Metabolism of Exogenous D-Beta-Hydroxybutyrate, an Energy Substrate Avidly Consumed by the Heart and Kidney
- 1Nestlé Health Science, Translation Research, Epalinges, Switzerland
- 2Nestlé Research, Clinical Development Unit, Lausanne, Switzerland
- 3Nestlé Research, Institute of Food Safety and Analytical Sciences, Lausanne, Switzerland
- 4Sherbrooke Molecular Imaging Center, Sherbrooke, QC, Canada
- 5Department of Medicine, Université de Sherbrooke, Sherbrooke, QC, Canada
- 6Research Center on Aging, Sherbrooke, QC, Canada
- 7CHUS Research Center, Sherbrooke, QC, Canada
- 8Department of Pharmacology and Physiology, Université de Sherbrooke, Sherbrooke, QC, Canada
There is growing interest in the metabolism of ketones owing to their reported benefits in neurological and more recently in cardiovascular and renal diseases. As an alternative to a very high fat ketogenic diet, ketones precursors for oral intake are being developed to achieve ketosis without the need for dietary carbohydrate restriction. Here we report that an oral D-beta-hydroxybutyrate (D-BHB) supplement is rapidly absorbed and metabolized in humans and increases blood ketones to millimolar levels. At the same dose, D-BHB is significantly more ketogenic and provides fewer calories than a racemic mixture of BHB or medium chain triglyceride. In a whole body ketone positron emission tomography pilot study, we observed that after D-BHB consumption, the ketone tracer 11C-acetoacetate is rapidly metabolized, mostly by the heart and the kidneys. Beyond brain energy rescue, this opens additional opportunities for therapeutic exploration of D-BHB supplements as a “super fuel” in cardiac and chronic kidney diseases.
Introduction
The ketogenic diet is a very low carbohydrate diet that has shown therapeutic benefits in drug resistant epilepsy (1, 2) and is gaining increased attention in other neurological diseases (3) and in healthy aging (4). Ketones (acetoacetate [AcAc] and D-beta-hydroxybutyrate [D-BHB]) are produced by the liver when blood glucose and insulin decrease (5). Blood ketones above 0.5 mM indicates a ketosis state that reaches 3–5 mM on the ketogenic diet. One of the main benefits of ketones is their ability to act as an alternative energy source to glucose or fatty acids for production of ATP by mitochondria. Caloric restriction and intermittent fasting also produce transient mild-moderate ketosis (6, 7).
As an alternative to the ketogenic diet, exogenous ketone precursors taken orally achieve mild ketosis in the absence of dietary restriction. They can be grouped in three categories (Figure 1): First, medium chain triglycerides (MCT) composed of a mixture of 8 and 10 carbons fatty acids are efficiently digested to free fatty acids (FFA), directly absorbed, and rapidly metabolized by the liver. The acute production of excess acetyl-CoA drives the production of AcAc and then D-BHB which are both secreted into the systemic circulation (8). While a high dose of MCT can provide a moderate increase in blood ketones (+0.5–1.0 mM), gastrointestinal intolerance and high caloric load limit their use. Second, ketone esters (KE) made of a BHB ester linked to butanediol provide one molecule of D-BHB after digestion, with the butanediol being further metabolized by the liver to D-BHB (9). KE increase blood ketones above 1 mM but are also limited at high dose by their gastric tolerability and severe bitterness (10). Third, perhaps the most physiologic way to raise blood ketones is via the oral intake of D-BHB itself. Exogenous D-BHB is directly absorbed into the circulation, with some of it being converted to AcAc by the liver, and both ketones being distributed throughout the body. Until recently, only racemic mixtures of dextro (D) and levo (L) BHB (D+L-BHB) were available and oral human studies with them have been reported (9, 11–14). As L-BHB is not metabolized significantly into energy intermediates and is slowly excreted in the urine (9, 15), D+L-BHB would be anticipated to be less ketogenic than pure D-BHB.
Once ketone precursors are absorbed and metabolized, the resulting ketones are taken up by extrahepatic tissues such as brain, heart, muscle, and kidney and metabolized to acetyl-CoA for ATP production in mitochondria (5). Understanding how ketones are utilized by different organs after the intake of a ketone precursor is therefore starting to gain in importance. For instance, brain energy is derived mostly from glucose, but ketones spare brain glucose consumption when they are available (16). When blood ketone levels are increased (either by ketogenic diet or exogenous ketones), the brain utilizes ketones preferentially. Brain ketone metabolism is directly proportional to plasma ketone level over a wide concentration range. An increase in brain ketone metabolism can increase overall brain energy supply in mild cognitive impairment and Alzheimer's disease (17–19). The heart is an energy omnivore and uses both FFA and glucose as major energy substrates (20). Increased blood ketones produced by acute intravenous infusion of D+L-BHB reduces myocardial glucose utilization without affecting myocardial FFA metabolism (21). The kidney uses FFA as its main energy source (22) and, although less well-studied, ketones have been shown to be preferred over FFA, lactate and other endogenous energy substrates for the kidney (23). Despite utilizing ~20% of total body energy intake, the liver cannot use ketones as a source of energy as it lacks the enzyme succinyl-CoA:3-oxoacid-CoA transferase (SCOT) required to convert AcAc back to acetyl-CoA (3). However, the liver contributes to the interconversion of AcAc to D-BHB via mitochondrial D-BHB dehydrogenase (BDH1).
Positron-emission tomography (PET) using the ketone tracer, 11C-AcAc, was developed initially to directly observe ketone metabolism in the brain of people developing MCI and AD (24, 25). It has been used to study heart energy metabolism in rodents (26) and has the potential to provide insight into whole body ketone metabolism in humans. Here, the objective was to compare the metabolism of a pure D-BHB oral supplement, i.e., the increase in blood D-BHB and AcAc after D-BHB, to that produced by the ingestion of the same amount of racemic D+L-BHB or MCT. A pilot study was also performed to assess the feasibility of using 11C-AcAc PET to observe organ ketone uptake after oral ingestion of D-BHB.
Materials and Methods
Test Products
D-BHB
14.1 g of pure salts of the D enantiomer (>99% enantiomeric excess) of D-BHB were used. The D-BHB supplement tested was formulated as a mixture of three salts: sodium D-beta-hydroxybutyrate (CAS Registry number 13613-65-5), magnesium (D-beta-hydroxybutyrate)2 (CAS Registry number 586976-57-0), and calcium (D-beta-hydroxybutyrate)2 (CAS Registry number 51899-07-1). Each oral serving provided 12 g D-beta-hydroxybutyric acid, 0.78 g sodium, 0.42 g magnesium, and 0.88 g calcium, citrus flavoring and sweetener (Stevia), dissolved in 150 mL of drinking water.
Chemical purity of beta-hydroxybutyric acid was determined by quantitative 1H-nuclear magnetic resonance (NMR). NMR spectra were recorded on a 600 MHz Bruker Avance III spectrometer equipped with a 5 mm TCI cryogenic probe at 300 K using a Topspin 3.5pl7 software (Bruker Biospin).
Enantiomeric purity was determined by chiral high-performance liquid chromatography (HPLC) using an HPLC-UV instrument from Agilent Technologies with a Sumichiral OA6100 (5 μm, 4.6 × 150 mm) column. The mobile phase consisted of 1 mM copper (II) sulfate in water at a flow rate of 1 mL/min. Detection of the peaks was carried out by ultraviolet detection at 254 and 210 nm. Calculation of enantiomeric excess (ee) was expressed in percentage (%) according to the following formula: ee% = [(area of D-BHB– area of L-BHB)/total area of both D and L-BHB combined] × 100.
D+L-BHB
14.5 g of an equimolar mixture of commercial D and L beta-hydroxybutyrate salt was used (KetoCaNa, KetoSports, USA). Each serving provided a mixture of 12 g D+L-Beta-hydroxybutyric acid, 1.3 g sodium, 1.2 g calcium, orange flavoring and stevia, dissolved in 150 mL of drinking water.
MCT
Fifteen grams of medium chain triglyceride (MCT) (60% caprylic C8 acid and 40% capric C10 acid) emulsified in 70 mL of a 5% aqueous milk protein solution.
Standard Breakfast
The meal consisted of 2 boiled eggs, 2 pieces of toast, 1 slice of cheese, and 1 portion of fruit jam, providing a total of 423 kcal (20 g fat, 24 g protein, 32 g carbohydrate). Water was provided ab-libitum. The amount of the breakfast was not adjusted to the weight of the participants.
Pharmacokinetic Study
Study Design and Outcomes
The ketogenic potential of D-BHB, D+L-BHB, and MCT was tested in 3 groups of 15 participants. The 3 groups had 11 participants in common and each participant had at least a 5-day washout period between each test product intake.
The groups had a mean age range of 36–38 years, body weight of 72–74 kg, BMI of 23–24 kg/m2, fasting plasma ketones of 98–185 μM, fasting plasma glucose of 5.3–5.5 mM, and fasting plasma insulin of 6.1–7.0 mU/L. Detailed demographics for each group are reported in Supplementary Material.
After an overnight fast, participants orally consumed 150 mL of the test product at time 0. At time 30 min, a standard breakfast was provided and consumed over 15 min to mimic the real life situation and explore any interference with the test product. Blood samples (7.5 mL) were taken at regular interval over 4 h [time (min): 0, 15, 30, 45, 60, 120, 180, 240] via a venous catheter. Plasma was analyzed for total ketones and D-BHB using Autokit Total Ketone Bodies and Autokit 3-HB (Wako Diagnostics, Mountain View, CA, USA), respectively. AcAc was then calculated by subtracting total BHB from total ketones. Plasma total BHB (D+L-BHB) was analyzed by ultra-high performance liquid chromatography, tandem mass spectrometry (UHPLC-MSMS; Vantage TSQ, ThermoFischer, Germany), based on the protocol described by Zeng and Cao (27). L-BHB was then calculated by subtracting D-BHB (measured by the enzymatic assay Autokit 3-HB above) from D+L-BHB measured by UHPLC-MSMS. Plasma glucose and insulin were measured using GLUC Flex® reagent cartridges (Siemens Healthcare Diagnostics Inc.) and an ARCHITECT Insulin 8K41 kit (Abbott Laboratories Diagnostics Division, Abbott Park, IL 60064 USA), respectively.
Concentration for maximum effect (Cmax) was calculated as the mean of the maximum concentration reached by each participant. Incremental area-under-the-curve (iAUC) was calculated as the mean of baseline-corrected iAUC for each individual over 4 h. Time for maximum effect (Tmax) was calculated as the mean of Tmax reached by each individual.
During the 4 h test period, gastro-intestinal tolerability was assessed with a visual analog scale (VAS; 0 to 100) for each of the following symptoms: (1) abdominal discomfort, (2) appetite, (3) gastric reflux, (4) nausea, (5) diarrhea, and (6) headache.
This study was approved by the Ethics Committee of Canton de Vaud (Switzerland) under the generic protocol reference 2018-00503, and all participants provided written informed consent. Procedures were conducted according to the principles of the Declaration of Helsinki. This trial is registered at ClinicalTrials.gov with the identification number NCT03603782.
Statistical Analysis
The sample size was based on previous determination of the coefficient of variation of plasma BHB iAUC (SD/mean = 0.0783). With this assumption, in order to detect a 10% difference between the iAUC of two products with a power of 80% and a type 1 error rate of 5%, about 12 participants per group were needed in a complete cross over design frame. Assuming 20% of non-evaluable participants, this resulted in enrollment of 15 participants/group.
To assess a potential carry-over effect, the joint modeling of iAUC and half-life (T1/2) was estimated by including product taken, previous product taken, and interaction of product taken and previous product taken as covariates. The covariate-associated coefficients were not different from zero, supporting the assumption that product-related effects were not carried forward over visits.
Exploratory inferential results were obtained with the non-parametric Wilcoxon rank-sum exact test (28). The alpha level was set at 0.05. Significance threshold was not adjusted for multiplicity and samples were considered independent even though some were correlated within participants due to the design of the study (partial cross-over).
11C-AcAc PET Pilot Study
The full method for the 11C-AcAc-PET tracer experiment has been reported previously (16, 17). On the day of the PET scan, prior to injecting the tracer 11C-AcAc at 0 min, a 66 year old healthy male (74 kg) fasted for ~6 h then consumed two servings of the D-BHB supplement at −75 min and again at −30 min.
PET images were acquired on a PET/CT (Gemini TF, Philips Healthcare, Eindhoven, the Netherlands). On the contralateral side from the radiotracer injection, blood was arterialized by warming the forearm with a heating pad at 44°C. Blood samples were taken at 3, 6, 8, 12, 20, and 28 min post-injection.
The acquisition protocol was as follows: 370 MBq of 11C-AcAc was injected followed by a 10-min dynamic brain acquisition, in list mode, with an isotropic voxel size of 2 mm3. Immediately after the dynamic brain acquisition, three whole-body (head to mid-thigh) acquisitions were performed at 18, 25, and 35 min post-injection. The acquisition times per bed position were 30, 45, and 60 s, respectively, for the three scans. Whole body acquisitions were performed with an isotropic voxel size of 4 mm3. Finally, an 8 frame per-cycle cardiac-gated acquisition of 15 min was performed 50 min after tracer injection.
PET tracer kinetics were analyzed for the brain (PMOD Technologies Ltd., Zurich, Switzerland). Brain ketone metabolism was assessed with graphical Patlak analysis of 11C-AcAc as previously described (16, 17). Briefly, Patlak linearization was used to quantify the brain uptake rate constants of acetoacetate (KAcAc) and the cerebral metabolic rate of AcAc and ketones (CMRAcAc and CMRketones). CMR was calculated using the following equation: CMR = K × Cp/LC, where K (min−1) is the uptake rate constant, Cp is the arterial plasma concentration, and LC is the lumped constant (1.0 for AcAc) accounting for uptake differences between the tracer and natural molecule. CMRketones calculation uses the plasma concentrations of AcAc and D-BHB, KAcAc, and rk (KAcAc/ Kketones) set at 1.2 as previously reported (21, 22). CMRketones = (KAcAcx[AcAc]) × (1+(1/rkx[AcAc]/[D-BHB])).
To characterize the effect of D-BHB supplementation on ketone uptake in organs besides the brain, 11C-AcAc uptake by the liver, kidneys and heart were segmented on the whole-body PET/CT fusion image, and the % dose/g was calculated from the organ volume and injected dose.
Heart reorientation and cardiac function analysis were performed using the cardiac module of PMOD 3.9 to obtain the ventricular volumes, ejection fraction, and polar map (29).
Plasma collected during the PET scan was analyzed for D-BHB and AcAc by automated colorimetric assay on a clinical chemistry analyser (Dimension Xpand Plus; Siemens, Deerfield, IL, USA) as previously described (8).
This PET study was approved by the CIUSSS de l'Estrie–CHUS Research Ethics Committee.
Results
Pharmacokinetics Study
Following intake of the D-BHB, blood ketones rapidly increased (Figure 2A, Supplementary Table 2) to a Cmax of 1.2 ± 0.1 mM. When the same amount of D+L-BHB or MCT was consumed, a ~50% lower Cmax from baseline was observed compared to D-BHB (Cmax D+L-BHB 0.62 ± 0.05 mM and MCT 0.62 ± 0.06 mM; p < 0.001 vs. D-BHB for both). Tmax was reached in about 1 h for each product (Supplementary Table 3), and ketone levels came back to baseline values after 3–4 h. The iAUC for D-BHB was ~1.5 fold higher than for D+L-BHB or MCT (Figure 3A, Supplementary Table 1; p < 0.005 for both).
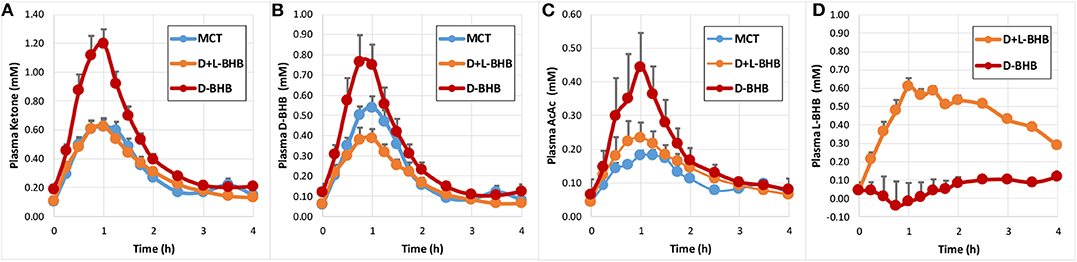
Figure 2. Blood ketone kinetics following gram-matched oral doses of D-BHB, D+L-BHB, and MCT in 15 fasted participants at rest. (A) Plasma ketone, (B) D-BHB, (C) AcAc, and (D) L-BHB levels over 4 h. Values are means + SEM.
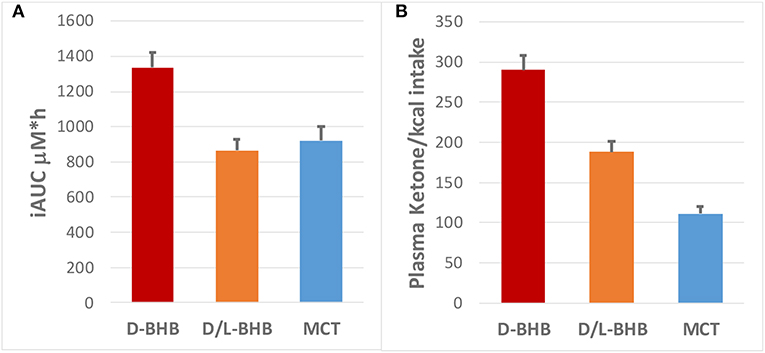
Figure 3. (A) Plasma ketone iAUC (μM*h) for D-BHB, D+L BHB and MCT, and (B) their respective ketone production per calorie consumed (iAUC/kcal).
Analysis of blood D-BHB and AcAc revealed a similar pattern for the three products (Figures 2B,C). However, MCT produced proportionally less blood AcAc and more blood D-BHB than oral D-BHB or D+L-BHB. The calculated ratio [mean AcAc iAUC/mean D-BHB iAUC] was 0.44 for MCT, a value ~30–40 % lower than for D-BHB (0.63) and D+L-BHB (0.76) (Supplementary Table 1; D-BHB vs. MCT p = 0.0102; D+L-BHB vs. MCT p < 0.0001; D+L-BHB vs. D-BHB p = 0.173). The baseline ratio AcAc/D-BHB was ~0.7, suggesting that MCT lowered the AcAc/D-BHB blood ratio while producing ketones. Similar ratios were obtained when using values derived from Cmax [mean AcAc Cmax/mean D-BHB Cmax] (Supplementary Table 2).
As expected, oral intake of D+L-BHB resulted in a significant increase in plasma L-BHB over the first hour (Figure 2D), follow by a slow decrease over the next 3 h, without coming back to baseline. In comparison, oral intake of D-BHB did not produce an increase in plasma L-BHB.
Ketone production per calorie ingested [MCT: 8.3 kcal/g; BHB: 4.6 kcal/g (42)] was significantly higher for D-BHB than for D+L-BHB and MCT (Figure 3B, p < 0.0001 for all three comparisons D-BHB vs. D+L BHB vs. MCT). Increased glucose and insulin levels as a result of the intake of the meal 30 min after each test product was consumed were not significantly different across the three groups (Supplementary Table 4 and Supplementary Figure 2). Tolerability of the three products, evaluated by a GI visual assessment score, was similar with no clinically significant differences (Supplementary Figure 1). Amongst some of the GI effect self-reported, decreased appetite and some diarrhea were the most common for the three products.
11C-AcAc PET Pilot Study
Plasma ketone levels were between 0.9 and 1.2 mM during the first 30 min of the PET scan acquisition. The first whole-body PET/CT biodistribution of 11C-AcAc 18 min post injection of the radiotracer is illustrated in Figure 4. The radiotracer was rapidly taken up mainly by the heart, where both ventricles and the atriums could be observed. 11C-AcAc uptake estimates per gram of tissue over three time points are summarized in Figure 5. Excretion of the radiotracer was observed in the urinary bladder and the salivary glands.
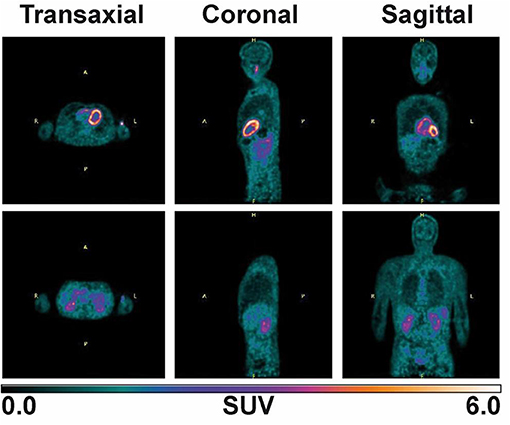
Figure 4. Whole body image after supplementation with two 15 g D-BHB doses, one at −75 min and the other at −30 min prior 11C-AcAc infusion (330 MBq). An 8 min scan (30 s per bed) was acquired 18 min post-injection of the radiotracer.
From the dynamic brain scan, CMRAcAc and KAcAc could be determined for all main regions of the brain (Supplementary Table 5) and compared to baseline values previously determined in healthy young adults (16). Overall and compared to baseline, each region demonstrated an increase in CMRAcAc and KAcAc of ~4.7 and 2.3-fold, respectively, about 1 h after taking D-BHB. This indicated that AcAc is effectively taken by the brain and by other organs particularly the heart and the kidney.
Despite the fact that a dynamic cardiac scan could not performed in the present study, assessment of cardiac function was still possible with the gated heart PET image (Figure 6). The values of the end-diastolic and end-systolic volumes as well as the ejection fraction of the left ventricle calculated from the 11C-AcAc metabolism were 100, 47 ml, and 52%, respectively.
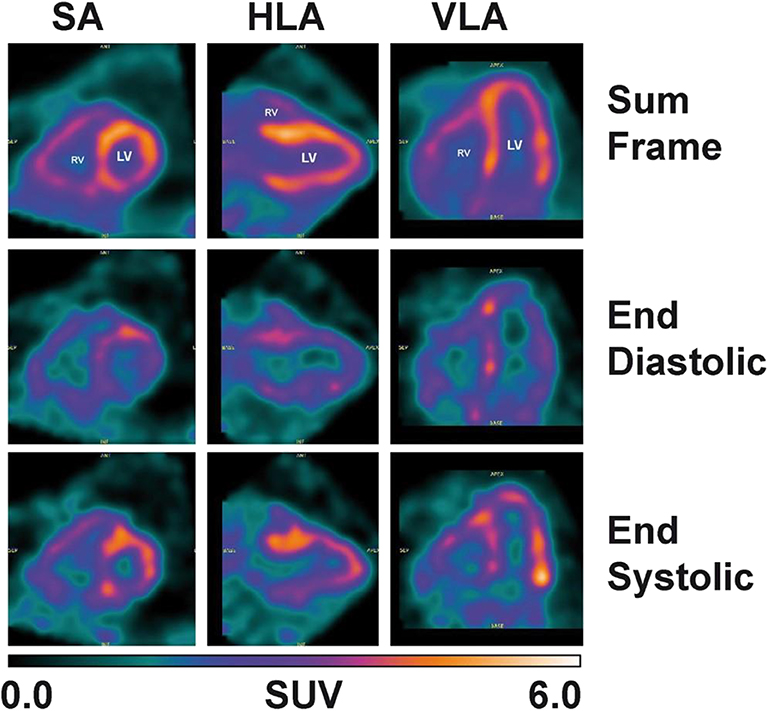
Figure 6. Heart image: 15 min cardiac-gated acquisition (8 frames) obtained 50 min post-11C-AcAc injection. Left ventricle (LV), right ventricle (RV), horizontal long axis (HLA), vertical long axis (VLA) and short axis (SA) images.
Discussion
To our knowledge, this is the first report of the metabolism of D-BHB in humans and its link to the organ distribution of the ketone, AcAc, by PET imaging. This study shows that D-BHB is rapidly absorbed and metabolized (Figure 2). It also increases blood ketones above 1 mM, a level ~1.7 fold greater than the same dose of D+L-BHB or MCT, and this despite the lower caloric load of D-BHB. Moreover, the results of the pilot whole body 11C-AcAc-PET experiment support the feasibility of this technique to measure organ-specific exogenous ketone utilization and they suggest that exogenous ketones are very actively utilized by the heart and kidney.
Ketone production from an exogenous dietary source has been traditionally achieved by MCT. This requires a bolus intake to saturate the liver with MCFA, producing excess acetyl-CoA which is then transformed to AcAc and BHB, which are released into systemic circulation. The Cmax achieved with MCT is usually between 300 and 600 μM, with higher values being difficult to reach due to GI side effects and liver saturation. Here we show that D-BHB, a natural and biologically active ketone isomer, raises blood ketone Cmax above 1 mM without noticeable side effects. In comparison, an equivalent dose of D+L-BHB or MCT only achieved half this ketone level, with similar Tmax at 1 h. Thus, compared to D+L-BHB, D-BHB significantly reduces the salt intake needed to achieve the same plasma ketone response.
Results from a previous study (9) comparing KE to D+L-BHB showed that at the same dose of D-BHB equivalent, the increase blood ketone iAUC had the same magnitude, suggesting that exogenous D-BHB and KE produce similar ketosis. We note that the Tmax of D+L-BHB was significantly longer (~90 min) compared to KE (~20 min) at the same dose, suggesting that D-BHB could have a longer duration of action than KE. We also found that D+L-BHB resulted in a significant increase of plasma L-BHB that eliminate slowly, consistent with a previous report (9) and indicating a difference in the metabolic utilization of D and L-BHB. While D-BHB is readily utilized as an energy source, the L-isoform is eliminated slowly in the urine and does not contribute significantly to the biological activity of ketone precursors as an energy source. Finally, D-BHB provided higher ketones per calorie consumed than MCT: the caloric density of D-BHB (4.6 kcal/g) is half that of MCT (8.2 kcal/g), making D-BHB a 2.6 fold more energetically efficient ketone precursor vs. MCT (based on iAUC over 4 h).
The present study also revealed that D-BHB conversion to AcAc provided a ~43% higher AcAc/D-BHB blood ratio (0.63) than the same dose of MCT (0.44). In this respect, KE seems to behave more like MCT, with a ratio around 0.2–0.4 (3), while on the ketogenic diet, this ratio remains unchanged around 0.5 (16). Since D-BHB needs to be converted to AcAc by BDH-1 within each organ before being metabolized to acetyl-CoA (3), a higher AcAc/D-BHB ratio could be energetically more effective. Moreover, as this conversion requires the co-factor, nicotinamide adenine dinucleotide (NAD+), to be reduced to NADH, a higher AcAc/D-BHB might better preserve the mitochondrial NAD+/NADH ratio. Interestingly, a higher NAD+/NADH ratio has been associated to better health (30, 31), and can be increased in the brain by nutritional ketosis (32, 33), suggesting that D-BHB might be superior to MCT or KE in this respect, a point meriting further investigation.
PET has been invaluable in measuring energy metabolism at the organ level in humans. For example, with 18F-FDG, it clearly shows that glucose is the main energy substrate of the brain and that a fatty acid such as 11C-palmitate is preferentially utilized by the heart and the liver (34, 35). Our pilot 11C-AcAc-PET experiment aimed simply to demonstrate the feasibility of measuring ketone uptake by the main organs when exogenous D-BHB is administered orally to humans. The key advantage of 11C-AcAc as a metabolic radiotracer is that it equilibrates rapidly with D-BHB in blood and is indistinguishable from endogenous AcAc, so reflects the actual metabolism of ketones. The whole-body scan revealed for the first time that the heart and kidney are major contributors to the metabolism of exogenous AcAc after ingestion of a D-BHB supplement.
Most of the heart's energy requirement is normally provided by fatty acids and glucose (20). Our results confirm that the heart has the capacity to utilize other substrates such as ketones (21) and that ketone metabolism appears to be linked to the metabolism and clearance of the radiotracer by the myocardium. On a per gram basis, the kidney consumes a large amount of energy mostly derived from fatty acids, but our data show that ketones can clearly also be used effectively for mitochondrial respiration in the kidney as well.
The cerebral metabolic rate for ketones (CMRketones) obtained here with D-BHB (3.0 μmol/100 g/min) exceeded that obtained after MCT supplementation in mild cognitive impairment [2.49 μmol/100 g/min; (17)] and Alzheimer's disease [1.9–2.0 μmol/100 g/min; (18)]. While the CMR values obtained here for D-BHB are preliminary, our data suggest that a D-BHB supplement represents a promising intervention to provide ketones that can rescue the brain energy glucose deficit in these conditions (17, 25).
11C-AcAc uptake by the liver (Figures 4, 5) could reflect both the conversion of 11C-AcAc to 11C-D-BHB by BHD-1 and the high blood reservoir capacity of the liver, but do not reflect ketone metabolism by the liver itself. We show here that the blood radioactivity values of 11C-AcAc were similar to those of the liver (see Figure 5) supporting the view that the liver produces but does not metabolize ketones (5). This is consistent with the liver radioactivity after 11C-AcAc infusion being due to blood and not to the conversion of AcAc into AcAc-CoA and other energy intermediates (5).
Recent studies have shown the potential importance of ketones in cardio-metabolic health. Infusion of D+L-BHB has beneficial hemodynamic effects in adult patients with heart failure and lower ejection fraction (36). In children with fatty acid oxidation defects such as multiple acyl-CoA dehydrogenase deficiency, D+L-BHB improved heart function and cognitive performance, which resulted in better walking ability and correction of neurological symptoms (37). Moreover, clinical trials in diabetes with sodium/glucose co-transporter-2 (SGLT-2) inhibitors, which increase plasma ketones (38), show markedly reduced risk of cardiovascular events and kidney failure (39). Given the significant dysregulation of energy metabolism in type 2 diabetes, it is plausible that part of the effect of SGLT2 inhibitors on heart and kidney is mediated via improved ATP production using ketones as an alternative energy fuel to fatty acid and glucose (38, 40). This opens additional opportunities for therapeutic exploration of D-BHB supplements beyond energy rescue of the brain.
This study has several limitations. First, the pharmacokinetic comparison of D-BHB, D+L-BHB and MCT was an acute, single 4 h study; the extent to which it reflects long-term differences in their metabolism remains to be determined. Second, the whole-body PET scan was done on a single person and (except for brain) was a semi-quantitative comparison across organs. The brain was chosen for the quantitative scan (CMRAcAc). Dynamic PET scanning is required in order to quantify the tracer uptake and cannot be done simultaneously on the brain as well as other organs. Cardiac gated image quality could be improved if the image was acquired sooner after tracer injection. Third, ketone production and metabolism vary depending on post-prandial metabolic status, which possibly influences organ ketone distribution. Hence, follow-up of this single observation under different feeding conditions will be needed to verify the relative differences across organs.
Conclusions
D-BHB appears to be a promising supplement to produce significantly higher blood ketones than D+L-BHB or MCT, and at a lower calorie intake for an equivalent dose. Moreover, exogenous D-BHB does not appear to lower the blood AcAc/D-BHB ratio, which, altogether, might make it a more effective “super fuel” compared to other ketone precursors such as MCT, D+L-BHB or KE.
The pilot 11C-AcAc-PET study clearly identifies the heart and kidney as significant consumers of exogenous ketones, in fact, more than the brain. Therefore, D-BHB supplementation could be tested in conditions such as heart failure and diabetic cardiomyopathy to improve cardiac energy efficiency and function, and in chronic kidney disease.
Data Availability Statement
All datasets generated for this study are included in the article/Supplementary Material.
Ethics Statement
The studies involving human participants were reviewed and approved by the Ethics Committee of Canton de Vaud (Switzerland) under the generic protocol reference 2018-00503, Trial registration number NCT03603782 (pharmacokinetic study), and by the CIUSSS de l'Estrie, CHUS Research Ethics Committee, Sherbrooke (Canada; PET study). The patients/participants provided their written informed consent to participate in this study. Written informed consent was obtained from the individual(s) for the publication of any potentially identifiable images or data included in this article.
Author Contributions
MH, EC, C-AC, SC, and BC designed the studies. Data collection was performed by MH, EC, C-AC, J-PG, and MH. C-AC and EC analyzed data. The manuscript was drafted by BC and SC and all authors discussed the results and revised critically the manuscript.
Funding
Funding was provided by Nestlé.
Conflict of Interest
BC, MH, and J-PG are employees of Nestlé. SC has consulted for Nestlé, Bulletproof and Accera, and received research funding and/or research materials from the Alzheimer Association (USA), Mitacs, FRQS, Abitec and Nestlé. AC declares research funding from CIHR, Canadian Diabetes Association, Fonds de recherche du Quebec–Santé, Janssen, Merck, UniQure, Caprion, Eli Lilly, Novo Nordisk, GlaxoSmithKline, Novartis, Pfizer, Philips, Sanofi, Siemens, and Amgen and consulting/advisory panel participation or conference fees from Merck, Amgen, Janssen, UniQure, Servier, Novo Nordisk, and Novartis. EC, MM, and C-AC declare no competing financial interests.
Acknowledgments
We thank the Nestlé Clinical Development Unit and Metabolic Unit staff for clinical management of the pharmacokinetic study and for their help in recruiting the volunteers; and thank Isabelle Breton, Irina Monnard, Shéhérazade Corbaz, and Simona Bartova from Nestlé Research for their skillful analytical assistance for measuring the D and L-BHB.
Supplementary Material
The Supplementary Material for this article can be found online at: https://www.frontiersin.org/articles/10.3389/fnut.2020.00013/full#supplementary-material
References
1. D'Andrea Meira I, Romão TT, Pires do Prado HJ, Krüger LT, Pires MEP, da Conceição PO. Ketogenic diet and epilepsy: what we know so far. Front Neurosci. (2019) 13:5. doi: 10.3389/fnins.2019.00005
2. Kim SH, Shaw A, Blackford R, Lowman W, Laux LC, Millichap JJ, et al. The ketogenic diet in children 3 years of age or younger: a 10-year single-center experience. Sci Rep. (2019) 9:8736. doi: 10.1038/s41598-019-45147-6
3. McDonald TJW, Cervenka MC. Lessons learned from recent clinical trials of ketogenic diet therapies in adults. Curr Opin Clin Nutr Metab Care. (2019) 22:418–24. doi: 10.1097/MCO.0000000000000596
4. Campisi J, Kapahi P, Lithgow GJ, Melov S, Newman JC, Verdin E. From discoveries in ageing research to therapeutics for healthy ageing. Nature. (2019) 571:183–92. doi: 10.1038/s41586-019-1365-2
5. Puchalska P, Crawford PA. Multi-dimensional roles of ketone bodies in fuel metabolism, signaling, and therapeutics. Cell Metab. (2017) 25:262–84. doi: 10.1016/j.cmet.2016.12.022
6. Anton SD, Moehl K, Donahoo WT, Marosi K, Lee SA, Mainous AG III, et al. Flipping the metabolic switch: understanding and applying the health benefits of fasting. Obesity. (2018) 26:254–68. doi: 10.1002/oby.22065
7. Stekovic S, Hofer SJ, Tripolt N, Aon MA, Royer P, Pein L, et al. Alternate day fasting improves physiological and molecular markers of aging in healthy, non-obese humans. Cell Metab. (2019) 30:462–76.e5. doi: 10.1016/j.cmet.2019.07.016
8. Courchesne-Loyer A, Fortier M, Tremblay-Mercier J, Chouinard-Watkins R, Roy M, Nugent S, et al. Stimulation of mild, sustained ketonemia by medium-chain triacylglycerols in healthy humans: estimated potential contribution to brain energy metabolism. Nutrition. (2013) 29:635–40. doi: 10.1016/j.nut.2012.09.009
9. Stubbs BJ, Cox PJ, Evans RD, Santer P, Miller JJ, Faull OK, et al. On the metabolism of exogenous ketones in humans. Front Physiol. (2017) 8:848. doi: 10.3389/fphys.2017.00848
10. Stubbs BJ, Cox PJ, Kirk T, Evans RD, Clarke K. Gastrointestinal effects of exogenous ketone drinks are infrequent, mild and vary according to ketone compound and dose. Int J Sport Nutr Exerc Metab. (2019) 29:1–23. doi: 10.1123/ijsnem.2019-0014
11. O'Connor A, Chang JL, Brownlow M, Contractor N. Acute oral intake of beta-hydroxybutyrate in a pilot study transiently increased its capillary levels in healthy volunteers. J Nutr Health Food Eng. (2018) 8:324–8. doi: 10.15406/jnhfe.2018.08.00289
12. Fischer T, Och U, Klawon I, Och T, Grüneberg M, Fobker M, et al. Effect of a sodium and calcium DL-β-hydroxybutyrate salt in healthy adults. J Nutr Metab. (2018) 2018:9812806. doi: 10.1155/2018/9812806
13. O'Malley T, Myette-Cote E, Durrer C, Little JP. Nutritional ketone salts increase fat oxidation but impair high-intensity exercise performance in healthy adult males. Appl Physiol Nutr Metab. (2017) 42:1031–35. doi: 10.1139/apnm-2016-0641
14. Holland AM, Qazi AS, Beasley KN, Bennett HR. Blood and cardiovascular health parameters after supplementing with ketone salts for six weeks. J. Insul. Resist. (2019) 4:a47. doi: 10.4102/jir.v4i1.47
15. Newman JC, Verdin E. β-Hydroxybutyrate: a signaling metabolite. Annu Rev Nutr. (2017) 37:51–76. doi: 10.1146/annurev-nutr-071816-064916
16. Courchesne-Loyer A, Croteau E, Castellano CA, St-Pierre V, Hennebelle M, Cunnane SC. Inverse relationship between brain glucose and ketone metabolism in adults during short-term moderate dietary ketosis: a dual tracer quantitative positron emission tomography study. J Cereb Blood Flow Metab. (2017) 37:2485–93. doi: 10.1177/0271678X16669366
17. Fortier M, Castellano CA, Croteau E, Langlois F, Bocti C, St-Pierre V, et al. A ketogenic drink improves brain energy and some measures of cognition in mild cognitive impairment. Alzheimers Dement. (2019) 15:625–34. doi: 10.1016/j.jalz.2018.12.017
18. Croteau E, Castellano CA, Richard MA, Fortier M, Nugent S, Lepage M, et al. Ketogenic medium chain triglycerides increase brain energy metabolism in Alzheimer's disease. J Alzheimers Dis. (2018) 64:551–61. doi: 10.3233/JAD-180202
19. Neth BJ, Mintz A, Whitlow C, Jung Y, Solingapuram Sai K, Register TC, et al. Modified ketogenic diet is associated with improved cerebrospinal fluid biomarker profile, cerebral perfusion, and cerebral ketone body uptake in older adults at-risk for Alzheimer's disease: a pilot study. Neurobiol Aging. (2020) 86:54–63. doi: 10.1016/j.neurobiolaging.2019.09.015
20. Lopaschuk GD. Metabolic modulators in heart disease: past, present, and future. Can J Cardiol. (2017) 33:838–49. doi: 10.1016/j.cjca.2016.12.013
21. Gormsen LC, Svart M, Thomsen HH, Søndergaard E, Vendelbo MH, Christensen N, et al. Ketone body infusion with 3-hydroxybutyrate reduces myocardial glucose uptake and increases blood flow in humans: a positron emission tomography study. J Am Heart Assoc. (2017) 6:e005066. doi: 10.1161/JAHA.116.005066
22. Nieth H, Schollmeyer P. Substrate-utilization of the human kidney. Nature. (1966) 209:1244–5. doi: 10.1038/2091244a0
23. Weidemann MJ, Krebs HA. The fuel of respiration of rat kidney cortex. Biochem J. (1969) 112:149–66. doi: 10.1042/bj1120149
24. Nugent S, Tremblay S, Chen KW, Ayutyanont N, Roontiva A, Castellano CA, et al. Brain glucose and acetoacetate metabolism: a comparison of young and older adults. Neurobiol Aging. (2014) 35:1386–95. doi: 10.1016/j.neurobiolaging.2013.11.027
25. Croteau E, Castellano CA, Fortier M, Bocti C, Fulop T, Paquet N, et al. A cross-sectional comparison of brain glucose and ketone metabolism in cognitively healthy older adults, mild cognitive impairment and early Alzheimer's disease. Exp Gerontol. (2018) 107:18–26. doi: 10.1016/j.exger.2017.07.004
26. Croteau E, Tremblay S, Gascon S, Dumulon-Perreault V, Labbé SM, Rousseau JA, et al. [(11)C]-Acetoacetate PET imaging: a potential early marker for cardiac heart failure. Nucl Med Biol. (2014) 41:863–70. doi: 10.1016/j.nucmedbio.2014.08.006
27. Zeng M, Cao H. Fast quantification of short chain fatty acids and ketone bodies by liquid chromatography-tandem mass spectrometry after facile derivatization coupled with liquid-liquid extraction. J Chromatogr B Analyt Technol Biomed Life Sci. (2018) 1083:137–45. doi: 10.1016/j.jchromb.2018.02.040
28. Wilcoxon F, Katty SK, Wilcox RA. Critical values and probability levels for the Wilcoxon rank sum test and the Wilcoxon signed rank test. In: Selected Tables in Mathematical Statistics, Vol. 1. (1970). p. 171–259.
29. Hesse B, Lindhardt TB, Acampa W, Anagnostopoulos C, Ballinger J, Bax JJ, et al. EANM/ESC guidelines for radionuclide imaging of cardiac function. Eur J Nucl Med Mol Imaging. (2008) 35:851–85. doi: 10.1007/s00259-007-0694-9
30. Konradi C, Öngür D. Role of mitochondria and energy metabolism in schizophrenia and psychotic disorders. Schizophr Res. (2017) 187:1–2. doi: 10.1016/j.schres.2017.07.007
31. Matasic DS, Brenner C, London B. Emerging potential benefits of modulating NAD+ metabolism in cardiovascular disease. Am J Physiol Heart Circ Physiol. (2018) 314:H839–52. doi: 10.1152/ajpheart.00409.2017
32. Xin L, Ipek Ö, Beaumont M, Shevlyakova M, Christinat N, Masoodi M, et al. Nutritional ketosis increases NAD+/NADH ratio in healthy human brain: an in vivo study by 31P-MRS. Front Nutr. (2018) 5:62. doi: 10.3389/fnut.2018.00062
33. Elamin M, Ruskin DN, Masino SA, Sacchetti P. Ketone-based metabolic therapy: is increased NAD+ a primary mechanism? Front Mol Neurosci. (2017) 10:377. doi: 10.3389/fnmol.2017.00377
34. Büsing KA, Schönberg SO, Brade J, Wasser K. Impact of blood glucose, diabetes, insulin, and obesity on standardized uptake values in tumors and healthy organs on 18F-FDG PET/CT. Nucl Med Biol. (2013) 40:206–13. doi: 10.1016/j.nucmedbio.2012.10.014
35. Christensen NL, Jakobsen S, Schacht AC, Munk OL, Alstrup AKO, Tolbod LP, et al. Whole-body biodistribution, dosimetry, and metabolite correction of [11C]palmitate: a PET tracer for imaging of fatty acid metabolism. Mol Imaging. (2017) 16:1536012117734485. doi: 10.1177/1536012117734485
36. Nielsen R, Møller N, Gormsen LC, Tolbod LP, Hansson NH, Sorensen J, et al. Cardiovascular effects of treatment with the ketone body 3-hydroxybutyrate in chronic heart failure patients. Circulation. (2019) 139:2129–41. doi: 10.1161/CIRCULATIONAHA.118.036459
37. Fischer T, Och U, Marquardt T. Long-term ketone body therapy of severe multiple acyl-CoA dehydrogenase deficiency: a case report. Nutrition. (2019) 60:122–8. doi: 10.1016/j.nut.2018.10.014
38. Mudaliar S, Alloju S, Henry RR. Can a shift in fuel energetics explain the beneficial cardiorenal outcomes in the EMPA-REG OUTCOME study? A unifying hypothesis. Diabetes Care. (2016) 39:1115–22. doi: 10.2337/dc16-0542
39. Garofalo C, Borrelli S, Liberti ME, Andreucci M, Conte G, Minutolo R, et al. SGLT2 inhibitors: nephroprotective efficacy and side effects. Medicina. (2019) 55:268. doi: 10.3390/medicina55060268
Keywords: ketones, acetoacetate, beta-hydroxybutyrate, energy metabolism, medium chain triglyceride, positron emission tomography, nicotinamide adenine dinucleotide
Citation: Cuenoud B, Hartweg M, Godin J-P, Croteau E, Maltais M, Castellano C-A, Carpentier AC and Cunnane SC (2020) Metabolism of Exogenous D-Beta-Hydroxybutyrate, an Energy Substrate Avidly Consumed by the Heart and Kidney. Front. Nutr. 7:13. doi: 10.3389/fnut.2020.00013
Received: 08 October 2019; Accepted: 31 January 2020;
Published: 19 February 2020.
Edited by:
Jonathan Cedernaes, Uppsala University, SwedenReviewed by:
Jose E. Galgani, Pontifical Catholic University of Chile, ChileTodd Hagobian, California Polytechnic State University, United States
David M. Diamond, University of South Florida, United States
Copyright © 2020 Cuenoud, Hartweg, Godin, Croteau, Maltais, Castellano, Carpentier and Cunnane. This is an open-access article distributed under the terms of the Creative Commons Attribution License (CC BY). The use, distribution or reproduction in other forums is permitted, provided the original author(s) and the copyright owner(s) are credited and that the original publication in this journal is cited, in accordance with accepted academic practice. No use, distribution or reproduction is permitted which does not comply with these terms.
*Correspondence: Bernard Cuenoud, bernard.cuenoud@nestle.com