SNARE Regulatory Proteins in Synaptic Vesicle Fusion and Recycling
- 1The Picower Institute for Learning and Memory, Department of Brain and Cognitive Sciences, Massachusetts Institute of Technology, Cambridge, MA, United States
- 2Department of Biology, Massachusetts Institute of Technology, Cambridge, MA, United States
Membrane fusion is a universal feature of eukaryotic protein trafficking and is mediated by the soluble N-ethylmaleimide sensitive factor attachment protein receptor (SNARE) family. SNARE proteins embedded in opposing membranes spontaneously assemble to drive membrane fusion and cargo exchange in vitro. Evolution has generated a diverse complement of SNARE regulatory proteins (SRPs) that ensure membrane fusion occurs at the right time and place in vivo. While a core set of SNAREs and SRPs are common to all eukaryotic cells, a specialized set of SRPs within neurons confer additional regulation to synaptic vesicle (SV) fusion. Neuronal communication is characterized by precise spatial and temporal control of SNARE dynamics within presynaptic subdomains specialized for neurotransmitter release. Action potential-elicited Ca2+ influx at these release sites triggers zippering of SNAREs embedded in the SV and plasma membrane to drive bilayer fusion and release of neurotransmitters that activate downstream targets. Here we discuss current models for how SRPs regulate SNARE dynamics and presynaptic output, emphasizing invertebrate genetic findings that advanced our understanding of SRP regulation of SV cycling.
Introduction
Eukaryotes rely on membrane-bound organelles to organize and transport material between cellular compartments (Wickner and Schekman, 2008). Transport between membrane-bound compartments and secretion of cellular cargo requires fusion of opposing lipid bilayers (Rothman, 1994; Jahn and Südhof, 1999). A large family of membrane associated SNARE proteins constitute the minimal molecular machinery required for membrane fusion by assembling into energetically favorable coiled-coil bundles that pull opposing lipid bilayers together to induce fusion (Söllner et al., 1993; Jahn and Scheller, 2006; Südhof and Rothman, 2009). Most cargo do not require a trigger for release and are trafficked into secretory vesicles destined for immediate fusion with the plasma membrane via the constitutive secretory pathway (Burgess and Kelly, 1987). Many cells including neurons also display a regulated secretion pathway for fast stimulus-dependent cargo release, typically in response to transient rises in intracellular Ca2+. Regulated secretion is mediated by a large cohort of SNARE regulatory proteins (SRPs) that control the timing and localization of SNARE assembly (Südhof and Rothman, 2009). Although some SRPs like N-ethylmaleimide sensitive factor (NSF), the soluble NSF attachment proteins (SNAPs) and Unc18 function in both constitutive and regulated secretion, others like Unc13, Complexin (Cpx), Synaptotagmin 1 (Syt1), Rab3-interacting molecule (RIM), and Tomosyn (Tom) provide unique temporal and spatial control of regulated secretion.
Many SRPs are present in all eukaryotes, suggesting they existed in the last common ancestor (Bennett and Scheller, 1993; Littleton, 2000; Lloyd et al., 2000; Varoqueaux and Fasshauer, 2017; Göhde et al., 2021). Others appeared later in multi-cellular eukaryotes that required more extensive cell-cell communication (Barber et al., 2009; Ryan and Grant, 2009). Gene duplication events occurring in vertebrate lineages generated orthologs of most SRPs in chordates. This redundancy is often absent in non-vertebrate lineages, facilitating genetic analysis of conserved membrane trafficking mechanisms in simpler model eukaryotes like the budding yeast Saccharomyces cerevisiae, the nematode Caenorhabditis elegans and the fruit fly Drosophila melanogaster (Novick et al., 1980; Bargmann, 1993; DiAntonio et al., 1993; Littleton and Bellen, 1995; Sato et al., 2014). Behavioral screens for temperature-sensitive (TS) paralytic mutants in Drosophila identified several conserved SRPs that contribute to SV release (Siddiqi and Benzer, 1976; Chen et al., 1991; van der Bliek and Meyerowitz, 1991; Kawasaki et al., 1998; Littleton et al., 1998, 2001b; Tolar and Pallanck, 1998; Kawasaki and Ordway, 1999; Rao et al., 2001; Babcock et al., 2004; Guan et al., 2005; Iyer et al., 2013). Similarly, screens for C. elegans mutants displaying motor paralysis, uncoordinated locomotion or altered sensitivity to the acetylcholinesterase inhibitor aldicarb have revealed key functions for multiple SRPs (Brenner, 1973; Hosono et al., 1992; Bargmann, 1993; Nguyen et al., 1995; Miller et al., 1996; Richmond et al., 1999; Sieburth et al., 2005). Given the conservation of SRPs across evolution, reverse genetic approaches have also been used to define functions for these proteins in Drosophila and nematodes (Schwarz, 1994; Richmond and Broadie, 2002; Harris and Littleton, 2015). The accessibility of peripheral neuromuscular junctions (NMJs) for electrophysiology and imaging has also facilitated characterization of SNARE and SRP function in SV cycling in Drosophila and C. elegans (Jan and Jan, 1976; Richmond and Jorgensen, 1999; Peled and Isacoff, 2011; Melom et al., 2013).
The SV cycle is initiated following action potential firing and depolarization of presynaptic terminals that cause transient voltage-gated Ca2+ channel opening (Katz, 1969; Sudhof, 2004). Subsequent spikes in local [Ca2+] trigger fusion of SVs that are docked and primed at specialized release sites known as active zones (AZs) (Zhai and Bellen, 2004; Ackermann et al., 2015; Ghelani and Sigrist, 2018). Following Ca2+ influx, SVs fuse at individual AZs in a probabilistic manner that is governed by a range of factors including local Ca2+ channel density and SV distance from the source of Ca2+ influx (Meinrenken et al., 2002; Bucurenciu et al., 2008; Böhme et al., 2016; Akbergenova et al., 2018; Neher and Brose, 2018). Release probability (Pr) for SV fusion can be approximated by measuring AZ Pr, which varies across neuronal subclasses and within the AZ population of a single neuron (Atwood and Karunanithi, 2002; Koester and Johnston, 2005; Peled and Isacoff, 2011; Holderith et al., 2012; Melom et al., 2013; Akbergenova et al., 2018; Neher and Brose, 2018; Karlocai et al., 2021). Most SVs are released via Ca2+-dependent evoked release, although some fuse in a stimulus-independent mode called spontaneous release. After fusion with the presynaptic plasma membrane, several endocytic routes for membrane and protein retrieval recover individual SVs, or larger membrane patches that traffic through endosomal compartments for further sorting (Soykan et al., 2016; Gan and Watanabe, 2018; Chanaday et al., 2019). Reformed SVs acidify through the action of the vesicular H+ pump, load neurotransmitters by vesicular H+ antiporters, and subsequently re-enter the SV pool for additional rounds of release (Sudhof, 2004).
In this review we examine current models for how SRPs guide SNAREs through their assembly/disassembly cycle, focusing on insights from invertebrate genetic studies of SV fusion. We also highlight biochemical approaches that guided reverse genetic experiments and provided context for interpreting genetic studies. The biochemistry and genetics of mammalian SV fusion have been described in prior reviews (Südhof, 2013; Neher and Brose, 2018; Rizo, 2018; Brunger et al., 2019). Key invertebrate and mammalian SRP phenotypes and their predicted molecular function are described in Table 1. This review begins with a description of the mechanism enabling SNAREs to overcome innate repulsion between opposing membranes, and the role of Unc13 and Unc18 in regulating SNARE availability for partial assembly. The SRPs Syt1 and Cpx then arrest SNARE assembly in a partially zippered state and subsequently promote Ca2+-dependent fusion. After fusion, NSF and SNAPs disassemble the SNARE complex to recharge individual SNARE proteins for further cycles of release. Intrinsic SNARE properties protect SNAREs from spontaneous reassembly post-fusion with help from the SRPs Unc18 and Tomosyn. Finally, RIM and Rab3 cooperate with Unc13 to re-position endocytosed SVs for subsequent docking and priming. Each of these steps provide avenues for modulation of SV release that can impact synaptic strength and plasticity.
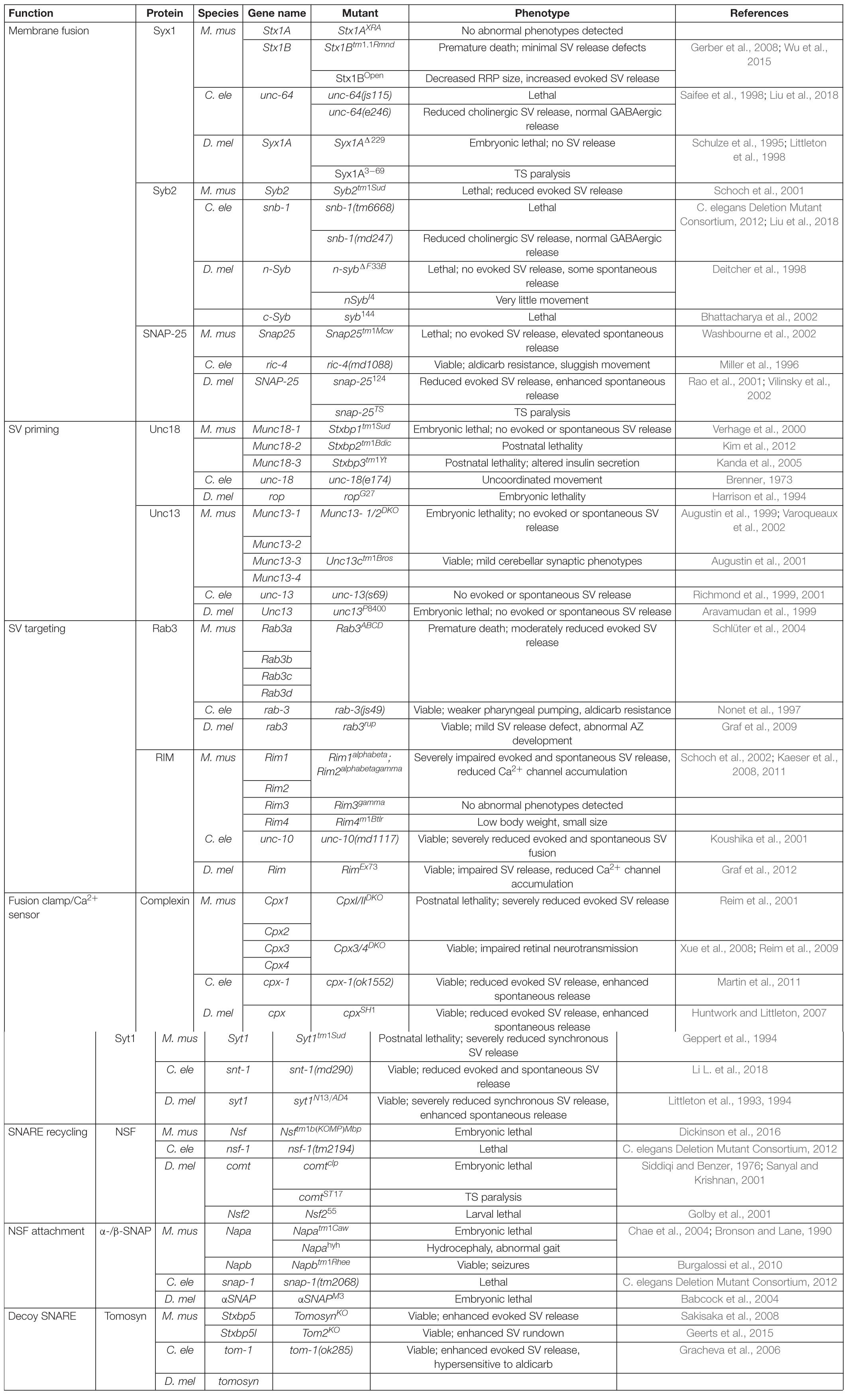
Table 1. Summary of synaptic and behavioral phenotypes in Mus musculus (M. mus), Caenorhabditis elegans (C. ele), and Drosophila melanogaster (D. mel) SNARE and SRP mutants.
SV Fusion Is Mediated by SNARE Complex Assembly
Lipids form stable bilayer membranes that innately repel each other through electrostatic forces and hydration repulsion (Milovanovic and Jahn, 2015; Robertson, 2018). Binding and assembly of SNARE proteins embedded in distinct bilayers is an energetically favored event that provides sufficient input to disrupt and fuse opposing membranes (Weber et al., 1998; McNew et al., 2000b; Tucker et al., 2004). SNAREs are a large protein family characterized by a ∼70 amino acid α-helical heptad repeat known as the SNARE motif. Based on their primary subcellular location, SNAREs are classified as vesicular (v-) or target (t-) membrane SNAREs. A secondary classification scheme defines the proteins as Q- or R-SNAREs depending on whether a glutamine (Q) or arginine (R) is encoded at a highly conserved central hydrophilic layer within the 16-layer SNARE coil. A fusion-competent SNARE complex is formed when three Q-SNARE helices combine with one R-SNARE helix of an opposing membrane (Weimbs et al., 1997; Fasshauer et al., 1998). Across species, the SNARE complex mediating SV fusion is composed of the v-SNARE Synaptobrevin 2 (Syb2, also known as vesicular associated membrane protein (VAMP)) and the t-SNAREs Syntaxin 1 (Syx1) and Synaptosomal associated protein of 25 kilodaltons (SNAP-25) (Söllner et al., 1993). Like all known SNARE complexes, the SV SNARE complex is composed of four α-helices, a Q-helix from Syx1, two Q-helices from SNAP-25 and one R-helix from Syb2. Syb2 and Syx1 are C-terminal anchored transmembrane proteins translated on cytosolic ribosomes and post-translationally inserted into membranes by the transmembrane recognition complex (TRC) (Trimble et al., 1988; Bennett et al., 1992b; Kutay et al., 1993; Borgese et al., 2003). SNAP-25 lacks a transmembrane domain and is post-translationally embedded in membranes via palmitoylation of a cysteine-rich central region (Oyler et al., 1989; Gonzalo and Linder, 1998).
In their native state, SNAREs are disordered filaments that project from their carrier membranes into the cytosol (Fasshauer, 2003). Each protein displays selective binding to a set of cognate SNAREs that zipper together to form a highly structured four-helical SNARE bundle (Sutton et al., 1998; McNew et al., 2000b). Incorporation of individual SNARE filaments into the structured SNARE complex releases free energy that is harnessed to overcome the innate repulsion between opposing lipid membranes. Two competing models for the order of SNARE incorporation into the SNARE complex have been described (Rizo, 2018). One model proposes t-SNARE dimers of Syx1 and SNAP-25 are formed before the v-SNARE Syb2 is engaged. A more recent model argues the SRP Unc18 chaperones assembly of Syx1 and Syb2, ensuring proper alignment prior to SNAP-25 incorporation (Ma et al., 2013; Jiao et al., 2018). These two models converge once cognate SNARE recognition is established to form a partially zippered SNARE configuration known as the trans-SNARE complex, with transmembrane segments residing on separate compartments and full SNARE assembly being temporarily arrested (Figure 1A). SNARE zippering is directional, initiating at the free N-terminal end and progressing through the membrane embedded C-termini (Sutton et al., 1998; Pobbati et al., 2006; Stein et al., 2009; Hernandez et al., 2012). Full zippering through the C-terminus drives fusion by converting the trans-SNARE complex to a cis-complex where all transmembrane segments are embedded in the same bilayer (Figure 1B). The specific arrangement of SNARE complexes between fusing membranes and the number of complexes required for SV fusion remain unclear. However, current models suggest efficient fusion requires several SNARE complexes to be arranged like “spokes on a wheel” around the fusion pore formed between opposing membranes (Hua and Scheller, 2001; Kümmel et al., 2011; Shi et al., 2012).
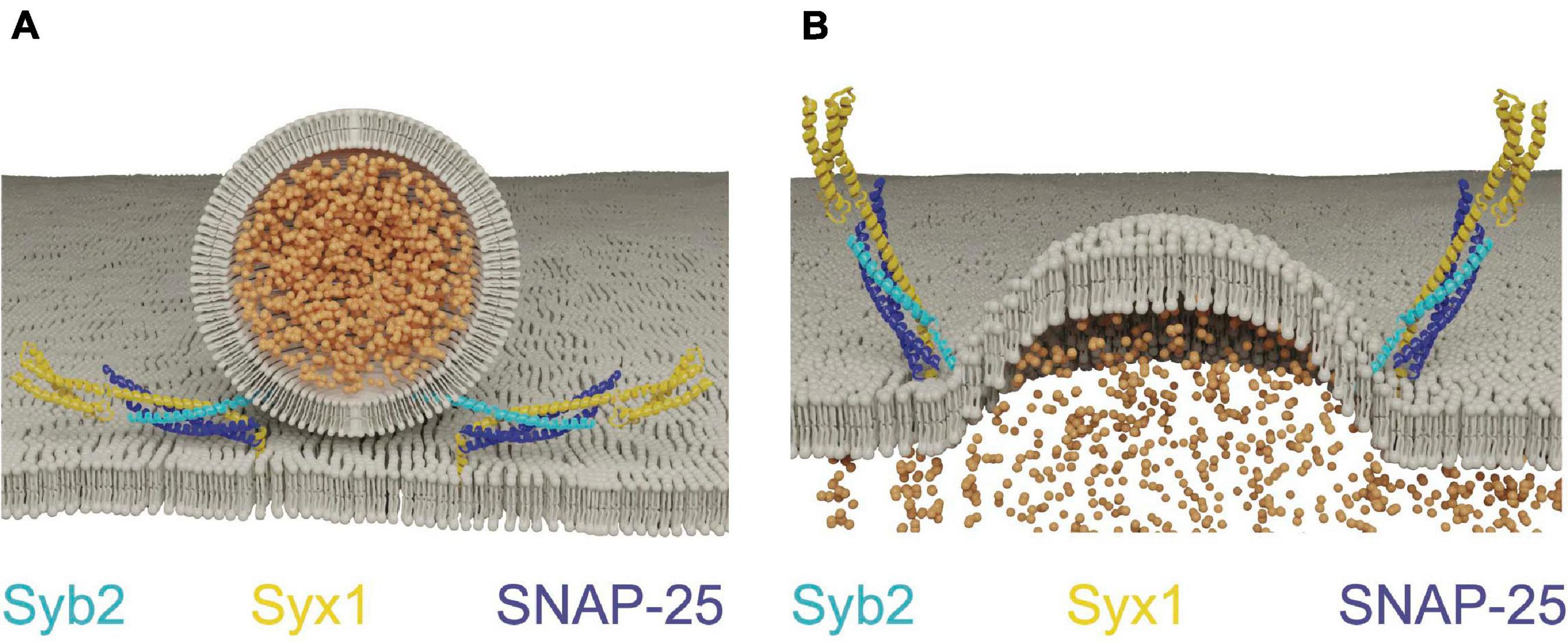
Figure 1. SNAREs assemble between opposing membranes to drive membrane fusion and neurotransmitter release. (A) One helix of the v-SNARE Syb2 assembles with three t-SNARE helices (one from Syx1 and two from SNAP-25) to form a four-helical trans-SNARE complex between opposing membranes. (B) Full SNARE zippering converts the trans-SNARE complex to a cis-SNARE complex to drive membrane fusion and neurotransmitter release. PDB structures in this and subsequent figures were obtained from the cited sources and rendered on membranes to highlight their role in SV fusion. Adapted from Fernandez et al. (1998); Sutton et al. (1998).
Genetic analysis of SNARE mutants in Drosophila and C. elegans support an essential and conserved role for the SNARE complex in mediating SV fusion. In Drosophila, Syx1 is essential for fusion of both SVs and post-Golgi vesicles with the plasma membrane (Broadie et al., 1995; Schulze et al., 1995; Schulze and Bellen, 1996; Burgess et al., 1997). This dual function has made it difficult to define the precise role of Syx1 in SV release, as complete absence of the protein prevents cell viability. Syx1 null mutants develop to the late embryonic stage due to maternal deposition of Syx1 mRNA. Development is arrested once maternal mRNAs are depleted and null embryos are paralyzed due to total absence of evoked and spontaneous SV release (Schulze et al., 1995). Structure-function studies targeting distinct regions of Syx1 and TS paralytic syx1 mutants identified in forward genetic screens are consistent with an essential role for Syx1 in SV fusion (Littleton et al., 1998; Wu et al., 1999; Stewart et al., 2000; Fergestad et al., 2001; Lagow et al., 2007). However, syx1 mutations in distinct regions of the protein differentially alter the amount of spontaneous versus evoked release, indicating Syx1 function can be altered to change either evoked or spontaneous SV fusion pathways. Consistent with an essential role for Syx1 in invertebrate SV fusion, null mutants in C. elegans Syx1 (unc-64) are immobile and lack detectable SV release (Ogawa et al., 1998; Saifee et al., 1998).
Genetic studies of the Drosophila Syb2 and SNAP-25 homologs have revealed phenotypes that are more challenging to interpret due to potential redundancy with other SNARE isoforms. Unlike Syx1, Syb2 function in SV and post-Golgi fusion is segregated between two Drosophila v-SNAREs, with c-Syb mediating post-Golgi fusion and n-Syb controlling SV release (Chin et al., 1993; DiAntonio et al., 1993; Broadie et al., 1995; Deitcher et al., 1998; Yoshihara et al., 1999). Although n-Syb null mutants show severe impairments in evoked release, a low rate of spontaneous fusion is preserved that indicates SV fusion is not eliminated. Consistently, high frequency stimulation elicits a low level of delayed evoked release (Yoshihara et al., 1999) and cleavage of n-Syb by tetanus toxin does not eliminate spontaneous fusion (Sweeney et al., 1995). n-Syb phenotypes can be rescued by overexpressing c-Syb, suggesting both proteins are capable of supporting SV release (Bhattacharya et al., 2002). Given overexpressed c-Syb supports relatively normal SV fusion in the absence of n-Syb, it is unclear why n-Syb mutants show defects primarily in evoked release. Perhaps endogenous neuronal c-Syb expression is too low to support evoked fusion, but high enough to contribute to residual spontaneous release. Alternatively, n-Syb may be specialized for evoked SV release, with spontaneous fusion supported by c-Syb and other Drosophila v-SNAREs (Littleton, 2000). Although no other v-SNARE beyond c-Syb has been shown to function in SV fusion in Drosophila, multiple v-SNAREs support spontaneous and asynchronous SV release at mammalian synapses (Ramirez et al., 2012; Lin et al., 2020). Similar to Drosophila, C. elegans null mutations in the Syb2 homolog (snb-1) are embryonic lethal, but retain uncoordinated movements that indicate a low level of residual SV release (Nonet et al., 1998).
Mutations in Drosophila SNAP-25 indicate redundancy may also compensate for loss of t-SNARE function. The first mutant in Drosophila SNAP-25 was isolated as a TS paralytic allele caused by an amino acid substitution at a highly conserved residue (G50E) in the second SNARE motif of the protein (Rao et al., 2001). Upon exposure to the non-permissive temperature of 37°C, adult animals rapidly paralyze. SNAP-25TS mutant larvae show elevated evoked and spontaneous release at room temperature and impaired release at 37°C. A Syx1 TS mutant (syx13–69; caused by a T254I substitution in the SNARE helix) displays a similar phenotype, indicating multiple t-SNARE mutations can alter SNARE dynamics in a manner that enhances fusion at lower temperatures and blocks release at elevated temperature (Littleton et al., 1998; Lagow et al., 2007; Bykhovskaia et al., 2013). While the mechanism underlying SNAP-25TS release enhancement is unknown, the T254I mutation in Syx1 has been suggested to enhance release by altering interactions between the fusion clamp Cpx and the SNARE complex (Bykhovskaia et al., 2013), as well as promoting C-terminal domain SNARE zippering (Ma et al., 2015). Subsequent studies on SNAP-25 revealed null mutants cause pupal lethality, but do not affect SV release in larvae due to compensation from the t-SNARE homolog SNAP-24 (Vilinsky et al., 2002). Together, these data suggest SNAP-25 normally excludes endogenous SNAP-24 from participating in the SV SNARE complex, though SNAP-24 can support normal SV release when SNAP-25 is absent. C. elegans SNAP-25 null mutants (ric-4) have not been characterized electrophysiologically though they display locomotor defects that suggest RIC-4 is essential for normal synaptic function (Miller et al., 1996). In summary, genetic approaches in Drosophila and C. elegans indicate an essential role for Syx1 in all forms of SV fusion, with spontaneous release persisting in the absence of Syb2 and SNAP-25 likely due to compensation from non-SV SNAREs.
Unc18 and Unc13 Restrict the Localization of SV SNARE Assembly to AZs by Regulating Syx1 Conformational Transitions
Although SNARE proteins are sufficient for membrane fusion in vitro, SRPs are required to regulate SNARE activity in vivo. Given SNARE complex formation is energetically favorable, the assembly process must be tightly controlled so it occurs at the right time and place for productive fusion (Rizo, 2018). The SM proteins (Sec1/Munc18, hereafter referred to as Unc18) and the AZ-localized Unc13 family are SRPs that control the subcellular localization of SNARE assembly. Unc18 is universally required for eukaryotic membrane fusion (Südhof and Rothman, 2009), while Unc13 functions only in regulated secretion (Aravamudan et al., 1999; Richmond et al., 1999). Both proteins act by controlling Syx1 availability and chaperoning SNARE complex assembly (Zhang and Hughson, 2021). Syx1 contains four α-helical domains with only the most C-terminal helix (termed the H3 domain) participating in SNARE complex formation (Fernandez et al., 1998; Sutton et al., 1998; Wu et al., 1999). The remaining N-terminal helices form a three-helix bundle called the Habc domain that folds back onto the H3 SNARE motif to generate a monomeric four stranded coiled-coil bundle. The Habc domain is separated from the H3 segment by a flexible hinge, allowing Syx1 to adopt an open or closed confirmation (Fernandez et al., 1998; Dulubova et al., 1999). In the closed state, the SNARE motif is locked into a grove along the length of the Habc domain and blocked from participating in SNARE complex formation. When converted to the open state, the H3 domain is relieved of Habc inhibition and SNARE complex formation can proceed. Point mutations in the hinge separating the Habc and H3 domains bias Syx1 toward the open conformation (open-Syx1) and enhance SV fusion in C. elegans (Richmond et al., 2001; Gerber et al., 2008). These observations indicate the Syx1 closed conformation is an autoinhibitory feature that must be overcome for SV fusion to proceed, with Unc13 and Unc18 controlling this conformational switch (Figure 2A).
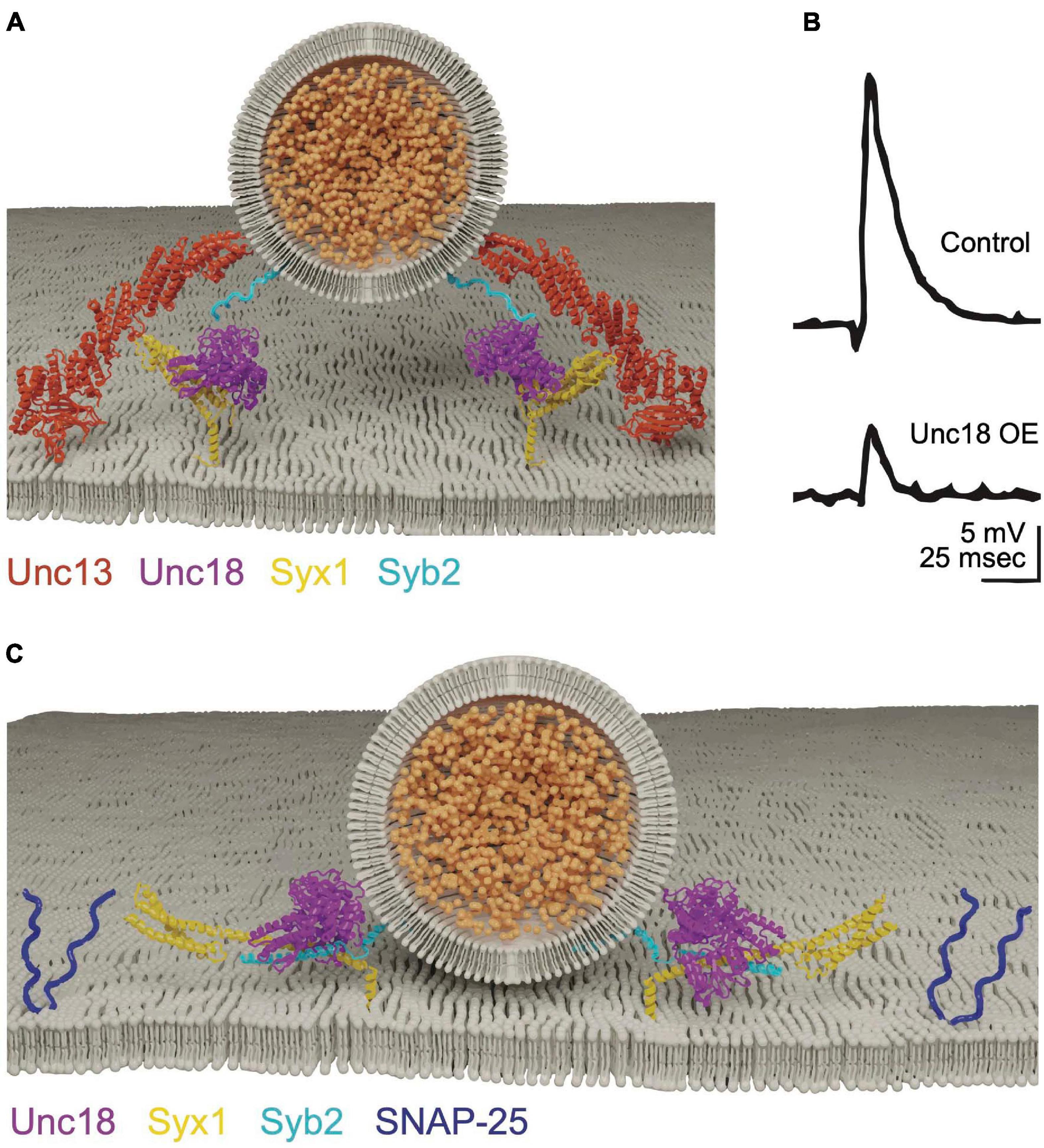
Figure 2. Unc13 and Unc18 chaperone SNARE complex assembly by regulating Syx1. (A) Unc18 holds Syx1 in a closed conformation prior to SNARE complex assembly. Unc13 bridges the SV and plasma membranes and interacts with Syx1 to drive transition to the open-Syx1 state. Adapted from Burkhardt et al. (2008); Liu et al. (2016); Xu et al. (2018). (B) Electrophysiological recordings of evoked responses at the Drosophila larval neuromuscular junction reveal overexpression of Unc18 impairs SV fusion. Adapted from Schulze et al. (1994). (C) Unc18 chaperones the assembly of Syb2 and Syx1 prior to SNAP-25 inclusion into the SNARE complex. Adapted from Burkhardt et al. (2008).
Unc18 proteins are cytosolic and bind to Syx1 in multiple conformational states (Hata et al., 1993; Pevsner et al., 1994; Yang et al., 2000; Dulubova et al., 2007; Khvotchev et al., 2007; Baker et al., 2015). These distinct binding interaction modes suggest Unc18 performs multiple roles in SNARE dynamics. Indeed, in vivo evidence indicates Unc18 both positively and negatively regulates SV release. The Drosophila Unc18 homolog ROP (Ras opposite) is essential for SV fusion, yet strongly inhibits both evoked and spontaneous release when overexpressed (Figure 2B; Harrison et al., 1994; Schulze et al., 1994; Wu et al., 1998). Like Syx1, ROP functions in all modes of cellular secretion and is required for SV and post-Golgi vesicle fusion (Harrison et al., 1994; DeBruhl et al., 2016). Unc18 proteins suppress Syx1 activity in part by holding the t-SNARE in its closed state (Pevsner et al., 1994; Yang et al., 2000). This interaction is required for transport of Syx1 through the secretory pathway, reducing its ability to form ectopic SNARE complexes at inappropriate times or subcellular locations (Rowe et al., 1999, 2001; Medine et al., 2007; McEwen and Kaplan, 2008). Overexpression of Unc18 is predicted to suppress neurotransmitter release by preventing formation of fusogenic SNARE complexes due to excessive inhibition of Syx1. Heterozygotes of Unc18 null mutants also display reduced evoked and spontaneous fusion, indicating SV release is impaired under conditions where Unc18 levels are limiting (Wu et al., 1998). Together, these data indicate SV release is bi-directionally sensitive to Unc18 abundance, suggesting synaptic levels of the protein are finely tuned for optimal presynaptic output.
Unc18 must also play a positive role in release given Unc18 null mutants show severe secretion defects (Harrison et al., 1994; Verhage et al., 2000; Weimer et al., 2003). Multiple positive effects of Unc18 on SV release have been described, including its ability to protect SNARE complexes from disassembly by NSF and α-SNAP. Assembly of the SNARE complex in vitro is blocked when NSF and α-SNAP are added, suggesting SNAREs must be protected from ongoing disassembly (Ma et al., 2013; Prinslow et al., 2019; Stepien et al., 2019). Addition of Unc18 and Unc13 to these in vitro assays restores the ability of SNAREs to trigger fusion, indicating the two proteins act in concert to ensure fusogenic SNARE zippering is not disrupted by premature disassembly. Unc18 also chaperones SNARE assembly by properly aligning individual SNARE helices during zippering of the 4-stranded helical bundle (Ma et al., 2013; Jiao et al., 2018). The SNARE complex is a coiled-coil structure divided into layers of hydrophobicity defined relative to the most central zero layer (Fasshauer et al., 1998). Misalignment of zippering decreases free energy released during SNARE assembly and alters the distance between fusing membranes (Fasshauer et al., 1998; Pobbati et al., 2006). In vitro data suggest Unc18 binds Syx1 and Syb2 in a prefusion intermediate where the two SNAREs are arrested in a partially zippered state and held in proper alignment prior to SNAP-25 arrival (Figure 2C; Jiao et al., 2018; Shu et al., 2020). This role of Unc18 in SNARE assembly is supported by crystal structures of several yeast homologs that hold individual v- and t-SNAREs in proper register (Baker et al., 2015). In summary, Unc18 likely supports SV fusion by templating SNARE complex assembly and inhibiting SNARE disassembly prior to fusion. How Unc18 transitions from inhibiting Syx1 availability by holding the protein in a closed conformation to templating Syx1 and Syb2 assembly is unclear, though Unc13 is hypothesized to regulate this transition in vivo.
Unc13 is one of several multidomain scaffold proteins enriched at presynaptic AZs. Unlike most AZ scaffolds, Unc13 is absolutely essential for both spontaneous and evoked release (Aravamudan et al., 1999; Augustin et al., 1999; Richmond et al., 1999). Unc13 contains C2, MUN and calmodulin binding domains that each have highly conserved binding interactions across evolution (Brose et al., 2000; Böhme et al., 2016). The lipid-binding C2 domains encoded at both termini of Unc13 enable simultaneous interaction with the SV and plasma membrane to facilitate SV capture (Liu et al., 2016). The MUN domain forms a long helical rod that extends from the AZ into the cytosol, similar to other vesicle tethering factors. The MUN domain plays a critical role in SV priming by converting Syx1 from its closed to open state, leading to subsequent v-SNARE engagement and SV docking (Betz et al., 1997; Li et al., 2011; Ma et al., 2011; Wang et al., 2011). Consistent with this model, open-Syx1 mutants rescue release defects in C. elegans Unc13 nulls, indicating Unc13 animals lack SV fusion due to insufficient conversion of Syx1 from its closed to open state (Richmond et al., 2001).
Syt1 and Cpx Regulate SNARE Assembly to Control the Timing of Ca2+-Dependent Fusion
Membrane fusion during constitutive secretion occurs spontaneously, with SNARE complex zippering hypothesized to occur in a single step. In contrast, SNARE assembly during regulated secretion is predicted to arrest in a partially zippered state, allowing membrane fusion to be tightly coupled to Ca2+ influx. Progressive step-wise zippering of the SV SNARE complex is supported by studies of the clostridial neurotoxins tetanus and botulinum that cleave individual SNAREs (Breidenbach and Brunger, 2005). After SV docking and priming, only a subset of toxin serotypes can access SNAREs for cleavage at each conformational state generated by progressive zippering (Hayashi et al., 1994; Bajohrs et al., 2004). Intermediate energy states along the trajectory of SNARE zippering are also observed in vitro using optical tweezers, further suggesting SNAREs assemble and disassemble in a step-wise manner (Gao et al., 2012; Zorman et al., 2014). The synaptic SRPs Cpx and Syt1 provide a neuronal-specific mechanism to further stall SNARE zippering until elevated Ca2+ triggers full SNARE assembly (Figure 3A). Cpx acts during trans-SNARE complex formation to arrest assembly in the partially zippered state (Giraudo et al., 2006; Malsam et al., 2012; Bykhovskaia et al., 2013), with Syt1 triggering full zippering and synchronous evoked fusion in response to Ca2+ (Chapman, 2008; Südhof, 2013; Quiñones-Frías and Littleton, 2021). The mechanisms by which these proteins regulate SNARE assembly and fusion are still being defined, but several models link their biochemical activities with defects in release observed in mutants disrupting their function.
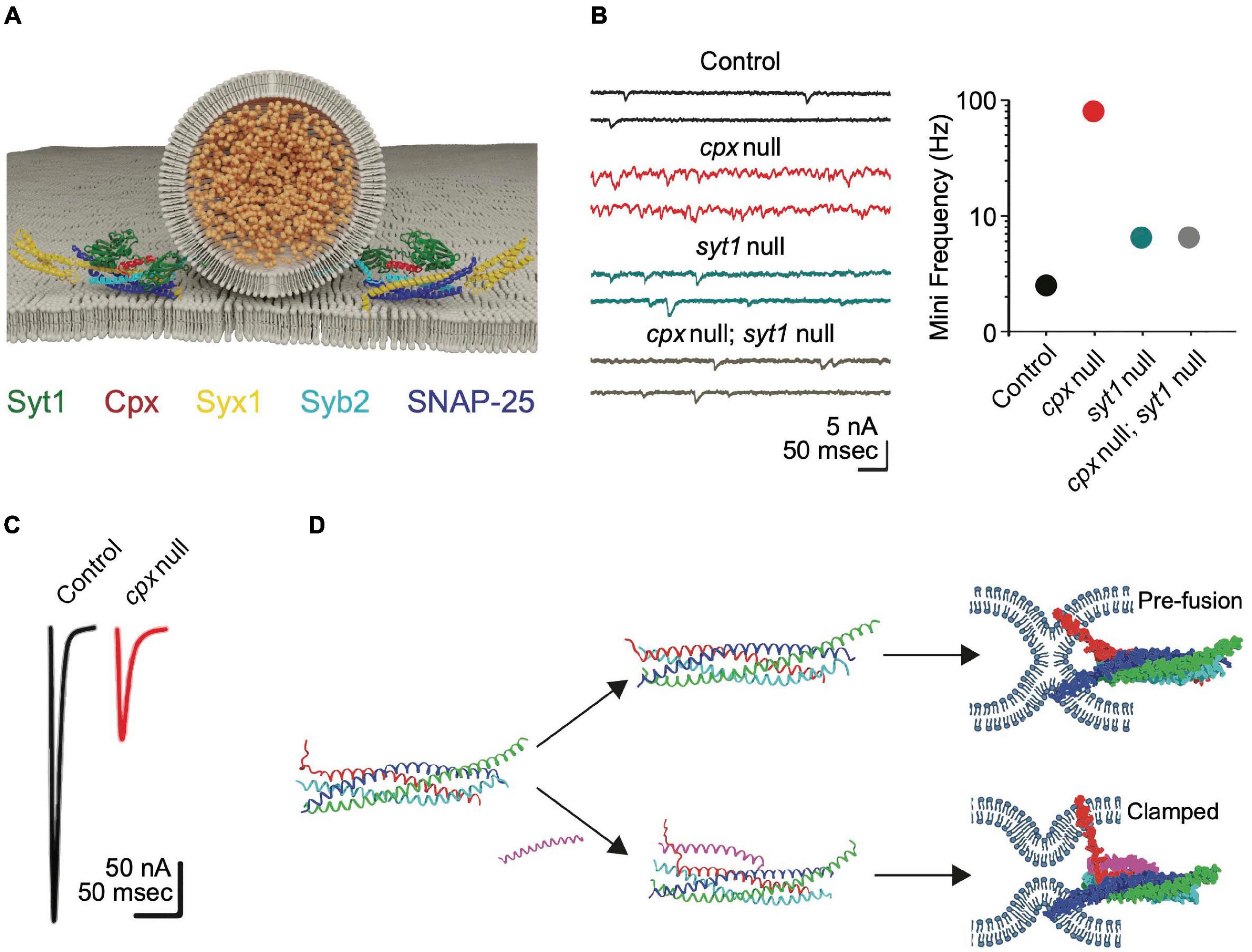
Figure 3. Cpx clamps the SNARE complex in a partially assembled state. (A) Cpx and Syt1 engage the SNARE complex on a shared binding interface to form a tripartite complex. Adapted from Zhou et al. (2017). (B) Electrophysiological recordings at Drosophila larval NMJs reveal cpx null mutants have a dramatically increased rate of spontaneous release. Spontaneous release is restored to near normal levels in syt1; cpx double mutants, indicating Syt1 is required for the elevated mini rate in cpx single mutants. Quantification of spontaneous release rate (mini frequency) is shown on the right for the indicated genotypes. (C) Evoked response amplitude is reduced in cpx null mutants, indicating Cpx is also required for efficient Ca2+-activated release. Panels (B,C) adapted from Jorquera et al. (2012). (D) Molecular-dynamics modeling suggests Cpx may clamp SNAREs in a partially assembled state by altering the confirmation of the C-terminus of Syb2 to prevent full SNARE zippering. Adapted from Bykhovskaia et al. (2013).
Cpx is small cytosolic α-helical protein identified through its binding affinity for the SNARE complex (McMahon et al., 1995). The protein is composed of an N-terminal accessory helix, a central SNARE-binding helix and an unstructured C-terminus that assembles into an amphipathic helix when bound to SV membranes (Pabst et al., 2000; Bowen et al., 2005; Xue et al., 2007; Cho et al., 2010; Kaeser-Woo et al., 2012; Buhl et al., 2013; Snead et al., 2014). Mouse Cpx mutants have decreased evoked release, suggesting the protein facilitates SV fusion (Reim et al., 2001). Subsequent in vitro assays indicated Cpx primarily functions to inhibit SNARE assembly and fusion (Giraudo et al., 2006; Malsam et al., 2020). In contrast to four Cpx genes in mammals, Drosophila contain a single Cpx that facilitates genetic analysis. Null mutants in Drosophila Cpx revealed both positive and negative functions in SV release, including a ∼100-fold increase in spontaneous fusion (Figure 3B) and a ∼50% decrease in evoked release (Figure 3C; Huntwork and Littleton, 2007). C. elegans Cpx null mutants display similar defects, indicating enhanced spontaneous fusion and decreased evoked release are conserved invertebrate phenotypes associated with loss of Cpx (Xue et al., 2009; Cho et al., 2010; Martin et al., 2011; Jorquera et al., 2012; Buhl et al., 2013; Iyer et al., 2013; Sabeva et al., 2017; Wragg et al., 2017). Cpx mutants also disrupt the speed of evoked release, with less synchronous fusion and increased release through the slower asynchronous pathway (Jorquera et al., 2012). In addition, Cpx participates in tethering SVs to release sites by interacting with the core AZ scaffolding protein Bruchpilot (BRP) (Scholz et al., 2019). Together, these observations indicate Cpx helps target SVs to release sites, facilitates the amount and speed of evoked release, and clamps SVs in a partially zippered state that limits spontaneous fusion.
In contrast to the dramatic increase in spontaneous fusion in invertebrate Cpx mutants, mouse Cpx knockouts do not display elevated spontaneous release (Xue et al., 2008, 2007; Yang et al., 2013; Chang et al., 2015; López-Murcia et al., 2019). However, mammalian Cpx is sufficient to clamp spontaneous release in both C. elegans and Drosophila Cpx mutants (Cho et al., 2010; Wragg et al., 2017), suggesting clamping properties are intrinsic to Cpx across phyla. Cpx3 is the most effective mammalian isoform for clamping SV fusion in Drosophila and C. elegans Cpx mutants. The primary difference between Cpx3 and other mammalian isoforms occurs in the C-terminus, suggesting this region harbors critical determinants for clamping fusion. Although it is unclear why mammalian synapses are more resistant to enhanced spontaneous release in Cpx mutants, Cpx can clamp SV fusion during the asynchronous phase of evoked release in mammals (Yang et al., 2010; Chang et al., 2015). This slower component of release occurs when Ca2+ levels are falling from their peak concentration that drives synchronous SV fusion. Therefore, higher baseline Ca2+ levels in invertebrate presynaptic terminals could account for the differences in Cpx clamping. Consistent with this hypothesis, presynaptic [Ca2+] can be reduced by long-term exposure to BAPTA and causes a ∼50% decrease in spontaneous release in Drosophila Cpx mutants (Jorquera et al., 2012). These data suggest Cpx clamping acts optimally at a slightly higher baseline [Ca2+], implying it may act in part by regulating the Ca2+ sensitivity of SV release.
Current data indicate the activating and inhibitory functions of Cpx can be genetically separated, though both require SNARE complex binding (Xue et al., 2007, 2010; Cho et al., 2010, 2014; Krishnakumar et al., 2011; Iyer et al., 2013). Several models for the inhibitory function of Cpx have been proposed. A “zig-zag” model based on structural evidence suggests the central helix of Cpx tucks into a partially zippered SNARE complex, while the accessory helix projects out at a 45-degree angle to bind a neighboring partial SNARE assembly (Kümmel et al., 2011). This mode would allow Cpx to bridge partial SNARE assemblies in an alternating zigzag chain sandwiched between docked SVs and the plasma membrane to clamp release before Ca2+ influx. Mutations predicted to abolish the zig-zag array have only mild effects on SV release in Drosophila, suggesting this binding mode is unlikely to represent the primary clamping configuration of Cpx (Cho et al., 2014). A second model from biochemical studies and molecular modeling suggests competition between Syb2 and Cpx for t-SNARE binding mediates clamping, with the Cpx N-terminal accessory helix binding partially assembled SNARE complexes in a grove between Syx1 and SNAP-25 in place of Syb2 (Figure 3D). This would allow Cpx to destabilize the final step of SNARE zippering by excluding Syb2 from the C-terminus of the SNARE complex (Bykhovskaia et al., 2013; Vasin et al., 2016; Brady et al., 2021). Although attractive, genetic analysis of Cpx and n-Syb mutations predicted to disrupt this mode of binding only partially disrupt clamping (Vasin et al., 2016). A modified version of the competition model has also been described where Syb2, a single helix of SNAP-25, and the Cpx accessory helix form a C-terminal helical bundle that displaces Syx1 from the SNARE complex at its C-terminus (Malsam et al., 2020). Mutations disrupting this binding mode do not affect evoked release but decrease the clamping efficiency for spontaneous fusion.
Regardless of its clamping configuration, enhanced spontaneous release in Cpx mutants is abolished in Drosophila Cpx, Syt1 double mutants (Figure 3B; Jorquera et al., 2012). Syt1 is a SV protein with tandem C2 domains (C2A and C2B, Figure 4A) that bind ∼ five Ca2+ ions via negatively charged aspartate residues encoded within protruding C2 loops (Ubach et al., 1998; Chapman, 2008). Ca2+ binding neutralizes the negative charge of these loops to allow C2•Ca2+ to partially insert into the plasma membrane (Davletov and Südhof, 1993; Chapman and Davis, 1998; Fernandez et al., 2001; Ubach et al., 2001). Ca2+ binding to the C2B domain of Drosophila Syt1 is critical for promoting evoked release (Figure 4B), with C2A-Ca2+ playing a supporting role (Littleton et al., 2001a; Mackler et al., 2002; Paddock et al., 2008, 2011; Yoshihara et al., 2010; Striegel et al., 2012; Lee et al., 2013; Bowers and Reist, 2020). The genetic interactions between Syt1 and Cpx suggest loss of Cpx may disrupt Syt1’s ability to link its fusion activation to Ca2+ binding. Following loss of Cpx, Syt1 may constitutively activate SNARE-dependent fusion in a Ca2+-independent manner, leading to elevated spontaneous fusion rates.
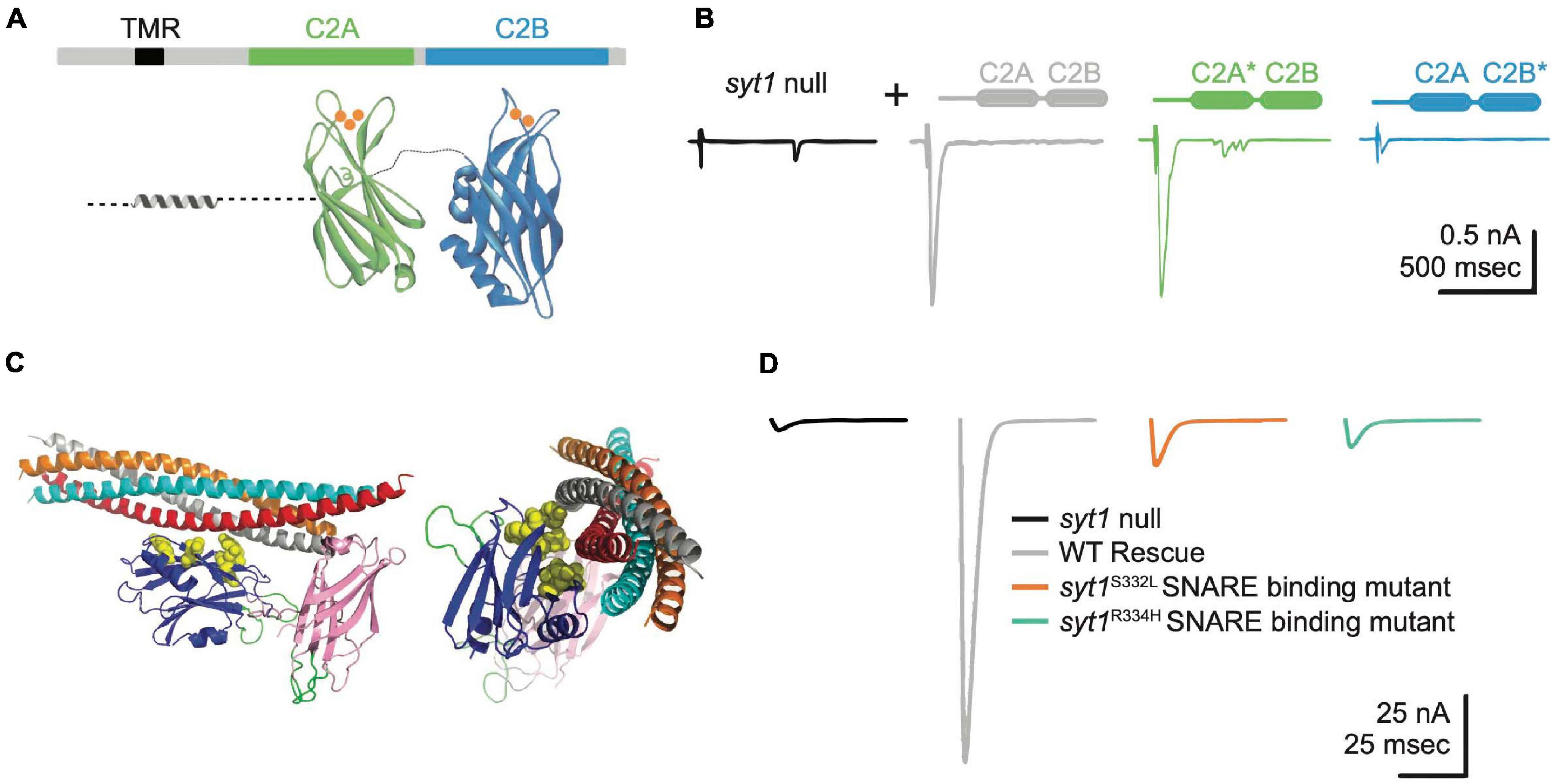
Figure 4. Syt1 binds Ca2+ and SNARE complexes to trigger synchronous SV fusion. (A) Syt1 is tethered to SV membranes via a transmembrane region (TMR) and has two Ca2+-binding C2 domains projecting into the cytosol. Ca2+ (orange) binds to polybasic loops projecting from each C2 domain, with C2A accommodating 3 Ca2+ ions and C2B binding 2 Ca2+ ions. (B) Two-electrode voltage clamp recordings demonstrate syt1 nulls have dramatically impaired evoked synchronous release at Drosophila larval NMJs. Unlike rescue with wildtype (WT) Syt1 (gray trace), transgenes with impaired C2B Ca2+ binding (C2B*, blue) severely impair evoked release while C2A Ca2+ binding mutants (C2A*, green) fail to prevent the enhanced asynchronous release observed in nulls (black). Adapted from Yoshihara et al. (2010). (C) The Syt1 C2B domain (blue) also interacts with the SNARE complex at a primary interface independent of the Cpx-associated tripartite binding site. Critical residues coordinating binding of the Syt1 C2B domain to the SNARE complex are highlighted in yellow and were identified from crystal structures of the complex and genetic screens in Drosophila. (D) Disrupting Syt1-SNARE binding at the primary interface with two independent alleles mimics the syt1 null phenotype. Panels (C,D) adapted from Guan et al. (2017).
Regulation of Syt1 activity is also likely to contribute to Cpx’s positive role in promoting fusion as mutations in either gene cause similar SV release defects, though Cpx phenotypes are generally milder than those found in Syt1 (Jorquera et al., 2012). Both Cpx and Syt1 mutants show impaired evoked synchronous release while enhancing the number of SVs released through the slower asynchronous pathway (Mackler et al., 2002; Yoshihara and Littleton, 2002; Saraswati et al., 2007; Yoshihara et al., 2010; Paddock et al., 2011; Jorquera et al., 2012; Striegel et al., 2012; Lee and Littleton, 2015; Guan et al., 2020; Shields et al., 2020). Both mutants show an increased rate of spontaneous fusion (Littleton et al., 1993, 1994; DiAntonio and Schwarz, 1994; Huntwork and Littleton, 2007; Lee et al., 2013), with Cpx playing the primary role in clamping release at invertebrate terminals and Syt1 assuming this function at mammalian synapses. The Ca2+ sensitivity of evoked release is also reduced in either mutant (Littleton et al., 1994; Jorquera et al., 2012), suggesting a greater number of Ca2+ ions are required to fuse SVs in their absence. Evoked release defects in Cpx mutants can be partially rescued with elevated extracellular [Ca2+], arguing Ca2+ sensitivity is impaired but not abolished (Jorquera et al., 2012). In contrast, Syt1 mutants display severely impaired release across the entire [Ca2+] range (Littleton et al., 1994; Yoshihara and Littleton, 2002). Finally, both mutants reduce the size of the readily releasable SV pool and alter the speed of SV fusion in a Ca2+-dependent manner (Yoshihara and Littleton, 2002; Mace et al., 2009; Jorquera et al., 2012; Lee and Littleton, 2015). Recent structural evidence provides a potential model explaining why these SRPs phenocopy each other. Syt1 and Cpx bind the outer surface of the SNARE complex to form a split but continuous α-helix as part of a “tripartite complex” (Figure 3A), suggesting Syt1 and Cpx may form a single regulatory unit that reduces the energy barrier needed for full SNARE zippering (Trimbuch and Rosenmund, 2016; Zhou et al., 2017).
Beyond the tripartite SNARE binding site with Cpx, a primary SNARE complex binding interface on a distinct surface of the Syt1 C2B domain is critical for triggering SV release (Zhou et al., 2015b; Guan et al., 2017). The five key residues that form the primary binding site based on structural data were independently identified in a genetic screen for Syt1 mutants in Drosophila (Figure 4C), indicating this interface is highly conserved and essential for Syt1 function (Guan et al., 2017). Disrupting SNARE binding at this site phenocopies Syt1 null mutants in critical ways (Figure 4D), including loss of synchronous fusion and elevated rates of asynchronous and spontaneous release. Current models for Syt1’s role in fusion suggest SNARE binding, together with Ca2+-independent lipid interactions mediated through a polybasic stretch on a separate C2B surface, sandwich Syt1 between the plasma membrane and the partially assembled SNARE complex. This positions the Ca2+ binding loops of Syt1’s C2 domains close to the plasma membrane, enabling rapid membrane insertion of the loops following Ca2+ entry to trigger a conformational rotation that pulls the two fusing membranes together and initiates full SNARE zippering (Quiñones-Frías and Littleton, 2021). Ca2+-dependent conformational changes in Syt1 may also dislodge Cpx to facilitate conversion from the trans- to cis-SNARE complex to drive the final fusion reaction. Displacement of Cpx would provide binding sites for α-SNAP to initiate subsequent NSF-mediated SNARE complex disassembly. Together, these data indicate Syt1 and Cpx cooperate to prevent full SNARE zippering during SV priming and later activate full fusion following Ca2+ influx.
The AAA+ ATPase NSF Disassembles SNARE Complexes to Support Continual SV Cycling
NSF proteins are highly conserved AAA+ ATPases that disassemble SNARE complexes for both constitutive and regulated membrane trafficking (Wilson et al., 1989; Pallanck et al., 1995a). Disassembly of the SNARE complex supplies the energy input required for membrane fusion by returning SNAREs to their disordered state for future rounds of assembly (Block et al., 1988; Wilson et al., 1992; Littleton et al., 1998). The cis-SNARE complex (also called the 7S complex based on its gradient sedimentation) is highly stable and resistant to SDS denaturation, indicating a large input of cellular energy is required to break the complex apart (Fasshauer et al., 2002). Full dissociation of the SNARE complex is estimated to consume between 12 and 50 ATP molecules, generating 65 kBT of free energy that can be used to drive membrane fusion during future cycles of SNARE assembly (Cipriano et al., 2013; Yoon and Munson, 2018). NSF proteins are composed of an N-terminal domain and two AAA+ ATPase domains termed D1 and D2 (Tagaya et al., 1993; White et al., 2018). The D2 domains promote multimerization of NSF into hexamers that assemble around a single SNARE complex via interactions between the NSF N-terminal domains and SNAP proteins that preferentially associate with assembled SNARE complexes (Zhao et al., 2015; White et al., 2018). Once the SNAP/NSF/SNARE complex is formed (termed the 20S complex, Figure 5A), the D1 domains of NSF use ATP hydrolysis to twist the four-helical SNARE bundle opposite to its assembled orientation until individual SNAREs are sufficiently destabilized to disassociate (Cipriano et al., 2013; Ryu et al., 2015; Zhao et al., 2015). Whether SNARE disassembly by NSF occurs in a multi-step process or all at once remains contentious. One model based on the processive mechanism of the bacterial AAA+ ATPase ClpXP suggests NSF may drive SNARE disassembly by progressing along the assembled SNARE complex in discrete steps that each require ATP hydrolysis (Saunders et al., 2020). An alternate model supported by in vitro single molecule assays suggests NSF may use a spring-loaded trigger mechanism to disassemble the SNARE complex in a single round of ATP hydrolysis (Ryu et al., 2015).
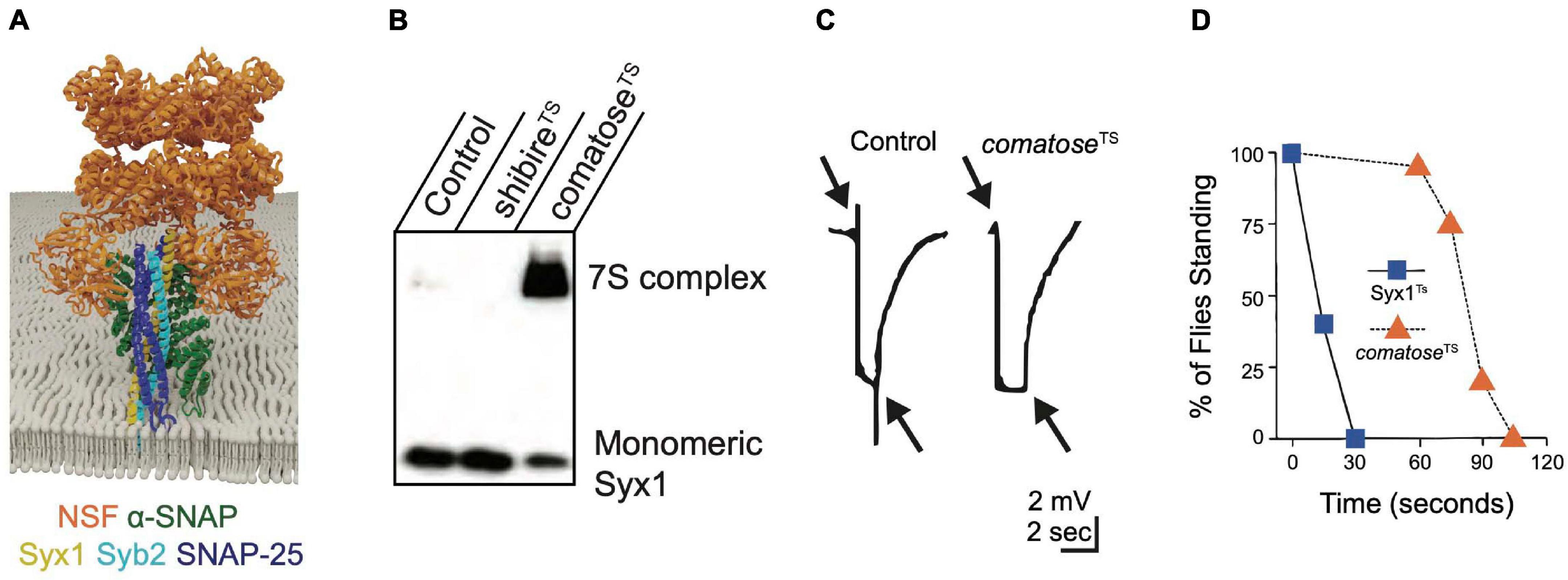
Figure 5. NSF and α-SNAP disassemble the cis-SNARE complex to maintain a pool of available free SNAREs for sustained release. (A) α-SNAP binds assembled SNARE complexes, enabling NSF to form a hexamer around the SNARE complex to initiate disassembly. Adapted from Zhao et al. (2015). (B) Western blot of Drosophila brain extracts with anti-Syx1 antisera demonstrates 7S complexes accumulate at the restrictive temperature in comatoseTS mutants at the expense of monomeric Syx1. Heat-shocked shibireTS mutants have reduced 7S complex compared to controls, indicating NSF continues to disassemble SNARE complexes within the plasma membrane when SVs are depleted. (C) Electroretinograms recorded from Drosophila TS comatose mutants reveal a loss of on and off transients (arrows) at elevated temperatures that occurs secondary to disrupted synaptic transmission from photoreceptors. Panels (B,C) adapted from Littleton et al. (2001b). (D) Drosophila TS mutants in Syx1 show rapid behavioral paralysis compared to the slower time-course for comatose TS mutations in NSF. Adapted from Littleton et al. (1998).
The Drosophila genome encodes two NSF proteins. NSF1 (comatose) mediates neuronal SNARE complex disassembly (Ordway et al., 1994; Boulianne and Trimble, 1995; Pallanck et al., 1995b) and NSF2 functions more broadly, including in the post-synaptic compartment (Golby et al., 2001; Stewart et al., 2002). TS behavioral screens in Drosophila uncovered numerous mutants in NSF1 that were originally named after their strong paralytic phenotype (comatose) (Siddiqi and Benzer, 1976; Pallanck et al., 1995a). Many comatose TS alleles result from single amino acid changes within a hinge region of the D1 domain that may impair the ability of NSF to twist the SNARE complex apart (Littleton et al., 2001b). Although restrictive temperatures are predicted to immediately disrupt NSF function, adult comatose animals behave normally for ∼ one minute before paralysis (Figure 4B). Accumulation of assembled 7S SNARE complexes (Figure 5B) and a progressive impairment of synaptic transmission within the visual system (Figure 5C) mirror the time course for paralysis (Littleton et al., 1998, 2001b; Tolar and Pallanck, 1998; Sanyal et al., 1999, 2001). These data suggest depletion of free SNAREs available to engage in SNARE complex assembly occurs after several rounds of SV fusion following loss of NSF1 function, leading to disrupted synaptic transmission and subsequent behavioral paralysis. The time course for recovery of comatose mutants correlates with the duration of the prior heat shock, likely due to the slow kinetics of NSF-mediated SNARE disassembly and the increasing depletion of free SNAREs as animals are maintained for longer periods at restrictive temperatures. The delayed onset and slow recovery from behavioral paralysis in NSF TS mutants contrasts with the rapid onset and recovery observed in Syx1 TS mutants (Syx13–69, Figure 5D). These kinetic differences highlight the requirement of Syx1 for immediate SV fusion and the large pre-existing pools of free SNAREs and SVs available to support ongoing release for several minutes after NSF inactivation (Littleton et al., 1998).
Although comatose mutations cause adult paralysis and synaptic transmission defects, the role of NSF at larval NMJs has been difficult to ascertain. NSF1 null mutants are lethal, but die over a developmental window that spans from late embryogenesis to the pharate adult stage (Golby et al., 2001; Littleton et al., 2001b; Sanyal and Krishnan, 2001). NSF1 null larvae display no obvious transmission defects at the NMJ even though lethality is rescued when NSF1 is re-expressed in the nervous system. NSF2 null mutants die during early larval development and are rescued by re-expression of NSF2 in mesodermal tissues, suggesting this isoform is predominantly active within muscles and other non-neuronal cells (Golby et al., 2001). NSF2 may also have SNARE-independent presynaptic functions given NSF2 mutants have defects in SV mobility due to decreased presynaptic actin filament assembly (Nunes et al., 2006). Overexpression of NSF2 in the nervous system is sufficient to rescue release defects in NSF1 mutants, suggesting differences in expression pattern and abundance are likely to account for their unique phenotypes. At the adult NMJ, comatose mutants display normal baseline synaptic transmission and a progressive activity-dependent reduction in evoked release during repetitive stimulation (Kawasaki et al., 1998; Kawasaki and Ordway, 1999). Together with an accumulation of docked SVs observed by EM at restrictive temperatures, these data suggest disassembly of SNARE complexes by NSF helps maintain SVs in a readily releasable state. Excess SNARE complexes in comatose mutants accumulate in both the presynaptic plasma membrane and on SVs (Tolar and Pallanck, 1998; Sanyal et al., 1999, 2001; Littleton et al., 2001b). Together, these data indicate NSF can break apart SNARE complexes in the plasma membrane that accumulate following fusion, as well as those already on SVs, to maintain a pool of free Syb2 to participate in trans-SNARE complex formation.
NSF relies on SNAP proteins to bind assembled SNARE complexes (Clary et al., 1990; Vivona et al., 2013). α-, β-, and γ-SNAP were initially purified from brain extracts based on their ability to promote NSF binding to SNARE-rich membranes. α-SNAP is the most extensively characterized and mediates association of NSF with the 7S SNARE complex in vitro and in vivo (Söllner et al., 1993; Barnard et al., 1997; Vivona et al., 2013; Zhao et al., 2015). The α-SNAP/SNARE complex binding interface includes residues from all SNAREs, suggesting it only recognizes assembled SNARE complexes (Whiteheart et al., 1992; Wilson et al., 1992; Marz et al., 2003). NSF hexamers bind SNAREs via four α-SNAP proteins that form a bridge between the SNARE complex and the NSF N-terminal domains (Zhao et al., 2015; Zhou et al., 2015a; Zhao and Brunger, 2016; White et al., 2018; Huang et al., 2019). In vitro evidence suggests NSF and α-SNAP indiscriminately disassemble SNARE complexes regardless of individual SNARE composition, suggesting they provide the energy for most cellular fusion reactions (Vivona et al., 2013; Zhao et al., 2015; Zhao and Brunger, 2016). Although the in vitro function of α-SNAP in SNARE disassembly has been well characterized, in vivo roles have not been extensively studied. In C. elegans, synaptic phenotypes resulting from NSF and α-SNAP mutations have not been reported, but Drosophila α-SNAP null mutants are embryonic lethal (Ordway et al., 1994; Babcock et al., 2004). Similar to Syx1 and Unc18, Drosophila α-SNAP mutants show defects in membrane trafficking in many cell types that indicate α-SNAP function is broadly required (Babcock et al., 2004). Like comatose mutants, α-SNAP hypomorphic alleles accumulate assembled 7S SNARE complexes but show no defects in synaptic transmission at larval NMJs (Babcock et al., 2004).
The functions of β- and γ-SNAP are less clear. α-SNAP is the only SNAP that restores in vitro fusion deficits in yeast sec17 (α-SNAP) mutants, suggesting SNAPs are not fully redundant (Clary et al., 1990; Griff et al., 1992; Peter et al., 1998). β-SNAP is a vertebrate-specific paralog of α-SNAP generated by a gene duplication event occurring after divergence of vertebrates and invertebrates. γ-SNAP is dissimilar in sequence to both α- and β- SNAP and may play a supportive role in NSF/SNARE complex dynamics (Whiteheart et al., 1992; Peter et al., 1998; Stenbeck, 1998). Many invertebrates lack a γ-SNAP homolog, though Drosophila has two γ-SNAP genes that have not been characterized. Although no γ-SNAP mutant has been reported in any species, RNAi knockdown of γ-SNAP indicates the protein mediates disassembly of an endosomal SNARE complex, suggesting a canonical SNAP function (Inoue et al., 2015). Although the requirement of NSF and SNAPs for SNARE complex disassembly has been well defined, the full complement of in vivo functions mediated by these proteins remains poorly characterized.
Multiple Modes of Endocytosis Mediate SV and SNARE Recycling
The SV and presynaptic plasma membranes become continuous during fusion, resulting in a temporary disruption in the spatial segregation of proteins. Many neurons can continue to release SVs for minutes to hours under high exocytotic demand, releasing far more SVs than observed in synaptic terminals by EM (Ceccarelli et al., 1973). To support further rounds of release, membrane proteins must be re-segregated and SV material selectively internalized to form new vesicles (Dittman and Ryan, 2009; Gan and Watanabe, 2018; Chanaday et al., 2019). SNARE disassembly by NSF is also required to free v-SNAREs from plasma membrane t-SNAREs after fusion. SNARE disassembly by NSF is hypothesized to occur in part at peri-active zones (PAZ), a presynaptic endocytotic domain surrounding AZs where SV material is retrieved from the plasma membrane (Estes et al., 1996; Littleton et al., 2001b; Rodal et al., 2008; Yu et al., 2011; Harris and Littleton, 2015; Maritzen and Haucke, 2018; Guan et al., 2020). Live imaging of NSF and α-SNAP show they redistribute from the cytoplasm to the peri-active zone (PAZ) to bind post-fusion SNARE complexes in Drosophila comatose mutants (Yu et al., 2011). Although endocytosis and SNARE disassembly can act within the same membrane compartment, how NSF activity is spatially and temporally coordinated with endocytosis is unknown. Three popular models have been proposed for SV endocytosis, including “kiss-and-run” endocytosis, ultrafast endocytosis and Clathrin-mediated endocytosis (CME) (Ceccarelli et al., 1973; Heuser and Reese, 1973; Fesce et al., 1994; Watanabe et al., 2013a,b).
Kiss-and-run is conceptually the simplest way to recover SV membrane proteins, with SNARE zippering causing brief fusion pore formation that releases neurotransmitters before the pore is quickly re-closed (Cremona and De Camilli, 1997; Rizzoli and Jahn, 2007; Chanaday et al., 2019). As such, SV material is never lost to the plasma membrane and SVs are immediately recovered without losing their identity. It is unclear how NSF-mediated disassembly works in this pathway given cis-SNARE complexes would not form in a fused membrane. Kiss-and-run has been documented for dense core vesicle (DCV) cargo release and for some SVs at a few central mammalian synapses (Artalejo et al., 1998; Aravanis et al., 2003; Xia et al., 2009; Zhang et al., 2009; Wen et al., 2017). However, experimental evidence does not support this mechanism at invertebrate synapses given kiss-and-run is a Clathrin-independent process (Henkel and Almers, 1996; Artalejo et al., 1998). Acute inactivation of Clathrin at Drosophila NMJs abolishes sustained release during repetitive stimulation and is accompanied by complete loss of SVs (Heerssen et al., 2008; Rodal and Littleton, 2008).
In contrast to kiss-and-run, CME enables SVs to be directly recovered from the plasma membrane after full collapse through progressive membrane invagination into reformed vesicles. Clathrin is a cytosolic protein that forms trimeric Y-shaped triskelions that progressively deform the plasma membrane by assembling on endocytic membrane patches to generate coated pits (Ungewickell and Branton, 1981; Smith et al., 1998). Further assembly shapes these pits into Clathrin-caged spheres that are budded from the plasma membrane (Heuser and Reese, 1973; Takei et al., 1996). GTP hydrolysis by the endocytic protein Dynamin provides energy to release nascent SVs from the plasma membrane by oligomerizing around the invaginating membrane stalk and pinching it to induce membrane fission (Hinshaw and Schmid, 1995; Sweitzer and Hinshaw, 1998; Ford et al., 2011). Hypomorphic alleles of the Drosophila Dynamin homolog Shibire were isolated in TS paralytic screens (Poodry et al., 1973; Koenig and Ikeda, 1989; Chen et al., 1991; van der Bliek and Meyerowitz, 1991; van de Goor et al., 1995; Delgado et al., 2000). Shibire mutants show fast synaptic depression and SV depletion at elevated temperatures, suggesting Dynamin is required to recover SVs for sustained release (Koenig and Ikeda, 1989; Delgado et al., 2000; Kawasaki et al., 2000; Wu et al., 2005).
Clathrin assembly is triggered by cytosolic adaptor proteins that recognize and cluster SV material into endocytic membrane patches (Haucke et al., 2011). Each SV protein is presumed to directly or indirectly associate with the general endocytic adaptor protein complex 2 (AP2) for retrieval from the membrane, with interactions between SV proteins likely contributing to AP2 recognition (Bennett et al., 1992a; Wittig et al., 2021). Syt1 is captured by the AP2 adaptor complex and Stonin2, while Syb2 is internalized by indirect AP2 association through the Clathrin adaptor AP180 (Zhang et al., 1994, 1998; Chapman et al., 1998; Nonet et al., 1999; Haucke et al., 2000; Littleton et al., 2001a; Martina et al., 2001; Walther et al., 2004; Bao et al., 2005; Diril et al., 2006; Kaempf et al., 2015). Mutations in the core α-adaptin subunit of the Drosophila AP2 complex cause embryonic lethality, with disrupted endocytosis and loss of SVs (González-Gaitán and Jäckle, 1997). Mutations in AP2 proteins in C. elegans also disrupt synaptic transmission and reduce SV numbers by up to 70%, with accumulation of large membrane vacuoles within synaptic terminals (Gu et al., 2008, 2013; Mullen et al., 2012). Loss of the Drosophila AP180 protein LAP causes a reduction in SV number, accumulation of cytosolic cisternae and increased SV size (Zhang et al., 1998), similar to mutants of the C. elegans AP180 homolog Unc11 that display an accumulation of Snb-1 (Syb2 homolog) on the plasma membrane, impaired neurotransmitter release and enlarged SVs (Nonet et al., 1999). NSF-mediated disassembly of the SNARE complex would expose Syb2 to AP180, providing one potential switch coupling SNARE disassembly to SV endocytosis. Neurotransmission is not fully eliminated in AP180 mutants, suggesting Syb2 can be retrieved from the plasma membrane via another mechanism. The abundant SV protein Synaptophysin simultaneously binds Syb2 and AP2 to provide a secondary pathway for SV internalization (Pennuto et al., 2003; Yelamanchili et al., 2005; Bonanomi et al., 2007; Gordon and Cousin, 2014, 2016; Gordon et al., 2016, 2011). Syx1 cannot bind Syb2 while associated with Synaptophysin, suggesting Syb2 would only be available for endocytosis recruitment after SNARE complex disassembly (Edelmann et al., 1995; Siddiqui et al., 2007). In addition, Synaptophysin may act to chaperone Syb2 and prevent premature SNARE complex re-assembly. Given the lack of a Synaptophysin homolog in Drosophila (Stevens et al., 2012), additional mechanisms to support v-SNARE endocytosis and chaperoning likely exist.
Ultrafast endocytosis is a newly proposed mechanism for Dynamin-dependent SV formation where the plasma membrane immediately buckles into the cytoplasm when SVs fuse (Watanabe et al., 2013a,b; Gan and Watanabe, 2018). Optically stimulated C. elegans motoneurons fixed within 25 milliseconds of SV release show large membrane invaginations at the periphery of the AZ that quickly resolve into cytosolic endosomes (Watanabe et al., 2013a). Following Dynamin activity, Clathrin-mediated fission of these endosomes generates new SVs. However, it is currently unclear whether the rapidly invaginated membrane compartments observed by EM contain SV proteins that were just exocytosed. This form of endocytosis may primarily act to relieve plasma membrane tension by internalizing lipids rather than fused SV proteins. Given the slow kinetics of NSF-mediated SNARE disassembly and the rapid time-course of ultrafast endocytosis, it is unlikely NSF could disassemble cis-SNARE complexes prior to plasma membrane internalization via this mechanism. As such, ultrafast endocytosis may internalize SV material lost during prior rounds of fusion. Indeed, some SV cargo like Syt1 and Syb2 are localized to the plasma membrane in resting mammalian neurons (Fernández-Alfonso et al., 2006), potentially allowing ultrafast endocytosis to draw from a pool of SV material normally found on the plasma membrane.
SNAREs, Rabs, and Rab Effectors Contribute to Target Specificity for Membrane Fusion
Following endocytosis, SVs navigate a host of non-target presynaptic membrane compartments as they traffic back to AZs. Dozens of t- and v-SNAREs are encoded in eukaryotic genomes, implying a large combinatorial assortment of possible SNARE complexes across different subcellular compartments. Only a subset of these theoretical complexes associate in vitro and in vivo to promote membrane fusion (Söllner et al., 1993; McNew et al., 2000a), suggesting cognate SNARE binding contributes to fusion specificity. SRPs also facilitate specificity in membrane trafficking, with Rab proteins and their effectors acting in vesicle-target recognition upstream of SNARE interactions. Rab proteins are monomeric GTPases that specify membrane identity by associating with one or a small subset of intracellular compartments via two cysteine-linked prenyl groups (Stenmark, 2009). These lipid anchors are exposed in the Rab GTP-bound active state and occluded following GTP hydrolysis (inactive GDP-bound state), allowing Rabs to cycle on and off membranes in a GTP-dependent manner (Pereira-Leal et al., 2001; Stenmark and Olkkonen, 2001). Rabs sculpt intracellular membrane composition by recruiting and activating effector proteins that include tethering factors, SNAREs, cytoskeleton modifiers, lipid kinases/phosphatases, endocytic proteins, and protein scaffolds (Grosshans et al., 2006; Stenmark, 2009). Among this group, tethering factors form critical Rab effectors for mediating membrane fusion specificity. These multimeric protein complexes project farther into the cytosol than SNARE proteins, identifying and luring vesicles via specific affinity for vesicular Rabs or SNAREs (Whyte and Munro, 2002; Yu and Hughson, 2010; Witkos and Lowe, 2015; Spang, 2016). Tethering complexes are phylogenetically diverse and include the Exocyst, Golgin, CORVET, and HOPS complexes, each tethering a distinct vesicle type to their cognate target membrane. After tethering factors bring vesicles to the appropriate target compartment, trans-SNARE assembly docks and primes them for fusion.
At the synapse, AZ components project into the cytoplasm to engage and recruit SVs to release sites via several large scaffolding proteins (Zhai and Bellen, 2004; Südhof, 2012; Ghelani and Sigrist, 2018). One of the most prominent AZ scaffold proteins in Drosophila is the ELKS/CAST-like protein BRP (Wagh et al., 2006). Brp null mutants lack the electron-dense T-bar structure at Drosophila AZs and have reduced evoked release secondary to decreased AZ Ca2+ channel density (Kittel et al., 2006; Wagh et al., 2006). Brp mutants lacking only the cytosolic C-terminus of the protein (Brpnude) have normal AZ dense body projections and Ca2+ channel clustering, but fail to accumulate SVs that normally surround the AZ due to defective Cpx binding (Hallermann et al., 2010; Scholz et al., 2019). Brpnude mutants display normal evoked release at low frequency, but severely reduced release following repetitive stimulation. These data indicate BRP is not an essential tethering factor for SV docking and priming, but clusters SVs for fast refilling of release sites to support sustained activity.
Among the AZ proteins that mediate SV targeting, RIM tethers vesicles to release sites via its interaction with Rab3 (Wang et al., 1997; Betz et al., 2001; Koushika et al., 2001; Schoch et al., 2002; Han et al., 2011). RIM mutants have severely impaired evoked release accompanied by a dramatic reduction in SV docking (Gracheva et al., 2008; Han et al., 2011; Graf et al., 2012; Kushibiki et al., 2019; Oh et al., 2021). Together with the AZ protein SYD-2/Liprin, the C. elegans RIM homolog Unc10 forms filamentous projections that extend from the AZ to capture and tether Rab3 bound SVs at release sites (Gracheva et al., 2008; Stigloher et al., 2011). Rab3 mutants also show defects in SV recruitment, but have more modest impairments in evoked release (Geppert et al., 1997; Nonet et al., 1997; Schlüter et al., 2006; Gracheva et al., 2008; Graf et al., 2009). Drosophila and C. elegans RIM/Rab3 double mutants do not have enhanced release defects, suggesting they operate in a similar pathway. Beyond SV tethering, Drosophila Rab3 mutants have defects in AZ maturation, with a subset of release sites lacking late AZ scaffold proteins required for efficient evoked release (Graf et al., 2009). This AZ maturation phenotype is not observed in RIM mutants, suggesting Rab3 interfaces with other Rab effector proteins to deliver AZ components during synaptic development.
RIM is hypothesized to partner with Unc13 to transition SVs from a tethered state to the downstream Unc18/Unc13-dependent priming mechanism (Liu et al., 2019). RIM and Unc13 display highly conserved interactions mediated by a C2 domain in Unc13 and a zinc finger domain of RIM. Disruption of the RIM/Unc13 interaction reduces the number of fusion competent SVs, suggesting efficient SV recruitment requires engagement with Unc13 (Betz et al., 2001). Homodimerization of Unc13 in C. elegans generates an autoinhibitory state that is relieved by RIM binding prior to SV priming (Liu et al., 2019). These biochemical interactions suggest a model where Unc13 autoinhibition is relieved by RIM before it primes Syx1 for SNARE complex assembly. Given the RIM zinc finger domain also interacts with Rab3 (Betz et al., 2001; Weimer et al., 2006; Liu et al., 2019), priming may be coupled to SV arrival through competition for RIM binding between Unc13 and SV-bound Rab3. Release of RIM from Rab3 would allow it to activate Unc13 for Syx1 priming, with open Syx1 engaging Unc18 on a distinct interaction surface that templates the onset of SNARE complex assembly between Syx1 and SV-localized Syb2. Release defects in both Unc10/RIM and Unc13 can be rescued by the open-Syx1 mutation (Tien et al., 2020), consistent with RIM-mediated SV tethering facilitating downstream SNARE-dependent SV priming.
Tomosyn Acts as a Decoy SNARE to Negatively Regulate SNARE Complex Assembly
SNARE proteins incompatible with membrane fusion, known as decoy SNAREs, provide an additional mechanism to ensure regulated SNARE assembly. Several decoy SNAREs have been described in mammals, but Tomosyn is the only known invertebrate decoy SNARE (Masuda et al., 1998; Lao et al., 2000; Scales et al., 2002; Gerst, 2003; Ashery et al., 2009). Tomosyn was originally identified for its ability to displace Syx1 from Unc18, and suggested to engage Syx1 and SNAP-25 in an intermediate stage of SNARE assembly preceding trans-SNARE complex formation (Fujita et al., 1998; Masuda et al., 1998; Pobbati et al., 2004). Subsequent experiments revealed Tomosyn prevents Syb2 binding to t-SNAREs, suggesting this complex is not a prefusion intermediate but may rather inhibit productive SNARE complex assembly (Yokoyama et al., 1999). Tomosyn is a large cytoplasmic protein with a Syb2-like R-SNARE motif at its C-terminus that forms a four-helical SNARE complex with synaptic t-SNAREs (Figure 6). However, the absence of a membrane anchor in Tomosyn prevents formation of fusogenic SNARE complexes. At the N-terminus, Tomosyn contains WD40 repeats organized into a propeller-like scaffold with homology to L(2)GL proteins (Lehman et al., 1999; Williams et al., 2011). In vivo studies suggest Tomosyn can also interact with SNAREs beyond its R-SNARE motif, as the yeast Tomosyn-like protein Sro7 lacks a SNARE motif and uses its scaffold domain to coordinate vesicle docking with SNARE assembly by binding the Sec9 t-SNARE (Lehman et al., 1999; McEwen et al., 2006; Yizhar et al., 2007; Yamamoto et al., 2010).
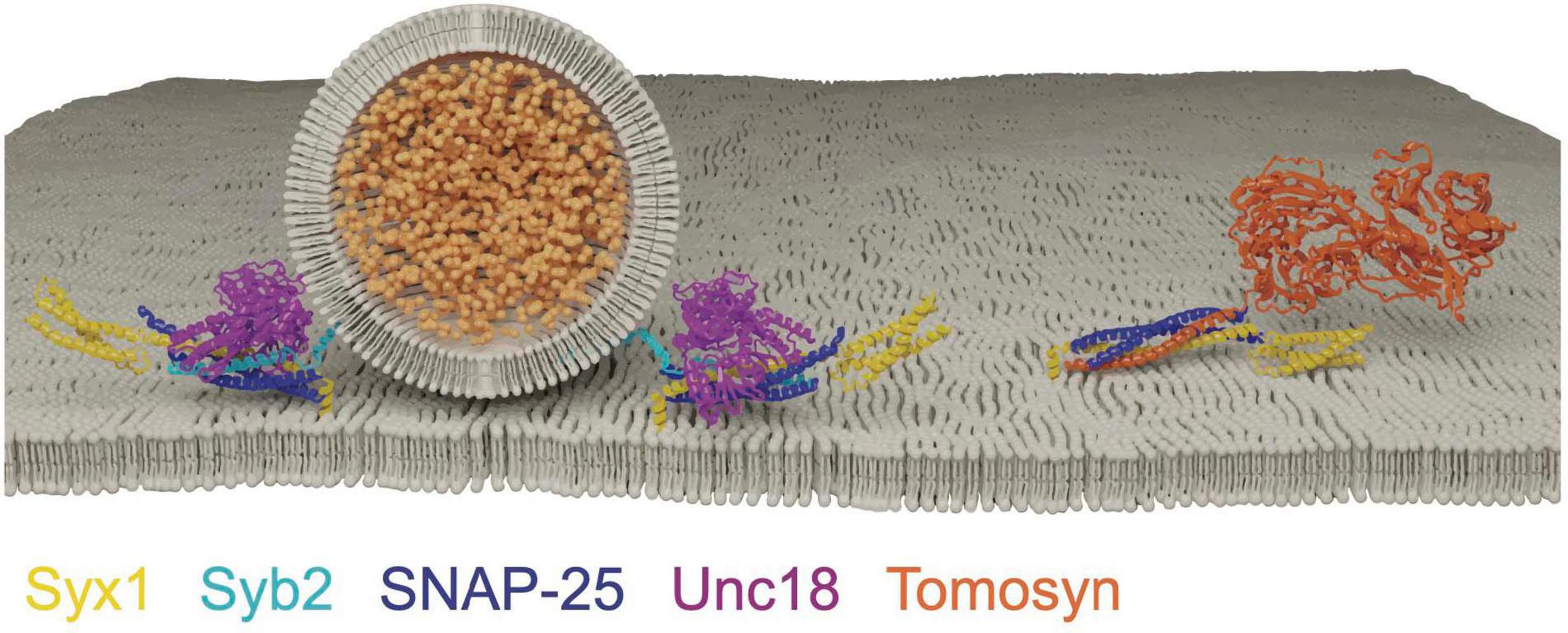
Figure 6. Tomosyn forms a decoy SNARE complex with Syx1 and SNAP-25 in an Unc18 and Syb2 independent manner. Adapted from Pobbati et al. (2004); Hattendorf et al. (2007).
Tomosyn overexpression reduces both constitutive and regulated secretion in a diverse set of eukaryotes, suggesting a highly conserved inhibitory role (Hatsuzawa et al., 2003; Yizhar et al., 2004; Gracheva et al., 2006; Zhang et al., 2006; Chen et al., 2011; Williams et al., 2011; Li et al., 2019). Null mutations in C. elegans Tomosyn (tom-1) show a dramatic increase in evoked neurotransmitter release (Gracheva et al., 2006; McEwen et al., 2006; Burdina et al., 2011). Whether this phenotype is caused by reduced decoy SNARE activity remains unclear given the scaffold and SNARE domains are both required in tandem to suppress release (Burdina et al., 2011). Genetic interactions in C. elegans suggest release suppression by Tomosyn may prevent Unc13/Unc-18-independent SNARE assembly. The total elimination of release in unc-13 and unc-18 single mutants indicate SV priming normally occurs exclusively via an Unc13/Unc18-dependent mechanism. Remarkably, double mutants of tom-1/unc-13 and tom-1/unc-18 partially restore the loss of evoked and spontaneous release in unc-13 and unc-18 single mutants, indicating Tomosyn suppresses a pathway that would otherwise bypass Unc13 and Unc18 to generate dysregulated priming (McEwen et al., 2006; Gracheva et al., 2010; Hu et al., 2013). In vitro reconstitution experiments indicate Tomosyn does not interfere with Unc13/Unc18-chaperoned SNARE assembly, suggesting Tomosyn can only engage Syx1 in an Unc13/Unc18-independent manner (Li Y. et al., 2018). NSF disassembly of the Tomosyn/t-SNARE complex leads to Unc18 capture of Syx1 for incorporation into productive SNARE complexes (Hatsuzawa et al., 2003; Li Y. et al., 2018). In vivo, tom-1 enhanced release is exaggerated by the open-Syx1 mutation, causing a further increase in tom-1 sensitivity to the acetylcholinesterase inhibitor aldicarb (Tien et al., 2020). Enhanced SV fusion in tom-1 exceeds the residual release in tom-1/unc-13 and tom-1/unc-18 double mutants, indicating Tomosyn also suppresses SNARE assembly within the traditional Unc13/Unc18 priming pathway. Together, these data indicate Tomosyn ensures tight regulation of SNARE complex assembly by acting as a failsafe to prevent dysregulated Unc13/Unc18-independent priming of Syx1.
Conclusion and Future Directions
SRPs guide SNARE interactions during multiple steps of the SV fusion cycle by localizing SNARE assembly, regulating Ca2+-dependent SNARE zippering, recycling SNAREs post-fusion and inhibiting dysregulated SV priming (Figure 7). Given their critical roles in synaptic communication, it is not surprising that mutations in these genes cause a host of severe human neurological disorders (Melom and Littleton, 2011; Engel et al., 2016; Lipstein et al., 2017; Redler et al., 2017; Salpietro et al., 2019; Abramov et al., 2021; Melland et al., 2021). SRPs and SNAREs are broadly expressed in all neurons and are ideally positioned to regulate intrinsic synaptic release strength and presynaptic plasticity. Given presynaptic output and plasticity mechanisms display wide heterogeneity across neuronal subtypes and at individual release sites, SRPs are likely subject to transcriptional and post-translational control that alters their function. Indeed, SRPs can regulate release differences by controlling SV availability, spontaneous release rate, and SV priming location (Hu et al., 2013; Melom et al., 2013; Cho et al., 2015; Böhme et al., 2016; Fulterer et al., 2018; Aponte-Santiago and Littleton, 2020; Pooryasin et al., 2021).
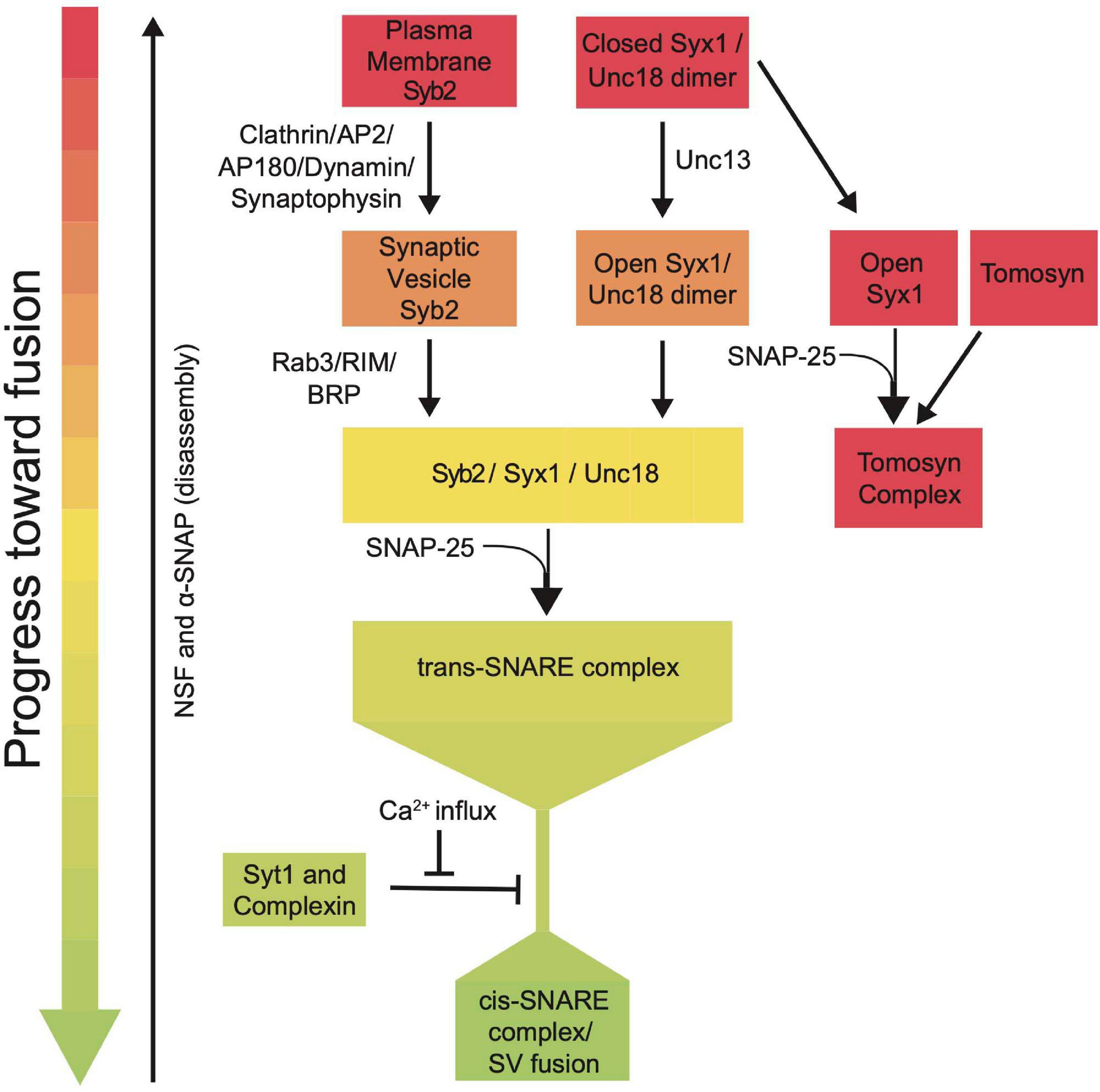
Figure 7. Summary flowchart showing current models for SRP regulation of SNARE complex assembly and SV fusion.
Several examples of SRP regulation that alter synaptic function or plasticity have been characterized. Phosphorylation of Tomosyn by protein kinase A (PKA) prevents its ability to inhibit SV availability, thereby enhancing SV release to facilitate plasticity and memory formation (Baba et al., 2005; Chen et al., 2011; Ben-Simon et al., 2015). Additionally, PKA phosphorylation of a Drosophila Cpx isoform occurs during activity-dependent retrograde signaling that reduces its clamping function, allowing activity-dependent increases in spontaneous SV release to promote synaptic growth and development (Yoshihara et al., 2005; Huntwork and Littleton, 2007; Barber et al., 2009; Choi et al., 2014; Cho et al., 2015; Andreae and Burrone, 2018). The synaptic signaling molecule nitric oxide (NO) enhances synaptic transmission by modifying Cpx function in Drosophila, with NO-regulated S-nitrosylation of the Cpx C-terminus altering SNARE-binding and enhancing evoked release (Robinson et al., 2018). Transcriptional regulation of Cpx can also modulate synaptic strength in Drosophila, with caloric intake and insulin signaling decreasing synaptic transmission by increasing Cpx levels downstream of the translational inhibitor FOXO (Mahoney et al., 2016). Similarly, the synaptic levels of Unc18 and Syx1 are finely tuned to regulate SV priming dynamics that are required to support presynaptic homeostatic plasticity at Drosophila NMJs (Ortega et al., 2018). Alternative splicing of Unc13 in Drosophila and C. elegans results in unique isoforms that alter the protein’s length, allowing the MUN domain to position and control SV priming at varying distances from AZ Ca2+ channel clusters (Hu et al., 2013; Böhme et al., 2016; Reddy-Alla et al., 2017; Fulterer et al., 2018; Pooryasin et al., 2021). In Drosophila, newly formed AZs first accumulate the long Unc13B splice variant before the shorter Unc13A variant arrives as AZs mature. Unc13B does not support efficient evoked release but is sufficient for spontaneous fusion, while Unc13A is required for SV priming for evoked but not spontaneous release (Böhme et al., 2016). Short isoforms of Unc13 in C. elegans also promote greater evoked release than longer isoforms (Hu et al., 2013), suggesting alternative Unc13 splicing represents a general mechanism for altering the efficiency of SV release by controlling where SV priming occurs along the AZ. These examples highlight only a subset of the mechanisms by which SNARE function might be dynamically controlled by SRPs. Future studies will certainly provide additional insights into how regulation of these essential presynaptic gatekeepers of brain communication contribute to the diversity of synaptic function and plasticity observed across distinct neuronal populations.
Author Contributions
Both authors wrote and edited the manuscript, contributed to the article, and approved the submitted version.
Funding
This work has been funded by NIH grants NS40296, NS117588, and MH104536 and the JPB Foundation.
Conflict of Interest
The authors declare that the research was conducted in the absence of any commercial or financial relationships that could be construed as a potential conflict of interest.
Publisher’s Note
All claims expressed in this article are solely those of the authors and do not necessarily represent those of their affiliated organizations, or those of the publisher, the editors and the reviewers. Any product that may be evaluated in this article, or claim that may be made by its manufacturer, is not guaranteed or endorsed by the publisher.
References
Abramov, D., Guiberson, N. G. L., and Burré, J. (2021). STXBP1 encephalopathies: clinical spectrum, disease mechanisms, and therapeutic strategies. J. Neurochem. 157, 165–178. doi: 10.1111/jnc.15120
Ackermann, F., Waites, C. L., and Garner, C. C. (2015). Presynaptic active zones in invertebrates and vertebrates. EMBO Rep. 16, 923–938. doi: 10.15252/embr.201540434
Akbergenova, Y., Cunningham, K. L., Zhang, Y. V., Weiss, S., and Littleton, J. T. (2018). Characterization of developmental and molecular factors underlying release heterogeneity at Drosophila synapses. eLife 7:e38268. doi: 10.7554/eLife.38268
Andreae, L. C., and Burrone, J. (2018). The role of spontaneous neurotransmission in synapse and circuit development. J. Neurosci. Res. 96, 354–359. doi: 10.1002/jnr.24154
Aponte-Santiago, N. A., and Littleton, J. T. (2020). Synaptic properties and plasticity mechanisms of invertebrate tonic and phasic neurons. Front. Physiol. 11:611982. doi: 10.3389/fphys.2020.611982
Aravamudan, B., Fergestad, T., Davis, W. S., Rodesch, C. K., and Broadie, K. (1999). Drosophila UNC-13 is essential for synaptic transmission. Nat. Neurosci. 2, 965–971. doi: 10.1038/14764
Aravanis, A. M., Pyle, J. L., and Tsien, R. W. (2003). Single synaptic vesicles fusing transiently and successively without loss of identity. Nature 423, 643–647. doi: 10.1038/nature01686
Artalejo, C. R., Elhamdani, A., and Palfrey, H. C. (1998). Secretion: dense-core vesicles can kiss-and-run too. Curr. Biol. 8, R62–R65. doi: 10.1016/s0960-9822(98)70036-3
Ashery, U., Bielopolski, N., Barak, B., and Yizhar, O. (2009). Friends and foes in synaptic transmission: the role of tomosyn in vesicle priming. Trends Neurosci. 32, 275–282. doi: 10.1016/j.tins.2009.01.004
Atwood, H. L., and Karunanithi, S. (2002). Diversification of synaptic strength: presynaptic elements. Nat. Rev. Neurosci. 3, 497–516. doi: 10.1038/nrn876
Augustin, I., Korte, S., Rickmann, M., Kretzschmar, H. A., Südhof, T. C., Herms, J. W., et al. (2001). The cerebellum-specific Munc13 isoform Munc13-3 regulates cerebellar synaptic transmission and motor learning in mice. J. Neurosci. 21, 10–17. doi: 10.1523/jneurosci.21-01-00010.2001
Augustin, I., Rosenmund, C., Südhof, T. C., and Brose, N. (1999). Munc13-1 is essential for fusion competence of glutamatergic synaptic vesicles. Nature 400, 457–461. doi: 10.1038/22768
Baba, T., Sakisaka, T., Mochida, S., and Takai, Y. (2005). PKA-catalyzed phosphorylation of tomosyn and its implication in Ca2+-dependent exocytosis of neurotransmitter. J. Cell Biol. 170, 1113–1125. doi: 10.1083/jcb.200504055
Babcock, M., Macleod, G. T., Leither, J., and Pallanck, L. (2004). Genetic analysis of soluble N-ethylmaleimide-sensitive factor attachment protein function in Drosophila reveals positive and negative secretory roles. J. Neurosci. 24, 3964–3973. doi: 10.1523/jneurosci.5259-03.2004
Bajohrs, M., Rickman, C., Binz, T., and Davletov, B. (2004). A molecular basis underlying differences in the toxicity of botulinum serotypes A and E. EMBO Rep. 5, 1090–1095. doi: 10.1038/sj.embor.7400278
Baker, R. W., Jeffrey, P. D., Zick, M., Phillips, B. P., Wickner, W. T., and Hughson, F. M. (2015). A direct role for the Sec1/Munc18-family protein Vps33 as a template for SNARE assembly. Science 349, 1111–1114. doi: 10.1126/science.aac7906
Bao, H., Daniels, R. W., MacLeod, G. T., Charlton, M. P., Atwood, H. L., and Zhang, B. (2005). AP180 maintains the distribution of synaptic and vesicle proteins in the nerve terminal and indirectly regulates the efficacy of Ca2+-triggered exocytosis. J. Neurophysiol. 94, 1888–1903. doi: 10.1152/jn.00080.2005
Barber, C. F., Jorquera, R. A., Melom, J. E., and Littleton, J. T. (2009). Postsynaptic regulation of synaptic plasticity by synaptotagmin 4 requires both C2 domains. J. Cell Biol. 187, 295–310. doi: 10.1083/jcb.200903098
Bargmann, C. I. (1993). Genetic and cellular analysis of behavior in C. elegans. Annu. Rev. Neurosci. 16, 47–71. doi: 10.1146/annurev.ne.16.030193.000403
Barnard, R. J., Morgan, A., and Burgoyne, R. D. (1997). Stimulation of NSF ATPase activity by alpha-SNAP is required for SNARE complex disassembly and exocytosis. J. Cell Biol. 139, 875–883. doi: 10.1083/jcb.139.4.875
Bennett, M. K., Calakos, N., Kreiner, T., and Scheller, R. H. (1992a). Synaptic vesicle membrane proteins interact to form a multimeric complex. J. Cell Biol. 116, 761–775. doi: 10.1083/jcb.116.3.761
Bennett, M. K., Calakos, N., and Scheller, R. H. (1992b). Syntaxin: a synaptic protein implicated in docking of synaptic vesicles at presynaptic active zones. Science 257, 255–259. doi: 10.1126/science.1321498
Bennett, M. K., and Scheller, R. H. (1993). The molecular machinery for secretion is conserved from yeast to neurons. Proc. Natl. Acad. Sci. U.S.A. 90, 2559–2563. doi: 10.1073/pnas.90.7.2559
Ben-Simon, Y., Rodenas-Ruano, A., Alviña, K., Lam, A. D., Stuenkel, E. L., Castillo, P. E., et al. (2015). A combined optogenetic-knockdown strategy reveals a major role of tomosyn in mossy fiber synaptic plasticity. Cell Rep. 12, 396–404. doi: 10.1016/j.celrep.2015.06.037
Betz, A., Okamoto, M., Benseler, F., and Brose, N. (1997). Direct interaction of the rat unc-13 homologue Munc13-1 with the N terminus of syntaxin. J. Biol. Chem. 272, 2520–2526. doi: 10.1074/jbc.272.4.2520
Betz, A., Thakur, P., Junge, H. J., Ashery, U., Rhee, J. S., Scheuss, V., et al. (2001). Functional interaction of the active zone proteins Munc13-1 and RIM1 in synaptic vesicle priming. Neuron 30, 183–196. doi: 10.1016/s0896-6273(01)00272-0
Bhattacharya, S., Stewart, B. A., Niemeyer, B. A., Burgess, R. W., McCabe, B. D., Lin, P., et al. (2002). Members of the synaptobrevin/vesicle-associated membrane protein (VAMP) family in Drosophila are functionally interchangeable in vivo for neurotransmitter release and cell viability. Proc. Natl. Acad. Sci. U.S.A. 99, 13867–13872. doi: 10.1073/pnas.202335999
Block, M. R., Glick, B. S., Wilcox, C. A., Wieland, F. T., and Rothman, J. E. (1988). Purification of an N-ethylmaleimide-sensitive protein catalyzing vesicular transport. Proc. Natl. Acad. Sci. U.S.A. 85, 7852–7856. doi: 10.1073/pnas.85.21.7852
Böhme, M. A., Beis, C., Reddy-Alla, S., Reynolds, E., Mampell, M. M., Grasskamp, A. T., et al. (2016). Active zone scaffolds differentially accumulate Unc13 isoforms to tune Ca(2+) channel-vesicle coupling. Nat. Neurosci. 19, 1311–1320. doi: 10.1038/nn.4364
Bonanomi, D., Rusconi, L., Colombo, C. A., Benfenati, F., and Valtorta, F. (2007). Synaptophysin I selectively specifies the exocytic pathway of synaptobrevin 2/VAMP2. Biochem. J. 404, 525–534. doi: 10.1042/BJ20061907
Borgese, N., Colombo, S., and Pedrazzini, E. (2003). The tale of tail-anchored proteins: coming from the cytosol and looking for a membrane. J. Cell Biol. 161, 1013–1019. doi: 10.1083/jcb.200303069
Boulianne, G. L., and Trimble, W. S. (1995). Identification of a second homolog of N-ethylmaleimide-sensitive fusion protein that is expressed in the nervous system and secretory tissues of Drosophila. Proc. Natl. Acad. Sci. U.S.A. 92, 7095–7099. doi: 10.1073/pnas.92.15.7095
Bowen, M. E., Weninger, K., Ernst, J., Chu, S., and Brunger, A. T. (2005). Single-molecule studies of synaptotagmin and complexin binding to the SNARE complex. Biophys. J. 89, 690–702. doi: 10.1529/biophysj.104.054064
Bowers, M. R., and Reist, N. E. (2020). The C2A domain of synaptotagmin is an essential component of the calcium sensor for synaptic transmission. PLoS One 15:e0228348. doi: 10.1371/journal.pone.0228348
Brady, J., Vasin, A., and Bykhovskaia, M. (2021). The accessory helix of complexin stabilizes a partially unzippered state of the SNARE complex and mediates the complexin clamping function in vivo. Eneuro 8:ENEURO.0526-20.2021. doi: 10.1523/ENEURO.0526-20.2021
Breidenbach, M. A., and Brunger, A. T. (2005). New insights into clostridial neurotoxin-SNARE interactions. Trends Mol. Med. 11, 377–381. doi: 10.1016/j.molmed.2005.06.012
Brenner, S. (1973). The genetics of behaviour. Br. Med. Bull. 29, 269–271. doi: 10.1093/oxfordjournals.bmb.a071019
Broadie, K., Prokop, A., Bellen, H. J., O’Kane, C. J., Schulze, K. L., and Sweeney, S. T. (1995). Syntaxin and synaptobrevin function downstream of vesicle docking in Drosophila. Neuron 15, 663–673. doi: 10.1016/0896-6273(95)90154-x
Bronson, R. T., and Lane, P. W. (1990). Hydrocephalus with hop gait (hyh): a new mutation on chromosome 7 in the mouse. Brain Res. Dev. Brain Res. 54, 131–136. doi: 10.1016/0165-3806(90)90073-8
Brose, N., Rosenmund, C., and Rettig, J. (2000). Regulation of transmitter release by Unc-13 and its homologues. Curr. Opin. Neurobiol. 10, 303–311. doi: 10.1016/S0959-4388(00)00105-7
Brunger, A. T., Choi, U. B., Lai, Y., Leitz, J., White, K. I., and Zhou, Q. (2019). The pre-synaptic fusion machinery. Curr. Opin. Struct. Biol. 54, 179–188. doi: 10.1016/j.sbi.2019.03.007
Bucurenciu, I., Kulik, A., Schwaller, B., Frotscher, M., and Jonas, P. (2008). Nanodomain coupling between Ca2+ channels and Ca2+ sensors promotes fast and efficient transmitter release at a cortical GABAergic synapse. Neuron 57, 536–545. doi: 10.1016/j.neuron.2007.12.026
Buhl, L. K., Jorquera, R. A., Akbergenova, Y., Huntwork-Rodriguez, S., Volfson, D., and Littleton, J. T. (2013). Differential regulation of evoked and spontaneous neurotransmitter release by C-terminal modifications of complexin. Mol. Cell. Neurosci. 52, 161–172. doi: 10.1016/j.mcn.2012.11.009
Burdina, A. O., Klosterman, S. M., Shtessel, L., Ahmed, S., and Richmond, J. E. (2011). In vivo analysis of conserved C. elegans tomosyn domains. PLoS One 6:e26185. doi: 10.1371/journal.pone.0026185
Burgalossi, A., Jung, S., Meyer, G., Jockusch, W. J., Jahn, O., Taschenberger, H., et al. (2010). SNARE protein recycling by αSNAP and βSNAP supports synaptic vesicle priming. Neuron 68, 473–487. doi: 10.1016/j.neuron.2010.09.019
Burgess, R. W., Deitcher, D. L., and Schwarz, T. L. (1997). The synaptic protein syntaxin1 is required for cellularization of Drosophila embryos. J. Cell Biol. 138, 861–875. doi: 10.1083/jcb.138.4.861
Burgess, T. L., and Kelly, R. B. (1987). Constitutive and regulated secretion of proteins. Annu. Rev. Cell Biol. 3, 243–293. doi: 10.1146/annurev.cb.03.110187.001331
Burkhardt, P., Hattendorf, D. A., Weis, W. I., and Fasshauer, D. (2008). Munc18a controls SNARE assembly through its interaction with the syntaxin N-peptide. EMBO J. 27, 923–933. doi: 10.1038/emboj.2008.37
Bykhovskaia, M., Jagota, A., Gonzalez, A., Vasin, A., and Littleton, J. T. (2013). Interaction of the complexin accessory helix with the C-terminus of the SNARE complex: molecular-dynamics model of the fusion clamp. Biophys. J. 105, 679–690. doi: 10.1016/j.bpj.2013.06.018
C. elegans Deletion Mutant Consortium (2012). Large-scale screening for targeted knockouts in the Caenorhabditis elegans genome. G3 2, 1415–1425. doi: 10.1534/g3.112.003830
Ceccarelli, B., Hurlbut, W. P., and Mauro, A. (1973). Turnover of transmitter and synaptic vesicles at the frog neuromuscular junction. J. Cell Biol. 57, 499–524. doi: 10.1083/jcb.57.2.499
Chae, T. H., Kim, S., Marz, K. E., Hanson, P. I., and Walsh, C. A. (2004). The hyh mutation uncovers roles for alpha Snap in apical protein localization and control of neural cell fate. Nat. Genet. 36, 264–270. doi: 10.1038/ng1302
Chanaday, N. L., Cousin, M. A., Milosevic, I., Watanabe, S., and Morgan, J. R. (2019). The synaptic vesicle cycle revisited: new insights into the modes and mechanisms. J. Neurosci. 39, 8209–8216. doi: 10.1523/JNEUROSCI.1158-19.2019
Chang, S., Reim, K., Pedersen, M., Neher, E., Brose, N., and Taschenberger, H. (2015). Complexin stabilizes newly primed synaptic vesicles and prevents their premature fusion at the mouse calyx of held synapse. J. Neurosci. 35, 8272–8290. doi: 10.1523/JNEUROSCI.4841-14.2015
Chapman, E. R. (2008). How does synaptotagmin trigger neurotransmitter release? Annu. Rev. Biochem. 77, 615–641. doi: 10.1146/annurev.biochem.77.062005.101135
Chapman, E. R., and Davis, A. F. (1998). Direct interaction of a Ca2+-binding loop of synaptotagmin with lipid bilayers. J. Biol. Chem. 273, 13995–14001. doi: 10.1074/jbc.273.22.13995
Chapman, E. R., Desai, R. C., Davis, A. F., and Tornehl, C. K. (1998). Delineation of the oligomerization, AP-2 binding, and synprint binding region of the C2B domain of synaptotagmin. J. Biol. Chem. 273, 32966–32972. doi: 10.1074/jbc.273.49.32966
Chen, K., Richlitzki, A., Featherstone, D. E., Schwärzel, M., and Richmond, J. E. (2011). Tomosyn-dependent regulation of synaptic transmission is required for a late phase of associative odor memory. Proc. Natl. Acad. Sci. U.S.A. 108, 18482–18487. doi: 10.1073/pnas.1110184108
Chen, M. S., Obar, R. A., Schroeder, C. C., Austin, T. W., Poodry, C. A., Wadsworth, S. C., et al. (1991). Multiple forms of dynamin are encoded by shibire, a Drosophila gene involved in endocytosis. Nature 351, 583–586. doi: 10.1038/351583a0
Chin, A. C., Burgess, R. W., Wong, B. R., Schwarz, T. L., and Scheller, R. H. (1993). Differential expression of transcripts from syb, a Drosophila melanogaster gene encoding VAMP (synaptobrevin) that is abundant in non-neuronal cells. Gene 131, 175–181. doi: 10.1016/0378-1119(93)90291-a
Cho, R. W., Buhl, L. K., Volfson, D., Tran, A., Li, F., Akbergenova, Y., et al. (2015). Phosphorylation of Complexin by PKA regulates activity-dependent spontaneous neurotransmitter release and structural synaptic plasticity. Neuron 88, 749–761. doi: 10.1016/j.neuron.2015.10.011
Cho, R. W., Kümmel, D., Li, F., Baguley, S. W., Coleman, J., Rothman, J. E., et al. (2014). Genetic analysis of the Complexin trans-clamping model for cross-linking SNARE complexes in vivo. Proc. Natl. Acad. Sci. U.S.A. 111, 10317–10322. doi: 10.1073/pnas.1409311111
Cho, R. W., Song, Y., and Littleton, J. T. (2010). Comparative analysis of Drosophila and mammalian complexins as fusion clamps and facilitators of neurotransmitter release. Mol. Cell. Neurosci. 45, 389–397. doi: 10.1016/j.mcn.2010.07.012
Choi, B. J., Imlach, W. L., Jiao, W., Wolfram, V., Wu, Y., Grbic, M., et al. (2014). Miniature neurotransmission regulates Drosophila synaptic structural maturation. Neuron 82, 618–634. doi: 10.1016/j.neuron.2014.03.012
Cipriano, D. J., Jung, J., Vivona, S., Fenn, T. D., Brunger, A. T., and Bryant, Z. (2013). Processive ATP-driven substrate disassembly by the N-ethylmaleimide-sensitive factor (NSF) molecular machine. J. Biol. Chem. 288, 23436–23445. doi: 10.1074/jbc.M113.476705
Clary, D. O., Griff, I. C., and Rothman, J. E. (1990). SNAPs, a family of NSF attachment proteins involved in intracellular membrane fusion in animals and yeast. Cell 61, 709–721. doi: 10.1016/0092-8674(90)90482-t
Cremona, O., and De Camilli, P. (1997). Synaptic vesicle endocytosis. Curr. Opin. Neurobiol. 7, 323–330. doi: 10.1016/S0959-4388(97)80059-1
Davletov, B. A., and Südhof, T. C. (1993). A single C2 domain from synaptotagmin I is sufficient for high affinity Ca2+/phospholipid binding. J. Biol. Chem. 268, 26386–26390. doi: 10.1016/s0021-9258(19)74326-9
DeBruhl, H., Albertson, R., Swider, Z., and Sullivan, W. (2016). Rop, the Sec1/Munc18 homolog in Drosophila, is required for furrow ingression and stable cell shape during cytokinesis. J. Cell Sci. 129, 430–443. doi: 10.1242/jcs.179200
Deitcher, D. L., Ueda, A., Stewart, B. A., Burgess, R. W., Kidokoro, Y., and Schwarz, T. L. (1998). Distinct requirements for evoked and spontaneous release of neurotransmitter are revealed by mutations in the Drosophila gene neuronal-synaptobrevin. J. Neurosci. 18, 2028–2039. doi: 10.1523/jneurosci.18-06-02028.1998
Delgado, R., Maureira, C., Oliva, C., Kidokoro, Y., and Labarca, P. (2000). Size of vesicle pools, rates of mobilization, and recycling at neuromuscular synapses of a Drosophila mutant, shibire. Neuron 28, 941–953. doi: 10.1016/S0896-6273(00)00165-3
DiAntonio, A., Burgess, R. W., Chin, A. C., Deitcher, D. L., Scheller, R. H., and Schwarz, T. L. (1993). Identification and characterization of Drosophila genes for synaptic vesicle proteins. J. Neurosci. 13, 4924–4935. doi: 10.1523/jneurosci.13-11-04924.1993
DiAntonio, A., and Schwarz, T. L. (1994). The effect on synaptic physiology of synaptotagmin mutations in Drosophila. Neuron 12, 909–920. doi: 10.1016/0896-6273(94)90342-5
Dickinson, M. E., Flenniken, A. M., Ji, X., Teboul, L., Wong, M. D., White, J. K., et al. (2016). High-throughput discovery of novel developmental phenotypes. Nature 537, 508–514. doi: 10.1038/nature19356
Diril, M. K., Wienisch, M., Jung, N., Klingauf, J., and Haucke, V. (2006). Stonin 2 is an AP-2-dependent endocytic sorting adaptor for synaptotagmin internalization and recycling. Dev. Cell 10, 233–244. doi: 10.1016/j.devcel.2005.12.011
Dittman, J., and Ryan, T. A. (2009). Molecular circuitry of endocytosis at nerve terminals. Annu. Rev. Cell Dev. Biol. 25, 133–160. doi: 10.1146/annurev.cellbio.042308.113302
Dulubova, I., Khvotchev, M., Liu, S., Huryeva, I., Südhof, T. C., and Rizo, J. (2007). Munc18-1 binds directly to the neuronal SNARE complex. Proc. Natl. Acad. Sci. U.S.A. 104, 2697–2702. doi: 10.1073/pnas.0611318104
Dulubova, I., Sugita, S., Hill, S., Hosaka, M., Fernandez, I., Südhof, T. C., et al. (1999). A conformational switch in syntaxin during exocytosis: role of munc18. EMBO J. 18, 4372–4382. doi: 10.1093/emboj/18.16.4372
Edelmann, L., Hanson, P. I., Chapman, E. R., and Jahn, R. (1995). Synaptobrevin binding to synaptophysin: a potential mechanism for controlling the exocytotic fusion machine. EMBO J. 14, 224–231. doi: 10.1002/j.1460-2075.1995.tb06995.x
Engel, A. G., Selcen, D., Shen, X.-M., Milone, M., and Harper, C. M. (2016). Loss of MUNC13-1 function causes microcephaly, cortical hyperexcitability, and fatal myasthenia. Neurol. Genet. 2:e105. doi: 10.1212/NXG.0000000000000105
Estes, P. S., Roos, J., van der Bliek, A., Kelly, R. B., Krishnan, K. S., and Ramaswami, M. (1996). Traffic of dynamin within individual Drosophila synaptic boutons relative to compartment-specific markers. J. Neurosci. 16, 5443–5456. doi: 10.1523/jneurosci.16-17-05443.1996
Fasshauer, D. (2003). Structural insights into the SNARE mechanism. Biochim. Biophys. Acta 1641, 87–97. doi: 10.1016/s0167-4889(03)00090-9
Fasshauer, D., Antonin, W., Subramaniam, V., and Jahn, R. (2002). SNARE assembly and disassembly exhibit a pronounced hysteresis. Nat. Struct. Biol. 9, 144–151. doi: 10.1038/nsb750
Fasshauer, D., Sutton, R. B., Brunger, A. T., and Jahn, R. (1998). Conserved structural features of the synaptic fusion complex: SNARE proteins reclassified as Q- and R-SNAREs. Proc. Natl. Acad. Sci. U.S.A. 95, 15781–15786. doi: 10.1073/pnas.95.26.15781
Fergestad, T., Wu, M. N., Schulze, K. L., Lloyd, T. E., Bellen, H. J., and Broadie, K. (2001). Targeted mutations in the syntaxin H3 domain specifically disrupt SNARE complex function in synaptic transmission. J. Neurosci. 21, 9142–9150. doi: 10.1523/jneurosci.21-23-09142.2001
Fernandez, I., Araç, D., Ubach, J., Gerber, S. H., Shin, O., Gao, Y., et al. (2001). Three-dimensional structure of the synaptotagmin 1 C2B-domain: synaptotagmin 1 as a phospholipid binding machine. Neuron 32, 1057–1069.
Fernandez, I., Ubach, J., Dulubova, I., Zhang, X., Südhof, T. C., and Rizo, J. (1998). Three-dimensional structure of an evolutionarily conserved N-terminal domain of syntaxin 1A. Cell 94, 841–849. doi: 10.1016/s0092-8674(00)81742-0
Fernández-Alfonso, T., Kwan, R., and Ryan, T. A. (2006). Synaptic vesicles interchange their membrane proteins with a large surface reservoir during recycling. Neuron 51, 179–186. doi: 10.1016/j.neuron.2006.06.008
Fesce, R., Grohovaz, F., Valtorta, F., and Meldolesi, J. (1994). Neurotransmitter release: fusion or “kiss-and-run”? Trends Cell Biol. 4, 1–4. doi: 10.1016/0962-8924(94)90025-6
Ford, M. G. J., Jenni, S., and Nunnari, J. (2011). The crystal structure of dynamin. Nature 477, 561–566. doi: 10.1038/nature10441
Fujita, Y., Shirataki, H., Sakisaka, T., Asakura, T., Ohya, T., Kotani, H., et al. (1998). Tomosyn: a syntaxin-1-binding protein that forms a novel complex in the neurotransmitter release process. Neuron 20, 905–915. doi: 10.1016/s0896-6273(00)80472-9
Fulterer, A., Andlauer, T. F. M., Ender, A., Maglione, M., Eyring, K., Woitkuhn, J., et al. (2018). Active zone scaffold protein ratios tune functional diversity across brain synapses. Cell Rep. 23, 1259–1274. doi: 10.1016/j.celrep.2018.03.126
Gan, Q., and Watanabe, S. (2018). Synaptic vesicle endocytosis in different model systems. Front. Cell Neurosci. 12:171. doi: 10.3389/fncel.2018.00171
Gao, Y., Zorman, S., Gundersen, G., Xi, Z., Ma, L., Sirinakis, G., et al. (2012). Single reconstituted neuronal SNARE complexes zipper in three distinct stages. Science 337, 1340–1343. doi: 10.1126/science.1224492
Geerts, C. J., Plomp, J. J., Koopmans, B., Loos, M., van der Pijl, E. M., van der Valk, M. A., et al. (2015). Tomosyn-2 is required for normal motor performance in mice and sustains neurotransmission at motor endplates. Brain Struct. Funct. 220, 1971–1982. doi: 10.1007/s00429-014-0766-0
Geppert, M., Goda, Y., Hammer, R. E., Li, C., Rosahl, T. W., Stevens, C. F., et al. (1994). Synaptotagmin I: a major Ca2+ sensor for transmitter release at a central synapse. Cell 79, 717–727. doi: 10.1016/0092-8674(94)90556-8
Geppert, M., Goda, Y., Stevens, C. F., and Südhof, T. C. (1997). The small GTP-binding protein Rab3A regulates a late step in synaptic vesicle fusion. Nature 387, 810–814. doi: 10.1038/42954
Gerber, S. H., Rah, J.-C., Min, S.-W., Liu, X., de Wit, H., Dulubova, I., et al. (2008). Conformational switch of syntaxin-1 controls synaptic vesicle fusion. Science 321, 1507–1510. doi: 10.1126/science.1163174
Gerst, J. E. (2003). SNARE regulators: matchmakers and matchbreakers. Biochim. Biophys. Acta 1641, 99–110. doi: 10.1016/s0167-4889(03)00096-x
Ghelani, T., and Sigrist, S. J. (2018). Coupling the structural and functional assembly of synaptic release sites. Front. Neuroanat. 12:81. doi: 10.3389/fnana.2018.00081
Giraudo, C. G., Eng, W. S., Melia, T. J., and Rothman, J. E. (2006). A clamping mechanism involved in SNARE-dependent exocytosis. Science 313, 676–680. doi: 10.1126/science.1129450
Göhde, R., Naumann, B., Laundon, D., Imig, C., McDonald, K., Cooper, B. H., et al. (2021). Choanoflagellates and the ancestry of neurosecretory vesicles. Philos. Trans. R. Soc. Lond. B Biol. Sci. 376:20190759. doi: 10.1098/rstb.2019.0759
Golby, J. A., Tolar, L. A., and Pallanck, L. (2001). Partitioning of N-ethylmaleimide-sensitive fusion (NSF) protein function in Drosophila melanogaster: dNSF1 is required in the nervous system, and dNSF2 is required in mesoderm. Genetics 158, 265–278. doi: 10.1093/genetics/158.1.265
González-Gaitán, M., and Jäckle, H. (1997). Role of Drosophila alpha-adaptin in presynaptic vesicle recycling. Cell 88, 767–776. doi: 10.1016/s0092-8674(00)81923-6
Gonzalo, S., and Linder, M. E. (1998). SNAP-25 palmitoylation and plasma membrane targeting require a functional secretory pathway. Mol. Biol. Cell 9, 585–597. doi: 10.1091/mbc.9.3.585
Gordon, S. L., and Cousin, M. A. (2014). The Sybtraps: control of synaptobrevin traffic by synaptophysin, α-synuclein and AP-180. Traffic 15, 245–254. doi: 10.1111/tra.12140
Gordon, S. L., and Cousin, M. A. (2016). The iTRAPs: guardians of synaptic vesicle cargo retrieval during endocytosis. Front. Synaptic Neurosci. 8:1. doi: 10.3389/fnsyn.2016.00001
Gordon, S. L., Harper, C. B., Smillie, K. J., and Cousin, M. A. (2016). A fine balance of synaptophysin levels underlies efficient retrieval of synaptobrevin II to synaptic vesicles. PLoS One 11:e0149457. doi: 10.1371/journal.pone.0149457
Gordon, S. L., Leube, R. E., and Cousin, M. A. (2011). Synaptophysin is required for synaptobrevin retrieval during synaptic vesicle endocytosis. J. Neurosci. 31, 14032–14036. doi: 10.1523/JNEUROSCI.3162-11.2011
Gracheva, E. O., Burdina, A. O., Holgado, A. M., Berthelot-Grosjean, M., Ackley, B. D., Hadwiger, G., et al. (2006). Tomosyn inhibits synaptic vesicle priming in Caenorhabditis elegans. PLoS Biol. 4:e261. doi: 10.1371/journal.pbio.0040261
Gracheva, E. O., Hadwiger, G., Nonet, M. L., and Richmond, J. E. (2008). Direct interactions between C. elegans RAB-3 and Rim provide a mechanism to target vesicles to the presynaptic density. Neurosci. Lett. 444, 137–142. doi: 10.1016/j.neulet.2008.08.026
Gracheva, E. O., Maryon, E. B., Berthelot-Grosjean, M., and Richmond, J. E. (2010). Differential regulation of synaptic vesicle tethering and docking by UNC-18 and TOM-1. Front. Syn. Neurosci. 2:141. doi: 10.3389/fnsyn.2010.00141
Graf, E. R., Daniels, R. W., Burgess, R. W., Schwarz, T. L., and DiAntonio, A. (2009). Rab3 dynamically controls protein composition at active zones. Neuron 64, 663–677. doi: 10.1016/j.neuron.2009.11.002
Graf, E. R., Valakh, V., Wright, C. M., Wu, C., Liu, Z., Zhang, Y. Q., et al. (2012). RIM promotes calcium channel accumulation at active zones of the Drosophila neuromuscular junction. J. Neurosci. 32, 16586–16596. doi: 10.1523/JNEUROSCI.0965-12.2012
Griff, I. C., Schekman, R., Rothman, J. E., and Kaiser, C. A. (1992). The yeast SEC17 gene product is functionally equivalent to mammalian alpha-SNAP protein. J. Biol. Chem. 267, 12106–12115. doi: 10.1016/s0021-9258(19)49812-8
Grosshans, B. L., Ortiz, D., and Novick, P. (2006). Rabs and their effectors: achieving specificity in membrane traffic. Proc. Natl. Acad. Sci. U.S.A. 103, 11821–11827. doi: 10.1073/pnas.0601617103
Gu, M., Liu, Q., Watanabe, S., Sun, L., Hollopeter, G., Grant, B. D., et al. (2013). AP2 hemicomplexes contribute independently to synaptic vesicle endocytosis. eLife 2:e00190. doi: 10.7554/eLife.00190
Gu, M., Schuske, K., Watanabe, S., Liu, Q., Baum, P., Garriga, G., et al. (2008). Mu2 adaptin facilitates but is not essential for synaptic vesicle recycling in Caenorhabditis elegans. J. Cell Biol. 183, 881–892. doi: 10.1083/jcb.200806088
Guan, Z., Bykhovskaia, M., Jorquera, R. A., Sutton, R. B., Akbergenova, Y., and Littleton, J. T. (2017). A synaptotagmin suppressor screen indicates SNARE binding controls the timing and Ca2+ cooperativity of vesicle fusion. eLife 6:e28409. doi: 10.7554/eLife.28409
Guan, Z., Quiñones-Frías, M. C., Akbergenova, Y., and Littleton, J. T. (2020). Drosophila synaptotagmin 7 negatively regulates synaptic vesicle release and replenishment in a dosage-dependent manner. eLife 9:e55443. doi: 10.7554/eLife.55443
Guan, Z., Saraswati, S., Adolfsen, B., and Littleton, J. T. (2005). Genome-wide transcriptional changes associated with enhanced activity in the Drosophila nervous system. Neuron 48, 91–107. doi: 10.1016/j.neuron.2005.08.036
Hallermann, S., Kittel, R. J., Wichmann, C., Weyhersmüller, A., Fouquet, W., Mertel, S., et al. (2010). Naked dense bodies provoke depression. J. Neurosci. 30, 14340–14345. doi: 10.1523/JNEUROSCI.2495-10.2010
Han, Y., Kaeser, P. S., Südhof, T. C., and Schneggenburger, R. (2011). RIM determines Ca2+ channel density and vesicle docking at the presynaptic active zone. Neuron 69, 304–316. doi: 10.1016/j.neuron.2010.12.014
Harris, K. P., and Littleton, J. T. (2015). Transmission, development, and plasticity of synapses. Genetics 201, 345–375. doi: 10.1534/genetics.115.176529
Harrison, S. D., Broadie, K., van de Goor, J., and Rubin, G. M. (1994). Mutations in the Drosophila Rop gene suggest a function in general secretion and synaptic transmission. Neuron 13, 555–566. doi: 10.1016/0896-6273(94)90025-6
Hata, Y., Slaughter, C. A., and Südhof, T. C. (1993). Synaptic vesicle fusion complex contains unc-18 homologue bound to syntaxin. Nature 366, 347–351. doi: 10.1038/366347a0
Hatsuzawa, K., Lang, T., Fasshauer, D., Bruns, D., and Jahn, R. (2003). The R-SNARE motif of tomosyn forms SNARE core complexes with syntaxin 1 and SNAP-25 and down-regulates exocytosis. J. Biol. Chem. 278, 31159–31166. doi: 10.1074/jbc.M305500200
Hattendorf, D. A., Andreeva, A., Gangar, A., Brennwald, P. J., and Weis, W. I. (2007). Structure of the yeast polarity protein Sro7 reveals a SNARE regulatory mechanism. Nature 446, 567–571. doi: 10.1038/nature05635
Haucke, V., Neher, E., and Sigrist, S. J. (2011). Protein scaffolds in the coupling of synaptic exocytosis and endocytosis. Nat. Rev. Neurosci. 12, 127–138. doi: 10.1038/nrn2948
Haucke, V., Wenk, M. R., Chapman, E. R., Farsad, K., and De Camilli, P. (2000). Dual interaction of synaptotagmin with mu2- and alpha-adaptin facilitates clathrin-coated pit nucleation. EMBO J. 19, 6011–6019. doi: 10.1093/emboj/19.22.6011
Hayashi, T., McMahon, H., Yamasaki, S., Binz, T., Hata, Y., Südhof, T. C., et al. (1994). Synaptic vesicle membrane fusion complex: action of clostridial neurotoxins on assembly. EMBO J. 13, 5051–5061. doi: 10.1002/j.1460-2075.1994.tb06834.x
Heerssen, H., Fetter, R. D., and Davis, G. W. (2008). Clathrin dependence of synaptic-vesicle formation at the Drosophila neuromuscular junction. Curr. Biol. 18, 401–409. doi: 10.1016/j.cub.2008.02.055
Henkel, A. W., and Almers, W. (1996). Fast steps in exocytosis and endocytosis studied by capacitance measurements in endocrine cells. Curr. Opin. Neurobiol. 6, 350–357. doi: 10.1016/s0959-4388(96)80119-x
Hernandez, J. M., Stein, A., Behrmann, E., Riedel, D., Cypionka, A., Farsi, Z., et al. (2012). Membrane fusion intermediates via directional and full assembly of the SNARE complex. Science 336, 1581–1584. doi: 10.1126/science.1221976
Heuser, J. E., and Reese, T. S. (1973). Evidence for recycling of synaptic vesicle membrane during transmitter release at the frog neuromuscular junction. J. Cell Biol. 57, 315–344. doi: 10.1083/jcb.57.2.315
Hinshaw, J. E., and Schmid, S. L. (1995). Dynamin self-assembles into rings suggesting a mechanism for coated vesicle budding. Nature 374, 190–192. doi: 10.1038/374190a0
Holderith, N., Lorincz, A., Katona, G., Rózsa, B., Kulik, A., Watanabe, M., et al. (2012). Release probability of hippocampal glutamatergic terminals scales with the size of the active zone. Nat. Neurosci. 15, 988–997. doi: 10.1038/nn.3137
Hosono, R., Hekimi, S., Kamiya, Y., Sassa, T., Murakami, S., Nishiwaki, K., et al. (1992). The unc-18 gene encodes a novel protein affecting the kinetics of acetylcholine metabolism in the nematode Caenorhabditis elegans. J. Neurochem. 58, 1517–1525. doi: 10.1111/j.1471-4159.1992.tb11373.x
Hu, Z., Tong, X.-J., and Kaplan, J. M. (2013). UNC-13L, UNC-13S, and Tomosyn form a protein code for fast and slow neurotransmitter release in Caenorhabditis elegans. eLife 2:e00967. doi: 10.7554/eLife.00967
Hua, Y., and Scheller, R. H. (2001). Three SNARE complexes cooperate to mediate membrane fusion. Proc. Natl. Acad. Sci. U.S.A. 98, 8065–8070. doi: 10.1073/pnas.131214798
Huang, X., Sun, S., Wang, X., Fan, F., Zhou, Q., Lu, S., et al. (2019). Mechanistic insights into the SNARE complex disassembly. Sci. Adv. 5:eaau8164. doi: 10.1126/sciadv.aau8164
Huntwork, S., and Littleton, J. T. (2007). A complexin fusion clamp regulates spontaneous neurotransmitter release and synaptic growth. Nat. Neurosci. 10, 1235–1237. doi: 10.1038/nn1980
Inoue, H., Matsuzaki, Y., Tanaka, A., Hosoi, K., Ichimura, K., Arasaki, K., et al. (2015). γ-SNAP stimulates disassembly of endosomal SNARE complexes and regulates endocytic trafficking pathways. J. Cell Sci. 128, 2781–2794. doi: 10.1242/jcs.158634
Iyer, J., Wahlmark, C. J., Kuser-Ahnert, G. A., and Kawasaki, F. (2013). Molecular mechanisms of COMPLEXIN fusion clamp function in synaptic exocytosis revealed in a new Drosophila mutant. Mol. Cell. Neurosci. 56, 244–254. doi: 10.1016/j.mcn.2013.06.002
Jahn, R., and Scheller, R. H. (2006). SNAREs-engines for membrane fusion. Nat. Rev. Mol. Cell Biol. 7, 631–643. doi: 10.1038/nrm2002
Jahn, R., and Südhof, T. C. (1999). Membrane fusion and exocytosis. Annu. Rev. Biochem. 68, 863–911. doi: 10.1146/annurev.biochem.68.1.863
Jan, L. Y., and Jan, Y. N. (1976). Properties of the larval neuromuscular junction in Drosophila melanogaster. J. Physiol. 262, 189–214. doi: 10.1113/jphysiol.1976.sp011592
Jiao, J., He, M., Port, S. A., Baker, R. W., Xu, Y., Qu, H., et al. (2018). Munc18-1 catalyzes neuronal SNARE assembly by templating SNARE association. eLife 7:e41771. doi: 10.7554/eLife.41771
Jorquera, R. A., Huntwork-Rodriguez, S., Akbergenova, Y., Cho, R. W., and Littleton, J. T. (2012). Complexin controls spontaneous and evoked neurotransmitter release by regulating the timing and properties of synaptotagmin activity. J. Neurosci. 32, 18234–18245. doi: 10.1523/JNEUROSCI.3212-12.2012
Kaempf, N., Kochlamazashvili, G., Puchkov, D., Maritzen, T., Bajjalieh, S. M., Kononenko, N. L., et al. (2015). Overlapping functions of stonin 2 and SV2 in sorting of the calcium sensor synaptotagmin 1 to synaptic vesicles. Proc. Natl. Acad. Sci. U.S.A. 112, 7297–7302. doi: 10.1073/pnas.1501627112
Kaeser, P. S., Deng, L., Wang, Y., Dulubova, I., Liu, X., Rizo, J., et al. (2011). RIM proteins tether Ca2+ channels to presynaptic active zones via a direct PDZ-domain interaction. Cell 144, 282–295. doi: 10.1016/j.cell.2010.12.029
Kaeser, P. S., Kwon, H.-B., Chiu, C. Q., Deng, L., Castillo, P. E., and Südhof, T. C. (2008). RIM1alpha and RIM1beta are synthesized from distinct promoters of the RIM1 gene to mediate differential but overlapping synaptic functions. J. Neurosci. 28, 13435–13447. doi: 10.1523/JNEUROSCI.3235-08.2008
Kaeser-Woo, Y. J., Yang, X., and Südhof, T. C. (2012). C-terminal complexin sequence is selectively required for clamping and priming but not for Ca2+ triggering of synaptic exocytosis. J. Neurosci. 32, 2877–2885. doi: 10.1523/JNEUROSCI.3360-11.2012
Kanda, H., Tamori, Y., Shinoda, H., Yoshikawa, M., Sakaue, M., Udagawa, J., et al. (2005). Adipocytes from Munc18c-null mice show increased sensitivity to insulin-stimulated GLUT4 externalization. J. Clin. Invest. 115, 291–301. doi: 10.1172/JCI22681
Karlocai, M. R., Heredi, J., Benedek, T., Holderith, N., Lorincz, A., and Nusser, Z. (2021). Variability in the Munc13-1 content of excitatory release site. eLife 10:e67468. doi: 10.7554/eLife.67468
Katz, B. S. (1969). The Release of Neural Transmitter Substances (Sherrington Lecture): Bernard S. Katz: 9780853230601: Amazon.com: Books. Liverpool: Liverpool University Press.
Kawasaki, F., Hazen, M., and Ordway, R. W. (2000). Fast synaptic fatigue in shibire mutants reveals a rapid requirement for dynamin in synaptic vesicle membrane trafficking. Nat. Neurosci. 3, 859–860. doi: 10.1038/78753
Kawasaki, F., Mattiuz, A. M., and Ordway, R. W. (1998). Synaptic physiology and ultrastructure in comatose mutants define an in vivo role for NSF in neurotransmitter release. J. Neurosci. 18, 10241–10249. doi: 10.1523/jneurosci.18-24-10241.1998
Kawasaki, F., and Ordway, R. W. (1999). The Drosophila NSF protein, dNSF1, plays a similar role at neuromuscular and some central synapses. J. Neurophysiol. 82, 123–130. doi: 10.1152/jn.1999.82.1.123
Khvotchev, M., Dulubova, I., Sun, J., Dai, H., Rizo, J., and Südhof, T. C. (2007). Dual modes of Munc18-1/SNARE interactions are coupled by functionally critical binding to syntaxin-1 N terminus. J. Neurosci. 27, 12147–12155. doi: 10.1523/JNEUROSCI.3655-07.2007
Kim, K., Petrova, Y. M., Scott, B. L., Nigam, R., Agrawal, A., Evans, C. M., et al. (2012). Munc18b is an essential gene in mice whose expression is limiting for secretion by airway epithelial and mast cells. Biochem. J. 446, 383–394. doi: 10.1042/BJ20120057
Kittel, R. J., Wichmann, C., Rasse, T. M., Fouquet, W., Schmidt, M., Schmid, A., et al. (2006). Bruchpilot promotes active zone assembly, Ca2+ channel clustering, and vesicle release. Science 312, 1051–1054. doi: 10.1126/science.1126308
Koenig, J. H., and Ikeda, K. (1989). Disappearance and reformation of synaptic vesicle membrane upon transmitter release observed under reversible blockage of membrane retrieval. J. Neurosci. 9, 3844–3860. doi: 10.1523/jneurosci.09-11-03844.1989
Koester, H. J., and Johnston, D. (2005). Target cell-dependent normalization of transmitter release at neocortical synapses. Science 308, 863–866. doi: 10.1126/science.1100815
Koushika, S. P., Richmond, J. E., Hadwiger, G., Weimer, R. M., Jorgensen, E. M., and Nonet, M. L. (2001). A post-docking role for active zone protein Rim. Nat. Neurosci. 4, 997–1005. doi: 10.1038/nn732
Krishnakumar, S. S., Radoff, D. T., Kümmel, D., Giraudo, C. G., Li, F., Khandan, L., et al. (2011). A conformational switch in complexin is required for synaptotagmin to trigger synaptic fusion. Nat. Struct. Mol. Biol. 18, 934–940. doi: 10.1038/nsmb.2103
Kümmel, D., Krishnakumar, S. S., Radoff, D. T., Li, F., Giraudo, C. G., Pincet, F., et al. (2011). Complexin cross-links prefusion SNAREs into a zigzag array. Nat. Struct. Mol. Biol. 18, 927–933. doi: 10.1038/nsmb.2101
Kushibiki, Y., Suzuki, T., Jin, Y., and Taru, H. (2019). RIMB-1/RIM-binding protein and UNC-10/RIM redundantly regulate presynaptic localization of the voltage-gated calcium channel in Caenorhabditis elegans. J. Neurosci. 39, 8617–8631. doi: 10.1523/JNEUROSCI.0506-19.2019
Kutay, U., Hartmann, E., and Rapoport, T. A. (1993). A class of membrane proteins with a C-terminal anchor. Trends Cell Biol. 3, 72–75. doi: 10.1016/0962-8924(93)90066-a
Lagow, R. D., Bao, H., Cohen, E. N., Daniels, R. W., Zuzek, A., Williams, W. H., et al. (2007). Modification of a hydrophobic layer by a point mutation in syntaxin 1A regulates the rate of synaptic vesicle fusion. PLoS Biol. 5:e72. doi: 10.1371/journal.pbio.0050072
Lao, G., Scheuss, V., Gerwin, C. M., Su, Q., Mochida, S., Rettig, J., et al. (2000). Syntaphilin: a syntaxin-1 clamp that controls SNARE assembly. Neuron 25, 191–201. doi: 10.1016/s0896-6273(00)80882-x
Lee, J., Guan, Z., Akbergenova, Y., and Littleton, J. T. (2013). Genetic analysis of synaptotagmin C2 domain specificity in regulating spontaneous and evoked neurotransmitter release. J. Neurosci. 33, 187–200. doi: 10.1523/JNEUROSCI.3214-12.2013
Lee, J., and Littleton, J. T. (2015). Transmembrane tethering of synaptotagmin to synaptic vesicles controls multiple modes of neurotransmitter release. Proc. Natl. Acad. Sci. U.S.A. 112, 3793–3798. doi: 10.1073/pnas.1420312112
Lehman, K., Rossi, G., Adamo, J. E., and Brennwald, P. (1999). Yeast homologues of tomosyn and lethal giant larvae function in exocytosis and are associated with the plasma membrane SNARE, Sec9. J. Cell Biol. 146, 125–140. doi: 10.1083/jcb.146.1.125
Li, B., Li, Y., Liu, F., Tan, X., Rui, Q., Tong, Y., et al. (2019). Overexpressed tomosyn binds syntaxins and blocks secretion during pollen development. Plant Physiol. 181, 1114–1126. doi: 10.1104/pp.19.00965
Li, L., Liu, H., Wang, W., Chandra, M., Collins, B. M., and Hu, Z. (2018). SNT-1 functions as the Ca2+ sensor for tonic and evoked neurotransmitter release in Caenorhabditis elegans. J. Neurosci. 38, 5313–5324. doi: 10.1523/JNEUROSCI.3097-17.2018
Li, W., Ma, C., Guan, R., Xu, Y., Tomchick, D. R., and Rizo, J. (2011). The crystal structure of a Munc13 C-terminal module exhibits a remarkable similarity to vesicle tethering factors. Structure 19, 1443–1455. doi: 10.1016/j.str.2011.07.012
Li, Y., Wang, S., Li, T., Zhu, L., and Ma, C. (2018). Tomosyn guides SNARE complex formation in coordination with Munc18 and Munc13. FEBS Lett. 592, 1161–1172. doi: 10.1002/1873-3468.13018
Lin, P.-Y., Chanaday, N. L., Horvath, P. M., Ramirez, D. M. O., Monteggia, L. M., and Kavalali, E. T. (2020). VAMP4 maintains a Ca2+-sensitive pool of spontaneously recycling synaptic vesicles. J. Neurosci. 40, 5389–5401. doi: 10.1523/JNEUROSCI.2386-19.2020
Lipstein, N., Verhoeven-Duif, N. M., Michelassi, F. E., Calloway, N., van Hasselt, P. M., Pienkowska, K., et al. (2017). Synaptic UNC13A protein variant causes increased neurotransmission and dyskinetic movement disorder. J. Clin. Invest. 127, 1005–1018. doi: 10.1172/JCI90259
Littleton, J. T. (2000). A genomic analysis of membrane trafficking and neurotransmitter release in Drosophila. J. Cell Biol. 150, F77–F82. doi: 10.1083/jcb.150.2.f77
Littleton, J. T., Bai, J., Vyas, B., Desai, R., Baltus, A. E., Garment, M. B., et al. (2001a). synaptotagmin mutants reveal essential functions for the C2B domain in Ca2+-triggered fusion and recycling of synaptic vesicles in vivo. J. Neurosci. 21, 1421–1433. doi: 10.1523/jneurosci.21-05-01421.2001
Littleton, J. T., Barnard, R. J., Titus, S. A., Slind, J., Chapman, E. R., and Ganetzky, B. (2001b). SNARE-complex disassembly by NSF follows synaptic-vesicle fusion. Proc. Natl. Acad. Sci. U.S.A. 98, 12233–12238. doi: 10.1073/pnas.221450198
Littleton, J. T., and Bellen, H. J. (1995). Presynaptic proteins involved in exocytosis in Drosophila melanogaster: a genetic analysis. Invert. Neurosci. 1, 3–13. doi: 10.1007/bf02331827
Littleton, J. T., Chapman, E. R., Kreber, R., Garment, M. B., Carlson, S. D., and Ganetzky, B. (1998). Temperature-sensitive paralytic mutations demonstrate that synaptic exocytosis requires SNARE complex assembly and disassembly. Neuron 21, 401–413. doi: 10.1016/s0896-6273(00)80549-8
Littleton, J. T., Stern, M., Perin, M., and Bellen, H. J. (1994). Calcium dependence of neurotransmitter release and rate of spontaneous vesicle fusions are altered in Drosophila synaptotagmin mutants. Proc. Natl. Acad. Sci. U.S.A. 91, 10888–10892. doi: 10.1073/pnas.91.23.10888
Littleton, J. T., Stern, M., Schulze, K., Perin, M., and Bellen, H. J. (1993). Mutational analysis of Drosophila synaptotagmin demonstrates its essential role in Ca(2+)-activated neurotransmitter release. Cell 74, 1125–1134. doi: 10.1016/0092-8674(93)90733-7
Liu, H., Li, L., Nedelcu, D., Hall, Q., Zhou, L., Wang, W., et al. (2019). Heterodimerization of UNC-13/RIM regulates synaptic vesicle release probability but not priming in C. elegans. eLife 8:e40585. doi: 10.7554/eLife.40585
Liu, H., Li, L., Wang, W., Gong, J., Yang, X., and Hu, Z. (2018). Spontaneous vesicle fusion is differentially regulated at cholinergic and gabaergic synapses. Cell Rep. 22, 2334–2345. doi: 10.1016/j.celrep.2018.02.023
Liu, X., Seven, A. B., Camacho, M., Esser, V., Xu, J., Trimbuch, T., et al. (2016). Functional synergy between the Munc13 C-terminal C1 and C2 domains. eLife 5:e13696. doi: 10.7554/eLife.13696
Lloyd, T. E., Verstreken, P., Ostrin, E. J., Phillippi, A., Lichtarge, O., and Bellen, H. J. (2000). A genome-wide search for synaptic vesicle cycle proteins in Drosophila. Neuron 26, 45–50. doi: 10.1016/s0896-6273(00)81136-8
López-Murcia, F. J., Reim, K., Jahn, O., Taschenberger, H., and Brose, N. (2019). Acute complexin knockout abates spontaneous and evoked transmitter release. Cell Rep. 26, 2521.e5–2530.e5. doi: 10.1016/j.celrep.2019.02.030
Ma, C., Li, W., Xu, Y., and Rizo, J. (2011). Munc13 mediates the transition from the closed syntaxin-Munc18 complex to the SNARE complex. Nat. Struct. Mol. Biol. 18, 542–549. doi: 10.1038/nsmb.2047
Ma, C., Su, L., Seven, A. B., Xu, Y., and Rizo, J. (2013). Reconstitution of the vital functions of Munc18 and Munc13 in neurotransmitter release. Science 339, 421–425. doi: 10.1126/science.1230473
Ma, L., Rebane, A. A., Yang, G., Xi, Z., Kang, Y., Gao, Y., et al. (2015). Munc18-1-regulated stage-wise SNARE assembly underlying synaptic exocytosis. eLife 4:e09580. doi: 10.7554/eLife.09580
Mace, K. E., Biela, L. M., Sares, A. G., and Reist, N. E. (2009). Synaptotagmin I stabilizes synaptic vesicles via its C2A polylysine motif. Genesis 47, 337–345. doi: 10.1002/dvg.20502
Mackler, J. M., Drummond, J. A., Loewen, C. A., Robinson, I. M., and Reist, N. E. (2002). The C(2)B Ca(2+)-binding motif of synaptotagmin is required for synaptic transmission in vivo. Nature 418, 340–344. doi: 10.1038/nature00846
Mahoney, R. E., Azpurua, J., and Eaton, B. A. (2016). Insulin signaling controls neurotransmission via the 4eBP-dependent modification of the exocytotic machinery. eLife 5:e16807. doi: 10.7554/eLife.16807
Malsam, J., Bärfuss, S., Trimbuch, T., Zarebidaki, F., Sonnen, A. F.-P., Wild, K., et al. (2020). Complexin suppresses spontaneous exocytosis by capturing the membrane-proximal regions of VAMP2 and SNAP25. Cell Rep. 32:107926. doi: 10.1016/j.celrep.2020.107926
Malsam, J., Parisotto, D., Bharat, T. A. M., Scheutzow, A., Krause, J. M., Briggs, J. A. G., et al. (2012). Complexin arrests a pool of docked vesicles for fast Ca2+-dependent release. EMBO J. 31, 3270–3281. doi: 10.1038/emboj.2012.164
Maritzen, T., and Haucke, V. (2018). Coupling of exocytosis and endocytosis at the presynaptic active zone. Neurosci. Res. 127, 45–52. doi: 10.1016/j.neures.2017.09.013
Martin, J. A., Hu, Z., Fenz, K. M., Fernandez, J., and Dittman, J. S. (2011). Complexin has opposite effects on two modes of synaptic vesicle fusion. Curr. Biol. 21, 97–105. doi: 10.1016/j.cub.2010.12.014
Martina, J. A., Bonangelino, C. J., Aguilar, R. C., and Bonifacino, J. S. (2001). Stonin 2: an adaptor-like protein that interacts with components of the endocytic machinery. J. Cell Biol. 153, 1111–1120. doi: 10.1083/jcb.153.5.1111
Marz, K. E., Lauer, J. M., and Hanson, P. I. (2003). Defining the SNARE complex binding surface of alpha-SNAP: implications for SNARE complex disassembly. J. Biol. Chem. 278, 27000–27008. doi: 10.1074/jbc.M302003200
Masuda, E. S., Huang, B. C., Fisher, J. M., Luo, Y., and Scheller, R. H. (1998). Tomosyn binds t-SNARE proteins via a VAMP-like coiled coil. Neuron 21, 479–480. doi: 10.1016/s0896-6273(00)80559-0
McEwen, J. M., and Kaplan, J. M. (2008). UNC-18 promotes both the anterograde trafficking and synaptic function of syntaxin. Mol. Biol. Cell 19, 3836–3846. doi: 10.1091/mbc.E08-02-0160
McEwen, J. M., Madison, J. M., Dybbs, M., and Kaplan, J. M. (2006). Antagonistic regulation of synaptic vesicle priming by Tomosyn and UNC-13. Neuron 51, 303–315. doi: 10.1016/j.neuron.2006.06.025
McMahon, H. T., Missler, M., Li, C., and Südhof, T. C. (1995). Complexins: cytosolic proteins that regulate SNAP receptor function. Cell 83, 111–119. doi: 10.1016/0092-8674(95)90239-2
McNew, J. A., Parlati, F., Fukuda, R., Johnston, R. J., Paz, K., Paumet, F., et al. (2000a). Compartmental specificity of cellular membrane fusion encoded in SNARE proteins. Nature 407, 153–159. doi: 10.1038/35025000
McNew, J. A., Weber, T., Parlati, F., Johnston, R. J., Melia, T. J., Söllner, T. H., et al. (2000b). Close is not enough: SNARE-dependent membrane fusion requires an active mechanism that transduces force to membrane anchors. J. Cell Biol. 150, 105–117. doi: 10.1083/jcb.150.1.105
Medine, C. N., Rickman, C., Chamberlain, L. H., and Duncan, R. R. (2007). Munc18-1 prevents the formation of ectopic SNARE complexes in living cells. J. Cell Sci. 120, 4407–4415. doi: 10.1242/jcs.020230
Meinrenken, C. J., Borst, J. G. G., and Sakmann, B. (2002). Calcium secretion coupling at calyx of Held governed by nonuniform channel-vesicle topography. J. Neurosci. 22, 1648–1667. doi: 10.1523/jneurosci.22-05-01648.2002
Melland, H., Carr, E. M., and Gordon, S. L. (2021). Disorders of synaptic vesicle fusion machinery. J. Neurochem. 157, 130–164. doi: 10.1111/jnc.15181
Melom, J. E., Akbergenova, Y., Gavornik, J. P., and Littleton, J. T. (2013). Spontaneous and evoked release are independently regulated at individual active zones. J. Neurosci. 33, 17253–17263. doi: 10.1523/JNEUROSCI.3334-13.2013
Melom, J. E., and Littleton, J. T. (2011). Synapse development in health and disease. Curr. Opin. Genet. Dev. 21, 256–261. doi: 10.1016/j.gde.2011.01.002
Miller, K. G., Alfonso, A., Nguyen, M., Crowell, J. A., Johnson, C. D., and Rand, J. B. (1996). A genetic selection for Caenorhabditis elegans synaptic transmission mutants. Proc. Natl. Acad. Sci. U.S.A. 93, 12593–12598. doi: 10.1073/pnas.93.22.12593
Milovanovic, D., and Jahn, R. (2015). Organization and dynamics of SNARE proteins in the presynaptic membrane. Front. Physiol. 6:89. doi: 10.3389/fphys.2015.00089
Mullen, G. P., Grundahl, K. M., Gu, M., Watanabe, S., Hobson, R. J., Crowell, J. A., et al. (2012). UNC-41/stonin functions with AP2 to recycle synaptic vesicles in Caenorhabditis elegans. PLoS One 7:e40095. doi: 10.1371/journal.pone.0040095
Neher, E., and Brose, N. (2018). Dynamically primed synaptic vesicle states: key to understand synaptic short-term plasticity. Neuron 100, 1283–1291. doi: 10.1016/j.neuron.2018.11.024
Nguyen, M., Alfonso, A., Johnson, C. D., and Rand, J. B. (1995). Caenorhabditis elegans mutants resistant to inhibitors of acetylcholinesterase. Genetics 140, 527–535. doi: 10.1093/genetics/140.2.527
Nonet, M. L., Holgado, A. M., Brewer, F., Serpe, C. J., Norbeck, B. A., Holleran, J., et al. (1999). UNC-11, a Caenorhabditis elegans AP180 homologue, regulates the size and protein composition of synaptic vesicles. Mol. Biol. Cell 10, 2343–2360. doi: 10.1091/mbc.10.7.2343
Nonet, M. L., Saifee, O., Zhao, H., Rand, J. B., and Wei, L. (1998). Synaptic transmission deficits in Caenorhabditis elegans synaptobrevin mutants. J. Neurosci. 18, 70–80.
Nonet, M. L., Staunton, J. E., Kilgard, M. P., Fergestad, T., Hartwieg, E., Horvitz, H. R., et al. (1997). Caenorhabditis elegans rab-3 mutant synapses exhibit impaired function and are partially depleted of vesicles. J. Neurosci. 17, 8061–8073. doi: 10.1523/jneurosci.17-21-08061.1997
Novick, P., Field, C., and Schekman, R. (1980). Identification of 23 complementation groups required for post-translational events in the yeast secretory pathway. Cell 21, 205–215. doi: 10.1016/0092-8674(80)90128-2
Nunes, P., Haines, N., Kuppuswamy, V., Fleet, D. J., and Stewart, B. A. (2006). Synaptic vesicle mobility and presynaptic F-actin are disrupted in a N-ethylmaleimide-sensitive factor allele of Drosophila. Mol. Biol. Cell 17, 4709–4719. doi: 10.1091/mbc.E06-03-0253
Ogawa, H., Harada, S., Sassa, T., Yamamoto, H., and Hosono, R. (1998). Functional properties of the unc-64 gene encoding a Caenorhabditis elegans syntaxin. J. Biol. Chem. 273, 2192–2198. doi: 10.1074/jbc.273.4.2192
Oh, K. H., Krout, M. D., Richmond, J. E., and Kim, H. (2021). UNC-2 CaV2 channel localization at presynaptic active zones depends on UNC-10/RIM and SYD-2/Liprin-α in Caenorhabditis elegans. J. Neurosci. 41, 4782–4794. doi: 10.1523/JNEUROSCI.0076-21.2021
Ordway, R. W., Pallanck, L., and Ganetzky, B. (1994). Neurally expressed Drosophila genes encoding homologs of the NSF and SNAP secretory proteins. Proc. Natl. Acad. Sci. U.S.A. 91, 5715–5719. doi: 10.1073/pnas.91.12.5715
Ortega, J. M., and Genç, Ö, and Davis, G. W. (2018). Molecular mechanisms that stabilize short term synaptic plasticity during presynaptic homeostatic plasticity. eLife 7:e40385. doi: 10.7554/eLife.40385
Oyler, G. A., Higgins, G. A., Hart, R. A., Battenberg, E., Billingsley, M., Bloom, F. E., et al. (1989). The identification of a novel synaptosomal-associated protein, SNAP-25, differentially expressed by neuronal subpopulations. J. Cell Biol. 109, 3039–3052. doi: 10.1083/jcb.109.6.3039
Pabst, S., Hazzard, J. W., Antonin, W., Südhof, T. C., Jahn, R., Rizo, J., et al. (2000). Selective interaction of complexin with the neuronal SNARE complex. Determination of the binding regions. J. Biol. Chem. 275, 19808–19818. doi: 10.1074/jbc.M002571200
Paddock, B. E., Striegel, A. R., Hui, E., Chapman, E. R., and Reist, N. E. (2008). Ca2+-dependent, phospholipid-binding residues of synaptotagmin are critical for excitation-secretion coupling in vivo. J. Neurosci. 28, 7458–7466. doi: 10.1523/JNEUROSCI.0197-08.2008
Paddock, B. E., Wang, Z., Biela, L. M., Chen, K., Getzy, M. D., Striegel, A., et al. (2011). Membrane penetration by synaptotagmin is required for coupling calcium binding to vesicle fusion in vivo. J. Neurosci. 31, 2248–2257. doi: 10.1523/JNEUROSCI.3153-09.2011
Pallanck, L., Ordway, R. W., and Ganetzky, B. (1995a). A Drosophila NSF mutant. Nature 376:25. doi: 10.1038/376025a0
Pallanck, L., Ordway, R. W., Ramaswami, M., Chi, W. Y., Krishnan, K. S., and Ganetzky, B. (1995b). Distinct roles for N-ethylmaleimide-sensitive fusion protein (NSF) suggested by the identification of a second Drosophila NSF homolog. J. Biol. Chem. 270, 18742–18744. doi: 10.1074/jbc.270.32.18742
Peled, E. S., and Isacoff, E. Y. (2011). Optical quantal analysis of synaptic transmission in wild-type and rab3-mutant Drosophila motor axons. Nat. Neurosci. 14, 519–526. doi: 10.1038/nn.2767
Pennuto, M., Bonanomi, D., Benfenati, F., and Valtorta, F. (2003). Synaptophysin I controls the targeting of VAMP2/synaptobrevin II to synaptic vesicles. Mol. Biol. Cell 14, 4909–4919. doi: 10.1091/mbc.E03-06-0380
Pereira-Leal, J. B., Hume, A. N., and Seabra, M. C. (2001). Prenylation of Rab GTPases: molecular mechanisms and involvement in genetic disease. FEBS Lett. 498, 197–200. doi: 10.1016/s0014-5793(01)02483-8
Peter, F., Wong, S. H., Subramaniam, V. N., Tang, B. L., and Hong, W. (1998). Alpha-SNAP but not gamma-SNAP is required for ER-Golgi transport after vesicle budding and the Rab1-requiring step but before the EGTA-sensitive step. J. Cell Sci. 111(Pt 17), 2625–2633. doi: 10.1242/jcs.111.17.2625
Pevsner, J., Hsu, S. C., Braun, J. E., Calakos, N., Ting, A. E., Bennett, M. K., et al. (1994). Specificity and regulation of a synaptic vesicle docking complex. Neuron 13, 353–361. doi: 10.1016/0896-6273(94)90352-2
Pobbati, A. V., Razeto, A., Böddener, M., Becker, S., and Fasshauer, D. (2004). Structural basis for the inhibitory role of tomosyn in exocytosis. J. Biol. Chem. 279, 47192–47200. doi: 10.1074/jbc.M408767200
Pobbati, A. V., Stein, A., and Fasshauer, D. (2006). N- to C-terminal SNARE complex assembly promotes rapid membrane fusion. Science 313, 673–676. doi: 10.1126/science.1129486
Poodry, C. A., Hall, L., and Suzuki, D. T. (1973). Developmental properties of Shibire: a pleiotropic mutation affecting larval and adult locomotion and development. Dev. Biol. 32, 373–386. doi: 10.1016/0012-1606(73)90248-0
Pooryasin, A., Maglione, M., Schubert, M., Matkovic-Rachid, T., Hasheminasab, S.-M., Pech, U., et al. (2021). Unc13A and Unc13B contribute to the decoding of distinct sensory information in Drosophila. Nat. Commun. 12:1932. doi: 10.1038/s41467-021-22180-6
Prinslow, E. A., Stepien, K. P., Pan, Y.-Z., Xu, J., and Rizo, J. (2019). Multiple factors maintain assembled trans-SNARE complexes in the presence of NSF and αSNAP. eLife 8:e38880. doi: 10.7554/eLife.38880
Quiñones-Frías, M. C., and Littleton, J. T. (2021). Function of Drosophila synaptotagmins in membrane trafficking at synapses. Cell Mol. Life Sci. 78, 4335–4364. doi: 10.1007/s00018-021-03788-9
Ramirez, D. M. O., Khvotchev, M., Trauterman, B., and Kavalali, E. T. (2012). Vti1a identifies a vesicle pool that preferentially recycles at rest and maintains spontaneous neurotransmission. Neuron 73, 121–134. doi: 10.1016/j.neuron.2011.10.034
Rao, S. S., Stewart, B. A., Rivlin, P. K., Vilinsky, I., Watson, B. O., Lang, C., et al. (2001). Two distinct effects on neurotransmission in a temperature-sensitive SNAP-25 mutant. EMBO J. 20, 6761–6771. doi: 10.1093/emboj/20.23.6761
Reddy-Alla, S., Böhme, M. A., Reynolds, E., Beis, C., Grasskamp, A. T., Mampell, M. M., et al. (2017). Stable positioning of unc13 restricts synaptic vesicle fusion to defined release sites to promote synchronous neurotransmission. Neuron 95, 1350.e12–1364.e12. doi: 10.1016/j.neuron.2017.08.016
Redler, S., Strom, T. M., Wieland, T., Cremer, K., Engels, H., Distelmaier, F., et al. (2017). Variants in CPLX1 in two families with autosomal-recessive severe infantile myoclonic epilepsy and ID. Eur. J. Hum. Genet. 25, 889–893. doi: 10.1038/ejhg.2017.52
Reim, K., Mansour, M., Varoqueaux, F., McMahon, H. T., Südhof, T. C., Brose, N., et al. (2001). Complexins regulate a late step in Ca2+-dependent neurotransmitter release. Cell 104, 71–81. doi: 10.1016/s0092-8674(01)00192-1
Reim, K., Regus-Leidig, H., Ammermüller, J., El-Kordi, A., Radyushkin, K., Ehrenreich, H., et al. (2009). Aberrant function and structure of retinal ribbon synapses in the absence of complexin 3 and complexin 4. J. Cell Sci. 122, 1352–1361. doi: 10.1242/jcs.045401
Richmond, J. E., and Broadie, K. S. (2002). The synaptic vesicle cycle: exocytosis and endocytosis in Drosophila and C. elegans. Curr. Opin. Neurobiol. 12, 499–507. doi: 10.1016/S0959-4388(02)00360-4
Richmond, J. E., Davis, W. S., and Jorgensen, E. M. (1999). UNC-13 is required for synaptic vesicle fusion in C. elegans. Nat. Neurosci. 2, 959–964. doi: 10.1038/14755
Richmond, J. E., and Jorgensen, E. M. (1999). One GABA and two acetylcholine receptors function at the C. elegans neuromuscular junction. Nat. Neurosci. 2, 791–797. doi: 10.1038/12160
Richmond, J. E., Weimer, R. M., and Jorgensen, E. M. (2001). An open form of syntaxin bypasses the requirement for UNC-13 in vesicle priming. Nature 412, 338–341. doi: 10.1038/35085583
Rizo, J. (2018). Mechanism of neurotransmitter release coming into focus. Protein Sci. 27, 1364–1391. doi: 10.1002/pro.3445
Rizzoli, S. O., and Jahn, R. (2007). Kiss-and-run, collapse and “readily retrievable” vesicles. Traffic 8, 1137–1144. doi: 10.1111/j.1600-0854.2007.00614.x
Robertson, J. L. (2018). The lipid bilayer membrane and its protein constituents. J. Gen. Physiol. 150, 1472–1483. doi: 10.1085/jgp.201812153
Robinson, S. W., Bourgognon, J.-M., Spiers, J. G., Breda, C., Campesan, S., Butcher, A., et al. (2018). Nitric oxide-mediated posttranslational modifications control neurotransmitter release by modulating complexin farnesylation and enhancing its clamping ability. PLoS Biol. 16:e2003611. doi: 10.1371/journal.pbio.2003611
Rodal, A. A., and Littleton, J. T. (2008). Synaptic endocytosis: illuminating the role of clathrin assembly. Curr. Biol. 18, R259–R261. doi: 10.1016/j.cub.2008.02.014
Rodal, A. A., Motola-Barnes, R. N., and Littleton, J. T. (2008). Nervous wreck and Cdc42 cooperate to regulate endocytic actin assembly during synaptic growth. J. Neurosci. 28, 8316–8325. doi: 10.1523/JNEUROSCI.2304-08.2008
Rothman, J. E. (1994). Mechanisms of intracellular protein transport. Nature 372, 55–63. doi: 10.1038/372055a0
Rowe, J., Calegari, F., Taverna, E., Longhi, R., and Rosa, P. (2001). Syntaxin 1A is delivered to the apical and basolateral domains of epithelial cells: the role of munc-18 proteins. J. Cell Sci. 114, 3323–3332. doi: 10.1242/jcs.114.18.3323
Rowe, J., Corradi, N., Malosio, M. L., Taverna, E., Halban, P., Meldolesi, J., et al. (1999). Blockade of membrane transport and disassembly of the Golgi complex by expression of syntaxin 1A in neurosecretion-incompetent cells: prevention by rbSEC1. J. Cell Sci. 112(Pt 12), 1865–1877. doi: 10.1242/jcs.112.12.1865
Ryan, T. J., and Grant, S. G. N. (2009). The origin and evolution of synapses. Nat. Rev. Neurosci. 10, 701–712. doi: 10.1038/nrn2717
Ryu, J.-K., Min, D., Rah, S.-H., Kim, S. J., Park, Y., Kim, H., et al. (2015). Spring-loaded unraveling of a single SNARE complex by NSF in one round of ATP turnover. Science 347, 1485–1489. doi: 10.1126/science.aaa5267
Sabeva, N., Cho, R. W., Vasin, A., Gonzalez, A., Littleton, J. T., and Bykhovskaia, M. (2017). Complexin mutants reveal partial segregation between recycling pathways that drive evoked and spontaneous neurotransmission. J. Neurosci. 37, 383–396. doi: 10.1523/JNEUROSCI.1854-16.2016
Saifee, O., Wei, L., and Nonet, M. L. (1998). The Caenorhabditis elegans unc-64 locus encodes a syntaxin that interacts genetically with synaptobrevin. Mol. Biol. Cell 9, 1235–1252. doi: 10.1091/mbc.9.6.1235
Sakisaka, T., Yamamoto, Y., Mochida, S., Nakamura, M., Nishikawa, K., Ishizaki, H., et al. (2008). Dual inhibition of SNARE complex formation by tomosyn ensures controlled neurotransmitter release. J. Cell Biol. 183, 323–337. doi: 10.1083/jcb.200805150
Salpietro, V., Malintan, N. T., Llano-Rivas, I., Spaeth, C. G., Efthymiou, S., Striano, P., et al. (2019). Mutations in the neuronal vesicular SNARE VAMP2 affect synaptic membrane fusion and impair human neurodevelopment. Am. J. Hum. Genet. 104, 721–730. doi: 10.1016/j.ajhg.2019.02.016
Sanyal, S., Basole, A., and Krishnan, K. S. (1999). Phenotypic interaction between temperature-sensitive paralytic mutants comatose and paralytic suggests a role for N-ethylmaleimide-sensitive fusion factor in synaptic vesicle cycling in Drosophila. J. Neurosci. 19:RC47.
Sanyal, S., and Krishnan, K. S. (2001). Lethal comatose mutation in Drosophila reveals possible role for NSF in neurogenesis. Neuroreport 12, 1363–1366. doi: 10.1097/00001756-200105250-00015
Sanyal, S., Tolar, L. A., Pallanck, L., and Krishnan, K. S. (2001). Genetic interaction between shibire and comatose mutations in Drosophila suggest a role for snap-receptor complex assembly and disassembly for maintenance of synaptic vesicle cycling. Neurosci. Lett. 311, 21–24. doi: 10.1016/s0304-3940(01)02125-5
Saraswati, S., Adolfsen, B., and Littleton, J. T. (2007). Characterization of the role of the Synaptotagmin family as calcium sensors in facilitation and asynchronous neurotransmitter release. Proc. Natl. Acad. Sci. U.S.A. 104, 14122–14127. doi: 10.1073/pnas.0706711104
Sato, K., Norris, A., Sato, M., and Grant, B. D. (2014). C. elegans as a Model for Membrane Traffic. London: WormBook, 1–47.
Saunders, R. A., Stinson, B. M., Baker, T. A., and Sauer, R. T. (2020). Multistep substrate binding and engagement by the AAA+ ClpXP protease. Proc. Natl. Acad. Sci. U.S.A. 117, 28005–28013. doi: 10.1073/pnas.2010804117
Scales, S. J., Hesser, B. A., Masuda, E. S., and Scheller, R. H. (2002). Amisyn, a novel syntaxin-binding protein that may regulate SNARE complex assembly. J. Biol. Chem. 277, 28271–28279. doi: 10.1074/jbc.M204929200
Schlüter, O. M., Basu, J., Südhof, T. C., and Rosenmund, C. (2006). Rab3 superprimes synaptic vesicles for release: implications for short-term synaptic plasticity. J. Neurosci. 26, 1239–1246. doi: 10.1523/JNEUROSCI.3553-05.2006
Schlüter, O. M., Schmitz, F., Jahn, R., Rosenmund, C., and Südhof, T. C. (2004). A complete genetic analysis of neuronal Rab3 function. J. Neurosci. 24, 6629–6637. doi: 10.1523/JNEUROSCI.1610-04.2004
Schoch, S., Castillo, P. E., Jo, T., Mukherjee, K., Geppert, M., Wang, Y., et al. (2002). RIM1alpha forms a protein scaffold for regulating neurotransmitter release at the active zone. Nature 415, 321–326. doi: 10.1038/415321a
Schoch, S., Deák, F., Königstorfer, A., Mozhayeva, M., Sara, Y., Südhof, T. C., et al. (2001). SNARE function analyzed in synaptobrevin/VAMP knockout mice. Science 294, 1117–1122. doi: 10.1126/science.1064335
Scholz, N., Ehmann, N., Sachidanandan, D., Imig, C., Cooper, B. H., Jahn, O., et al. (2019). Complexin cooperates with Bruchpilot to tether synaptic vesicles to the active zone cytomatrix. J. Cell Biol. 218, 1011–1026. doi: 10.1083/jcb.201806155
Schulze, K. L., and Bellen, H. J. (1996). Drosophila syntaxin is required for cell viability and may function in membrane formation and stabilization. Genetics 144, 1713–1724. doi: 10.1093/genetics/144.4.1713
Schulze, K. L., Broadie, K., Perin, M. S., and Bellen, H. J. (1995). Genetic and electrophysiological studies of Drosophila syntaxin-1A demonstrate its role in nonneuronal secretion and neurotransmission. Cell 80, 311–320. doi: 10.1016/0092-8674(95)90414-x
Schulze, K. L., Littleton, J. T., Salzberg, A., Halachmi, N., Stern, M., Lev, Z., et al. (1994). rop, a Drosophila homolog of yeast Sec1 and vertebrate n-Sec1/Munc-18 proteins, is a negative regulator of neurotransmitter release in vivo. Neuron 13, 1099–1108. doi: 10.1016/0896-6273(94)90048-5
Schwarz, T. L. (1994). Genetic analysis of neurotransmitter release at the synapse. Curr. Opin. Neurobiol. 4, 633–639. doi: 10.1016/0959-4388(94)90003-5
Shi, L., Shen, Q.-T., Kiel, A., Wang, J., Wang, H.-W., Melia, T. J., et al. (2012). SNARE proteins: one to fuse and three to keep the nascent fusion pore open. Science 335, 1355–1359. doi: 10.1126/science.1214984
Shields, M. C., Bowers, M. R., Kramer, H. L., Fulcer, M. M., Perinet, L. C., Metz, M. J., et al. (2020). The role of the C2A domain of synaptotagmin 1 in asynchronous neurotransmitter release. PLoS One 15:e0232991. doi: 10.1371/journal.pone.0232991
Shu, T., Jin, H., Rothman, J. E., and Zhang, Y. (2020). Munc13-1 MUN domain and Munc18-1 cooperatively chaperone SNARE assembly through a tetrameric complex. Proc. Natl. Acad. Sci. U.S.A. 117, 1036–1041. doi: 10.1073/pnas.1914361117
Siddiqi, O., and Benzer, S. (1976). Neurophysiological defects in temperature-sensitive paralytic mutants of Drosophila melanogaster. Proc. Natl. Acad. Sci. U.S.A. 73, 3253–3257. doi: 10.1073/pnas.73.9.3253
Siddiqui, T. J., Vites, O., Stein, A., Heintzmann, R., Jahn, R., and Fasshauer, D. (2007). Determinants of synaptobrevin regulation in membranes. Mol. Biol. Cell 18, 2037–2046. doi: 10.1091/mbc.e07-01-0049
Sieburth, D., Ch’ng, Q., Dybbs, M., Tavazoie, M., Kennedy, S., Wang, D., et al. (2005). Systematic analysis of genes required for synapse structure and function. Nature 436, 510–517. doi: 10.1038/nature03809
Smith, C. J., Grigorieff, N., and Pearse, B. M. (1998). Clathrin coats at 21 A resolution: a cellular assembly designed to recycle multiple membrane receptors. EMBO J. 17, 4943–4953. doi: 10.1093/emboj/17.17.4943
Snead, D., Wragg, R. T., Dittman, J. S., and Eliezer, D. (2014). Membrane curvature sensing by the C-terminal domain of complexin. Nat. Commun. 5:4955. doi: 10.1038/ncomms5955
Söllner, T., Whiteheart, S. W., Brunner, M., Erdjument-Bromage, H., Geromanos, S., Tempst, P., et al. (1993). SNAP receptors implicated in vesicle targeting and fusion. Nature 362, 318–324. doi: 10.1038/362318a0
Soykan, T., Maritzen, T., and Haucke, V. (2016). Modes and mechanisms of synaptic vesicle recycling. Curr. Opin. Neurobiol. 39, 17–23. doi: 10.1016/j.conb.2016.03.005
Spang, A. (2016). Membrane tethering complexes in the endosomal system. Front. Cell Dev. Biol. 4:35. doi: 10.3389/fcell.2016.00035
Stein, A., Weber, G., Wahl, M. C., and Jahn, R. (2009). Helical extension of the neuronal SNARE complex into the membrane. Nature 460, 525–528. doi: 10.1038/nature08156
Stenbeck, G. (1998). Soluble NSF-attachment proteins. Int. J. Biochem. Cell Biol. 30, 573–577. doi: 10.1016/s1357-2725(97)00064-2
Stenmark, H. (2009). Rab GTPases as coordinators of vesicle traffic. Nat. Rev. Mol. Cell Biol. 10, 513–525. doi: 10.1038/nrm2728
Stenmark, H., and Olkkonen, V. M. (2001). The Rab GTPase family. Genome Biol. 2:REVIEWS3007. doi: 10.1186/gb-2001-2-5-reviews3007
Stepien, K. P., Prinslow, E. A., and Rizo, J. (2019). Munc18-1 is crucial to overcome the inhibition of synaptic vesicle fusion by αSNAP. Nat. Commun. 10:4326. doi: 10.1038/s41467-019-12188-4
Stevens, R. J., Akbergenova, Y., Jorquera, R. A., and Littleton, J. T. (2012). Abnormal synaptic vesicle biogenesis in Drosophila synaptogyrin mutants. J. Neurosci. 32, 18054–18067. doi: 10.1523/JNEUROSCI.2668-12.2012
Stewart, B. A., Mohtashami, M., Rivlin, P., Deitcher, D. L., Trimble, W. S., and Boulianne, G. L. (2002). Dominant-negative NSF2 disrupts the structure and function of Drosophila neuromuscular synapses. J. Neurobiol. 51, 261–271. doi: 10.1002/neu.10059
Stewart, B. A., Mohtashami, M., Trimble, W. S., and Boulianne, G. L. (2000). SNARE proteins contribute to calcium cooperativity of synaptic transmission. Proc. Natl. Acad. Sci. U.S.A. 97, 13955–13960. doi: 10.1073/pnas.250491397
Stigloher, C., Zhan, H., Zhen, M., Richmond, J., and Bessereau, J.-L. (2011). The presynaptic dense projection of the Caenorhabditis elegans cholinergic neuromuscular junction localizes synaptic vesicles at the active zone through SYD-2/liprin and UNC-10/RIM-dependent interactions. J. Neurosci. 31, 4388–4396. doi: 10.1523/JNEUROSCI.6164-10.2011
Striegel, A. R., Biela, L. M., Evans, C. S., Wang, Z., Delehoy, J. B., Sutton, R. B., et al. (2012). Calcium binding by synaptotagmin’s C2A domain is an essential element of the electrostatic switch that triggers synchronous synaptic transmission. J. Neurosci. 32, 1253–1260. doi: 10.1523/JNEUROSCI.4652-11.2012
Sudhof, T. C. (2004). The synaptic vesicle cycle. Annu. Rev. Neurosci. 27, 509–547. doi: 10.1146/annurev.neuro.26.041002.131412
Südhof, T. C. (2012). The presynaptic active zone. Neuron 75, 11–25. doi: 10.1016/j.neuron.2012.06.012
Südhof, T. C. (2013). Neurotransmitter release: the last millisecond in the life of a synaptic vesicle. Neuron 80, 675–690. doi: 10.1016/j.neuron.2013.10.022
Südhof, T. C., and Rothman, J. E. (2009). Membrane fusion: grappling with SNARE and SM proteins. Science 323, 474–477. doi: 10.1126/science.1161748
Sutton, R. B., Fasshauer, D., Jahn, R., and Brunger, A. T. (1998). Crystal structure of a SNARE complex involved in synaptic exocytosis at 2.4 A resolution. Nature 395, 347–353. doi: 10.1038/26412
Sweeney, S. T., Broadie, K., Keane, J., Niemann, H., and O’Kane, C. J. (1995). Targeted expression of tetanus toxin light chain in Drosophila specifically eliminates synaptic transmission and causes behavioral defects. Neuron 14, 341–351. doi: 10.1016/0896-6273(95)90290-2
Sweitzer, S. M., and Hinshaw, J. E. (1998). Dynamin undergoes a GTP-dependent conformational change causing vesiculation. Cell 93, 1021–1029. doi: 10.1016/s0092-8674(00)81207-6
Tagaya, M., Wilson, D. W., Brunner, M., Arango, N., and Rothman, J. E. (1993). Domain structure of an N-ethylmaleimide-sensitive fusion protein involved in vesicular transport. J. Biol. Chem. 268, 2662–2666. doi: 10.1016/s0021-9258(18)53825-4
Takei, K., Mundigl, O., Daniell, L., and De Camilli, P. (1996). The synaptic vesicle cycle: a single vesicle budding step involving clathrin and dynamin. J. Cell Biol. 133, 1237–1250. doi: 10.1083/jcb.133.6.1237
Tien, C.-W., Yu, B., Huang, M., Stepien, K. P., Sugita, K., Xie, X., et al. (2020). Open syntaxin overcomes exocytosis defects of diverse mutants in C. elegans. Nat. Commun. 11:5516. doi: 10.1038/s41467-020-19178-x
Tolar, L. A., and Pallanck, L. (1998). NSF function in neurotransmitter release involves rearrangement of the SNARE complex downstream of synaptic vesicle docking. J. Neurosci. 18, 10250–10256. doi: 10.1523/jneurosci.18-24-10250.1998
Trimble, W. S., Cowan, D. M., and Scheller, R. H. (1988). VAMP-1: a synaptic vesicle-associated integral membrane protein. Proc. Natl. Acad. Sci. U.S.A. 85, 4538–4542. doi: 10.1073/pnas.85.12.4538
Trimbuch, T., and Rosenmund, C. (2016). Should I stop or should I go? The role of complexin in neurotransmitter release. Nat. Rev. Neurosci. 17, 118–125. doi: 10.1038/nrn.2015.16
Tucker, W. C., Weber, T., and Chapman, E. R. (2004). Reconstitution of Ca2+-regulated membrane fusion by synaptotagmin and SNAREs. Science 304, 435–438. doi: 10.1126/science.1097196
Ubach, J., Lao, Y., Fernandez, I., Arac, D., Südhof, T. C., and Rizo, J. (2001). The C2B domain of synaptotagmin I is a Ca2+-binding module. Biochemistry 40, 5854–5860. doi: 10.1021/bi010340c
Ubach, J., Zhang, X., Shao, X., Südhof, T. C., and Rizo, J. (1998). Ca2+ binding to synaptotagmin: how many Ca2+ ions bind to the tip of a C2-domain? EMBO J. 17, 3921–3930. doi: 10.1093/emboj/17.14.3921
Ungewickell, E., and Branton, D. (1981). Assembly units of clathrin coats. Nature 289, 420–422. doi: 10.1038/289420a0
van de Goor, J., Ramaswami, M., and Kelly, R. (1995). Redistribution of synaptic vesicles and their proteins in temperature-sensitive shibire(ts1) mutant Drosophila. Proc. Natl. Acad. Sci. U.S.A. 92, 5739–5743. doi: 10.1073/pnas.92.12.5739
van der Bliek, A. M., and Meyerowitz, E. M. (1991). Dynamin-like protein encoded by the Drosophila shibire gene associated with vesicular traffic. Nature 351, 411–414. doi: 10.1038/351411a0
Varoqueaux, F., and Fasshauer, D. (2017). Getting nervous: an evolutionary overhaul for communication. Annu. Rev. Genet. 51, 455–476. doi: 10.1146/annurev-genet-120116-024648
Varoqueaux, F., Sigler, A., Rhee, J.-S., Brose, N., Enk, C., Reim, K., et al. (2002). Total arrest of spontaneous and evoked synaptic transmission but normal synaptogenesis in the absence of Munc13-mediated vesicle priming. Proc. Natl. Acad. Sci. U.S.A. 99, 9037–9042. doi: 10.1073/pnas.122623799
Vasin, A., Volfson, D., Littleton, J. T., and Bykhovskaia, M. (2016). Interaction of the complexin accessory helix with synaptobrevin regulates spontaneous fusion. Biophys. J. 111, 1954–1964. doi: 10.1016/j.bpj.2016.09.017
Verhage, M., Maia, A. S., Plomp, J. J., Brussaard, A. B., Heeroma, J. H., Vermeer, H., et al. (2000). Synaptic assembly of the brain in the absence of neurotransmitter secretion. Science 287, 864–869. doi: 10.1126/science.287.5454.864
Vilinsky, I., Stewart, B. A., Drummond, J., Robinson, I., and Deitcher, D. L. (2002). A Drosophila SNAP-25 null mutant reveals context-dependent redundancy with SNAP-24 in neurotransmission. Genetics 162, 259–271. doi: 10.1093/genetics/162.1.259
Vivona, S., Cipriano, D. J., O’Leary, S., Li, Y. H., Fenn, T. D., and Brunger, A. T. (2013). Disassembly of all SNARE complexes by N-ethylmaleimide-sensitive factor (NSF) is initiated by a conserved 1:1 interaction between α-soluble NSF attachment protein (SNAP) and SNARE complex. J. Biol. Chem. 288, 24984–24991. doi: 10.1074/jbc.M113.489807
Wagh, D. A., Rasse, T. M., Asan, E., Hofbauer, A., Schwenkert, I., Dürrbeck, H., et al. (2006). Bruchpilot, a protein with homology to ELKS/CAST, is required for structural integrity and function of synaptic active zones in Drosophila. Neuron 49, 833–844. doi: 10.1016/j.neuron.2006.02.008
Walther, K., Diril, M. K., Jung, N., and Haucke, V. (2004). Functional dissection of the interactions of stonin 2 with the adaptor complex AP-2 and synaptotagmin. Proc. Natl. Acad. Sci. U.S.A. 101, 964–969. doi: 10.1073/pnas.0307862100
Wang, S., Yang, J., Tsai, A., Kuca, T., Sanny, J., Lee, J., et al. (2011). Drosophila adducin regulates Dlg phosphorylation and targeting of Dlg to the synapse and epithelial membrane. Dev. Biol. 357, 392–403. doi: 10.1016/j.ydbio.2011.07.010
Wang, Y., Okamoto, M., Schmitz, F., Hofmann, K., and Südhof, T. C. (1997). Rim is a putative Rab3 effector in regulating synaptic-vesicle fusion. Nature 388, 593–598. doi: 10.1038/41580
Washbourne, P., Thompson, P. M., Carta, M., Costa, E. T., Mathews, J. R., Lopez-Benditó, G., et al. (2002). Genetic ablation of the t-SNARE SNAP-25 distinguishes mechanisms of neuroexocytosis. Nat. Neurosci. 5, 19–26. doi: 10.1038/nn783
Watanabe, S., Liu, Q., Davis, M. W., Hollopeter, G., Thomas, N., Jorgensen, N. B., et al. (2013a). Ultrafast endocytosis at Caenorhabditis elegans neuromuscular junctions. eLife 2:e00723. doi: 10.7554/eLife.00723
Watanabe, S., Rost, B. R., Camacho-Pérez, M., Davis, M. W., Söhl-Kielczynski, B., Rosenmund, C., et al. (2013b). Ultrafast endocytosis at mouse hippocampal synapses. Nature 504, 242–247. doi: 10.1038/nature12809
Weber, T., Zemelman, B. V., McNew, J. A., Westermann, B., Gmachl, M., Parlati, F., et al. (1998). SNAREpins: minimal machinery for membrane fusion. Cell 92, 759–772. doi: 10.1016/s0092-8674(00)81404-x
Weimbs, T., Low, S. H., Chapin, S. J., Mostov, K. E., Bucher, P., and Hofmann, K. (1997). A conserved domain is present in different families of vesicular fusion proteins: a new superfamily. Proc. Natl. Acad. Sci. U.S.A. 94, 3046–3051. doi: 10.1073/pnas.94.7.3046
Weimer, R. M., Gracheva, E. O., Meyrignac, O., Miller, K. G., Richmond, J. E., and Bessereau, J.-L. (2006). UNC-13 and UNC-10/rim localize synaptic vesicles to specific membrane domains. J. Neurosci. 26, 8040–8047. doi: 10.1523/JNEUROSCI.2350-06.2006
Weimer, R. M., Richmond, J. E., Davis, W. S., Hadwiger, G., Nonet, M. L., and Jorgensen, E. M. (2003). Defects in synaptic vesicle docking in unc-18 mutants. Nat. Neurosci. 6, 1023–1030. doi: 10.1038/nn1118
Wen, X., Saltzgaber, G. W., and Thoreson, W. B. (2017). Kiss-and-run is a significant contributor to synaptic exocytosis and endocytosis in photoreceptors. Front. Cell Neurosci. 11:286. doi: 10.3389/fncel.2017.00286
White, K. I., Zhao, M., Choi, U. B., Pfuetzner, R. A., and Brunger, A. T. (2018). Structural principles of SNARE complex recognition by the AAA+ protein NSF. eLife 7:e38888. doi: 10.7554/eLife.38888
Whiteheart, S. W., Brunner, M., Wilson, D. W., Wiedmann, M., and Rothman, J. E. (1992). Soluble N-ethylmaleimide-sensitive fusion attachment proteins (SNAPs) bind to a multi-SNAP receptor complex in Golgi membranes. J. Biol. Chem. 267, 12239–12243. doi: 10.1016/s0021-9258(19)49830-x
Whyte, J. R. C., and Munro, S. (2002). Vesicle tethering complexes in membrane traffic. J. Cell Sci. 115, 2627–2637. doi: 10.1242/jcs.115.13.2627
Wickner, W., and Schekman, R. (2008). Membrane fusion. Nat. Struct. Mol. Biol. 15, 658–664. doi: 10.1038/nsmb.1451
Williams, A. L., Bielopolski, N., Meroz, D., Lam, A. D., Passmore, D. R., Ben-Tal, N., et al. (2011). Structural and functional analysis of tomosyn identifies domains important in exocytotic regulation. J. Biol. Chem. 286, 14542–14553. doi: 10.1074/jbc.M110.215624
Wilson, D. W., Whiteheart, S. W., Wiedmann, M., Brunner, M., and Rothman, J. E. (1992). A multisubunit particle implicated in membrane fusion. J. Cell Biol. 117, 531–538. doi: 10.1083/jcb.117.3.531
Wilson, D. W., Wilcox, C. A., Flynn, G. C., Chen, E., Kuang, W. J., Henzel, W. J., et al. (1989). A fusion protein required for vesicle-mediated transport in both mammalian cells and yeast. Nature 339, 355–359. doi: 10.1038/339355a0
Witkos, T. M., and Lowe, M. (2015). The golgin family of coiled-coil tethering proteins. Front. Cell Dev. Biol. 3:86. doi: 10.3389/fcell.2015.00086
Wittig, S., Ganzella, M., Barth, M., Kostmann, S., Riedel, D., and Pérez-Lara, Á, et al. (2021). Cross-linking mass spectrometry uncovers protein interactions and functional assemblies in synaptic vesicle membranes. Nat. Commun. 12:858. doi: 10.1038/s41467-021-21102-w
Wragg, R. T., Parisotto, D. A., Li, Z., Terakawa, M. S., Snead, D., Basu, I., et al. (2017). Evolutionary divergence of the c-terminal domain of complexin accounts for functional disparities between vertebrate and invertebrate complexins. Front. Mol. Neurosci. 10:146. doi: 10.3389/fnmol.2017.00146
Wu, M. N., Fergestad, T., Lloyd, T. E., He, Y., Broadie, K., and Bellen, H. J. (1999). Syntaxin 1A interacts with multiple exocytic proteins to regulate neurotransmitter release in vivo. Neuron 23, 593–605. doi: 10.1016/s0896-6273(00)80811-9
Wu, M. N., Littleton, J. T., Bhat, M. A., Prokop, A., and Bellen, H. J. (1998). ROP, the Drosophila Sec1 homolog, interacts with syntaxin and regulates neurotransmitter release in a dosage-dependent manner. EMBO J. 17, 127–139. doi: 10.1093/emboj/17.1.127
Wu, Y., Kawasaki, F., and Ordway, R. W. (2005). Properties of short-term synaptic depression at larval neuromuscular synapses in wild-type and temperature-sensitive paralytic mutants of Drosophila. J. Neurophysiol. 93, 2396–2405. doi: 10.1152/jn.01108.2004
Wu, Y.-J., Tejero, R., Arancillo, M., Vardar, G., Korotkova, T., Kintscher, M., et al. (2015). Syntaxin 1B is important for mouse postnatal survival and proper synaptic function at the mouse neuromuscular junctions. J. Neurophysiol. 114, 2404–2417. doi: 10.1152/jn.00577.2015
Xia, X., Lessmann, V., and Martin, T. F. J. (2009). Imaging of evoked dense-core-vesicle exocytosis in hippocampal neurons reveals long latencies and kiss-and-run fusion events. J. Cell Sci. 122, 75–82. doi: 10.1242/jcs.034603
Xu, Y., Kuhlmann, J., Brennich, M., Komorowski, K., Jahn, R., Steinem, C., et al. (2018). Reconstitution of SNARE proteins into solid-supported lipid bilayer stacks and X-ray structure analysis. Biochim. Biophys. Acta Biomembr. 1860, 566–578. doi: 10.1016/j.bbamem.2017.10.023
Xue, M., Craig, T. K., Xu, J., Chao, H.-T., Rizo, J., and Rosenmund, C. (2010). Binding of the complexin N terminus to the SNARE complex potentiates synaptic-vesicle fusogenicity. Nat. Struct. Mol. Biol. 17, 568–575. doi: 10.1038/nsmb.1791
Xue, M., Lin, Y. Q., Pan, H., Reim, K., Deng, H., Bellen, H. J., et al. (2009). Tilting the balance between facilitatory and inhibitory functions of mammalian and Drosophila Complexins orchestrates synaptic vesicle exocytosis. Neuron 64, 367–380. doi: 10.1016/j.neuron.2009.09.043
Xue, M., Reim, K., Chen, X., Chao, H.-T., Deng, H., Rizo, J., et al. (2007). Distinct domains of complexin I differentially regulate neurotransmitter release. Nat. Struct. Mol. Biol. 14, 949–958. doi: 10.1038/nsmb1292
Xue, M., Stradomska, A., Chen, H., Brose, N., Zhang, W., Rosenmund, C., et al. (2008). Complexins facilitate neurotransmitter release at excitatory and inhibitory synapses in mammalian central nervous system. Proc. Natl. Acad. Sci. U.S.A. 105, 7875–7880. doi: 10.1073/pnas.0803012105
Yamamoto, Y., Fujikura, K., Sakaue, M., Okimura, K., Kobayashi, Y., Nakamura, T., et al. (2010). The tail domain of tomosyn controls membrane fusion through tomosyn displacement by VAMP2. Biochem. Biophys. Res. Commun. 399, 24–30. doi: 10.1016/j.bbrc.2010.07.026
Yang, B., Steegmaier, M., Gonzalez, L. C., and Scheller, R. H. (2000). nSec1 binds a closed conformation of syntaxin1A. J. Cell Biol. 148, 247–252. doi: 10.1083/jcb.148.2.247
Yang, X., Cao, P., and Südhof, T. C. (2013). Deconstructing complexin function in activating and clamping Ca2+-triggered exocytosis by comparing knockout and knockdown phenotypes. Proc. Natl. Acad. Sci. U.S.A. 110, 20777–20782. doi: 10.1073/pnas.1321367110
Yang, X., Kaeser-Woo, Y. J., Pang, Z. P., Xu, W., and Südhof, T. C. (2010). Complexin clamps asynchronous release by blocking a secondary Ca(2+) sensor via its accessory α helix. Neuron 68, 907–920. doi: 10.1016/j.neuron.2010.11.001
Yelamanchili, S. V., Reisinger, C., Becher, A., Sikorra, S., Bigalke, H., Binz, T., et al. (2005). The C-terminal transmembrane region of synaptobrevin binds synaptophysin from adult synaptic vesicles. Eur. J. Cell Biol. 84, 467–475. doi: 10.1016/j.ejcb.2004.11.007
Yizhar, O., Lipstein, N., Gladycheva, S. E., Matti, U., Ernst, S. A., Rettig, J., et al. (2007). Multiple functional domains are involved in tomosyn regulation of exocytosis. J. Neurochem. 103, 604–616. doi: 10.1111/j.1471-4159.2007.04791.x
Yizhar, O., Matti, U., Melamed, R., Hagalili, Y., Bruns, D., Rettig, J., et al. (2004). Tomosyn inhibits priming of large dense-core vesicles in a calcium-dependent manner. Proc. Natl. Acad. Sci. U.S.A. 101, 2578–2583. doi: 10.1073/pnas.0308700100
Yokoyama, S., Shirataki, H., Sakisaka, T., and Takai, Y. (1999). Three splicing variants of tomosyn and identification of their syntaxin-binding region. Biochem. Biophys. Res. Commun. 256, 218–222. doi: 10.1006/bbrc.1999.0300
Yoon, T.-Y., and Munson, M. (2018). SNARE complex assembly and disassembly. Curr. Biol. 28, R397–R401. doi: 10.1016/j.cub.2018.01.005
Yoshihara, M., Adolfsen, B., Galle, K. T., and Littleton, J. T. (2005). Retrograde signaling by Syt 4 induces presynaptic release and synapse-specific growth. Science 310, 858–863. doi: 10.1126/science.1117541
Yoshihara, M., Guan, Z., and Littleton, J. T. (2010). Differential regulation of synchronous versus asynchronous neurotransmitter release by the C2 domains of synaptotagmin 1. Proc. Natl. Acad. Sci. U.S.A. 107, 14869–14874. doi: 10.1073/pnas.1000606107
Yoshihara, M., and Littleton, J. T. (2002). Synaptotagmin I functions as a calcium sensor to synchronize neurotransmitter release. Neuron 36, 897–908. doi: 10.1016/s0896-6273(02)01065-6
Yoshihara, M., Ueda, A., Zhang, D., Deitcher, D. L., Schwarz, T. L., and Kidokoro, Y. (1999). Selective effects of neuronal-synaptobrevin mutations on transmitter release evoked by sustained versus transient Ca2+ increases and by cAMP. J. Neurosci. 19, 2432–2441. doi: 10.1523/jneurosci.19-07-02432.1999
Yu, I.-M., and Hughson, F. M. (2010). Tethering factors as organizers of intracellular vesicular traffic. Annu. Rev. Cell Dev. Biol. 26, 137–156. doi: 10.1146/annurev.cellbio.042308.113327
Yu, W., Kawasaki, F., and Ordway, R. W. (2011). Activity-dependent interactions of NSF and SNAP at living synapses. Mol. Cell. Neurosci. 47, 19–27. doi: 10.1016/j.mcn.2011.02.002
Zhai, R. G., and Bellen, H. J. (2004). The architecture of the active zone in the presynaptic nerve terminal. Physiology 19, 262–270. doi: 10.1152/physiol.00014.2004
Zhang, B., Koh, Y. H., Beckstead, R. B., Budnik, V., Ganetzky, B., and Bellen, H. J. (1998). Synaptic vesicle size and number are regulated by a clathrin adaptor protein required for endocytosis. Neuron 21, 1465–1475. doi: 10.1016/s0896-6273(00)80664-9
Zhang, J. Z., Davletov, B. A., Südhof, T. C., and Anderson, R. G. (1994). Synaptotagmin I is a high affinity receptor for clathrin AP-2: implications for membrane recycling. Cell 78, 751–760. doi: 10.1016/s0092-8674(94)90442-1
Zhang, Q., Li, Y., and Tsien, R. W. (2009). The dynamic control of kiss-and-run and vesicular reuse probed with single nanoparticles. Science 323, 1448–1453. doi: 10.1126/science.1167373
Zhang, W., Lilja, L., Mandic, S. A., Gromada, J., Smidt, K., Janson, J., et al. (2006). Tomosyn is expressed in beta-cells and negatively regulates insulin exocytosis. Diabetes Metab. Res. Rev. 55, 574–581. doi: 10.2337/diabetes.55.03.06.db05-0015
Zhang, Y., and Hughson, F. M. (2021). Chaperoning SNARE folding and assembly. Annu. Rev. Biochem. 17, 465–479. doi: 10.1146/annurev-biochem-081820-103615
Zhao, M., and Brunger, A. T. (2016). Recent advances in deciphering the structure and molecular mechanism of the AAA+ ATPase N-ethylmaleimide-sensitive factor (NSF). J. Mol. Biol. 428, 1912–1926. doi: 10.1016/j.jmb.2015.10.026
Zhao, M., Wu, S., Zhou, Q., Vivona, S., Cipriano, D. J., Cheng, Y., et al. (2015). Mechanistic insights into the recycling machine of the SNARE complex. Nature 518, 61–67. doi: 10.1038/nature14148
Zhou, Q., Huang, X., Sun, S., Li, X., Wang, H.-W., and Sui, S.-F. (2015a). Cryo-EM structure of SNAP-SNARE assembly in 20S particle. Cell Res. 25, 551–560. doi: 10.1038/cr.2015.47
Zhou, Q., Lai, Y., Bacaj, T., Zhao, M., Lyubimov, A. Y., Uervirojnangkoorn, M., et al. (2015b). Architecture of the synaptotagmin-SNARE machinery for neuronal exocytosis. Nature 525, 62–67. doi: 10.1038/nature14975
Zhou, Q., Zhou, P., Wang, A. L., Wu, D., Zhao, M., Südhof, T. C., et al. (2017). The primed SNARE-complexin-synaptotagmin complex for neuronal exocytosis. Nature 548, 420–425. doi: 10.1038/nature23484
Keywords: SNARE, synapse, synaptic vesicle, neurotransmitter release, membrane trafficking, Drosophila melanogaster, Caenorhabditis elegans
Citation: Sauvola CW and Littleton JT (2021) SNARE Regulatory Proteins in Synaptic Vesicle Fusion and Recycling. Front. Mol. Neurosci. 14:733138. doi: 10.3389/fnmol.2021.733138
Received: 29 June 2021; Accepted: 20 July 2021;
Published: 06 August 2021.
Edited by:
Zhiyong Shao, Fudan University, ChinaReviewed by:
Ege Kavalali, Vanderbilt University, United StatesYongli Zhang, Yale University, United States
Copyright © 2021 Sauvola and Littleton. This is an open-access article distributed under the terms of the Creative Commons Attribution License (CC BY). The use, distribution or reproduction in other forums is permitted, provided the original author(s) and the copyright owner(s) are credited and that the original publication in this journal is cited, in accordance with accepted academic practice. No use, distribution or reproduction is permitted which does not comply with these terms.
*Correspondence: Chad W. Sauvola, sauvolac@mit.edu; J. Troy Littleton, troy@mit.edu