The thalamostriatal system in normal and diseased states
- 1Yerkes National Primate Research Center, Emory University, Atlanta, GA, USA
- 2Department of Neurology, Emory University, Atlanta, GA, USA
- 3Udall Center of Excellence for Parkinson’s Disease, Emory University, Atlanta, GA, USA
- 4Department of Pharmacology, MRC Anatomical Neuropharmacology Unit, Oxford, UK
Because of our limited knowledge of the functional role of the thalamostriatal system, this massive network is often ignored in models of the pathophysiology of brain disorders of basal ganglia origin, such as Parkinson’s disease (PD). However, over the past decade, significant advances have led to a deeper understanding of the anatomical, electrophysiological, behavioral and pathological aspects of the thalamostriatal system. The cloning of the vesicular glutamate transporters 1 and 2 (vGluT1 and vGluT2) has provided powerful tools to differentiate thalamostriatal from corticostriatal glutamatergic terminals, allowing us to carry out comparative studies of the synaptology and plasticity of these two systems in normal and pathological conditions. Findings from these studies have led to the recognition of two thalamostriatal systems, based on their differential origin from the caudal intralaminar nuclear group, the center median/parafascicular (CM/Pf) complex, or other thalamic nuclei. The recent use of optogenetic methods supports this model of the organization of the thalamostriatal systems, showing differences in functionality and glutamate receptor localization at thalamostriatal synapses from Pf and other thalamic nuclei. At the functional level, evidence largely gathered from thalamic recordings in awake monkeys strongly suggests that the thalamostriatal system from the CM/Pf is involved in regulating alertness and switching behaviors. Importantly, there is evidence that the caudal intralaminar nuclei and their axonal projections to the striatum partly degenerate in PD and that CM/Pf deep brain stimulation (DBS) may be therapeutically useful in several movement disorders.
Introduction
Although the evolution of the thalamus and striatum pre-dates the expansion of the cerebral cortex (Butler, 1994; Reiner, 2010; Stephenson-Jones et al., 2011), our knowledge about the functional anatomy and behavioral role of the connections between them is minimal compared to the amount of information that has been gathered about the corticostriatal system (Kemp and Powell, 1971; Alexander et al., 1986; Parent and Hazrati, 1995). However, significant progress has been made in our understanding of the anatomical and functional organization of the thalamostriatal system since its first description in the early 1940’s (Vogt and Vogt, 1941; Cowan and Powell, 1956). Research in the last decade has resulted in a much better understanding of various aspects of the synaptic properties of the thalamostriatal projection(s), and their potential roles in cognition. Furthermore, evidence that the center median/parafascicular (CM/Pf) complex, the main source of thalamostriatal connections, is severely degenerated in Parkinson’s disease (PD), combined with the fact that lesion or deep brain stimulation (DBS) of this nuclear group alleviates some of the motor and non-motor symptoms of Tourette’s syndrome (TS) and PD, has generated significant interest in these projections. In this review, we will discuss these topics and provide an overview of our current knowledge of the functional anatomy, synaptology, and physiology of the mammalian thalamostriatal system, as well as the involvement of these projections in disease processes. Because of space limitation, we will focus mostly on recent developments in this field. Readers are referred to previous reviews for additional information and a broader coverage of the early literature on the thalamostriatal projections (Groenewegen and Berendse, 1994; Parent and Hazrati, 1995; Mengual et al., 1999; Haber and Mcfarland, 2001; Van der Werf et al., 2002; Kimura et al., 2004; Smith et al., 2004, 2009, 2010, 2011; McHaffie et al., 2005; Haber and Calzavara, 2009; Halliday, 2009; Minamimoto et al., 2009; Sadikot and Rymar, 2009; Galvan and Smith, 2011; Bradfield et al., 2013b).
Thalamostriatal Circuitry and Synaptic Connectivity
Functionally Segregated Basal Ganglia-Thalamostriatal Circuits Through the Center Median/Parafascicular (CM/Pf) Complex
Although the thalamostriatal system originates from most thalamic nuclei, the CM/Pf (or the Pf in rodents) is the main source of thalamostriatal projections in primates and non-primates (Smith and Parent, 1986; Berendse and Groenewegen, 1990; Francois et al., 1991; Sadikot et al., 1992a,b; Deschenes et al., 1996a,b; Mengual et al., 1999; McFarland and Haber, 2000; Mcfarland and Haber, 2001; Smith et al., 2004, 2009; Castle et al., 2005; McHaffie et al., 2005; Parent and Parent, 2005; Raju et al., 2006; Lacey et al., 2007). CM/Pf neurons send massive and topographically organized projections to specific regions of the dorsal striatum, but provide only minor inputs to the cerebral cortex (Sadikot et al., 1992a; Parent and Parent, 2005; Galvan and Smith, 2011; Figure 1). Single cell tracing studies in monkeys have shown that more than half of all CM neurons innervate densely and focally the striatum without significant input to the cerebral cortex, while about one third innervates diffusely the cerebral cortex without significant projections to the striatum, and the remaining neurons project to both targets with a preponderance of innervation of the dorsal striatum (Parent and Parent, 2005).
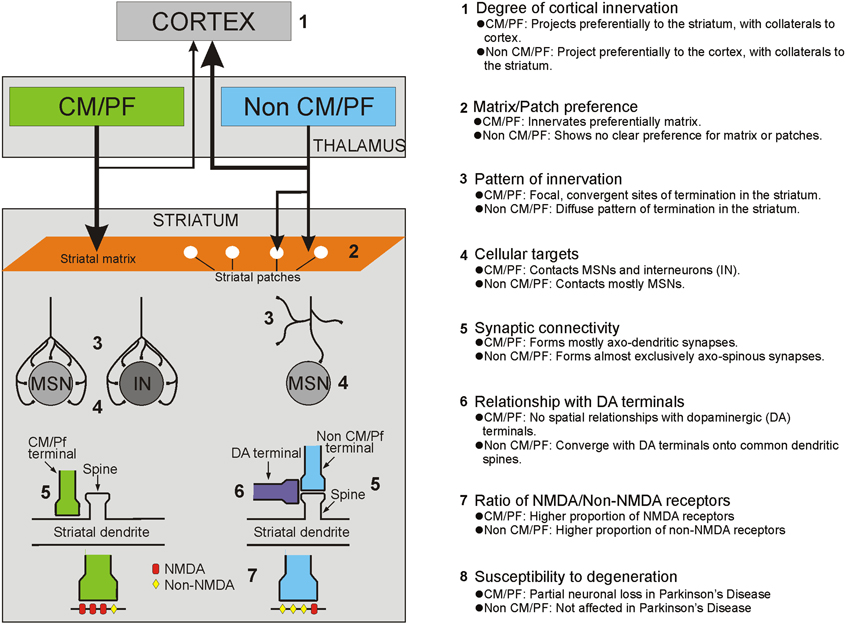
Figure 1. Summary of the anatomical, functional and pathological characteristics that differentiate thalamostriatal projections from the CM/Pf vs. other thalamic nuclei (i.e., non CM/Pf).
Based on its preferential targeting of specific functional territories, the primate CM/Pf complex is divided into five major sub-regions: (1) the rostral third of Pf which innervates mainly the nucleus accumbens; (2) the caudal two thirds of Pf which project to the caudate nucleus; (3) the dorsolateral extension of Pf (Pfdl) which targets selectively the anterior putamen; (4) the medial two thirds of CM (CMm) which innervates the post-commissural putamen; and (5) the lateral third of CM (CMl) which is the source of inputs the primary motor cortex (M1). Through these projections, the CM/Pf gains access to the entire striatal complex, thereby making the CM/Pf-striatal system a functionally organized network that may broadly affect motor and non-motor basal ganglia functions (Smith et al., 2004, 2009, 2010; Galvan and Smith, 2011). In rodents, the lateral part of Pf is considered to be the homologue of the primate CM and projects mainly to the sensorimotor region (i.e., the dorsolateral part) of the caudate-putamen complex, whereas the medial rodent Pf displays strong similarities with the primate Pf, projecting to associative and limbic striatal regions of the striatum (Groenewegen and Berendse, 1994).
Thalamostriatal Systems From Non-Center Median/Parafascicular (CM/Pf) Thalamic Nuclei
In addition to the CM/Pf complex, thalamostriatal projections originate from several other rostral intralaminar and non-intralaminar thalamic nuclei. In primates and non-primates, the rostral intralaminar nuclei (central lateral, paracentral, central medial), the mediodorsal nucleus (MD), the pulvinar, the lateral posterior nucleus, the medial posterior nucleus, midline, anterior and the ventral motor nuclear group are prominent sources of thalamostriatal projections to the caudate nucleus and putamen (Royce, 1978; Beckstead, 1984; Smith and Parent, 1986; Groenewegen and Berendse, 1994; Smith et al., 2004, 2009; Alloway et al., 2014). In contrast to the projections from the CM/Pf complex, these nuclei send major projections to the cerebral cortex, while contributing a modest or sparse innervation of the dorsal and ventral striatum (Royce, 1983; Macchi et al., 1984; Deschenes et al., 1996a,b; Smith et al., 2004, 2009; Figure 1). In rats, the topography of these projections corresponds to the functionally segregated organization of the striatum, so that sensorimotor-, associative- and limbic-related thalamic nuclei innervate functionally corresponding regions of the dorsal and ventral striatum (Berendse and Groenewegen, 1990, 1991; Groenewegen and Berendse, 1994). Although such detailed analyses have not been done in primates (except for projections from the ventral anterior/ventral lateral (VA/VL) complex), evidence from retrograde and anterograde labeling studies indicate that the primate non-CM/Pf thalamostriatal projections also display a strict functional topography (Parent et al., 1983; Smith and Parent, 1986; Fenelon et al., 1991; McFarland and Haber, 2000; Mcfarland and Haber, 2001). The thalamostriatal projections from the ventral motor thalamic nuclei have received particular attention in these studies. It appears that projections from the pars oralis of the VL (VLo), the main recipient of sensorimotor internal globus pallidus (GPi) outflow, terminate preferentially in the post-commissural putamen, whereas projections from the magnocellular division of the VA, the principal target of the substantia nigra pars reticulata (SNr) and associative GPi outflow, innervate the caudate nucleus (Mcfarland and Haber, 2001). Within striatal territories, VA/VL projections terminate in a patchy manner in the striatum, indicating that additional organizational principles may be at work that have not yet been elucidated (Groenewegen and Berendse, 1994; McFarland and Haber, 2000; Mcfarland and Haber, 2001; Smith et al., 2004, 2009; Raju et al., 2006).
Through the use of trans-synaptic viral tracing studies, Strick and colleagues have suggested that thalamostriatal projections from the VL and other intralaminar thalamic nuclei that receive cerebellar outflow from the dentate nucleus may be the underlying connections through which the cerebellum communicates with the basal ganglia (Bostan and Strick, 2010; Bostan et al., 2010, 2013). Dysfunctions of these cerebello-thalamo-basal ganglia interactions may underlie some aspects of the pathophysiology of dystonia and other movement disorders (Jinnah and Hess, 2006; Neychev et al., 2008; Calderon et al., 2011).
The Superior Colliculus: A Potential Driver of the Thalamostriatal System in Mammals
Anatomical studies have suggested that additional sub-cortical tecto-basal ganglia loops exist that connect the superficial and deep layers of the superior colliculus with specific thalamic nuclei, which then gain access to the basal ganglia circuitry via thalamostriatal connections (McHaffie et al., 2005; Redgrave et al., 2010). The existence of these connections could resolve some of the fundamental issues associated with short-latency responses to biologically salient stimuli (Smith et al., 2011; Alloway et al., 2014). As discussed in more detail below, Pf neurons exhibit short-latency excitatory responses to salient stimuli. Redgrave and colleagues have suggested that the superior colliculus (optic tectum in lower species) displays the evolutionary profile, anatomical connectivity and physiological features that would allow it to mediate such effects upon thalamic neurons (McHaffie et al., 2005; Redgrave et al., 2010). The basal ganglia and the superior colliculus are neural structures that appeared early (>400 million years ago) and have been highly conserved throughout the evolution of the vertebrate brain (Reiner, 2010; Stephenson-Jones et al., 2011), thereby suggesting that they are part of fundamental processing units that play basic functions in mammalian behavior. The superior colliculus has direct access to primary sensory information, and studies have shown that stimuli associated with positive or negative outcomes activate different sub-regions of this nucleus that engage various tecto-thalamo-striatal loops (Redgrave et al., 1999; Alloway et al., 2014). Through these loops, the primary sensory events could be rapidly transmitted to the striatum and affect the basal ganglia circuitry which, in turn, could lead to basal ganglia-mediated disinhibition of different sub-regions of the superior colliculus that could help select and reinforce some sensory stimuli over others (Redgrave et al., 1999), most likely through regulation of corticostriatal plasticity via dopaminergic and cholinergic intrastriatal mechanisms (Ding et al., 2010; Smith et al., 2011). Through these processes, the short-latency sensory-driven activity in the superior colliculus could be used by the basal ganglia to reinforce the development of novel habits or procedures (Redgrave et al., 2010; Smith et al., 2011).
Synaptic Organization and Prevalence of Thalamostriatal Vs. Corticostriatal Terminals
Anterograde tracing studies in several species have shown that the thalamostriatal projections give rise to asymmetric (or Gray’s Type 1) synapses. In rodents, the principal synaptic target of most non-CM/Pf thalamostriatal projections are dendritic spines of striatal medium spiny neurons (MSNs), a pattern of synaptic connectivity similar to the corticostriatal system (Kemp and Powell, 1971; Dube et al., 1988; Xu et al., 1991; Raju et al., 2006; Lacey et al., 2007; Figures 1, 2). In contrast, striatal afferents from CM/Pf (or Pf in rodents) establish asymmetric synapses principally with dendritic shafts of MSNs (Dube et al., 1988; Sadikot et al., 1992b; Smith et al., 1994; Sidibe and Smith, 1996; Raju et al., 2006, 2008; Lacey et al., 2007) and several types of striatal interneurons including cholinergic interneurons (Meredith and Wouterlood, 1990; Lapper and Bolam, 1992; Sidibe and Smith, 1999) and parvalbumin-positive GABA interneurons (Rudkin and Sadikot, 1999; Sidibe and Smith, 1999; Figure 1). Overall, 70–90% of CM/Pf (or Pf) terminals form axo-dendritic synapses in the rat and monkey striatum (Dube et al., 1988; Sadikot et al., 1992b; Raju et al., 2006; Lacey et al., 2007). However, single cell filling studies have revealed that the pattern of synaptic connection of individual Pf neurons is highly variable in rats. For instance, some Pf neurons were found to be the sources of terminals that terminate almost exclusively on dendritic spines, whereas others predominantly target dendritic shafts (Lacey et al., 2007). It is not known whether these neurons represent functionally different subpopulations of Pf-striatal cells.
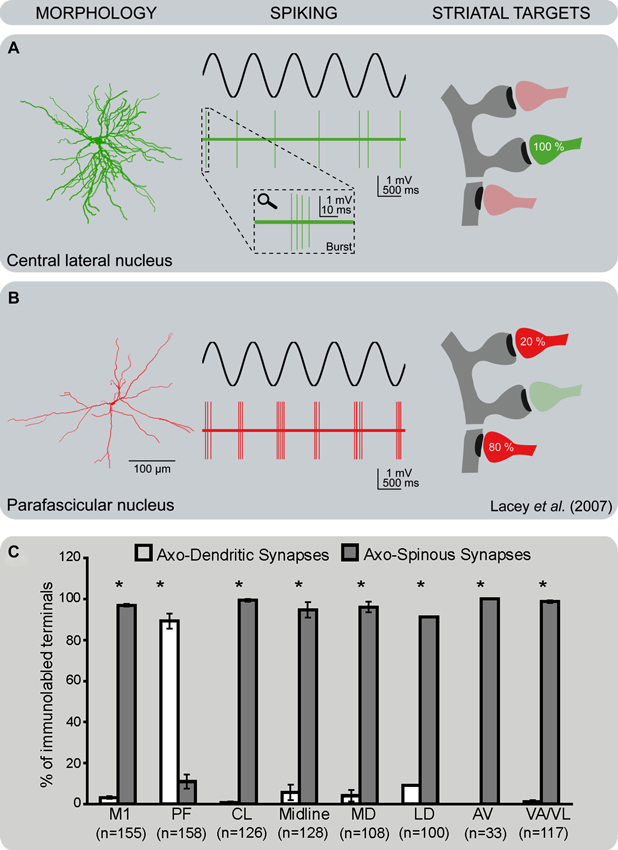
Figure 2. Differential pattern of activity and synaptic connectivity of CM/Pf vs. non-CM/Pf thalamostriatal terminals. (A) Thalamostriatal neurons of the central lateral nucleus (non-CM/Pf) have a compact bushy dendritic arbor. They tend to fire brief bursts of spikes at high frequency (2–5 spikes at ~150 Hz) in time with the active part of the cortical slow oscillation, reminiscent of low-threshold Ca2+ spike bursts. At the ultrastructural level, terminals from the central lateral nucleus almost exclusively target spines in the striatum. (B) Thalamostriatal neurons of the parafascicular nucleus have a reticular dendritic arbor with long and infrequently branching dendrites. They also tend to fire bursts of action potentials in time with the active part of the cortical slow oscillation, but the burst spike frequency is significantly lower and more variable than those of central lateral neurons. At the ultrastructural level, terminals from the parafascicular nucleus predominantly target the dendritic shafts of striatal neurons (see Lacey et al., 2005, 2007 for more details). (C) Histogram showing the frequency of axo-dendritic vs. axo-spinous synapses formed by anterogradely labeled boutons from various thalamic nuclei and the primary motor cortex (M1) in the rat striatum. Note the striking difference in the pattern of synaptic connectivity of terminals from Pf vs. other thalamic nuclei (see Raju et al., 2006 for more details). Abbreviations: AV: Anteroventral nucleus; CL: Central lateral nucleus; LD: Laterodorsal nucleus; MD: Mediodorsal nucleus; M1: Primary motor cortex; Pf: Parafascicular nucleus; VA/VL: Ventral anterior/ventral lateral nucleus.
The cloning of the vesicular glutamate transporters 1 or 2 (vGluT1 or vGluT2) (Fremeau et al., 2001, 2004) and the demonstration that these transporters are differentially expressed in corticostriatal (vGluT1-positive) or thalamostriatal (vGluT2-positive) terminals (but see Barroso-Chinea et al., 2008) have helped with the assessment of the relative prevalence, and the characterization of the synaptic connectivity of corticostriatal and thalamostriatal terminals in rodents and nonhuman primates. These studies showed that 95% of all vGluT1-positive corticostriatal projections terminate on dendritic spines of striatal neurons, and 5% on dendrites in both rodents and primates. This pattern is different from that of vGluT2-containing thalamostriatal boutons. For instance, only 50–65% (depending on the striatal region) of vGluT2-containing terminals contact dendritic spines in monkeys, while the remaining form asymmetric synapses with dendritic shafts (Raju et al., 2008). In rats, as many as 80% of vGluT2-positive terminals form axo-spinous synapses in the striatum (Raju et al., 2006; Lacey et al., 2007). Whether these differences in the proportion of vGluT2 terminals in contact with striatal spines represent a genuine species difference in the microcircuitry of the thalamostriatal system between primates and non-primates remain to be determined.
It is also important to note that the synaptic connectivity of vGluT2-containing terminals differs between the patch/striosome and the matrix compartments of the striatum in rats. While the ratio of axo-spinous and axo-dendritic synapses for vGluT2-immunoreactive terminals is 90:10 in the patch compartment, it is about 55:45 in the matrix (Raju et al., 2006; Figure 1). The fact that the massive thalamostriatal projection from Pf, a predominant source of axo-dendritic glutamatergic synapses, terminates exclusively in the striatal matrix accounts for this difference in the overall synaptology of vGluT2-containing terminals between the two striatal compartments (Herkenham and Pert, 1981; Sadikot et al., 1992b; Raju et al., 2006). Evidence that activity imbalances between the patch/striosome and matrix compartments may be involved in various basal ganglia disorders (Crittenden and Graybiel, 2011) highlights the potential significance of this compartmental segregation of CM/Pf inputs to the mammalian striatum. In contrast to vGluT2, the pattern of synaptic innervation of corticostriatal vGluT1-positive terminals does not differ between patch/striosome and matrix compartments (Raju et al., 2006).
The use of vGluT1 and vGluT2 also allowed the quantification of the relative prevalence of cortical and thalamic terminals in the rat and monkey striatum. In the monkey post-commissural putamen, ~50% of putative glutamatergic terminals (i.e., those forming asymmetric synapses) express vGluT1, ~25% contain vGluT2 and ~25% do not display immunoreactivity for either vGluT1 or vGluT2 (Raju et al., 2008). In rats, the differences in the prevalence of vGluT1 over vGluT2 terminals is not as striking (35% vGluT1 vs. 25% vGluT2 in rats), and the percentage of putative glutamatergic terminals unlabeled for either transporter subtype is higher (~40%) than in monkeys (Kaneko and Fujiyama, 2002; Fujiyama et al., 2004; Lacey et al., 2005; Fujiyama et al., 2006; Huerta-Ocampo et al., 2013). It remains unclear whether the large proportion of putative glutamatergic terminals that are not immunopositive for vGluT1 or vGluT2 are simply cortical and thalamic boutons that express undetectable levels of vGluT1 or vGluT2, whether they express other, yet unidentified, vGluT(s) or whether they are non-glutamatergic.
Thalamic Inputs to Direct Vs. Indirect Pathway Neurons
The striatum comprises two main populations of output neurons characterized by their differential dopamine receptors and neuropeptides expression (D1/substance P/dynorphin or D2/enkephalin) (Gerfen, 1984). These so-called “direct” and “indirect pathway” MSNs receive synaptic inputs from the thalamus and the cerebral cortex (Somogyi et al., 1981; Hersch et al., 1995; Sidibe and Smith, 1999; Lanciego et al., 2004; Lei et al., 2004, 2013; Huerta-Ocampo et al., 2013). In rats, the proportion of thalamic (vGluT2-positive) or cortical (vGluT1-positive) terminals in contact with direct or indirect pathway MSNs is very similar when considered as a population (Doig et al., 2010; Lei et al., 2013), but the total number of cortical terminals is higher than the number of thalamic boutons in contact with individual MSNs (Huerta-Ocampo et al., 2013). Tract-tracing studies in monkeys suggested that afferents from the CM preferentially innervate direct pathway MSNs in the putamen (Sidibe and Smith, 1996). However, because the CM/Pf (or Pf in rodents) has a unique pattern of synaptic connectivity in the striatum compared with other thalamic nuclei (Figures 1, 2), and because axonal tracers labeled only a subset of CM terminals in this study, the potentially preferential innervation of direct pathway neurons needs to be further assessed using vGluT2 or other more general marker of the CM/Pf-striatal system.
The convergence of thalamic and cortical inputs upon single MSNs is consistent with in vivo and in vitro electrophysiological analyses showing that single direct or indirect pathway neurons respond to both cortical and thalamic stimulation in rodents (Kocsis and Kitai, 1977; Vandermaelen and Kitai, 1980; Ding et al., 2008; Ellender et al., 2011, 2013; Huerta-Ocampo et al., 2013). Recent studies have suggested that thalamic inputs may gate corticostriatal transmission via regulation of striatal cholinergic interneurons, and that this interaction may modulate behavioral switching and attentional set-shifting (Kimura et al., 2004; Ding et al., 2010; Smith et al., 2011; Sciamanna et al., 2012; Bradfield et al., 2013a,b).
Relationships Between Thalamic or Cortical Terminals and Dopaminergic or Histaminergic Afferents
The modulation of excitatory inputs from the cerebral cortex by dopaminergic afferents from the substantia nigra pars compacta is central to our understanding of the functional properties of the basal ganglia. The post-synaptic cortical-induced excitatory responses are modulated by dopamine acting through a wide variety of pre- and/or post-synaptic mechanisms dependent on the type and localization of dopamine receptors and the physiological state of striatal MSNs (Gonon, 1997; Reynolds et al., 2001; Cragg and Rice, 2004; Surmeier et al., 2007; Rice and Cragg, 2008; Ma et al., 2012). Although the regulatory effects of dopamine on thalamic glutamatergic transmission have not been directly assessed, the similarity between the overall pattern of synaptic connectivity of non-CM/Pf thalamic and cortical terminals with MSNs (Moss and Bolam, 2008) suggests that these two pathways may be regulated in the same manner by nigrostriatal dopamine afferents (Figure 1). The dopaminergic modulation of corticostriatal transmission relies in part on the synaptic convergence of dopaminergic and cortical synapses on individual spines of striatal MSNs (Freund et al., 1984; Bolam and Smith, 1990; Smith et al., 1994) and/or pre-synaptic dopamine-mediated regulation of glutamate release from neighboring cortical terminals (Surmeier et al., 2007). Our recent quantitative ultrastructural analyses of rat tissue immunolabelled to reveal both dopaminergic axons and cortical (vGluT1-positive) or thalamic (vGluT2-positive) terminals in rats showed that both glutamatergic systems display the same structural relationships with dopaminergic afferents, i.e., all thalamic and cortical glutamatergic terminals are located within 1 µm of a dopaminergic synapse suggesting that synaptically released and spilled over dopamine may modulate most glutamatergic terminals in the rodent striatum (Arbuthnott et al., 2000; Arbuthnott and Wickens, 2007; Moss and Bolam, 2008; Rice and Cragg, 2008; but see Xu et al., 2012). However, it is unclear whether this general concept of interaction between dopaminergic and thalamostriatal afferents also applies to CM/Pf-striatal terminals that form axo-dendritic synapses. In light of our previous tracing studies which showed that axo-dendritic thalamic inputs from CM and dopaminergic terminals do not display significant structural relationships on the dendritic surface of striatal neurons (Smith et al., 1994), it is likely that the interactions between dopaminergic afferents and CM/Pf or non-CM/Pf thalamostriatal synapses differ (Figure 1).
Glutamatergic inputs from the cerebral cortex and thalamus to both direct and indirect pathway MSNs are also modulated pre-synaptically by histamine (Ellender et al., 2011). Histaminergic projections to the striatum that originate in the hypothalamus negatively modulate corticostriatal and thalamostriatal transmission through histamine H3 receptors.
Afferent Connections of Center Median/Parafascicular (CM/Pf)
In addition to the massive GABAergic projections from the GPi and SNr (see above), the CM receives inputs from motor, premotor and somatosensory cortices (Mehler, 1966; Kuypers and Lawrence, 1967; Kunzle, 1976, 1978; Catsman-Berrevoets and Kuypers, 1978; DeVito and Anderson, 1982), while the Pf is the main target of the frontal and supplementary eye fields (Huerta et al., 1986; Leichnetz and Goldberg, 1988) and associative areas of the parietal cortex (Ipekchyan, 2011). The CM/Pf complex also receives significant afferents from various subcortical sources, including the superior colliculus (Grunwerg et al., 1992; Redgrave et al., 2010), the pedunculopontine tegmental nucleus (Pare et al., 1988; Parent et al., 1988; Barroso-Chinea et al., 2011), the cerebellum (Royce et al., 1991; Ichinohe et al., 2000), the raphe nuclei, the locus coeruleus (Lavoie and Parent, 1991; Royce et al., 1991; Vertes et al., 2010), and from the mesencephalic, pontine and medullary reticular formation (Comans and Snow, 1981; Steriade and Glenn, 1982; Hallanger et al., 1987; Cornwall and Phillipson, 1988; Vertes and Martin, 1988; Royce et al., 1991; Newman and Ginsberg, 1994).
Physiology of the Thalamostriatal Projections
In Vivo Recording Studies
Experiments to study the role of the projections from the CM/Pf to the striatum date back to the 1970s. These studies described that electrical stimulation of the intralaminar nuclei induces short-latency excitatory post-synaptic potentials (EPSPs) in anesthetized cats and rats (Kocsis and Kitai, 1977; Vandermaelen and Kitai, 1980), confirming the existence of a direct glutamatergic thalamostriatal connection. Later, Wilson and colleagues demonstrated that these responses occurred in striatal MSNs (Wilson et al., 1983) and in cholinergic interneurons (Wilson et al., 1990). In addition to short-latency EPSPs, both types of neurons also showed prolonged inhibitions, or long-latency excitations (Wilson et al., 1983, 1990), indicating that the thalamic stimulation also engaged polysynaptic pathways.
In more recent studies in awake monkeys, we carried out extracellular recordings in the striatum during electrical stimulation of the CM/Pf complex (Nanda et al., 2009; Figure 3). We found that striatal cells did not respond to single-pulse stimulation, but that many neurons showed long-latency (tens of milliseconds) responses to burst stimulation (100 Hz, 1 s) of the CM/Pf. While phasically active neurons (PANs), which likely correspond to striatal MSNs, responded mainly with increases in firing, tonically active striatal neurons (TANs, likely to be cholinergic interneurons) often showed combinations of increases and decreases in firing (Nanda et al., 2009; Figure 3). Both types of responses were most likely generated by activation of the intrastriatal circuitry. As mentioned above, anatomical observations support the idea that CM/Pf terminals contact both striatal GABAergic and cholinergic elements in primates and non-primates (Sidibe and Smith, 1999), and that cholinergic interneurons receive GABAergic synaptic inputs from collaterals of direct and indirect pathway MSNs (Gonzales et al., 2013). These collaterals may mediate the decreases in firing after the activation of the presumably excitatory thalamostriatal projections.
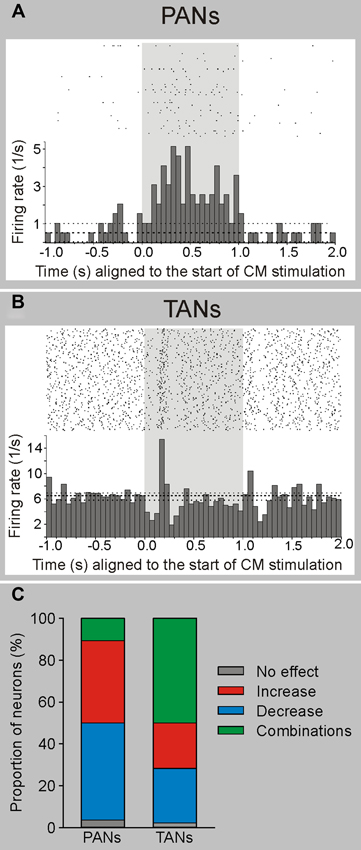
Figure 3. Electrophysiological responses of striatal neurons to electrical stimulation of CM in awake monkeys. Electrical stimulation (100 Hz, 100 pulses-shaded area) of CM evokes responses in PANs (putatively MSNs) and TANs (putatively cholinergic interneurons) in awake rhesus monkeys. (A) Example of a PAN responding with increased firing to electrical CM stimulation. (B) Example of a TAN responding with a brief decrease followed by an increase in firing to CM stimulation. The histograms and rasters are aligned to the start of stimulation trains. (C) Summary of responses. The majority of PANs display increases or decreases in firing rate, while most TANs present combinatory (increases and decreases) responses following CM stimulation (see Nanda et al., 2009 for more details).
The eventual effects of CM stimulation on the striatal circuitry may depend on the experimental conditions chosen. For instance, pharmacological stimulation of the Pf in rats, or electrical stimulation of the CM/Pf in monkeys, was shown to reduce striatal acetylcholine levels, an effect that can be reversed by intrastriatal administration of GABAA receptor antagonists (Zackheim and Abercrombie, 2005; Nanda et al., 2009). The reduction in acetylcholine levels could be explained assuming that the thalamic activation drives intrastriatal GABAergic neurons that then secondarily inhibit cholinergic interneurons. However, other studies showed that electrical stimulation of Pf increased the level of acetylcholine in the rat striatum (Consolo et al., 1996a) in an NMDA-receptor dependent manner.
In Vitro Recordings in Brain Slices
A new rat brain slice preparation that partly preserved thalamostriatal axons (Smeal et al., 2007) has enabled studies of the chemical and functional properties of thalamostriatal synapses and the potential relationships between thalamostriatal and corticostriatal systems in normal state (Ding et al., 2008; Smeal et al., 2008). Using this preparation, the ratio of NMDA/non-NMDA glutamatergic receptors was found to be higher at thalamic than cortical synapses (Ding et al., 2008; Smeal et al., 2008), an observation that extends earlier neurochemical studies in adult rats (Baldi et al., 1995; Consolo et al., 1996a,b). This slice preparation has also lead to additional data suggesting that the thalamostriatal system gates corticostriatal signaling via activation of striatal cholinergic interneurons, and that this functional interaction might be altered in mouse model of dystonia (Ding et al., 2008; Sciamanna et al., 2012). However, it is a limitation of this preparation that thalamostriatal projections from CM/Pf cannot be distinguished from those originating in other parts of the thalamus.
The introduction of optogenetic methods helped to further characterize the properties of specific thalamostriatal synapses in rats (Ellender et al., 2013). Thus, neurons in the central lateral nucleus (CL) have bushy, frequently branching, dendrites and, under anesthesia, fire action potentials in the form of low-threshold Ca2+ spike bursts, while Pf neurons have long, infrequently branching dendrites and give rise to action potentials that are only rarely in the form of low-threshold bursts (Lacey et al., 2007; Figure 2). In the striatum, thalamostriatal terminals from the CL terminate almost exclusively on dendritic spines, while Pf boutons target predominantly dendritic shafts (Figure 2). In vitro optogenetic activation of the different pathways combined with whole-cell, patch-clamp recordings of direct or indirect pathway MSNs in adult mice (Ellender et al., 2013) revealed that stimulation of CL synapses leads to large amplitude, predominantly AMPA-receptor mediated, excitatory responses that display short-term facilitation. In contrast, stimulation of Pf synapses gives rise to small amplitude responses that display short-term depression and are largely mediated by post-synaptic NMDA receptors (Ellender et al., 2013; Figure 4). The high frequency Ca2+ spike bursts in CL neurons together with the synaptic properties of CL thalamostriatal synapses suggests that thalamic inputs from CL are well suited to driving MSNs to depolarization and hence firing (Ellender et al., 2013). In contrast, the firing characteristics and properties of Pf neurons and their thalamostriatal synapses suggest that these are better suited to exert modulatory effects on striatal MSNs, which could be in the form of facilitating Ca2+-dependent processes. Furthermore, pairing Pf pre-synaptic stimulation with action potentials in MSNs leads to NMDA receptor- and Ca2+-dependent long-term depression at these synapses (Ellender et al., 2013; Figure 4).
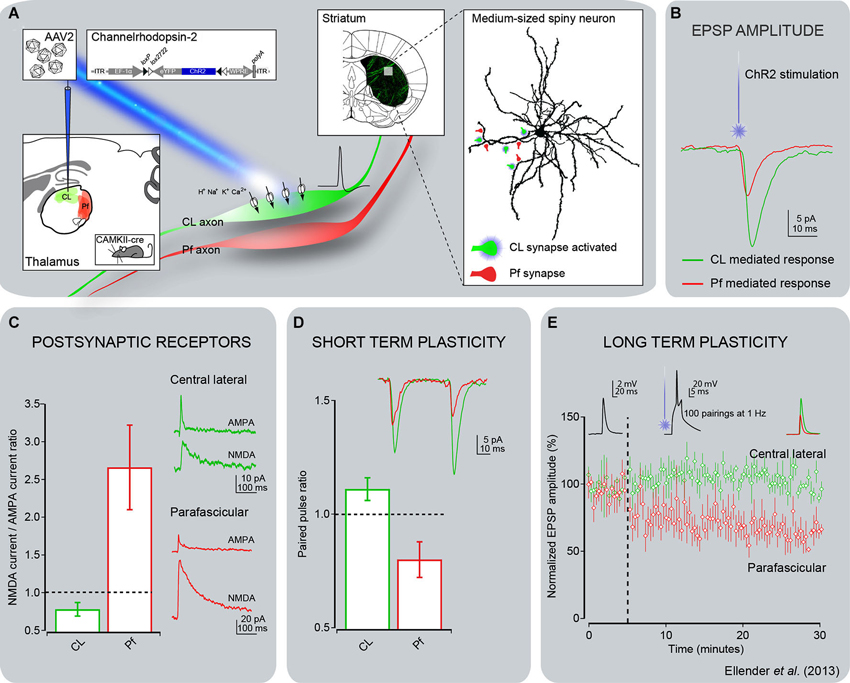
Figure 4. Electrophysiological responses of striatal MSNs to optogenetic activation of thalamostriatal terminals from CL or Pf in mice. (A) Channelrhodopsin-2 (ChR2) was delivered to either the CL or Pf thalamic nucleus using a stereotaxic injection of adeno-associated virus containing the double-floxed sequence for ChR2-YFP in CAMKII-cre mice. This approach enabled expression of ChR2 in the excitatory thalamic neurons of either the CL or Pf nucleus including in their axonal arbor. Thalamic axons expressing ChR2-YFP were readily visible in acute striatal slices and this method allowed for activation of only those synapses originating from neurons from the injected thalamic nucleus by illumination of these slices with blue (473 nm) laser or LED light. (B) Whole-cell patch-clamp recordings of striatal MSNs showed that activation of CL synapses led to consistently larger post-synaptic responses than activation of Pf synapses. (C) Detailed investigation of the glutamate receptor-mediated currents revealed the CL synapses exhibit predominantly AMPA receptor-mediated currents in response to light activation and the Pf synapses exhibit predominantly NMDA receptor-mediated currents. (D) The short term plastic properties of these synapses were investigated by repetitive activation of the synapses in close succession. This revealed that inputs from CL exhibit short term facilitation, in which the response to the second activation has a larger amplitude than the response to the first activation, whereas inputs from Pf exhibit short term depression. (E) The long term plastic properties of these synapses were investigated using a spike timing-dependent plasticity protocol, consisting of the pairing of optical activation of a pre-synaptic thalamic input with a post-synaptic action potential in a MSN. This protocol induced a clear long term depression of synaptic efficacy at Pf synapses, but no plasticity was observed at CL synapses. The same observation was made using either a pre-post or post-pre pairing, with only the pre-post pairing shown for clarity (see Ellender et al., 2013 for more details).
The Role of the Center Median/Parafascicular (CM/Pf) Thalamostriatal System in Cognition
The CM/Pf-striatal system is now thought to be critical in mediating basal ganglia responses to attention-related stimuli, and may be engaged in behavioral switching and reinforcement functions (Kimura et al., 2004; Minamimoto et al., 2009; Smith et al., 2011; Bradfield et al., 2013a).
Responses of Center Median/Parafascicular (CM/Pf) Neurons in Attention-Related Tasks
Because the intralaminar nuclei receive massive ascending projections from the reticular formation and various brainstem regions (see above), and have long been known as the source(s) of widely distributed “nonspecific” thalamocortical projections, these thalamic nuclei are considered part of the ascending “reticular activating system” that regulates arousal and attention (as reviewed in Van der Werf et al., 2002). In line with this concept, functional imaging studies in humans demonstrated a significant increase of activity in CM/Pf during processing of attention-related stimuli (Kinomura et al., 1996; Hulme et al., 2010; Metzger et al., 2010). More recent observations in primates showed that CM and Pf neurons respond to behaviorally salient visual, auditory and somatosensory stimuli (Matsumoto et al., 2001; Minamimoto and Kimura, 2002; Minamimoto et al., 2005; Figure 5). In these studies, the response latencies of Pf neurons were much shorter than those of CM neurons (Figure 5). Compatible with the view that responses of CM/Pf neurons to external events are related to attention, the initially vigorous responses fade quickly upon repeated stimulus presentation if stimuli were not followed by reward, and, thus, lose their salience (Matsumoto et al., 2001; Minamimoto and Kimura, 2002; Kimura et al., 2004; Minamimoto et al., 2005). Acute pharmacological inactivation of Pf in monkeys disrupts attention processing more efficiently than CM inactivation (Minamimoto and Kimura, 2002). The functional responses of CM/Pf neurons to attention-related stimuli thus suggest a role of the CM/Pf-striatal system in cognition, most particularly related to attention shifting, behavior switching and reinforcement processes (Matsumoto et al., 2001; Minamimoto and Kimura, 2002; Kimura et al., 2004; Minamimoto et al., 2005; Smith et al., 2011; Bradfield et al., 2013a,b). There is also evidence that sensory-responsive CM neurons may be involved in mechanisms needed for decision-making and biasing actions (Minamimoto et al., 2005).
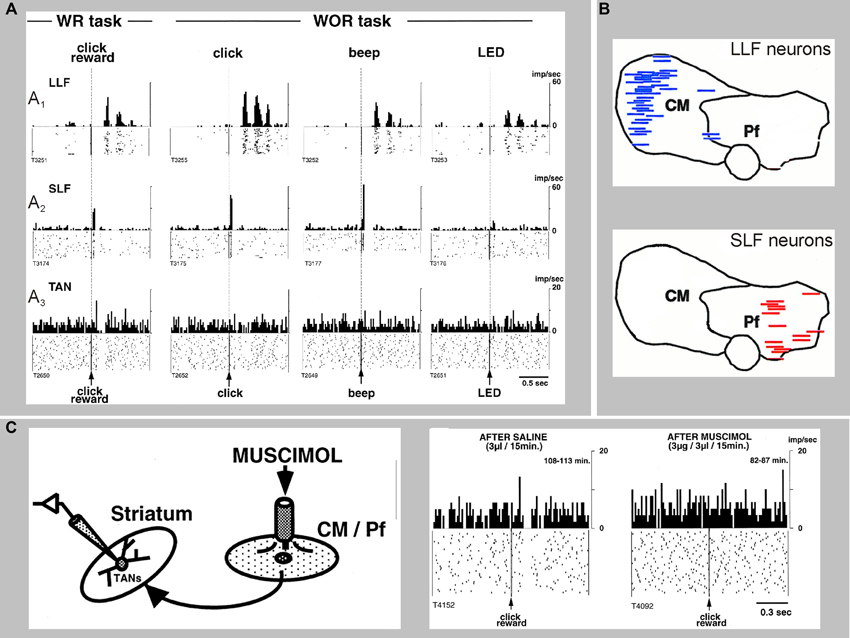
Figure 5. Sensory responses of two types of CM/Pf neurons, and a striatal TAN recorded during the presentation of a stimulus with or without reward (WR vs. WOR). The spike rasters and histograms are aligned to the time of presentation of the stimulus. (A1) Representative activity of a CM neuron with long-latency facilitation (LLF) following stimulus presentation. (A2) Activity of a Pf neuron showing short-latency facilitation (SLF) after stimulus. (A3) Activity of a TAN. Note that thalamic responses occur in both WR and WOR tasks, whereas TAN responses occur only in the WR task. (B) Localization of LLF and SLF neurons in the monkey CM/Pf complex. Note the complete segregation of these two populations of neurons in CM or Pf. (C) Application of the GABAA receptor agonist muscimol in CM/Pf alters the pattern of responses of striatal TANs to the presentation of reward-related sensory stimuli in awake monkeys, The rasters and histograms on the right demonstrate that the pauses in the TAN responses are abolished after application of muscimol in CM/Pf (reproduced with permission from Matsumoto et al., 2001).
Additional evidence for a “cognitive” role of the CM/Pf projections to the striatum comes from studies in mice in which selective immunotoxin lesions or pharmacological inactivation of the Pf-striatal projection, impair performance in a discrimination learning task (Brown et al., 2010; Kato et al., 2011). Furthermore, recent evidence indicates the Pf projection to the posterior dorsomedial striatum is involved in regulating the interaction between new and previously learned stimuli (Bradfield et al., 2013a,b). It is noteworthy that neither lesion nor pharmacological inactivation of CM/Pf in monkeys or rodents lead to motor impairments (Matsumoto et al., 2001; Minamimoto and Kimura, 2002; Brown et al., 2010; Kato et al., 2011).
Center Median/Parafascicular (CM/Pf)-Striatal System Regulation of Striatal Cholinergic Interneurons
Reward-associated events evoke pause responses in striatal TANs (which are likely to be cholinergic interneurons) (Goldberg and Reynolds, 2011). These responses are regulated in part by the CM/Pf-striatal system, because they are almost completely abolished by chemical inactivation of the CM/Pf complex in monkeys (Matsumoto et al., 2001; Figure 5). Furthermore, the removal of Pf inputs to cholinergic interneurons reduces the firing rate of these neurons and produces an enduring deficit in goal-directed learning (Bradfield et al., 2013a). These observations are consistent with the fact that cholinergic interneurons receive synaptic inputs from CM/Pf (Lapper and Bolam, 1992; Sidibe and Smith, 1999), that CM stimulation strongly affects TAN activity patterns (Wilson et al., 1990; Nanda et al., 2009) and that CM/Pf alterations affect striatal acetylcholine release (Consolo et al., 1996a,b; Zackheim and Abercrombie, 2005; Nanda et al., 2009). As mentioned above, several mechanisms have been proposed to explain how activation of the glutamatergic CM/Pf-striatal projection evokes inhibitions or pauses in TAN firing, including the involvement of intercalated GABAergic and dopaminergic elements, as well as intrinsic properties of cholinergic interneurons (Ding et al., 2010; Goldberg and Reynolds, 2011; Sciamanna et al., 2012; Threlfell et al., 2012).
Degeneration of the Thalamostriatal System in Parkinson’s Disease (PD)
Postmortem studies have shown that 30–40% of CM/Pf neurons are lost in PD patients with mild motor deficits, and that the extent of CM/Pf degeneration does not further progress with the severity of the Parkinsonian motor signs (Xuereb et al., 1991; Heinsen et al., 1996; Henderson et al., 2000a,b,2005; Brooks and Halliday, 2009; Halliday, 2009). We have recently found a similarly robust loss of CM/Pf neurons in monkeys that were chronically treated with low doses of the neurotoxin 1-methyl-4-phenyl-1,2,3,6-tetrahydropyridine (MPTP), even in motor asymptomatic animals with minimal nigrostriatal dopaminergic denervation (Villalba et al., 2014; Figure 6). In PD patients and MPTP-treated monkeys, this thalamic degeneration predominantly affects CM/Pf (Henderson et al., 2000a,b; Villalba et al., 2014), although significant neuronal loss was also reported in the parataenial, cucullar and central lateral nuclei of PD patients (Halliday, 2009). In the brain of PD patients, α-synuclein deposition was found in the latter three nuclei, but not as much in CM/Pf (Brooks and Halliday, 2009). Parvalbumin-negative neurons are particularly affected in CM (Halliday, 2009). It is noteworthy that robust CM/Pf neuronal loss is not only found in PD, but has also been found in other neurodegenerative diseases, including progressive supranuclear palsy and Huntington’s disease (Heinsen et al., 1996; Henderson et al., 2000a,b). The cellular properties of CM/Pf neurons needs to be studied in more detail to determine the potential factors that make them more sensitive to degeneration than other thalamic cells in these diseases (see also below).
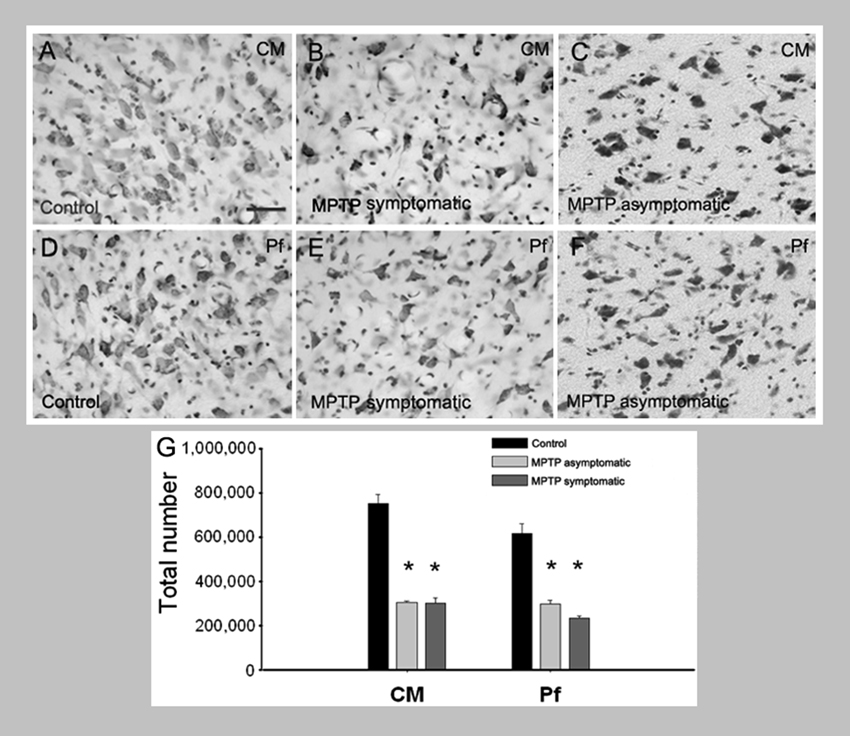
Figure 6. Loss of CM/Pf neurons in MPTP-treated monkeys. (A–G) Light micrographs of neurons in CM or Pf of control monkeys (A, D), MPTP-treated motor symptomatic (B, E) and MPTP-treated motor asymptomatic (C, F) animals. The motor asymptomatic animals had ~40% striatal dopamine loss and did not display any Parkinsonian motor symptoms, while the motor symptomatic monkeys had more than 80% striatal dopamine loss and displayed moderate to severe Parkinsonism. Note the significant reduction in neuronal density in CM and Pf of both symptomatic and asymptomatic MPTP-treated monkeys. (G) Stereological cell counts demonstrate a significant loss of neurons in both CM and Pf of the two groups of MPTP-treated monkeys compared with controls.
In rodent and primate models of PD, striatal dopamine loss is associated with a loss of glutamatergic synapses (Ingham et al., 1998; Villalba et al., 2013), which is consistent with a loss of spines on striatal MSNs in PD postmortem material (see below). Stereological estimates of the number of glutamatergic synapses formed by vGluT1- (marker of cortical terminals) or vGluT2- (marker of thalamic terminals) containing terminals in the putamen of MPTP-treated monkeys showed that the number of vGluT2-positive terminals, but not that of vGluT1-containing boutons, is substantially reduced in Parkinsonian animals (Villalba et al., 2013). This suggests that the loss of thalamic glutamatergic inputs to the striatum outweighs the loss of cortical terminals in MPTP-treated monkeys (Villalba et al., 2013). Because CM/Pf inputs to striatal MSNs predominantly terminate on dendritic shafts (see above), these findings are in line with our previous studies which had demonstrated that MPTP treatment reduces the relative prevalence of vGluT2-positive axo-dendritic synapses in the putamen more strongly than that of axo-spinous synapses (Raju et al., 2008). Together, these results support the hypothesis that the loss of CM/Pf inputs to the striatum prominently contributes to the glutamatergic deafferentation of MSNs in PD. It is not yet clear whether the loss of striatal inputs from CM/Pf also affects the glutamatergic innervation of cholinergic interneurons.
The loss of glutamatergic afferents is reflected in a profound loss of dendritic spines of striatal MSNs in PD patients (Stephens et al., 2005; Zaja-Milatovic et al., 2005), rodent models of PD (Ingham et al., 1989; Day et al., 2006; Kusnoor et al., 2009) and in MPTP-treated monkeys (Raju et al., 2008; Smith et al., 2009; Villalba et al., 2009, 2013; Villalba and Smith, 2010, 2011, 2013). In the latter studies, a 40–50% spine loss was found in the sensorimotor striatum, similar to findings in PD patients (Zaja-Milatovic et al., 2005; Villalba et al., 2009). The link between the aforementioned striatal glutamatergic deafferentation and spine loss remains uncertain. For instance, it remains unclear whether the CM/Pf degeneration and the resulting loss of glutamatergic inputs to the striatum contribute to the changes in the number and morphology of dendritic spines in PD. As a further complication, the use of dopaminergic drugs can also affect spine growth and morphology. Thus, a recent study showed aberrant restoration of axo-spinous synapses that affect corticostriatal, but not thalamostriatal synapses, in unilaterally 6-hydroxydopamine (OHDA)-lesioned rats that developed L-3,4-didroxyphenylalanine (L-DOPA)-induced dyskinesias (Zhang et al., 2013), suggesting a differential impairment of the two glutamatergic systems in dyskinesia. Details about the extent of Pf neuronal loss in these animals are needed to translate these findings to human PD patients with L-DOPA-induced dyskinesia.
Potential Reasons for Center Median/Parafascicular (CM/Pf) Neuron Loss in PD
Although it remains unclear why CM/Pf neurons are particularly sensitive to neurodegeneration in PD and other disorders (Halliday et al., 2005; Halliday, 2009), their chemical phenotype and sensitivity to chronic MPTP administration in non-human primates (Villalba et al., 2014) may provide clues. The CM/Pf nuclei do not carry a specifically high level of α-synuclein deposits in PD patients (Brooks and Halliday, 2009), so that their vulnerability in PD cannot be explained by the burden of α-synuclein aggregation.
In rodents, striatal terminals from the Pf express immunoreactivity for the protein cerebellin 1, a neurochemical feature that appears to be specific for the CM/Pf-striatal system, and may also regulate the morphology of striatal spines (Kusnoor et al., 2010). It remains to be established whether this unique molecular characteristic amongst thalamic neurons contributes to their susceptibility. It is also possible that the absence of calcium binding proteins expression in CM/Pf neurons determines their differential vulnerability. In humans, postmortem studies have shown that subpopulations of parvalbumin-containing neurons are mainly affected in Pf, while non-parvalbumin/non-calbindin neurons are more specifically targeted in CM (Henderson et al., 2000a). In MPTP-treated animals, the degeneration of CM/Pf could instead be due to direct toxic effects of the MPTP metabolite, 1-methyl-4-phenylpyridinium (MPP+), on the thalamus, independent of its effects on the nigrostriatal system (Villalba et al., 2014). Consistent with this possibility, injections of MPP+ into the rodent striatum resulted in a major neuronal loss in Pf, without significant effects on cortical neurons (Ghorayeb et al., 2002).
The Center Median/Parafascicular (CM/Pf) as a Target of Neurosurgical Interventions in Brain Disorders
Although the physiological properties of the caudal intralaminar nuclei and their projections remain poorly characterized, these nuclei have been used as targets for surgical interventions, aimed at treating pain, seizures, impairments of consciousness, or movement disorders. We will focus our discussion on the use of neurosurgical procedures as treatment of movement disorders, because this use is most easily linked to the interactions between CM/Pf and the basal ganglia. These procedures have been used specifically in patients with disabling TS, or with PD. The mechanisms of action of CM/Pf interventions in these diseases, and the specifics of the optimal surgical approach and DBS characteristics remain matters of speculation. Furthermore, inclusion and exclusion criteria for trials of these interventions are only beginning to emerge for TS patients, while no formal criteria have yet been developed for trials in patients with PD.
Ablative Surgeries of Center Median/Parafascicular (CM/Pf)
Since the 1960s, unilateral or bilateral lesions of the intralaminar and medial thalamic nuclei, as well as the nucleus ventro-oralis internus (Voi) have been empirically used to treat patients with TS (see below) (Hassler and Dieckmann, 1970, 1973; de Divitiis et al., 1977; Hassler, 1982). These studies have reported impressive reductions in tic frequency in these patients, along with a lesser reversal of compulsive symptoms. The effects of CM/Pf lesions in other movement disorders (such as Parkinsonism) have not been extensively characterized. However, in rodents, Pf lesions were shown to prevent the neurochemical changes produced by dopamine denervation in different basal ganglia nuclei (Kerkerian-Le Goff et al., 2009). Similar experiments in MPTP-treated primates have not resulted in significant anti-parkinsonian effects (Lanciego et al., 2008).
CM/Pf Deep Brain Stimulation and TS
TS is a neuropsychiatric disorder of childhood onset. Patients develop rapid, stereotyped movements (tics) which typically peak in preadolescence and decline in the later teenage years. Many TS patients also suffer from psychiatric comorbidities, such as obsessive compulsive disorder, attention-deficit hyperactivity disorder, or depression. Most patients are successfully (albeit partially) treated with neuroleptics and other drugs or with behavioral therapy. However, a few patients continue to experience severe tics in adulthood. These patients are candidates for neurosurgical procedures. Although still under considerable debate, TS may be the result of abnormal GABAergic or dopaminergic transmission in the basal ganglia (see, e.g., Buse et al., 2013; Worbe et al., 2013), involving both motor and limbic basal ganglia-thalamocortical circuitry.
Although there is little evidence linking the pathology or functional disturbances of CM/Pf to TS, this nuclear complex has been a major focus of surgical treatment of this condition, mostly because of the early empirical evidence with ablative treatments (see above). Early investigations of the use of stimulation of CM/Pf in movement disorders were carried out in patients that were enrolled in pain treatment studies, but also suffered from movement disorders (Andy, 1980; Krauss et al., 2002). Significant symptomatic improvements of motor dysfunctions were reported in these studies, but the stimulation parameters were not communicated. Since then, impressive reductions in tic frequency and severity, perhaps with greater effectiveness against motor than vocal tics, have been reported, although the number of TS patients treated with CM/Pf DBS remains small (Visser-Vandewalle et al., 2003, 2004, 2006; Temel and Visser-Vandewalle, 2004; Houeto et al., 2005; Ackermans et al., 2006, 2008, 2010, 2011; Bajwa et al., 2007; Maciunas et al., 2007; Servello et al., 2008, 2010; Shields et al., 2008; Porta et al., 2009; Hariz and Robertson, 2010; Sassi et al., 2011; Maling et al., 2012; Savica et al., 2012; Visser-Vandewalle and Kuhn, 2013). The time course of tic improvement varies between individuals, ranging from immediate effects (Visser-Vandewalle et al., 2003; Maciunas et al., 2007) to a more protracted time course (Maciunas et al., 2007; Servello et al., 2008). In addition to the motor symptoms of the disease, CM/Pf DBS also effectively alleviated some of the psychiatric components of TS, including obsessive-compulsive behaviors and anxiety (Houeto et al., 2005; Mink, 2006; Visser-Vandewalle et al., 2006; Neuner et al., 2009; Krack et al., 2010; Sassi et al., 2011). The mechanisms of action of CM/Pf stimulation on TS signs and symptoms remain unclear, but the anatomy and potential role of the thalamostriatal system from CM/Pf in cognition, as well as functional studies indicating the key role of CM/Pf in regulating striatal cholinergic interneurons activity (see above) in animals, suggest that CM/Pf stimulation may mediate its effects through complex regulation of striatal microcircuits that influence both motor and non-motor basal ganglia-thalamocortical and thalamostriatal networks (Nanda et al., 2009; Kim et al., 2013).
Center Median/Parafascicular (CM/Pf) DBS and Parkinson’s Disease (PD)
CM/Pf DBS was found to provide significant anti-parkinsonian benefits in 6-OHDA-treated rats (Jouve et al., 2010). CM/Pf DBS has also been used in a few PD patients. These studies have suggested that CM/Pf DBS may have anti-dyskinetic effects and reduce freezing of gait, a symptom that is not satisfactorily treated with either medications or conventional DBS approaches directed at subthalamic and pallidal targets (Caparros-Lefebvre et al., 1999; Mazzone et al., 2006). More recent studies have suggested that CM/Pf DBS may also reduce Parkinsonian tremor (Peppe et al., 2008; Stefani et al., 2009).
Concluding Remarks and Future Studies
Despite significant progress in our understanding of the anatomy, physiology and pathophysiology of the thalamostriatal systems, many unresolved issues remain. The cloning of vGluT1 and vGluT2 has had a significant impact in our understanding of the anatomical and synaptic organization of the thalamostriatal systems, allowing us to further appreciate that the thalamus is a massive source of extrinsic glutamatergic inputs to the striatum that originates either in the CM/Pf nuclear complex, or in the numerous non-CM/Pf thalamic nuclei. Future studies aimed at understanding the physiological role of these multiple thalamostriatal circuits, and their functional interactions with the corticostriatal and nigrostriatal systems, are warranted to decipher the mechanisms by which these extrinsic afferents regulate basal ganglia functions.
Because of the complex relationships between the cerebral cortex, thalamus and striatum, the use of traditional stimulation or lesion methods has had a limited impact in our understanding of the physiology and synaptic properties of thalamostriatal connections. However, as presented in this review, optogenetic approaches help overcome some of these technical challenges, setting the stage for a rigorous and detailed characterization of the physiology and pathophysiology of the CM/Pf vs. non-CM/Pf-striatal systems in normal and diseased states.
The major breakthroughs that were recently made in characterizing some aspects of the role of the CM/Pf-striatal system in regulating the physiological responses of striatal cholinergic interneurons to attention-related salient sensory stimuli provide a deeper understanding of the mechanisms involved by which the basal ganglia regulate activities such as behavioral switching, attentional set-shifting and reinforcement. Because the CM/Pf-striatal system undergoes massive degeneration in PD, future studies aimed at assessing the effects of this degeneration upon attention and other basal ganglia-related cognitive functions are needed. A better understanding of the respective role played by the thalamostriatal vs. the nigrostriatal dopamine systems in the regulation of cholinergic interneuron activity is another area of great interest for future studies.
In light of neuropathology studies of the thalamus in human patients with PD (Halliday, 2009), combined with studies in MPTP-treated non-human primates, it appears that CM/Pf neurons are particularly sensitive to degeneration in PD, neurodegenerative diseases, and neurotoxic insults. Thus, future studies aimed at elucidating the chemical, physiological and pharmacological properties of CM/Pf neurons vs. other thalamic cells are essential to determine the basis for the selective vulnerability of CM/Pf neurons in brain disorders.
Finally, another area of great interest is the use of DBS of CM/Pf in movement disorders, most particularly in TS. We need to understand better how stimulation of the thalamostriatal system from CM/Pf alleviates tics and psychiatric symptoms of this disease. Furthermore, rigorous blinded trials in a large number of patients still need to be done before this treatment can be recommended for patients with TS.
Conflict of Interest Statement
The authors declare that the research was conducted in the absence of any commercial or financial relationships that could be construed as a potential conflict of interest.
References
Ackermans, L., Duits, A., Temel, Y., Winogrodzka, A., Peeters, F., Beuls, E. A., et al. (2010). Long-term outcome of thalamic deep brain stimulation in two patients with Tourette syndrome. J. Neurol. Neurosurg. Psychiatry 81, 1068–1072. doi: 10.1136/jnnp.2009.176859
Ackermans, L., Duits, A., Van Der Linden, C., Tijssen, M., Schruers, K., Temel, Y., et al. (2011). Double-blind clinical trial of thalamic stimulation in patients with Tourette syndrome. Brain 134, 832–844. doi: 10.1093/brain/awq380
Ackermans, L., Temel, Y., Cath, D., Van Der Linden, C., Bruggeman, R., Kleijer, M., et al. (2006). Deep brain stimulation in Tourette’s syndrome: two targets? Mov. Disord. 21, 709–713. doi: 10.1002/mds.20816
Ackermans, L., Temel, Y., and Visser-Vandewalle, V. (2008). Deep brain stimulation in Tourette’s Syndrome. Neurotherapeutics 5, 339–344. doi: 10.1016/j.nurt.2008.01.009
Alexander, G. E., Delong, M. R., and Strick, P. L. (1986). Parallel organization of functionally segregated circuits linking basal ganglia and cortex. Annu. Rev. Neurosci. 9, 357–381. doi: 10.1146/annurev.neuro.9.1.357
Alloway, K. D., Smith, J. B., and Watson, G. D. (2014). Thalamostriatal projections from the medial posterior and parafascicular nuclei have distinct topographic and physiologic properties. J. Neurophysiol. 111, 36–50. doi: 10.1152/jn.00399.2013
Andy, O. J. (1980). Parafascicular-center median nuclei stimulation for intractable pain and dyskinesia (painful-dyskinesia). Appl. Neurophysiol. 43, 133–144.doi: 10.1159/000102247
Arbuthnott, G. W., and Wickens, J. (2007). Space, time and dopamine. Trends Neurosci. 30, 62–69. doi: 10.1016/j.tins.2006.12.003
Arbuthnott, G. W., Ingham, C. A., and Wickens, J. R. (2000). Dopamine and synaptic plasticity in the neostriatum. J. Anat. 196, 587–596. doi: 10.1046/j.1469-7580.2000.19640587.x
Bajwa, R. J., De Lotbiniere, A. J., King, R. A., Jabbari, B., Quatrano, S., Kunze, K., et al. (2007). Deep brain stimulation in Tourette’s syndrome. Mov. Disord. 22, 1346–1350. doi: 10.1002/mds.21398
Baldi, G., Russi, G., Nannini, L., Vezzani, A., and Consolo, S. (1995). Trans-synaptic modulation of striatal ACh release in vivo by the parafascicular thalamic nucleus. Eur. J. Neurosci. 7, 1117–1120. doi: 10.1111/j.1460-9568.1995.tb01100.x
Barroso-Chinea, P., Castle, M., Aymerich, M. S., and Lanciego, J. L. (2008). Expression of vesicular glutamate transporters 1 and 2 in the cells of origin of the rat thalamostriatal pathway. J. Chem. Neuroanat. 35, 101–107. doi: 10.1016/j.jchemneu.2007.08.001
Barroso-Chinea, P., Rico, A. J., Conte-Perales, L., Gomez-Bautista, V., Luquin, N., Sierra, S., et al. (2011). Glutamatergic and cholinergic pedunculopontine neurons innervate the thalamic parafascicular nucleus in rats: changes following experimental parkinsonism. Brain Struct. Funct. 216, 319–330. doi: 10.1007/s00429-011-0317-x
Beckstead, R. M. (1984). The thalamostriatal projection in the cat. J. Comp. Neurol. 223, 313–346. doi: 10.1002/cne.902230302
Berendse, H. W., and Groenewegen, H. J. (1990). Organization of the thalamostriatal projections in the rat, with special emphasis on the ventral striatum. J. Comp. Neurol. 299, 187–228. doi: 10.1002/cne.902990206
Berendse, H. W., and Groenewegen, H. J. (1991). Restricted cortical termination fields of the midline and intralaminar thalamic nuclei in the rat. Neuroscience 42, 73–102. doi: 10.1016/0306-4522(91)90151-d
Bolam, J. P., and Smith, Y. (1990). The GABA and substance P input to dopaminergic neurones in the substantia nigra of the rat. Brain Res. 529, 57–78. doi: 10.1016/0006-8993(90)90811-o
Bostan, A. C., Dum, R. P., and Strick, P. L. (2010). The basal ganglia communicate with the cerebellum. Proc. Natl. Acad. Sci. U S A 107, 8452–8456. doi: 10.1073/pnas.1000496107
Bostan, A. C., and Strick, P. L. (2010). The cerebellum and basal ganglia are interconnected. Neuropsychol. Rev. 20, 261–270. doi: 10.1007/s11065-010-9143-9
Bostan, A. C., Dum, R. P., and Strick, P. L. (2013). Cerebellar networks with the cerebral cortex and basal ganglia. Trends Cogn. Sci. 17, 241–254. doi: 10.1016/j.tics.2013.03.003
Bradfield, L. A., Bertran-Gonzalez, J., Chieng, B., and Balleine, B. W. (2013a). The thalamostriatal pathway and cholinergic control of goal-directed action: interlacing new with existing learning in the striatum. Neuron 79, 153–166. doi: 10.1016/j.neuron.2013.04.039
Bradfield, L. A., Hart, G., and Balleine, B. W. (2013b). The role of the anterior, mediodorsal and parafascicular thalamus in instrumental conditioning. Front. Syst. Neurosci. 7:51. doi: 10.3389/fnsys.2013.00051
Brooks, D., and Halliday, G. M. (2009). Intralaminar nuclei of the thalamus in lewy body diseases. Brain Res. Bull. 78, 97–104. doi: 10.1016/j.brainresbull.2008.08.014
Brown, H. D., Baker, P. M., and Ragozzino, M. E. (2010). The parafascicular thalamic nucleus concomitantly influences behavioral flexibility and dorsomedial striatal acetylcholine output in rats. J. Neurosci. 30, 14390–14398. doi: 10.1523/jneurosci.2167-10.2010
Buse, J., Schoenefeld, K., Munchau, A., and Roessner, V. (2013). Neuromodulation in Tourette syndrome: dopamine and beyond. Neurosci. Biobehav. Rev. 37, 1069–1084. doi: 10.1016/j.neubiorev.2012.10.004
Butler, A. B. (1994). The evolution of the dorsal pallium in the telencephalon of amniotes: cladistic analysis and a new hypothesis. Brain Res. Brain Res. Rev. 19, 66–101. doi: 10.1016/0165-0173(94)90004-3
Calderon, D. P., Fremont, R., Kraenzlin, F., and Khodakhah, K. (2011). The neural substrates of rapid-onset Dystonia-Parkinsonism. Nat. Neurosci. 14, 357–365. doi: 10.1038/nn.2753
Caparros-Lefebvre, D., Blond, S., Feltin, M. P., Pollak, P., and Benabid, A. L. (1999). Improvement of levodopa induced dyskinesias by thalamic deep brain stimulation is related to slight variation in electrode placement: possible involvement of the centre median and parafascicularis complex. J. Neurol. Neurosurg. Psychiatry 67, 308–314. doi: 10.1136/jnnp.67.3.308
Castle, M., Aymerich, M. S., Sanchez-Escobar, C., Gonzalo, N., Obeso, J. A., and Lanciego, J. L. (2005). Thalamic innervation of the direct and indirect basal ganglia pathways in the rat: Ipsi- and contralateral projections. J. Comp. Neurol. 483, 143–153. doi: 10.1002/cne.20421
Catsman-Berrevoets, C. E., and Kuypers, H. G. (1978). Differential laminar distribution of corticothalamic neurons projecting to the VL and the center median. An HRP study in the cynomolgus monkey. Brain Res. 154, 359–365. doi: 10.1016/0006-8993(78)90706-0
Comans, P. E., and Snow, P. J. (1981). Ascending projections to nucleus parafascicularis of the cat. Brain Res. 230, 337–341. doi: 10.1016/0006-8993(81)90411-x
Consolo, S., Baldi, G., Giorgi, S., and Nannini, L. (1996a). The cerebral cortex and parafascicular thalamic nucleus facilitate in vivo acetylcholine release in the rat striatum through distinct glutamate receptor subtypes. Eur. J. Neurosci. 8, 2702–2710. doi: 10.1111/j.1460-9568.1996.tb01565.x
Consolo, S., Baronio, P., Guidi, G., and Di Chiara, G. (1996b). Role of the parafascicular thalamic nucleus and N-methyl-D-aspartate transmission in the D1-dependent control of in vivo acetylcholine release in rat striatum. Neuroscience 71, 157–165. doi: 10.1016/0306-4522(95)00421-1
Cornwall, J., and Phillipson, O. T. (1988). Afferent projections to the parafascicular thalamic nucleus of the rat, as shown by the retrograde transport of wheat germ agglutinin. Brain Res. Bull. 20, 139–150. doi: 10.1016/0361-9230(88)90171-2
Cowan, W. M., and Powell, T. P. (1956). A study of thalamo-striate relations in the monkey. Brain 79, 364–390. doi: 10.1093/brain/79.2.364
Cragg, S. J., and Rice, M. E. (2004). DAncing past the DAT at a DA synapse. Trends Neurosci. 27, 270–277. doi: 10.1016/j.tins.2004.03.011
Crittenden, J. R., and Graybiel, A. M. (2011). Basal Ganglia disorders associated with imbalances in the striatal striosome and matrix compartments. Front. Neuroanat. 5:59. doi: 10.3389/fnana.2011.00059
Day, M., Wang, Z., Ding, J., An, X., Ingham, C. A., Shering, A. F., et al. (2006). Selective elimination of glutamatergic synapses on striatopallidal neurons in Parkinson disease models. Nat. Neurosci. 9, 251–259. doi: 10.1038/nn1632
de Divitiis, E., D’errico, A., and Cerillo, A. (1977). Stereotactic surgery in Gilles de la Tourette syndrome. Acta Neurochir. (Wien) (Suppl. 24), 73. doi: 10.1007/978-3-7091-8482-0_12
Deschenes, M., Bourassa, J., Doan, V. D., and Parent, A. (1996a). A single-cell study of the axonal projections arising from the posterior intralaminar thalamic nuclei in the rat. Eur. J. Neurosci. 8, 329–343. doi: 10.1111/j.1460-9568.1996.tb01217.x
Deschenes, M., Bourassa, J., and Parent, A. (1996b). Striatal and cortical projections of single neurons from the central lateral thalamic nucleus in the rat. Neuroscience 72, 679–687. doi: 10.1016/0306-4522(96)00001-2
DeVito, J. L., and Anderson, M. E. (1982). An autoradiographic study of efferent connections of the globus pallidus in Macaca mulatta. Exp. Brain Res. 46, 107–117. doi: 10.1007/bf00238104
Ding, J. B., Guzman, J. N., Peterson, J. D., Goldberg, J. A., and Surmeier, D. J. (2010). Thalamic gating of corticostriatal signaling by cholinergic interneurons. Neuron 67, 294–307. doi: 10.1016/j.neuron.2010.06.017
Ding, J., Peterson, J. D., and Surmeier, D. J. (2008). Corticostriatal and thalamostriatal synapses have distinctive properties. J. Neurosci. 28, 6483–6492. doi: 10.1523/jneurosci.0435-08.2008
Doig, N. M., Moss, J., and Bolam, J. P. (2010). Cortical and thalamic innervation of direct and indirect pathway medium-sized spiny neurons in mouse striatum. J. Neurosci. 30, 14610–14618. doi: 10.1523/jneurosci.1623-10.2010
Dube, L., Smith, A. D., and Bolam, J. P. (1988). Identification of synaptic terminals of thalamic or cortical origin in contact with distinct medium-size spiny neurons in the rat neostriatum. J. Comp. Neurol. 267, 455–471. doi: 10.1002/cne.902670402
Ellender, T. J., Harwood, J., Kosillo, P., Capogna, M., and Bolam, J. P. (2013). Heterogeneous properties of central lateral and parafascicular thalamic synapses in the striatum. J. Physiol. 591, 257–272. doi: 10.1113/jphysiol.2012.245233
Ellender, T. J., Huerta-Ocampo, I., Deisseroth, K., Capogna, M., and Bolam, J. P. (2011). Differential modulation of excitatory and inhibitory striatal synaptic transmission by histamine. J. Neurosci. 31, 15340–15351. doi: 10.1523/jneurosci.3144-11.2011
Fenelon, G., Francois, C., Percheron, G., and Yelnik, J. (1991). Topographic distribution of the neurons of the central complex (centre median-parafascicular complex) and of other thalamic neurons projecting to the striatum in macaques. Neuroscience 45, 495–510. doi: 10.1016/0306-4522(91)90244-i
Francois, C., Percheron, G., Parent, A., Sadikot, A. F., Fenelon, G., and Yelnik, J. (1991). Topography of the projection from the central complex of the thalamus to the sensorimotor striatal territory in monkeys. J. Comp. Neurol. 305, 17–34. doi: 10.1002/cne.903050104
Fremeau, R. T. Jr., Troyer, M. D., Pahner, I., Nygaard, G. O., Tran, C. H., Reimer, R. J., et al. (2001). The expression of vesicular glutamate transporters defines two classes of excitatory synapse. Neuron 31, 247–260. doi: 10.1016/s0896-6273(01)00344-0
Fremeau, R. T. Jr., Voglmaier, S., Seal, R. P., and Edwards, R. H. (2004). VGLUTs define subsets of excitatory neurons and suggest novel roles for glutamate. Trends Neurosci. 27, 98–103. doi: 10.1016/j.tins.2003.11.005
Freund, T. F., Powell, J. F., and Smith, A. D. (1984). Tyrosine hydroxylase-immunoreactive boutons in synaptic contact with identified striatonigral neurons, with particular reference to dendritic spines. Neuroscience 13, 1189–1215. doi: 10.1016/0306-4522(84)90294-x
Fujiyama, F., Kuramoto, E., Okamoto, K., Hioki, H., Furuta, T., Zhou, L., et al. (2004). Presynaptic localization of an AMPA-type glutamate receptor in corticostriatal and thalamostriatal axon terminals. Eur. J. Neurosci. 20, 3322–3330. doi: 10.1111/j.1460-9568.2004.03807.x
Fujiyama, F., Unzai, T., Nakamura, K., Nomura, S., and Kaneko, T. (2006). Difference in organization of corticostriatal and thalamostriatal synapses between patch and matrix compartments of rat neostriatum. Eur. J. Neurosci. 24, 2813–2824. doi: 10.1111/j.1460-9568.2006.05177.x
Galvan, A., and Smith, Y. (2011). The primate thalamostriatal systems: anatomical organization, functional roles and possible involvement in Parkinson’s disease. Basal Ganglia 1, 179–189. doi: 10.1016/j.baga.2011.09.001
Gerfen, C. R. (1984). The neostriatal mosaic: compartmentalization of corticostriatal input and striatonigral output systems. Nature 311, 461–464. doi: 10.1038/312172a0
Ghorayeb, I., Fernagut, P. O., Hervier, L., Labattu, B., Bioulac, B., and Tison, F. (2002). A ‘single toxin-double lesion’ rat model of striatonigral degeneration by intrastriatal 1-methyl-4-phenylpyridinium ion injection: a motor behavioural analysis. Neuroscience 115, 533–546. doi: 10.1016/S0306-4522(02)00401-3
Goldberg, J. A., and Reynolds, J. N. (2011). Spontaneous firing and evoked pauses in the tonically active cholinergic interneurons of the striatum. Neuroscience 198, 27–43. doi: 10.1016/j.neuroscience.2011.08.067
Gonon, F. (1997). Prolonged and extrasynaptic excitatory action of dopamine mediated by D1 receptors in the rat striatum in vivo. J. Neurosci. 17, 5972–5978.
Gonzales, K. K., Pare, J. F., Wichmann, T., and Smith, Y. (2013). GABAergic inputs from direct and indirect striatal projection neurons onto cholinergic interneurons in the primate putamen. J. Comp. Neurol. 521, 2502–2522. doi: 10.1002/cne.23295
Groenewegen, H. J., and Berendse, H. W. (1994). The specificity of the ‘nonspecific’ midline and intralaminar thalamic nuclei. Trends Neurosci. 17, 52–57. doi: 10.1016/0166-2236(94)90074-4
Grunwerg, B. S., Krein, H., and Krauthamer, G. M. (1992). Somatosensory input and thalamic projeciton of pedunculopontine tegmental neurons. Neuroreport 3, 673–675. doi: 10.1097/00001756-199208000-00004
Haber, S. N., and Calzavara, R. (2009). The cortico-basal ganglia integrative network: the role of the thalamus. Brain Res. Bull. 78, 69–74. doi: 10.1016/j.brainresbull.2008.09.013
Haber, S., and Mcfarland, N. R. (2001). The place of the thalamus in frontal cortical-basal ganglia circuits. Neuroscientist 7, 315–324. doi: 10.1177/107385840100700408
Hallanger, A. E., Levey, A. I., Lee, H. J., Rye, D. B., and Wainer, B. H. (1987). The origins of cholinergic and other subcortical afferents to the thalamus in the rat. J. Comp. Neurol. 262, 105–124. doi: 10.1002/cne.902620109
Halliday, G. M. (2009). Thalamic changes in Parkinson’s disease. Parkinsonism Relat. Disord. 15(Suppl. 3), S152–S155. doi: 10.1016/S1353-8020(09)70804-1
Halliday, G. M., Macdonald, V., and Henderson, J. M. (2005). A comparison of degeneration in motor thalamus and cortex between progressive supranuclear palsy and Parkinson’s disease. Brain 128, 2272–2280. doi: 10.1093/brain/awh596
Hariz, M. I., and Robertson, M. M. (2010). Gilles de la Tourette syndrome and deep brain stimulation. Eur. J. Neurosci. 32, 1128–1134. doi: 10.1111/j.1460-9568.2010.07415.x.
Hassler, R. (1982). “Stereotaxic surgery for psychiatric disturbances,” in Stereotaxy of the Human Brain, eds G. Schaltenbrand and A. E. Walker (New York: Thieme-Stratton), 570–590.
Hassler, R., and Dieckmann, G. (1970). Stereotaxic treatment of tics and inarticulate cries or coprolalia considered as motor obsessional phenomena in Gilles de la Tourette’s disease. Rev. Neurol.(Paris) 123, 89–100.
Hassler, R., and Dieckmann, G. (1973). “Relief of obsessive-compulsive disorders, phobias and tics by stereotactic coagulations of the rostral intralaminar and medial-thalamic nuclei,” in Surgical Approaches in Psychiatry. Proceedings of the Third International Congress of Psychosurgery, eds L. V. Laitinen and K. Livingston (Cambridge, UK: Garden City Press), 206–212.
Heinsen, H., Rub, U., Gangnus, D., Jungkunz, G., Bauer, M., Ulmar, G., et al. (1996). Nerve cell loss in the thalamic centromedian-parafascicular complex in patients with Huntington’s disease. Acta Neuropathol. 91, 161–168. doi: 10.1007/s004010050408
Henderson, J. M., Carpenter, K., Cartwright, H., and Halliday, G. M. (2000a). Degeneration of the centre median-parafascicular complex in Parkinson’s disease. Ann. Neurol. 47, 345–352. doi: 10.1002/1531-8249(200003)47:3<345::AID-ANA10>3.0.CO;2-V
Henderson, J. M., Carpenter, K., Cartwright, H., and Halliday, G. M. (2000b). Loss of thalamic intralaminar nuclei in progressive supranuclear palsy and Parkinson’s disease: clinical and therapeutic implications. Brain 123, 1410–1421. doi: 10.1093/brain/123.7.1410
Henderson, J. M., Schleimer, S. B., Allbutt, H., Dabholkar, V., Abela, D., Jovic, J., et al. (2005). Behavioural effects of parafascicular thalamic lesions in an animal model of parkinsonism. Behav. Brain Res. 162, 222–232. doi: 10.1016/j.bbr.2005.03.017
Herkenham, M., and Pert, C. (1981). Mosaic distribution of opiate receptors, parafascicular projections and acetylcholinesterase in rat striatum. Nature 291, 415–418. doi: 10.1038/291415a0
Hersch, S. M., Ciliax, B. J., Gutekunst, C. A., Rees, H. D., Heilman, C. J., Yung, K. K., et al. (1995). Electron microscopic analysis of D1 and D2 dopamine receptor proteins in the dorsal striatum and their synaptic relationships with motor corticostriatal afferents. J. Neurosci. 15, 5222–5237.
Houeto, J. L., Karachi, C., Mallet, L., Pillon, B., Yelnik, J., Mesnage, V., et al. (2005). Tourette’s syndrome and deep brain stimulation. J. Neurol. Neurosurg. Psychiatry 76, 992–995. doi: 10.1136/jnnp.2004.043273
Huerta, M. F., Krubitzer, L. A., and Kaas, J. H. (1986). Frontal eye field as defined by intracortical microstimulation in squirrel monkeys, owl monkeys and macaque monkeys: I. subcortical connections. J. Comp. Neurol. 253, 415–439. doi: 10.1002/cne.902530402
Huerta-Ocampo, I., Mena-Segovia, J., and Bolam, J. P. (2013). Convergence of cortical and thalamic input to direct and indirect pathway medium spiny neurons in the striatum. Brain Struct. Funct. doi: 10.1007/s00429-013-0601-z. [Epub ahead of print].
Hulme, O. J., Whiteley, L., and Shipp, S. (2010). Spatially distributed encoding of covert attentional shifts in human thalamus. J. Neurophysiol. 104, 3644–3656. doi: 10.1152/jn.00303.2010
Ichinohe, N., Mori, F., and Shoumura, K. (2000). A di-synaptic projection from the lateral cerebellar nucleus to the laterodorsal part of the striatum via the central lateral nucleus of the thalamus in the rat. Brain Res. 880, 191–197. doi: 10.1016/s0006-8993(00)02744-x
Ingham, C. A., Hood, S. H., and Arbuthnott, G. W. (1989). Spine density on neostriatal neurones changes with 6-hydroxydopamine lesions and with age. Brain Res. 503, 334–338. doi: 10.1016/0006-8993(89)91686-7
Ingham, C. A., Hood, S. H., Taggart, P., and Arbuthnott, G. W. (1998). Plasticity of synapses in the rat neostriatum after unilateral lesion of the nigrostriatal dopaminergic pathway. J. Neurosci. 18, 4732–4743.
Ipekchyan, N. M. (2011). Comparative analysis of the quantitative characteristics of the corticothalamic projections of parietal cortex fields 5 and 7. Neurosci. Behav. Physiol. 41, 10–12. doi: 10.1007/s11055-010-9369-2
Jinnah, H. A., and Hess, E. J. (2006). A new twist on the anatomy of dystonia: the basal ganglia and the cerebellum? Neurology 67, 1740–1741. doi: 10.1212/01.wnl.0000246112.19504.61
Jouve, L., Salin, P., Melon, C., and Kerkerian-Le Goff, L. (2010). Deep brain stimulation of the center median-parafascicular complex of the thalamus has efficient anti-parkinsonian action associated with widespread cellular responses in the basal ganglia network in a rat model of Parkinson’s disease. J. Neurosci. 30, 9919–9928. doi: 10.1523/JNEUROSCI.1404-10.2010
Kaneko, T., and Fujiyama, F. (2002). Complementary distribution of vesicular glutamate transporters in the central nervous system. Neurosci. Res. 42, 243–250. doi: 10.1016/s0168-0102(02)00009-3
Kato, S., Kuramochi, M., Kobayashi, K., Fukabori, R., Okada, K., Uchigashima, M., et al. (2011). Selective neural pathway targeting reveals key roles of thalamostriatal projection in the control of visual discrimination. J. Neurosci. 31, 17169–17179. doi: 10.1523/JNEUROSCI.4005-11.2011
Kemp, J. M., and Powell, T. P. (1971). The termination of fibres from the cerebral cortex and thalamus upon dendritic spines in the caudate nucleus: a study with the Golgi method. Philos. Trans. R. Soc. Lond. B Biol. Sci. 262, 429–439. doi: 10.1098/rstb.1971.0105
Kerkerian-Le Goff, L., Bacci, J. J., Jouve, L., Melon, C., and Salin, P. (2009). Impact of surgery targeting the caudal intralaminar thalamic nuclei on the pathophysiological functioning of basal ganglia in a rat model of Parkinson’s disease. Brain. Res. Bull. 78, 80–84. doi: 10.1016/j.brainresbull.2008.08.010
Kim, J. P., Min, H. K., Knight, E. J., Duffy, P. S., Abulseoud, O. A., Marsh, M. P., et al (2013). Centromedian-parafascicular deep brain stimulation induces differential functional inhibition of the motor, associative and limbic circuits in large animals. Biol. Psychiatry 74, 917–926. doi: 10.1016/j.biopsych.2013.06.024
Kimura, M., Minamimoto, T., Matsumoto, N., and Hori, Y. (2004). Monitoring and switching of cortico-basal ganglia loop functions by the thalamo-striatal system. Neurosci. Res. 48, 355–360. doi: 10.1016/j.neures.2003.12.002
Kinomura, S., Larsson, J., Gulyas, B., and Roland, P. E. (1996). Activation by attention of the human reticular formation and thalamic intralaminar nuclei. Science 271, 512–515. doi: 10.1126/science.271.5248.512
Kocsis, J. D., and Kitai, S. T. (1977). Dual excitatory inputs to caudate spiny neurons from substantia nigra stimulation. Brain Res. 138, 271–283. doi: 10.1016/0006-8993(77)90745-4
Krack, P., Hariz, M. I., Baunez, C., Guridi, J., and Obeso, J. A. (2010). Deep brain stimulation: from neurology to psychiatry? Trends Neurosci. 33, 474–484. doi: 10.1016/j.tins.2010.07.002
Krauss, J. K., Pohle, T., Weigel, R., and Burgunder, J. M. (2002). Deep brain stimulation of the centre median-parafascicular complex in patients with movement disorders. J. Neurol. Neurosurg. Psychiatry 72, 546–548.
Kunzle, H. (1976). Thalamic projections from the precentral motor cortex in macaca fascicularis. Brain Res. 105, 253–267. doi: 10.1016/0006-8993(76)90424-8
Kunzle, H. (1978). An autoradiographic analysis of the efferent connections from premotor and adjacent prefrontal regions (areas 6 and 9) in macaca fascicularis. Brain Behav. Evol. 15, 185–234. doi: 10.1159/000123780
Kusnoor, S. V., Muly, E. C., Morgan, J. I., and Deutch, A. Y. (2009). Is the loss of thalamostriatal neurons protective in parkinsonism? Parkinsonism Relat. Disord. 15(Suppl. 3), S162–S166. doi: 10.1016/s1353-8020(09)70806-5
Kusnoor, S. V., Parris, J., Muly, E. C., Morgan, J. I., and Deutch, A. Y. (2010). Extracerebellar role for Cerebellin1: modulation of dendritic spine density and synapses in striatal medium spiny neurons. J. Comp. Neurol. 518, 2525–2537. doi: 10.1002/cne.22350
Kuypers, H. G. J. M., and Lawrence, D. G. (1967). Cortical projections to the red nucleus and the brain stem in the Rhesus monkey. Brain Res. 4, 151–188. doi: 10.1016/0006-8993(67)90004-2
Lacey, C. J., Bolam, J. P., and Magill, P. J. (2007). Novel and distinct operational principles of intralaminar thalamic neurons and their striatal projections. J. Neurosci. 27, 4374–4384. doi: 10.1523/jneurosci.5519-06.2007
Lacey, C. J., Boyes, J., Gerlach, O., Chen, L., Magill, P. J., and Bolam, J. P. (2005). GABA(B) receptors at glutamatergic synapses in the rat striatum. Neuroscience 136, 1083–1095. doi: 10.1016/j.neuroscience.2005.07.013
Lanciego, J. L., Gonzalo, N., Castle, M., Sanchez-Escobar, C., Aymerich, M. S., and Obeso, J. A. (2004). Thalamic innervation of striatal and subthalamic neurons projecting to the rat entopeduncular nucleus. Eur. J. Neurosci. 19, 1267–1277. doi: 10.1111/j.1460-9568.2004.03244.x
Lanciego, J. L., Rodriguez-Oroz, M. C., Blesa, F. J., Alvarez-Erviti, L., Guridi, J., Barroso-Chinea, P., et al (2008). Lesion of the centromedian thalamic nucleus in MPTP-treated monkeys. Mov. Disord. 23, 708–715. doi: 10.1002/mds.21906
Lapper, S. R., and Bolam, J. P. (1992). Input from the frontalcortex and the parafascicular nucleus to cholinergic interneurons in the dorsal striatum of the rat. Neuroscience 51, 533–545. doi: 10.1016/0306-4522(92)90293-b
Lavoie, B., and Parent, A. (1991). Serotoninergic innervation of the thalamus in the primate: an immunohistochemical study. J. Comp. Neurol. 312, 1–18. doi: 10.1002/cne.903120102
Lei, W., Deng, Y., Liu, B., Mu, S., Guley, N. M., Wong, T., et al. (2013). Confocal laser scanning microscopy and ultrastructural study of VGLUT2 thalamic input to striatal projection neurons in rats. J. Comp. Neurol. 521, 1354–1377. doi: 10.1002/cne.23235
Lei, W., Jiao, Y., Del Mar, N., and Reiner, A. (2004). Evidence for differential cortical input to direct pathway versus indirect pathway striatal projection neurons in rats. J. Neurosci. 24, 8289–8299. doi: 10.1523/jneurosci.1990-04.2004
Leichnetz, G. R., and Goldberg, M. E. (1988). Higher centers concerned with eye movement and visual attention: cerebral cortex and thalamus. Rev. Oculomot. Res. 2, 365–429.
Ma, Y., Smith, Y., and Wichmann, T. (2012). Effects of dopamine on corticostriatal transmission in monkeys. Mov. Disord. 27, S1.
Macchi, G., Bentivoglio, M., Molinari, M., and Minciacchi, D. (1984). The thalamo-caudate versus thalamo-cortical projections as studied in the cat with fluorescent retrograde double labeling. Exp. Brain Res. 54, 225–239. doi: 10.1007/bf00236222
Maciunas, R. J., Maddux, B. N., Riley, D. E., Whitney, C. M., Schoenberg, M. R., Ogrocki, P. J., et al. (2007). Prospective randomized double-blind trial of bilateral thalamic deep brain stimulation in adults with Tourette syndrome. J. Neurosurg. 107, 1004–1014. doi: 10.3171/jns.2007.107.5.1004
Maling, N., Hashemiyoon, R., Foote, K. D., Okun, M. S., and Sanchez, J. C. (2012). Increased thalamic gamma band activity correlates with symptom relief following deep brain stimulation in humans with Tourette’s syndrome. PLoS One 7:e44215. doi: 10.1371/journal.pone.0044215
Matsumoto, N., Minamimoto, T., Graybiel, A. M., and Kimura, M. (2001). Neurons in the thalamic CM-Pf complex supply striatal neurons with information about behaviorally significant sensory events. J. Neurophysiol. 85, 960–976.
Mazzone, P., Stocchi, F., Galati, S., Insola, A., Altibrandi, M. G., Modugno, N., et al. (2006). Bilateral implantation of centromedian-parafascicularis complex and GPi: a new combination of unconventional targets for deep brain stimulation in severe Parkinson disease. Neuromodulation 9, 221–228. doi: 10.1111/j.1525-1403.2006.00063.x
McFarland, N. R., and Haber, S. N. (2000). Convergent inputs from thalamic motor nuclei and frontal cortical areas to the dorsal striatum in the primate. J. Neurosci. 20, 3798–3813.
Mcfarland, N. R., and Haber, S. N. (2001). Organization of thalamostriatal terminals from the ventral motor nuclei in the macaque. J. Comp. Neurol. 429, 321–336. doi: 10.1002/1096-9861(20000108)429:2<321::aid-cne11>3.0.co;2-a
McHaffie, J. G., Stanford, T. R., Stein, B. E., Coizet, V., and Redgrave, P. (2005). Subcortical loops through the basal ganglia. Trends Neurosci. 28, 401–407. doi: 10.1016/j.tins.2005.06.006
Mehler, W. R. (1966). The posterior thalamic region in man. Confin. Neurol. 27, 18–29. doi: 10.1159/000103928
Mengual, E., De Las Heras, S., Erro, E., Lanciego, J. L., and Gimenez-Amaya, J. M. (1999). Thalamic interaction between the input and the output systems of the basal ganglia. J. Chem. Neuroanat. 16, 187–200. doi: 10.1016/s0891-0618(99)00010-1
Meredith, G. E., and Wouterlood, F. G. (1990). Hippocampal and midline thalamic fibers and terminals in relation to the choline acetyltransferase-immunoreactive neurons in nucleus accumbens of the rat: a light and electron microscopic study. J. Comp. Neurol. 296, 204–221. doi: 10.1002/cne.902960203
Metzger, C. D., Eckert, U., Steiner, J., Sartorius, A., Buchmann, J. E., Stadler, J., et al. (2010). High field FMRI reveals thalamocortical integration of segregated cognitive and emotional processing in mediodorsal and intralaminar thalamic nuclei. Front. Neuroanat. 4:138. doi: 10.3389/fnana.2010.00138
Minamimoto, T., Hori, Y., and Kimura, M. (2005). Complementary process to response bias in the centromedian nucleus of the thalamus. Science 308, 1798–1801. doi: 10.1126/science.1109154
Minamimoto, T., Hori, Y., and Kimura, M. (2009). Roles of the thalamic CM-PF complex-Basal ganglia circuit in externally driven rebias of action. Brain Res. Bull. 78, 75–79. doi: 10.1016/j.brainresbull.2008.08.013
Minamimoto, T., and Kimura, M. (2002). Participation of the thalamic CM-Pf complex in attentional orienting. J. Neurophysiol. 87, 3090–3101.
Mink, J. W. (2006). Neurobiology of basal ganglia and Tourette syndrome: basal ganglia circuits and thalamocortical outputs. Adv. Neurol. 99, 89–98.
Moss, J., and Bolam, J. P. (2008). A dopaminergic axon lattice in the striatum and its relationship with cortical and thalamic terminals. J. Neurosci. 28, 11221–11230. doi: 10.1523/jneurosci.2780-08.2008
Nanda, B., Galvan, A., Smith, Y., and Wichmann, T. (2009). Effects of stimulation of the centromedian nucleus of the thalamus on the activity of striatal cells in awake rhesus monkeys. Eur. J. Neurosci. 29, 588–598. doi: 10.1111/j.1460-9568.2008.06598.x
Neuner, I., Podoll, K., Janouschek, H., Michel, T. M., Sheldrick, A. J., and Schneider, F. (2009). From psychosurgery to neuromodulation: deep brain stimulation for intractable Tourette syndrome. World J. Biol. Psychiatry 10, 366–376. doi: 10.1080/15622970802513317
Newman, D. B., and Ginsberg, C. Y. (1994). Brainstem reticular nuclei that project to the thalamus in rats: a retrograde tracer study. Brain Behav. Evol. 44, 1–39. doi: 10.1159/000113566
Neychev, V. K., Fan, X., Mitev, V. I., Hess, E. J., and Jinnah, H. A. (2008). The basal ganglia and cerebellum interact in the expression of dystonic movement. Brain 131, 2499–2509. doi: 10.1093/brain/awn168
Pare, D., Smith, Y., Parent, A., and Steriade, M. (1988). Projections of brainstem core cholinergic and non-cholinergic neurons of cat to intralaminar and reticular thalamic nuclei. Neuroscience 25, 69–86. doi: 10.1016/0306-4522(88)90007-3
Parent, A., and Hazrati, L. N. (1995). Functional anatomy of the basal ganglia. I. The cortico-basal ganglia-thalamo-cortical loop. Brain Res. Brain Res. Rev. 20, 91–127. doi: 10.1016/0165-0173(94)00007-c
Parent, A., Mackey, A., and De Bellefeuille, L. (1983). The subcortical afferents to caudate nucleus and putamen in primate: a fluorescence retrograde double labeling study. Neuroscience 10, 1137–1150. doi: 10.1016/0306-4522(83)90104-5
Parent, A., Pare, D., Smith, Y., and Steriade, M. (1988). Basal forebrain cholinergic and noncholinergic projections to the thalamus and brainstem in cats and monkeys. J. Comp. Neurol. 277, 281–301. doi: 10.1002/cne.902770209
Parent, M., and Parent, A. (2005). Single-axon tracing and three-dimensional reconstruction of centre median-parafascicular thalamic neurons in primates. J. Comp. Neurol. 481, 127–144. doi: 10.1002/cne.20348
Peppe, A., Gasbarra, A., Stefani, A., Chiavalon, C., Pierantozzi, M., Fermi, E., et al. (2008). Deep brain stimulation of CM/PF of thalamus could be the new elective target for tremor in advanced Parkinson’s Disease? Parkinsonism Relat. Disord. 14, 501–504. doi: 10.1016/j.parkreldis.2007.11.005
Porta, M., Brambilla, A., Cavanna, A. E., Servello, D., Sassi, M., Rickards, H., et al. (2009). Thalamic deep brain stimulation for treatment-refractory Tourette syndrome: two-year outcome. Neurology 73, 1375–1380. doi: 10.1212/wnl.0b013e3181bd809b
Raju, D. V., Ahern, T. H., Shah, D. J., Wright, T. M., Standaert, D. G., Hall, R. A., et al. (2008). Differential synaptic plasticity of the corticostriatal and thalamostriatal systems in an MPTP-treated monkey model of parkinsonism. Eur. J. Neurosci. 27, 1647–1658. doi: 10.1111/j.1460-9568.2008.06136.x
Raju, D. V., Shah, D. J., Wright, T. M., Hall, R. A., and Smith, Y. (2006). Differential synaptology of vGluT2-containing thalamostriatal afferents between the patch and matrix compartments in rats. J. Comp. Neurol. 499, 231–243. doi: 10.1002/cne.21099
Redgrave, P., Prescott, T., and Gurney, K. N. (1999). The basal ganglia: a vertebrate solution to the selection problem? Neuroscience 89, 1009–1023. doi: 10.1016/s0306-4522(98)00319-4
Redgrave, P., Rodriguez, M., Smith, Y., Rodriguez-Oroz, M. C., Lehericy, S., Bergman, H., et al. (2010). Goal-directed and habitual control in the basal ganglia: implications for Parkinson’s disease. Nat. Rev. Neurosci. 11, 760–772. doi: 10.1038/nrn2915
Reiner, A. J. (2010). “The conservative evolution of the vertebrate basal ganglia,” in Handbook of Basal Ganglia Structure and Function, eds. H. Steiner and K. Y. Tseng (New York, NY: Elsevier), 29–62.
Reynolds, J. N., Hyland, B. I., and Wickens, J. R. (2001). A cellular mechanism of reward-related learning. Nature 413, 67–70. doi: 10.1038/35092560
Rice, M. E., and Cragg, S. J. (2008). Dopamine spillover after quantal release: rethinking dopamine transmission in the nigrostriatal pathway. Brain Res. Rev. 58, 303–313. doi: 10.1016/j.brainresrev.2008.02.004
Royce, G. J. (1978). Cells of origin of subcortical afferents to the caudate nucleus: a horseradish peroxidase study in the cat. Brain Res. 153, 465–475. doi: 10.1016/0006-8993(78)90332-3
Royce, G. J. (1983). Single thalamic neurons which project to both the rostral cortex and caudate nucleus studied with the fluorescent double labeling method. Exp. Neurol. 79, 773–784. doi: 10.1016/0014-4886(83)90041-9
Royce, G. J., Bromley, S., and Gracco, C. (1991). Subcortical projections to the centromedian and parafascicular thalamic nuclei in the cat. J. Comp. Neurol. 306, 129–155. doi: 10.1002/cne.903060110
Rudkin, T. M., and Sadikot, A. F. (1999). Thalamic input to parvalbumin-immunoreactive GABAergic interneurons: organization in normal striatum and effect of neonatal decortication. Neuroscience 88, 1165–1175. doi: 10.1016/s0306-4522(98)00265-6
Sadikot, A. F., Parent, A., and Francois, C. (1992a). Efferent connections of the centromedian and parafascicular thalamic nuclei in the squirrel monkey: a PHA-L study of subcortical projections. J. Comp. Neurol. 315, 137–159. doi: 10.1002/cne.903150203
Sadikot, A. F., Parent, A., Smith, Y., and Bolam, J. P. (1992b). Efferent connections of the centromedian and parafascicular thalamic nuclei in the squirrel monkey: a light and electron microscopic study of the thalamostriatal projection in relation to striatal heterogeneity. J. Comp. Neurol. 320, 228–242. doi: 10.1002/cne.903200207
Sadikot, A. F., and Rymar, V. V. (2009). The primate centromedian-parafascicular complex: anatomical organization with a note on neuromodulation. Brain Res. Bull. 78, 122–130. doi: 10.1016/j.brainresbull.2008.09.016
Sassi, M., Porta, M., and Servello, D. (2011). Deep brain stimulation therapy for treatment-refractory Tourette’s syndrome: a review. Acta Neurochir. (Wien) 153, 639–645.
Savica, R., Stead, M., Mack, K. J., Lee, K. H., and Klassen, B. T. (2012). Deep brain stimulation in tourette syndrome: a description of 3 patients with excellent outcome. Mayo Clin. Proc. 87, 59–62. doi: 10.1016/j.mayocp.2011.08.005
Sciamanna, G., Tassone, A., Mandolesi, G., Puglisi, F., Ponterio, G., Martella, G., et al. (2012). Cholinergic dysfunction alters synaptic integration between thalamostriatal and corticostriatal inputs in DYT1 dystonia. J. Neurosci. 32, 11991–12004. doi: 10.1523/jneurosci.0041-12.2012
Servello, D., Porta, M., Sassi, M., Brambilla, A., and Robertson, M. M. (2008). Deep brain stimulation in 18 patients with severe Gilles de la Tourette syndrome refractory to treatment: the surgery and stimulation. J. Neurol. Neurosurg. Psychiatry 79, 136–142. doi: 10.1136/jnnp.2006.104067
Servello, D., Sassi, M., Brambilla, A., Defendi, S., and Porta, M. (2010). Long-term, post-deep brain stimulation management of a series of 36 patients affected with refractory gilles de la tourette syndrome. Neuromodulation 13, 187–194. doi: 10.1111/j.1525-1403.2009.00253.x
Shields, D. C., Cheng, M. L., Flaherty, A. W., Gale, J. T., and Eskandar, E. N. (2008). Microelectrode-guided deep brain stimulation for Tourette syndrome: within-subject comparison of different stimulation sites. Stereotact Funct. Neurosurg. 86, 87–91. doi: 10.1159/000112429
Sidibe, M., and Smith, Y. (1996). Differential synaptic innervation of striatofugal neurones projecting to the internal or external segments of the globus pallidus by thalamic afferents in the squirrel monkey. J. Comp. Neurol. 365, 445–465. doi: 10.1002/(sici)1096-9861(19960212)365:3<445::aid-cne8>3.3.co;2-8
Sidibe, M., and Smith, Y. (1999). Thalamic inputs to striatal interneurons in monkeys: synaptic organization and co-localization of calcium binding proteins. Neuroscience 89, 1189–1208. doi: 10.1016/s0306-4522(98)00367-4
Smeal, R. M., Gaspar, R. C., Keefe, K. A., and Wilcox, K. S. (2007). A rat brain slice preparation for characterizing both thalamostriatal and corticostriatal afferents. J. Neurosci. Methods 159, 224–235. doi: 10.1016/j.jneumeth.2006.07.007
Smeal, R. M., Keefe, K. A., and Wilcox, K. S. (2008). Differences in excitatory transmission between thalamic and cortical afferents to single spiny efferent neurons of rat dorsal striatum. Eur. J. Neurosci. 28, 2041–2052. doi: 10.1111/j.1460-9568.2008.06505.x
Smith, Y., Bennett, B. D., Bolam, J. P., Parent, A., and Sadikot, A. F. (1994). Synaptic relationships between dopaminergic afferents and cortical or thalamic input in the sensorimotor territory of the striatum in monkey. J. Comp. Neurol. 344, 1–19. doi: 10.1002/cne.903440102
Smith, Y., Galvan, A., Raju, D., and Wichmann, T. (2010). “Anatomical and functional organization of the thalamostriatal systems,” in Handbook of Basal Ganglia Structure and Function: A Decade of Progress, eds. H. Steiner and K. Y. Tseng (New York, NY: Elsevier), 381–392.
Smith, Y., and Parent, A. (1986). Differential connections of caudate nucleus and putamen in the squirrel monkey (Saimiri sciureus). Neuroscience 18, 347–371.
Smith, Y., Raju, D., Nanda, B., Pare, J. F., Galvan, A., and Wichmann, T. (2009). The thalamostriatal systems: anatomical and functional organization in normal and parkinsonian states. Brain Res. Bull. 78, 60–68. doi: 10.1016/j.brainresbull.2008.08.015
Smith, Y., Raju, D. V., Pare, J. F., and Sidibe, M. (2004). The thalamostriatal system: a highly specific network of the basal ganglia circuitry. Trends Neurosci. 27, 520–527. doi: 10.1016/j.tins.2004.07.004
Smith, Y., Surmeier, D. J., Redgrave, P., and Kimura, M. (2011). Thalamic contributions to Basal Ganglia-related behavioral switching and reinforcement. J. Neurosci. 31, 16102–16106. doi: 10.1523/jneurosci.4634-11.2011
Somogyi, P., Bolam, J. P., and Smith, A. D. (1981). Monosynaptic cortical input and local axon collaterals of identified striatonigral neurons. A light and electron microscopic study using the Golgi-peroxidase transport-degeneration procedure. J. Comp. Neurol. 195, 567–584. doi: 10.1002/cne.901950403
Stefani, A., Peppe, A., Pierantozzi, M., Galati, S., Moschella, V., Stanzione, P., et al. (2009). Multi-target strategy for parkinsonian patients: the role of deep brain stimulation in the centromedian-parafascicularis complex. Brain Res. Bull. 78, 113–118. doi: 10.1016/j.brainresbull.2008.08.007
Stephens, B., Mueller, A. J., Shering, A. F., Hood, S. H., Taggart, P., Arbuthnott, G. W., et al. (2005). Evidence of a breakdown of corticostriatal connections in parkinson’s disease. Neuroscience 132, 741–754. doi: 10.1016/j.neuroscience.2005.01.007
Stephenson-Jones, M., Samuelsson, E., Ericsson, J., Robertson, B., and Grillner, S. (2011). Evolutionary conservation of the basal ganglia as a common vertebrate mechanism for action selection. Curr. Biol. 21, 1081–1091. doi: 10.1016/j.cub.2011.05.001
Steriade, M., and Glenn, L. L. (1982). Neocortical and caudate projections of intralaminar thalamic neurons and their synaptic excitation from midbrain reticular core. J. Neurophysiol. 48, 352–371.
Surmeier, D. J., Ding, J., Day, M., Wang, Z., and Shen, W. (2007). D1 and D2 dopamine-receptor modulation of striatal glutamatergic signaling in striatal medium spiny neurons. Trends Neurosci. 30, 228–235. doi: 10.1016/j.tins.2007.03.008
Temel, Y., and Visser-Vandewalle, V. (2004). Surgery in tourette syndrome. Mov. Disord. 19, 3–14. doi: 10.1002/mds.10649
Threlfell, S., Lalic, T., Platt, N. J., Jennings, K. A., Deisseroth, K., and Cragg, S. J. (2012). Striatal dopamine release is triggered by synchronized activity in cholinergic interneurons. Neuron 75, 58–64. doi: 10.1016/j.neuron.2012.04.038
Van der Werf, Y. D., Witter, M. P., and Groenewegen, H. J. (2002). The intralaminar and midline nuclei of the thalamus. Anatomical and functional evidence for participation in processes of arousal and awareness. Brain Res. Brain Res. Rev. 39, 107–140. doi: 10.1016/s0165-0173(02)00181-9
Vandermaelen, C. P., and Kitai, S. T. (1980). Intracellular analysis of synaptic potentials in rat neostriatum following stimulation of the cerebral cortex, thalamus and substantia nigra. Brain Res. Bull. 5, 725–733. doi: 10.1016/0361-9230(80)90212-9
Vertes, R. P., Linley, S. B., and Hoover, W. B. (2010). Pattern of distribution of serotonergic fibers to the thalamus of the rat. Brain Struct. Funct. 215, 1–28. doi: 10.1007/s00429-010-0249-x
Vertes, R. P., and Martin, G. F. (1988). Autoradiographic analysis of ascending projections from the pontine and mesencephalic reticular formation and the median raphe nucleus in the rat. J. Comp. Neurol. 275, 511–541. doi: 10.1002/cne.902750404
Villalba, R. M., Lee, H., and Smith, Y. (2009). Dopaminergic denervation and spine loss in the striatum of MPTP-treated monkeys. Exp. Neurol. 215, 220–227. doi: 10.1016/j.expneurol.2008.09.025
Villalba, R. M., and Smith, Y. (2010). Striatal spine plasticity in Parkinson’s disease. Front. Neuroanat. 4:133. doi: 10.3389/fnana.2010.00133
Villalba, R. M., and Smith, Y. (2011). Neuroglial plasticity at striatal glutamatergic synapses in Parkinson’s disease. Front. Syst. Neurosci. 5:68. doi: 10.3389/fnsys.2011.00068
Villalba, R. M., and Smith, Y. (2013). Differential striatal spine pathology in Parkinson’s disease and cocaine addiction: a key role of dopamine? Neuroscience 251, 2–20. doi: 10.1016/j.neuroscience.2013.07.011
Villalba, R. M., Wichmann, T., and Smith, Y. (2014). Neuronal loss in the caudal intralaminar thalamic nuclei in a primate model of Parkinson’s disease. Brain Struct. Funct. 219, 381–394.
Villalba, R. M., Wichmann, T., and Smith, Y. (2013). Preferential loss of thalamostriatal over corticostriatal glutamatergic synapses in MPTP-treated parkinsonian monkeys. Soc. of Neurosci. Annu. Meet. Abstr. 240.02.
Visser-Vandewalle, V., Ackermans, L., Van Der Linden, C., Temel, Y., Tijssen, M. A., Schruers, K. R., et al. (2006). Deep brain stimulation in Gilles de la Tourette’s syndrome. Neurosurgery 58:E590. doi: 10.1093/med/9780199543717.003.0018
Visser-Vandewalle, V., and Kuhn, J. (2013). Deep brain stimulation for tourette syndrome. Handb. Clin. Neurol. 116, 251–258. doi: 10.1016/B978-0-444-53497-2.00020-6
Visser-Vandewalle, V., Temel, Y., Boon, P., Vreeling, F., Colle, H., Hoogland, G., et al. (2003). Chronic bilateral thalamic stimulation: a new therapeutic approach in intractable tourette syndrome. Report of three cases. J. Neurosurg. 99, 1094–1100. doi: 10.3171/jns.2003.99.6.1094
Visser-Vandewalle, V., Temel, Y., Van Der Linden, C., Ackermans, L., and Beuls, E. (2004). Deep brain stimulation in movement disorders. The applications reconsidered. Acta Neurol. Belg. 104, 33–36.
Vogt, C., and Vogt, O. (1941). Thalamusstudien I-III: I. Zur Einführung. II. Homogenität and Grenzgestaltung der Grisea des Thalamus. III. Das Griseum centrale (Centrum medianum Luys). J. Psychol. Neurol. 50, 32–154.
Wilson, C. J., Chang, H. T., and Kitai, S. T. (1983). Origins of post synaptic potentials evoked in spiny neostriatal projection neurons by thalamic stimulation in the rat. Exp. Brain Res. 51, 217–226. doi: 10.1007/bf00237197
Wilson, C. J., Chang, H. T., and Kitai, S. T. (1990). Firing patterns and synaptic potentials of identified giant aspiny interneurons in the rat neostriatum. J. Neurosci. 10, 508–519.
Worbe, Y., Sgambato-Faure, V., Epinat, J., Chaigneau, M., Tande, D., Francois, C., et al. (2013). Towards a primate model of Gilles de la tourette syndrome: anatomo-behavioural correlation of disorders induced by striatal dysfunction. Cortex 49, 1126–1140. doi: 10.1016/j.cortex.2012.08.020
Xu, Z. C., Wilson, C. J., and Emson, P. C. (1991). Restoration of thalamostriatal projections in rat neostriatal grafts: an electron microscopic analysis. J. Comp. Neurol. 303, 22–34. doi: 10.1002/cne.903030104
Xu, C., Zhao, J., Liu, Y., Zeng, X., Jia, Y., Wang, Y., et al. (2012). Dopaminergic axons preferentially innervate dendritic spines with hyperactive glutamatergic synapses in the rat striatum. Brain Res. 1486, 92–102. doi: 10.1016/j.brainres.2012.09.032
Xuereb, J. H., Perry, R. H., Candy, J. M., Perry, E. K., Marshall, E., and Bonham, J. R. (1991). Nerve cell loss in the thalamus in Alzheimer’s disease and Parkinson’s disease. Brain 114, 1363–1379. doi: 10.1093/brain/114.3.1363
Zackheim, J., and Abercrombie, E. D. (2005). Thalamic regulation of striatal acetylcholine efflux is both direct and indirect and qualitatively altered in the dopamine-depleted striatum. Neuroscience 131, 423–436. doi: 10.1016/j.neuroscience.2004.11.006
Zaja-Milatovic, S., Milatovic, D., Schantz, A. M., Zhang, J., Montine, K. S., Samii, A., et al. (2005). Dendritic degeneration in neostriatal medium spiny neurons in Parkinson disease. Neurology 64, 545–547. doi: 10.1212/01.wnl.0000150591.33787.a4
Zhang, Y., Meredith, G. E., Mendoza-Elias, N., Rademacher, D. J., Tseng, K. Y., and Steece-Collier, K. (2013). Aberrant restoration of spines and their synapses in L-DOPA-induced dyskinesia: involvement of corticostriatal but not thalamostriatal synapses. J. Neurosci. 33, 11655–11667. doi: 10.1523/jneurosci.0288-13.2013
Keywords: thalamus, Parkinson’s disease, intralaminar nuclei, glutamate, vesicular glutamate transporter, attention, striatum, Tourette’s syndrome
Citation: Smith Y, Galvan A, Ellender TJ, Doig N, Villalba RM, Huerta-Ocampo I, Wichman T and Bolam JP (2014) The thalamostriatal system in normal and diseased states. Front. Syst. Neurosci. 8:5. doi: 10.3389/fnsys.2014.00005
Received: 12 November 2013; Accepted: 11 January 2014;
Published online: 30 January 2014.
Edited by:
Hagai Bergman, The Hebrew University, IsraelReviewed by:
Jose L. Lanciego, University of Navarra, SpainAntonio Pisani, Università di Roma “Tor Vergata”, Italy
Copyright © 2014 Smith, Galvan, Ellender, Doig, Villalba, Huerta-Ocampo, Wichman and Bolam. This is an open-access article distributed under the terms of the Creative Commons Attribution License (CC BY). The use, distribution or reproduction in other forums is permitted, provided the original author(s) or licensor are credited and that the original publication in this journal is cited, in accordance with accepted academic practice. No use, distribution or reproduction is permitted which does not comply with these terms.
*Correspondence: Yoland Smith, Yerkes National Primate Research Center, Emory University, 954, Gatewood Road NE, Atlanta, GA 30329, USA e-mail: ysmit01@emory.edu