Spatiotemporal Regulation of Transcript Isoform Expression in the Hippocampus
- 1Fralin Biomedical Research Institute, Center for Neurobiology Research, Virginia Tech Carilion, Roanoke, VA, United States
- 2Department of Biomedical Sciences and Pathobiology, Virginia-Maryland College of Veterinary Medicine, Virginia Tech, Blacksburg, VA, United States
- 3Virginia Tech Carilion School of Medicine, Roanoke, VA, United States
Proper development and plasticity of hippocampal neurons require specific RNA isoforms to be expressed in the right place at the right time. Precise spatiotemporal transcript regulation requires the incorporation of essential regulatory RNA sequences into expressed isoforms. In this review, we describe several RNA processing strategies utilized by hippocampal neurons to regulate the spatiotemporal expression of genes critical to development and plasticity. The works described here demonstrate how the hippocampus is an ideal investigative model for uncovering alternate isoform-specific mechanisms that restrict the expression of transcripts in space and time.
Introduction
The transcription and translation of RNA must be meticulously organized for cells to function properly. Neurons, in particular, are morphologically complex cells that require gene expression to be regulated in specific subcellular compartments at specific times for proper development and function. As a result, many different forms of RNA regulation coordinate to ensure proper transcript expression in the right place at the right time. Every step of RNA metabolism, from trafficking to translation and degradation is dictated in an mRNA-specific fashion by the nucleotide sequence and the combination of factors that associate with it, otherwise known as the RNA regulation code (Moore, 2005; Raj and Blencowe, 2015). Alternative isoform expression is one regulatory mechanism well poised to mediate spatial and temporal gene expression in neurons. Alternative isoform expression results from the interplay of many different RNA regulatory processes, including alternative promoter usage (alternative first exon; Twine et al., 2013), alternative exon splicing (Ha et al., 2021; Joglekar et al., 2021), alternative last exon usage (Taliaferro et al., 2016), and alternative polyadenylation (APA; Fontes et al., 2017; Ha et al., 2021; Figure 1). This coordinated inclusion or exclusion of specific cis RNA sequences can determine the spatiotemporal expression profile of a given transcript via differential binding of trans-acting factors. These include RNA binding proteins (RBPs) and microRNAs, that then recruit other post-transcriptional regulators, such as motor proteins or translational regulators (Wang et al., 2009; Mayya and Duchaine, 2019; Yee et al., 2019). Alternative isoform expression is regulated by a complex interplay of splicing factors (Fischer et al., 2011; Carey and Wickramasinghe, 2018), epigenetic modifications (Zhang et al., 2020), transcription factors (Thompson et al., 2019), enhancers/repressors (Conboy, 2021), and RNA binding proteins (Yee et al., 2019). Neuron-enriched RBPs, such as RBFOX1 (Jacko et al., 2018; Begg et al., 2020), ELAVL (Hinman et al., 2013; Yokoi et al., 2017), NOVA (Jensen et al., 2000; Ule et al., 2006), and MBNL2 (Wang et al., 2012, 2015; Taliaferro et al., 2016), regulate neuronal-specific, or even neuron class-specific (Feng et al., 2021), splicing programs by binding to highly conserved sequence motifs in pre-mRNAs and recruiting spliceosome factors to promote or inhibit splicing of specific exons.
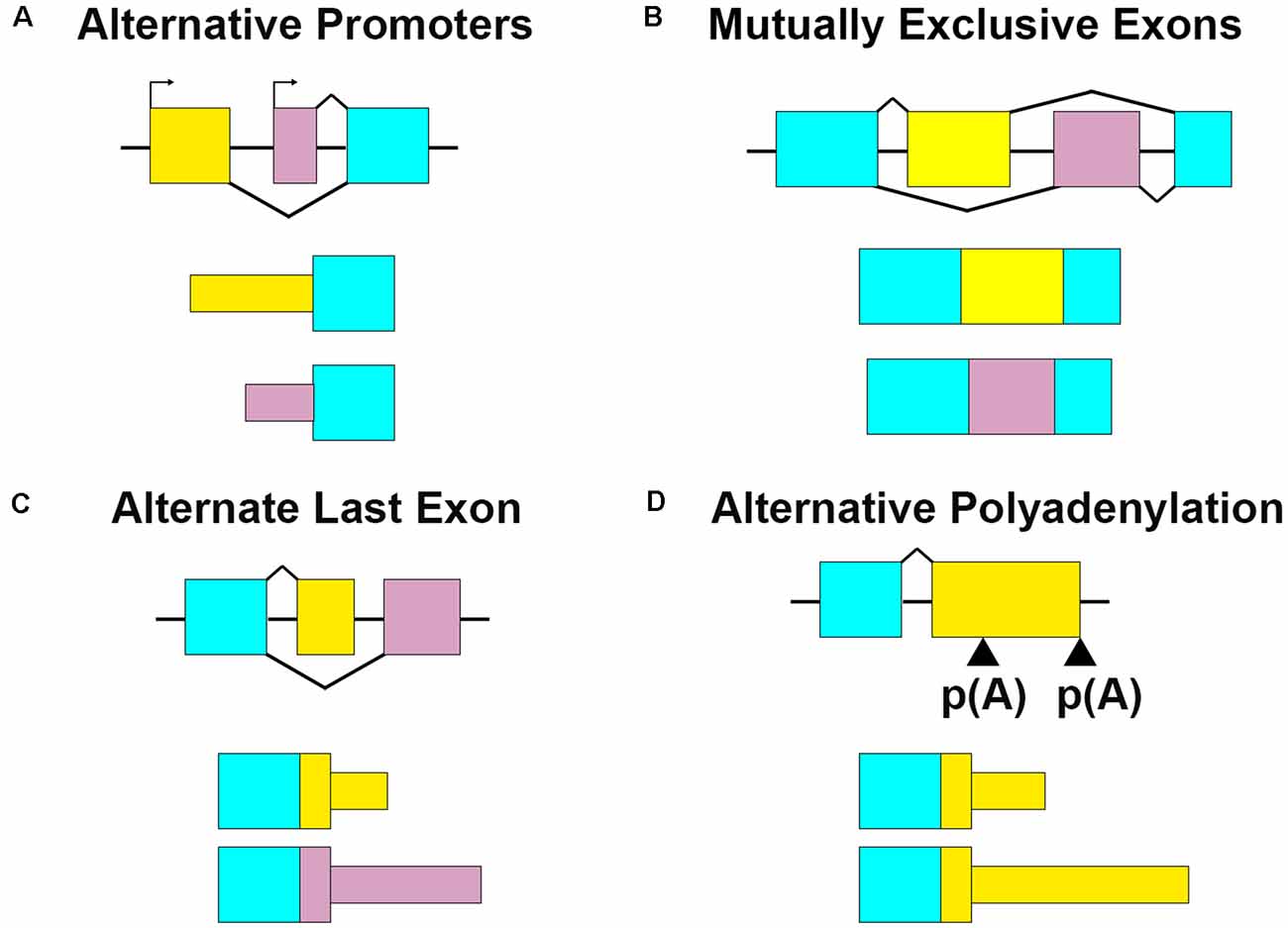
Figure 1. Multiple RNA processing mechanisms contribute to mRNA sequence variation. (A) Alternative promoters change the 5′ ends of transcripts by initiating transcription from different start sites. For Bdnf, the alternate promoter determines its dendritic localization. (B) Mutually exclusive exons retain one exon or the other. Gria2 uses this mechanism in combination with RNA editing to modify RNA localization and channel characteristics. (C) Alternate last exons retain one or the other mutually exclusive terminal exons. Cdc42 uses two alternate last exons to vary the terminal coding and 3′UTR sequences, which affect transcript localization and protein properties. (D) Alternative polyadenylation (APA) terminates transcription at multiple places within the same last exon. Also termed tandem 3′UTR, this results in a shortened or elongated 3′UTR. Camk2a harbors multiple 3′UTR lengths through this process, which modifies its posttranscriptional regulatory capacity.
Alternative isoform expression greatly expands the diversity of RNA transcripts and can be used to change transcriptome profiles at different developmental stages (Su et al., 2018). Over 95% of human multi-exon genes are alternatively spliced, and alternative splicing occurs at a higher rate in the brain than other tissues, demonstrating the importance of RNA variation in neurons (Yeo et al., 2004; Pan et al., 2008). Temporally-controlled isoform expression is critical for shaping neuron function across development by impacting ion channel composition (Gray et al., 2007), intracellular junction formation (Grabowski and Black, 2001; Iijima et al., 2016; Südhof, 2017), plasticity-related protein localization (Hermey et al., 2017; Furlanis and Scheiffele, 2018), and microRNA-mediated control over local translation (Hu and Li, 2017; Corradi and Baudet, 2020). Uncovering the mechanisms and impact of alternative isoform regulation on the spatiotemporal organization of gene expression is crucial to our understanding of neuronal biology.
RNA localization is a process by which transcripts are transported to different areas of the cell where they can be locally translated. This is especially important in neurons, where functionally distinct compartments demand specialized gene expression at discrete time points (Steward and Schuman, 2003). RNA localization is indispensable for compartment-specific proteomes, as almost half of all neurite-localized proteins are translated locally (Zappulo et al., 2017). Certain alternative isoform expression modes, such as alternative 3′ untranslated region (UTR) usage, can drive RNA localization. Over 70% of neuron-enriched genes have at least two alternative 3′UTRs, and longer 3′UTRs are concentrated in neurites over soma, demonstrating their importance in RNA trafficking (Ouwenga et al., 2017; Tushev et al., 2018). Longer 3′UTR sequences, acquired by alternative last exon usage or APA, allow for a greater number of regulatory sequence motifs to control the localization, stability, and translation transcripts (Andreassi and Riccio, 2009). Other RNA regulatory mechanisms, such as RNA editing and microRNA processing, work in tandem with alternative isoform expression to facilitate accurate localized gene expression and local translation. RNA editing can mediate localization through RNA sequence changes, and microRNAs can repress target RNAs in a spatially restricted manner (Kumar and Carmichael, 1997; Zhang and Carmichael, 2001; Schratt, 2009).
The hippocampus serves as an ideal model for studying RNA regulation dynamics because its principal neurons are physically separated into distinct cell bodies and dendritic layers, allowing for easy identification of synaptically localized RNAs. Due to its high propensity for plasticity, the hippocampus requires constant changes in gene expression to meet and maintain synaptic demands. In response to synaptic activity, hippocampal synapses readily undergo plasticity to strengthen or weaken connections in processes called Long-term potentiation (LTP) and Long-term depression (LTD), respectively (Bliss and Lomo, 1973; Dudek and Bear, 1992; Neves et al., 2008). The local translation of newly transcribed and/or synaptically localized transcripts is required to maintain activity-dependent changes to synaptic efficacy (Frey and Morris, 1997; Nguyen and Kandel, 1997; Holt et al., 2019). The hippocampus is also an area of robust splicing. It is estimated that every gene expresses an average of 3.9 alternative splice isoforms in the rat hippocampus (Wang et al., 2019). Our lab identified 3,298 differentially spliced transcript isoforms from 2,111 unique genes across the mouse hippocampal subregions and compartments, showing how splicing and localization are prevalent in this region (Farris et al., 2019). In this review, we cover several compelling examples in which splicing and RNA localization aid in hippocampal development and plasticity (Table 1). We further highlight recent work cataloging the extent of compartment-specific isoform regulation in the hippocampus and underscore the field’s need for mechanistic studies to reveal how alternate isoforms and their interactors functionally impact local translation.
Developmentally-Regulated Isoform Expression Guides Ion Channel Function
Splicing regulation of ion channels is critical to shaping the electrical activity required for hippocampal development (Grabowski and Black, 2001; Lipscombe, 2005). Mislocalization or mistiming of ion channel expression has been linked to neurodevelopmental diseases such as cortical malformations and epilepsies (Smith and Walsh, 2020). Thus, the investigation of how developing hippocampal neurons use RNA regulation to manage ion channel expression is an actively investigated topic. Here we discuss two examples of how splicing can be used to ensure the proper localization of ion channels during critical developmental timepoints.
The localization of hyperpolarization-activated cyclic nucleotide-gated 1 (HCN1), a channel responsible for the Ih current that modulates action potential firing frequency, is mediated by the splicing of peroxisomal biogenesis factor 5 like (Pex5l; Santoro et al., 2004). Neuronal Pex5l is an HCN1 channel subunit chaperone transcribed from two alternate promoters, 1a and 1b (Lewis et al., 2009). The nomenclature for the 11 Pex5l splice variants uses a parenthesis after Pex5l to indicate which N-terminal exons are spliced to the common exons 5–16 (Han et al., 2020). PEX5L variants containing exon 1b appear to oppose exon 1a-containing variants by decreasing HCN1 expression and Ih current through increased endocytosis of surface HCN1 channels. Specifically, PEX5L (1b-2–4) reduces HCN1 expression, and (1b-2) results in the sequestering of HCN1 to intracellular puncta in hippocampal CA1 (Lewis et al., 2009; Santoro et al., 2009). These two (1b−) variants make up almost all of the PEX5L (1b−) variants in the mouse brain, with their mRNA accounting for 20–30% of all Pex5l mRNA (Santoro et al., 2009). Independently, PEX5L (1a) prevents the axonal expression of HCN1, while PEX5L (1a-4) localizes HCN1 to the distal dendritic layer of CA1 (Lewis et al., 2009; Santoro et al., 2009; Piskorowski et al., 2011). While all Pex5l transcripts containing the 1a exon are highly expressed in the rat hippocampus from P2 to adulthood, the expression of isoforms containing exon 1b appears later, around P13 (Lewis et al., 2009). This suggests developmental regulation of the alternate exon 1b promoter in the hippocampus. Indeed, indicators of transcriptional activation (histone-3 lysine 4 trimethylation, H3K4me3) cluster almost exclusively to the exon 1a promotor in neural progenitors (Meissner et al., 2008; Lipscombe and Pan, 2009). In whole brain lysates, there are H3K4me3 peaks at both exon 1a and 1b, showing activation of the 1b promoter later in development. This early inhibition of the Ih -reducing exon 1b may facilitate the role of Ih in generating giant depolarizing potentials in the initial postnatal period that are critical for synaptic strengthening (Bender et al., 2001, 2005; Kasyanov et al., 2004) or as a pacemaker for early network oscillation (Garaschuk et al., 1998). The oscillations mediated by HCN channels may provide a synchronous activity that is essential to the hippocampal maturation of glutamatergic neurotransmission (Durand et al., 1996). Thus, restriction of exon 1b-containing PEX5L variant expression during early development allows for proper hippocampal activity and maturation through the localization and expression of HCN1.
Like PEX5L, alternative splicing of discs large MAGUK scaffold protein 3 (Dlg3, also known as synapse-associated protein 102 or Sap102) regulates the localization of ion channel subunits during development, specifically glutamate ionotropic N-methyl-d-aspartate receptors (NMDARs) that mediate synaptic plasticity (Chen et al., 2011). During development, there is an NMDAR subunit composition switch from NMDA type subunit 2B (GRIN2B) to 2A (GRIN2A), leading to an increase in the activation threshold for LTP (Monyer et al., 1994; Watanabe et al., 1994). This switch is mediated by Dlg3, a member of the PSD-95-like membrane-associated guanylate kinase family of synaptic scaffolding proteins (Müller et al., 1996; Smith et al., 1996). DLG3 expression in CA1 synapses closely mirrors that of GRIN2B at CA1 during development, and DLG3 has been found to control GRIN2B expression in the synapse (Sans et al., 2000; Chen et al., 2012). Mammalian Dlg3 has three isoforms which can be differentiated based on the presence or absence of two alternatively spliced regions (Müller et al., 1996). The I1 region at the N terminus binds GRIN2A, whereas the I2 region found towards the C-terminal end binds GRIN2B and localizes it to synapses (Chen et al., 2011; Wei et al., 2015, 2018). Knockdown of Dlg3 I1 in hippocampal neurons increases GRIN2B surface expression, and knockdown of Dlg3 I2 increases surface expression of GRIN2A (Chen et al., 2012; Wei et al., 2015). In mice, the expression of Dlg3 mRNA containing I1 increases from P1 to P20 (Chen et al., 2011). Conversely, Dlg3 mRNA containing I2 decreases relative to the other variants from P2 to P20, and I2 phosphorylation, which clusters DLG3 at spines, plateaus after P8 (Chen et al., 2011; Wei et al., 2015, 2018). Thus, developmentally regulated splicing from I2 containing- to I1 containing-Dlg3 aids in the stage-specific subunit composition switch of GRIN2B to GRIN2A containing NMDARs at maturing hippocampal synapses. In this way, hippocampal neurons developmentally tune activity patterns via the splicing regulation of ion channel chaperones.
Multiple RNA Processing Mechanisms Contribute to Proper Ion Channel Subunit Expression
As illustrated with Pex5l and Dlg3, splicing serves as an important regulator of hippocampal channel localization. Controlling the flow of ions is the basis for neuronal signaling, and making sure the correct ion channels localize to their appropriate compartments is crucial to hippocampal function. One strategy to accomplish this is by directing channel subunit isoforms to specific areas to regulate both the channel localization and properties. Several different posttranscriptional processing modes are utilized to achieve this, including alternative last exons, mutually exclusive splicing, and RNA editing. Kcnj6 and Gria2 demonstrate how channel subunit splicing can affect localization, and how RNA editing adds another layer to this regulation.
Potassium inwardly-rectifying channel subfamily J member 6 (Kcnj6) expresses splice variants whose RNAs localize differently. Known more widely as G protein-gated inwardly rectifying K+ channel 2 (Girk2), it is a G-protein dependent hyperpolarizing potassium channel that maintains resting membrane potentials and has been shown to depotentiate LTP in hippocampal cultures (Sakura et al., 1995; Chung et al., 2009; Hibino et al., 2010). Two Girk2 splice isoforms, GIRK2a and GIRK2c, differ due to alternate last exons, resulting in different 3′UTRs and 11 amino acids at the C-terminus (Wei et al., 1998). Although both mRNA variants are expressed in the CA1 neuropil, Girk2c RNA and protein have a much higher expression (Marron Fernandez de Velasco et al., 2017). In addition, GIRK2a protein is mostly restricted to the cell body of hippocampal pyramidal neurons (Marron Fernandez de Velasco et al., 2017). GIRK2c’s PDZ binding motif allows its binding to several synaptic proteins that GIRK2a cannot, such as PSD-95 (Inanobe et al., 1999). Experiments with Girk2 knockout mouse hippocampal slices indicate that GIRK2a mediates slow inhibitory postsynaptic currents in CA1 proximal dendritic fields via Schaeffer collaterals, while GIRK2c mediates them in the distal dendritic fields via perforant/temporoammonic path (Marron Fernandez de Velasco et al., 2017). Furthermore, caspase-3 cleaves GIRK2a at the alternatively spliced C-terminus after prolonged activity in rat hippocampal cultures (Baculis et al., 2017). This cleavage decreases binding to its G-protein Gβγ and GIRK1, preventing GIRK2’s activity and surface expression. Thus, splicing of GIRK2 to the Girk2c variant localizes the RNA and protein to the neuropil, and only GIRK2a is cleaved after sustained activity.
Another channel subunit gene, glutamate ionotropic receptor AMPA type subunit 2 (Gria2), can undergo two modes of RNA regulation that affect its activity and localization- splicing and editing (Tanaka et al., 2000; Barbon and Barlati, 2011). GRIA2 is a subunit of alpha-amino-3-hydroxy-5-methyl-4-isoxazole-propionic acid receptors (AMPARs) that mediate fast excitatory synaptic transmission (Lüscher et al., 1999; Tanaka et al., 2000). Insertion and recycling of AMPARs, and thus GRIA2, mediates changes in synaptic strength in response to learning (Lüscher et al., 1999; Ehlers, 2000; Zheng et al., 2015). RNA editing is a process through which protein sequences are altered via nucleoside modification of target transcripts. It is estimated that 85% of all human pre-mRNAs undergo the most common form of RNA editing, A-to-I editing (Athanasiadis et al., 2004). This editing results in the chemical conversion of an adenosine to inosine, which is read as a guanosine (Bass and Weintraub, 1987, 1988; Wagner et al., 1989; Walkley and Li, 2017). Gria2 undergoes A-to-I RNA editing at two positions known as the Q/R site and the R/G site. Q/R site editing changes the amino acid at position 607 from glutamine to arginine (Higuchi et al., 1993; Yang et al., 1995). Gria2 that is unedited at the Q/R site is readily trafficked to the membrane, while the edited form is sequestered in the ER (Araki et al., 2010; Gurung et al., 2018). A mutation of Gria2 such that it can no longer be edited at position 607 results in loss of neurons in CA1 (Konen et al., 2020). Q/R editing makes AMPAR less permeable to Ca2+ (Egebjerg and Heinemann, 1993). Gria2 mRNA is also edited at the R/G site to change an arginine in position 764 to glycine (Lomeli et al., 1994). This has a drastic effect on its localization, as 56% of Gria2 mRNA in the rat hippocampus is edited at the R/G site, while only 12% of Gria2 mRNA is R/G edited in the synaptosomal fraction (La Via et al., 2013). The R/G edit increases the recovery rate of AMPAR from desensitization (Lomeli et al., 1994).
Gria2 undergoes alternative splicing at the mutually exclusive “flip/flop” exons near the R/G site (Sommer et al., 1990). GRIA2 variants containing the flop exon (GRIA2Flop) have a faster desensitization rate, faster deactivation rate, and lower steady-state current than those containing the GRIA2Flip variant (Wen et al., 2017). Gria2flip accounts for 41% of total Gria2 mRNA in rat hippocampus, with the number rising to 96% in the synaptoneurosomal fraction (La Via et al., 2013). In the rat CA1, Gria2flip mRNA localizes to the cell soma (stratum pyramidale) and dendritic (stratum radiatum) layers, while Gria2flop mRNA is expressed exclusively in the cell soma (La Via et al., 2013). Blocking sodium or calcium currents in rodent CA1 with tetrodotoxin or nifedipine, respectively, results in upregulation of Gria2flop RNA (Sommer et al., 1990; Penn et al., 2012). While flip/flop splicing does not remove the R/G site, it interacts with the editing effect. Unedited GRIA2Flip is trafficked to dendrites more readily than edited GRIA2Flip (La Via et al., 2013). Editing of the R/G site in GRIA2Flop, but not GRIA2Flip, results in a faster channel closing rate and a faster desensitization rate (Wen et al., 2017). Thus, splicing to the flip variant or leaving the R/G site unedited localizes Gria2 RNA to the synapses while affecting desensitization kinetics of AMPAR. Gria2 RNA editing and splicing can change both the RNA and AMPAR localization while modifying channel properties. These two modifications interact, adding another layer to its regulation. With splicing and RNA editing being so common, it is important that we study their interactions, as they may have profound effects throughout the localized transcriptome.
Alternative Untranslated Regions Drive RNA Localization and Local Translation
Untranslated regions (UTRs) serve important isoform-specific roles by regulating many aspects of mRNA metabolism, including RNA localization, stability, and translation. Utilization of alternate UTRs leads to the incorporation of different cis-regulatory sequences, which can be bound by distinct complements of RBPs for differential localization, or microRNAs for translational control. Multiple mechanisms can alter UTRs, such as alternate promoter usage, alternate last exons, and alternative polyadenylation. Here we will discuss examples where varying 5′ and 3′UTRs mediate local gene expression regulation in hippocampal neurites.
Alternative promoters allow for transcription to begin at different first exons and often results in isoforms with different 5′UTRs. In the aged human brain, 60% of genes utilize alternative promoters (Pardo et al., 2013). 5′UTRs can influence various aspects of RNA regulation including localization and translational regulation. For example, transcription of the inducible transcription factor Npas4 from an upstream promoter results in a variant with a longer 5′UTR that preferentially localizes to hippocampal CA1 dendrites (Brigidi et al., 2019). In the hippocampal slice, excitatory postsynaptic potentials, but not action potentials, lead to selective CA1 dendritic translation of the Npas4 long 5′UTR variant and its compartment-specific interactor ARNT1. The locally synthesized NPAS1-ARNT1 heterodimers undergo retrograde translocation to the nucleus and activate different sets of genes than when Npas4 is activated by action potentials. This results in an isoform-specific, UTR-dependent mechanism to decode stimulus-specific synaptic activity into a distinct genomic response (Brigidi et al., 2019).
Brain-derived neurotrophic factor (Bdnf) is another example with transcript isoforms that differ only in the UTRs. BDNF is a critical neurotrophin that regulates hippocampal plasticity (Leal et al., 2014). In humans and mice, it has nine alternate promotors and two alternative polyadenylation sites, producing at least 22 transcript variants (Aid et al., 2007; Colliva and Tongiorgi, 2021). Each of the alternate promoters produces an entirely different 5′UTR. The resulting alternate Bdnf isoforms have differing roles, regulations, and localizations despite harboring identical amino acid sequences (Metsis et al., 1993; Timmusk et al., 1993; Pruunsild et al., 2007; Foltran and Diaz, 2016). Constitutive and activity-dependent dendritic targeting elements have been identified in the Bdnf CDS and 3′UTRs (Oe and Yoneda, 2010; Vicario et al., 2015). Bdnf isoform-specific localization is thought to be mediated by permissive or repressive interactions between the 5′UTRs and CDS cis- elements (Colliva and Tongiorgi, 2021). Some of the RBPs required for 3′UTR mediated Bdnf dendritic targeting have been identified, including CPEB-1 and several ELAV and FXR family proteins (Oe and Yoneda, 2010; Vicario et al., 2015). The RBPs associated with the different 5′UTRs have been bioinformatically identified, but not yet validated (Colliva and Tongiorgi, 2021). Here we will focus on Bdnf transcribed from the fourth promoter, also known as promoter IV and previously as the third promoter/promoter III until ~2007 (Zheng et al., 2011). Bdnf mRNA transcribed from promoter IV is expressed in an activity-dependent fashion across the rat hippocampus, where it is retained mostly in the soma and proximal dendrites (Chiaruttini et al., 2008; Chapman et al., 2012). The 5′UTR transcribed from the fourth promoter causes the retention of Bdnf RNA to the proximal dendrites, whereas the 5′UTRs transcribed from other promoters, including VI, permit localization to distal hippocampal dendrites (Chiaruttini et al., 2008; Baj et al., 2011). Additionally, the alternate 5′UTRs may serve to control the translational efficiency of the RNA. Bdnf 5′UTRs repress translation of a reporter construct in rat cultured hippocampal neurons, with promoter IV 5′UTR repressing reporter translation the least, and promoter VI 5′UTR repressing the most (Vanevski and Xu, 2015). Bdnf promoter-specific knockout mice show hippocampal cell- and –compartment-specific deficits in dendritic arborization, namely promoter IV knockout mice show decreased apical dendritic arborization in CA1, but not neighboring CA3 (Maynard et al., 2017). Overexpression of BDNF from promoter IV increases the number of primary and secondary dendrites in rat hippocampal neurons at 7 DIV (days in vitro) and 18 DIV, respectively (Baj et al., 2011). Collectively, these data support a model in which transcription of Bdnf mRNA from promoters IV and VI localize to proximal or distal dendrites, respectively, in a UTR-dependent manner, establishing isoform-specific regulation of different dendritic compartments (Figure 2A; Baj et al., 2011; Colliva and Tongiorgi, 2021).
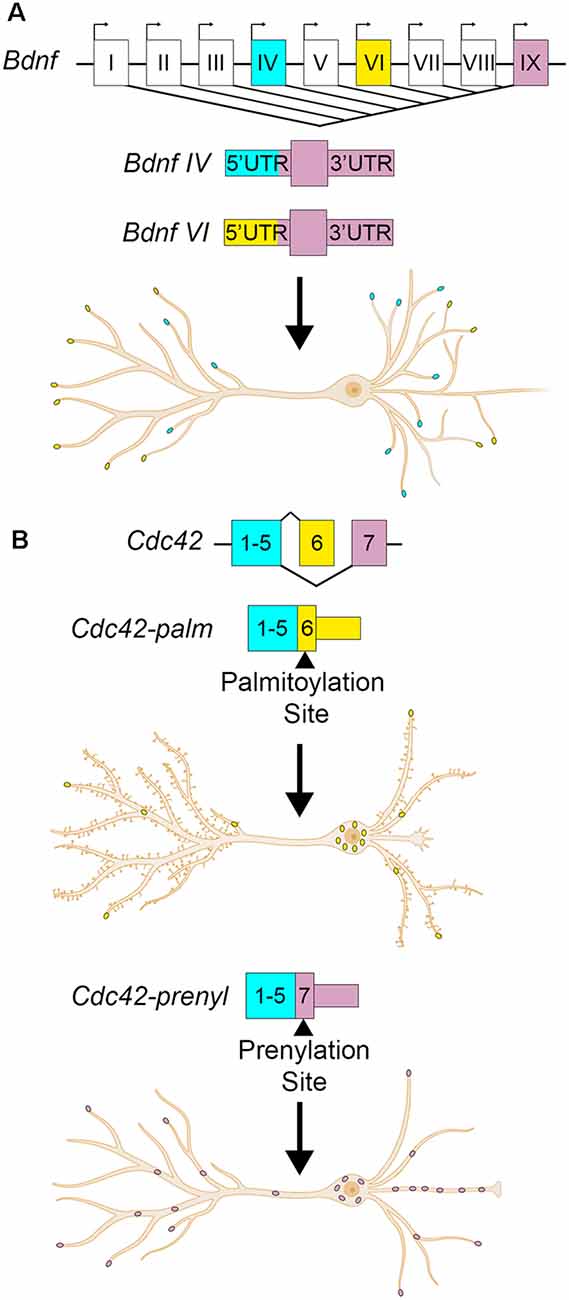
Figure 2. Alternate UTR usage can modify the localization and developmental effects of a transcript. (A) Bdnf transcription can begin from one of nine alternate promoters. The promoter choice affects the 5′UTR sequence, but not the coding sequence or 3′UTR. Bdnf transcribed from the fourth promoter (cyan dots) localizes to proximal dendrites, while transcription from the sixth promoter localizes it to distal dendrites (yellow dots). Bdnf IV localization to proximal dendrites promotes dendritic formation. (B) Cdc42 has alternate last exons that determine its 3′UTR. The upstream last exon localizes Cdc42 mostly to the cell body of the neuron (yellow dots), and contains a palmitoylation site. This 3′UTR variant promotes spine formation. The downstream last exon containing a prenylation site enriches the transcript to neurites and axons (pink dots) and promotes axogenesis.
Activity-dependent transcription at promoter IV is regulated by cAMP response element binding protein 1 (CREB1) and methyl-CpG binding protein 2 (MECP2). Calcium influx upon neuronal activation causes the nuclear translocation of γCAMKII, which leads to phosphorylation of CREB1 (Bito et al., 1996; Deisseroth et al., 1996; Ma et al., 2014; Cohen et al., 2018). CREB1 then binds to the calcium/cyclic AMP response element (CRE) in promoter IV, initiating transcription (Shieh et al., 1998; Tao et al., 1998; Pruunsild et al., 2011). On the other hand, MECP2 can bind to methylated CpG islands in promoter IV, preventing its transcription (Chen et al., 2003; Martinowich et al., 2003; Chang et al., 2006; Zhou et al., 2006; KhorshidAhmad et al., 2016). MECP2 can be phosphorylated in a calcium-dependent manner following neuronal activation, allowing for its dissociation from promoter IV (Chen et al., 2003; Zhou et al., 2006). Bdnf at promoter IV is an elegant example of UTR-specific regulation influencing RNA localization. By simply initiating and/or inactivating transcription from a specific promoter, the plasticity at specific synapses can be regulated in a UTR-dependent manner.
Alternate 3′UTRs primarily take two forms- alternate last exons and APA. In the former, the terminal exon is different among isoforms, resulting in completely distinct 3′UTRs. For example, cell division cycle 42 (Cdc42) is a Rho-family GTPase that is required for plasticity at CA1 spines (Murakoshi et al., 2011; Kim et al., 2014; Shibata et al., 2021) and has two splice variants with alternate last exons resulting in different C-terminal protein sequences and 3′UTRs (Figure 2B; Munemitsu et al., 1990; Shinjo et al., 1990; Marks and Kwiatkowski, 1996; Olenik et al., 1997). The proximal last exon is known as exon 6 or exon 6B, and is spliced into the brain-exclusive variant CDC42-palm (due to its palmitoylation modification, also known as CDC42-v2, CDC42-E6, CDC42b, and bCDC42, where b stands for brain; Marks and Kwiatkowski, 1996). The distal last exon is termed exon 6A or exon 7, and is spliced into the ubiquitously expressed CDC42-prenyl (due to its prenylation modification, also known as CDC42-v1, CDC42E7, and CDC42u where u stands for ubiquitous; Kang et al., 2008; Roberts et al., 2008). For the rest of this review, CDC42-palm and CDC42-prenyl will be used. Cdc42-prenyl is expressed ubiquitously due to its last exon having a stronger 3′ splice acceptor (Yap et al., 2016). In non-neuronal cells, the splicing regulators PTBP1 and PTBP2 repress the inclusion of the Cdc42-palm alternate last exon (Yap et al., 2016; Ciolli Mattioli et al., 2019). Downregulation of these factors in early neuron development promotes the inclusion of the Cdc42-palm alternate last exon with the weaker 3′splice acceptor (Zheng et al., 2012; Yap et al., 2016). While the RNAs of each isoform localize to both neurites and soma, Cdc42-prenyl has higher expression than Cdc42-palm in cultured neurites (Taliaferro et al., 2016; Ciolli Mattioli et al., 2019; Lee et al., 2021) and hippocampal neuropil (Farris et al., 2019). Cdc42-prenyl mRNA has a longer 3′UTR that mediates its localization to neurites in mouse cortical neurons and mouse embryonic stem cell-derived neurons, where it is locally translated (Marks and Kwiatkowski, 1996; Ciolli Mattioli et al., 2019). CDC42-prenyl promotes axogenesis in hippocampal neurons (Yap et al., 2016). While the role of CDC42-prenyl prenylation in neurons is not yet understood, it may anchor the protein to axons since this modification allows its association with the membrane in S. cerevisiae (Estravís et al., 2017). Palmitoylation of CDC42-palm attaches the fatty acid palmitate to either Cys188 or Cys189, which is required for induction of dendritic filopodia, and the development of long protrusions in cultured neurons (Wirth et al., 2013). Palmitoylation of CDC42-palm also stabilizes hippocampal spines and rescues spine density in 22q11DS transgenic mouse models (Moutin et al., 2017). Glutamate treatment of hippocampal cultures induces a rapid depalmitoylaion of CDC42-palm and its dispersal from spines (Kang et al., 2008). Thus, the alternate 3′UTRs mediate the isoform-dependent localization of Cdc42 in hippocampal neurons, while posttranslational modifications can alter their activity and function.
Another mode of alternative 3′UTR generation is alternative polyadenylation, which results from transcription termination at different points along the same terminal exon, resulting in shortened or elongated versions of the 3′UTR. 79% of mouse coding genes have alternative polyadenylation sites (Hoque et al., 2013). APA is especially prevalent in the brain, where tissue-specific 3′UTR lengthening is enriched (Miura et al., 2013). In excitatory neuron-differentiated mESCs, 4,149 genes were found to have differentially localized alternate 3′UTRs of which 3,675 corresponded to APA isoforms, and 474 to alternate last exons (Ciolli Mattioli et al., 2019). While the specific mechanisms governing preferential localization of alternate UTR isoforms to specific compartments remains unknown for the majority of transcripts, compartment localized RNAs in the adult hippocampus have longer 3′UTRs that are more stable and contain more microRNA seed sequences and RBP motifs compared with non-localized RNAs (Tushev et al., 2018), providing greater opportunity for regulation. Indeed, neurite-enriched 3′UTRs contain binding motifs for localization-linked RBPs such as muscleblind-like (MBNL) family RNA-binding proteins (Taliaferro et al., 2016; Tushev et al., 2018), zip-code-binding protein (ZBP1; Tushev et al., 2018), and fragile X mental retardation protein (FMRP; Tushev et al., 2018). Longer 3′UTRs allow for more microRNAs to bind and repress translation (Legendre et al., 2006; Hu and Li, 2017; Epple et al., 2021). There is evidence that up to 88% of mouse hippocampal neurite mRNAs are targeted by synaptic microRNAs (Epple et al., 2021), suggesting that noncoding RNAs have an underappreciated role in localized gene expression (Hu and Li, 2017; Epple et al., 2021).
Calcium calmodulin-dependent protein kinase II alpha (Camk2a) is a well-characterized gene involved in memory formation that encodes alterative isoforms with different length 3′UTRs due to alternative polyadenylation (Figure 3A). Camk2a mRNA containing the longer 3′UTR is enriched in hippocampal neuropil (Tushev et al., 2018). Camk2a dendritic localization is dependent on elements within its 3′UTR (Mayford et al., 1996; Mori et al., 2000) as transgenic reporter mice containing only the Camk2a 3′UTR is sufficient to localize a lacZ transcript to hippocampal dendrites (Mayford et al., 1996). miR-181a is a locally processed microRNA that controls hippocampal plasticity via alternative 3′UTR-mediated translational repression (Sambandan et al., 2017). miR-181a is present in rodent hippocampal dendrites and synaptosomes (Epple et al., 2021). miR-181a is present in rat CA1 dendrites in its pre-miR form and is processed locally to its mature form by DICER upon synaptic activation (Sambandan et al., 2017). After maturation, miR-181a inhibits the local translation of Camk2a, which is required for memory formation (Miller et al., 2002; Sambandan et al., 2017). A GFP reporter construct containing the Camk2a long 3′UTR showed decreased activity-dependent translation in hippocampal dendrites compared to a construct containing the short Camk2a 3′UTR lacking the miR-181a seed sequence (Sambandan et al., 2017). Thus, the inclusion of miR-181a seed sequence in Camk2a long 3′UTR allows for local translational repression at active synapses (Figures 3B,C). miR-181a also downregulates the expression of rat hippocampal Gria2 mRNA (Saba et al., 2012). miR-181a expression in the rat dorsal hippocampus increases after learning tasks and is associated with inhibition of protein kinase AMP-activated catalytic subunit alpha 1 (Prkaa1) RNA, the catalytic subunit of AMP-activated protein kinase (AMPK), a regulator of plasticity (Potter et al., 2010; Zhang et al., 2017). Thus, local activity-dependent pre-miR-181a processing leads to the repression of several synaptic transcripts that are essential to plasticity.
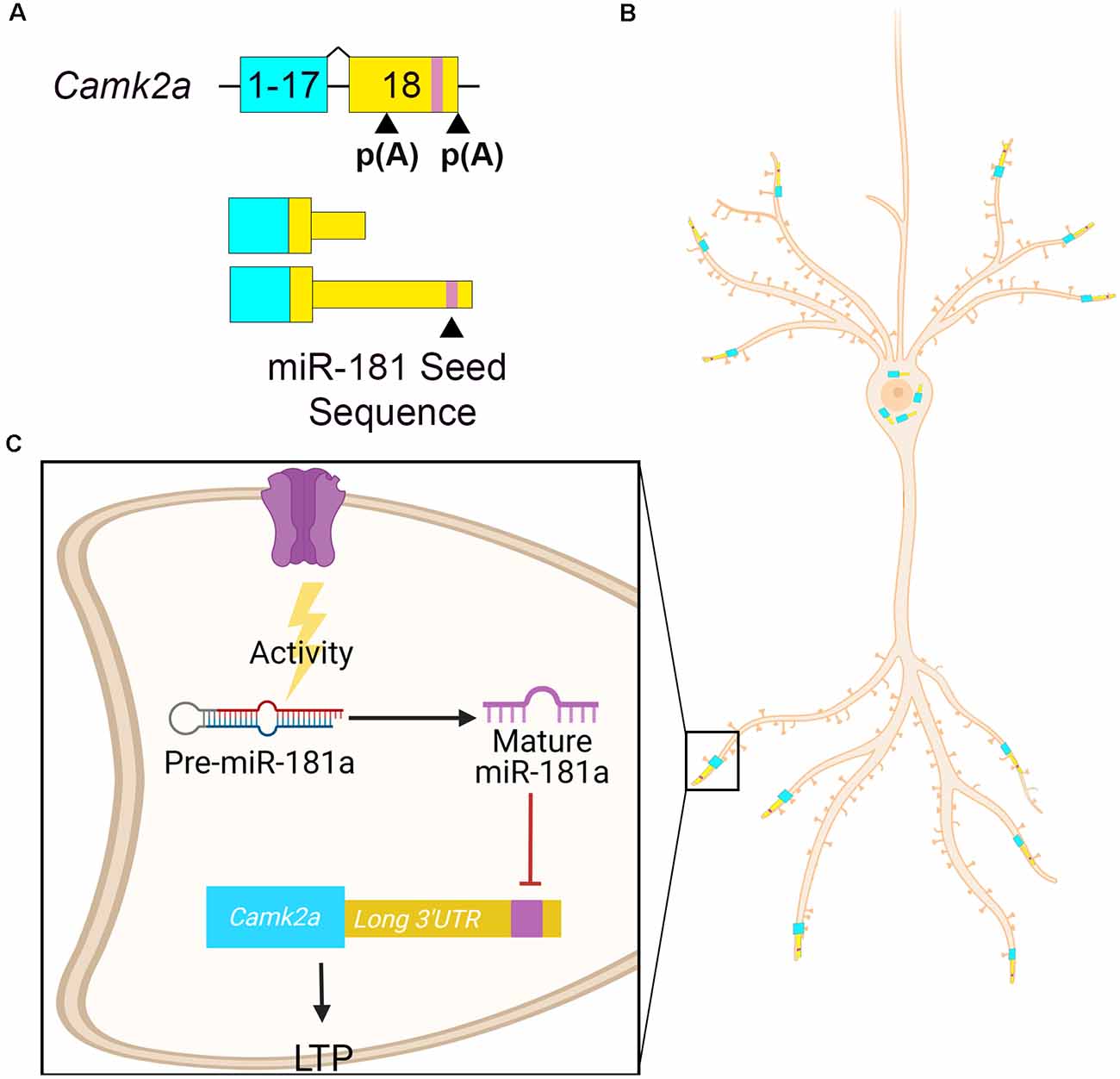
Figure 3. Camk2a APA drives its localization and activity-dependent miRNA regulation. (A) Camk2a APA [p(A)] in exon 18 determines the 3′UTR length. The distal p(A) site results in a longer 3′UTR that contains a miR-181a binding site (purple). (B) Camk2a with the shorter 3′UTR localizes to the cell soma, whereas Camk2a with the longer 3′UTR localizes to neurites. (C) Pre-miR-181a is present at hippocampal synapses. In response to synaptic activity, it is processed by DICER into its mature active form. Mature miR-181a then represses the translation of Camk2a by binding to its seed sequence in the long 3′UTR. This results in the inhibition of long-term potentiation (LTP). Expressing the longer 3′UTR increases Camk2a’s regulatory potential.
miR-181a demonstrates how isoform expression can interact with microRNA-mediated local translational repression through alternative 3′UTRs. In fact, 43.3% of predicted microRNA target sites in human 3′UTRs are found in alternative UTR segments (Majoros and Ohler, 2007). Furthermore, miR-181a is predicted to target a significant number of 3′UTRs which undergo alternative splicing (Wu et al., 2013). By including a longer 3′UTR, RNAs can be localized to neurites more efficiently, possibly through additional RBP binding or greater RNA stability (Tushev et al., 2018), where they can be regulated by microRNAs in an activity-dependent manner.
Discussion
Each step of post-transcriptional regulation from RNA localization to stability to translation and degradation can be altered by changing the cis RNA regulatory sequences in a given transcript. In morphologically complex cells like neurons, alternative isoform expression is used to tune these regulatory processes and provide distinct subcellular compartments with the necessary transcripts to support specialized functions. Unraveling this code is vital to fully understanding how RNA is regulated across different contexts and cell types to support neuronal functions. The genes presented in this review illustrate how alternate isoform expression mediates localized RNA regulation to ensure proper hippocampal neuron development and plasticity.
Are There Subregion-Specific Splicing Programs in The Hippocampus?
Isoform-specific regulation of Pex5l and Dlg3 leads to the proper expression and localization of HCN1 and NMDAR subunits, respectively, which are vital for the development of proper electrical activity for synaptic maturation (Ewald and Cline, 2009; Stoenica et al., 2013). Pex5l exon 1a expression is highest in CA1, consistent with the critical role of HCN1 in CA1 pyramidal cells (Magee, 1999; Piskorowski et al., 2011). However, it remains unknown how subregion-specific splicing programs in the hippocampus are implemented and thus, further investigation is needed. The hippocampus has cell- and compartment-specific transcriptomes, as it is divided into 4 major subregions (CA1, CA2, CA3, and the dentate gyrus) with diverse gene expression schemes to control subregion-specific properties and functions (Masser et al., 2014; Cembrowski et al., 2016; Farris et al., 2019). Several studies have identified alternative splicing programs that readily distinguish neuron cell classes (glutamatergic, GABAergic, glia; Zhang et al., 2014; Furlanis et al., 2019; Sapkota et al., 2019; Feng et al., 2021; Joglekar et al., 2021) and to a lesser extent distinguish neuron subclasses (CA1, CA3; Furlanis et al., 2019; Joglekar et al., 2021), indicating that alternate isoform expression is a driver of functional specification (Furlanis et al., 2019). Cell-specific expression of transcription factors and epigenetic modifiers likely induce expression of these specialized transcriptomes, but it is still unknown how they communicate with the splicing machinery to induce expression of one isoform over another. Cell-specific transcriptomes must further change at specific developmental windows (Weyn-Vanhentenryck et al., 2018) and also be actively maintained into adulthood (McCann et al., 2021). There are likely many players involved, as neuronal splicing regulatory networks require combinatorial interactions between splicing factors and RBPs (Raj and Blencowe, 2015; Vuong et al., 2016). The identification of these players using cell- and isoform-specific profiling approaches in combination with gene knockout methods will shed light on the cell- and context-dependent interplay between spliceosome components, transcription factors, and RBPs.
What Regulatory Elements Influence Localized Isoform Expression?
As evidenced by Npas4, Bdnf, Cdc42, and Camk2a, both 5′ and 3′UTRs can direct the trafficking of transcripts to hippocampal dendrites. The inclusion of different cis sequences in the UTRs increases the capacity for regulating RNA characteristics, such as localization and stability. With alternative promoters, alternative polyadenylation, and alternative last exons being so pervasive in neurons (Pardo et al., 2013; Tushev et al., 2018), this leads to an underappreciated amount of diversity in UTR-dependent regulation. The functional impact of this UTR diversity on local RNA regulation is still largely unknown. The regulation and trafficking of UTR-dependent RNA localization is mediated by RNA-binding proteins (Miura et al., 2013; Mayya and Duchaine, 2019; Bae and Miura, 2020), but for the overwhelming majority of RNAs, the binding partners and mechanisms that mediate localization along with the contexts under which it happens are still being discovered. Indeed, hippocampal compartment-specific sequencing studies have identified thousands of synaptically localized RNAs that display cell-type specificity (Cajigas et al., 2012; Farris et al., 2019), hundreds of which have alternate UTRs (Tushev et al., 2018), underscoring the diversity and complexity of RNA regulation occurring in dendrites. While RBP motifs and dendritic targeting elements in specific transcripts have been characterized, it remains unknown how combinations of cis sequences work in concert to induce specific patterns of localization. and how different contexts or dynamic systems expressing distinct complements of RNAs and RBPs affect these interactions. The tools in this regard have improved over time to include in silico motif predictions to pair with cross-linking immunoprecipitation (CLIP) data in order to identify the locations of RBPs along target RNA transcripts. Cell-specific RNA-RBP prediction networks that modulate local transcriptomes can be generated by combining compartment-specific transcriptome data with RBP-CLIP data, or when technically feasible, performing compartment-specific RBP-CLIP in different contexts. Fully elucidating UTR-mediated local RNA regulation by unraveling the RNA localization code may be beyond our technological reach at this time. However, advances in highly-multiplexed and iterative single-molecule imaging techniques to map RBP-RNA interactions in situ provide much optimism.
RNA editing is another tool that can regulate the localization of plasticity-related transcripts. By interacting with splicing, RNA editing provides an additional regulatory layer through which transcripts can be modified in response to activity. Of the 17,831 known RNA editing sites in the mouse genome, 682 have been detected in the hippocampus (Stilling et al., 2014). It is clear that editing plays a role in the hippocampus, but its relationship with splicing still requires further study. We have seen an example of splicing-editing interaction in Gria2, but the exact molecular players remain unidentified. RNA editing can occur co-transcriptionally like splicing, and some RNA editing enzymes have been implicated in splicing (Kapoor et al., 2020). It will be interesting to determine whether RNA editing proteins and splicing factors co-localize to coordinate editing with splicing.
microRNA regulation is another way that splicing can impact local RNA expression. Alternate UTRs increase the regulatory potential of microRNAs. By utilizing 3′UTRs containing a different mix of seed sequences, microRNA-mediated regulation can be adjusted to meet specific regulatory needs. Activity is a key signaling step in the maturation process of miR-181a, allowing it to be loaded onto the RNA-induced silencing complex (RISC) to repress translation of target transcripts such as the long 3′UTR neurite-localized Camk2a. How the RISC identifies miR-181a and how it recruits target transcripts remains unknown. Indeed, the prevalence and mechanisms of microRNA-mediated control of localized RNA need further elucidation, as it is a potentially widespread method of local gene expression regulation. Understanding the full extent of this regulatory mechanism may be key to understanding the role of local translation and its role in synaptic plasticity.
It remains an open question as to how alternate isoforms impact local translational capacity. Genome-wide measures of isoform-specific transcription and translation in mature neuronal cultures from differentiated human embryonic stem cells demonstrate that regulatory sequences in long 5′ and 3′UTRs generally repress translation, with the strongest effect from 3′UTRs (Blair et al., 2017). This is consistent with the fact that localized RNAs have longer UTRs and require translational repression for transport. Structural features detected in some dendritic transcripts, such as high G-C content in 5′UTRs and upstream open reading frames, are also consistent with translational repression (Falley et al., 2009). Low translational capacity is often equated with monosome association and a recent study showed that localized transcripts in CA1 exhibit a preference for monosome translation (Biever et al., 2020). The monosome preferring transcripts encoded a full range of low- to high-abundance proteins in the neuropil, suggesting that monosomes are an essential source of synaptic proteins (Biever et al., 2020). As technologies continue to advance for lower input samples, it will be critically important to directly test the impact of alternate isoforms on local translational capacity.
What Other Emerging Forms of Splicing Warrant Further Study?
Several other forms of splicing require further study to understand their roles in local RNA regulation as it relates to hippocampal neuron function. Circular RNAs (circRNAs) are non-linear transcripts without a distinct 3′ or 5′ end that are produced as a result of back-splicing (Gokool et al., 2020). In the hippocampus, circRNAs derived from synapse-related genes such as Homer1, Elavl, and Nlgn1 are present at hippocampal synapses (You et al., 2015). The linear mRNA for Staufen2 (Stau2) is almost exclusively present in the cytoplasm, while circStau2 localizes to synapses in both mouse and human neurons, suggesting a synaptic role for some circRNAs (Rybak-Wolf et al., 2015). Retained introns are another form of splicing in which introns are included in the mRNA. Retained introns can contain cis- elements such as constitutive transport elements (CTE) that are bound by RBPs (Li et al., 2006, 2016). Staufen2 protein has been shown to localize Calm3 mRNA to hippocampal dendrites in a retained-intron-3′UTR-dependent manner (Sharangdhar et al., 2017). NXF1 regulates the nuclear export of its own mRNA with a retained intron, leading to its protein isoform colocalizing in RNA granules with Staufen2 (Li et al., 2016). Microexons are 3–27 nucleotide long sequences that insert small stretches of in-frame amino acids into protein products (Gonatopoulos-Pournatzis and Blencowe, 2020). In the mouse hippocampus, differential splicing leads to significant changes in protein isoform expression, and microexons are enriched in alternative splicing events that lead to proteome changes in neurons (Reixachs-Solé et al., 2020). Microexons programs are highly conserved and are involved in critical neuronal functions such as neurogenesis, vesicle trafficking, and RNA binding proteins (Gonatopoulos-Pournatzis and Blencowe, 2020). The disruption of neuronal microexons is associated with autism spectrum disorders. RNAseq on postmortem brains showed that 30% of detected microexons were significantly different between autistic and control brains (Irimia et al., 2014). Further work will be needed to reveal the cell- and compartment-specific role for these mechanisms in regulating localized gene expression.
Conclusion
Developmental and activity-regulated gene expression programs can utilize cell- and context-specific RNA codes necessary to support compartment-specific functions. Despite all the elegant work described in this review, we have only scratched the surface of transcript isoform-dependent local RNA regulation. Recent work has cataloged cell- and compartment-specific isoform expression, but many questions remain with regards to the functional roles that specific sequences and their interactors play in regulating local transcriptomes. Advances in long-read technologies, such as nanopore sequencing, have illuminated the extent of previously underestimated levels of RNA isoform diversity. Nanopore sequencing allows for the direct sequencing of full-length transcripts, instead of the shorter reads utilized by RNAseq. This technology was able to identify 33,984 isoforms from 10,793 genes in human cells, of which 52.6% had an unannotated splice junction (Workman et al., 2019). This technology can be used to analyze isoforms in different cellular compartments, as nanopore sequencing across 13 cell lines revealed that there are major differences in isoforms expressed in the nucleus vs. cytoplasm, including intron-retention enrichment in nuclear transcripts (Zeng and Hamada, 2020). Moving forward, insights revealed from sequencing methodologies such as long read (Bolisetty et al., 2015; Deamer et al., 2016) and 5′/3′ end sequencing need to be integrated with functional assays and spatially resolved single-molecule imaging techniques to fully unravel the ways in which the RNA code impacts local RNA regulation in specific contexts. The hippocampus remains a useful model system to uncover splicing-dependent regulation of localized transcriptomes to impact neuron function.
Author Contributions
All authors contributed to the article and approved the submitted version.
Funding
This work was supported by the National Institute of Mental Health grant R00MH109626.
Conflict of Interest
The authors declare that the research was conducted in the absence of any commercial or financial relationships that could be construed as a potential conflict of interest.
References
Aid, T., Kazantseva, A., Piirsoo, M., Palm, K., and Timmusk, T. (2007). Mouse and rat BDNF gene structure and expression revisited. J. Neurosci. Res. 85, 525–535. doi: 10.1002/jnr.21139
Andreassi, C., and Riccio, A. (2009). To localize or not to localize: mRNA fate is in 3′UTR ends. Trends Cell Biol. 19, 465–474. doi: 10.1016/j.celrep.2020.02.047
Araki, Y., Lin, D.-T., and Huganir, R. L. (2010). Plasma membrane insertion of the AMPA receptor GluA2 subunit is regulated by NSF binding and Q/R editing of the ion pore. Proc. Natl. Acad. Sci. U S A 107, 11080–11085. doi: 10.1073/pnas.1006584107
Athanasiadis, A., Rich, A., and Maas, S. (2004). Widespread A-to-I RNA editing of Alu-containing mRNAs in the human transcriptome. PLoS Biol. 2:e391. doi: 10.1371/journal.pbio.0020391
Baculis, B. C., Weiss, A. C., Pang, W., Jeong, H. G., Lee, J. H., Liu, D.-C., et al. (2017). Prolonged seizure activity causes caspase dependent cleavage and dysfunction of G-protein activated inwardly rectifying potassium channels. Sci. Rep. 7:12313. doi: 10.1038/s41598-017-12508-y
Bae, B., and Miura, P. (2020). Emerging roles for 3′UTRs in neurons. Int. J. Mol. Sci. 21:3413. doi: 10.3390/ijms21103413
Baj, G., Leone, E., Chao, M. V., and Tongiorgi, E. (2011). Spatial segregation of BDNF transcripts enables BDNF to differentially shape distinct dendritic compartments. Proc. Natl. Acad. Sci. U S A 108, 16813–16818. doi: 10.1073/pnas.1014168108
Barbon, A., and Barlati, S. (2011). Glutamate receptor RNA editing in health and disease. Biochemistry (Mosc) 76, 882–889. doi: 10.1134/S0006297911080037
Bass, B. L., and Weintraub, H. (1987). A developmentally regulated activity that unwinds RNA duplexes. Cell 48, 607–613. doi: 10.1016/0092-8674(87)90239-x
Bass, B. L., and Weintraub, H. (1988). An unwinding activity that covalently modifies its double-stranded RNA substrate. Cell 55, 1089–1098. doi: 10.1016/0092-8674(88)90253-x
Begg, B. E., Jens, M., Wang, P. Y., Minor, C. M., and Burge, C. B. (2020). Concentration-dependent splicing is enabled by Rbfox motifs of intermediate affinity. Nat. Struct. Mol. Biol. 27, 901–912. doi: 10.1038/s41594-020-0475-8
Bender, R. A., Brewster, A., Santoro, B., Ludwig, A., Hofmann, F., Biel, M., et al. (2001). Differential and age-dependent expression of hyperpolarization-activated, cyclic nucleotide-gated cation channel isoforms 1-4 suggests evolving roles in the developing rat hippocampus. Neuroscience 106, 689–698. doi: 10.1016/s0306-4522(01)00314-1
Bender, R. A., Galindo, R., Mameli, M., Gonzalez-Vega, R., Valenzuela, C. F., and Baram, T. Z. (2005). Synchronized network activity in developing rat hippocampus involves regional hyperpolarization-activated cyclic nucleotide-gated (HCN) channel function. Eur. J. Neurosci. 22, 2669–2674. doi: 10.1111/j.1460-9568.2005.04407.x
Biever, A., Glock, C., Tushev, G., Ciirdaeva, E., Dalmay, T., Langer, J. D., et al. (2020). Monosomes actively translate synaptic mRNAs in neuronal processes. Science 367:eaay4991. doi: 10.1126/science.aay4991
Bito, H., Deisseroth, K., and Tsien, R. W. (1996). CREB phosphorylation and dephosphorylation: a Ca(2+)− and stimulus duration-dependent switch for hippocampal gene expression. Cell 87, 1203–1214. doi: 10.1016/s0092-8674(00)81816-4
Blair, J. D., Hockemeyer, D., Doudna, J. A., Bateup, H. S., and Floor, S. N. (2017). Widespread translational remodeling during human neuronal differentiation. Cell Rep. 21, 2005–2016. doi: 10.1016/j.celrep.2017.10.095
Bliss, T. V., and Lomo, T. (1973). Long-lasting potentiation of synaptic transmission in the dentate area of the anaesthetized rabbit following stimulation of the perforant path. J. Physiol. 232, 331–356. doi: 10.1113/jphysiol.1973.sp010273
Bolisetty, M. T., Rajadinakaran, G., and Graveley, B. R. (2015). Determining exon connectivity in complex mRNAs by nanopore sequencing. Genome Biol. 16:204. doi: 10.1186/s13059-015-0777-z
Brigidi, G. S., Hayes, M. G. B., Delos Santos, N. P., Hartzell, A. L., Texari, L., Lin, P.-A., et al. (2019). Genomic decoding of neuronal depolarization by stimulus-specific NPAS4 heterodimers. Cell 179, 373.e27–391.e27. doi: 10.1016/j.cell.2019.09.004
Cajigas, I. J., Tushev, G., Will, T. J., tom Dieck, S., Fuerst, N., and Schuman, E. M. (2012). The local transcriptome in the synaptic neuropil revealed by deep sequencing and high-resolution imaging. Neuron 74, 453–466. doi: 10.1016/j.neuron.2012.02.036
Carey, K. T., and Wickramasinghe, V. O. (2018). Regulatory potential of the RNA processing machinery: implications for human disease. Trends Genet. 34, 279–290. doi: 10.1016/j.tig.2017.12.012
Cembrowski, M. S., Wang, L., Sugino, K., Shields, B. C., and Spruston, N. (2016). Hipposeq: a comprehensive RNA-seq database of gene expression in hippocampal principal neurons. eLife 5:e14997. doi: 10.7554/eLife.14997
Chang, Q., Khare, G., Dani, V., Nelson, S., and Jaenisch, R. (2006). The disease progression of Mecp2 mutant mice is affected by the level of BDNF expression. Neuron 49, 341–348. doi: 10.1016/j.neuron.2005.12.027
Chapman, T. R., Barrientos, R. M., Ahrendsen, J. T., Hoover, J. M., Maier, S. F., and Patterson, S. L. (2012). Aging and infection reduce expression of specific brain-derived neurotrophic factor mRNAs in hippocampus. Neurobiol. Aging 33, e1–e14. doi: 10.1016/j.neurobiolaging.2011.07.015
Chen, W. G., Chang, Q., Lin, Y., Meissner, A., West, A. E., Griffith, E. C., et al. (2003). Derepression of BDNF transcription involves calcium-dependent phosphorylation of MeCP2. Science 302, 885–889. doi: 10.1126/science.1086446
Chen, B.-S., Gray, J. A., Sanz-Clemente, A., Wei, Z., Thomas, E. V., Nicoll, R. A., et al. (2012). SAP102 mediates synaptic clearance of NMDA receptors. Cell Rep. 2, 1120–1128. doi: 10.1016/j.celrep.2012.09.024
Chen, B.-S., Thomas, E. V., Sanz-Clemente, A., and Roche, K. W. (2011). NMDA receptor-dependent regulation of dendritic spine morphology by SAP102 splice variants. J. Neurosci. 31, 89–96. doi: 10.1523/JNEUROSCI.1034-10.2011
Chiaruttini, C., Sonego, M., Baj, G., Simonato, M., and Tongiorgi, E. (2008). BDNF mRNA splice variants display activity-dependent targeting to distinct hippocampal laminae. Mol. Cell Neurosci. 37, 11–19. doi: 10.1016/j.mcn.2007.08.011
Chung, H. J., Ge, W.-P., Qian, X., Wiser, O., Jan, Y. N., and Jan, L. Y. (2009). G protein-activated inwardly rectifying potassium channels mediate depotentiation of long-term potentiation. Proc. Natl. Acad. Sci. U S A 106, 635–640. doi: 10.1073/pnas.0811685106
Ciolli Mattioli, C., Rom, A., Franke, V., Imami, K., Arrey, G., Terne, M., et al. (2019). Alternative 3′UTRs direct localization of functionally diverse protein isoforms in neuronal compartments. Nucleic Acids Res. 47, 2560–2573. doi: 10.1093/nar/gky1270
Cohen, S. M., Suutari, B., He, X., Wang, Y., Sanchez, S., Tirko, N. N., et al. (2018). Calmodulin shuttling mediates cytonuclear signaling to trigger experience-dependent transcription and memory. Nat. Commun. 9:2451. doi: 10.1038/s41467-018-04705-8
Colliva, A., and Tongiorgi, E. (2021). Distinct role of 5′UTR sequences in dendritic trafficking of BDNF mRNA: additional mechanisms for the BDNF splice variants spatial code. Mol. Brain 14:10. doi: 10.1186/s13041-020-00680-8
Conboy, J. G. (2021). Unannotated splicing regulatory elements in deep intron space. Wiley Interdiscip. Rev. RNA. [Online ahead of print]. doi: 10.1002/wrna.1656
Corradi, E., and Baudet, M.-L. (2020). In the right place at the right time: miRNAs as key regulators in developing axons. Int. J. Mol. Sci. 21:8726. doi: 10.3390/ijms21228726
Deamer, D., Akeson, M., and Branton, D. (2016). Three decades of nanopore sequencing. Nat. Biotechnol. 34, 518–524. doi: 10.1038/nbt.3423
Deisseroth, K., Bito, H., and Tsien, R. W. (1996). Signaling from synapse to nucleus: postsynaptic CREB phosphorylation during multiple forms of hippocampal synaptic plasticity. Neuron 16, 89–101. doi: 10.1016/s0896-6273(00)80026-4
Dudek, S. M., and Bear, M. F. (1992). Homosynaptic long-term depression in area CA1 of hippocampus and effects of N-methyl-D-aspartate receptor blockade. Proc. Natl. Acad. Sci. U S A 89, 4363–4367. doi: 10.1073/pnas.89.10.4363
Durand, G. M., Kovalchuk, Y., and Konnerth, A. (1996). Long-term potentiation and functional synapse induction in developing hippocampus. Nature 381, 71–75. doi: 10.1038/381071a0
Egebjerg, J., and Heinemann, S. F. (1993). Ca2+ permeability of unedited and edited versions of the kainate selective glutamate receptor GluR6. Proc. Natl. Acad. Sci. U S A 90, 755–759. doi: 10.1073/pnas.90.2.755
Ehlers, M. D. (2000). Reinsertion or degradation of AMPA receptors determined by activity-dependent endocytic sorting. Neuron 28, 511–525. doi: 10.1016/s0896-6273(00)00129-x
Epple, R., Krüger, D., Berulava, T., Brehm, G., Islam, R., Köster, S., et al. (2021). The coding and small non-coding hippocampal synaptic RNAome. Mol. Neurobiol. 58, 2940–2953. doi: 10.1007/s12035-021-02296-y
Estravís, M., Rincón, S. A., Portales, E., Pérez, P., and Santos, B. (2017). Cdc42 activation state affects its localization and protein levels in fission yeast. Microbiology (Reading) 163, 1156–1166. doi: 10.1099/mic.0.000503
Ewald, R. C., and Cline, H. T. (2009). “NMDA receptors and brain development,” in Biology of the NMDA Receptor, ed A. M. Van Dongen (Boca Raton, FL: CRC Press/Taylor & Francis).
Falley, K., Schütt, J., Iglauer, P., Menke, K., Maas, C., Kneussel, M., et al. (2009). Shank1 mRNA: dendritic transport by kinesin and translational control by the 5′untranslated region. Traffic 10, 844–857. doi: 10.1111/j.1600-0854.2009.00912.x
Farris, S., Ward, J. M., Carstens, K. E., Samadi, M., Wang, Y., and Dudek, S. M. (2019). Hippocampal subregions express distinct dendritic transcriptomes that reveal differences in mitochondrial function in CA2. Cell Rep. 29, 522.e6–539.e6. doi: 10.1016/j.celrep.2019.08.093
Feng, H., Moakley, D. F., Chen, S., McKenzie, M. G., Menon, V., and Zhang, C. (2021). Complexity and graded regulation of neuronal cell-type-specific alternative splicing revealed by single-cell RNA sequencing. Proc. Natl. Acad. Sci. U S A 118:e2013056118. doi: 10.1073/pnas.2013056118
Fischer, U., Englbrecht, C., and Chari, A. (2011). Biogenesis of spliceosomal small nuclear ribonucleoproteins. Wiley Interdiscip. Rev. RNA 2, 718–731. doi: 10.1002/wrna.87
Foltran, R. B., and Diaz, S. L. (2016). BDNF isoforms: a round trip ticket between neurogenesis and serotonin. J. Neurochem. 138, 204–221. doi: 10.1111/jnc.13658
Fontes, M. M., Guvenek, A., Kawaguchi, R., Zheng, D., Huang, A., Ho, V. M., et al. (2017). Activity-dependent regulation of alternative cleavage and polyadenylation during hippocampal long-term potentiation. Sci. Rep. 7:17377. doi: 10.1038/s41598-017-17407-w
Frey, U., and Morris, R. G. (1997). Synaptic tagging and long-term potentiation. Nature 385, 533–536. doi: 10.1038/385533a0
Furlanis, E., and Scheiffele, P. (2018). Regulation of neuronal differentiation, function and plasticity by alternative splicing. Annu. Rev. Cell Dev. Biol. 34, 451–469. doi: 10.1146/annurev-cellbio-100617-062826
Furlanis, E., Traunmüller, L., Fucile, G., and Scheiffele, P. (2019). Landscape of ribosome-engaged transcript isoforms reveals extensive neuronal-cell-class-specific alternative splicing programs. Nat. Neurosci. 22, 1709–1717. doi: 10.1038/s41593-019-0465-5
Garaschuk, O., Hanse, E., and Konnerth, A. (1998). Developmental profile and synaptic origin of early network oscillations in the CA1 region of rat neonatal hippocampus. J. Physiol. 507, 219–236. doi: 10.1111/j.1469-7793.1998.219bu.x
Gokool, A., Loy, C. T., Halliday, G. M., and Voineagu, I. (2020). Circular RNAs: the brain transcriptome comes full circle. Trends Neurosci. 43, 752–766. doi: 10.1016/j.tins.2020.07.007
Gonatopoulos-Pournatzis, T., and Blencowe, B. J. (2020). Microexons: at the nexus of nervous system development, behaviour and autism spectrum disorder. Curr. Opin. Genet. Dev. 65, 22–33. doi: 10.1016/j.gde.2020.03.007
Grabowski, P. J., and Black, D. L. (2001). Alternative RNA splicing in the nervous system. Prog. Neurobiol. 65, 289–308. doi: 10.1016/s0301-0082(01)00007-7
Gray, A. C., Raingo, J., and Lipscombe, D. (2007). Neuronal calcium channels: splicing for optimal performance. Cell Calcium 42, 409–417. doi: 10.1016/j.ceca.2007.04.003
Gurung, S., Evans, A. J., Wilkinson, K. A., and Henley, J. M. (2018). ADAR2-mediated Q/R editing of GluK2 regulates kainate receptor upscaling in response to suppression of synaptic activity. J. Cell Sci. 131:jcs222273. doi: 10.1242/jcs.222273
Ha, K. C. H., Sterne-Weiler, T., Morris, Q., Weatheritt, R. J., and Blencowe, B. J. (2021). Differential contribution of transcriptomic regulatory layers in the definition of neuronal identity. Nat. Commun. 12:335. doi: 10.1038/s41467-020-20483-8
Han, Y., Lyman, K. A., Foote, K. M., and Chetkovich, D. M. (2020). The structure and function of TRIP8b, an auxiliary subunit of hyperpolarization-activated cyclic-nucleotide gated channels. Channels (Austin) 14, 110–122. doi: 10.1080/19336950.2020.1740501
Hermey, G., Blüthgen, N., and Kuhl, D. (2017). Neuronal activity-regulated alternative mRNA splicing. Int. J. Biochem. Cell Biol. 91, 184–193. doi: 10.1016/j.biocel.2017.06.002
Hibino, H., Inanobe, A., Furutani, K., Murakami, S., Findlay, I., and Kurachi, Y. (2010). Inwardly rectifying potassium channels: their structure, function and physiological roles. Physiol. Rev. 90, 291–366. doi: 10.1152/physrev.00021.2009
Higuchi, M., Single, F. N., Köhler, M., Sommer, B., Sprengel, R., and Seeburg, P. H. (1993). RNA editing of AMPA receptor subunit GluR-B: a base-paired intron-exon structure determines position and efficiency. Cell 75, 1361–1370. doi: 10.1016/0092-8674(93)90622-w
Hinman, M. N., Zhou, H.-L., Sharma, A., and Lou, H. (2013). All three RNA recognition motifs and the hinge region of HuC play distinct roles in the regulation of alternative splicing. Nucleic Acids Res. 41, 5049–5061. doi: 10.1093/nar/gkt166
Holt, C. E., Martin, K. C., and Schuman, E. M. (2019). Local translation in neurons: visualization and function. Nat. Struct. Mol. Biol. 26, 557–566. doi: 10.1038/s41594-019-0263-5
Hoque, M., Ji, Z., Zheng, D., Luo, W., Li, W., You, B., et al. (2013). Analysis of alternative cleavage and polyadenylation by 3′ region extraction and deep sequencing. Nat. Methods 10, 133–139. doi: 10.1038/nmeth.2288
Hu, Z., and Li, Z. (2017). miRNAs in synapse development and synaptic plasticity. Curr. Opin. Neurobiol. 45, 24–31. doi: 10.1016/j.conb.2017.02.014
Iijima, T., Hidaka, C., and Iijima, Y. (2016). Spatio-temporal regulations and functions of neuronal alternative RNA splicing in developing and adult brains. Neurosci. Res. 109, 1–8. doi: 10.1016/j.neures.2016.01.010
Inanobe, A., Yoshimoto, Y., Horio, Y., Morishige, K. I., Hibino, H., Matsumoto, S., et al. (1999). Characterization of G-protein-gated K+ channels composed of Kir3.2 subunits in dopaminergic neurons of the substantia nigra. J. Neurosci. 19, 1006–1017. doi: 10.1523/JNEUROSCI.19-03-01006.1999
Irimia, M., Weatheritt, R. J., Ellis, J. D., Parikshak, N. N., Gonatopoulos-Pournatzis, T., Babor, M., et al. (2014). A highly conserved program of neuronal microexons is misregulated in autistic brains. Cell 159, 1511–1523. doi: 10.1016/j.cell.2014.11.035
Jacko, M., Weyn-Vanhentenryck, S. M., Smerdon, J. W., Yan, R., Feng, H., Williams, D. J., et al. (2018). Rbfox splicing factors promote neuronal maturation and axon initial segment assembly. Neuron 97, 853.e6–868.e6. doi: 10.1016/j.neuron.2018.01.020
Jensen, K. B., Dredge, B. K., Stefani, G., Zhong, R., Buckanovich, R. J., Okano, H. J., et al. (2000). Nova-1 regulates neuron-specific alternative splicing and is essential for neuronal viability. Neuron 25, 359–371. doi: 10.1016/s0896-6273(00)80900-9
Joglekar, A., Prjibelski, A., Mahfouz, A., Collier, P., Lin, S., Schlusche, A. K., et al. (2021). A spatially resolved brain region- and cell type-specific isoform atlas of the postnatal mouse brain. Nat. Commun. 12:463. doi: 10.1038/s41467-020-20343-5
Kang, R., Wan, J., Arstikaitis, P., Takahashi, H., Huang, K., Bailey, A. O., et al. (2008). Neural palmitoyl-proteomics reveals dynamic synaptic palmitoylation. Nature 456, 904–909. doi: 10.1038/nature07605
Kapoor, U., Licht, K., Amman, F., Jakobi, T., Martin, D., Dieterich, C., et al. (2020). ADAR-deficiency perturbs the global splicing landscape in mouse tissues. Genome Res. 30, 1107–1118. doi: 10.1101/gr.256933.119
Kasyanov, A. M., Safiulina, V. F., Voronin, L. L., and Cherubini, E. (2004). GABA-mediated giant depolarizing potentials as coincidence detectors for enhancing synaptic efficacy in the developing hippocampus. Proc. Natl. Acad. Sci. U S A 101, 3967–3972. doi: 10.1073/pnas.0305974101
KhorshidAhmad, T., Acosta, C., Cortes, C., Lakowski, T. M., Gangadaran, S., and Namaka, M. (2016). Transcriptional regulation of brain-derived neurotrophic factor (BDNF) by methyl CpG binding protein 2 (MeCP2) a novel mechanism for re-myelination and/or myelin repair involved in the treatment of multiple sclerosis (MS). Mol. Neurobiol. 53, 1092–1107. doi: 10.1007/s12035-014-9074-1
Kim, I. H., Wang, H., Soderling, S. H., and Yasuda, R. (2014). Loss of Cdc42 leads to defects in synaptic plasticity and remote memory recall. eLife 3:e02839. doi: 10.7554/eLife.02839
Konen, L. M., Wright, A. L., Royle, G. A., Morris, G. P., Lau, B. K., Seow, P. W., et al. (2020). A new mouse line with reduced GluA2 Q/R site RNA editing exhibits loss of dendritic spines, hippocampal CA1-neuron loss, learning and memory impairments and NMDA receptor-independent seizure vulnerability. Mol. Brain 13:27. doi: 10.1186/s13041-020-0545-1
Kumar, M., and Carmichael, G. G. (1997). Nuclear antisense RNA induces extensive adenosine modifications and nuclear retention of target transcripts. Proc. Natl. Acad. Sci. U S A 94, 3542–3547. doi: 10.1073/pnas.94.8.3542
La Via, L., Bonini, D., Russo, I., Orlandi, C., Barlati, S., and Barbon, A. (2013). Modulation of dendritic AMPA receptor mRNA trafficking by RNA splicing and editing. Nucleic Acids Res. 41, 617–631. doi: 10.1093/nar/gks1223
Leal, G., Comprido, D., and Duarte, C. B. (2014). BDNF-induced local protein synthesis and synaptic plasticity. Neuropharmacology 76, 639–656. doi: 10.1016/j.neuropharm.2013.04.005
Lee, S. J., Zdradzinski, M. D., Sahoo, P. K., Kar, A. N., Patel, P., Kawaguchi, R., et al. (2021). Selective axonal translation of the mRNA isoform encoding prenylated Cdc42 supports axon growth. J. Cell Sci. 134:jcs251967. doi: 10.1242/jcs.251967
Legendre, M., Ritchie, W., Lopez, F., and Gautheret, D. (2006). Differential repression of alternative transcripts: a screen for miRNA targets. PLoS Comput. Biol. 2:e43. doi: 10.1371/journal.pcbi.0020043
Lewis, A. S., Schwartz, E., Chan, C. S., Noam, Y., Shin, M., Wadman, W. J., et al. (2009). Alternatively spliced isoforms of TRIP8b differentially control h channel trafficking and function. J. Neurosci. 29, 6250–6265. doi: 10.1523/JNEUROSCI.0856-09.2009
Li, Y., Bor, Y.-C., Fitzgerald, M. P., Lee, K. S., Rekosh, D., and Hammarskjold, M.-L. (2016). An NXF1 mRNA with a retained intron is expressed in hippocampal and neocortical neurons and is translated into a protein that functions as an Nxf1 cofactor. Mol. Biol. Cell 27, 3903–3912. doi: 10.1091/mbc.E16-07-0515
Li, Y., Bor, Y.-C., Misawa, Y., Xue, Y., Rekosh, D., and Hammarskjöld, M.-L. (2006). An intron with a constitutive transport element is retained in a Tap messenger RNA. Nature 443, 234–237. doi: 10.1038/nature05107
Lipscombe, D. (2005). Neuronal proteins custom designed by alternative splicing. Curr. Opin. Neurobiol. 15, 358–363. doi: 10.1016/j.conb.2005.04.002
Lipscombe, D., and Pan, J. Q. (2009). Tripping the HCN breaker. Neuron 62, 747–750. doi: 10.1016/j.neuron.2009.06.003
Lomeli, H., Mosbacher, J., Melcher, T., Höger, T., Geiger, J. R., Kuner, T., et al. (1994). Control of kinetic properties of AMPA receptor channels by nuclear RNA editing. Science 266, 1709–1713. doi: 10.1126/science.7992055
Lüscher, C., Xia, H., Beattie, E. C., Carroll, R. C., von Zastrow, M., Malenka, R. C., et al. (1999). Role of AMPA receptor cycling in synaptic transmission and plasticity. Neuron 24, 649–658. doi: 10.1016/s0896-6273(00)81119-8
Ma, H., Groth, R. D., Cohen, S. M., Emery, J. F., Li, B., Hoedt, E., et al. (2014). γCaMKII shuttles Ca2+/CaM to the nucleus to trigger CREB phosphorylation and gene expression. Cell 159, 281–294. doi: 10.1016/j.cell.2014.09.019
Magee, J. C. (1999). Dendritic I h normalizes temporal summation in hippocampal CA1 neurons. Nat. Neurosci. 2, 508–514.
Majoros, W. H., and Ohler, U. (2007). Spatial preferences of microRNA targets in 3′ untranslated regions. BMC Genomics 8:152. doi: 10.1186/1471-2164-8-152
Marks, P. W., and Kwiatkowski, D. J. (1996). Genomic organization and chromosomal location of murine Cdc42. Genomics 38, 13–18. doi: 10.1006/geno.1996.0586
Marron Fernandez de Velasco, E., Zhang, L., N Vo, B., Tipps, M., Farris, S., Xia, Z., et al. (2017). GIRK2 splice variants and neuronal G protein-gated K+ channels: implications for channel function and behavior. Sci. Rep. 7:1639. doi: 10.1038/s41598-017-01820-2
Martinowich, K., Hattori, D., Wu, H., Fouse, S., He, F., Hu, Y., et al. (2003). DNA methylation-related chromatin remodeling in activity-dependent BDNF gene regulation. Science 302, 890–893. doi: 10.1126/science.1090842
Masser, D. R., Bixler, G. V., Brucklacher, R. M., Yan, H., Giles, C. B., Wren, J. D., et al. (2014). Hippocampal subregions exhibit both distinct and shared transcriptomic responses to aging and nonneurodegenerative cognitive decline. J. Gerontol. A Biol. Sci. Med. Sci. 69, 1311–1324. doi: 10.1093/gerona/glu091
Mayford, M., Baranes, D., Podsypanina, K., and Kandel, E. R. (1996). The 3′-untranslated region of CaMKII alpha is a cis-acting signal for the localization and translation of mRNA in dendrites. Proc. Natl. Acad. Sci. U S A 93, 13250–13255. doi: 10.1073/pnas.93.23.13250
Maynard, K. R., Hobbs, J. W., Sukumar, M., Kardian, A. S., Jimenez, D. V., Schloesser, R. J., et al. (2017). Bdnf mRNA splice variants differentially impact CA1 and CA3 dendrite complexity and spine morphology in the hippocampus. Brain Struct. Funct. 222, 3295–3307. doi: 10.1007/s00429-017-1405-3
Mayya, V. K., and Duchaine, T. F. (2019). Ciphers and executioners: how 3′-untranslated regions determine the fate of messenger RNAs. Front. Genet. 10:6. doi: 10.3389/fgene.2019.00006
McCann, K. E., Lustberg, D. J., Shaughnessy, E. K., Carstens, K. E., Farris, S., Alexander, G. M., et al. (2021). Novel role for mineralocorticoid receptors in control of a neuronal phenotype. Mol. Psychiatry 26, 350–364. doi: 10.1038/s41380-019-0598-7
Meissner, A., Mikkelsen, T. S., Gu, H., Wernig, M., Hanna, J., Sivachenko, A., et al. (2008). Genome-scale DNA methylation maps of pluripotent and differentiated cells. Nature 454, 766–770. doi: 10.1038/nature07107
Metsis, M., Timmusk, T., Arenas, E., and Persson, H. (1993). Differential usage of multiple brain-derived neurotrophic factor promoters in the rat brain following neuronal activation. Proc. Natl. Acad. Sci. U S A 90, 8802–8806. doi: 10.1073/pnas.90.19.8802
Miller, S., Yasuda, M., Coats, J. K., Jones, Y., Martone, M. E., and Mayford, M. (2002). Disruption of dendritic translation of CaMKIIalpha impairs stabilization of synaptic plasticity and memory consolidation. Neuron 36, 507–519. doi: 10.1016/s0896-6273(02)00978-9
Miura, P., Shenker, S., Andreu-Agullo, C., Westholm, J. O., and Lai, E. C. (2013). Widespread and extensive lengthening of 3′UTRs in the mammalian brain. Genome Res. 23, 812–825. doi: 10.1101/gr.146886.112
Monyer, H., Burnashev, N., Laurie, D. J., Sakmann, B., and Seeburg, P. H. (1994). Developmental and regional expression in the rat brain and functional properties of four NMDA receptors. Neuron 12, 529–540. doi: 10.1016/0896-6273(94)90210-0
Moore, M. J. (2005). From birth to death: the complex lives of eukaryotic mRNAs. Science 309, 1514–1518. doi: 10.1126/science.1111443
Mori, Y., Imaizumi, K., Katayama, T., Yoneda, T., and Tohyama, M. (2000). Two cis-acting elements in the 3′ untranslated region of α-CaMKII regulate its dendritic targeting. Nat. Neurosci. 3, 1079–1084. doi: 10.1038/80591
Moutin, E., Nikonenko, I., Stefanelli, T., Wirth, A., Ponimaskin, E., De Roo, M., et al. (2017). Palmitoylation of cdc42 promotes spine stabilization and rescues spine density deficit in a mouse model of 22q11.2 deletion syndrome. Cereb. Cortex 27, 3618–3629. doi: 10.1093/cercor/bhw183
Müller, B. M., Kistner, U., Kindler, S., Chung, W. J., Kuhlendahl, S., Fenster, S. D., et al. (1996). SAP102, a novel postsynaptic protein that interacts with NMDA receptor complexes in vivo. Neuron 17, 255–265. doi: 10.1055/s-0041-1730312
Munemitsu, S., Innis, M. A., Clark, R., McCormick, F., Ullrich, A., and Polakis, P. (1990). Molecular cloning and expression of a G25K cDNA, the human homolog of the yeast cell cycle gene CDC42. Mol. Cell. Biol. 10, 5977–5982. doi: 10.1128/mcb.10.11.5977-5982.1990
Murakoshi, H., Wang, H., and Yasuda, R. (2011). Local, persistent activation of Rho GTPases during plasticity of single dendritic spines. Nature 472, 100–104. doi: 10.1038/nature09823
Neves, G., Cooke, S. F., and Bliss, T. V. P. (2008). Synaptic plasticity, memory and the hippocampus: a neural network approach to causality. Nat. Rev. Neurosci. 9, 65–75. doi: 10.1038/nrn2303
Nguyen, P. V., and Kandel, E. R. (1997). Brief theta-burst stimulation induces a transcription-dependent late phase of LTP requiring cAMP in area CA1 of the mouse hippocampus. Learn. Mem. 4, 230–243. doi: 10.1101/lm.4.2.230
Oe, S., and Yoneda, Y. (2010). Cytoplasmic polyadenylation element-like sequences are involved in dendritic targeting of BDNF mRNA in hippocampal neurons. FEBS Lett. 584, 3424–3430. doi: 10.1016/j.febslet.2010.06.040
Olenik, C., Barth, H., Just, I., Aktories, K., and Meyer, D. K. (1997). Gene expression of the small GTP-binding proteins RhoA, RhoB, Rac1 and Cdc42 in adult rat brain. Mol. Brain Res. 52, 263–269. doi: 10.1016/s0169-328x(97)00270-2
Ouwenga, R., Lake, A. M., O’Brien, D., Mogha, A., Dani, A., and Dougherty, J. D. (2017). Transcriptomic analysis of Ribosome-bound mRNA in cortical neurites in vivo. J. Neurosci. 37, 8688–8705. doi: 10.1523/JNEUROSCI.3044-16.2017
Pan, Q., Shai, O., Lee, L. J., Frey, B. J., and Blencowe, B. J. (2008). Deep surveying of alternative splicing complexity in the human transcriptome by high-throughput sequencing. Nat. Genet. 40, 1413–1415. doi: 10.1038/ng.259
Pardo, L. M., Rizzu, P., Francescatto, M., Vitezic, M., Leday, G. G. R., Sanchez, J. S., et al. (2013). Regional differences in gene expression and promoter usage in aged human brains. Neurobiol. Aging 34, 1825–1836. doi: 10.1016/j.neurobiolaging.2013.01.005
Penn, A. C., Balik, A., Wozny, C., Cais, O., and Greger, I. H. (2012). Activity-mediated AMPA receptor remodeling, driven by alternative splicing in the ligand-binding domain. Neuron 76, 503–510. doi: 10.1016/j.neuron.2012.08.010
Piskorowski, R., Santoro, B., and Siegelbaum, S. A. (2011). TRIP8b splice forms act in concert to regulate the localization and expression of HCN1 channels in CA1 pyramidal neurons. Neuron 70, 495–509. doi: 10.1016/j.neuron.2011.03.023
Potter, W. B., O’Riordan, K. J., Barnett, D., Osting, S. M. K., Wagoner, M., Burger, C., et al. (2010). Metabolic regulation of neuronal plasticity by the energy sensor AMPK. PLoS One 5:e8996. doi: 10.1371/journal.pone.0008996
Pruunsild, P., Kazantseva, A., Aid, T., Palm, K., and Timmusk, T. (2007). Dissecting the human BDNF locus: bidirectional transcription, complex splicing and multiple promoters. Genomics 90, 397–406. doi: 10.1016/j.ygeno.2007.05.004
Pruunsild, P., Sepp, M., Orav, E., Koppel, I., and Timmusk, T. (2011). Identification of cis-elements and transcription factors regulating neuronal activity-dependent transcription of human BDNF gene. J. Neurosci. 31, 3295–3308. doi: 10.1523/JNEUROSCI.4540-10.2011
Raj, B., and Blencowe, B. J. (2015). Alternative splicing in the mammalian nervous system: recent insights into mechanisms and functional roles. Neuron 87, 14–27. doi: 10.1016/j.neuron.2015.05.004
Reixachs-Solé, M., Ruiz-Orera, J., Albà, M. M., and Eyras, E. (2020). Ribosome profiling at isoform level reveals evolutionary conserved impacts of differential splicing on the proteome. Nat. Commun. 11:1768. doi: 10.1038/s41467-020-15634-w
Roberts, P. J., Mitin, N., Keller, P. J., Chenette, E. J., Madigan, J. P., Currin, R. O., et al. (2008). Rho Family GTPase modification and dependence on CAAX motif-signaled posttranslational modification. J. Biol. Chem. 283, 25150–25163. doi: 10.1074/jbc.M800882200
Rybak-Wolf, A., Stottmeister, C., Glažar, P., Jens, M., Pino, N., Giusti, S., et al. (2015). Circular RNAs in the mammalian brain are highly abundant, conserved and dynamically expressed. Mol. Cell 58, 870–885. doi: 10.1016/j.molcel.2015.03.027
Saba, R., Störchel, P. H., Aksoy-Aksel, A., Kepura, F., Lippi, G., Plant, T. D., et al. (2012). Dopamine-regulated microRNA MiR-181a controls GluA2 surface expression in hippocampal neurons. Mol. Cell. Biol. 32, 619–632. doi: 10.1128/MCB.05896-11
Sakura, H., Bond, C., Warren-Perry, M., Horsley, S., Kearney, L., Tucker, S., et al. (1995). Characterization and variation of a human inwardly-rectifying-K-channel gene (KCNJ6): a putative ATP-sensitive K-channel subunit. FEBS Lett. 367, 193–197. doi: 10.1016/0014-5793(95)00498-x
Sambandan, S., Akbalik, G., Kochen, L., Rinne, J., Kahlstatt, J., Glock, C., et al. (2017). Activity-dependent spatially localized miRNA maturation in neuronal dendrites. Science 355, 634–637. doi: 10.1126/science.aaf8995
Sans, N., Petralia, R. S., Wang, Y. X., Blahos, J., Hell, J. W., and Wenthold, R. J. (2000). A developmental change in NMDA receptor-associated proteins at hippocampal synapses. J. Neurosci. 20, 1260–1271. doi: 10.1523/JNEUROSCI.20-03-01260.2000
Santoro, B., Piskorowski, R. A., Pian, P., Hu, L., Liu, H., and Siegelbaum, S. A. (2009). TRIP8b splice variants form a family of auxiliary subunits that regulate gating and trafficking of HCN channels in the brain. Neuron 62, 802–813. doi: 10.1016/j.neuron.2009.05.009
Santoro, B., Wainger, B. J., and Siegelbaum, S. A. (2004). Regulation of HCN channel surface expression by a novel C-terminal protein-protein interaction. J. Neurosci. 24, 10750–10762. doi: 10.1523/JNEUROSCI.3300-04.2004
Sapkota, D., Lake, A. M., Yang, W., Yang, C., Wesseling, H., Guise, A., et al. (2019). Cell-type-specific profiling of alternative translation identifies regulated protein isoform variation in the mouse brain. Cell Rep. 26, 594.e7–607.e7. doi: 10.1016/j.celrep.2018.12.077
Sharangdhar, T., Sugimoto, Y., Heraud-Farlow, J., Fernández-Moya, S. M., Ehses, J., Ruiz de Los Mozos, I., et al. (2017). A retained intron in the 3′-UTR of Calm3 mRNA mediates its Staufen2- and activity-dependent localization to neuronal dendrites. EMBO Rep. 18, 1762–1774. doi: 10.15252/embr.201744334
Shibata, A. C. E., Ueda, H. H., Eto, K., Onda, M., Sato, A., Ohba, T., et al. (2021). Photoactivatable CaMKII induces synaptic plasticity in single synapses. Nat. Commun. 12:751. doi: 10.1038/s41467-021-21025-6
Shieh, P. B., Hu, S. C., Bobb, K., Timmusk, T., and Ghosh, A. (1998). Identification of a signaling pathway involved in calcium regulation of BDNF expression. Neuron 20, 727–740. doi: 10.1016/s0896-6273(00)81011-9
Shinjo, K., Koland, J. G., Hart, M. J., Narasimhan, V., Johnson, D. I., Evans, T., et al. (1990). Molecular cloning of the gene for the human placental GTP-binding protein Gp (G25K): identification of this GTP-binding protein as the human homolog of the yeast cell-division-cycle protein CDC42. Proc. Natl. Acad. Sci. U S A 87, 9853–9857. doi: 10.1073/pnas.87.24.9853
Smith, S. A., Holik, P., Stevens, J., Mazoyer, S., Melis, R., Williams, B., et al. (1996). Isolation of a gene (DLG3) encoding a second member of the discs-large family on chromosome 17q12–q21. Genomics 31, 145–150. doi: 10.1006/geno.1996.0025
Smith, R. S., and Walsh, C. A. (2020). Ion channel functions in early brain development. Trends Neurosci. 43, 103–114. doi: 10.1016/j.tins.2019.12.004
Sommer, B., Keinänen, K., Verdoorn, T. A., Wisden, W., Burnashev, N., Herb, A., et al. (1990). Flip and flop: a cell-specific functional switch in glutamate-operated channels of the CNS. Science 249, 1580–1585. doi: 10.1126/science.1699275
Steward, O., and Schuman, E. M. (2003). Compartmentalized synthesis and degradation of proteins in neurons. Neuron 40, 347–359. doi: 10.1016/s0896-6273(03)00635-4
Stilling, R. M., Benito, E., Gertig, M., Barth, J., Capece, V., Burkhardt, S., et al. (2014). De-regulation of gene expression and alternative splicing affects distinct cellular pathways in the aging hippocampus. Front. Cell. Neurosci. 8:373. doi: 10.3389/fncel.2014.00373
Stoenica, L., Wilkars, W., Battefeld, A., Stadler, K., Bender, R., and Strauss, U. (2013). HCN1 subunits contribute to the kinetics of Ih in neonatal cortical plate neurons. Dev. Neurobiol. 73, 785–797. doi: 10.1002/dneu.22104
Su, C.-H., Dhananjaya, D., and Tarn, W.-Y. (2018). Alternative splicing in neurogenesis and brain development. Front. Mol. Biosci. 5:12. doi: 10.3389/fmolb.2018.00012
Südhof, T. C. (2017). Synaptic neurexin complexes: a molecular code for the logic of neural circuits. Cell 171, 745–769. doi: 10.1055/s-0041-1726373
Taliaferro, J. M., Vidaki, M., Oliveira, R., Olson, S., Zhan, L., Saxena, T., et al. (2016). Distal alternative last exons localize mRNAs to neural projections. Mol. Cell 61, 821–833. doi: 10.1016/j.molcel.2016.01.020
Tanaka, H., Grooms, S. Y., Bennett, M. V., and Zukin, R. S. (2000). The AMPAR subunit GluR2: still front and center-stage. Brain Res. 886, 190–207. doi: 10.1016/s0006-8993(00)02951-6
Tao, X., Finkbeiner, S., Arnold, D. B., Shaywitz, A. J., and Greenberg, M. E. (1998). Ca2+ influx regulates BDNF transcription by a CREB family transcription factor-dependent mechanism. Neuron 20, 709–726. doi: 10.1016/s0896-6273(00)81010-7
Thompson, M., Bixby, R., Dalton, R., Vandenburg, A., Calarco, J. A., and Norris, A. D. (2019). Splicing in a single neuron is coordinately controlled by RNA binding proteins and transcription factors. eLife 8:e46726. doi: 10.7554/eLife.46726
Timmusk, T., Palm, K., Metsis, M., Reintam, T., Paalme, V., Saarma, M., et al. (1993). Multiple promoters direct tissue-specific expression of the rat BDNF gene. Neuron 10, 475–489. doi: 10.1016/0896-6273(93)90335-o
Tushev, G., Glock, C., Heumüller, M., Biever, A., Jovanovic, M., and Schuman, E. M. (2018). Alternative 3′UTRs modify the localization, regulatory potential, stability and plasticity of mRNAs in neuronal compartments. Neuron 98, 495.e6–511.e6. doi: 10.1016/j.neuron.2018.03.030
Twine, N. A., Janitz, C., Wilkins, M. R., and Janitz, M. (2013). Sequencing of hippocampal and cerebellar transcriptomes provides new insights into the complexity of gene regulation in the human brain. Neurosci. Lett. 541, 263–268. doi: 10.1016/j.neulet.2013.02.034
Ule, J., Stefani, G., Mele, A., Ruggiu, M., Wang, X., Taneri, B., et al. (2006). An RNA map predicting Nova-dependent splicing regulation. Nature 444, 580–586. doi: 10.1038/nature05304
Vanevski, F., and Xu, B. (2015). HuD interacts with Bdnf mRNA and is essential for activity-induced BDNF synthesis in dendrites. PLoS One 10:e0117264. doi: 10.1371/journal.pone.0117264
Vicario, A., Colliva, A., Ratti, A., Davidovic, L., Baj, G., Gricman, Ł., et al. (2015). Dendritic targeting of short and long 3′ UTR BDNF mRNA is regulated by BDNF or NT-3 and distinct sets of RNA-binding proteins. Front. Mol. Neurosci. 8:62. doi: 10.3389/fnmol.2015.00062
Vuong, C. K., Black, D. L., and Zheng, S. (2016). The neurogenetics of alternative splicing. Nat. Rev. Neurosci. 17, 265–281. doi: 10.1038/nrn.2016.27
Wagner, R. W., Smith, J. E., Cooperman, B. S., and Nishikura, K. (1989). A double-stranded RNA unwinding activity introduces structural alterations by means of adenosine to inosine conversions in mammalian cells and Xenopus eggs. Proc. Natl. Acad. Sci. U S A 86, 2647–2651. doi: 10.1073/pnas.86.8.2647
Walkley, C. R., and Li, J. B. (2017). Rewriting the transcriptome: adenosine-to-inosine RNA editing by ADARs. Genome Biol. 18:205. doi: 10.1186/s13059-017-1347-3
Wang, E. T., Cody, N. A. L., Jog, S., Biancolella, M., Wang, T. T., Treacy, D. J., et al. (2012). Transcriptome-wide regulation of pre-mRNA splicing and mRNA localization by muscleblind proteins. Cell 150, 710–724. doi: 10.1016/j.cell.2012.06.041
Wang, E. T., Ward, A. J., Cherone, J. M., Giudice, J., Wang, T. T., Treacy, D. J., et al. (2015). Antagonistic regulation of mRNA expression and splicing by CELF and MBNL proteins. Genome Res. 25, 858–871. doi: 10.1101/gr.184390.114
Wang, X., Wang, K., Radovich, M., Wang, Y., Wang, G., Feng, W., et al. (2009). Genome-wide prediction of cis-acting RNA elements regulating tissue-specific pre-mRNA alternative splicing. BMC Genomics 10:S4. doi: 10.1186/1471-2164-10-S1-S4
Wang, X., You, X., Langer, J. D., Hou, J., Rupprecht, F., Vlatkovic, I., et al. (2019). Full-length transcriptome reconstruction reveals a large diversity of RNA and protein isoforms in rat hippocampus. Nat. Commun. 10:5009. doi: 10.1038/s41467-019-13037-0
Watanabe, M., Mishina, M., and Inoue, Y. (1994). Distinct spatiotemporal expressions of five NMDA receptor channel subunit mRNAs in the cerebellum. J. Comp. Neurol. 343, 513–519. doi: 10.1002/cne.903430402
Wei, Z., Behrman, B., Wu, W.-H., and Chen, B.-S. (2015). Subunit-specific regulation of N-methyl-D-aspartate (NMDA) receptor trafficking by SAP102 protein splice variants. J. Biol. Chem. 290, 5105–5116. doi: 10.1074/jbc.M114.599969
Wei, J., Hodes, M. E., Piva, R., Feng, Y., Wang, Y., Ghetti, B., et al. (1998). Characterization of murine Girk2 transcript isoforms: structure and differential expression. Genomics 51, 379–390. doi: 10.1006/geno.1998.5369
Wei, Z., Wu, G., and Chen, B.-S. (2018). Regulation of SAP102 synaptic targeting by phosphorylation. Mol. Neurobiol. 55, 6215–6226. doi: 10.1007/s12035-017-0836-4
Wen, W., Lin, C.-Y., and Niu, L. (2017). R/G editing in GluA2Rflop modulates the functional difference between GluA1 flip and flop variants in GluA1/2R heteromeric channels. Sci. Rep. 7:13654. doi: 10.1038/s41598-017-13233-2
Weyn-Vanhentenryck, S. M., Feng, H., Ustianenko, D., Duffié, R., Yan, Q., Jacko, M., et al. (2018). Precise temporal regulation of alternative splicing during neural development. Nat. Commun. 9:2189. doi: 10.1038/s41467-018-04559-0
Wirth, A., Chen-Wacker, C., Wu, Y.-W., Gorinski, N., Filippov, M. A., Pandey, G., et al. (2013). Dual lipidation of the brain-specific Cdc42 isoform regulates its functional properties. Biochem. J. 456, 311–322. doi: 10.1042/BJ20130788
Workman, R. E., Tang, A. D., Tang, P. S., Jain, M., Tyson, J. R., Razaghi, R., et al. (2019). Nanopore native RNA sequencing of a human poly(A) transcriptome. Nat. Methods 16, 1297–1305. doi: 10.1038/s41592-019-0617-2
Wu, C.-T., Chiou, C.-Y., Chiu, H.-C., and Yang, U.-C. (2013). Fine-tuning of microRNA-mediated repression of mRNA by splicing-regulated and highly repressive microRNA recognition element. BMC Genomics 14:438. doi: 10.1186/1471-2164-14-438
Yang, J. H., Sklar, P., Axel, R., and Maniatis, T. (1995). Editing of glutamate receptor subunit B pre-mRNA in vitro by site-specific deamination of adenosine. Nature 374, 77–81. doi: 10.1038/374077a0
Yap, K., Xiao, Y., Friedman, B. A., Je, H. S., and Makeyev, E. V. (2016). Polarizing the neuron through sustained co-expression of alternatively spliced isoforms. Cell Rep. 15, 1316–1328. doi: 10.1016/j.celrep.2016.04.012
Yee, B. A., Pratt, G. A., Graveley, B. R., Van Nostrand, E. L., and Yeo, G. W. (2019). RBP-Maps enables robust generation of splicing regulatory maps. RNA 25, 193–204. doi: 10.1261/rna.069237.118
Yeo, G., Holste, D., Kreiman, G., and Burge, C. B. (2004). Variation in alternative splicing across human tissues. Genome Biol. 5:R74. doi: 10.1186/gb-2004-5-10-r74
Yokoi, S., Udagawa, T., Fujioka, Y., Honda, D., Okado, H., Watanabe, H., et al. (2017). 3′UTR length-dependent control of synGAP isoform α2 mRNA by FUS and ELAV-like proteins promotes dendritic spine maturation and cognitive function. Cell Rep. 20, 3071–3084. doi: 10.1016/j.celrep.2017.08.100
You, X., Vlatkovic, I., Babic, A., Will, T., Epstein, I., Tushev, G., et al. (2015). Neural circular RNAs are derived from synaptic genes and regulated by development and plasticity. Nat. Neurosci. 18, 603–610. doi: 10.1038/nn.3975
Zappulo, A., van den Bruck, D., Ciolli Mattioli, C., Franke, V., Imami, K., McShane, E., et al. (2017). RNA localization is a key determinant of neurite-enriched proteome. Nat. Commun. 8:583. doi: 10.1038/s41467-017-00690-6
Zeng, C., and Hamada, M. (2020). RNA-seq analysis reveals localization-associated alternative splicing across 13 cell lines. Genes (Basel) 11:820. doi: 10.3390/genes11070820
Zhang, Z., and Carmichael, G. G. (2001). The fate of dsRNA in the nucleus: a p54(nrb)-containing complex mediates the nuclear retention of promiscuously A-to-I edited RNAs. Cell 106, 465–475. doi: 10.1016/s0092-8674(01)00466-4
Zhang, Y., Chen, K., Sloan, S. A., Bennett, M. L., Scholze, A. R., O’Keeffe, S., et al. (2014). An RNA-sequencing transcriptome and splicing database of glia, neurons and vascular cells of the cerebral cortex. J. Neurosci. 34, 11929–11947. doi: 10.1523/JNEUROSCI.1860-14.2014
Zhang, S., Chen, J., Zhang, J., and Xu, J. (2017). miR-181a involves in the hippocampus-dependent memory formation via targeting PRKAA1. Sci. Rep. 7:8480. doi: 10.1038/s41598-017-09095-3
Zhang, J., Zhang, Y.-Z., Jiang, J., and Duan, C.-G. (2020). The crosstalk between epigenetic mechanisms and alternative rna processing regulation. Front. Genet. 11:998. doi: 10.3389/fgene.2020.00998
Zheng, S., Gray, E. E., Chawla, G., Porse, B. T., O’Dell, T. J., and Black, D. L. (2012). PSD-95 is post-transcriptionally repressed during early neural development by PTBP1 and PTBP2. Nat. Neurosci. 15, 381–388. doi: 10.1038/nn.3026
Zheng, N., Jeyifous, O., Munro, C., Montgomery, J. M., and Green, W. N. (2015). Synaptic activity regulates AMPA receptor trafficking through different recycling pathways. eLife 4:e06878. doi: 10.7554/eLife.06878
Zheng, F., Zhou, X., Luo, Y., Xiao, H., Wayman, G., and Wang, H. (2011). Regulation of brain-derived neurotrophic factor exon IV transcription through calcium responsive elements in cortical neurons. PLos One 6:e28441. doi: 10.1371/journal.pone.0028441
Keywords: RNA localization, alternative splicing, subcellular localization, hippocampus, Cdc42, BDNF, alternative isoform expression, alternate UTR
Citation: Park J and Farris S (2021) Spatiotemporal Regulation of Transcript Isoform Expression in the Hippocampus. Front. Mol. Neurosci. 14:694234. doi: 10.3389/fnmol.2021.694234
Received: 12 April 2021; Accepted: 15 June 2021;
Published: 08 July 2021.
Edited by:
Dezhi Liao, University of Minnesota Twin Cities, United StatesReviewed by:
Sulagna Das, Albert Einstein College of Medicine, United StatesBongmin Bae, University of Nevada, Reno, United States
Copyright © 2021 Park and Farris. This is an open-access article distributed under the terms of the Creative Commons Attribution License (CC BY). The use, distribution or reproduction in other forums is permitted, provided the original author(s) and the copyright owner(s) are credited and that the original publication in this journal is cited, in accordance with accepted academic practice. No use, distribution or reproduction is permitted which does not comply with these terms.
*Correspondence: Shannon Farris, farrissl@vtc.vt.edu