Cluster decays of 12Be excited states
- 1Ruđer Bošković Institute, Zagreb, Croatia
- 2University of Birmingham, Birmingham, United Kingdom
- 3TRIUMF, Vancouver, BC, Canada
- 4University of Edinburgh, Edinburgh, United Kingdom
- 5INFN Laboratori Nazionali del Sud, Catania, Italy
- 6McMaster University, Hamilton, ON, Canada
An experimental study of the helium-cluster decays of the 12Be excited states has been performed using the 9Li + 7Li → α + α + 8He and 9Li + 7Li → α + 6He + 6He reactions at the 9Li beam energy of 74.8 MeV. At excitation energies between 10 and 25 MeV, the 12Be excited states decaying into the 4He + 8He, 6He + 6He, and 6He + 6He*(1.8 MeV, Jπ = 2+) have been observed. Most of the observed states decaying into the 4He + 8He and 6He + 6He correspond to previously reported states. The decay to the 6He + 6He*(1.8 MeV, Jπ = 2+) channel has been observed for the first time. Two of the five states observed in this new decay channel are also observed in the ground state channel 6He + 6He, while two may correspond to the 4He + 8He decaying states. The states around 13.5 and 20.0 MeV decay to the 6He + 6He and 4He + 8He channels, while the state around 22.3 MeV decays into all three decay channels examined. This experiment is complementary to all previous studies of the 12Be cluster states because of the different reaction mechanisms used for the population of the 12Be cluster states. The observation of the states at high excitations with these exotic decay properties strongly supports the molecular structure of the 12Be excited states.
1 Introduction
Detailed data on the properties of nuclei away from the stability line are essential to improve the understanding of nuclear structure and reaction mechanisms. In light nuclei, both aspects of nuclear structure, single-particle dynamics, and nucleon correlations, which result in clustering, are the most pronounced due to the small number of important degrees of freedom. Understanding the correlations and formation of clusters is closely related to fine details of the nuclear force as well as to spatial and quantum symmetries within the nuclei. Modern nuclear theories are able to realistically model A
The key nuclei to understand α-clustering are beryllium and carbon isotopes. The 8Be and 12C low excitation level schemes correspond to ones for an assembly of two and three α-particles, respectively. The structure of heavier beryllium and carbon isotopes, as well as the effects of additional neutrons to these cluster structures is currently not fully understood. Nuclear molecules are one of the quite exotic structural phenomena discovered away from the stability line [2, 3]. A nuclear molecule is a system built from α-clusters and valence neutrons residing in the orbitals which can be constructed as linear combinations of orbitals around the individual α-particles. The covalent exchange of the neutrons between the α-cores increases the stability of the system, similar to the binding of covalent atomic molecules. The two-center nuclear molecular structures have been identified in 9Be [4–6] and 10Be [7–9]. To establish the nuclear molecule as a general type of structure in neutron-rich nuclei, it is essential to experimentally confirm it in heavier beryllium nuclei, particularly in the 12Be nucleus in which four valence neutrons may occupy shell model orbitals, atomic-like orbitals around individual α-core, or molecular-like orbitals around both α-cores [10].
The experimental results on the cluster decays of the 12Be excited states are very limited and often inconsistent. The earliest measurements of the 12Be projectile inelastic breakup [11–14] provided evidence for a number of states decaying into the 4He + 8He and 6He + 6He clusters. The estimated spins of some of the observed states indicated that they are members of a rotational band of a deformed, exotic 6He + 6He cluster structure in 12Be, which may be linked to an α-4n-α molecule [12, 13]. This molecular rotational band was not confirmed in later measurement of the 12Be inelastic breakup at higher beam energy [15]. The excitation energy spectra for both helium-cluster decay channels obtained in this work show fewer structures than the ones in [12, 13] with a large background of unclear origin. Both experiments used a composite CH2 target and events originating from different target components were not fully distinguished. This introduced a large uncertainty in the reconstructed 12Be excitation energy spectra in both of these experiments. Although spectra for the assumed reaction on the hydrogen show structures identified as the 12Be excited states, spectra for the reaction on carbon are largely structureless. The most recent measurement of the 12Be breakup off the 12C target [16, 17] at the beam energy similar to one in [12] identified new states close to each helium-cluster decay threshold and provided results in agreement with [12, 13] in the overlapping low excitation energy region. These results are interpreted as confirmation of the proposed molecular rotational band and evidence for a well-developed cluster structure in 12Be. Another experimental approach used to examine the cluster structure of the 12Be excited states is the measurement of the 8He + 4He resonant scattering using a radioactive 8He beam on a thick 4He gas target [18]. The obtained results did not improve the understanding of the 12Be cluster structure as no sharp resonances were observed in the reconstructed 12Be excitation energy spectra for both 8He + 4He and 6He + 6He decay channels in the measured excitation energy range from 13.7 to 16.4 MeV.
From the theoretical side, the structure of the 12Be excited states above the thresholds for their decay into the 4He + 8He (8.9 MeV), 6He + 6He (10.1 MeV), and 5He + 7He (12.4 MeV) has attracted significant interest as an excellent candidate to characterize the evolution of exotic clustering with the addition of neutrons. Some of the recent studies use the no-core shell model (NCSH) [19], the generalized two-center cluster model (GTCM) [10, 20–22], the microscopic generator-coordinate method and complemented NCSM calculations [23], and the antisymmetrized molecular dynamic (AMD) model [24]. The common result of all of these calculations is a well-developed cluster structure with states possessing the 4He + 8He and 6He + 6He structure and grouped into various rotational bands. Another important result is the stabilization of the classical α-cluster structure by excess neutrons. These exotic clustering modes, together with the breakdown of the N = 8 shell closure required to understand the structure of the ground and low-lying excited states [25, 26], set the 12Be nucleus as a key neutron-rich system to benchmark newly developed theoretical models, and improve the knowledge of nuclear structure.
This study reports the results of the experiment using an approach different from all previous studies of clustering in 12Be. This method, resonant particle spectroscopy measurements of many-nucleon or cluster transfer reactions, was successfully applied in earlier research on the clustering in light nuclei close to the stability line. For example, it was applied in previous studies of clustering in 10Be using the 7Li + 7Li → α + α + 6He reaction, which led to the first observation of the 6He decay of the 10Be excited states [9]. The 7Li nucleus has prominent α + t cluster structure in the ground state (Jπ = 3/2−) with clusters being in l = 1 relative motion, making it a perfect system for the triton transfer reactions. Two additional neutrons in the 9Li reduce clustering of its ground state (Jπ = 3/2−) compared to the 7Li. The overall 9Li + 7Li system has large excess of neutrons (N/Z = 5/3) making this reaction suitable for the study of the neutron-rich light nuclei. In addition, the reaction 9Li + 7Li → α + 12Be has a large positive Q-value of 12.36 MeV, making it especially suitable for the study of excited states at high 12Be excitations. This experiment is complementary to all previous studies of the 12Be cluster states since it uses the different reaction mechanisms to populate the 12Be cluster states: the expected main reaction mechanisms are triton transfer from 7Li to 9Li and t + 2n transfer from 9Li to 7Li. These transfer reactions may populate exotic molecular structures such as the ones proposed in [20].
2 Experimental details and data analysis
The experiment was performed at the ISAC-II rare-isotope ion beam facility at TRIUMF in Vancouver using the 9Li beam of energy of 74.8 MeV and LiF targets of thickness of ≈ 1 mg/cm2. The average beam intensity during the 6 days of the measurement was ≈4 × 106 pps. No beam contaminants were observed in the recorded data. The beam was focused through a set of collimators resulting in the spot size on the target of
The reaction products were detected using a large solid angle silicon strip detector array assembled of six wedge-shaped telescopes, each having a ΔE detector of thickness ≈70 μm and E detector of thickness ≈1.5 mm, arranged in a “lampshade” geometry which covered an azimuthal angle of 360° (Figure 1). Both ΔE and E detectors were single-sided strip detectors with 16 circular strips of 5 mm wide separated by 100 μm of SiO2, specifically YY1 design made by Micron Semiconductor Ltd. [27]. The polar angular range covered by the detector array was from 16.6° to 47.8° with an angular resolution of ≈2°. The resolution in the azimuthal angle was
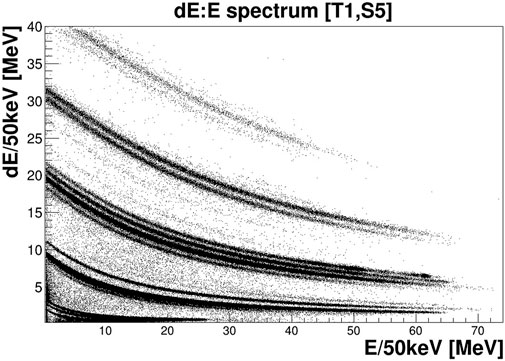
FIGURE 2. Typical particle identification of ΔE–E spectrum obtained for one strip in the E detector: here, the spectrum for strip 5 of telescope T1 is shown. Observed loci correspond to the (from bottom to top) 1,2,3H, 3,4,6,8He, 6,7,8,9Li, 7,9,10,11,12Be, and 10,11,12B.
The kinematically complete measurements of the following reactions were performed to examine the helium-cluster decays of the 12Be excited states:
The reaction products were detected in coincidence events and each detected product was identified using graphical cuts imposed on the ΔE–E plots such as the one presented in Figure 2. Coincident detection of two out of the three reaction products allows the reaction exit channel identification and consequently a full kinematical reconstruction of the event by the application of momentum and energy conservation. From the measured energies in the ΔE and E detectors for each identified nucleus, its energy in the reaction was reconstructed accounting for all possible energy losses in the target and inactive layers of the detectors. A detailed hit reconstruction was also performed by taking into account the geometrical overlap of the strips in the ΔE and E detectors, as well as the signal sharing between two adjacent strips in the detectors. A careful reconstruction of double hits in adjacent strips was also performed in the data analysis. The calibration, fine-tuning of the detector geometry, and hit reconstruction procedure were verified using the elastic and inelastic scattering of the 9Li beam on 7Li and 19F target components, elastic scattering on 27Al target backing and 184W target contaminant, and the 1H(9Li,4He6He) reaction on the hydrogen target contaminant. The double hit procedure in the case of hits in adjacent and separated strips was verified by reconstructing the 8Be ground state for two detected α-particles in the same detector. All reconstructed excitation energy spectra confirmed that the applied procedures produced correct results and that the experimental setup was stable during all data taking time [29]. The stability of the electronics was validated by location in the raw ADC data of constant amplitude signal generated in a pulser during the full data taking time. As an example, the 19F + 9Li elastic scattering peak for the whole detector array and for all recorded data is at correct energy and has a FWHM of 650 keV. For the same set of data, the 7Li + 9Li elastic scattering peak is at correct energy and has a FWHM of 1.3 MeV. The large FWHM value is due to unresolved contribution of the first excited state of 7Li at 478 keV. The excited states of the 19F [30] and 7Li [31] at low excitations were also correctly reconstructed. Another illustration of the data quality is the 8Be ground state reconstructed from the set of data of triple coincidence events 4He + 4He + 6He detected in the whole detector array and for all taken data which is positioned at the correct energy and has an experimental FWHM of 70 keV.
The reconstructed energy and angular position of the two detected and identified reaction products, under the assumption of the mass of the third, undetected nucleus, determine the energy and emission angle of the third reaction product. The sum of energies of all reaction products is equal to the total energy in the reaction exit channel, from which the reaction Q-value can be calculated. The reaction Q-value was calculated for each recorded coincidence event, and it was used to identify an origin of the event from various possible reactions of the 9Li beam with different target constituents. This procedure relies on the correct identification of the undetected reaction product. For composite targets, such as the one used in this experiment, this may lead to misidentification of the reaction exit channel in the case of strong background contributions and low energy/angular resolution. For this reason, an additional procedure for the identification of the reaction exit channel, independent of the mass of the undetected nucleus, was applied [32]. Two kinematical quantities,
were calculated for each coincident event and plotted in the so-called Catania plot [32]. In this plot, events from the specific reaction gather in a locus around the line with a slope inversely proportional to the mass number of the undetected nucleus which intersects the ordinate at the negative value of the reaction Q-value (Eq. 5, Figure 3). The selection of the events originating from a particular three-body nuclear reaction was performed using both the calculated Q-value and a graphical cut applied around the reaction line in the Catania plot, in order to remove the background contributions as much as possible.
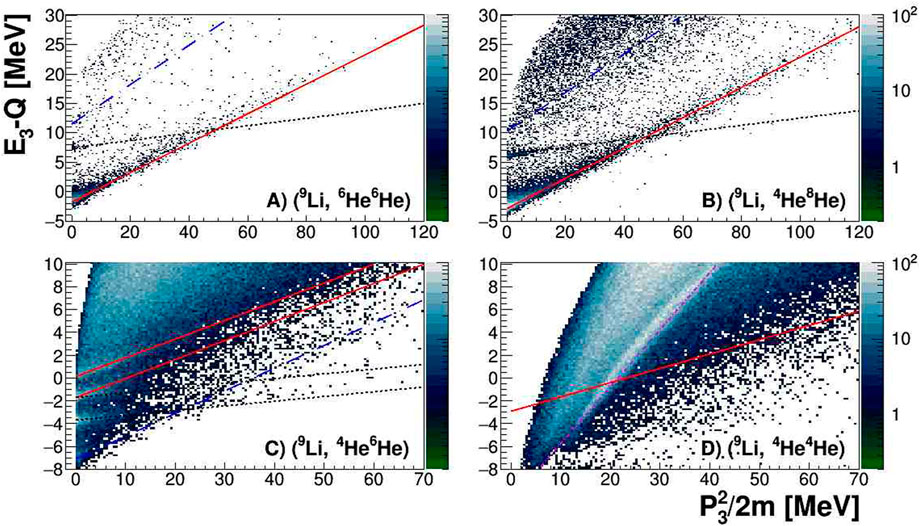
FIGURE 3. Examples of the correlation plots for the identification of events originating from various reaction exit channels: Catania plots reconstructed for the coincident detection of reaction product pairs relevant for the 12Be excited states. The spectra (A,C) correspond to the data for the 6He + 6He decay of the 12Be excited states, i.e. reaction (2) with Q value of 2.253 MeV. The spectra (B,D) are for the 4He + 8He decay and reaction (1) with Q = 3.402 MeV. In all the cases, reaction products are detected as dT = 3 events. Red lines mark loci associated with the reaction on the 7Li, blue dashed lines on the 6Li, and black dotted lines on the 19F. Additional red and black lines in plot C correspond to undetected 6He in its first excited state at 1.8 MeV for reaction on 7Li, and to undetected 18O in its first excited state at 1.99 MeV for reaction on 19F, respectively. On plot D, intense locus of the events marked with purple line corresponds to the reaction on hydrogen content of the target.
The aforementioned calculations require knowledge of the relative azimuthal angle between two detected reaction products, which cannot be measured using single-sided strip detectors used in the experiment. Thus, nominal values of the relative azimuthal angle were used in the calculations: for hits in the telescopes on the opposite sides of the beam, labeled as dT = 3 events, the nominal value used was 180°; for hits in telescopes separated by one detector segment, labeled as dT = 2, the nominal value of 120° was used; for hits in two adjacent telescopes (dT = 1), the nominal value was 60°; and for the hits in the same telescope (dT = 0), the nominal value was 0°. Real angular ranges of the relative azimuthal angles are [125°–180°] for dT = 3, [65°–175°] for dT = 2, [5°–115°] for dT = 1, and [0°–55°] for the dT = 0 case.
The large uncertainty in the relative azimuthal angle of the detected reaction products results in a large uncertainty in the reconstructed momentum of the undetected reaction product and degrades the resolution in the reaction Q-value and other kinematical quantities calculated for identification of the reaction. This is especially true for the dT = 2 and dT = 1 events. Consequently, for reaction exit channels with Q-values differing by less than 2.5 MeV in dT = 1, 2 sets of data, a clean separation of the corresponding events was not possible. For this reason, experimental data for each reaction exit channel were compared to detailed Monte Carlo simulations of all reaction channels that may contribute to that set of coincidence events. Monte Carlo simulations were performed using the UNISim MC framework [33] and included a realistic description of the experimental setup with all relevant sources of uncertainties, assuming isotropic formation of the 12Be excited state in the first step of the reaction and its subsequent isotropic decay [29]. The results of these simulations guided the selection of events associated with a particular reaction, minimizing background contributions at the cost of reduced event statistics.
For selected events of the particular reaction exit channel, the relative energy between each pair of the reaction products was calculated, in other words, the excitation energy spectra were reconstructed for all nuclei which are possible pathways to the same exit channel. Since all the reaction products were identified at this stage, it was possible to reconstruct the relative azimuthal angle from energies and polar angles of two detected nuclei. This procedure significantly improves the excitation energy resolution. For wrongly associated events, the relative azimuthal angle, and consequently the excitation energy are incorrect and degrade the spectral resolution, but do not lead to prominent peaks in the spectrum [29]. The excitation energy is obtained by adding the calculated value of the relative energy to the corresponding threshold energy for a particular decay channel. For example, the final state α + α + 8He may have proceeded through the excited states of 8Be decaying into α + α and two possible combinations of the 12Be excited states decaying into α + 8He. The relative energy between detected reaction products depends on their energies, polar angles, and relative azimuthal angle, while the relative energy between one detected and undetected reaction product is calculated using the energy and polar angle of other detected reaction product.
The 12Be excitation energy spectra were reconstructed for two possible helium-cluster decay channels: for the 6He + 6He decay, the 4He + 6He and 6He + 6He coincident events were analyzed and for the 4He + 8He decays, the 4He + 8He and 4He + 4He coincident events were analyzed. All selected events presented in the excitation energy spectra originate from the 9Li + 7Li reaction. The calculated excitation energies for each selected event are plotted in 2D correlation spectra for three possible pairs of reaction products, thus in three 2D spectra in total, of which the most relevant one for a particular set of data are presented in Figure 4. The excited state decaying into the particular pair of nuclei can be identified as a straight locus perpendicular to the corresponding axis. In the 4He + 6He + 6He exit channel, strong background contributions from the 10Be states decaying into the 4He + 6He were observed. These results will be presented in a separate publication. Similarly, the 8Be excited states decaying into two α-particles produced a strong background in the 12Be excitation energy spectra for the 4He + 8He decay. These observed states, known 10Be states at 9.56, 10.15, and 11.76 MeV [34] decaying into the 4He + 6He, and known 8Be states from the ground state up to 20 MeV in excitation [34] decaying into two α-particles, were excellent test samples for quality checks of the data analysis procedure, for verification of the Monte Carlo simulations, and for estimation of the uncertainty and resolution in the 12Be excitation energy. The strongest peaks in these background contributions were excluded from the final 12Be excitation energy spectra by applying cuts on the regions in the 2D correlation spectra which were projected on the 12Be axis. The remaining background contributions in the 12Be excitation energy spectra produce broad structures mainly at large excitation energies.
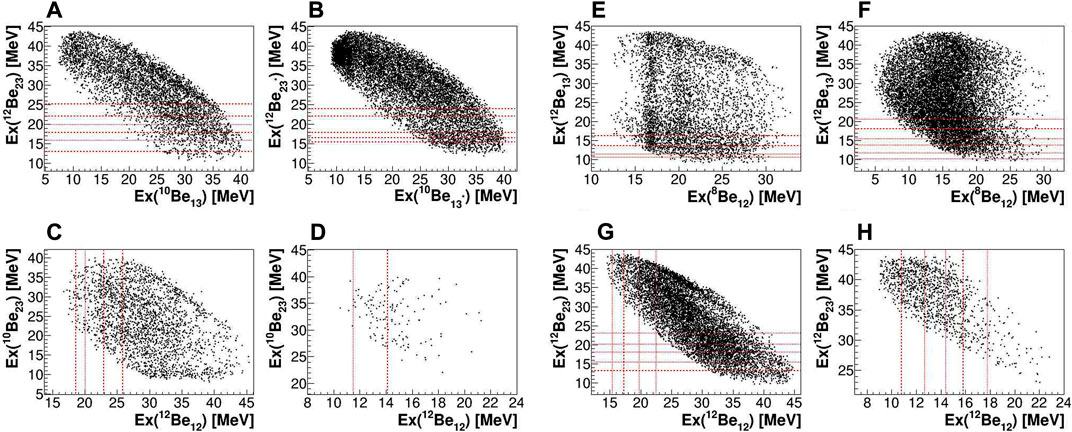
FIGURE 4. Typical correlation plots for the identification of excited states: 2D plots of excitation energy spectra reconstructed for composite systems made of various pairs of the three final reaction products. Plots A, B, C, and D are reconstructed for events associated with the 6He + 6He decay, plots E, F, G, and H for the 4He + 8He decay of the 12Be excited states. Spectra are reconstructed for the 4He + 6He dT = 3 coincidences for undetected 6He in the ground state (A) and the first excited state at 1.8 MeV (B); 6He + 6He coincidences for dT = 3 (C) and dT = 1 (D); 4He + 4He coincidences for dT = 3 (E) and dT = 2 (F); 4He + 8He coincidences for dT = 3 (G) and dT = 1 (H). The candidates for the 12Be states are marked with the lines.
The data were analyzed and are presented here separately for all types of xHe + yHe coincidence events and for all possible pairs of the detector array segments (dT = 0, 1, 2, 3), since different sets of events span different regions of the 12Be excitation energy, have different uncertainties, and contain different background contributions. The observed peaks in the 12Be excitation energy spectra were fitted with a Gaussian function on the top of smooth background contributions estimated by the polynomial function and, in some cases, broad Gaussian function at higher excitations to account for increased background contributions. The detection efficiency was also taken into consideration in the spectra analysis. Typical examples of the spectra are shown in Figures 5, 6. It can be seen that excitation energy spectra have rather low resolution and that most of the peaks have limited data statistics.
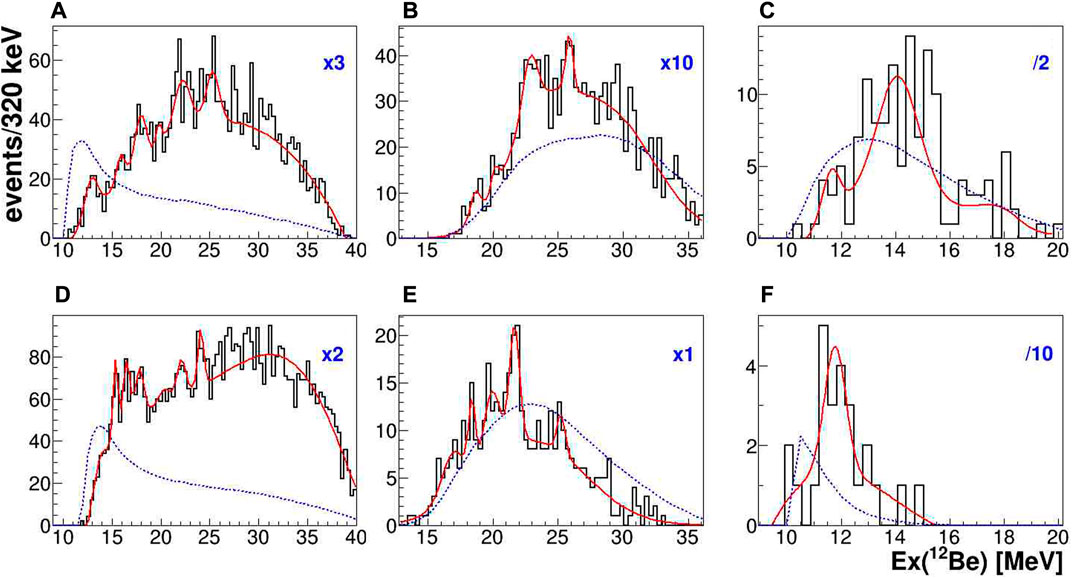
FIGURE 5. Examples of the 12Be excitation energy spectra for the 6He + 6He decay. Spectra are reconstructed for the 4He + 6He coincidences for dT = 3 and undetected 6He in the ground state (A); 6He + 6He coincidences for dT = 3 (B) and dT = 1 (C); 4He + 6He coincidences for dT = 3 and undetected 6He in the first excited state (D); 6He + 6He coincidences for dT = 2 (E) and dT = 0 (F). Solid red curves are results of the best fit using Gaussian curves for the peaks on the estimated background. Dotted blue curves represent the detection efficiency calculated by Monte Carlo simulations multiplied by the labeled factor.
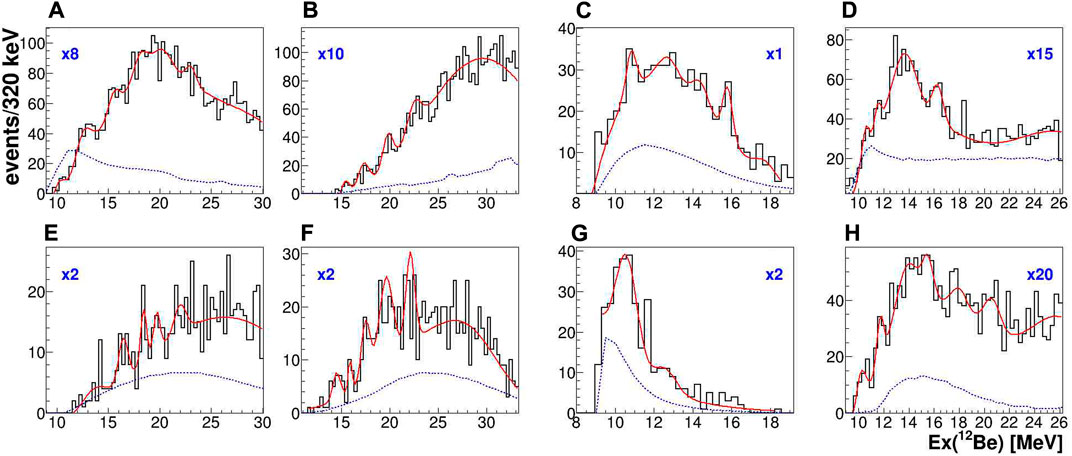
FIGURE 6. Examples of the 12Be excitation energy spectra for the 4He + 8He decay. Spectra are reconstructed for the 4He + 8He coincidences for dT = 3 and 8He paired with undetected 4He (A) and detected 4He (B); 4He + 8He coincidences for dT = 1 and 8He paired with detected 4He (C); 4He + 4He coincidences for dT = 3 and summed contributions of the 8He paired with undetected and detected 4He (D); 4He + 8He coincidences for dT = 2 and 8He paired with undetected 4He (E) and detected 4He (F); 4He + 8He coincidences for dT = 0 and 8He paired with detected 4He (G); 4He + 4He coincidences for dT = 2 and summed contributions of the 8He paired with undetected and detected 4He (H). Solid red curves are results of the best fit using Gaussian curves for the peaks on the estimated background. Dotted blue curves represent the detection efficiency calculated by Monte Carlo simulations multiplied by the labeled factor.
The excitation energy spectra presented have binning of 320 keV, and it was tested that main structures in the spectra are not artifacts of the data presentation by plotting spectra with binnings from 200 to 500 keV. The criteria to interpret the observed peak in the excitation energy spectrum as a candidate for the 12Be excited state are as follows:
- peak observation in the corresponding 2D correlation plots of excitation energies, examples are presented in Figure 4
- peak observation in at least two independent sets of data
- agreement in fit parameters of the state for different datasets: excitation energy difference of less than 350 keV for the low excitations and up to 600 keV for the highest excitations, and the FWHM value difference smaller than 350 keV
The only exception from the last two criteria are the states in the 6He + 6He*(1.8 MeV) decay, because corresponding coincidence events could have been separated from the data for the 6He ground state decay channel only for one dataset, the dT = 3 4He + 6He coincidences.
The Monte Carlo simulations provided detection efficiency curves for the excitation energy spectra and estimations of the excitation energy resolution. For these purposes, simulated data were analyzed in the same way as experimental data and these values were assigned from the generated spectra. The correctness of the simulations and a quality check of the data filtering criteria were validated by the analysis of the triple coincidence events 4He + 6He + 6He and 4He + 4He + 8He. Because all three reaction products for the reactions on the 7Li target were detected in these events, reaction exit channel identification was background-free and the resolution in the 8,10,12Be excitation energies was superior to the ones for the double coincidence data. Reconstructed spectra for simulated events were in excellent agreement with spectra from the experimental data. However, these subsets of triple coincidence data covered more limited phase space of the three-body reaction, had much lower detection efficiency, and resulted in much lower event statistics than the double coincidence data. In the 4He + 6He + 6He triple coincidence events, only the 10Be states were observed while in the 4He + 4He + 8He triple coincidences, in addition to the more intense 8Be states, also two 12Be states were observed.
3 Results and discussion
Here are presented some typical spectra from the full set of the results given in [29] which best illustrate the results obtained for the 12Be excited states. The first set of spectra presented in Figure 3 show correlation plots used to identify the events produced in the various possible reaction exit channels—Catania plots. On these plots are presented selected coincidence events of identified reaction product pairs relevant for the study of the 12Be excited states. In all of the cases presented here, reaction products were detected in the telescopes on opposite sides of the beam trajectory (dT = 3). The nominal relative azimuthal angle between detected reaction products of 180° was used initially in the event reconstruction and this led to a small shift in the corresponding Q-value, which in the worst case was 500 keV. The spectra A and C correspond to the data for the 6He + 6He decay of the 12Be excited states, that is, the reaction (2) with a Q-value of 2.253 MeV. The spectra B and D are for the 4He + 8He decay and reaction (1) with Q = 3.402 MeV. In all of the spectra, red lines mark the loci associated with the reaction on 7Li, blue dashed lines are for the reaction on 6Li, and black dotted lines are for the reaction on 19F. Additional red and black lines in plot C correspond to the undetected 6He in its first excited state at 1.8 MeV for the reaction on 7Li and to the 18O in its first excited state at 1.99 MeV for the reaction on 19F, respectively (the Q-values are more negative for the excited state than those for the ground state reaction). In plot D, there appears an intense locus of the events having a large slope and marked with purple line, which corresponds to the reaction on the hydrogen content of the target. These spectra show that for the 6He + 6He, 4He + 8He ,and 4He + 6He coincidence events, clean identification of events originating from the reaction on 7Li is possible, while for the 4He + 4He case, the selected events may contain some background contributions from other reactions, even if events at the crossing with intense hydrogen locus were excluded from further analysis.
The 2D excitation energy correlation plots for identification of the excited states in the composite systems, made of various pairs of the final reaction products, are presented in Figure 4. For the selected events of the particular reaction, the excitation energy spectra are reconstructed: spectra A, B, C, and D are for selected events associated with the 6He + 6He decay, while plots E, F, G, and H are for the 4He + 8He decay of the 12Be states. Spectra are presented for the 4He + 6He coincidences in opposite telescopes (dT = 3), for undetected 6He in the ground state (A) and the first excited state at 1.8 MeV (B), 6He + 6He coincidences for dT = 3 (C) and dT = 1 (D) case, 4He + 4He coincidences for dT = 3 (E) and dT = 2 (F) case, and 4He + 8He coincidences for dT = 3 (G) and dT = 1 (H) case. In the spectra A, B, and C, the intense loci correspond to the 10Be states but also loci associated with the 12Be states are visible. In the spectra E and F, the strongest loci are for the 8Be states which are observed as diagonal loci on the spectra G and H. In these spectra, the 12Be states can be also observed.
These 2D correlation plots were used to produce the final 12Be excitation energy spectra as projections on the 12Be axis, excluding in these projections regions with intense contributions of the 8Be/10Be states. The 12Be excitation energy spectra obtained for the 6He + 6He and 6He + 6He*(1.8 MeV) decays are presented in Figure 5, and the spectra for the 4He + 8He decay are presented in Figure 6. Figure 5 presents spectra for the 4He + 6He coincidences for dT = 3 case and undetected 6He in the ground state (A), 6He + 6He coincidences for dT = 3 (B) and dT = 1 (C), 4He + 6He coincidences for dT = 3 and undetected 6He in the first excited state (D), and 6He + 6He coincidences for dT = 2 (E) and dT = 0 (F). Figure 6 presents spectra for the 4He + 8He coincidences for dT = 3 and 8He paired with undetected 4He (A) and detected 4He (B); 4He + 8He coincidences for dT = 1 and 8He paired with detected 4He (C); 4He + 4He events for dT = 3 and summed contributions of the 8He paired with undetected and detected 4He (D), 4He + 8He for dT = 2 and 8He paired with undetected 4He (E) and detected 4He (F), 4He + 8He for dT = 0 and 8He plus detected 4He (G), and 4He + 4He events for dT = 2 and summed contributions of the 8He with undetected and detected 4He (H). In these figures, solid red curves are the results of the best fit using Gaussian curves for the peaks on the background represented by the polynomial function and, in some spectra, broad Gaussian function at the highest excitations. Dotted blue curves present detection efficiency calculated by Monte Carlo simulations multiplied by the labeled factor.
As already mentioned earlier, in all of the event selection processes, rigorous criteria were used in the attempt to minimize background contributions and filter as clean data as possible. This procedure significantly reduced the final number of accepted events and resulted in a small number of counts in the peaks associated with the 12Be excited states. Due to the limitations of the experimental setup, also the resolution in the excitation energy is rather low. The results of the simulations provided the estimates of experimental excitation energy resolution as follows: for excitation energy 3 MeV above the decay threshold for dT = 1 events FWHM = 950 keV; for excitation energy 6 MeV above the decay threshold for dT = 2 events FWHM = 1,050 keV and for dT = 3 FWHM = 750 keV; for excitation energy 10 MeV above the threshold for dT = 2 FWHM = 1,150 keV and for dT = 3 FWHM = 820 keV. It should be mentioned that the same problems, limited data statistics, poor resolution, and large background, can be seen in all published results on the helium decays of the 12Be states.
The 12Be states observed in this work that decay to 6He + 6He are listed in Table 1, to 6He + 6He*(1.8 MeV) in Table 2, and to 4He + 8He in Table 3. In these tables, all datasets on the 7Li target in which the particular decay channel can be observed are listed. For some datasets, it was not possible to separate different reaction exit channels, for example, in the 4He + 6He coincidences, for dT = 1, 2 cases, the events of the 6He decays into the ground and the first excited state of the undetected 6He cannot be separated. Also, the datasets for which the relative energy of the reaction product pair corresponding to the 12Be is at very high excitation are not considered, for example, the 4He + 6He coincidences for dT = 0. The dataset labels indicate the reaction exit channel in the form t(p,1 2)3, the identifier of the pair of detector segments in which reaction products were detected (dT = 0,1,2,3), and a pair of the reaction products forming the 12Be state. For each dataset and each observed 12Be state, the level of confidence of the peak observation is indicated: clear peak, indication of the peak (one with a small number of counts), and possible peak (one seen in other datasets, but in this case, it is obscured by contributions from the background or other peaks or affected by the detection efficiency). An empty position in the table means that the particular dataset does not cover the 12Be excitation energy interval of the peak observed in other datasets. The peaks with clear observation in, at least, two datasets are considered as the 12Be states. Other peaks in the tables, all of them satisfying aforementioned criteria for a candidate for the state, are considered as tentative states. Estimated uncertainty in their positions is ±250 keV for excited states from the decay thresholds to 14 MeV in excitation and ±400 keV for the states at higher excitations.

TABLE 1. 12Be excited states observed in the 6He+6He decay. Observation of a clear peak is labeled with •, indication of a peak with ◦, and a possible peak obscured by the background or other peaks, or affected by the detection efficiency, with ×. Tentative states are in parenthesis.

TABLE 2. 12Be excited states observed in the 6He+6He*(1.8 MeV) decay. Observation of a clear peak is labeled with •, indication of a peak with ◦, and possible peak obscured by the background or other peaks, or affected by the detection efficiency, with ×. Tentative states are in parenthesis.
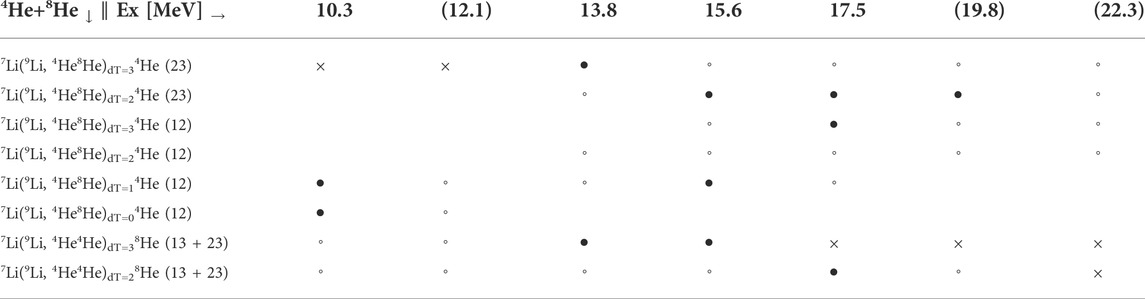
TABLE 3. 12Be excited states observed in the 4He+8He decay. Observation of a clear peak is labeled with •, indication of a peak with ◦, and a possible peak obscured by the background or other peaks, or affected by the detection efficiency, with ×. Tentative states are in parenthesis.
Taking into account the quoted uncertainty in the excitation energy and experimental resolution, most of the observed states can be associated with the states seen in the previous experiments [12–18]. The 12Be decay to 6He + 6He*, where one 6He is in its first excited state at 1.8 MeV and with Jπ = 2+, is observed for the first time in this work. Two of the observed five states in this decay channel most likely correspond to the states decaying to the ground state channel 6He + 6He, while the other two may correspond to the states decaying into the 4He + 8He decay channel.
At the low excitations, from the 4He + 8He decay threshold at 8.96 MeV, and the 6He + 6He threshold at 10.11 MeV, up to 14 MeV, states are observed in the 4He + 8He decay channel at 10.3 and 13.8 MeV, as well as the possible state at 12.1 MeV. The 13.8 MeV state is also observed in the spectra for the 4He + 4He + 8He triple coincidence events. In the 6He + 6He decay channel, a state is observed at 13.5 MeV and indications for the state at 11.7 MeV are found. The 10.3 MeV state was observed in [16, 17], where its spin 0+ was deduced and it was proposed as the band head of the 4He + 8He rotational band. In the same work, two other states at 12.1 and 13.6 MeV were observed, for the 13.6 MeV state, its spin/parity 4+ was deduced and it was claimed that these states are 2+ and 4+ members of the rotational band with very high deformation. The 12.1 MeV state was observed in [12, 13], as well as a state around 14 MeV. The broad peak around 13.6 MeV was observed in [15]. In [12, 13], a spin of 4+ was deduced for the 12.1 MeV and 14 MeV states. The states which may correspond to here propose 11.7 MeV state in the 6He + 6He channel were observed in [14, 16, 17], while the state around 13.5 MeV was observed in [12–17]. It is likely that the 13.5 MeV state in the 6He + 6He channel is the same state seen at 13.8 MeV in the 4He + 8He channel. The experimental FWHM values obtained in fits of the spectra in this work support this claim.
In the 12Be excitation energy ranging from 14 MeV to 19 MeV, in this work, in the 4He + 8He decay channel, the states are observed at 15.6 and 17.5 MeV. The 17.5 MeV state also appears in the spectra for the 4He + 4He + 8He triple coincidence events. In this decay channel, the states were observed close to 15.6 MeV in [12, 13, 15]. In [12, 13], also, the states at 17.4 and 18.2 MeV are reported. The peak around 17.5 MeV can be seen in this decay channel in spectra published in [15]. In the 6He + 6He decay channel, a state is observed in this work at 18.5 MeV and indications for the state at 16.5 MeV are found. In [12, 13], the states were reported at 16.1, 17.8, and 18.6 MeV, all with deduced Jπ = 6+, while the spectra published in [15] show peak around 18 MeV. In the 6He + 6He*(1.8 MeV) decay channel, the states are observed at 15.4, 16.5, and 17.8 MeV. The 15.4 and 17.8 MeV states may correspond to the 15.6 and 17.5 MeV states observed in the 4He + 8He channel. The 16.5 MeV state is the only one of three observed states which may be associated to the 6He ground state decay channel.
This decay scheme is different than the one observed in the 10Be states. It was observed in [35, 36] that the same 10Be states decay to the ground and the first excited state of 6He, both for the states with neutrons in pure σ orbitals and with a mixture of σ and π orbitals. It is not straightforward to link helium-cluster decay modes of these two nuclei, because 12Be is a more complex system with one proposed cluster, 8He, having neutron halo structure. If this difference in the 6He decay modes is not the result of limited quality of the presented 12Be experimental results, then it is related to the structural differences of these two Be isotopes due to bonding of additional two neutrons around the α—α cores.
At higher 12Be excitations, from 19 to 25 MeV, in this work, in the 6He + 6He decay channel, the states are observed at 22.5 and 25.4 MeV and in the 6He + 6He*(1.8 MeV) channel, at 22.1 and 24.0 MeV. There are also indications for the state at 20.0 MeV in the 6He ground state decay channel. The 6He*(1.8 MeV) decaying state at 22.1 MeV may correspond to the state observed in the decay to the 6He ground state at 22.5 MeV. The state at 24.0 MeV does not have its counterpart in other two decay channels. In the 4He + 8He decay channel, a state is observed at 19.8 and indications for the state at 22.3 MeV are found. It is plausible to correlate these states to the ones seen in the 6He decay channels. In this excitation energy range, states are reported [12, 13] at 19.4 and 20.7 MeV in the 4He + 8He channel and at 19.3, 20.9, 22.8, 24.0, and 25.1 in the 6He + 6He channel.
The summary of the 12Be states observed in this work to decay by the helium-cluster emission is given in Table 4. All observed states in the three decay channels examined are listed, with tentative states in parenthesis. The states which correlate with previously observed states are in bold. The states observed in the 6He + 6He decay channel are at 13.5, 18.5, 22.5, and 25.4 MeV, and indications for additional states at 11.7, 16.5, and 20.0 MeV are found. Five states are observed in the 6He + 6He*(1.8 MeV) decay channel, at 15.4, 16.5, 17.8, 22.1, and 24.0 MeV. In the 4He + 8He channel, states are observed at 10.3, 13.8, 15.6, and 17.5 MeV, and indications for the states at 12.1, 19.8, and 22.3 MeV are found.

TABLE 4. 12Be states observed in this work in the three helium-cluster decays. Tentative states are in parenthesis. The states observed in the previous studies are in bold.
The 4He + 8He* decay, with 8He in its first excited state, was examined in the 4He + 4He coincidences, but due to the large background in this dataset (Figure 3D) and the large width of the 8He excited state, there was no signature of this decay of the 12Be states. The data analysis for possible 9Li + 3H decay did not provide any indication for this decay of the 12Be states. This decay channel was studied only in [15], and the result was structureless spectrum with an indication of a state at 17.7 MeV. In these sets of data, the known excited states of 7Li decaying into 4He + 3H and excited states of 13B are observed. The 13B results will be presented in a separate publication. Data analysis for the neutron decay to the 11Be ground and first excited state is also performed but due to the detector array geometry, the 11Be + 4He coincidence data scan very high excitation energies in 12Be and provide the structureless spectrum. The present experimental data cannot provide information on the spin and parity of the observed states, not only due to low statistics and unfavorable resolution but also due to the non-zero spin projectile and target nuclei (both 9Li and 7Li have Jπ = 3/2−). The only hint on the possible spin/parity of the observed states is coming from its decay mode: the states decaying into the 6He + 6He must have even spin and positive parity (0+, 2+, 4+ etc.) because of identical 0+ constituents, while the states decaying into the 4He + 8He must be natural parity states (0+, 1−, 2+, 3− etc.) as both constituents are 0+ nuclei.
An attempt was made in the data analysis to get some hint on the reaction mechanisms of the 9Li + 7Li reaction which populates the helium-decaying states in 12Be. It was already mentioned that the expected main reaction mechanisms are the triton transfer from 7Li to 9Li and the t + 2n transfer from 9Li to 7Li. For this reason, the detector array geometry was optimized to increase the detection efficiency for reaction products of these transfer reactions using AUSA software tools [37]. Unfortunately, this detector geometry also optimizes detection of the background events from the decays of the 8Be states into 4He + 4He and of the 10Be states into 4He + 6He. These 8,10Be* helium-cluster decays were populated in much simpler transfer reactions with larger cross sections: the proton, for the 8Be, and triton, for the 10Be, transfer from 9Li to 7Li, and the proton transfer from 7Li to 9Li for 10Be. In the cluster transfer reactions, the residual part of the nucleus weakly interacts in the reaction and will slightly change its state of motion. Thus, if a triton is transferred from the 7Li target to 9Li beam to form 12Be, recoil α from 7Li will have small kinetic energy. Similarly, if the t + 2n are transferred from 9Li beam to 7Li, the recoil α from 9Li will slightly change the velocity it had as a part of the beam particle. With kinematical consideration of the momentum of the recoil α-particle, it was possible to select events most likely originating from these transfer reactions. Indeed, it is found that all observed helium-decaying 12Be states are also seen in these subsets of the data. However, it is also found that for some of these states, for which the detection efficiency permitted detection of corresponding events and which are not obstructed by events originated in decays of the 8,10Be states, the recoil α-particles have a broad range of the momentum. This would imply that the helium-decaying 12Be states were also produced in reactions such as fusion followed by α-particle emission. Unfortunately, low data statistics and unfavorable energy and angular resolution are prohibiting detailed analysis of the reaction process. It should be mentioned that non-observation of the 12Be states decaying into the 9Li + 3H channel implies that there is no resonant contribution in the triton transfer process from 7Li to 9Li.
4 Conclusion
An experimental study of the 12Be excited states decaying into 4He + 8He and 6He + 6He has been performed using the 9Li + 7Li → α + 12Be* → α + α + 8He and 9Li + 7Li → α + 12Be* → α + 6He + 6He reactions. The 9Li beam of energy of 74.8 MeV and LiF targets of thickness ≈ 1 mg/cm2 were used in the experiment. A large solid angle silicon strip detector array assembled of six wedge-shaped detector telescopes was used to detect reaction products. The reaction products were identified using the ΔE–E technique. The data analysis consisted of a sequence of event reconstruction and event selection procedures aimed to reject events originating from the background processes and to achieve a clean set of events for further analysis. In each step, rigorous criteria for selection of the events were applied and quality checks were performed by comparing the results obtained with the well-known properties of the nuclei involved in the considered reactions.
The 12Be excitation energy spectra were reconstructed for two possible helium-cluster decay channels: for the 6He + 6He decay, the 4He + 6He and 6He + 6He coincidences and for the 4He + 8He decay, the 4He + 8He and 4He + 4He coincidences were analyzed. In the 4He + 6He + 6He exit channel, strong background contributions from the 10Be states decaying into the 4He + 6He were observed. The 8Be excited states decaying into two α-particles produced a strong background in the 12Be excitation energy spectra for the 4He + 8He decay. The 8,10Be* nuclei were produced by much simpler reaction mechanisms than 12Be*, so the cross sections for their production are much larger than the ones for the 12Be*. Contributions of the strongest 8,10Be states were excluded from the final 12Be excitation energy spectra. The datasets of various xHe + yHe coincidence events detected in all possible pairs of the detector array segments were analyzed, and the results are presented separately. The excitation energy spectra obtained have significant background contributions, rather low resolution and observed peaks mostly have limited data statistics, but the same problems can be seen in all published results on the helium decays of the 12Be states. A peak in the excitation energy spectrum is considered as a candidate for the 12Be excited state if it was seen in corresponding 2D correlation plots of excitation energies in at least two independent datasets and if the fit parameters of the state agreed for different datasets. The data for the 6He + 6He*(1.8 MeV) decay could only be separated from the data for the 6He ground state decay channel for one dataset, the dT = 3 4He + 6He coincidences, and this is the only exception from these criteria. The candidate states are further filtered by the criterion of clean observation in at least two independent datasets, the candidate states which did not pass this criterion are considered as tentative states.
The results are the 12Be excitation energy spectra with the following observed excited states: 1) 13.5, 18.5, 22.5, and 25.4 MeV states, and tentative states at 11.7, 16.5, and 20.0 MeV in the 6He + 6He decay channel; 2) 15.4, 16.5, 17.8, 22.1, and 24.0 MeV states decaying into 6He + 6He*(1.8 MeV, Jπ = 2+); and 3) the states at 10.3, 13.8, 15.6, and 17.5 MeV, and tentative states at 12.1, 19.8, and 22.3 MeV, decaying into 4He + 8He. The estimated uncertainty in their positions is ±250 keV for excited states from the decay threshold energies up to 14 MeV and ±400 keV for the states at higher excitations. Most of the observed states were seen in the previous experiments. The 6He + 6He*(1.8 MeV) decay of the 12Be states is observed for the first time. Two of the five states observed in this decay channel most likely also decay to the ground state channel 6He + 6He, while two may correspond to the 4He + 8He decaying states. The 24.0 MeV state does not have a counterpart in the other two decay channels. The states around 13.5 and 20.0 MeV decay to the 6He + 6He and 4He + 8He channels, while the state around 22.3 MeV decays to all three decay channels examined. The experimental results presented here cannot provide information on the spin and parity of the observed states, due to the non-zero spin projectile and target nuclei. The decay modes of the observed helium-cluster decaying states provide limited information on their possible spin/parity as the states decaying into the 6He + 6He must have even spin and positive parity (0+, 2+, 4+, etc.), while the states decaying into 4He + 8He must be natural parity states (0+, 1−, 2+, 3−, etc.).
The data analysis for the 4He + 8He* decay with 8He in its first excited state, for the 9Li + 3H decay and for the neutron decays to the 11Be ground and first excited state for excitations higher than 25 MeV, was also performed, but the results obtained do not provide evidence for these decays of the 12Be excited states. The limited quality of obtained results and the reduced reaction phase space explored in the final dataset, due to the applied rigorous event selection criteria, do not permit detailed analysis of the reaction mechanism populating the helium-cluster decaying 12Be states, but simple considerations of the momentum of recoil α-particle imply that various complex reaction mechanisms, from triton transfer to compound nucleus reactions, may populate these states.
In the presented experimental work, it was not possible to identify proposed rotational bands because this measurement could not provide information on the spin, parity, and partial decay widths of the observed 12Be states. Even if the presented results provide limited evidence for the molecular structure in 12Be, they are one important step forward in the understanding of its structure because of the new spectroscopy information and the observation of the new decay mode of the states. Consequently, this advance in understanding of the 12Be structure may improve the understanding of the structure of light neutron-rich nuclei in general. This experiment is the first measurement aimed to study 12Be by use of the complex nuclear reactions to populate exotic cluster structures in the neutron-rich light nuclei. The observation of the helium-cluster decays of the 12Be states validates this approach and opens the path for future studies of other neutron-rich light nuclei (such as 14,15B and 15,16,17C) by the use of re-accelerated neutron-rich ion beams such as the 9Li beam at the ISAC-II facility at TRIUMF. The observed helium-cluster decaying 12Be excited states are in agreement with results of the previous experiments, particularly [12, 13, 16, 17], all performed by the use of breakup after inelastic excitation of the 12Be fragmentation beams. These new results show that previous results are not artifacts of the applied experimental technique. It is evident that in the excitation energy interval from 10 to 25 MeV, there are the 12Be excited states decaying into the 4He + 8He and 6He + 6He, and, as it is presented here for the first time, into the 6He + 6He*(1.8 MeV) channel. The observation of the states at these high excitations with these exotic decay properties is strongly supporting the molecular structure in the 12Be. An inspection of the decay threshold energies in 12Be (Table 5) leads to the conclusion that these states should have some peculiar property which inhibits their decay into the 11Be + n and 10Be + 2n channels, most probable decay modes for states at the excitations with very high density of the n/2n states and enables their decay into the helium-decaying channels. This peculiar property should be their special structure, most likely the cluster molecular structure of four neutrons orbiting two α-clusters, as it is proposed in a number of theoretical studies [10, 19–24].
Evidently, new experimental efforts are needed to uncover the structure of 12Be, one of the key nuclei to improve the knowledge of the clustering in the neutron-rich nuclei. For example, measurements of the reactions studied in this work with an improved detector array with better angular resolution and larger angular coverage could provide high-quality data which will result in improved characterization of the states, including the partial widths for the various decay channels, the important information to confirm the molecular nature of the states. The use of the large solid angle segmented neutron detector in these measurements would enable determination of the partial widths for various possible neutron-decay channels and would significantly enhance the knowledge of the 12Be structure.
Data availability statement
The raw data supporting the conclusions of this article will be made available by the authors, without undue reservation.
Author contributions
NS and MF were spokespersons of the experiment S1620 at TRIUMF, they proposed and led the experiment. NV did simulations for the optimization of the experimental setup and analyzed the experimental data under mentorship of NS. All authors contributed to setup of the experiment and the measurements. NS prepared the first draft, the revised versions and the final version of the manuscript. All authors contributed to manuscript revisions and read and approved the submitted version.
Funding
This work has been supported in part by the Croatian Science Foundation under Project no. 7194 and Project no. IP-2018-01-1257, by the European Regional Development Fund for the “Center of Excellence for Advanced Materials and Sensing Devices“ (Grant No. KK.01.1.1.01.0001) and for the Competitiveness and Cohesion Operational Programme (Grant No. KK.01.1.1.06), as well as by the European Union’s Horizon 2020 research and Innovation programme under grant agreement No. 669014. Part of the work has been supported by the Natural Sciences and Engineering Research Council of Canada under grants SAPPJ-2019-00039 and SAPMR 2020-00004. AD acknowledges support provided by the Istituto Nazionale di Fisica Nucleare. TD acknowledges support provided by the UK Science and Technology Facilities Council.
Acknowledgments
The authors thank Antonio Massara from INFN LNS Catania for provided targets and Peter Machule from TRIUMF for technical support in the experimental setup. The authors acknowledge the technical support of the TRIUMF operations and beam delivery groups during the measurements. NS thanks Alison Laird from the University of York for providing an additional detector during the measurements. NS and NV thank Oliver Kirsebom and Michael Munch from the University of Aarhus for their support in running AUSA tools for the experimental setup optimization and Matko Milin from the University of Zagreb for fruitful discussions on the obtained results.
Conflict of interest
The authors declare that the research was conducted in the absence of any commercial or financial relationships that could be construed as a potential conflict of interest.
Publisher’s note
All claims expressed in this article are solely those of the authors and do not necessarily represent those of their affiliated organizations, or those of the publisher, the editors, and the reviewers. Any product that may be evaluated in this article, or claim that may be made by its manufacturer, is not guaranteed or endorsed by the publisher.
References
1. Freer M, Horiuchi H, Kanada-En’yo Y, Lee D, Meissner U. Microscopic clustering in light nuclei. Rev Mod Phys (2018) 90:035004. doi:10.1103/RevModPhys.90.035004
2. von Oertzen W, Freer M, Kanada-En’yo Y. Nuclear clusters and nuclear molecules. Phys Rep (2006) 432:43–113. doi:10.1016/j.physrep.2006.07.001
3. Freer M. The clustered nucleus — Cluster structures in stable and unstable nuclei. Rep Prog Phys (2007) 70:2149–210. doi:10.1088/0034-4885/70/12/R03
4. von Oertzen W. Two-center molecular states in 9B, 9Be, 10Be and 10B. Z Phys A — Hadrons Nuclei (1996) 354:37–43. doi:10.1007/s002180050010
5. von Oertzen W. Dimers based on the α + α potential and chain states of carbon isotopes. Z Phys A - Particles Fields (1997) 357:355–65. doi:10.1007/s002180050255
6. Freer M. Molecules in nuclei. Comptes Rendus Physique (2003) 4:475–87. doi:10.1016/S1631-0705(03)00050-1
7. Freer M, Casarejos E, Achouri L, Angulo C, Ashwood NI, Curtis N, et al. α:2n:α molecular band in 10Be. Phys Rev Lett (2006) 96:042501. doi:10.1103/PhysRevLett.96.042501
8. Milin M, Zadro M, Cherubini S, Davinson T, DiPietro A, Figuera P, et al. Sequential decay reactions induced by a 18 MeV 6He beam on 6Li and 7Li. Nucl Phys A (2005) 753:263–87. doi:10.1016/j.nuclphysa.2005.02.154
9. Soić N, Blagus S, Bogovac M, Fazinić S, Lattuada M, Milin M, et al. 6He + α clustering in 10Be. Europhys Lett (1996) 34:7–12. doi:10.1209/epl/i1996-00407-y
10. Ito M, Itagaki N, Sakurai H, Ikeda K. Coexistence of covalent superdeformation and molecular resonances in an unbound region of 12Be. Phys Rev Lett (2008) 100:182502. doi:10.1103/PhysRevLett.100.182502
11. Korsheninnikov A, Nikolskii E, Kobayashi T, Aleksandrov D, Fujimaki M, Kumagai H, et al. Spectroscopy of 12Be and 13Be using a 12Be radioactive beam. Phys Lett B (1995) 343:53–8. doi:10.1016/0370-2693(94)01435-F
12. Freer M, Angélique JC, Axelsson L, Benoit B, Bergmann U, Catford WN, et al. Exotic molecular states in 12Be. Phys Rev Lett (1999) 82:1383–6. doi:10.1103/PhysRevLett.82.1383
13. Freer M, Angélique JC, Axelsson L, Benoit B, Bergmann U, Catford WN, et al. Helium breakup states in 10Be and 12Be. Phys Rev C (2001) 63:034301. doi:10.1103/PhysRevC.63.034301
14. Saito A, Shimoura S, Takeuchi S, Motobayashi T, Minemura T, Matsuyama YU, et al. Molecular states in neutron-rich beryllium isotopes. Nucl Phys A (2004) 738:337–41. doi:10.1016/j.nuclphysa.2004.04.057
15. Charity RJ, Komarov SA, Sobotka LG, Clifford J, Bazin D, Gade A, et al. Particle decay of 12Be excited states. Phys Rev C (2007) 76:064313. doi:10.1103/PhysRevC.76.064313
16. Yang Z, Ye Y, Li Z, Lou J, Wang J, Jiang D, et al. Observation of enhanced monopole strength and clustering in 12Be. Phys Rev Lett (2014) 112:162501. doi:10.1103/PhysRevLett.112.162501
17. Yang ZH, Ye YL, Li ZH, Lou JL, Wang JS, Jiang DX, et al. Helium-helium clustering states in 12Be. Phys Rev C (2015) 91:024304. doi:10.1103/PhysRevC.91.024304
18. Freer M, Ashwood NI, Achouri NL, Catford WN, Curtis N, Delaunay F, et al. Elastic scattering of 8He + 4He and two-neutron transfer and the influence of resonances in 12Be. Phys Lett B (2017) 775:58–62. doi:10.1016/j.physletb.2017.10.063
19. Maris P, Caprio MA, Vary JP. Emergence of rotational bands in ab initio no-core configuration interaction calculations of the Be isotopes. Phys Rev C (2015) 91:014310. doi:10.1103/PhysRevC.91.014310
20. Ito M, Ikeda K. Unified studies of chemical bonding structures and resonant scattering in light neutron-excess systems, 10, 12Be. Rep Prog Phys (2014) 77:096301. doi:10.1088/0034-4885/77/9/096301
21. Ito M. Formations of loose clusters in an unbound region of 12Be. Phys Rev C (2012) 85:044308. doi:10.1103/PhysRevC.85.044308
22. Ito M, Itagaki N, Ikeda K. Cluster correlations for low-lying intruder states of 12Be. Phys Rev C (2012) 85:014302. doi:10.1103/PhysRevC.85.014302
23. Dufour MPD, Nowacki F. Microscopic investigation of the 12Be spectroscopy. Nucl Phys A (2010) 836:242–55. doi:10.1016/j.nuclphysa.2010.02.002
24. Kanada-En’yo Y, Horiuchi H. Cluster structures of the ground and excited states of 12Be studied with antisymmetrized molecular dynamics. Phys Rev C (2003) 68:014319. doi:10.1103/PhysRevC.68.014319
25. Caurier E, Martínez-Pinedo G, Nowacki F, Poves A, Zuker AP. The shell model as a unified view of nuclear structure. Rev Mod Phys (2005) 77:427–88. doi:10.1103/RevModPhys.77.427
26. Lyu M, Yoshida K, Kanada-En’yo Y, Ogata K. Direct probing of the cluster structure in 12Be via the α-knockout reaction. Phys Rev C (2019) 99:064610. doi:10.1103/PhysRevC.99.064610
27.Micron semiconductor (2022). YY1 design. Available from: http://www.micronsemiconductor.co.uk/product/yy1/.
28. Davinson T. Multistrip silicon detectors for studies with low energy RNBs. Nucl Phys A (2004) 746:188–94. doi:10.1016/j.nuclphysa.2004.09.145
29. Vukman N. PhD thesis: Clustering in neutron-rich light nuclei produced in reactions of9Li beam on LiF target. Zagreb, Croatia: University of Zagreb (2022).
30. Tilley D, Weller H, Cheves C. Energy levels of light nuclei A = 16-17. Nucl Phys A (1993) 564:1–183. doi:10.1016/0375-9474(93)90073-7
31. Tilley D, Cheves C, Godwin L, Hale G, Hofmann H, Kelley J, et al. Energy levels of light nuclei A=5, 6, 7. Nucl Phys A (2002) 708:3–163. doi:10.1016/S0375-9474(02)00597-3
32. Costanzo E, Lattuada M, Romano S, Vinciguerra D, Zadro M. A procedure for the analysis of the data of a three body nuclear reaction. Nucl Instr Methods Phys Res Section A: Acc Spectrometers Detectors Associated Equipment (1990) 295:373–6. doi:10.1016/0168-9002(90)90715-I
33. Dell’Aquila D (2021). UNISim MC framework Available from: https://github.com/dellaquilamaster/unisim-tool.
34. Tilley D, Kelley J, Godwin J, Millener D, Purcell J, Sheu C, et al. Energy levels of light nuclei. Nucl Phys A (2004) 745:155–362. doi:10.1016/j.nuclphysa.2004.09.059
35. Miljanic D, Soić N, Blagus S, Cherubini S, Costanzo E, Lattuada M, et al. 10Be and molecular states. Fizika B Zagreb (2001) 10:235.
36. Soić N. PhD thesis: Nuclear reactions with 7Li and cluster structure of light nuclei. Zagreb, Croatia: University of Zagreb (1999).
37.Aarhus Subatomic Group. AUSA software tools. (2017). Available from: https://git.kern.phys.au.dk/ausa.
Keywords: nuclear structure, helium clustering, nuclear molecule, 12Be, neutron-rich light nuclei, transfer reactions, radioactive ion beam
Citation: Vukman N, Soić N, Freer M, Alcorta M, Connolly D, Čolović P, Davinson T, Di Pietro A, Lennarz A, Psaltis A, Ruiz C, Uroić M and Williams M (2022) Cluster decays of 12Be excited states. Front. Phys. 10:1009421. doi: 10.3389/fphy.2022.1009421
Received: 01 August 2022; Accepted: 29 September 2022;
Published: 20 October 2022.
Edited by:
Daniele Dell’Aquila, University of Sassari, ItalyReviewed by:
Magda Cicerchia, University of Padua, ItalyLibertad Barrón-Palos, Universidad Nacional Autónoma de México, Mexico
Copyright © 2022 Vukman, Soić, Freer, Alcorta, Connolly, Čolović, Davinson, Di Pietro, Lennarz, Psaltis, Ruiz, Uroić and Williams. This is an open-access article distributed under the terms of the Creative Commons Attribution License (CC BY). The use, distribution or reproduction in other forums is permitted, provided the original author(s) and the copyright owner(s) are credited and that the original publication in this journal is cited, in accordance with accepted academic practice. No use, distribution or reproduction is permitted which does not comply with these terms.
*Correspondence: Neven Soić, soic@irb.hr