Understanding regulation in complex environments: a route to enhance photosynthetic light-reactions in microalgae photobioreactors
- Department of Biology, University of Padova, Padova, Italy
Microalgae are recognized as a sustainable source of biomass to produce a wide range of bioproducts. To maximize the positive environmental impact and achieve economic competitiveness of microalgae-based products, it is however still essential to improve the biomass productivity during large-scale cultivation. Microalgae large-scale cultures are generally limited by light availability and thus the efficiency in conversion of radiation energy into biomass is a major factor impacting productivity. Natural light is a highly variable environmental parameter, and it constantly changes following seasons, time of day, and weather conditions. The artificial environment of large-scale microalgae cultures generates a further layer of complexity added to these natural light dynamics. In fact, because of biomass density and cell self-shading, light is unevenly distributed in the mass culture. Moreover, because of mixing, cells move between different parts of the volume, generating abrupt fluctuations in light exposure. Although microalgae evolved various regulatory mechanisms to cope with dynamic light conditions, these are not adapted to respond to the complex mixture of natural and artificial fluctuations commonly encountered in large-scale cultures, often causing reduction in photosynthetic efficiency. In the past years, genetic approaches to improve the light reactions of photosynthesis have been explored to optimise the composition and regulation of the photosynthetic machinery to large-scale cultivation. These approaches have shown promising results at the laboratory scale but have yet to be fully proven at the industrial scale. This can be explained by the fact that the complexity of the cultivation environment on microalgae photosynthesis and its impact on productivity is underestimated. This work aims for a systematic discussion on the complex role played by the growth environment in determining microalgae photosynthetic performances upon cultivation at industrial scale, with the objective of maximizing the impact of genetic modifications and ultimately fully realize the potential of microalgae for biomass productivity.
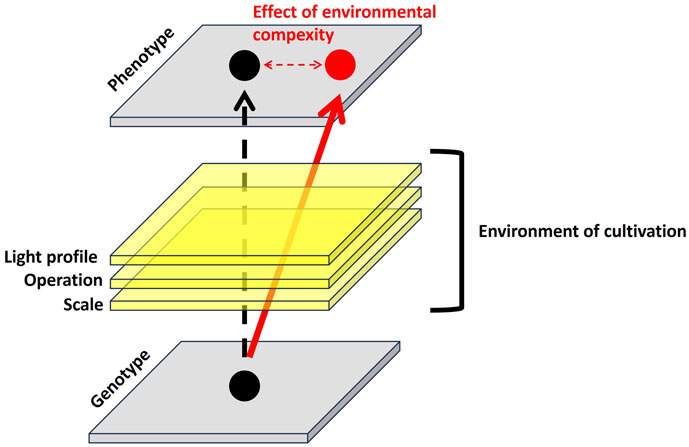
GRAPHICAL ABSTRACT | Effect of environmental complexity on performances of improved microalgae in PBR.
Potential of microalgae as sustainable source of biomass
Currently, global economy is responsible for a massive release of greenhouse gases (GHG) and other pollutants in the atmosphere (Gies, 2017), with heavy negative consequences for the wellbeing of our society on the long term (Walsh et al., 2015). Photosynthetic organisms fix CO2 into biomass using only the energy from sunlight and provide a relevant contribution in mitigating current anthropogenic GHG emissions.
Plants are at the base of food production, but their biomass also find many other applications in energy, nutraceutical and pharmaceutics sectors. However, if the current society aims to deploy a more sustainable economy, plants alone cannot satisfy such an expected growing demand for biomass (Lynch et al., 2021), especially if the food production must be increased to respond to a growing global population. This motivates the search for alternative sources of biomass that do not compete with the current food production chain for the same limiting factors, such as fertile land, fresh water and fertilizers (Rulli et al., 2016).
Microalgae have long been recognised as a valuable alternative biomass source, as they are photosynthetic organisms whose cultivation does not need arable land and can use civil and industrial wastewaters as nutrients’ source for their cultivation, thus avoiding competition with limiting factors for agriculture (Perin and Morosinotto, 2019b). In microalgae, photon-to-biomass-conversion efficiency (PBCE) is higher than in plants [i.e., approx. 3%–4% in lab-scale conditions with crops in the field limited to only 0.2%–1% (Melis, 2009; De Vree et al., 2015);]. This is not due to a more efficient metabolism per se but to other factors like: i) the whole biomass is photosynthetically active, unlike plants that have non-photosynthetic tissues (e.g., roots); ii) microalgae are always photosynthetically active, regardless of season, unlike crops that show growth cycles limited to few months in temperate climates, curbing the total biomass producible per year.
The larger PBCE of microalgae is also responsible for an increased capacity for CO2 sequestration, enabling the cultivation of these microorganisms in connection with industrial sites for a direct mitigation of anthropogenic GHG emissions. Higher growth rates also mean a faster sequestration of nutrients from waste-streams, bringing these organisms also at the forefront for the establishment of a circular nutrients economy, to preserve our current food production system whilst mitigating its heavy environmental impact (Perin et al., 2019b).
Microalgae are a group of organisms with a large genetic and metabolic diversity and their biomass can represent a feedstock to produce many different bio-commodities, from food/feed, biofertilizers to biofuels. They also contain many valuable molecules for various applications, like pigments (i.e., carotenoids and keto-carotenoids) and fatty acids (i.e., omega-3 fatty acids), some already commercialized in nutraceutical and pharmaceutical markets (Bilal et al., 2017; Ng et al., 2017; Koutra et al., 2018; Maeda et al., 2018; Renuka et al., 2018). While not all the possible applications are compatible with each other, e.g., cultivation on wastewaters poses some limitations for the following exploitation of the biomass as food or feed supplement, still it is clear that many different products can be extracted from the biomass.
Independently from the specific desired application, however, in most cases the biomass productivity values currently achievable are too low, leading to high prices necessary to compensate for production, harvesting and processing costs of an intensive large-scale cultivation (Lam et al., 2018), making microalgae-based products not competitive in large commodity markets (e.g., biofuels, biofertilizers) (Perin and Jones, 2019).
One of the reasons for this low productivity is that microalgae cultivated at large scale still show a substantially lower PBCE [i.e. 0.5% (De Vree et al., 2015);] than in laboratory conditions with cultures limited by light availability (Perin et al., 2022). One unavoidable challenge to make the industrial production of microalgae biomass competitive in large markets and maximize the potential environmental benefits associated with the cultivation of these organisms is to improve their photon-to-biomass conversion efficiency (PBCE) at large scale.
Light environment of microalgae cultures at industrial scale
Microalgae at industrial scale are cultivated in photobioreactors (PBRs) or ponds where they often reach high cell densities to maximize biomass productivity. This leads to an inhomogeneous light distribution because of cells’ self-shading. As an example, for a culture with a 5 cm depth and 1 g L-1 biomass concentration, it can be estimated that the first cm of the volume absorbs approx. 60% of incident radiation (Figure 1A), whilst most of the remaining is harvested by the second cm of culture, leaving the rest almost in the dark (Formighieri et al., 2012; Perin et al., 2019a).
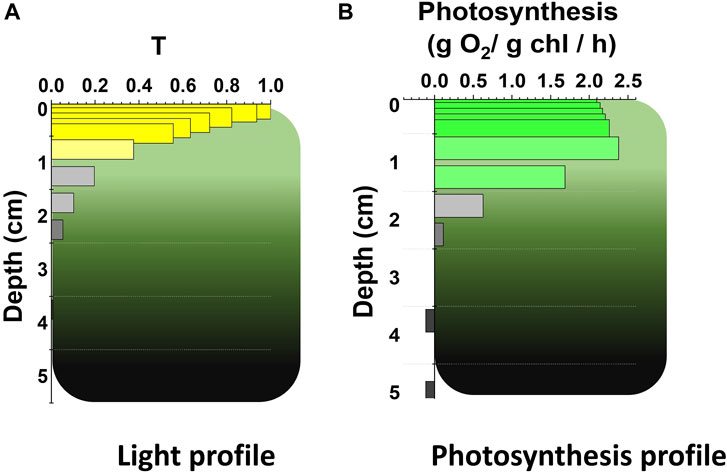
FIGURE 1. Light attenuation profile and effect on photosynthesis in photobioreactor. Quantitative prediction of (A) the light attenuation profile, as transmittance (T, i.e., the ratio between transmitted and incident irradiance) and of (B) the photosynthetic activity, as oxygen evolution (Perin et al., 2015), as a function of the depth of microalgae in photobioreactor (in centimeters, cm). Predictions are based on experimental data from a Nannochloropsis culture at 1 g L−1 concentration in cylindric PBR with 5 cm diameter (Perin et al., 2017b), exposed to 1,500 μmol photons m−2 s−1, using the mathematical model of (Perin et al., 2017a). Nutrients and CO2 were provided in excess to ensure growth was only dependent on PBCE. Cultures were operated in semi-continuous mode and were thus acclimated to the growth conditions.
The peculiar light profile which microalgae are exposed to during cultivation at scale is expected to have major consequences on their photosynthetic metabolism. Quantitative models indeed suggest that the photosynthetic rate of microalgae in dense cultures decreases with the culture’s depth, following the reduction of light intensity (Perin et al., 2019a). The highest photosynthetic rate in the culture is indeed expected in the very first layer of cells that absorb most of the radiation (Figure 1B). However, here cells are exposed to excess light and are therefore experiencing photosynthesis saturation. Excess light is still efficiently absorbed but the fraction not useable for photochemical reactions drives the generation of longer life-time excited chlorophyll states (i.e., triplet state chlorophyll, 3Chl*), that interact with molecular oxygen and generate reactive oxygen species (i.e., ROS; e.g., singlet oxygen, 1O2). ROS can oxidize pigments, proteins and lipids, leading to a broad alteration of the central metabolism. ROS also find in the D1 subunit of photosystem II (PSII) a major target (Kale et al., 2017), and thus have a direct effect in the reduction of photosynthetic functionality. Consequently, in these cells light is efficiently harvested but exploited with low efficiency.
Photosynthesis saturation in these first layers of the culture is expected to be a widespread phenomenon for microalgae cultivated outdoor. As an example, in the eukaryotic microalga Nannochloropsis, photosynthesis saturates approx. at 150 µmol photons m-2 s-1 (Sforza et al., 2012), a value well below intensities reached during full sunlight exposure (i.e. 500-2000 µmol photons m-2 s-1 in temperate climates depending on the season).
It is also worth noting that, even if data originated from specific experiments, the scheme of Figure 1 represents the light distribution profile of any dense industrial microalgae culture, regardless of the species. In fact, despite different microalgae species show a wide variability in absorption spectra, due to differences in the content of Carotenoids, accessory Chlorophylls and Phycobilisomes (in the case of red microalgae), Chl a is the main pigment that always provides the largest contribution to absorption. Furthermore, the eventual spectral differences are likely less relevant in a dense microalgae culture. In fact, the portion of the light spectrum that is less efficiently absorbed (e.g., green wavelengths) by the first layers of cells is eventually absorbed by the deeper, light limited, layers of the mass culture.
While in more external layers cells are saturated, in the deepest layers of the culture light is no longer sufficient for driving a net photosynthetic oxygen evolution and net oxygen is instead consumed by respiration for cell maintenance, curbing the overall photon-to-biomass conversion efficiency (Figure 1B). Here cells do not receive energy to generate enough ATP and NADPH to sustain the Calvin-Benson cycle, which thus limits the CO2 fixation activity of these layers. As these light-limited regions can easily occupy the largest fraction of the culture volume (e.g., >70%, Perin et al., 2017b), they represent a major limitation to biomass productivity of large-scale microalgae cultivation plants. To reduce light-limitation in deeper layers and consequently preventing part of the culture volume to have a null or even negative productivity, the cultures’ depth for microalgae large scale cultivation is often limited and normally it does not go beyond 20 cm (Chisti, 2016). It is worth mentioning that microalgae can also be cultivated in diluted cultures, therefore increasing the amount of light available at the backside and reducing the impact of some of these phenomena. Another possibility to minimize this negative effect is to develop systems with a shorter light path. In the former case, however, low cell density reduces total biomass productivity and increases costs of downstream processing. In the latter case, instead, construction costs increase significantly. Overall, even if in different systems this phenomenon can have different impact, all systems for microalgae cultivation are affected by an inhomogeneous light distribution.
In assessing their growth environment, it should be considered that microalgal cultures are actively mixed to improve CO2 and nutrients supply, with consequences also on light utilization. Because of mixing, in fact, microalgae are forced to move between different layers of the mass culture and thus from regions where light is saturating (more exposed layers) to others where it is limiting (inner layers) and vice versa. Cells therefore experience abrupt changes in irradiance, with a millisecond to seconds timescale, depending on the design of the cultivation system (Carvalho et al., 2011).
Microalgae evolved mechanisms to cope with saturating irradiance and thus increase the chances of survival in a highly variable natural environment, where light intensity often suddenly becomes saturating due to weather conditions. Upon exposure to saturating light, when the protein D1 is damaged by ROS, it is degraded and re-synthesized to restore photosynthetic functionality (Theis and Schroda, 2016). Also carotenoids or tocopherol, that show an antioxidant activity, are accumulated to directly scavenge reactive molecules, like 3Chl* or 1O2 (Rodrigues et al., 2012). Non-photochemical quenching (NPQ) is another photoprotection mechanism that come into play to drive the non-radiative de-excitation of excited chlorophyll (Chl) states, resulting in the dissipation of excess energy as heat. These mechanisms are also expected to be deployed by those cells populating the more exposed regions of large-scale microalgae cultures (Perin et al., 2017a).
Carotenoids are per se important for detoxification of reactive molecular species (e.g., ROS), but are also involved in NPQ activation in microalgae. Zeaxanthin indeed plays a major influence in NPQ in many algal species (Kuczynska et al., 2015). This oxygenated pigment is synthesized from violaxanthin by Violaxanthin De-Epoxidase (VDE), upon exposure to saturating irradiance. Once experiencing limiting light conditions, zeaxanthin is converted back to violaxanthin by Zeaxanthin Epoxidase (ZE), closing the so-called xanthophyll cycle.
Despite all these regulatory mechanisms of photosynthesis have a fundamental role to preserve photosynthetic functionality and consequently the fitness of microalgae cells, they are nevertheless expected to impact photon-to-biomass conversion efficiency too. As an example, the repair mechanism of PSII requires the investment of ATP and NADPH for protein synthesis and can represent a major sink of such metabolic resources in microalgae (Weisz et al., 2019).
Similarly, NPQ activation comes at a cost for the cell. This mechanism evolved to dissipate up to 90% of the absorbed light energy as heat, when irradiance exceeds the photochemical capacity of the cell. While NPQ is seminal to maintain photosynthetic fitness upon exposure to saturating irradiance, it also drives to a reduction in the amount of energy that is channelled to photochemistry, and this could be detrimental for PBCE if NPQ is active when not needed.
How does microalgae photosynthetic light reactions respond to large-scale cultivation?
Microalgae are mostly cultivated outdoors to use sunlight as energy input (Abu-Ghosh et al., 2016) and in these conditions microalgae cultures are exposed to the natural variability of irradiance, which depends on the seasons, the time of the day and the weather conditions.
Microalgae, as all photosynthetic organisms, evolved a sophisticated light-sensing system to monitor light availability and adapt their physiology to light conditions (Kianianmomeni and Hallmann, 2014; Agarwal et al., 2023), but the eventual impact of photoreceptors in sensing light changes upon cultivation in industrial conditions have not been investigated yet. What is more studied are the several mechanisms for the regulation of photosynthesis, that microalgae evolved to respond to natural variations of irradiance, going from Sun flecks, diurnal cycles to seasonal changes, all with different time kinetics (Figure 2).
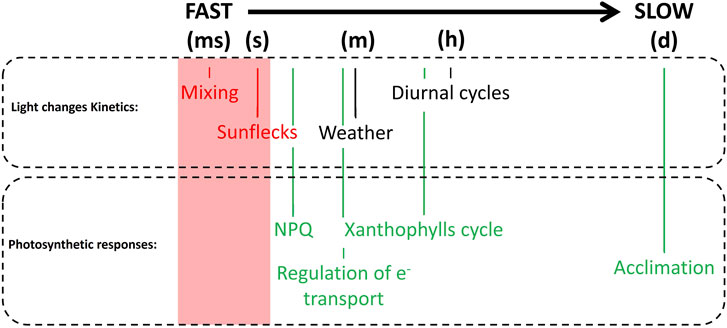
FIGURE 2. Complexity of light conditions impacting microalgae light-use efficiency in photobioreactors outdoors. Light changes are divided according to the speed of their kinetics, from the fastest [from milliseconds (ms) to seconds (s)] to the slowest [from hours (h) to days (d)]. Regulatory mechanisms of photosynthesis are organized according to their kinetics of operation, from the fastest [NPQ, from seconds (s) to minutes (m)], to the slowest (Acclimation). It is worth mentioning that charge separation happens on a faster time-scale than that showed in the figure. This was done intentionally as many of the processes we described within a photobioreactor are relatively slower (ms is already a very fast mixing kinetic).
Seasons and diurnal cycles cause light changes in the timeframe of days-hours and microalgae can adapt their photosynthetic functionality via longer-term regulatory mechanisms. These responses are globally called acclimation, where the modulation of the composition of the photosynthetic machinery (i.e., proteins and other macromolecules (e.g., pigments)) enables the regulation of photosynthetic functionality. In microalgae, as in many other photosynthetic organisms, acclimation involves the reduction of the Chl content, followed by an increased in the content of photoprotective Car and xanthophylls, upon exposure to saturating irradiance. This phenomenon also correlates with an increased photosynthetic capacity for electron transport that are delivered to an increased number of sinks (e.g., enzymes of the Clavin-Benson cycle). While most information on acclimation comes from lab scale experiments so far, it has also been observed that microalgae cultivated outdoors activate an acclimation response to the illumination conditions, suggesting this is a regulatory framework that needs to be considered (Perin et al., 2022).
Light availability can also change with shorter kinetics, e.g., hours-minutes, because of weather conditions and microalgae evolved short-term regulatory mechanisms to enable a faster modulation of photosynthesis, without necessarily involving a large modulation of proteins’ synthesis or degradation (Roach and Krieger-Liszkay, 2019). Short-term mechanisms in fact depend on either macromolecules already present in the cell or pools of molecules rapidly synthesized/degraded upon changes in irradiance.
One mechanism that does not call for synthesis of newly synthesized macromolecules is state transition. The latter involves the translocation in the thylakoid membrane of the antenna complexes upon their phosphorylation/dephosphorylation, for their binding to the core complexes of either of the photosystems to increase or decrease their absorption cross section (Bolychevtseva et al., 2021). Despite state transition was originally described in red and green microalgae more than 50 years ago (Bonaventura and Myers, 1969; Murata, 1969), its impact in microalgae upon cultivation in photobioreactor has not been described in the available literature.
Among the short-term mechanisms, NPQ and the xanthophylls cycle are among the most studied for the protection of PSII (Figure 3), with PSI overreduction instead prevented by the so-called photosynthetic control, that is activated by the proton gradient across the thylakoids’ membrane generated in excess light and leads to the inhibition of the Cytochrome b6f to reduce the flux of electrons reaching this photosystem (Barbato et al., 2020). Cyclic (CEF) and pseudo-cyclic (PCEF) electron flows are also involved in avoiding PSI over-reduction by activating alternative pathways that recycle electrons back to the plastoquinone pool or Oxygen (Shikanai, 2014) (Figure 3), as reviewed in (Shikanai and Yamamoto, 2017; Storti et al., 2019; Ma et al., 2021). NPQ, xanthophylls cycle and regulation of photosynthetic electron transport all have activation kinetics in the range of tens of seconds - minutes and are therefore fast enough to respond to dynamic weather conditions, even when microalgae are cultivated at large-scale (Figure 2).
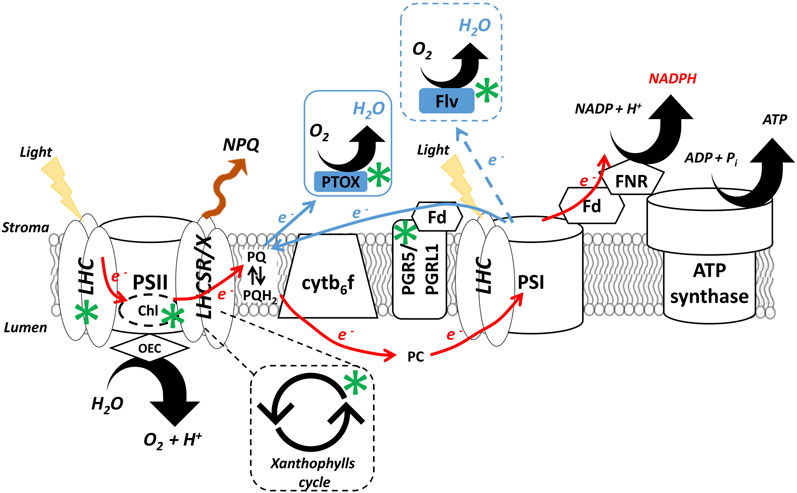
FIGURE 3. Regulatory mechanisms of microalgae photosynthesis and potential targets for improvement. NPQ depends on the activity of light-harvesting complexes stress related (i.e., LHCSR in the green lineage and LHCX in heterokonts), that enable the dissipation of excess energy as heat (red wave). The xanthophylls cycle is indicated by two circular arrows and its kinetics control NPQ induction and relaxation. The linear electron flow (red solid arrows) moves electrons from water to NADP+ and generates both reducing power (i.e., NADPH) and a proton gradient across the thylakoid membrane that is ultimately used to drive ATP synthesis by ATP synthase. The alternative electron pathways (blue solid arrows) include both the PGR5/PGRL1 cyclic electron flow around PSI and the PTOX-mediated water to water cycle. The pseudo-cyclic electron flow instead redirects electrons downstream PSI to water by non-native FLV proteins (dashed blue arrow). Reactions are not balanced. The targets for microalgae photosynthesis improvement in photobioreactors, discussed in this work, are indicated by green asterisks. Cytb6f, cytochrome b6f; Fd, ferredoxin; Flv, flavodiiron protein; FNR, ferredoxin:NADP+ reductase; LHC, Light Harvesting Complexes; OEC, oxygen evolving complex; PC, plastocyanin; PQ, plastoquinone; PGH, 2 plastoquinol; PGR5, proton gradient regulation 5; PGRL1, PGR5-like protein 1; PSI, photosystem I; PSII, photosystem II; PTOX, plastid terminal oxidase. Adapted from (Perin and Morosinotto, 2019a).
The most challenging conditions for regulation of photosynthesis are those, like sunflecks, where excess light exposure is not long enough for the full activation of protection mechanisms (Figure 2). Indeed, exposure of microalgae to light fluctuations of different frequencies showed that the most critical conditions, causing a major growth reduction, are those where light fluctuations are in the 10–100 s range (Bellan et al., 2020; Perin et al., 2022).
In addition to all the natural light dynamics, during large-sale cultivation, microalgae cultures are also actively mixed to: i) ensure optimal gas exchanges and avoid CO2 limitations or oxygen accumulation; ii) guarantee optimal provision of nutrients; iii) improve the average exposure of cells to sunlight (Brennan and Owende, 2010).
One major consequence of mixing is that microalgae abruptly move between different regions of the culture and thus experience drastically different light conditions, introducing one additional source of light dynamics associated with artificial cultivation. As shown in Figure 2, light changes associated with mixing fall in the milliseconds-seconds time frame are and thus highly challenging for an effective activation of regulatory mechanisms of photosynthesis (Eberhard et al., 2008).
As an example, the full activation of photoprotection takes some minutes in Nannochloropsis (Perin et al., 2023) and thus microalgae cells moving from the inner, light-limited, layers of photobioreactors to the most external, light-saturated, regions on a milliseconds/seconds timeframe can experience light damage (Figure 4A).
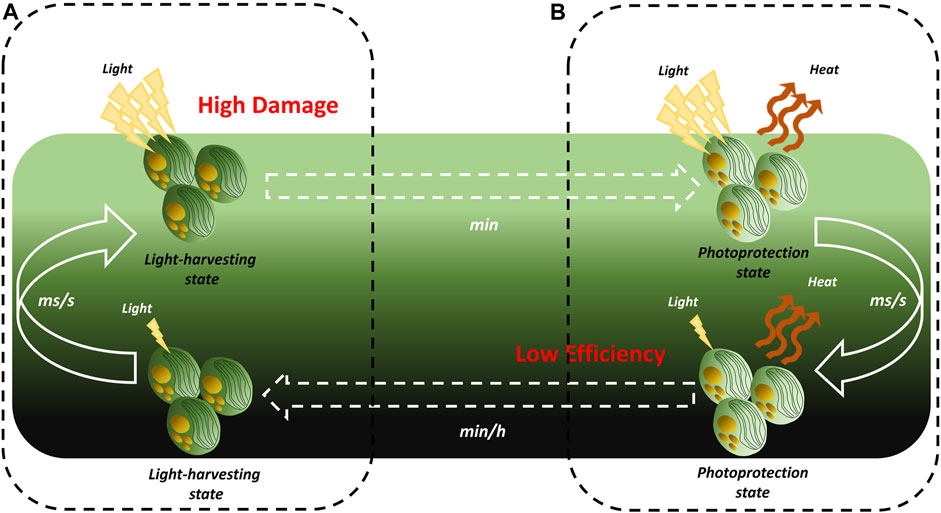
FIGURE 4. Effect of culture mixing on photosynthesis regulation during cultivation in photobioreactors. Microalgae in photobioreactors are mixed faster (milliseconds to seconds timeframe) than the activation/relaxation kinetics of the xanthophylls cycle (minutes to hours). (A) When microalgae move from the inner, light-limited, layers to the external, light-saturated, regions of a PBR they cannot activate photoprotection fast enough and are locked in a light-harvesting state that expose them to high photo-damage (B). When microalgae move in the opposite direction, they cannot relax photoprotection fast enough and are locked in a photoprotective state that lowers their light-use efficiency.
On the other hand, the conversion of zeaxanthin to violaxanthin starts after several minutes of exposure to limiting light and its full completion takes hours (Goss and Jakob, 2010). Therefore, the relaxation of photoprotection in cells moving in the opposite direction (i.e., from the most external to the inner regions of the culture) is not fast enough, locking these cells in a photoprotective state, which keeps dissipating a significant fraction of the absorbed energy as heat even if light is limiting, reducing photosynthetic efficiency (Figure 4B). Given that most microalgae culture volume is light-limited, this inefficient relaxation of photoprotection is expected to be one of the most relevant causes of the low PBCE values of state-of-the-art microalgae cultivation plants.
To maximize PBCE and consequently biomass productivity in this environment, it is therefore seminal to modify the regulatory mechanisms of photosynthesis to optimally balance light-use and photo-protection efficiency for the specific artificial conditions of large-scale, high-density cultures.
Metabolic engineering of photosynthesis to balance light-use and photoprotection efficiencies
In nature, the main objective of a unicellular organism is surviving. During industrial cultivation, the objective is instead the maximization of the growth of the whole population. Consequently, cells should be optimized to have the maximal efficiency, even if this implies additional risk of exposure to strong stress for a fraction of the culture. Since the selection principle is different, it is possible that a change in the balance between light-use efficiency and photoprotection, favouring the former, may be positive.
Metabolic engineering of photosynthesis represents a powerful tool to meet this rationale. Different strategies have been already attempted to domesticate microalgae to the intended cultivation environment, with the objective to optimize those biological traits positively selected during evolution for survival in the natural environment, but detrimental for an optimal light-use vs photoprotection efficiency balance during large-scale cultivation.
As an example, in nature microalgae must harvest as much light as possible and there is no advantage in leaving energy available to other cells. Consequently, microalgae evolved large macromolecular complexes to maximize the number of chlorophyll molecules involved in light-harvesting (i.e., light-harvesting complexes or antenna). During large-scale cultivation, instead, cells should not compete, and each should ideally harvest only enough light to run efficiently photochemistry, leaving energy also to the other cells in the culture.
To address this limitation, the reduction of the Chl content has long been pursued as valuable strategy to improve both i) the light distribution profile within a microalgae dense culture and ii) the saturation limit of photosynthesis (Perin et al., 2014; Sharon-Gojman et al., 2017; Nymark et al., 2019; Kumar et al., 2021). As an example, quantitative models indeed show that in WT microalgae the fraction of inhibited photosynthetic units (PSUs), is larger in the first, and most light exposed, cm of the culture (Figure 5A). A reduction of Chl content, by decreasing the amount of harvested light, it is thus also expected to lower the fraction of inhibited PSUs in the most exposed layers of the culture (Figures 5B, C).
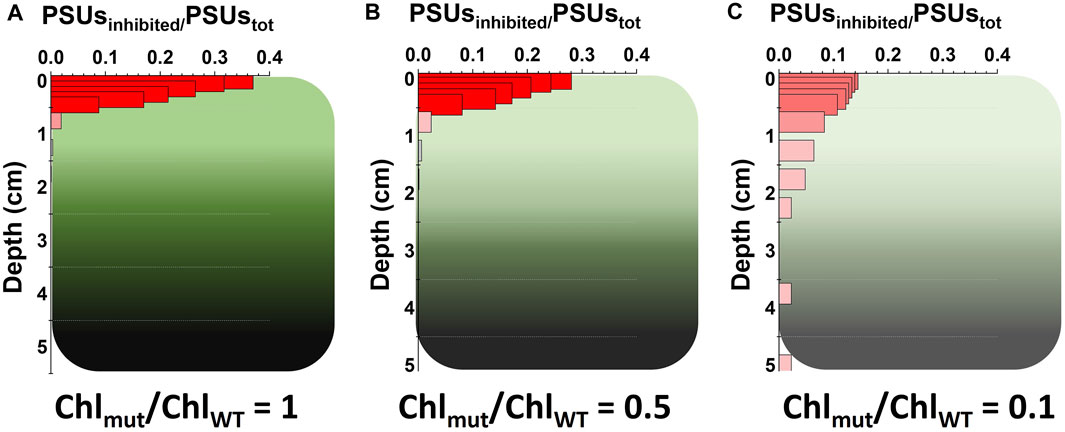
FIGURE 5. Reduction of Chl content and expected impact on photoinhibition in photobioreactors. Quantitative prediction of the photo-inhibition level, expressed as fraction of photo-inhibited PSUs (Photosynthetic Units) out of the total, as function of the PBR depth in centimeters (cm), for the WT strain (A) and for domesticated Nannochloropsis strains with 50% (B) and 90% (C) reduction of the Chl content of the parental strain. Experimental data and modelling approach are the same of Figure 1. These predictions are based on data collected from Nannochloropsis cells, a group of microalgae containing only Chl a and not any other accessory forms of Chl (Perin et al., 2015). Therefore, the 90% reduction refers to a reduction of the content of Chl a. It is worth mentioning that carotenoids content is generally relatively maintained in these strains, namely, with a reduction proportional to that of the Chl a content.
Research efforts in this direction indeed succeeded to achieve both the two objectives discussed above (Figure 3) and led to an improved light-use-efficiency and biomass productivity, demonstrated at least at the lab-scale. These results were confirmed in different microalgae species, validating the robustness of the approach (Cazzaniga et al., 2014; Dall’Osto et al., 2019; Negi et al., 2020; Sengupta et al., 2023).
However, it is worth noting that a substantial reduction of the Chl content is also expected to have an indiscriminate impact on the amount of pigment-binding (i.e., antenna) complexes. In all photosynthetic organisms, different classes of antenna complexes exist and they are not only involved in light harvesting but also in photoprotection and their deletion may thus cause negative consequences.
Another strategy to balance light-use and photoprotection efficiencies of microalgae for an improved PBCE is the optimization of the regulatory mechanisms of photosynthesis to respond more efficiently to the light fluctuation profiles experienced in large-scale outdoor systems (Figure 2). Whilst the reduction of the NPQ activation intensity is expected to have detrimental effects on microalgae fitness, as most exposed layers must preserve their ability to withstand excess irradiation to survive (Perin et al., 2017a), another viable approach is targeting the kinetics of photoprotection.
This strategy, originally demonstrated viable in plants in the field [e.g., tobacco and soybean (Kromdijk et al., 2016; De Souza AP et al., 2022),], has been recently successfully applied also in microalgae (Perin et al., 2023). Speeding up the conversion of zeaxanthin into violaxanthin (Figure 3) was demonstrated to increase microalgae fitness and biomass productivity in dense cultures, likely because cells that move from the most external, light-saturated, layers to the inner, light-limited, regions of photobioreactors can relax photoprotection faster, increasing the channelling of irradiance towards photochemistry.
Another approach involves the optimization of electron transport reactions of photosynthesis, a strategy that has only been hypothesized so far. At difference from PSII, PSI did not evolve efficient repair mechanisms (Tiwari et al., 2016), making it extremely vulnerable to photodamage when the electron transport chain is over-saturated, with major negative consequences on growth (Larosa et al., 2017). Some photosynthetic organisms can modulate their photosynthetic electron transport and protect PSI, with cyclic and pseudo-cyclic contributions playing a major role (Shikanai, 2014).
Despite being found in all photosynthetic organisms, regulatory mechanisms for the control of electron transport are not conserved (Alboresi et al., 2018), opening the possibility of implementation via synthetic biology approaches. As an example, in cyanobacteria, pseudo-cyclic electron transport avoids over-reduction of the electron transport chain in fluctuating light conditions (Yamamoto et al., 2016; Alboresi et al., 2018; Gómez et al., 2018), returning electrons to oxygen. This activity was lost in some eukaryotic microalgae species, and it might represent a promising regulatory mechanism to implement, potentially beneficial to respond to the artificial light fluctuations experienced during large-scale cultivation (Figure 3). However, if not properly regulated, new-to-host alternative electron transports might lead to redirect electrons away from the photochemical reactions, thus reducing light-use ability with possible detrimental consequences on the optimization of the balance between photo-protection and efficiency.
Performance of improved microalgae strains in lab vs. outdoor conditions
Despite more of a decade of research efforts to domesticate the light reactions of photosynthesis in microalgae to boost their PBCE and biomass productivity (Leister, 2023), the improvements currently documented have been only proven at the lab-scale, with mixed results when strains have been tested at higher scales of cultivation. As an example, strains that were isolated for an improved photosynthesis and growth in the lab, once moved to pilot plants, in some cases showed an improved productivity (Cazzaniga et al., 2014), in others no advantage at all (De Mooij et al., 2014). It has indeed been observed that the complexity of the cultivation environment has a major impact on performances of genetically improved strains, with mutants showing improved performances depending on the cultivation conditions (Perin et al., 2017b).
This variability of outcomes is likely largely dependent on the environmental conditions experienced at higher scales of cultivation, which are more complex than those experienced at the lab-scale (Perin and Morosinotto, 2019a) and with an impact on the photosynthetic light-utilization ability that is still under-investigated. The larger the scale of cultivation, the higher the environmental complexity microalgae are exposed to, and the smaller the knowledge currently available of its impact on the photosynthetic metabolism. As shown in the examples in Figure 6, in different systems depending on the size different environmental parameters become effective and impactful.
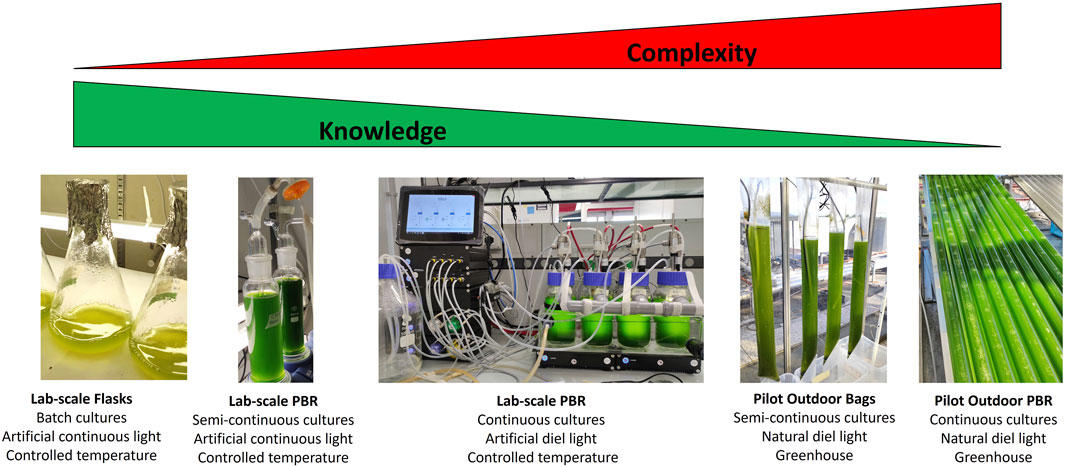
FIGURE 6. Relationship between complexity and knowledge at different scales of microalgae cultivation. Pictures show the different scales of microalgae cultivation platforms from the lab to the pilot scale outdoors (from left to right). The main relevant features of the different scales of cultivation are indicated below each picture. From left to right the different platforms are arranged from the lowest to the highest degree of operational complexity and from the highest to the lowest level of knowledge available on how microalgae photosynthesis responds. This figure has no ambition of being an exhaustive representation of all the different cultivation systems currently existing. For a detailed description of the differences among the available microalgae cultivation systems at scale we recommend the recent literature (Acién et al., 2017).
As an example, microalgae mutants showing a significant reduction of Chl content with respect to the parental strain do not always maintain the differences when cultivated in a more complex environment, such as lab-scale PBRs (Figure 7). Our understanding of the molecular mechanisms behind microalgae acclimation to the cultivation environment is still limited and only through the inclusion of this further layer of biological complexity the scientific community would be capable to design more robust genetic modifications to improve growth at large scale outdoors.
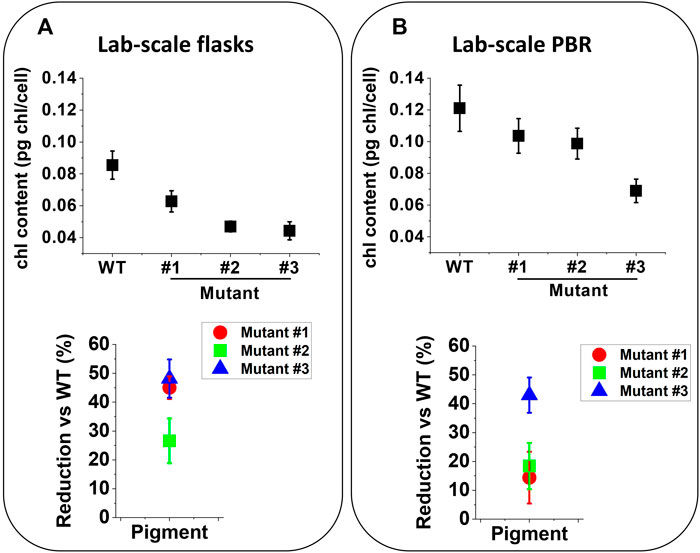
FIGURE 7. Acclimation of optimized microalgae strains to the complexity of the cultivation environment. Chlorophyll (Chl) content of WT and domesticated Nannochloropsis strains isolated with a lower Chl content than the parental strain. Values of Chl content measured in flasks (A) at a low operational complexity are compared to those measured in lab-scale PBRs (B) at a higher operational complexity. The two systems correspond to the first two on the left in Figure 6. Data come from previously published studies, where additional information, including growth rate and biomass productivity can be found (Perin et al., 2017a; Perin et al., 2017b).
One of the main reasons why these important biological responses are understudied is that most of the relevant phenomena affecting microalgae productivity in industrial plants are only measurable at large scale and thus their investigation is very time and resource consuming. Moreover, the identification of the most relevant genetic targets by a trial-and-error approach is ineffective and time consuming. For this reason, tools capable of predicting the performance of genetic modifications in the settings of a large-scale cultivation plant would be highly valuable to make strain improvement faster and more efficient. Mathematical models might come to help to deal with this complexity, enabling the quantification of the impact of each parameter. Several modelling approaches have been already developed (Bernardi et al., 2014; 2017; Darvehei et al., 2018) to include the effect of light as one major environmental parameter and are indeed capable to describe phototrophic microalgae growth. Some models are already enabling the simulation in silico of hypothetical genetic modifications of light reactions of photosynthesis to predict their impact on growth in more complex growth conditions (e.g., from low-complexity flasks to higher-complexity lab-scale PBRs) (Perin et al., 2017a).
However, so far this potentiality has only been validated at the lab scale and before this technology makes a difference at larger scales, typical of PBRs outdoors, models must be improved to account for the effect of photo-acclimation and to include a comprehensive description of the effect on photosynthesis of the highly dynamic natural and artificial light fluctuations that microalgae are exposed to during large scale growth. The inclusion of more input variables is therefore expected to increase the value of these modelling approaches and improve their reliability for outdoor predictions (Perin et al., 2019a), potentially largely increasing the impact of these tools.
Conclusion
Microalgae cultivated at large-scale experience a complex mixture of natural and artificial fluctuations of irradiance that the regulatory mechanisms of photosynthesis are only partially able to respond to. This partial absence of coordination between regulatory mechanisms of photosynthesis and light dynamics in PBRs is responsible for energy losses that contribute to curb photon-to-biomass conversion efficiency and biomass productivity.
A deeper understanding of the complexity of the growth environment in outdoor large-scale systems and its impact on the light reactions of photosynthesis in microalgae is essential to close the current gap between lab- and PBRs-scale growth performances and to translate more effectively the potential of genetic improvements of light reactions of photosynthesis to a relevant scale of cultivation for microalgae industrial applications.
Quantitative models represent a powerful tool to achieve this objective and quantify the impact of genetic modifications identifying the ones with the largest impact on biomass productivity at large-scale outdoors. The mathematical description of photo-acclimation as well as the inclusion of the effect of the highly dynamic natural and artificial fluctuations of irradiance to which microalgae are exposed to in PBRs will be seminal for success.
Author contributions
GP: Conceptualization, Writing–original draft. TM: Conceptualization, Writing–review and editing.
Funding
The author(s) declare financial support was received for the research, authorship, and/or publication of this article. TM acknowledges the support from European Union H2020 Project 862087-GAIN4CROPS and Marie Skłodowska-Curie No. 955520 Digitalgae. GP acknowledges the support from the University of Padova STARS Project WWBiomass.
Conflict of interest
The authors declare that the research was conducted in the absence of any commercial or financial relationships that could be construed as a potential conflict of interest.
Publisher’s note
All claims expressed in this article are solely those of the authors and do not necessarily represent those of their affiliated organizations, or those of the publisher, the editors and the reviewers. Any product that may be evaluated in this article, or claim that may be made by its manufacturer, is not guaranteed or endorsed by the publisher.
References
Abu-Ghosh, S., Fixler, D., Dubinsky, Z., and Iluz, D. (2016). Flashing light in microalgae biotechnology. Bioresour. Technol. 203, 357–363. doi:10.1016/j.biortech.2015.12.057
Acién, F. G., Molina, E., Reis, A., Torzillo, G., Zittelli, G. C., Sepúlveda, C., et al. (2017). “1 - photobioreactors for the production of microalgae,” in Microalgae-based biofuels and bioproducts woodhead publishing series in energy. Editors C. Gonzalez-Fernandez, and R. Muñoz (Sawston, United Kingdom: Woodhead Publishing), 1–44. doi:10.1016/B978-0-08-101023-5.00001-7
Agarwal, A., Levitan, O., Cruz de Carvalho, H., and Falkowski, P. G. (2023). Light-dependent signal transduction in the marine diatom Phaeodactylum tricornutum. Proc. Natl. Acad. Sci. 120, e2216286120. doi:10.1073/pnas.2216286120
Alboresi, A., Storti, M., and Morosinotto, T. (2018). Balancing protection and efficiency in the regulation of photosynthetic electron transport across plant evolution. New phytologist 221, 105–109. doi:10.1111/nph.15372
Barbato, R., Tadini, L., Cannata, R., Peracchio, C., Jeran, N., Alboresi, A., et al. (2020). Higher order photoprotection mutants reveal the importance of ΔpH-dependent photosynthesis-control in preventing light induced damage to both photosystem II and photosystem I. Sci. Rep. 10, 6770–6814. doi:10.1038/s41598-020-62717-1
Bellan, A., Bucci, F., Perin, G., Alboresi, A., and Morosinotto, T. (2020). Photosynthesis regulation in response to fluctuating light in the secondary endosymbiont alga nannochloropsis gaditana. Plant Cell. Physiology 61, 41–52. doi:10.1093/pcp/pcz174
Bernardi, A., Nikolaou, A., Meneghesso, A., Chachuat, B., Morosinotto, T., and Bezzo, F. (2017). Semi-empirical modeling of microalgae photosynthesis in different acclimation states – application to N. gaditana. J. Biotechnol. 259, 63–72. doi:10.1016/j.jbiotec.2017.08.002
Bernardi, A., Perin, G., Sforza, E., Galvanin, F., Morosinotto, T., and Bezzo, F. (2014). An identifiable state model to describe light intensity influence on microalgae growth. Industrial Eng. Chem. Res. 53, 6738–6749. doi:10.1021/ie500523z
Bilal, M., Rasheed, T., Ahmed, I., Iqbal, H. M. N., and Sada, E. G. (2017). High-value compounds from microalgae with industrial exploitability – a review. Front. Biosci. 9, 319–342. doi:10.2741/s490
Bolychevtseva, Y. V., Tropin, I. V., and Stadnichuk, I. N. (2021). State 1 and state 2 in photosynthetic apparatus of red microalgae and cyanobacteria. Biochem. Mosc. 86, 1181–1191. doi:10.1134/S0006297921100023
Bonaventura, C., and Myers, J. (1969). Fluorescence and oxygen evolution from Chlorella pyrenoidosa. Biochim. Biophys. Acta 189, 366–383. doi:10.1016/0005-2728(69)90168-6
Brennan, L., and Owende, P. (2010). Biofuels from microalgae—a review of technologies for production, processing, and extractions of biofuels and co-products. Renew. Sustain. Energy Rev. 14, 557–577. doi:10.1016/j.rser.2009.10.009
Carvalho, A. P., Silva, S. O., Baptista, J. M., and Malcata, F. X. (2011). Light requirements in microalgal photobioreactors: an overview of biophotonic aspects. Appl. Microbiol. Biotechnol. 89, 1275–1288. doi:10.1007/s00253-010-3047-8
Cazzaniga, S., Dall’Osto, L., Szaub, J., Scibilia, L., Ballottari, M., Purton, S., et al. (2014). Domestication of the green alga Chlorella sorokiniana: reduction of antenna size improves light-use efficiency in a photobioreactor. Biotechnol. biofuels 7, 157. doi:10.1186/s13068-014-0157-z
Chisti, Y. (2016). Large-scale production of algal biomass: raceway ponds. Algae Biotechnol. 58, 21–40. doi:10.1007/978-3-319-12334-9_2
Dall’Osto, L., Cazzaniga, S., Guardini, Z., Barera, S., Benedetti, M., Mannino, G., et al. (2019). Combined resistance to oxidative stress and reduced antenna size enhance light-to-biomass conversion efficiency in Chlorella vulgaris cultures. Biotechnol. Biofuels 12, 221. doi:10.1186/s13068-019-1566-9
Darvehei, P., Bahri, P. A., and Moheimani, N. R. (2018). Model development for the growth of microalgae: a review. Renew. Sustain. Energy Rev. 97, 233–258. doi:10.1016/j.rser.2018.08.027
De Mooij, T., Janssen, M., Cerezo-Chinarro, O., Mussgnug, J. H., Kruse, O., Ballottari, M., et al. (2014). Antenna size reduction as a strategy to increase biomass productivity: a great potential not yet realized. J. Appl. Phycol. 27, 1063–1077. doi:10.1007/s10811-014-0427-y
De Souza, A. P., Burgess, S. J., Doran, L., Hansen, J., Manukyan, L., Gotarkar, D., et al. (2022). Response to Comments on "Soybean photosynthesis and crop yield is improved by accelerating recovery from photoprotection". Science 379, eadf2189. doi:10.1126/science.adf2189
De Vree, J. H., Bosma, R., Janssen, M., Barbosa, M. J., and Wijffels, R. H. (2015). Comparison of four outdoor pilot-scale photobioreactors. Biotechnol. Biofuels 8, 215–312. doi:10.1186/s13068-015-0400-2
Eberhard, S., Finazzi, G., and Wollman, F. A. (2008). The dynamics of photosynthesis. Annu. Rev. Genet. 42, 463–515. doi:10.1146/annurev.genet.42.110807.091452
Formighieri, C., Franck, F., and Bassi, R. (2012). Regulation of the pigment optical density of an algal cell: filling the gap between photosynthetic productivity in the laboratory and in mass culture. J. Biotechnol. 162, 115–123. doi:10.1016/j.jbiotec.2012.02.021
Gies, E. (2017). The real cost of energy. Nature 551, S145-S147–S147. doi:10.1038/d41586-017-07510-3
Gómez, R., Carrillo, N., Morelli, M. P., Tula, S., Shahinnia, F., Hajirezaei, M. R., et al. (2018). Faster photosynthetic induction in tobacco by expressing cyanobacterial flavodiiron proteins in chloroplasts. Photosynth. Res. 136, 129–138. doi:10.1007/s11120-017-0449-9
Goss, R., and Jakob, T. (2010). Regulation and function of xanthophyll cycle-dependent photoprotection in algae. Photosynth. Res. 106, 103–122. doi:10.1007/s11120-010-9536-x
Kale, R., Hebert, A. E., Frankel, L. K., Sallans, L., Bricker, T. M., and Pospíšil, P. (2017). Amino acid oxidation of the D1 and D2 proteins by oxygen radicals during photoinhibition of Photosystem II. Proc. Natl. Acad. Sci. U. S. A. 114, 2988–2993. doi:10.1073/pnas.1618922114
Kianianmomeni, A., and Hallmann, A. (2014). Algal photoreceptors: in vivo functions and potential applications. Planta 239, 1–26. doi:10.1007/s00425-013-1962-5
Koutra, E., Economou, C. N., Tsafrakidou, P., and Kornaros, M. (2018). Bio-based products from microalgae cultivated in digestates. Trends Biotechnol. 36, 819–833. doi:10.1016/j.tibtech.2018.02.015
Kromdijk, J., Głowacka, K., Leonelli, L., Gabilly, S. T., Iwai, M., Niyogi, K. K., et al. (2016). Improving photosynthesis and crop productivity by accelerating recovery from photoprotection. Sci. (New York, N.Y.) 354, 857–861. doi:10.1126/science.aai8878
Kuczynska, P., Jemiola-Rzeminska, M., and Strzalka, K. (2015). Photosynthetic pigments in diatoms. Mar. drugs 13, 5847–5881. doi:10.3390/md13095847
Kumar, V., Sharma, N., Jaiswal, K. K., Vlaskin, M. S., Nanda, M., Tripathi, M. K., et al. (2021). Microalgae with a truncated light-harvesting antenna to maximize photosynthetic efficiency and biomass productivity: recent advances and current challenges. Process Biochem. 104, 83–91. doi:10.1016/j.procbio.2021.03.006
Lam, G. P., Vermuë, M. H., Eppink, M. H. M., Wijffels, R. H., and Berg, C. V. D. (2018). Multi-product microalgae biorefineries: from concept towards reality. Trends Biotechnol. 36, 216–227. doi:10.1016/j.tibtech.2017.10.011
Larosa, V., Meneghesso, A., La Rocca, N., Steinbeck, J., Hippler, M., Szabo, I., et al. (2017). Mitochondria affect photosynthetic electron transport and photo-sensitivity in a green alga. Plant physiol. 176, 2305–2314. doi:10.1104/pp.17.01249
Leister, D. (2023). Enhancing the light reactions of photosynthesis: strategies, controversies, and perspectives. Mol. Plant 16, 4–22. doi:10.1016/j.molp.2022.08.005
Lynch, J., Cain, M., Frame, D., and Pierrehumbert, R. (2021). Agriculture’s contribution to climate change and role in mitigation is distinct from predominantly fossil CO2-emitting sectors. Front. Sustain. Food Syst. 4, 300. doi:10.3389/fsufs.2020.518039
Ma, M., Liu, Y., Bai, C., Yang, Y., Sun, Z., Liu, X., et al. (2021). The physiological functionality of PGR5/PGRL1-dependent cyclic electron transport in sustaining photosynthesis. Front. Plant Sci. 12, 702196. doi:10.3389/fpls.2021.702196
Maeda, Y., Yoshino, T., Matsunaga, T., Matsumoto, M., and Tanaka, T. (2018). Marine microalgae for production of biofuels and chemicals. Curr. Opin. Biotechnol. 50, 111–120. doi:10.1016/j.copbio.2017.11.018
Melis, A. (2009). Solar energy conversion efficiencies in photosynthesis: minimizing the chlorophyll antennae to maximize efficiency. Plant Sci. 177, 272–280. doi:10.1016/j.plantsci.2009.06.005
Murata, N. (1969). Control of excitation transfer in photosynthesis. I. Light-induced change of chlorophyll a fluorescence in Porphyridium cruentum. Biochimica Biophysica Acta (BBA) - Bioenergetics 172, 242–251. doi:10.1016/0005-2728(69)90067-X
Negi, S., Perrine, Z., Friedland, N., Kumar, A., Tokutsu, R., Minagawa, J., et al. (2020). Light regulation of light-harvesting antenna size substantially enhances photosynthetic efficiency and biomass yield in green algae. Plant J. 103, 584–603. doi:10.1111/TPJ.14751
Ng, I., Tan, S., Kao, P., Chang, Y., and Chang, J. (2017). Recent developments on genetic engineering of microalgae for biofuels and bio-based chemicals. Biotechnol. J. 12, 1600644. doi:10.1002/biot.201600644
Nymark, M., Volpe, C., Hafskjold, M. C. G., Kirst, H., Serif, M., Vadstein, O., et al. (2019). Loss of ALBINO3b insertase results in truncated light-harvesting antenna in diatoms. Plant Physiol. 181, 1257–1276. doi:10.1104/pp.19.00868
Perin, G., Bellan, A., Bernardi, A., Bezzo, F., and Morosinotto, T. (2019a). The potential of quantitative models to improve microalgae photosynthetic efficiency. Physiol. Plant. 166, 380–391. doi:10.1111/ppl.12915
Perin, G., Bellan, A., Michelberger, T., Lyska, D., Wakao, S., Niyogi, K. K., et al. (2023). Modulation of xanthophyll cycle impacts biomass productivity in the marine microalga Nannochloropsis. Proc. Natl. Acad. Sci. U. S. A. 120, e2214119120. doi:10.1073/pnas.2214119120
Perin, G., Bellan, A., Segalla, A., Meneghesso, A., Alboresi, A., and Morosinotto, T. (2015). Generation of random mutants to improve light-use efficiency of Nannochloropsis gaditana cultures for biofuel production. Biotechnol. biofuels 8, 161. doi:10.1186/s13068-015-0337-5
Perin, G., Bernardi, A., Bellan, A., Bezzo, F., and Morosinotto, T. (2017a). A mathematical model to guide genetic engineering of photosynthetic metabolism. Metab. Eng. 44, 337–347. doi:10.1016/j.ymben.2017.11.002
Perin, G., Gambaro, F., and Morosinotto, T. (2022). Knowledge of regulation of photosynthesis in outdoor microalgae cultures is essential for the optimization of biomass productivity. Front. plant Sci. 13, 846496. doi:10.3389/FPLS.2022.846496
Perin, G., and Jones, P. R. (2019). Economic feasibility and long-term sustainability criteria on the path to enable a transition from fossil fuels to biofuels. Curr. Opin. Biotechnol. 57, 175–182. doi:10.1016/j.copbio.2019.04.004
Perin, G., and Morosinotto, T. (2019a). “Optimization of microalgae photosynthetic metabolism to close the gap with potential productivity,” in Grand challenges in biology and biotechnology (Dordrecht, NL: Springer Cham), 223–248. doi:10.1007/978-3-030-25233-5_6
Perin, G., and Morosinotto, T. (2019b). Potential of microalgae biomass for the sustainable production of bio-commodities. Cham: Springer, 243–276. doi:10.1007/124_2019_30
Perin, G., Segalla, A., Basso, S., Simionato, D., Meneghesso, A., Sforza, E., et al. (2014). Biotechnological optimization of light use efficiency in nannochloropsis cultures for biodiesel production. Chem. Eng. Trans. 37, 763–768. doi:10.3303/CET1437128
Perin, G., Simionato, D., Bellan, A., Carone, M., Occhipinti, A., Maffei, M. E., et al. (2017b). Cultivation in industrially relevant conditions has a strong influence on biological properties and performances of Nannochloropsis gaditana genetically modified strains. Algal Res. 28, 88–99. doi:10.1016/j.algal.2017.10.013
Perin, G., Yunus, I. S., Valton, M., Alobwede, E., and Jones, P. R. (2019b). Sunlight-driven recycling to increase nutrient use-efficiency in agriculture. Algal Res. 41, 101554. doi:10.1016/j.algal.2019.101554
Renuka, N., Guldhe, A., Prasanna, R., Singh, P., and Bux, F. (2018). Microalgae as multi-functional options in modern agriculture: current trends, prospects and challenges. Biotechnol. Adv. 36, 1255–1273. doi:10.1016/j.biotechadv.2018.04.004
Roach, T., and Krieger-Liszkay, A. (2019). Photosynthetic regulatory mechanisms for efficiency and prevention of photo-oxidative stress. Annu. Plant Rev. Online 2, 273–306. doi:10.1002/9781119312994.APR0666
Rodrigues, E., Mariutti, L. R. B., and Mercadante, A. Z. (2012). Scavenging capacity of marine carotenoids against reactive oxygen and nitrogen species in a membrane-mimicking system. Mar. Drugs 10, 1784–1798. doi:10.3390/md10081784
Rulli, M. C., Bellomi, D., Cazzoli, A., Carolis, G. D., and Odorico, P. D. (2016). The water-land-food nexus of first-generation biofuels. London, United Kingdom: Springer Nature, 1–10. doi:10.1038/srep22521
Sengupta, A., Bandyopadhyay, A., Schubert, M. G., Church, G. M., and Pakrasi, H. B. (2023). Antenna modification in a fast-growing cyanobacterium synechococcus elongatus UTEX 2973 leads to improved efficiency and carbon-neutral productivity. Microbiol. Spectr. 11, e0050023–23. doi:10.1128/spectrum.00500-23
Sforza, E., Simionato, D., Giacometti, G. M., Bertucco, A., and Morosinotto, T. (2012). Adjusted light and dark cycles can optimize photosynthetic efficiency in algae growing in photobioreactors. PloS one 7, e38975. doi:10.1371/journal.pone.0038975
Sharon-Gojman, R., Leu, S., and Zarka, A. (2017). Antenna size reduction and altered division cycles in self-cloned, marker-free genetically modified strains of Haematococcus pluvialis. Algal Res. 28, 172–183. doi:10.1016/J.ALGAL.2017.09.015
Shikanai, T. (2014). Central role of cyclic electron transport around photosystem I in the regulation of photosynthesis. Curr. Opin. Biotechnol. 26, 25–30. doi:10.1016/j.copbio.2013.08.012
Shikanai, T., and Yamamoto, H. (2017). Contribution of cyclic and pseudo-cyclic electron transport to the formation of proton motive force in chloroplasts. Mol. plant 10, 20–29. doi:10.1016/j.molp.2016.08.004
Storti, M., Alboresi, A., Gerotto, C., Aro, E. M., Finazzi, G., and Morosinotto, T. (2019). Role of cyclic and pseudo-cyclic electron transport in response to dynamic light changes in Physcomitrella patens. Plant, Cell. & Environ. 42, 1590–1602. doi:10.1111/pce.13493
Theis, J., and Schroda, M. (2016). Revisiting the photosystem II repair cycle. Plant Signal Behav. 11, e1218587. doi:10.1080/15592324.2016.1218587
Tiwari, A., Mamedov, F., Grieco, M., Suorsa, M., Jajoo, A., Styring, S., et al. (2016). Photodamage of iron–sulphur clusters in photosystem I induces non-photochemical energy dissipation. Nat. Plants 2, 16035. doi:10.1038/nplants.2016.35
Walsh, B. J., Rydzak, F., Palazzo, A., Kraxner, F., Herrero, M., Schenk, P. M., et al. (2015). New feed sources key to ambitious climate targets. Carbon Balance Manag. 10, 26. doi:10.1186/s13021-015-0040-7
Weisz, D. A., Johnson, V. M., Niedzwiedzki, D. M., Shinn, M. K., Liu, H., Klitzke, C. F., et al. (2019). A novel chlorophyll protein complex in the repair cycle of photosystem II. Proc. Natl. Acad. Sci. 116, 21907–21913. doi:10.1073/pnas.1909644116
Keywords: microalgae, photosynthesis regulation, photobioreactor, biomass, genetic engineering
Citation: Perin G and Morosinotto T (2023) Understanding regulation in complex environments: a route to enhance photosynthetic light-reactions in microalgae photobioreactors. Front. Photobiol. 1:1274525. doi: 10.3389/fphbi.2023.1274525
Received: 09 August 2023; Accepted: 27 October 2023;
Published: 10 November 2023.
Edited by:
Eduardo Jacob-Lopes, Federal University of Santa Maria, BrazilReviewed by:
Marcel Janssen, Wageningen University and Research, NetherlandsIskander M. Ibrahim, Towson University, United States
Copyright © 2023 Perin and Morosinotto. This is an open-access article distributed under the terms of the Creative Commons Attribution License (CC BY). The use, distribution or reproduction in other forums is permitted, provided the original author(s) and the copyright owner(s) are credited and that the original publication in this journal is cited, in accordance with accepted academic practice. No use, distribution or reproduction is permitted which does not comply with these terms.
*Correspondence: Tomas Morosinotto, tomas.morosinotto@unipd.it