Current Status of Tissue Engineering in the Management of Severe Hypospadias
- 1Department of Health Science and Technology, Faculty of Medicine, Aalborg University, Aalborg, Denmark
- 2Department of Pediatric Surgery and Urology, Hamad General Hospital, Doha, Qatar
- 3College of Medicine, Qatar University, Doha, Qatar
- 4Department of Mechanical and Industrial Engineering, Qatar University, Doha, Qatar
- 5Department of Urology, Hamad Medical Corporation, Doha, Qatar
Hypospadias, characterized by misplacement of the urinary meatus in the lower side of the penis, is a frequent birth defect in male children. Because of the huge variation in the anatomic presentation of hypospadias, no single urethroplasty procedure is suitable for all situations. Hence, many surgical techniques have emerged to address the shortage of tissues required to bridge the gap in the urethra particularly in the severe forms of hypospadias. However, the rate of postoperative complications of currently available surgical procedures reaches up to one-fourth of the patients having severe hypospadias. Moreover, these urethroplasty techniques are technically demanding and require considerable surgical experience. These limitations have fueled the development of novel tissue engineering techniques that aim to simplify the surgical procedures and to reduce the rate of complications. Several types of biomaterials have been considered for urethral repair, including synthetic and natural polymers, which in some cases have been seeded with cells prior to implantation. These methods have been tested in preclinical and clinical studies, with variable degrees of success. This review describes the different urethral tissue engineering methodologies, with focus on the approaches used for the treatment of hypospadias. At present, despite many significant advances, the search for a suitable tissue engineering approach for use in routine clinical applications continues.
Introduction
Hypospadias is a frequent genitourinary congenital malformation with an incidence of around 1 per 300 male newborns, although its frequency varies among populations from 0.3 to 7.0 per 1,000 live births (1, 2). Hypospadias results from the malposition of the urinary meatus on the ventral aspect of the penis following incomplete closure of the urethral folds during early gestational weeks. This condition has been associated with hereditary and/or environmental factors that have not been completely identified yet (3). Three abnormalities that are most frequently associated with hypospadias are an abnormal location of the meatus, a curvature of the penis (chordee), and an incomplete ventral prepuce. In general, severe forms of hypospadias are associated with a significant chordee and a urethral meatus located proximal to the mid-shaft of the penis. The ventral axis components of the penis may also display abnormalities, including atrophy of the cavernous corpora and stiffening of the corpus spongiosum (4). Advances in microsurgical instrumentation, imaging devices, suture materials, and reconstructive techniques have significantly improved the outcome of hypospadias surgical repair during the last decade. However, the current management techniques for proximal hypospadias still present several limitations, which are mainly associated with the highly demanding surgical procedures and the graft donor site morbidity. Moreover, complication and reoperation rates remain unacceptably high. These constraints have initiated a drive toward the development of tissue engineering approaches that may offer a better alternative for pediatric urethral reconstruction. In this review, we summarize the limitations of the current surgical management of severe hypospadias and examine the recent tissue engineering developments toward repair of the urethra, with special focus on the research targeted to severe hypospadias in children.
Current Management of Severe Hypospadias and Its Limitations
Hundreds of different urethroplasty techniques have been reported for hyspospadias repair over the years (5). Treatment of distal hypospadias with one-stage urethroplasty approaches, such as onlay preputial island flaps or tubularized incised plate (TIP) repair, is currently associated with a relatively high success rate. However, management of severe hypospadias remains a challenge, as no single technique is applicable to all patients. There is still an ongoing discussion whether it is most appropriate to perform a single-stage or a two-stage approach. Recent reports indicate that more patients appear to develop complications following single-stage procedures (6) and that staged procedures may result in overall better functional outcomes (7). Yet, retrospective studies of patients undergoing two-stage repair have evidenced complication rates ranging from 50 to 68% (8, 9). The frequency of postoperative complications appears to increase with the anatomic severity of hypospadias no matter what surgical technique is employed (6). The most frequent complications include fistulas and urethral strictures, but can also include dehiscence of the repair or recurrence of chordee.
The outcome of the urethroplasty not only depends on the quality of the anatomical structures and the surgical approach but also on the availability of an appropriate source for the graft, since patients with severe hypospadias frequently require extra tissue to restore the missing urethra. Autologous sources of grafts utilized for urethral replacement include skin from genital areas or extra-genital regions (10–12). These grafts have been superseded by buccal mucosa free skin grafts which is currently the most widely used source (13). Harvesting of oral mucosal grafts represents an easy procedure, causing minimal discomfort for the patient and an acceptable degree of morbidity (14). However, the amounts of tissue available for harvesting are limited and complications may appear, including donor site bleeding, infection, pain, parotid duct injury, graft contracture, and numbness (15).
Further complexities may arise following the pubertal period, in that a failed neo-urethra may result in a scarcity of hair-free surrounding skin for urethral replacement. Moreover, a surgically constructed neo-urethra may fail to develop along with the penis as the affected person matures to post-pubertal age, causing stricture and secondary chordee. Balanitis xerotica obliterans, resulting from the use of adjacent affected urethral or penile tissues for repair, may also cause recurrent urethral strictures in patients who had undergone previous surgery for hypospadias. Eventually, any form of substitution urethroplasty seems to worsen over time, as complication rates appear to increase with the duration of the follow-up (16).
The Rise of Urethral Tissue Engineering
Overall, the disappointingly low success rates, the complications related to graft harvesting, and the tendency of grafts to deteriorate over time, are prompting us “to think outside the box” (Figure 1). Tissue engineering may hold the key to finding novel techniques and tissue sources to replace the missing urethra. Tissue-engineered grafts could be tailored with characteristics like those of urethral mucosa, but conveniently available “off the shelf.” Ideally, tissue-engineered constructs for urethral replacement should be biocompatible, able to be well vascularized and biodegradable (17, 18). The biodegradation process should follow with the regeneration timeframe of the local surrounding tissues to allow the generation of a fully differentiated functional urothelium (19). Moreover, optimal constructs should also be compliant enough to accommodate jets of propelled urine during voiding. Neo-urethral compliance can be adjusted by manipulating the mechanical properties of constructs to optimize stretch functionality (20). Furthermore, the construct should be impermeable to urine, as urine is cytotoxic to surrounding tissues (21).
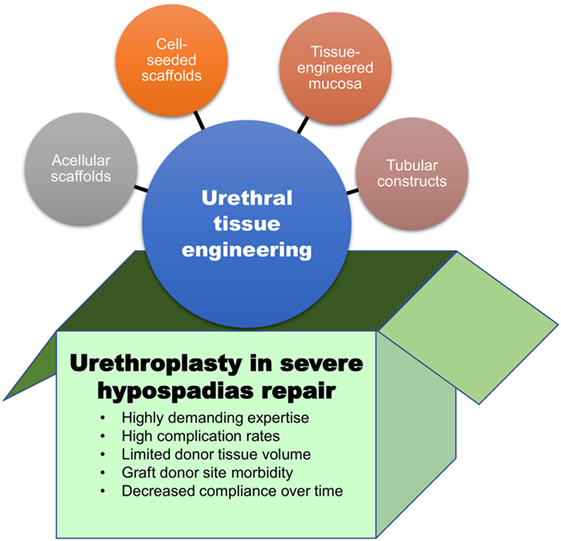
Figure 1. The box contains the main limitations of current surgical approaches for hypospadias repair. Outside the box, urethral tissue engineering approaches that may represent valuable therapeutic options for children with severe hypospadias.
To overcome the difficulties associated with current urethral repair techniques, during the last couple of decades, extensive research has been performed to investigate biomaterials and cells that could be used alone or in combination for urethral replacement. Table 1 provides some examples of the diverse types of biomaterials and cells that have been assessed for urethral tissue engineering. These sources will be described and discussed in the following sections.
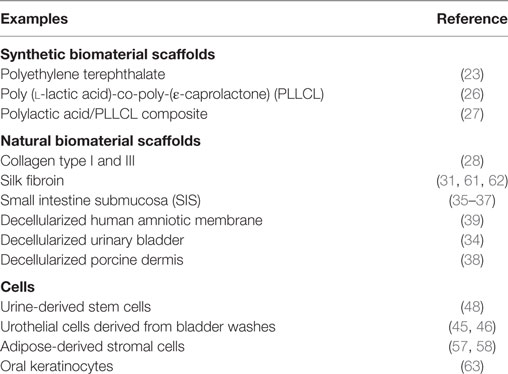
Table 1. Examples of biomaterials and cells that have been investigated for urethral tissue engineering.
Polymeric and Extracellular Matrix (ECM)-Derived Scaffolds
The scaffold acts as a supporting skeleton for tissue regeneration, maintaining the structural balance of the regenerating tissue and allowing its three-dimensional (3D) regeneration. The scaffolds can be categorized according to their biodegradability (non-biodegradable or biodegradable) or according to their source (synthetic, naturally derived, or a combination of both) (22).
Some of the synthetic non-degradable polymers that have been explored for urinary tract reconstruction include polytetrafluoroethylene and poly(ethylene terephthalate). These materials do not readily promote cellular attachment and, therefore, require a surface treatment to allow for urothelial cell adhesion (23). In general, non-degradable materials used in urinary tract reconstruction have been mainly used as temporary supports in specific clinical scenarios, as they have in general exhibited poor results, with the occurrence of complications including calcification, fistulae, chronic hematuria, encrustation, migration, and significant shortening (24, 25).
Synthetic biodegradable scaffolds, on the other hand, include those made of polymers, including poly-l-lactide (PLL), polycaprolactone (PCL), and poly(lactic-co-glycolic acid) (PLGA). The mechanical properties of these polymers, such as porosity and degradation rate, can be easily tailored to satisfy the requirements of a urethral construct. Polymeric composite membranes fabricated using PLL–PCL have demonstrated to possess mechanical properties suitable for urothelial tissue engineering, supporting growth and phenotype maintenance of human urothelial cells (26, 27). Synthetic biomaterials, however, usually require surface treatments to promote cell attachment, as they lack specific molecular elements for interaction with cells and proteins (22).
Natural polymers, on the other hand, exhibit specific cell adhesion ligands that favor the attachment and growth of cells onto their surface. Different naturally derived biomaterials have been explored for urethral replacement, including collagen (28), hyaluronic acid derivatives, alginate, and chitosan (29). Another promising biomaterial is silk fibroin (SF), a natural polymer obtained from Bombyx mori cocoons. This material has shown excellent biocompatibility, with reduced immunogenicity and fewer inflammatory reactions as compared to other biological materials. The mechanical characteristics of SF, including its elasticity and shape memory, were found to be well suited for urologic tissue engineering applications (30). Different cell types relevant for urethral reconstruction have been successfully grown onto porous SF scaffolds, including keratinocytes and fibroblasts (31). SF can also be blend with a synthetic polymer, such as PCL, to facilitate the fabrication of electrospun nanofiber allowing successful growth of oral mucosal epithelial (EP) cells (32).
Extracellular matrix obtained after chemical decellularization of xenogeneic or allogeneic tissues has also been extensively investigated as a scaffold for urethral reconstruction. ECM scaffolds exhibit very rapid biodegradability in vivo, releasing degradation components that orchestrate healing and regeneration through a process known as constructive tissue remodeling (33). Decellularized tissues retain most of the structural collagens and proteoglycans, which contribute not only to preserve the structural integrity but also to retain bioactive growth factors promoting ingrowth of endothelial and smooth muscle cells (SMCs) (34). Matrices prepared from small intestine submucosa (SIS) are those that have been most frequently investigated for urethral repair, thanks to their high content of collagens, fibronectin, elastin, and glycosaminoglycans (35–37). Other acellular matrices include decellularized porcine dermis (38), human amniotic membrane (39), and urinary bladder (40). A promising new source for generation of autologous tissue-engineered constructs is decellularized ECM obtained from cultures of progenitor cells (41, 42). These in vitro cell-derived matrices might be prepared using urethral-specific cells to provide a mixture of specific ECM components and biological factors that may provide the environmental signals controlling the fate of the diverse types of cells comprising the urethral tissue.
Cell-Seeded Scaffolds
No consensus has yet been reached on the potential beneficial effects of cell seeding of tissue-engineered scaffolds for use in the urogenital system, although it appears that cells are required for urethral repair of defects that are >0.5 cm in length (43). Although cells harvested from the urinary tract may be the optimal choice for urethral tissue engineering, other sources may be effective as well. A recent study was performed to determine whether EP cells from urinary and non-urinary sources may behave different in terms of their clonogenic capacity and ability to proliferate. Since only few differences were observed between oral mucosal and urethral cells, the authors suggested that these two sources may be similarly efficient to generate stratified epithelium for urethral reconstruction (44).
Several studies have examined the effects of seeding scaffolds with urothelial cells, which may be obtained invasively or non-invasively. Disadvantages of invasive techniques (e.g., open bladder biopsy) include the harvesting of an inadequate number of cells and their requirement for general anesthesia. Moreover, the associated morbidity of the donor site can lead to significant risks, including bleeding and infection. Alternatively, various non-invasive methods have been used to harvest the needed cells as well. For example, urothelial cells have been obtained from bladder washings, a method that has shown to be safe and highly reproducible in adults and children (45, 46). Urine-derived stem cells have also shown the ability to expand and to differentiate into both urothelial and smooth muscle phenotypes (47, 48). Cell proliferation is markedly influenced by the mechanical properties of the polymer scaffolds, and coculture of several cell types was shown to be superior to culture of individual cell types (38), perhaps because the former represents a more physiological condition, involving paracrine signaling among different cell types.
Fabrication and Addition of Bioactive Factors
Although several approaches have been utilized to fabricate these scaffolds, an attractive method of fabricating scaffolds for urethral replacement consists of producing cell-seeded tubular grafts that self-assemble (Figure 2) (49). Optimal results require adjusting the mechanical properties of these constructs, including surface topography that is important for ideal growth and differentiation of the cells. For example, attachment of cellular proteins important for urothelial cell adhesion can be enhanced by increasing the external roughness of these scaffolds (50, 51). Scaffold porosity is another parameter that needs to be controlled during fabrication to offer a route for the diffusion of nutrients to the cells, as well as for the elimination of metabolic products (52, 53). Exogenous trophic factors, including natural ECM proteins and growth factors, have been used to bio-functionalize scaffolds creating a microenvironment that simulates the integration of the tissue-engineered constructs (54–56).
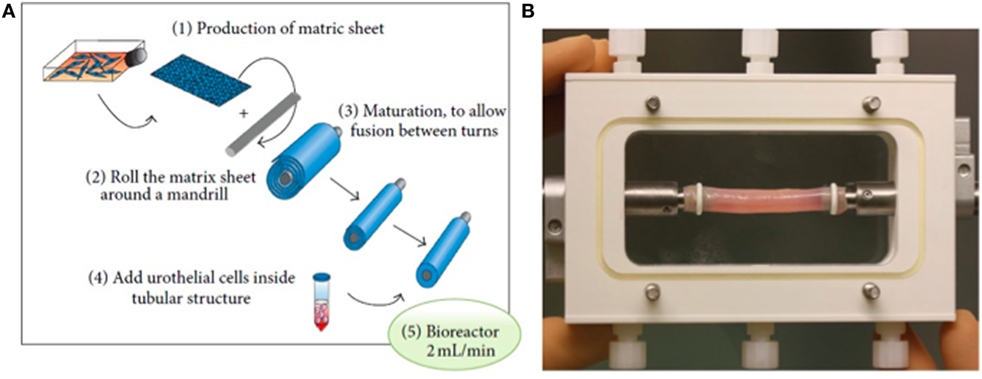
Figure 2. Fabrication of urethral scaffold tubes: (A) A matrix sheet of fibroblasts is rolled to form a tube, and urothelial cells are seeded in the lumen. (B) Illustration of a bioreactor for tubular cell-seeded grafts to stimulate differentiation and formation of a watertight mucosal layer [reprinted with permission from Ref. (49)]. © 2013 by Orabi et al.
Recent Translational and Clinical Studies Toward Tissue-Engineered Urethral Replacement
Several engineered urethral substitutes have been examined in animal models over the past three decades. Although some studies have demonstrated positive results, the experiments were performed in small series with short follow-up. Notwithstanding, the results of these animal studies have yielded several important conclusions. For example, failure of a urothelial layer to develop on the internal surface of the implanted constructs resulted in leakage of urine, with associated inflammation and fibrosis, leading to re-stricturing. Moreover, seeded constructs seem necessary to treat longer strictures, because the survival of these constructs does not depend on the ingrowth of EP cells from the surrounding healthy tissues but mainly on the pre-seeded cells (49). Efforts to identify better cell sources to seed the constructs have led to the use of adipose stem cells to reconstruct urinary tract epithelium (24, 57, 58) and SMCs (59) in urethral tissue engineering. A recent systematic review showed that, regardless of the type of scaffold material, cell seeding significantly reduced the risk of morbidities. Moreover, the addition of cells reduced failure rates when inlay or full urethroplasties were performed (60).
On the other hand, extent of vascularization represents a key factor for the success of the implanted constructs. In this direction, vascularization has been analyzed immunohistochemically in a rabbit model following ventral onlay grafts of SF (Figure 3). The samples of tissue-engineered urethra revealed SMC differentiation, EP maturation, and de novo vascularization and innervation processes (61). Several other preclinical studies strongly support the use of SF as a material for urethral tissue engineering, showing improved vascularization and reduced immunogenicity in comparison to conventional SIS scaffolds (61, 62).
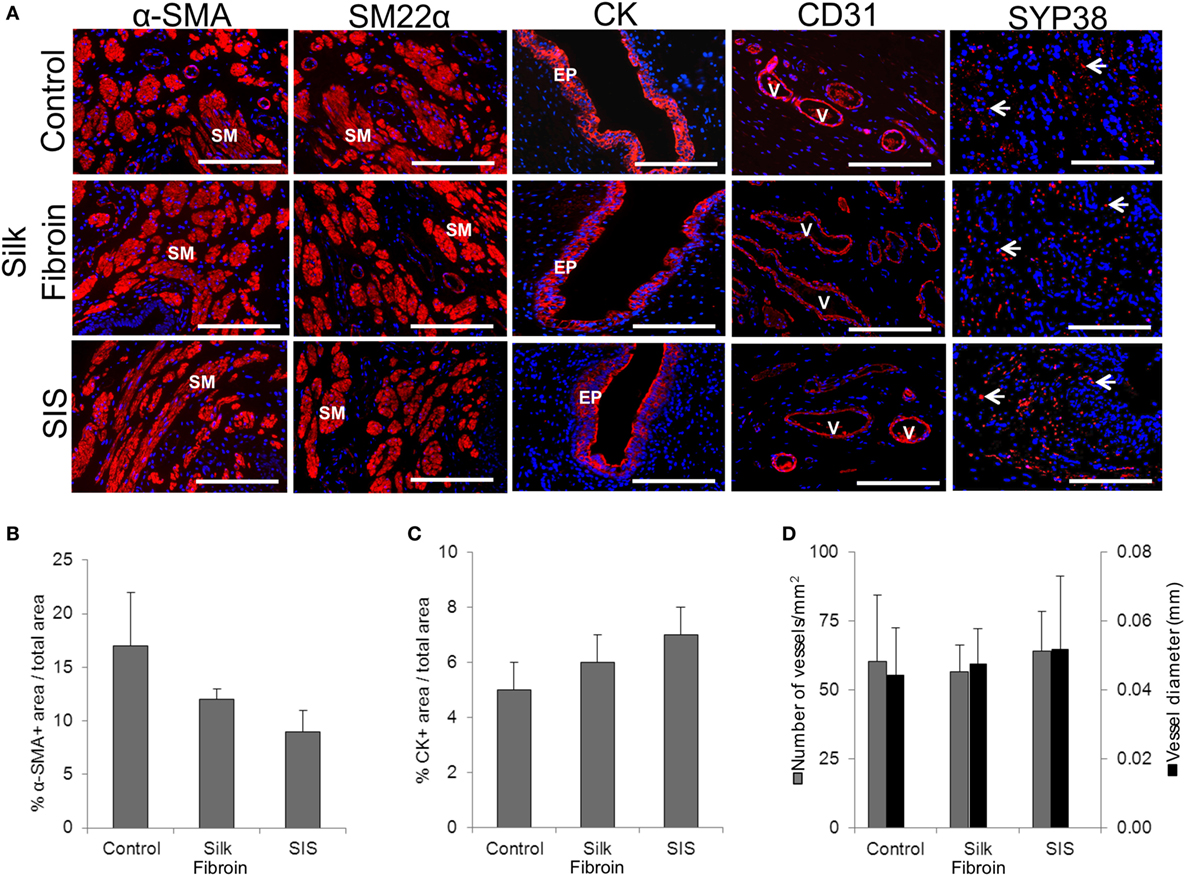
Figure 3. Urethral tissue regeneration following implantation of acellular silk fibroin scaffolds in rabbits. (A) Immunohistochemical assays showing the expression of smooth muscle (SM) contractile markers; epithelial (EP)-associated cytokeratins (CK); and endothelial markers. V indicates blood vessels and arrows denote cells of neuronal lineages. Scale bars denote 200 µm in all panels. Panels (B–D) display histomorphometric data from alpha-smooth muscle actin positive (a-SMA+) regions (B), CK positive cells (C), and CD31 positive vessels (D) obtained from control and scaffold implanted animals. [reprinted with permission from Ref. (61)]. © 2014 by Chung et al.
When considering the type of the reconstruction and the related shape of the construct, animal studies showed that synthetic materials did not perform as well for inlay repair as compared to full repair (60). It is also desirable to produce an artificial graft with characteristics similar to those of buccal mucosa and a tissue-engineered buccal mucosa for urethral replacement showed optimal short-term results in a rabbit model (63). Long-term results, on the other hand, showed that the collagen fibers in the construct were significantly more disordered than those in normal urethral submucosa (64).
Intriguingly, a recent meta-analysis comparing outcomes of several urethral tissue engineering preclinical and clinical studies has revealed that the favorable results seen in animal models are not always translated into the patients (60). A feasible explanation for these differences could be the fact that preclinical studies use animal models with a healthy urethra, limiting the level of evidence provided by the animal model.
Concerning clinical trials, several studies have been carried out to assess the performance of tissue-engineered grafts in pediatric patients (summarized in Table 2). In general, results using scaffolds or tissue-engineered constructs have been suboptimal in patients who had preceding failed urethroplasties and those with unhealthy vascular beds (65).
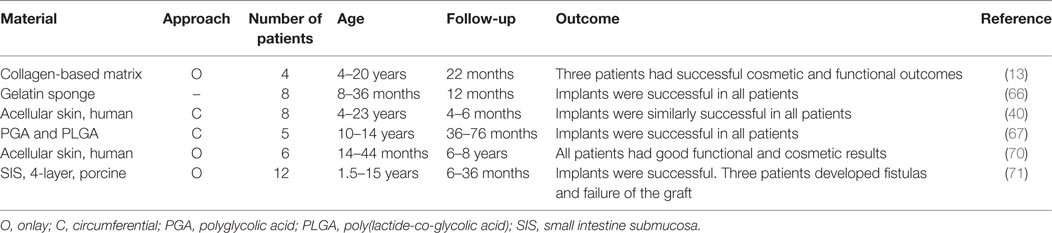
Table 2. Outcomes of clinical studies of tissue-engineered urethral replacement in pediatric patients [adapted from Ref. (15, 60)].
In 1999, Atala et al. showed that the use of bladder submucosa and a collagen-based inert matrix for urethroplasty in four patients with hypospadias yielded positive results (13). Despite the small number of patients, the short duration of follow-up (22 months) and the development of a fistula in one patient, these findings suggest that tissue-engineered materials should be tested in larger numbers of patients. 10 years later, use of a porous gelatin scaffold and preputial mucosa or a urethral plate graft combined with local flap to repair severe hypospadias in eight patients revealed that all were successful after 1-year follow-up (66).
A porous gelatin scaffold was also shown to be successful in eight patients with hypospadias, of mean age 13 years in a consecutive experiment (40). Another clinical trial, in which patients were followed up for 6 years, found that tubularized constructs composed of PGA:PLGA meshes seeded with bladder cells were successful in managing urethral trauma in pediatric patients (67). However, degradation products of polyester-based scaffolds can induce chronic inflammation in vivo (68) and may, therefore, adversely affect the long-term fate of implanted constructs (69).
In 2013, Fossum et al. tested the long-term (about 8 years) effects of cultured autologous urothelial cell implants in hypospadias patients (70). That study found that use of these implants in patients with severe hypospadias resulted in high complication rates, but with results equal to or better than expected for their phenotypes. However, the limitations of this study included the small number of patients and the absence of a control group.
A recent clinical study found that SIS grafts were successful in circumcised patients with hypospadias or undergoing repeat hypospadias repair (71) (Figure 4). Three of the 12 patients developed fistula, which may have been caused by the occurrence of infection, but these fistulas were easy to repair. Limitations included the heterogeneity of the hypospadias (distal, mid-shaft, and proximal), the heterogeneity of patient age (from infants to teenagers), and the inclusion of untreated patients and those who had failed previous repair, all of which might generate confusion in the interpretation of results.
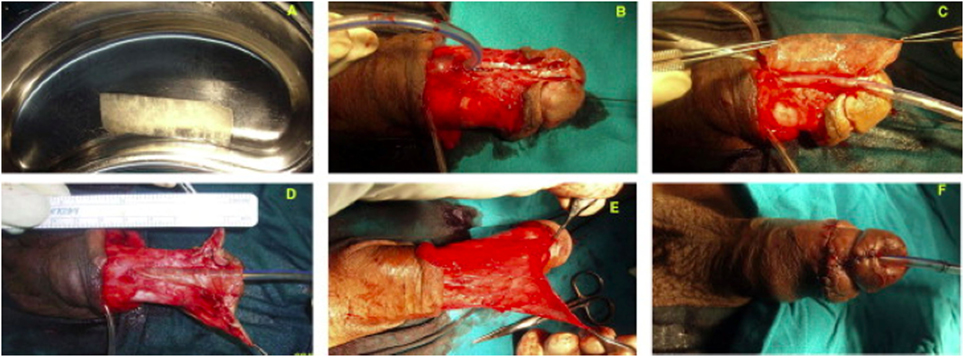
Figure 4. (A) Gross appearance of a small intestine submucosa (SIS) graft; (B) penile skin degloving through a subcoronal incision, preserving the urethral plate; (C) suturing of the SIS graft in an onlay fashion; (D) suturing of the completed onlay SIS graft; (E) splitting of the dartos flap into two halves to form the second layer of coverage for the graft; and (F) final postoperative appearance [reprinted with permission from Ref. (71)]. © 2013 by Elsevier B.V.
Conclusion and Future Directions
Current urethroplasty techniques for the management of severe hypospadias are associated with significant morbidities and limitations that strongly mandates the need to “think outside the box.” Advances in urethral tissue engineering biomaterials and fabrication methods have built a solid base on which future urethral replacement approaches can stand on. The currently available experimental evidence suggests that long and complex urethral defects would require tubular cell loaded constructs in contrast to onlay grafts, which have shown benefit in shorter defects. Although the search for an “ideal” combination of biomaterials and cells for urethral replacement continues, it appears that optimal results could be obtained with composite materials seeded what different cell types (stromal and EP). Future research in this field should take into consideration more realistic animal models to bridge the translational gap between animal models and clinical studies and the importance of longer follow-up periods. We believe that biodegradability is a crucial factor for successful urethral tissue-engineered regeneration in children, which may accompany the natural growth of penile size at puberty.
Author Contributions
TA conceived the article, planned and prepared its structure, and performed the bibliographical search. TA wrote the manuscript draft under supervision of CP. EM, AA, AH, and CP edited sections of the manuscript and contributed to the critical revision of the final draft.
Conflict of Interest Statement
The authors declare that the research was conducted in the absence of any commercial or financial relationships that could be construed as a potential conflict of interest.
References
1. Springer A, van den Heijkant M, Baumann S. Worldwide prevalence of hypospadias. J Pediatr Urol (2016) 12:152.e1–7. doi:10.1016/j.jpurol.2015.12.002
2. Fisch H, Hyun G, Hensle TW. Rising hypospadias rates: disproving a myth. J Pediatr Urol (2010) 6:37–9. doi:10.1016/j.jpurol.2009.05.005
3. Kalfa N, Sultan C, Baskin LS. Hypospadias: etiology and current research. Urol Clin North Am (2010) 37:159–66. doi:10.1016/j.ucl.2010.03.010
4. Camoglio FS, Bruno C, Zambaldo S, Zampieri N. Hypospadias anatomy: elastosonographic evaluation of the normal and hypospadic penis. J Pediatr Urol (2016) 12:199.e1–5. doi:10.1016/j.jpurol.2016.02.007
5. Springer A, Tekgul S, Subramaniam R. An update of current practice in hypospadias surgery. Eur Urol Suppl (2017) 16:8–15. doi:10.1016/j.eursup.2016.09.006
6. Long CJ, Chu DI, Tenney RW, Morris AR, Weiss DA, Shukla AR, et al. Intermediate-term followup of proximal hypospadias repair reveals high complication rate. J Urol (2017) 197:852–8. doi:10.1016/j.juro.2016.11.054
7. Pippi Salle JL, Sayed S, Salle A, Bagli D, Farhat W, Koyle M, et al. Proximal hypospadias: a persistent challenge. Single institution outcome analysis of three surgical techniques over a 10-year period. J Pediatr Urol (2016) 12:28.e1–7. doi:10.1016/j.jpurol.2015.06.011
8. McNamara ER, Schaeffer AJ, Logvinenko T, Seager C, Rosoklija I, Nelson CP, et al. Management of proximal hypospadias with 2-stage repair: 20-year experience. J Urol (2015) 194:1080–5. doi:10.1016/j.juro.2015.04.105
9. Stanasel I, Le HK, Bilgutay A, Roth DR, Gonzales ET, Janzen N, et al. Complications following staged hypospadias repair using transposed preputial skin flaps. J Urol (2015) 194:512–6. doi:10.1016/j.juro.2015.02.044
10. Altarac S, Papeš D, Bracka A. Surgery illustrated surgical atlas: two-stage hypospadias repair with inner preputial layer Wolfe graft (Aivar Bracka repair). BJU Int (2012) 110:460–73. doi:10.1111/j.1464-410X.2012.11304.x
11. Duan C, Huang X, Chen B, Li Q, Chen W, Li S. [Repair of hypospadias using scrotal septum vascular pedicle flap with two wing-like split-thickness skin graft]. Zhongguo Xiu Fu Chong Jian Wai Ke Za Zhi (2009) 23:316–8. (in Chinese).
12. Schwentner C, Seibold J, Colleselli D, Alloussi SH, Schilling D, Stenzl A, et al. Single-stage dorsal inlay full-thickness genital skin grafts for hypospadias reoperations: extended follow up. J Pediatr Urol (2011) 7:65–71. doi:10.1016/j.jpurol.2010.01.016
13. Atala A, Guzman L, Retik AB. A novel inert collagen matrix for hypospadias repair. J Urol (1999) 162:1148–51. doi:10.1016/S0022-5347(01)68105-9
14. Barbagli G, Vallasciani S, Romano G, Fabbri F, Guazzoni G, Lazzeri M. Morbidity of oral mucosa graft harvesting from a single cheek. Eur Urol (2010) 58(1):33–41. doi:10.1016/j.eururo.2010.01.012
15. De Kemp V, De Graaf P, Fledderus JO, Bosch JLHR, De Kort LMO. Tissue engineering for human urethral reconstruction: systematic review of recent literature. PLoS One (2015) 10:e0118653. doi:10.1371/journal.pone.0118653
16. Mousavi SA, Aarabi M. Tubularized incised plate urethroplasty for hypospadias reoperation: a review and meta-analysis. Int Braz J Urol (2014) 40:588–95. doi:10.1590/S1677-5538.IBJU.2014.05.02
17. O’Brien FJ. Biomaterials & scaffolds for tissue engineering. Mater Today (2011) 14:88–95. doi:10.1016/S1369-7021(11)70058-X
18. Ulery BD, Nair LS, Laurencin CT. Biomedical applications of biodegradable polymers. J Polym Sci B Polym Phys (2011) 49:832–64. doi:10.1002/polb.22259
19. Atala A, Bauer SB, Soker S, Yoo JJ, Retik AB. Tissue-engineered autologous bladders for patients needing cystoplasty. Lancet (2006) 367:1241–6. doi:10.1016/S0140-6736(06)68438-9
20. Lu SH, Sacks MS, Chung SY, Gloeckner DC, Pruchnic R, Huard J, et al. Biaxial mechanical properties of muscle-derived cell seeded small intestinal submucosa for bladder wall reconstitution. Biomaterials (2005) 26:443–9. doi:10.1016/j.biomaterials.2004.05.006
21. Vaegler M, Maurer S, Toomey P, Amend B, Sievert KD. Tissue engineering in urothelium regeneration. Adv Drug Deliv Rev (2015) 82:64–8. doi:10.1016/j.addr.2014.11.021
22. Lin HK, Madihally SV, Palmer B, Frimberger D, Fung KM, Kropp BP. Biomatrices for bladder reconstruction. Adv Drug Deliv Rev (2015) 82:47–63. doi:10.1016/j.addr.2014.11.020
23. Bisson I, Hilborn J, Wurm F, Meyrat B, Frey P. Human urothelial cells grown on collagen adsorbed to surface-modified polymers. Urology (2002) 60:176–80. doi:10.1016/S0090-4295(02)01642-4
24. Li H, Xu Y, Xie H, Li C, Song L, Feng C, et al. Epithelial-differentiated adipose-derived stem cells seeded bladder acellular matrix grafts for urethral reconstruction: an animal model. Tissue Eng Part A (2014) 20:774–84. doi:10.1089/ten.TEA.2013.0122
25. Tammela T. Temporary urethral stents. In: Denstedt J, Atala A, editors. Biomaterials and Tissue Engineering in Urology. Woodhead Publishing (2009). p. 208–25.
26. Sartoneva R, Haimi S, Miettinen S, Mannerström B, Haaparanta A-M, Sándor GK, et al. Comparison of a poly-L-lactide-co-ε-caprolactone and human amniotic membrane for urothelium tissue engineering applications. J R Soc Interface (2011) 8:671–7. doi:10.1098/rsif.2010.0520
27. Sartoneva R, Haaparanta A-M, Lahdes-Vasama T, Mannerstrom B, Kellomaki M, Salomaki M, et al. Characterizing and optimizing poly-L-lactide-co-ε-caprolactone membranes for urothelial tissue engineering. J R Soc Interface (2012) 9:3444–54. doi:10.1098/rsif.2012.0458
28. Sabbagh W, Masters JRW, Duffy PG, Herbage D, Brown RA. In vitro assessment of a collagen sponge for engineering urothelial grafts. Br J Urol (1998) 82:888–94. doi:10.1046/j.1464-410X.1998.00828.x
29. Scriven SD, Trejdosiewicz LK, Thomas DFM, Southgate J. Urothelial cell transplantation using biodegradable synthetic scaffolds. J Mater Sci Mater Med (2001) 12:991–6. doi:10.1023/A:1012869318205
30. Sack BS, Mauney JR, Estrada CR. Silk fibroin scaffolds for urologic tissue engineering. Curr Urol Rep (2016) 17:1–10. doi:10.1007/s11934-015-0567-x
31. Xie M, Xu Y, Song L, Wang J, Lv X, Zhang Y. Tissue-engineered buccal mucosa using silk fibroin matrices for urethral reconstruction in a canine model. J Surg Res (2014) 188:1–7. doi:10.1016/j.jss.2013.11.1102
32. Wei G, Li C, Fu Q, Xu Y, Li H. Preparation of PCL/silk fibroin/collagen electrospun fiber for urethral reconstruction. Int Urol Nephrol (2015) 47:95–9. doi:10.1007/s11255-014-0854-3
33. Brown BN, Badylak SF. Extracellular matrix as an inductive scaffold for functional tissue reconstruction. Transl Res (2014) 163:268–85. doi:10.1016/j.trsl.2013.11.003
34. Yang B, Zhang Y, Zhou L, Sun Z, Zheng J, Chen Y, et al. Development of a porcine bladder acellular matrix with well-preserved extracellular bioactive factors for tissue engineering. Tissue Eng Part C Methods (2010) 16:1201–11. doi:10.1089/ten.tec.2009.0311
35. Fiala R, Vidlar A, Vrtal R, Belej K, Student V. Porcine small intestinal submucosa graft for repair of anterior urethral strictures. Eur Urol (2007) 51:1702–8. doi:10.1016/j.eururo.2007.01.099
36. Donkov II, Bashir A, Elenkov CHG, Panchev PK. Dorsal onlay augmentation urethroplasty with small intestinal submucosa: modified Barbagli technique for strictures of the bulbar urethra. Int J Urol (2006) 13:1415–7. doi:10.1111/j.1442-2042.2006.01587.x
37. Palminteri E, Berdondini E, Fusco F, De Nunzio C, Salonia A. Long-term results of small intestinal submucosa graft in bulbar urethral reconstruction. Urology (2012) 79:695–701. doi:10.1016/j.urology.2011.09.055
38. Kimuli M, Eardley I, Southgate J. In vitro assessment of decellularized porcine dermis as a matrix for urinary tract reconstruction. BJU Int (2004) 94:859–66. doi:10.1111/j.1464-410X.2004.05047.x
39. Chen C, Zheng S, Zhang X, Dai P, Gao Y, Nan L, et al. Transplantation of amniotic scaffold-seeded mesenchymal stem cells and/or endothelial progenitor cells from bone marrow to efficiently repair 3-cm circumferential urethral defect in model dogs. Tissue Eng Part A (2017). doi:10.1089/ten.tea.2016.0518
40. Yang W, Guo J, Zhang Y, Ma T, Li Y, An F. Tissue engineered patch treatment of the urinary leakage after hypospadias repair. J Clin Rehabil Tissue Eng Res (2011) 15:7973–6. doi:10.3969/j.issn.1673-8225.2011.42.043
41. Hyldig K, Riis S, Pennisi CP, Zachar V, Fink T. Implications of extracellular matrix production by adipose tissue-derived stem cells for development of wound healing therapies. Int J Mol Sci (2017) 18:1167. doi:10.3390/ijms18061167
42. Hoshiba T. Cultured cell-derived decellularized matrices: a review towards the next decade. J Mater Chem B (2017) 5:4322–31. doi:10.1039/C7TB00074J
43. Dorin RP, Pohl HG, De Filippo RE, Yoo JJ, Atala A. Tubularized urethral replacement with unseeded matrices: what is the maximum distance for normal tissue regeneration? World J Urol (2008) 26:323–6. doi:10.1007/s00345-008-0316-6
44. Corradini F, Zattoni M, Barbagli G, Bianchi G, Giovanardi M, Serafini C, et al. Comparative assessment of cultures from oral and urethral stem cells for urethral regeneration. Curr Stem Cell Res Ther (2016) 11:643–51. doi:10.2174/1574888X10666150902094644
45. Fossum M, Gustafson C-J, Nordenskjold A, Kratz G. Isolation and in vitro cultivation of human urothelial cells from bladder washings of adult patients and children. Scand J Plast Reconstr Surg Hand Surg (2003) 37:41–5. doi:10.1080/alp.37.1.41.45
46. Nagele U, Maurer S, Feil G, Bock C, Krug J, Sievert KD, et al. In vitro investigations of tissue-engineered multilayered urothelium established from bladder washings. Eur Urol (2008) 54:1414–22. doi:10.1016/j.eururo.2008.01.072
47. Bharadwaj S, Liu G, Shi Y, Wu R, Yang B, He T, et al. Multipotential differentiation of human urine-derived stem cells: potential for therapeutic applications in urology. Stem Cells (2013) 31:1840–56. doi:10.1002/stem.1424
48. Liu Y, Ma W, Liu B, Wang Y, Chu J, Xiong G, et al. Urethral reconstruction with autologous urine-derived stem cells seeded in three-dimensional porous small intestinal submucosa in a rabbit model. Stem Cell Res Ther (2017) 8:63. doi:10.1186/s13287-017-0500-y
49. Orabi H, Bouhout S, Morissette A, Rousseau A, Chabaud S, Bolduc S. Tissue engineering of urinary bladder and urethra: advances from bench to patients. ScientificWorldJournal (2013) 2013:1–13. doi:10.1155/2013/154564
50. Yang Y, Leong KW. Nanoscale surfacing for regenerative medicine. Wiley Interdiscip Rev Nanomed Nanobiotechnol (2010) 2:478–95. doi:10.1002/wnan.74
51. Chun YW, Lim H, Webster TJ, Haberstroh KM. Nanostructured bladder tissue replacements. Wiley Interdiscip Rev Nanomed Nanobiotechnol (2011) 3:134–45. doi:10.1002/wnan.89
52. Murphy CM, Duffy GP, Schindeler A, O’Brien FJ. Effect of collagen-glycosaminoglycan scaffold pore size on matrix mineralization and cellular behavior in different cell types. J Biomed Mater Res A (2016) 104:291–304. doi:10.1002/jbm.a.35567
53. Brehmer B, Rohrmann D, Becker C, Rau G, Jakse G. Different types of scaffolds for reconstruction of the urinary tract by tissue engineering. Urol Int (2007) 78:23–9. doi:10.1159/000096930
54. Mondalek FG, Ashley RA, Roth CC, Kibar Y, Shakir N, Ihnat MA, et al. Enhanced angiogenesis of modified porcine small intestinal submucosa with hyaluronic acid-poly(lactide-co-glycolide) nanoparticles: from fabrication to preclinical validation. J Biomed Mater Res A (2010) 94:712–9. doi:10.1002/jbm.a.32748
55. Kanematsu A, Yamamoto S, Noguchi T, Ozeki M, Tabata Y, Ogawa O. Bladder regeneration by bladder acellular matrix combined with sustained release of exogenous growth factor. J Urol (2003) 170:1633–8. doi:10.1097/01.ju.0000084021.51099.8a
56. Mauney JR, Adam RM. Dynamic reciprocity in cell-scaffold interactions. Adv Drug Deliv Rev (2015) 82:77–85. doi:10.1016/j.addr.2014.10.016
57. Shi J-G, Fu W-J, Wang X-X, Xu Y-D, Li G, Hong B-F, et al. Transdifferentiation of human adipose-derived stem cells into urothelial cells: potential for urinary tract tissue engineering. Cell Tissue Res (2012) 347:737–46. doi:10.1007/s00441-011-1317-0
58. Zhang M, Peng Y, Zhou Z, Zhou J, Wang Z, Lu M. Differentiation of human adipose-derived stem cells co-cultured with urothelium cell line toward a urothelium-like phenotype in a nude murine model. Urology (2013) 81:465.e15–22. doi:10.1016/j.urology.2012.10.030
59. Fu Q, Deng CL, Zhao RY, Wang Y, Cao Y. The effect of mechanical extension stimulation combined with epithelial cell sorting on outcomes of implanted tissue-engineered muscular urethras. Biomaterials (2014) 35:105–12. doi:10.1016/j.biomaterials.2013.09.067
60. Versteegden LRM, de Jonge PKJD, IntHout J, van Kuppevelt TH, Oosterwijk E, Feitz WFJ, et al. Tissue engineering of the urethra: a systematic review and meta-analysis of preclinical and clinical studies. Eur Urol (2017) 72:594–606. doi:10.1016/j.eururo.2017.03.026
61. Chung YG, Tu D, Franck D, Gil ES, Algarrahi K, Adam RM, et al. Acellular bi-layer silk fibroin scaffolds support tissue regeneration in a rabbit model of onlay urethroplasty. PLoS One (2014) 9:e91592. doi:10.1371/journal.pone.0091592
62. Wang Y, Rudym DD, Walsh A, Abrahamsen L, Kim H-J, Kim HS, et al. In vivo degradation of three-dimensional silk fibroin scaffolds. Biomaterials (2008) 29:3415–28. doi:10.1016/j.biomaterials.2008.05.002
63. Li C, Xu YM, Song LJ, Fu Q, Cui L, Yin S. Urethral reconstruction using oral keratinocyte seeded bladder acellular matrix grafts. J Urol (2008) 180:1538–42. doi:10.1016/j.juro.2008.06.013
64. Li C, Xu Y-M, Liu Z-S, Li H-B. Engineering and RNA interference techniques. Urology (2013) 81:1075–80. doi:10.1016/j.urology.2013.01.041
65. El Kassaby A, AbouShwareb T, Atala A. Randomized comparative study between buccal mucosal and acellular bladder matrix grafts in complex anterior urethral strictures. J Urol (2008) 179:1432–6. doi:10.1016/j.juro.2007.11.101
66. Li P, Li S, Zhao M, Shi B, Li Q, Wang Y, et al. [Urethral reconstruction using gelatin sponge and micro-mucosa graft combined with local flap]. Zhongguo Xiu Fu Chong Jian Wai Ke Za Zhi (2009) 23:313–5. (in Chinese).
67. Raya-Rivera A, Esquiliano DR, Yoo JJ, Lopez-Bayghen E, Soker S, Atala A. Tissue-engineered autologous urethras for patients who need reconstruction: an observational study. Lancet (2011) 377:1175–82. doi:10.1016/S0140-6736(10)62354-9
68. Ceonzo K, Gaynor A, Shaffer L, Kojima K, Vacanti CA, Stahl GL. Polyglycolic acid-induced inflammation: role of hydrolysis and resulting complement activation. Tissue Eng (2006) 12:301–8. doi:10.1089/ten.2006.12.301
69. Gomez P, Gil ES, Lovett ML, Rockwood DN, Di Vizio D, Kaplan DL, et al. The effect of manipulation of silk scaffold fabrication parameters on matrix performance in a murine model of bladder augmentation. Biomaterials (2011) 32:7562–70. doi:10.1016/j.biomaterials.2011.06.067
70. Fossum M, Skikuniene J, Orrego A, Nordenskjold A. Prepubertal follow-up after hypospadias repair with autologous in vitro cultured urothelial cells. Acta Paediatr (2012) 101:755–60. doi:10.1111/j.1651-2227.2012.02659.x
Keywords: hypospadias, urethra, urethroplasty, postoperative complications, tissue engineering, biomaterials
Citation: Abbas TO, Mahdi E, Hasan A, AlAnsari A and Pennisi CP (2018) Current Status of Tissue Engineering in the Management of Severe Hypospadias. Front. Pediatr. 5:283. doi: 10.3389/fped.2017.00283
Received: 27 October 2017; Accepted: 13 December 2017;
Published: 22 January 2018
Edited by:
Miguel Alfedo Castellan, University of Miami, United StatesReviewed by:
Yuval Bar-Yosef, Dana-Dwek Children’s Hospital, IsraelMarcos Raymond Perez-Brayfield, University of Puerto Rico, Puerto Rico
Santiago Vallasciani, King Faisal Specialist Hospital & Research Centre, Saudi Arabia
Copyright: © 2018 Abbas, Mahdi, Hasan, AlAnsari and Pennisi. This is an open-access article distributed under the terms of the Creative Commons Attribution License (CC BY). The use, distribution or reproduction in other forums is permitted, provided the original author(s) or licensor are credited and that the original publication in this journal is cited, in accordance with accepted academic practice. No use, distribution or reproduction is permitted which does not comply with these terms.
*Correspondence: Tariq O. Abbas, tariq2c@hotmail.com, abbas@hst.aau.dk