PET tracers in glioblastoma: Toward neurotheranostics as an individualized medicine approach
- 1Cancer Research Center, RAZAVI Hospital, Imam Reza International University, Mashhad, Iran
- 2The Persian Gulf Nuclear Medicine Research Center, Department of Molecular Imaging and Theranostics, Bushehr Medical University Hospital, School of Medicine, Bushehr University of Medical Sciences, Bushehr, Iran
- 3Department of Neurology, Bushehr Medical University Hospital, School of Medicine, Bushehr University of Medical Sciences, Bushehr, Iran
- 4Department of Radiology, Cleveland Clinic, Cleveland, Ohio
Over the past decade, theragnostic radiopharmaceuticals have been used in nuclear medicine for both diagnosis and treatment of various tumors. In this review, we carried out a literature search to investigate and explain the role of radiotracers in the theragnostic approach to glioblastoma multiform (GBM). We primarily focused on basic and rather common positron emotion tomography (PET) radiotracers in these tumors. Subsequently, we introduced and evaluated the preclinical and clinical results of theranostic-based biomarkers including integrin receptor family, prostate-specific membrane antigen (PSMA), fibroblast activated protein (FAP), somatostatin receptors (SRS), and chemokine receptor-4 (CXCR4) for patients with GBM to confer the benefit of personalized therapy. Moreover, promising research opportunities that could have a profound impact on the treatment of GBM over the next decade are also highlighted. Preliminary results showed the potential feasibility of the theragnostic approach using theses biomarkers in GBM patients.
Introduction
Glioblastoma Multiforme (GBM), which develops from glial cells, astrocytes or oligodendrocytes, is a heterogeneous invasive brain tumor that causes death in the first two years after diagnosis. According to the World Health Organization (WHO), brain tumor classification has been updated to include genotypic markers, histological markers, and grade (1). A low survival rate and very few treatment options for GBM make it a particularly acute health challenge.
There are few effective treatments for primary brain tumors despite significant advances in revealing their molecular underpinnings over the past decade. The most important barriers to developing effective treatments are the tumor blood-brain barrier (BBB), intra-, and intertumoral heterogeneity, and intrinsic resistance to chemotherapy and radiotherapy (2, 3). A number of strategies are currently being investigated including immunotherapy, gene therapy, and novel drug-delivery technologies that bypass the blood-brain barrier. A combination of therapies may also be necessary to achieve a broad, durable antitumor response.
Targeted therapies play an important role in modern treatment concepts. Additionally, the molecular heterogeneity of the tumors, including GBM, also makes this entity an ideal candidate for individualized and targeted treatments (4). To enhance patient selection and predict patient responses to treatment, nuclear imaging appears to be an important component of patient care and personalized medicine. Through a theragnostic approach, as an innovative approach in this field, radiotracer pairs with identical chemical and biological characteristics (but labeled with different isotopes) are used for both diagnosis and therapeutic purposes. In order to develop a “theragnostic” agent, it is necessary to combine targeted therapy with diagnostic imaging, such as scintigraphy, single photon emission tomography (SPECT), SPECT/computed tomography (CT), positron emotion tomography (PET)/CT, and/or PET/magnetic resonance imaging (MRI), in order to identify patients who may benefit from the treatment. Imaging systems that use specific theranostic probes allow physicians to visualize and assess disease targets and control or eliminate them as appropriate (5). To date, many PET agents have been developed for GBM. The already-established PET tracers are focused on general cancer hallmarks that are not specific to any tumor type. Most of them are sustained proliferation markers that indicate an increase in glucose metabolism, protein synthesis, or DNA replication. Neurotheranostics aims to revolutionize staging and improve diagnostic and therapeutic outcomes for neurological disorders. This review comprehensively addresses PET radiotracers and recent advances in neurotheranostics for GBM including integrin receptor family, prostate-specific membrane antigen (PSMA), fibroblast activated protein (FAP), somatostatin receptors (SRS), and chemokine receptor-4 (CXCR4). Moreover, promising research opportunities that could have a profound impact on the treatment of GBM over the next decade are also highlighted.
Glucose metabolism PET
While differentiation between primary brain tumor from metastasis is problematic using conventional imaging, 18F-fluorodeoxyglucose (18F-FDG) PET may be helpful in depicting involved lesions or localizing the primary cancer site (6, 7). Heterogeneous primary brain tumors may show low or high uptake, especially in GBM with necrosis. For instance, astrocytomas and gangliogliomas show relatively high FDG uptake despite their low grade (8). To overcome the greatest clinical challenge of 18F-FDG PET in brain tumor imaging, delayed 18F-FDG imaging can sometimes improve discrimination between tumor and normal lesion due to prolonged radiotracer retention in the tumor relative to the gray matter and radio-necrosis (9, 10). It has been revealed that in low-grade tumors, the FDG uptake is similar to the white matter, while the uptake can be in the range of the normal gray matter in high-grade tumors. Generally, the tumor to white matter uptake ratios greater than 1.5 or the tumor to gray matter uptake ratios greater than 0.6 are used to differentiate benign tumors (grades I and II) from malignant tumors (grades III and IV) (11). Likewise, contrast-enhanced (CE)-MRI using gadolinium cannot reliably distinguish active tumors from radio-necrosis (12). Therefore, PET/MRI may indicate the active metabolic margins of the tumor as a guided biopsy procedure as well as correct tumor delineation during treatment planning and checking treatment response (13, 14). FDG PET/MR imaging with and without CE has higher specificity (97%) compare to MRI scan alone (23%) for the detection of recurrence, and higher FDG uptake in the tumor with recurrent glioma indicates a worse survival (15). Medulloblastomas as malignant brain tumors have high FDG uptake (16, 17).
According to the RANO guidelines, amino acid PET is superior to FDG PET imaging; therefore, the use of amino acids PET is recommended against 18F-FDG for brain tumor imaging (18).
Amino acid PET tracers
Due to the high tumor-to-brain contrast in malignant tissues and the low uptake in normal brain tissue, radiolabeled amino acid tracers are in the first line of brain tumor imaging, including O-(2-18F-fluoroethyl)-L-tyrosine (FET) (18F-FET), Carbon-11-methyl-L-methionine (MET) (11C-MET), 3,4-dihydroxy-6-18F-fluoro-L-phenylalanine (FDOPA) (18F-FDOPA), α-[11C]Methyl-l-tryptophan (AMT) (11C-AMT), and 18F-fluciclovine (18F-FACBC) (19–24). Tumor delineation is highly effective for planning stereotactic biopsies, resection, and treatment planning in radiotherapy (25–28). In this regard, 11C-MET and 18F-FET amino acid-based imaging modalities have a high accuracy for delineation of the tumor extent in non-enhancing gliomas (27). A disadvantage of these tracers is the small amino acid uptake (30%) in low-grade II gliomas; therefore, they do not exclude gliomas (29–31). Because of the short half-life of 11C (20 min) from 11C-MET in the clinical studies, 18F-FET was developed (32, 33) and used as its alternative with more sensitivity. 18F-FET PET combined with MRI may improve tumor diagnosis and increase specificity (21). Moreover, amino acid PET tracers provide valuable information for diagnosis and differentiation of radiation injury from treatment-related changes due to the tumor relapse (21, 34–37).
FET-PET is superior to FDG-PET with higher sensitivity. The sensitivity is about 94% for high-grade tumors (WHO III-IV) and 68% for low-grade tumors (WHO I–II) for differentiation of high from low-grade gliomas by low uptake in the low-grade tumors (29–32, 38, 39). The diagnostic accuracy of 11C-MET is slightly lower than FET (11, 40), which is related to the higher affinity of 11C-MET for inflammation (37). FDOPA as a dopamine analog metabolize through monoamine oxidases or catechol-O-methyltransferase, it labels to 18F (18F-FDOPA) and used for neuroendocrine tumors (41) and neurological applications. A study found that FDOPA could diagnose the recurrence lesions with a sensitivity of 100% and specificity of 85.7% vs. 47.6% and 100% of FDG PET, respectively (42). Therefore, FDOPA is superior to 18F-FDG PET for assessment of recurrence in low-grade tumors while it makes no significant difference in high-grade gliomas (42, 43). A comparative study found no significant difference between FDOPA and FET in high-grade gliomas, while uptake ratios were 10%–15% higher for FET compared to FDOPA (44). High levels of 11C-AMT accumulate in gliomas via the Kynurenine pathway, which is responsible for producing NAD + from the degradation of tryptophan, an essential amino acid (45). In patients with positive recurrent glioma on MRI, AMT uptake by the tumor may differentiate recurrent glioma from radiation injury in PET study (46). Low FLT uptake in the brain cortex caused to diagnose the small malignant lesions (47). Previous investigations revealed that 18F-FACBC PET had a potential for use in primary staging in gliomas (48–52). In a preliminary study of 6 patients, Tsuyuguchi et al. found that 18F-FACBC might provide a better response than 11C-MET for the initial detection as well as the detection of high-grade recurrent gliomas (49). Unsurprisingly, the results of 18F-FACBC PET/MR imaging were significantly better than the results of 11C-MET PET/MRI (82% vs. 10%). In summary, compared to 11C-MET, 18F-FACBC has a higher detection rate in recurrent and progressing gliomas and their images have a better contrast due to the lower background in the normal brain cortex (51). Figure 1 illustrates a 58-year-old man with high-grade glioma who underwent brain 18F-FET PET/CT imaging. On 18F-FET PET/CT, there was intense radiotracer uptake in the left parietal lobe whereas T2-W MRI showed a lesion with no change in enhancement in post-contrast imaging.
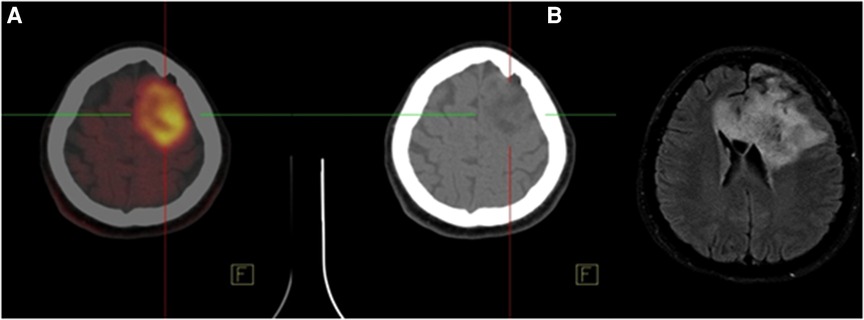
Figure 1. A 58-year-old man with high-grade glioma underwent brain 18F-FET PET/CT indicated intense radiotracer uptake (SUVmax = 7.68) in the left parietal lobe. (A) Accordingly, the tumor showed no change in Gd enhancement on FLAIR axial MRI with Gd. (B) Therefore, recurrence or residual disease was noted in the left parietal lobe.
In a systematic review of the recent studies, both amino acid PET tracers and perfusion-weighted imaging modalities were able to detect high-grade tumors, identify tumor recurrence, differentiate recurrence from treatment effects, and predict survival. Amino acid PET tracers performed better on these measures than perfusion-weighted imaging; however, they had the strongest results when combined. Studies of amino acid PET tracers with magnetic resonance spectroscopy (MRS) demonstrated that both modalities had diagnostic potential but MET PET and FDOPA PET performed better than MRS (53).
New imaging targets
Tumor hypoxia
Hypoxia is a main factor in treatment outcome and prognosis in different cancers, including glioma (54). Multiple radiotracers can be used to visualize hypoxia or sense oxygen levels. The tracer 18F-Fluoromisoinodazole (18F-FMISO) was developed for glioma imaging; however, its success is limited due to low sensitivity for differentiating normoxic from hypoxic tissue as well as a low blood brain barrier permeability (55, 56). Nevertheless, some results such as immunohistochemistry (IHC) findings, suggest that additional clinical studies are needed to evaluate 18F-FMISO as an imaging tool for hypoxia (57, 58). Moreover, the use of multiple PET radiopharmaceuticals is suggested to improve the planning for patient treatment and monitoring due to enhancing tumor detection and metabolic and genetic characterization. However, further research is required to eliminate the technical and logical issues of this technique and develop the concept of simultaneous multiplexing of 18F-FMISO with other PET oncology tracers (54). 18F-labeled flouroazomycin arabinoside (18F-FAZA) has the potential to be a promising alternative to 18F-FMISO offering an improved tumor-to-background ratio regarding faster blood clearance (59, 60). There is preliminary evidence that 18F-FAZA PET may be useful for assessing radiotherapy response in glioblastoma patients (61).
Translocator protein (TSPO)
Like peripheral cancers, brain cancer cells trigger inflammation as part of the immune response. A wide range of neuroinflammatory conditions, including high-grade GBM, have been studied with translocator protein 18 kDa (TSPO) PET imaging (62–66). The TSPO, previously known as the peripheral benzodiazepine receptor, is highly expressed in GBM and plays a critical role in essential mitochondria-based physiological processes (67, 68). According to several studies, there is a positive correlation between TSPO expression and the grade of malignancy and glioma cell proliferation; however, it has a negative correlation with survival in patients with glioma (69–71).
Cellular proliferation
3′-deoxy-3′-fluorothymidine (FLT) was used as an antiviral compound in patients with HIV for the first time (72). It's absorbed by cells and is subsequently phosphorylated by thymidine kinase 1 (TK1), which causes intracellular trapping within the cell. In this way, FLT retention within the cell provides a measure of TK activity, a key enzyme for cell proliferation (73). The increase in TK1 activity in normal cells occurs only during the DNA synthetic phase (72), while it occurs more and permanently in malignant cells. 18F-FLT uptake has a strong correlation between SUV of 18F-FLT and the proliferative status in lung cancer, colorectal carcinoma, non-Hodgkin's lymphoma, and melanoma (74–77). 18F-FLT is also considered to be an attractive PET tracer for the imaging of brain tumors due to the low uptake of 18F-FLT in the intact brain tissue and providing a low-background cerebral image (78). Furthermore, unlike 18F-FET, 18F-FLT requires a breakdown of the blood-brain barrier for it to be absorbed (79). Based on the results of a preliminary study in a low number patients with newly diagnosed gliomas, FLT uptake heterogeneity using textural features seemed to be useful for the assessment of proliferation and potential prediction of survival in these patients (80).
18F-FLT PET as a marker of cellular proliferation was compared with 18F-FDG in 25 patients with newly diagnosed or previously treated glioma. The results showed that 18F-FLT was more sensitive than 18F-FDG, correlated better with Ki-67 values, and appeared to be a promising tracer as a substitute marker of proliferation in these patients (81). For recurrent malignant glioma patients treated with bevacizumab and irinotecan, kinetic parameters from FLT provided sufficient information to predict the overall survival compared to those from FDOPA (82). A meta-analysis study indicated that 18F-FLT PET had a moderately better overall accuracy for diagnosing glioma recurrence compared with 18F-FDG (83).
Fernandez et al. determined the probability of combining 18F-FLT PET and MRI to improve the detection of tumoral tissue compared to MRI alone in 13 patients with newly diagnosed glioma. They also evaluated whether 18F-FLT uptake had a prognostic value by studying its association with histopathological features. According to the results, a combination of MRI and 18F-FLT PET detected additional tumor tissue, which might lead to a more complete surgical resection. The negative predictive value of a negative PET was also increased when combined with a negative MRI. Nevertheless, 18F-FLT underestimated the margins of the lesion and did not correlate with histopathological findings (84).
According to Nikaki et al. 18F-FLT accumulates in metastatic lesions, and it is highly sensitive and accurate. They reported that it could be used as a complementary tool to other imaging procedures. The tumor-to-background ratio and SUVmax provided the best diagnostic accuracy, while PV50% (proliferation × volume) and PV75% were of less diagnostic significance (85).
Poly ADP-ribose polymerase (PARP-1)
One of the most abundant isoforms of PARP enzymes is nuclear enzyme poly(ADP-ribose) polymerase 1 (PARP-1) (86). Among the 18 members of the PARP family, PARP-1 is the founder and major isoform with diverse regional expression, subcellular distribution, and domain composition with mono (ADP-ribosylation) and poly (ADP-ribosylation) activities (87).
Several physiological functions of Poly(ADP-ribosyl)ation (PARylation) have been implicated, including DNA repair, gene transcription, cell cycle progression, cell death, chromatin function, and genomic stability (88). Since PARP becomes activated in response to DNA breaks, understanding how various endogenous species can induce DNA strand breaks, and thus activate PARP, in various diseases has become increasingly important. Several studies have demonstrated that neutralizing peroxynitrite and/or inhibiting PARP pharmacologically or genetically is effective in treating cardiovascular, inflammatory, vascular, and neurodegenerative diseases by preventing cell death as well as down-regulating multiple inflammatory pathways (89–91). Despite PARP1's therapeutic potential, it has also attracted attention as a target for imaging agents. Reiner et al. developed a PARP agent for PET/CT imaging based on the Olaparib scaffold using rapid bioorthogonal conjugation chemistries (18F-BO) (92). 18F-BO (biorthogonal olaparib) was produced as the first inhibitor for PET imaging. Another specific target, 18F-PARPi, has been successfully used in GBM cell lines with high binding to peripheral tumors. It has been shown that the biodistribution and uptake of this tracer in the brain is very low around 2 h post-injection. In comparison to previous amino acid-based tracers, PARPi has a lower uptake in brain tumors with a lower background uptake in the cortex (93). As an important point, small PARPi molecules are needed for penetrating through the BBB as a major obstacle for any successful radiotracer targeting GBM. This limitation is mostly related to poor BBB penetration and high chemoresistance of GBM cells (94). With a high precision and good signal/noise ratio, 18F-PARPi can be used to visualize glioblastoma in xenograft and orthotopic mouse models. It offers new opportunities to image tumor growth and monitor interventions non-invasively (95).
18F-PARPi-FL, as a bimodal fluorescent/PET agent, is used for PARP1 imaging in glioblastoma cells. A study found that PARPi-FL could be radiolabeled and used to augment the limitations of the “fluorescence only” intraoperative imaging molecule (96). For this purpose, PET and SPECT imaging were performed in orthotopic glioblastoma models with 124I- and 131I-I2-PARP inhibitor (Olaparib) (97). The results showed the specific binding of the I2-PARPi tracer to PARP1 indicating the potential of this tracer for glioblastoma detection. Moreover, the results of an important preclinical study by Pirovano et al. provided the first full characterization of an auger-emitting PARP inhibitor, which showed a survival benefit in mouse models of GBM and verified the high potential of 123I-MAPi for clinical translation (98). With their short range of action, auger electrons possess high biological efficacy, which allows them to inflict DNA damage and cell death precisely. In addition to imaging tumor progression and monitoring therapy response, 123I-MAPi can also be used to calculate patient dosimetry.
Sigma-1, sigma-2
Human tumor cell lines including C6 glioma, neuroblastoma, and NG108-15 neuroblastoma-glioma hybrid express sigma 1 and sigma 2 receptors. Sigma 1 receptors are associated with invasiveness, while sigma 2 receptors are associated with proliferation. These two receptors are interesting targets for PET imaging in glioblastomas (99). Initially, 11C-SA4503 sigma-1 based tracer was prepared as the first PET ligand for GBM (100). Moreover, fluorinated sigma-1 receptor ligands were developed such as 18F-(S)-Fluspidine, 18F-FTC-146 and 18F-FPS with high binding affinity. Further efforts by radiopharmacists led to 125I- PIMBA and 18F-RHM-4 with high background binding to S2R (101, 102).
Epidermal growth factor receptor
Several malignancies have been associated with the human epidermal growth factor receptor (EGFR) family. All four members of this family, including HER1 (EGFR, ErbB1), HER2 (Neu, ErbB2), HER3 (ErbB3), and HER4 (ErbB4), play an important role in cell growth regulation, proliferation, and tumor migration (103). Epidermal growth factor receptor overexpression and activation of downstream signaling pathways, including RASYRAFYMAPK and PI3K/AKT, is considered to play a critical role in developing and progressing malignant gliomas (104, 105). Therefore, it is imperative to develop various treatment options, including tyrosine kinase inhibitors and monoclonal antibodies targeting EGFR, HER2, or both. Additionally, molecular imaging radiopharmaceuticals targeting EGFR family can improve the sensitivity and specificity of glioma detection (106). Following the use of 64Cu-DOTA-trastuzumab PET in breast cancer patients, it was found to be effective in detecting brain metastases (107). As part of a pilot study conducted in 2014, 11 patients with histopathologically proven GBM underwent PET/CT examination before surgery using 11C-PD153035. Six of the 8 patients with GBM were clearly visualized by 11C-PD153035 PET/CT, while 2 patients with GBM, 1 with anaplastic astrocytoma, and 2 with oligodendroglioma did not show any significant uptake. According to the results, 11C-PD153035 PET/CT was a promising tool for EGFR-targeted molecular imaging of GBM, which could translate into clinical applications to select patients suitable for EGFR-targeted therapies and to assess the early response of malignant gliomas to such therapies (108). A recent study evaluated the potential use of 89Zr-DFO-nimotuzumab in assessing the EGFR status in glioma (109).
Potential theranostics-based receptors
Integrin receptor family
Integrins are heterodimeric transmembrane glycoproteins containing 24 αβ (18 different α and 8 β) subunits that regulate cell survival, proliferation, and differentiation through their ability to transduce signals and interact with other cellular receptors [Table 1] (110).
Among them, the pro-angiogenic αvβ3 has been found to be highly expressed in high-grade brain tumors (112). This integrin belongs to the subclass of integrins that recognize the tripeptide sequence Arg-Gly-Asp (RGD) and is found in numerous extracellular matrix proteins, including fibronectin. While αvβ3 integrins were first introduced as a marker of tumor progression and angiogenesis, specifically on both tumor-associated EC and GB cells, αvβ5 is also used as a complementary attractive therapeutic target in GB (113, 114, 119, 127–129). Numerous studies confirmed the pro-tumorigenic and pro-angiogenic activity of αvβ3/αvβ5 and most recently α6β1 in GBM (130, 131), which makes them suitable for anticancer therapy (132). In fact, comparative IHC of integrins in GB has shown an overexpression of α2, α3, α4, α5, α6 and β1 (133) as well as β3/αvβ3, αvβ5, and αvβ8 (134). Moreover, αvβ3 is preferentially expressed at the invasion front of GBM. Integrins, as the main link between a cell and extracellular matrix, play an essential role in tumor invasion in the complex regulatory network of tumor progression (135). Due to its inherent safety and ability to target both tumor and endothelial cells, RGD peptide can be used as a selective carrier for delivering anti-cancer drugs. In this regard, a number of RGD peptide drug conjugates have been proposed as targeted drug delivery systems (136, 137). Radiolabeled cyclic RGD peptides in combination with radio-immunotherapy enhance therapeutic regimens with rapid and accurate detection of tumor growth and metastasis (138, 139).
Over the past decades, several radiotracers targeting αvβ3 have been developed and investigated for clinical applications. Many of them are based on tripeptide RGD due to its high affinity and specificity for integrin αvβ3. All of the investigated RGD peptides have shown very similar in vivo distribution with high uptake in the urinary tract, due to the urinary elimination of the tracer, as well as moderate liver and intestine uptake. In one study, a sugar amino acid was conjugated to the cyclic peptide c(RGDfK) to design 18F-galacto-RGD PET tracer (140). In 18F-Galacto-RGD PET images, the tracer uptake significantly correlated with the amount of integrin 3 expressed in tumor samples based on IHC examination. The data suggested that 18F-Galacto-RGD PET might be a promising tool for planning and monitoring individualized cancer therapies targeting this integrin. A first-in-human study of 64Cu-labeled long-acting integrin αvβ3 targeting molecule, 64Cu-NOTA-EB-RGD, indicated the safety, favorable dosimetry profile, and pharmacokinetic properties. In three GBM patients, the EB-derived albumin-binding moiety present in 64Cu-NOTA-EB-RGD resulted in more efficient pharmacokinetics and a higher contrast. Theranostic applications for 64Cu-labeled EB-RGD such as 67Cu, 177Lu, 90Y or 225Ac were recommended (141). Recently, the in vitro and in vivo characteristics of a 68Ga-radiolabeled dimeric RGD, 68Ga-NODAGA-c(RGDyK)2, were investigated in a glioblastoma multiforme tumor model. 68Ga-RGD peptides showed better imaging properties than 18F-FDG and 18F-FLT in a glioblastoma tumor model in mice. Except in the excretory organs, 68Ga-RGD peptides showed higher tumor-to-background ratios (142). A study evaluated the efficacy of 68Ga-NOTA-RGD dimer for noninvasive integrin αvβ3 imaging in preoperative patients with different grades of glioma. According to the results, it could evaluate glioma demarcation more precisely compared to 18F-FDG PET/CT because of being specific to brain tumors, but not to brain parenchyma, except for the choroid plexus. Moreover, 68Ga-RGD SUVmax had a significant correlation with glioma grade (143). Lo et al. developed a long-circulation integrin-targeted molecule consisting of a DOTA chelator, a truncated Evans blue dye (EB), a modified linker, and cRGDfK peptide. 111In-DOTA-EB-cRGDfK showed significant tumor accumulation with a high tumor-to-background ratio at 24 h post-injection in the preclinical phase. The data suggested that the ability to specifically bind to αvβ3-expressing lesions present in the radiolabeled DOTA-EB-cRGDfK could be considered as a promising factor for glioblastoma tumor imaging indicating its potential for use as a theranostic radiopharmaceutical (144).
Chemokine receptor-4 (CXCR4)
Chemokine receptor-4 (CXCR4) was introduced as a member of the G-protein-coupled chemokine receptor family. It affects embryogenesis, neo-angiogenesis, hematopoiesis, and inflammation. In addition to hematological malignancies, solid neoplasias express CXCR4 chemokine receptors. The activation of CXCR4 by CXCL12 induces migration and/or survival of neoplastic cells, such as tumor cells from brain tumors (neuronal and glial tumors), colorectal cancers, prostate cancer, melanoma, renal cell cancer, neuroblastoma cells, and ovarian cancer (145). One study found that in human glioma cell lines and primary tumors, CXCR4 expression correlated directly with malignancy. CXCR4 activation induced tumor cell chemotaxis and increased the production of vascular endothelial growth factor. Additionally, glioma cells expressing higher levels of CXCR4 formed more rapidly growing and lethal tumors in nude mice (146). A poorer prognosis was observed after surgery in patients with CXCR4-positive gliomas (137).
68Ga-Pentixafor was developed as a radiolabeled cyclic pentapeptide with high affinity to CXCR4 (147). The researchers found that 68Ga-Pentixafor PET imaging would be a promising radiotracer for the management of treatment planning in high-grade gliomas. Lapa et al. demonstrated that non-invasive imaging of CXCR4 in human malignant glioma using 68Ga-Pentixafor was feasible (147). They showed that 68Ga-Pentixafor PET in high-grade glioma yielded positive and receptor expression of malignant cells could be confirmed by immunohistochemical workup. Due to the extremely low background activity uptake of 68Ga-Pentixafor in the brain, it is very favorable and has a significantly higher tumor uptake compared to amino acid tracers such as 18F-FET, which confirms the high specificity of the tracer. In this regard, radioligand agents such as 177Lu in combination with Pentixafor seem to be a promising new approach for glioblastoma therapy. CXCR4-directed therapy alone or with temozolomide has already yielded promising results.
Figure 2 shows a 62-year-old man with high-grade glioma who underwent 68Ga-CXCR4 PET/CT. A large hypo-dense cerebral lesion with good tumor delineation was observed in the right parieto-occipital lobe. Figure 3 demonstrates 68Ga-Pentixather and 68Ga-FAPI imaging in a 55-year-old patient with a history of GBM in the right frontotemporal lobe that underwent surgery and external beam radiation therapy and received 177Lu-DOTATATE.
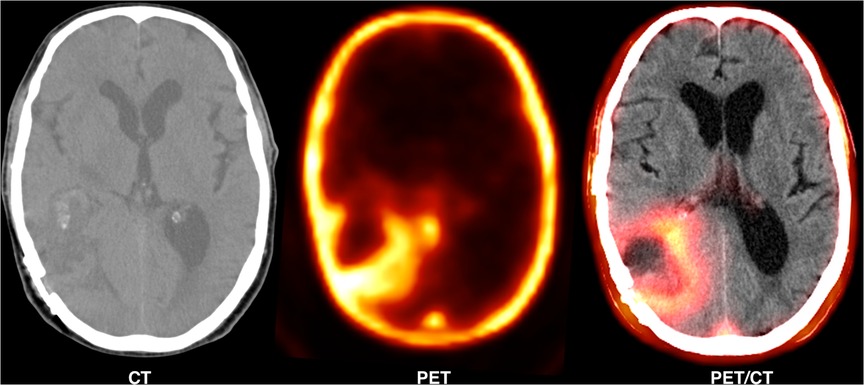
Figure 2. A 62-year-old man with high-grade glioma (WHO IV) that underwent 68Ga-pentixafor PET/CT. A large hypo-dense cerebral lesion (7*5.5 cm) was observed in the right parieto-occipital lobe with 68Ga-Pentixafor (Pars-CixaforTM) uptake (SUVmax: 1.87), central photopenia (probably necrosis) and good tumor delineation.
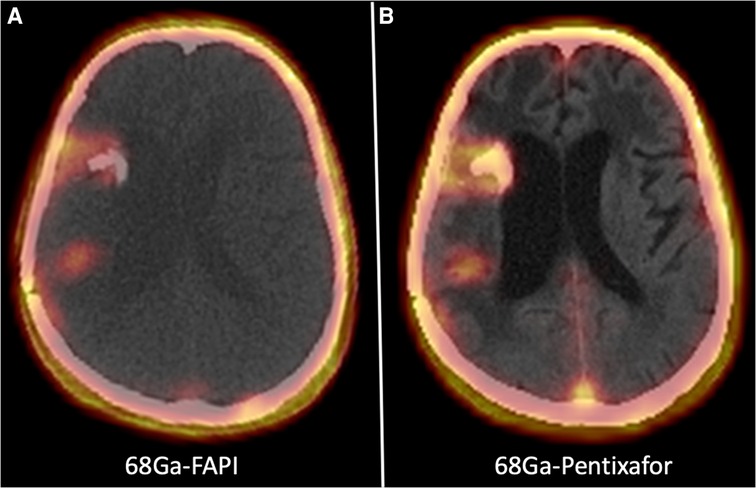
Figure 3. A 55-year-old patient with a history of GBM in the right frontotemporal lobe that underwent surgery and external beam radiation therapy and received 177Lu-DOTATATE. On 68Ga-Pentixather and 68Ga-FAPI imaging, there were three cerebral mass lesions in the right frontal and temporoparietal lobs, including a 11 × 15 mm lesion in the temporal lobe with SUV max = 4.15 (68Ga-Pentixather) and SUVmax = 4.154 (68Ga-FAPI), a 27 × 15 mm lesion in the temporal lobe (peri ventricular) with SUVmax = 1.97 (68Ga-Pentixather) and SUVmax = 2.68 (68Ga-FAPI), and a third lesion with a diameter of 12 × 14 mm in the right temporal lobe with SUVmax = 2.23 (68Ga-Pentixather) and 1.82 (68Ga-FAPI).
Somatostatin receptor
As a cyclic neuropeptide, somatostatin is well known in nuclear medicine for diagnosis and treatment of neuroendocrine tumors (148). The majority of the human tumors overexpress one or more of the five distinct human SSTR subtype receptors (149). Based on preliminary results from the MAURITIUS trial, of 43 glioblastoma patients treated with 400 to 3,700 MBq of 90Y-DOTA-lanreotide in fractionated one-to-six cycles, 5 cases showed regressive disease (reduction of more than 25% of tumor size), 14 cases showed stable disease, and 24 patients had progressive disease (increase of more than 25% of tumor size). In addition, 5 patients reported a subjective improvement in the quality of life measures (150). The authors reported that preclinical data and clinical studies confirmed the promise usefulness of radiolabeled lanreotide for tumor diagnosis and therapy. A case study reported that locally injected 90Y-DOTATOC in three patients with grade IV recurrent glioblastoma was feasible and well tolerated. An epileptic seizure recurred in one patient and a transient mild headache occurred on the day of the radiopharmaceutical application in another patient. This approach showed an attractive strategy for treating locally recurring or progressing glioblastoma (151).
Prostate-specific membrane antigen (PSMA)
PSMA is a type II transmembrane glycoprotein. Its overexpression is positively associated with higher tumor grades and stages in prostate cancer. PSMA expression is not limited to prostate cancers. Some tissues, such as salivary glands, express PSMA physiologically, and monoclonal antibodies to the extracellular domain of PSMA are also highly reactive with tumor vasculature in a variety of cancers, such as lung, breast, and colon carcinoma (152, 153). In one study, a single case of a glioblastoma patient was analyzed in whom the tumor also expressed PSMA on its neo-vasculature (154). Multiple immunohistopathological studies have confirmed PSMA expression in the tumoral vessels of high-grade gliomas (155–159).
With the development of 68Ga-labeled PSMA, a PSMA-targeted small molecule labeled with 68Ga, the researchers also use it as a therapeutic target with different radionuclides, e.g., 177Lu. 68Ga-PSMA is increasingly used in the routine clinical assessment of prostate cancer patients in the initial staging of intermediate or high-risk prostate cancers and in restaging after biochemical clue of tumor recurrence. With its extremely low background uptake in the normal brain tissue and a high tumor-to-brain ratio, 68Ga-PSMA-11 PET/CT is highly promising for the diagnosis of recurrent GBM (160). Nevertheless, a low tumor-to-liver ratio observed in a study by Kunikowska et al. suggested that radiolabeled PSMA ligands may have a limited role in targeted radionuclide therapy of recurrent GBM (160). In 2021, a re-resection of a recurrent tumor following radiochemotherapy and subsequent chemotherapy was performed in 16 patients with de-novo glioblastoma (161). The results showed that both the initial diagnosis and recurrence of glioblastoma exhibited PSMA expression. The authors argued that high vascular PSMA expression upon recurrence appeared to be associated with a poor prognosis; therefore, PSMA expression in recurrent GBM might serve as a promising target for theranostic approaches. The authors recommended further studies on PSMA PET imaging and PSMA-directed radioligand therapy in patients with brain tumors. PSMA PET imaging was used to assess the potential of PMSA radioligand therapy in syngeneic GL261 GBM models in one study (162). The results showed that although 18F-PSMA-1007 PET imaging of GL261 tumor-bearing mice was feasible and produced high tumor-to-background ratio, absolute tumor uptake values remained low, suggesting the limited application of the GL261 model for PSMA-directed therapy studies.
Fibroblast activated protein
During recent decades, numerous radiotracers and their application in neuro-oncology have been investigated in gliomas. Functional imaging techniques, as complementary tools to structural MRI, are highly needed for tumor entities such as anaplastic astrocytoma and GBM (163). As discussed earlier, while amino acid-based tracers such as FET have shown good results in comparison with FDG, other promising radiotracers such as fibroblast activated protein inhibitors (FAPIs) can also detect tumors based on the expression of fibroblast activation protein in the tumor stroma in cancer-activated fibroblasts of GBM.
Various types of FAPI have been already established such as FAPI-02/04-46/74 and their biodistribution in GBM patients is currently under investigation (164, 165). As a pioneer researcher in the production of FAP, Giesel et al. assessed the biodistribution of 68Ga-FAPI-02/04 in eight patients with head and neck cancer (166). The results showed that tumor-to-background ratios of FAP-specific PET were almost similar to 18F-FDG-PET. Röhrich et al. showed increased FAPI-02/04 uptake in 13 patients with grade III/IV gliomas and high-grade glioblastomas (167). Additionally, Chen et al. compared 68Ga-DOTA-FAPI-04 and 18F-FDG PET/CT for the diagnosis of primary and metastatic lesions in patients with various types of cancer including four glioma patients (two GBM, one grade II glioma, and one grade III glioma). However, the absolute uptake of FAP for tumor was low and the tumor-to-background ratio was high, lower uptake of FAP with a higher tumor-to-background ratio were observed in gliomas in other brain studies (168–170).
A case report study by Ballal1 et al. showed the possibility of a theranostic approach of 68Ga-DOTA.SA.FAPi PET/CT-guided 177Lu-DOTA.SA.FAPi radionuclide therapy in an end-stage breast cancer patient with confirmed brain metastasis (171). The patient received a single cycle of 177Lu-DOTA.SA.FAPi. There was physiological uptake of 177Lu-DOTA.SA.FAPi in the liver, kidneys, pancreas, and background muscle, and an intense accumulation of radiotracer was noted in all lesions in agreement with 68Ga-DOTA.SA.FAPi PET/CT scans. The authors declared that in patients not responding to conventional treatment options, 68Ga-DOTA.SA.FAPi PET/CT-guided 177Lu-DOTA.SA.FAPi therapy might offer a new opportunity for a theranostic approach in breast cancer therapy.
Moon et al. reported better tumor retention by introducing squaric acid (SA) as a linker into the FAP inhibitor yielding DATA5m.SA.FAPi and DOTA.SA.FAPi (172). Wang et al. demonstrated that 68Ga-DOTA.SA.FAPi PET/CT and 18F-FDG PET/CT had similarly high SUL uptake values in the primary site of head and neck cancers (173). In another study by Ballal et al. 68Ga-DOTA.SA.FAPi identified additional lesions in the brain that could not be detected on 18F-FDG PET/CT (174). According to the results, lower cortex uptake of 68Ga-DOTA.SA.FAPi in the brain makes it an ideal radiotracer where 18F-FDG PET/CT fails to detect brain tumors.
Figure 4. A 55-year-old man with recurrent high-grade glioma tumor undergoing 68Ga-FAPI PET/CT (left side) and one cycle of 177Lu-FAPI (right side).
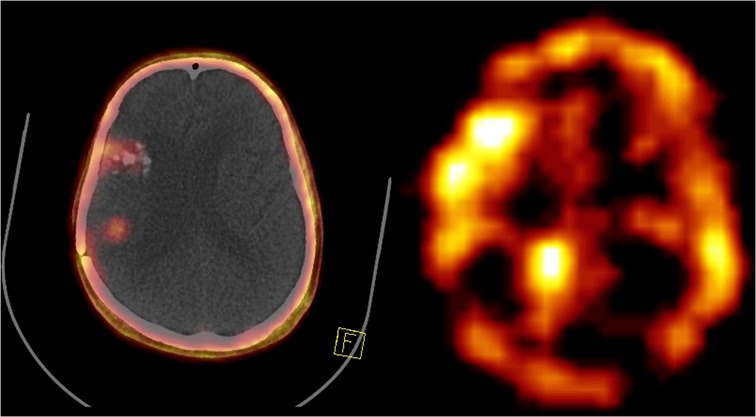
Figure 4. A 55-year-old man with recurrent high-grade glioma in the right temporoparietal region underwent 68Ga-FAPI PET/CT showing three cerebral lesions in the right frontal and right temporoparietal regions (left side). The patient received one cycle of 177Lu-FAPI (right side).
Conclusion
Glioblastoma multiforme is a fast-growing, invasive brain tumor that is typically associated with fatal outcomes. In recent years, new PET biomarkers have opened new horizons to evaluating a wide range of biochemical processes in terms of the diagnosis, staging, treatment planning, and monitoring disease progression and response to therapy of GBM. Along with the therapeutic potential of nuclear medicine and its successful theranostic applications in different malignancies, it could serve as an additional therapeutic option as neurotheranostics for GBM. Although the majority of these compounds require further clinical investigation, they may have a great potential for combined therapies.
Author contributions
All authors contributed to the article and approved the submitted version.
Conflict of interest
The authors declare that the research was conducted in the absence of any commercial or financial relationships that could be construed as a potential conflict of interest.
Publisher's note
All claims expressed in this article are solely those of the authors and do not necessarily represent those of their affiliated organizations, or those of the publisher, the editors and the reviewers. Any product that may be evaluated in this article, or claim that may be made by its manufacturer, is not guaranteed or endorsed by the publisher.
References
1. Louis DN, Perry A, Reifenberger G, Von Deimling A, Figarella-Branger D, Cavenee WK, et al. The 2016 world health organization classification of tumors of the central nervous system: a summary. Acta Neuropathol. (2016) 131(6):803–20. doi: 10.1007/s00401-016-1545-1
2. Qazi MA, Vora P, Venugopal C, Sidhu SS, Moffat J, Swanton C, et al. Intratumoral heterogeneity: pathways to treatment resistance and relapse in human glioblastoma. Ann Oncol. (2017) 28(7):1448–56. doi: 10.1093/annonc/mdx169
3. Tirosh I, Suvà ML. Tackling the many facets of glioblastoma heterogeneity. Cell Stem Cell. (2020) 26(3):303–4. doi: 10.1016/j.stem.2020.02.005
4. Pene F, Courtine E, Cariou A, Mira JP. Toward theragnostics. Crit Care Med. (2009) 37(1 Suppl):S50–8. doi: 10.1097/CCM.0b013e3181921349
5. Sikkandhar MG, Nedumaran AM, Ravichandar R, Singh S, Santhakumar I, Goh ZC, et al. Theranostic probes for targeting tumor microenvironment: an overview. Int J Mol Sci. (2017) 18(5):1036. doi: 10.3390/ijms18051036
6. White ML, Zhang Y, Yu F, Kazmi SJ. Diffusion tensor MR imaging of cerebral gliomas: evaluating fractional anisotropy characteristics. Am J Neuroradiol. (2011) 32(2):374–81. doi: 10.3174/ajnr.A2267
7. Zlotnik A, Burkhardt AM, Homey B. Homeostatic chemokine receptors and organ-specific metastasis. Nat Rev Immunol. (2011) 11(9):597–606. doi: 10.1038/nri3049
8. Santra A, Kumar R, Sharma P, Bal C, Kumar A, Julka PK, et al. F-18 FDG PET-CT in patients with recurrent glioma: comparison with contrast enhanced MRI. Eur J Radiol. (2012) 81(3):508–13. doi: 10.1016/j.ejrad.2011.01.080
9. Spence C, Pavani F, Driver J. Spatial constraints on visual-tactile cross-modal distractor congruency effects. Cogn Affect Behav Neurosci. (2004) 4(2):148–69. doi: 10.3758/CABN.4.2.148.15460922
10. Valk PE, Dillon WP. Radiation injury of the brain. Am J Neuroradiol. (1991) 12(1):45–62. PMID: 7502957; PMCID: PMC8367566.7502957
11. Delbeke D, Meyerowitz C, Lapidus RL, Maciunas RJ, Jennings MT, Moots PL, et al. Optimal cutoff levels of F-18 fluorodeoxyglucose uptake in the differentiation of low-grade from high-grade brain tumors with PET. Radiology. (1995) 195(1):47–52. doi: 10.1148/radiology.195.1.7892494
12. Kumar AJ, Leeds NE, Fuller GN, Van Tassel P, Maor MH, Sawaya RE, et al. Malignant gliomas: MR imaging spectrum of radiation therapy-and chemotherapy-induced necrosis of the brain after treatment. Radiology. (2000) 217(2):377–84. doi: 10.1148/radiology.217.2.r00nv36377
13. Grosu AL, Weber WA, Franz M, Stärk S, Piert M, Thamm R, et al. Reirradiation of recurrent high-grade gliomas using amino acid PET (SPECT)/CT/MRI image fusion to determine gross tumor volume for stereotactic fractionated radiotherapy. Int J Radiat Oncol Biol Phys. (2005) 63(2):511–9. doi: 10.1016/j.ijrobp.2005.01.056
14. Levivier M, Goldman S, Pirotte B, Brucher J-M, Balériaux D, Luxen A, et al. Diagnostic yield of stereotactic brain biopsy guided by positron emission tomography with [18F] fluorodeoxyglucose. J Neurosurg. (1995) 82(3):445–52. doi: 10.3171/jns.1995.82.3.0445
15. Santra A, Kumar R, Sharma P, Bal C, Julka PK, Malhotra A. F-18 FDG PET-CT for predicting survival in patients with recurrent glioma: a prospective study. Neuroradiology. (2011) 53(12):1017–24. doi: 10.1007/s00234-011-0898-3
16. Herholz K, Heiss WD. Positron emission tomography in clinical neurology. Mol Imaging Biol. (2004) 6(4):239–69. doi: 10.1016/j.mibio.2004.05.002
17. Ullrich RT, Kracht LW, Jacobs AH. Neuroimaging in patients with gliomas. Semin Neurol. (2008) 28(4):484–94. doi: 10.1055/s-0028-1083696
18. Albert NL, Weller M, Suchorska B, Galldiks N, Soffietti R, Kim MM, et al. Response assessment in neuro-oncology working group and European association for neuro-oncology recommendations for the clinical use of PET imaging in gliomas. Neuro Oncol. (2016) 18(9):1199–208. doi: 10.1093/neuonc/now058
19. Albert B, Zhang J, Noyvirt A, Setchi R, Sjaaheim H, Velikova S, et al. Automatic EEG processing for the early diagnosis of traumatic brain injury. Procedia Comput Sci. (2016) 96:703–12. doi: 10.1016/j.procs.2016.08.253
20. Drake LR, Hillmer AT, Cai Z. Approaches to PET imaging of glioblastoma. Molecules. (2020) 25(3):568. doi: 10.3390/molecules25030568
21. Galldiks N, Lohmann P, Albert NL, Tonn JC, Langen K-J. Current status of PET imaging in neuro-oncology. Neuro-oncol Adv. (2019) 1(1):vdz010. doi: 10.1093/noajnl/vdz010
22. Langen K-J, Galldiks N, Hattingen E, Shah NJ. Advances in neuro-oncology imaging. Nat Rev Neurol. (2017) 13(5):279–89. doi: 10.1038/nrneurol.2017.44
23. Langen K-J, Watts C. Amino acid PET for brain tumours—ready for the clinic? Nat Rev Neurol. (2016) 12(7):375–6. doi: 10.1038/nrneurol.2016.80
24. Law I, Albert NL, Arbizu J, Boellaard R, Drzezga A, Galldiks N, et al. Joint EANM/EANO/RANO practice guidelines/SNMMI procedure standards for imaging of gliomas using PET with radiolabelled amino acids and [18F] FDG: version 1.0. Eur J Nucl Med Mol Imaging. (2019) 46(3):540–57. doi: 10.1007/s00259-018-4207-9
25. Hayes AF. Partial, conditional, and moderated moderated mediation: quantification, inference, and interpretation. Commun Monogr. (2018) 85(1):4–40. doi: 10.1080/03637751.2017.1352100
26. Lohmann P, Stavrinou P, Lipke K, Bauer EK, Ceccon G, Werner J-M, et al. FET PET reveals considerable spatial differences in tumour burden compared to conventional MRI in newly diagnosed glioblastoma. Eur J Nucl Med Mol Imaging. (2019) 46(3):591–602. doi: 10.1007/s00259-018-4188-8
27. Kracht LW, Miletic H, Busch S, Jacobs AH, Voges J, Hoevels M, et al. Delineation of brain tumor extent with [11C]L-methionine positron emission tomography: local comparison with stereotactic histopathology. Clin Cancer Res. (2004) 10(21):7163–70. doi: 10.1158/1078-0432.CCR-04-0262
28. Lopez WO, Cordeiro JG, Albicker U, Doostkam S, Nikkhah G, Kirch RD, et al. Correlation of (18)F-fluoroethyl tyrosine positron-emission tomography uptake values and histomorphological findings by stereotactic serial biopsy in newly diagnosed brain tumors using a refined software tool. Onco Targets Ther. (2015) 8:3803–15. doi: 10.2147/OTT.S87126
29. Hutterer M, Nowosielski M, Putzer D, Jansen NL, Seiz M, Schocke M, et al. [18f]-fluoro-ethyl-L-tyrosine PET: a valuable diagnostic tool in neuro-oncology, but not all that glitters is glioma. Neuro Oncol. (2013) 15(3):341–51. doi: 10.1093/neuonc/nos300
30. Jansen NL, Graute V, Armbruster L, Suchorska B, Lutz J, Eigenbrod S, et al. MRI-suspected low-grade glioma: is there a need to perform dynamic FET PET? Eur J Nucl Med Mol Imaging. (2012) 39(6):1021–9. doi: 10.1007/s00259-012-2109-9
31. Pichler BJ, Kolb A, Nägele T, Schlemmer H-P. PET/MRI: paving the way for the next generation of clinical multimodality imaging applications. J Nucl Med. (2010) 51(3):333–6. doi: 10.2967/jnumed.109.061853
32. Kratochwil C, Combs SE, Leotta K, Afshar-Oromieh A, Rieken S, Debus J, et al.. Intra-individual comparison of 18F-FET and 18F-DOPA in PET imaging of recurrent brain tumors. Neuro Oncol. (2014) 16(3):434–40. doi: 10.1093/neuonc/not199
33. Galldiks N, Langen K-J, Albert NL, Chamberlain M, Soffietti R, Kim MM, et al. PET Imaging in patients with brain metastasis—report of the RANO/PET group. Neuro Oncol. (2019) 21(5):585–95. doi: 10.1093/neuonc/noz003
34. Kebir S, Fimmers R, Galldiks N, Schäfer N, Mack F, Schaub C, et al. Late pseudoprogression in glioblastoma: diagnostic value of dynamic O-[2-(18F) fluoroethyl]-L-tyrosine PET. Clin Cancer Res. (2016) 22(9):2190–6. doi: 10.1158/1078-0432.CCR-15-1334
35. Pöpperl G, Götz C, Rachinger W, Gildehaus F-J, Tonn J-C, Tatsch K. Value of O-[2-(18F) fluoroethyl]-L-tyrosine PET for the diagnosis of recurrent glioma. Eur J Nucl Med Mol Imaging. (2004) 31(11):1464–70. doi: 10.1007/s00259-004-1590-1
36. Rachinger W, Goetz C, Pöpperl G, Gildehaus FJ, Kreth FW, Holtmannspötter M, et al. Positron emission tomography with O-[2-(18F) fluoroethyl]-l-tyrosine versus magnetic resonance imaging in the diagnosis of recurrent gliomas. Neurosurgery. (2005) 57(3):505–11. doi: 10.1227/01.NEU.0000171642.49553.B0
37. Tomura N, Kokubun M, Saginoya T, Mizuno Y, Kikuchi Y. Differentiation between treatment-induced necrosis and recurrent tumors in patients with metastatic brain tumors: comparison among 11C-methionine-PET, FDG-PET, MR permeability imaging, and MRI-ADC—preliminary results. Am J Neuroradiol. (2017) 38(8):1520–7. doi: 10.3174/ajnr.A5252
38. Unterrainer M, Vettermann F, Brendel M, Holzgreve A, Lifschitz M, Zähringer M, et al. Towards standardization of 18F-FET PET imaging: do we need a consistent method of background activity assessment? EJNMMI Res. (2017) 7(1):1–8. doi: 10.1186/s13550-017-0295-y
39. Brandsma D, van den Bent MJ. Pseudoprogression and pseudoresponse in the treatment of gliomas. Curr Opin Neurol. (2009) 22(6):633–8. doi: 10.1097/WCO.0b013e328332363e
40. Milker-Zabel S, Zabel-du Bois A, Henze M, Huber P, Schulz-Ertner D, Hoess A, et al. Improved target volume definition for fractionated stereotactic radiotherapy in patients with intracranial meningiomas by correlation of CT, MRI, and [68 Ga]-DOTATOC-PET. Int J Radiat Oncol Biol Phys. (2006) 65(1):222–7. doi: 10.1016/j.ijrobp.2005.12.006
41. Minn H, Kauhanen S, Seppänen M, Nuutila P. 18F-FDOPA: a multiple-target molecule. J Nucl Med. (2009) 50(12):1915–8. doi: 10.2967/jnumed.109.065664
42. Karunanithi S, Sharma P, Kumar A, Khangembam BC, Bandopadhyaya GP, Kumar R, et al. 18F-FDOPA PET/CT for detection of recurrence in patients with glioma: prospective comparison with 18F-FDG PET/CT. Eur J Nucl Med Mol Imaging. (2013) 40(7):1025–35. doi: 10.1007/s00259-013-2384-0
43. Karunanithi S, Sharma P, Kumar A, Khangembam BC, Bandopadhyaya GP, Kumar R, et al. Comparative diagnostic accuracy of contrast-enhanced MRI and 18F-FDOPA PET-CT in recurrent glioma. Eur Radiol. (2013) 23(9):2628–35. doi: 10.1007/s00330-013-2838-6
44. Herholz K. Brain tumors: an update on clinical PET research in gliomas. Semin Nucl Med. (2017) 7(1):5–17. doi: 10.1053/j.semnuclmed.2016.09.004
45. Chen Y, Guillemin GJ. Kynurenine pathway metabolites in humans: disease and healthy states. Int J Tryptophan Res. (2009) 2:S2097. doi: 10.4137/IJTR.S2097
46. Alkonyi B, Barger GR, Mittal S, Muzik O, Chugani DC, Bahl G, et al. Accurate differentiation of recurrent gliomas from radiation injury by kinetic analysis of α-11C-methyl-L-tryptophan PET. J Nucl Med. (2012) 53(7):1058–64. doi: 10.2967/jnumed.111.097881
47. Yamamoto M, Sato Y, Serizawa T, Kawabe T, Higuchi Y, Nagano O, et al. Subclassification of recursive partitioning analysis class II patients with brain metastases treated radiosurgically. Int J Radiat Oncol Biol Phys. (2012) 83(5):1399–405. doi: 10.1016/j.ijrobp.2011.10.018
48. Bogsrud T, Loendalen A, Brandal P, Saxhaug C, Bach-Gansmo T. 18F-fluciclovine (FACBC) PET/CT in residual or recurrent gliomas. Soc Nuclear Med. (2016) 44(8):605–11. doi: 10.1097/RLU.0000000000002641
49. Tsuyuguchi N, Terakawa Y, Uda T, Nakajo K, Kanemura Y. Diagnosis of brain tumors using amino acid transport PET imaging with 18F-fluciclovine: a comparative study with L-methyl-11C-methionine PET imaging. Asia Ocean J Nucl Med Biol. (2017) 5(2):85. doi: 10.22038/aojnmb.2017.8843
50. Shoup TM, Olson J, Hoffman JM, Votaw J, Eshima D, Eshima L, et al. Synthesis and evaluation of [18F] 1-amino-3-fluorocyclobutane-1-carboxylic acid to image brain tumors. J Nucl Med. (1999) 40(2):331–8. PMID: 10025843.10025843
51. Kondo A, Ishii H, Aoki S, Suzuki M, Nagasawa H, Kubota K, et al. Phase IIa clinical study of [18F] fluciclovine: efficacy and safety of a new PET tracer for brain tumors. Ann Nucl Med. (2016) 30(9):608–18. doi: 10.1007/s12149-016-1102-y
52. Parent EE, Benayoun M, Ibeanu I, Olson JJ, Hadjipanayis CG, Brat DJ, et al. [18 F] fluciclovine PET discrimination between high-and low-grade gliomas. EJNMMI Res. (2018) 8(1):1–11. doi: 10.1186/s13550-018-0415-3
53. Stopa BM, Juhász C, Mittal S. Comparison of amino acid PET to advanced and emerging MRI techniques for neurooncology imaging: a systematic review of the recent studies. Mol Imaging. (2021) 2021:1–19. doi: 10.1155/2021/8874078
54. Bell C, Dowson N, Fay M, Thomas P, Puttick S, Gal Y, et al. Hypoxia imaging in gliomas with 18F-fluoromisonidazole PET: toward clinical translation. Semin Nucl Med. (2015) 45(2):136–50. doi: 10.1053/j.semnuclmed.2014.10.001
55. Valk PE, Mathis CA, Prados MD, Gilbert JC, Budinger TF. Hypoxia in human gliomas: demonstration by PET with fluorine-18-fluoromisonidazole. J Nucl Med. (1992) 33(12):2133–7. PMID: 1334136.1334136
56. Challapalli A, Carroll L, Aboagye EO. Molecular mechanisms of hypoxia in cancer. Clin Transl Imaging. (2017) 5(3):225–53. doi: 10.1007/s40336-017-0231-1
57. Kawai N, Lin W, Cao WD, Ogawa D, Miyake K, Haba R, et al. Correlation between ¹⁸F-fluoromisonidazole PET and expression of HIF-1α and VEGF in newly diagnosed and recurrent malignant gliomas. Eur J Nucl Med Mol Imaging. (2014) 41(10):1870–8. doi: 10.1007/s00259-014-2776-9
58. Kaur B, Khwaja FW, Severson EA, Matheny SL, Brat DJ, Van Meir EG. Hypoxia and the hypoxia-inducible-factor pathway in glioma growth and angiogenesis. Neuro Oncol. (2005) 7(2):134–53. doi: 10.1215/S1152851704001115
59. Piert M, Machulla HJ, Picchio M, Reischl G, Ziegler S, Kumar P, et al. Hypoxia-specific tumor imaging with 18F-fluoroazomycin arabinoside. J Nucl Med. (2005) 46(1):106–13. PMID: 15632040.15632040
60. Postema EJ, McEwan AJ, Riauka TA, Kumar P, Richmond DA, Abrams DN, et al. Initial results of hypoxia imaging using 1-alpha-D: -[5-deoxy-5-(18F)-fluoroarabinofuranosyl]-2-nitroimidazole (18F-FAZA). Eur J Nucl Med Mol Imaging. (2009) 36(10):1565–73. doi: 10.1007/s00259-009-1154-5
61. Mapelli P, Zerbetto F, Incerti E, Conte GM, Bettinardi V, Fallanca F, et al. 18F-FAZA PET/CT hypoxia imaging of high-grade glioma before and after radiotherapy. Clin Nucl Med. (2017) 42(12):e525–e6. doi: 10.1097/RLU.0000000000001850
62. Werry EL, Bright FM, Piguet O, Ittner LM, Halliday GM, Hodges JR, et al. Recent developments in TSPO PET imaging as A biomarker of neuroinflammation in neurodegenerative disorders. Int J Mol Sci. (2019) 20(13):3161. doi: 10.3390/ijms20133161
63. Amhaoul H, Hamaide J, Bertoglio D, Reichel SN, Verhaeghe J, Geerts E, et al. Brain inflammation in a chronic epilepsy model: evolving pattern of the translocator protein during epileptogenesis. Neurobiol Dis. (2015) 82:526–39. doi: 10.1016/j.nbd.2015.09.004
64. Banati RB, Newcombe J, Gunn RN, Cagnin A, Turkheimer F, Heppner F, et al. The peripheral benzodiazepine binding site in the brain in multiple sclerosis: quantitative in vivo imaging of microglia as a measure of disease activity. Brain. (2000) 123(Pt 11):2321–37. doi: 10.1093/brain/123.11.2321
65. Hasnain N, Mustafa RM, Bakhshi SK, Shamim MS. Efficacy of positron emission tomography in distinguishing brain tumours from inflammation. J Pak Med Assoc. (2020) 70(12(a)):2291–3. PMID: 33475617.33475617
66. Zinnhardt B, Müther M, Roll W, Backhaus P, Jeibmann A, Foray C, et al. TSPO imaging-guided characterization of the immunosuppressive myeloid tumor microenvironment in patients with malignant glioma. Neuro Oncol. (2020) 22(7):1030–43. doi: 10.1093/neuonc/noaa023
67. Papadopoulos V, Baraldi M, Guilarte TR, Knudsen TB, Lacapère JJ, Lindemann P, et al. Translocator protein (18 kDa): new nomenclature for the peripheral-type benzodiazepine receptor based on its structure and molecular function. Trends Pharmacol Sci. (2006) 27(8):402–9. doi: 10.1016/j.tips.2006.06.005
68. Casellas P, Galiegue S, Basile AS. Peripheral benzodiazepine receptors and mitochondrial function. Neurochem Int. (2002) 40(6):475–86. doi: 10.1016/S0197-0186(01)00118-8
69. Miettinen H, Kononen J, Haapasalo H, Helén P, Sallinen P, Harjuntausta T, et al. Expression of peripheral-type benzodiazepine receptor and diazepam binding inhibitor in human astrocytomas: relationship to cell proliferation. Cancer Res. (1995) 55(12):2691–5. PMID: 7780986.7780986
70. Vlodavsky E, Soustiel JF. Immunohistochemical expression of peripheral benzodiazepine receptors in human astrocytomas and its correlation with grade of malignancy, proliferation, apoptosis and survival. J Neurooncol. (2007) 81(1):1–7. doi: 10.1007/s11060-006-9199-9
71. Unterrainer M, Fleischmann DF, Vettermann F, Ruf V, Kaiser L, Nelwan D, et al. TSPO PET, tumour grading and molecular genetics in histologically verified glioma: a correlative (18)F-GE-180 PET study. Eur J Nucl Med Mol Imaging. (2020) 47(6):1368–80. doi: 10.1007/s00259-019-04491-5
72. Flexner C, van der Horst C, Jacobson MA, Powderly W, Duncanson F, Ganes D, et al. Relationship between plasma concentrations of 3′-deoxy-3′-fluorothymidine (alovudine) and antiretroviral activity in two concentration-controlled trials. J Infect Dis. (1994) 170(6):1394–403. doi: 10.1093/infdis/170.6.1394
73. Shields AF, Grierson JR, Dohmen BM, Machulla HJ, Stayanoff JC, Lawhorn-Crews JM, et al. Imaging proliferation in vivo with [F-18]FLT and positron emission tomography. Nat Med. (1998) 4(11):1334–6. doi: 10.1038/3337
74. Buck AK, Schirrmeister H, Hetzel M, Von Der Heide M, Halter G, Glatting G, et al. 3-deoxy-3-[(18)F]fluorothymidine-positron Emission tomography for noninvasive assessment of proliferation in pulmonary nodules. Cancer Res. (2002) 62(12):3331–4. PMID: 12067968.12067968
75. Cobben DC, Jager PL, Elsinga PH, Maas B, Suurmeijer AJ, Hoekstra HJ. 3′-18F-fluoro-3′-deoxy-L-thymidine: a new tracer for staging metastatic melanoma? J Nucl Med. (2003) 44(12):1927–32. PMID: 14660718.14660718
76. Wagner M, Seitz U, Buck A, Neumaier B, Schultheiss S, Bangerter M, et al. 3′-[18F]fluoro-3′-deoxythymidine [(18F)-FLT] as positron emission tomography tracer for imaging proliferation in a murine B-cell lymphoma model and in the human disease. Cancer Res. (2003) 63(10):2681–7. PMID: 12750297.12750297
77. Been LB, Suurmeijer AJ, Cobben DC, Jager PL, Hoekstra HJ, Elsinga PH. [18f]FLT-PET in oncology: current status and opportunities. Eur J Nucl Med Mol Imaging. (2004) 31(12):1659–72. doi: 10.1007/s00259-004-1687-6
78. Jacobs AH, Thomas A, Kracht LW, Li H, Dittmar C, Garlip G, et al. 18F-fluoro-L-thymidine And 11C-methylmethionine as markers of increased transport and proliferation in brain tumors. J Nucl Med. (2005) 46(12):1948–58. PMID: 16330557.16330557
79. Nowosielski M, DiFranco MD, Putzer D, Seiz M, Recheis W, Jacobs AH, et al. An intra-individual comparison of MRI, [18F]-FET and [18F]-FLT PET in patients with high-grade gliomas. PLoS ONE. (2014) 9(4):e95830. doi: 10.1371/journal.pone.0095830
80. Mitamura K, Yamamoto Y, Kudomi N, Maeda Y, Norikane T, Miyake K, et al. Intratumoral heterogeneity of (18)F-FLT uptake predicts proliferation and survival in patients with newly diagnosed gliomas. Ann Nucl Med. (2017) 31(1):46–52. doi: 10.1007/s12149-016-1129-0
81. Chen W, Cloughesy T, Kamdar N, Satyamurthy N, Bergsneider M, Liau L, et al. Imaging proliferation in brain tumors with 18F-FLT PET: comparison with 18F-FDG. J Nucl Med. (2005) 46(6):945–52. PMID: 15937304.15937304
82. Wardak M, Schiepers C, Cloughesy TF, Dahlbom M, Phelps ME, Huang SC. ¹⁸F-FLT and ¹⁸F-FDOPA PET kinetics in recurrent brain tumors. Eur J Nucl Med Mol Imaging. (2014) 41(6):1199–209. doi: 10.1007/s00259-013-2678-2
83. Li Z, Yu Y, Zhang H, Xu G, Chen L. A meta-analysis comparing 18F-FLT PET with 18F-FDG PET for assessment of brain tumor recurrence. Nucl Med Commun. (2015) 36(7):695–701. doi: 10.1097/MNM.0000000000000302
84. Fernandez P, Zanotti-Fregonara P, Eimer S, Gimbert E, Monteil P, Penchet G, et al. Combining 3′-deoxy-3′-[18F] fluorothymidine and MRI increases the sensitivity of glioma volume detection. Nucl Med Commun. (2019) 40(10):1066–71. doi: 10.1097/MNM.0000000000001056
85. Nikaki A, Papadopoulos V, Valotassiou V, Efthymiadou R, Angelidis G, Tsougos I, et al. Evaluation of the performance of 18F-Fluorothymidine positron emission tomography/computed tomography (18F-FLT-PET/CT) in metastatic brain lesions. Diagnostics (Basel). (2019) 9(1):17. doi: 10.3390/diagnostics9010017
86. Barr TL, Conley YP. Poly(ADP-ribose) polymerase-1 and its clinical applications in brain injury. J Neurosci Nurs. (2007) 39(5):278–84. doi: 10.1097/01376517-200710000-00004
87. Narne P, Pandey V, Simhadri PK, Phanithi PB. Poly(ADP-ribose)polymerase-1 hyperactivation in neurodegenerative diseases: the death knell tolls for neurons. Semin Cell Dev Biol. (2017) 63:154–66. doi: 10.1016/j.semcdb.2016.11.007
88. Pacher P, Szabo C. Role of the peroxynitrite-poly(ADP-ribose) polymerase pathway in human disease. Am J Pathol. (2008) 173(1):2–13. doi: 10.2353/ajpath.2008.080019
89. Szabó C, Ischiropoulos H, Radi R. Peroxynitrite: biochemistry, pathophysiology and development of therapeutics. Nat Rev Drug Discov. (2007) 6(8):662–80. doi: 10.1038/nrd2222
90. Pacher P, Beckman JS, Liaudet L. Nitric oxide and peroxynitrite in health and disease. Physiol Rev. (2007) 87(1):315–424. doi: 10.1152/physrev.00029.2006
91. Slade D. PARP And PARG inhibitors in cancer treatment. Genes Dev. (2020) 34(5-6):360–94. doi: 10.1101/gad.334516.119
92. Reiner T, Lacy J, Keliher EJ, Yang KS, Ullal A, Kohler RH, et al. Imaging therapeutic PARP inhibition in vivo through bioorthogonally developed companion imaging agents. Neoplasia. (2012) 14(3):169–77. doi: 10.1593/neo.12414
93. Kossatz S, Carney B, Schweitzer M, Carlucci G, Miloushev VZ, Maachani UB, et al. Biomarker-Based PET imaging of diffuse intrinsic pontine glioma in mouse models. Cancer Res. (2017) 77(8):2112–23. doi: 10.1158/0008-5472.CAN-16-2850
94. Drake LR, Hillmer AT, Cai Z. Approaches to PET imaging of glioblastoma. Molecules. (2020) 25(3):568. doi: 10.3390/molecules25030568
95. Carney B, Carlucci G, Salinas B, Di Gialleonardo V, Kossatz S, Vansteene A, et al. Non-invasive PET imaging of PARP1 expression in glioblastoma models. Mol Imaging Biol. (2016) 18(3):386–92. doi: 10.1007/s11307-015-0904-y
96. Carlucci G, Carney B, Brand C, Kossatz S, Irwin CP, Carlin SD, et al. Dual-Modality optical/PET imaging of PARP1 in glioblastoma. Mol Imaging Biol. (2015) 17(6):848–55. doi: 10.1007/s11307-015-0858-0
97. Salinas B, Irwin CP, Kossatz S, Bolaender A, Chiosis G, Pillarsetty N, et al. Radioiodinated PARP1 tracers for glioblastoma imaging. EJNMMI Res. (2015) 5(1):123. doi: 10.1186/s13550-015-0123-1
98. Pirovano G, Jannetti SA, Carter LM, Sadique A, Kossatz S, Guru N, et al. Targeted brain tumor radiotherapy using an auger emitter. Clin Cancer Res. (2020) 26(12):2871–81. doi: 10.1158/1078-0432.CCR-19-2440
99. Vilner BJ, John CS, Bowen WD. Sigma-1 and sigma-2 receptors are expressed in a wide variety of human and rodent tumor cell lines. Cancer Res. (1995) 55(2):408–13. PMID: 7812973.7812973
100. Toyohara J, Kobayashi T, Mita S, Ishiwata K. Application of [¹¹C]SA4503 to selection of novel σ₁ selective agonists. Nucl Med Biol. (2012) 39(8):1117–21. doi: 10.1016/j.nucmedbio.2012.06.004
101. Kashiwagi H, McDunn JE, Simon PO, Goedegebuure PS, Xu J, Jones L, et al. Selective sigma-2 ligands preferentially bind to pancreatic adenocarcinomas: applications in diagnostic imaging and therapy. Mol Cancer. (2007) 6(1):1–12. doi: 10.1186/1476-4598-6-48
102. John CS, Bowen WD, Fisher SJ, Lim BB, Geyer BC, Vilner BJ, et al. Synthesis, in vitro pharmacologic characterization, and preclinical evaluation of N-[2-(1′-piperidinyl) ethyl]-3-[125I] iodo-4-methoxybenzamide [P (125I) MBA] for imaging breast cancer. Nucl Med Biol. (1999) 26(4):377–82. doi: 10.1016/S0969-8051(98)00104-8
103. Arienti C, Pignatta S, Tesei A. Epidermal growth factor receptor family and its role in gastric cancer. Front Oncol. (2019) 9:1308. doi: 10.3389/fonc.2019.01308
104. Moriai T, Kobrin MS, Hope C, Speck L, Korc M. A variant epidermal growth factor receptor exhibits altered type alpha transforming growth factor binding and transmembrane signaling. Proc Natl Acad Sci U S A. (1994) 91(21):10217–21. doi: 10.1073/pnas.91.21.10217
105. Hackel PO, Zwick E, Prenzel N, Ullrich A. Epidermal growth factor receptors: critical mediators of multiple receptor pathways. Curr Opin Cell Biol. (1999) 11(2):184–9. doi: 10.1016/S0955-0674(99)80024-6
106. Bublil EM, Yarden Y. The EGF receptor family: spearheading a merger of signaling and therapeutics. Curr Opin Cell Biol. (2007) 19(2):124–34. doi: 10.1016/j.ceb.2007.02.008
107. Tamura K, Kurihara H, Yonemori K, Tsuda H, Suzuki J, Kono Y, et al. 64Cu-DOTA-trastuzumab PET imaging in patients with HER2-positive breast cancer. J Nucl Med. (2013) 54(11):1869–75. doi: 10.2967/jnumed.112.118612
108. Sun J, Cai L, Zhang K, Zhang A, Pu P, Yang W, et al. A pilot study on EGFR-targeted molecular imaging of PET/CT with 11C-PD153035 in human gliomas. Clin Nucl Med. (2014) 39(1):e20–6. doi: 10.1097/RLU.0b013e3182a23b73
109. Tang Y, Hu Y, Liu W, Chen L, Zhao Y, Ma H, et al. A radiopharmaceutical [(89)Zr]Zr-DFO-nimotuzumab for immunoPET with epidermal growth factor receptor expression in vivo. Nucl Med Biol. (2019) 70:23–31. doi: 10.1016/j.nucmedbio.2019.01.007
110. Campbell ID, Humphries MJ. Integrin structure, activation, and interactions. Cold Spring Harbor Perspect Biol. (2011) 3(3):a004994. doi: 10.1101/cshperspect.a004994
111. Anderson LR, Owens TW, Naylor MJ. Integrins in development and cancer. Biophys Rev. (2014) 6(2):191–202. doi: 10.1007/s12551-013-0123-1
112. Gladson CL. Expression of integrin alpha v beta 3 in small blood vessels of glioblastoma tumors. J Neuropathol Exp Neurol. (1996) 55(11):1143–9. doi: 10.1097/00005072-199611000-00005
113. Roth P, Silginer M, Goodman SL, Hasenbach K, Thies S, Maurer G, et al. Integrin control of the transforming growth factor-β pathway in glioblastoma. Brain. (2013) 136(Pt 2):564–76. doi: 10.1093/brain/aws351
114. Scaringi C, Minniti G, Caporello P, Enrici RM. Integrin inhibitor cilengitide for the treatment of glioblastoma: a brief overview of current clinical results. Anticancer Res. (2012) 32(10):4213–23. PMID: 23060541.23060541
115. Dijkgraaf I, Terry SY, McBride WJ, Goldenberg DM, Laverman P, Franssen GM, et al. Imaging integrin alpha-v-beta-3 expression in tumors with an 18F-labeled dimeric RGD peptide. Contrast Media Mol Imaging. (2013) 8(3):238–45. doi: 10.1002/cmmi.1523
116. Malric L, Monferran S, Gilhodes J, Boyrie S, Dahan P, Skuli N, et al. Interest of integrins targeting in glioblastoma according to tumor heterogeneity and cancer stem cell paradigm: an update. Oncotarget. (2017) 8(49):86947. doi: 10.18632/oncotarget.20372
117. Capasso D, Del Gatto A, Comegna D, Russo L, Fattorusso R, Saviano M, et al. Selective targeting of αvβ5 integrin in HepG2 cell line by RGDechi15D peptide. Molecules. (2020) 25(18):4298. doi: 10.3390/molecules25184298
118. Echavidre W, Picco V, Faraggi M, Montemagno C. Integrin-αvβ3 as a therapeutic target in glioblastoma: back to the future? Pharmaceutics. (2022) 14(5):1053. doi: 10.3390/pharmaceutics14051053
119. Delamarre E, Taboubi S, Mathieu S, Bérenguer C, Rigot V, Lissitzky JC, et al. Expression of integrin alpha6beta1 enhances tumorigenesis in glioma cells. Am J Pathol. (2009) 175(2):844–55. doi: 10.2353/ajpath.2009.080920
120. Takada Y, Ye X, Simon S. The integrins. Genome Biol. (2007) 8(5):1–9. doi: 10.1186/gb-2007-8-5-215
121. Hehlgans S, Haase M, Cordes N. Signalling via integrins: implications for cell survival and anticancer strategies. Biochim Biophys Acta. (2007) 1775(1):163–80. doi: 10.1016/j.bbcan.2006.09.001
122. Safa AR, Saadatzadeh MR, Cohen-Gadol AA, Pollok KE, Bijangi-Vishehsaraei K. Glioblastoma stem cells (GSCs) epigenetic plasticity and interconversion between differentiated non-GSCs and GSCs. Genes Dis. (2015) 2(2):152–63. doi: 10.1016/j.gendis.2015.02.001
123. Lathia JD, Heddleston JM, Venere M, Rich JN. Deadly teamwork: neural cancer stem cells and the tumor microenvironment. Cell Stem Cell. (2011) 8(5):482–5. doi: 10.1016/j.stem.2011.04.013
124. Gladson CL, Wilcox JN, Sanders L, Gillespie GY, Cheresh DA. Cerebral microenvironment influences expression of the vitronectin gene in astrocytic tumors. J Cell Sci. (1995) 108(Pt 3):947–56. doi: 10.1242/jcs.108.3.947
125. Humphries M. Integrin structure. Biochem Soc Trans. (2000) 28(4):311–40. doi: 10.1042/bst0280311
126. Veeravalli KK, Ponnala S, Chetty C, Tsung AJ, Gujrati M, Rao JS. Integrin α9β1-mediated cell migration in glioblastoma via SSAT and Kir4. 2 potassium channel pathway. Cell Signal. (2012) 24(1):272–81. doi: 10.1016/j.cellsig.2011.09.011
127. Gingras MC, Roussel E, Bruner JM, Branch CD, Moser RP. Comparison of cell adhesion molecule expression between glioblastoma multiforme and autologous normal brain tissue. J Neuroimmunol. (1995) 57(1–2):143–53. doi: 10.1016/0165-5728(94)00178-Q
128. Janssen M, Oyen WJ, Massuger LF, Frielink C, Dijkgraaf I, Edwards DS, et al. Comparison of a monomeric and dimeric radiolabeled RGD-peptide for tumor targeting. Cancer Biother Radiopharm. (2002) 17(6):641–6. doi: 10.1089/108497802320970244
129. Schittenhelm J, Schwab EI, Sperveslage J, Tatagiba M, Meyermann R, Fend F, et al. Longitudinal expression analysis of αv integrins in human gliomas reveals upregulation of integrin αvβ3 as a negative prognostic factor. J Neuropathol Exp Neurol. (2013) 72(3):194–210. doi: 10.1097/NEN.0b013e3182851019
130. Yoshimoto M, Ogawa K, Washiyama K, Shikano N, Mori H, Amano R, et al. Alpha(v)beta(3) integrin-targeting radionuclide therapy and imaging with monomeric RGD peptide. Int J Cancer. (2008) 123(3):709–15. doi: 10.1002/ijc.23575
131. Ellert-Miklaszewska A, Poleszak K, Pasierbinska M, Kaminska B. Integrin signaling in glioma pathogenesis: from biology to therapy. Int J Mol Sci. (2020) 21(3):888. doi: 10.3390/ijms21030888
132. Bello L, Francolini M, Marthyn P, Zhang J, Carroll RS, Nikas DC, et al. Alpha(v)beta3 and alpha(v)beta5 integrin expression in glioma periphery. Neurosurgery. (2001) 49(2):380–9; discussion 90. doi: 10.1097/00006123-200108000-00022
133. Marshall GR. Peptide interactions with G-protein coupled receptors. Biopolymers. (2001) 60(3):246–77. doi: 10.1002/1097-0282(2001)60:3%3C246::AID-BIP10044%3E3.0.CO;2-V
134. Liu Z, Shi J, Jia B, Yu Z, Liu Y, Zhao H, et al. Two ⁹⁰Y-labeled multimeric RGD peptides RGD4 and 3PRGD2 for integrin targeted radionuclide therapy. Mol Pharm. (2011) 8(2):591–9. doi: 10.1021/mp100403y
135. Conte M, Casas-Tintò S, Soler J. Modeling invasion patterns in the glioblastoma battlefield. PLoS Comput Biol. (2021) 17(1):e1008632. doi: 10.1371/journal.pcbi.1008632
136. Katsamakas S, Chatzisideri T, Thysiadis S, Sarli V. RGD-mediated delivery of small-molecule drugs. Future Med Chem. (2017) 9(6):579–604. doi: 10.4155/fmc-2017-0008
137. Battistini L, Bugatti K, Sartori A, Curti C, Zanardi F. RGD Peptide-drug conjugates as effective dual targeting platforms: recent advances. Eur J Org Chem. (2021) 2021(17):2506–28. doi: 10.1002/ejoc.202100240
138. Chen X, Park R, Tohme M, Shahinian AH, Bading JR, Conti PS. MicroPET and autoradiographic imaging of breast cancer αv-integrin expression using 18F-and 64Cu-labeled RGD peptide. Bioconjugate Chem. (2004) 15(1):41–9. doi: 10.1021/bc0300403
139. Haubner R, Wester H-J, Weber WA, Mang C, Ziegler SI, Goodman SL, et al. Noninvasive imaging of αvβ3 integrin expression using 18F-labeled RGD-containing glycopeptide and positron emission tomography. Cancer Res. (2001) 61(5):1781–5. PMID: 11280722.11280722
140. Schnell O, Krebs B, Carlsen J, Miederer I, Goetz C, Goldbrunner RH, et al. Imaging of integrin alpha(v)beta(3) expression in patients with malignant glioma by [18F] galacto-RGD positron emission tomography. Neuro Oncol. (2009) 11(6):861–70. doi: 10.1215/15228517-2009-024
141. Zhang J, Li D, Niu G, Baum R, Zhu Z, Chen X. First-in-Human study of a64Cu-labeled long-acting integrin αvβ3 targeting Molecule64Cu-NOTA-EB-RGD in healthy volunteers and GBM patients. Soc Nuclear Med. (2020):349.
142. Novy Z, Stepankova J, Hola M, Flasarova D, Popper M, Petrik M. Preclinical evaluation of radiolabeled peptides for PET imaging of glioblastoma Multiforme. Molecules. (2019) 24(13):2496. doi: 10.3390/molecules24132496
143. Li D, Zhao X, Zhang L, Li F, Ji N, Gao Z, et al. 68Ga-PRGD2 PET/CT in the evaluation of glioma: a prospective study. Mol Pharm. (2014) 11(11):3923–9. doi: 10.1021/mp5003224
144. Lo W-L, Lo S-W, Chen S-J, Chen M-W, Huang Y-R, Chen L-C, et al. Molecular imaging and preclinical studies of radiolabeled long-term RGD peptides in U-87 MG tumor-bearing mice. Int J Mol Sci. (2021) 22(11):5459. doi: 10.3390/ijms22115459
145. Burger JA, Kipps TJ. CXCR4: a key receptor in the crosstalk between tumor cells and their microenvironment. Blood. (2006) 107(5):1761–7. doi: 10.1182/blood-2005-08-3182
146. Bian XW, Yang SX, Chen JH, Ping YF, Zhou XD, Wang QL, et al. Preferential expression of chemokine receptor CXCR4 by highly malignant human gliomas and its association with poor patient survival. Neurosurgery. (2007) 61(3):570–8. discussion 8–9. doi: 10.1227/01.NEU.0000290905.53685.A2
147. Lapa C, Lückerath K, Kleinlein I, Monoranu CM, Linsenmann T, Kessler AF, et al. (68)Ga-pentixafor-PET/CT for imaging of chemokine receptor 4 expression in glioblastoma. Theranostics. (2016) 6(3):428–34. doi: 10.7150/thno.13986
148. Strosberg J, El-Haddad G, Wolin E, Hendifar A, Yao J, Chasen B, et al. Phase 3 trial of (177)Lu-dotatate for midgut neuroendocrine tumors. N Engl J Med. (2017) 376(2):125–35. doi: 10.1056/NEJMoa1607427
149. Bailly C, Vidal A, Bonnemaire C, Kraeber-Bodéré F, Chérel M, Pallardy A, et al. Potential for nuclear medicine therapy for glioblastoma treatment. Front Pharmacol. (2019) 10:772. doi: 10.3389/fphar.2019.00772
150. Virgolini I, Britton K, Buscombe J, Moncayo R, Paganelli G, Riva P. In- and Y-DOTA-lanreotide: results and implications of the MAURITIUS trial. Semin Nucl Med. (2002) 32(2):148–55. doi: 10.1053/snuc.2002.31565
151. Heute D, Kostron H, von Guggenberg E, Ingorokva S, Gabriel M, Dobrozemsky G, et al. Response of recurrent high-grade glioma to treatment with (90)Y-DOTATOC. J Nucl Med. (2010) 51(3):397–400. doi: 10.2967/jnumed.109.072819
152. Chang SS, O'Keefe DS, Bacich DJ, Reuter VE, Heston WD, Gaudin PB. Prostate-specific membrane antigen is produced in tumor-associated neovasculature. Clin Cancer Res. (1999) 5(10):2674–81. PMID: 10537328.10537328
153. Troyer JK, Beckett ML, Wright GL Jr. Detection and characterization of the prostate-specific membrane antigen (PSMA) in tissue extracts and body fluids. Int J Cancer. (1995) 62(5):552–8. doi: 10.1002/ijc.2910620511
154. Chang SS, Reuter VE, Heston WD, Bander NH, Grauer LS, Gaudin PB. Five different anti-prostate-specific membrane antigen (PSMA) antibodies confirm PSMA expression in tumor-associated neovasculature. Cancer Res. (1999) 59(13):3192–8. PMID: 10397265.10397265
155. Mhawech-Fauceglia P, Zhang S, Terracciano L, Sauter G, Chadhuri A, Herrmann FR, et al. Prostate-specific membrane antigen (PSMA) protein expression in normal and neoplastic tissues and its sensitivity and specificity in prostate adenocarcinoma: an immunohistochemical study using mutiple tumour tissue microarray technique. Histopathology. (2007) 50(4):472–83. doi: 10.1111/j.1365-2559.2007.02635.x
156. Nomura N, Pastorino S, Jiang P, Lambert G, Crawford JR, Gymnopoulos M, et al. Prostate specific membrane antigen (PSMA) expression in primary gliomas and breast cancer brain metastases. Cancer Cell Int. (2014) 14(1):26. doi: 10.1186/1475-2867-14-26
157. Saffar H, Noohi M, Tavangar SM, Saffar H, Azimi S. Expression of prostate-specific membrane antigen (PSMA) in brain glioma and its correlation with tumor grade. Iran J Pathol. (2018) 13(1):45–53. doi: 10.30699/ijp.2019.89854.1849
158. Wernicke AG, Edgar MA, Lavi E, Liu H, Salerno P, Bander NH, et al. Prostate-specific membrane antigen as a potential novel vascular target for treatment of glioblastoma multiforme. Arch Pathol Lab Med. (2011) 135(11):1486–9. doi: 10.5858/arpa.2010-0740-OA
159. Mahzouni P, Shavakhi M. Prostate-Specific membrane antigen expression in neovasculature of glioblastoma Multiforme. Adv Biomed Res. (2019) 8:18. doi: 10.4103/abr.abr_209_18
160. Kunikowska J, Kuliński R, Muylle K, Koziara H, Królicki L. 68Ga-Prostate-Specific Membrane antigen-11 PET/CT: a new imaging option for recurrent glioblastoma Multiforme? Clin Nucl Med. (2020) 45(1):11–8. doi: 10.1097/RLU.0000000000002806
161. Holzgreve A, Biczok A, Ruf VC, Liesche-Starnecker F, Steiger K, Kirchner MA, et al. PSMA Expression in glioblastoma as a basis for theranostic approaches: a retrospective, correlational panel study including immunohistochemistry, clinical parameters and PET imaging. Front Oncol. (2021) 11:646387. doi: 10.3389/fonc.2021.646387
162. Kirchner MA, Holzgreve A, Brendel M, Orth M, Ruf VC, Steiger K, et al. PSMA PET imaging in glioblastoma: a preclinical evaluation and theranostic outlook. Front Oncol. (2021) 11:774017. doi: 10.3389/fonc.2021.774017
163. Lapa C, Reiter T, Kircher M, Schirbel A, Werner RA, Pelzer T, et al. Somatostatin receptor based PET/CT in patients with the suspicion of cardiac sarcoidosis: an initial comparison to cardiac MRI. Oncotarget. (2016) 7(47):77807. doi: 10.18632/oncotarget.12799
164. Siebermair J, Köhler M, Kupusovic J, Nekolla S, Kessler L, Ferdinandus J, et al. Cardiac fibroblast activation detected by ga-68 FAPI PET imaging as a potential novel biomarker of cardiac injury/remodeling. J Nucl Cardiol. (2021) 28(3):812–21. doi: 10.1007/s12350-020-02307-w
165. Pan Q, Luo Y, Zhang W. Recurrent immunoglobulin G4-related disease shown on 18F-FDG and 68Ga-FAPI PET/CT. Clin Nucl Med. (2020) 45(4):312–3. doi: 10.1097/RLU.0000000000002919
166. Kratochwil C, Flechsig P, Lindner T, Abderrahim L, Altmann A, Mier W, et al. 68Ga-FAPI PET/CT: tracer uptake in 28 different kinds of cancer. J Nucl Med. (2019) 60(6):801–5. doi: 10.2967/jnumed.119.227967
167. Röhrich M, Floca R, Loi L, Adeberg S, Windisch P, Giesel FL, et al. FAP-specific PET signaling shows a moderately positive correlation with relative CBV and no correlation with ADC in 13 IDH wildtype glioblastomas. Eur J Radiol. (2020) 127:109021. doi: 10.1016/j.ejrad.2020.109021
168. Giesel FL, Heussel CP, Lindner T, Röhrich M, Rathke H, Kauczor H-U, et al. FAPI-PET/CT improves staging in a lung cancer patient with cerebral metastasis. Eur J Nucl Med Mol Imaging. (2019) 46(8):1754–5. doi: 10.1007/s00259-019-04346-z
169. Windisch P, Zwahlen DR, Koerber SA, Giesel FL, Debus J, Haberkorn U, et al. Clinical results of fibroblast activation protein (FAP) specific PET and implications for radiotherapy planning: systematic review. Cancers. (2020) 12(9):2629. doi: 10.3390/cancers12092629
170. Chen H, Pang Y, Wu J, Zhao L, Hao B, Wu J, et al. Comparison of [68 Ga]Ga-DOTA-FAPI-04 and [18F] FDG PET/CT for the diagnosis of primary and metastatic lesions in patients with various types of cancer. Eur J Nucl Med Mol Imaging. (2020) 47(8):1820–32. doi: 10.1007/s00259-020-04769-z
171. Ballal S, Yadav MP, Kramer V, Moon ES, Roesch F, Tripathi M, et al. A theranostic approach of [68 Ga]Ga-DOTA.SA.FAPi PET/CT-guided [177Lu]Lu-DOTA.SA.FAPi radionuclide therapy in an end-stage breast cancer patient: new frontier in targeted radionuclide therapy. Eur J Nucl Med Mol Imaging. (2021) 48(3):942–4. doi: 10.1007/s00259-020-04990-w
172. Moon ES, Elvas F, Vliegen G, De Lombaerde S, Vangestel C, De Bruycker S, et al. Targeting fibroblast activation protein (FAP): next generation PET radiotracers using squaramide coupled bifunctional DOTA and DATA(5 m) chelators. EJNMMI Radiopharm Chem. (2020) 5(1):19. doi: 10.1186/s41181-020-00102-z
173. Wang H, Wu Q, Liu Z, Luo X, Fan Y, Liu Y, et al. Downregulation of FAP suppresses cell proliferation and metastasis through PTEN/PI3K/AKT and ras-ERK signaling in oral squamous cell carcinoma. Cell Death Dis. (2014) 5(4):e1155. doi: 10.1038/cddis.2014.122
174. Ballal S, Yadav MP, Moon ES, Kramer VS, Roesch F, Kumari S, et al. Biodistribution, pharmacokinetics, dosimetry of [(68)Ga]Ga-DOTA.SA.FAPi, and the head-to-head comparison with [(18)F]F-FDG PET/CT in patients with various cancers. Eur J Nucl Med Mol Imaging. (2021) 48(6):1915–31. doi: 10.1007/s00259-020-05132-y
Keywords: theranostics, neuro-Oncology, glioblastoma multiform (GBM), prostate-specific membrane antigen (PSMA), fibroblast activated protein (FAP), somatostatin receptors (SRS), chemokine receptor-4 (CXCR4)
Citation: Dadgar H, Jokar N, Nemati R, Larvie M and Assadi M (2023) PET tracers in glioblastoma: Toward neurotheranostics as an individualized medicine approach. Front. Nucl. Med. 3:1103262. doi: 10.3389/fnume.2023.1103262
Received: 20 November 2022; Accepted: 23 January 2023;
Published: 27 February 2023.
Edited by:
Gaetano Paone, Imaging Institute of Southern Switzerland, SwitzerlandReviewed by:
Yoichi Shimizu, Kyoto University, JapanLaurence Carroll, Johns Hopkins University, United States
© 2023 Dadgar, Jokar, Nemati, Larvie and Assadi. This is an open-access article distributed under the terms of the Creative Commons Attribution License (CC BY). The use, distribution or reproduction in other forums is permitted, provided the original author(s) and the copyright owner(s) are credited and that the original publication in this journal is cited, in accordance with accepted academic practice. No use, distribution or reproduction is permitted which does not comply with these terms.
*Correspondence: Majid Assadi assadipoya@yahoo.com asadi@bpums.ac.ir
†These authors have contributed equally to this work
Specialty Section: This article was submitted to PET and SPECT, a section of the journal Frontiers in Nuclear Medicine