Research status of rare-earth-ion-doped infrared laser
- 1Center for Advanced Laser Technology, Hebei University of Technology, Tianjin, China
- 2Innovation and Research Institute of Hebei University of Technology in Shijiazhuang, Shijiazhuang, China
- 3Hebei Key Laboratory of Advanced Laser Technology and Equipment, Tianjin, China
Infrared lasers have an extensive range of applications in sensing, detection, communication, medicine, and other fields. The principle of directly pumping solid-state lasers is simple, and it can easily achieve high-power and high-efficiency laser output, which is one of the important means to obtain infrared lasers. Incorporating rare earth ions into the substrate as the gain medium for directly pumping solid-state lasers can alter their optical performance and further enhance the performance of the laser. Lasers based on rare earth ion doping have a small volume, high conversion efficiency, good beam quality, wide tuning range, and multiple operating modes. Therefore, the proportion of rare earth ions doped as the gain medium for activating ions is currently very large. In this review, Ho3+, Tm3+, and Er3+ are selected as the representative rare earth ions, and their optical properties, such as luminous power and fluorescence lifetime, when doped in different substrates, such as crystals, ceramics, and fibers, are introduced, respectively, to illustrate their feasibility as infrared laser gain media. In addition, we show the different optical properties when doped with two ions, three ions, and four ions, demonstrating their great potential as infrared laser gain media.
1 Introduction
Due to the quick development of infrared laser technology, it is now widely used in different industries. Near-infrared lasers are commonly used in laser weapons, clinical surgery, precision machining, encrypted communications, etc. Mid-infrared lasers can be applied to spectral measurement, medical treatment, and remote sensing, while long-wave infrared lasers can achieve atmospheric detection and infrared photoelectric countermeasures [1]. There are many methods to obtain infrared lasers, including semiconductor quantum cascade lasers, free electron lasers, chemical lasers, gas lasers, frequency-doubling lasers, and directly pumping solid-state lasers. For semiconductor quantum cascade lasers, their advantages are their small size and high efficiency, but their structure is complex, the output power is small, and the beam quality is poor. Free electron lasers can generate high power and are easy to continuously tune, but they are bulky and expensive. Chemical lasers rely on chemical reactions to provide energy, with high output energy and good beam quality. However, toxic chemical byproducts are produced during chemical reactions, and the required raw materials are also relatively expensive. As a nonlinear laser, frequency-doubling lasers have a wide tunable spectral range and high repetition rate, but they also suffer from complex structures and expensive prices. The principle of direct solid-state lasers is relatively simple, and they can easily achieve high-power and high-efficiency laser output.
Directly pumping solid-state lasers can be divided into two groups based on gain media: solid-state lasers doped with rare earth ions and solid-state lasers doped with transition metal ions. Transition metal ion-doped solid-state lasers lack suitable pump sources, and it is hard to attain high energy output at room temperature with significantly reduced upper level lifetime. For rare earth ion doping, 11 trivalent ions, namely, Ce, Dy, Ho, Nd, Tm, Sm, Pr, Eu, Tb, Er, and Yb, and three divalent ions, namely, Dy, Sm, and Tm, have achieved laser output in rare earth elements. Their energy level structure is rich. The same ion has multiple emission peaks, which can achieve a multi-wavelength laser output. The mid-infrared solid laser based on rare earth ion doping has a small volume, high conversion efficiency, good beam quality, a wide tuning range, and many operation modes. Therefore, the proportion of gain medium doped with rare earth ions as active ions is very large at present, which has become a research hotspot in this field [2].
However, low damage thresholds, poor thermal performance, and poor stability of the matrix materials limit the power increase. Therefore, the selection of suitable matrix materials is an urgent problem to be solved. Therefore, in this review, Ho3+, Tm3+, and Er3+, that is, three kinds of ions that have been extensively studied at home and abroad, are selected as representatives, and their optical properties when doped into different crystals, ceramics, fibers, and other different substrates are introduced, respectively, which shows the feasibility of using them as infrared laser gain media. In addition, we show the different optical properties when doped with two ions, three ions, and four ions, demonstrating their great potential as infrared laser gain media.
2 Characterization of Ho3+ doping in different substrates
Ho3+ is a kind of rare earth ion, which is the main doped material in the gain medium of the laser. In different substrates, the laser performance can be different. A wide-band emission from 1930 nm to 2130 nm was achieved using holmium-doped Al2O3 glass as the gain medium in the first, which was an important step from 2 microns to a silicon-based laser source on a high-performance chip in the 2.2-micron wavelength range [3]. Holmium ion-doped aluminum germanate glass suitable for potassium–sodium ion exchange waveguides was made using the high-temperature melting method. The Ho3+-doped aluminum germanate glass has effective near-infrared fluorescence emission and stable waveguide performance when using 644-nm pumping light, which indicates that the Ho3+-doped aluminum germanate glass is a potential gain medium for ∼1.2-μm waveguide lasers [4].
In Ho3+-doped substrates, optical fibers, such as ZrF4-BaF2-LaF3-AlF3-YF3 glass fiber, are most commonly used. When Ho3+ is doped into the ZBYA glass fiber, the single cladding structure of the Ho3+-doped ZBYA glass fiber can be obtained under the pump of a 1150-nm laser to achieve a laser output of ∼2.9 μm [5]. In addition to glass fibers, there are CaYAlO4 single-crystal fibers. It is now possible to grow CALYO single-crystal fibers doped with different concentrations of Ho3+ by the micro-pulling-down method. After measuring and analyzing the polarization absorption spectrum at ambient temperature, fluorescence spectrum, and fluorescence decay curve, it was found that Ho3+ has a higher gain cross section and longer 5I7 level lifetime than other rare earth ions, which provides a higher power reserve capacity for pulsed laser operation, so Ho3+ ions are utilized to achieve laser emission at a wavelength of approximately 2 μm [6]. The polarized absorption spectra of Ho:CALYO crystal fibers doped with 0.5 and 2.0 at% of Ho3+ in the wavelength range of 300–2100 nm at room temperature are shown in Figures 1A, B. The spectra of Ho:CALYO crystal fibers consist of seven groups of bands, which are attributed to the transitions from the 5I8 ground state to the excited states, namely, 5G4, 5G5, 5F1+5G6, 5F4+5S2, 5F5, 5I6, and 5I7, respectively.
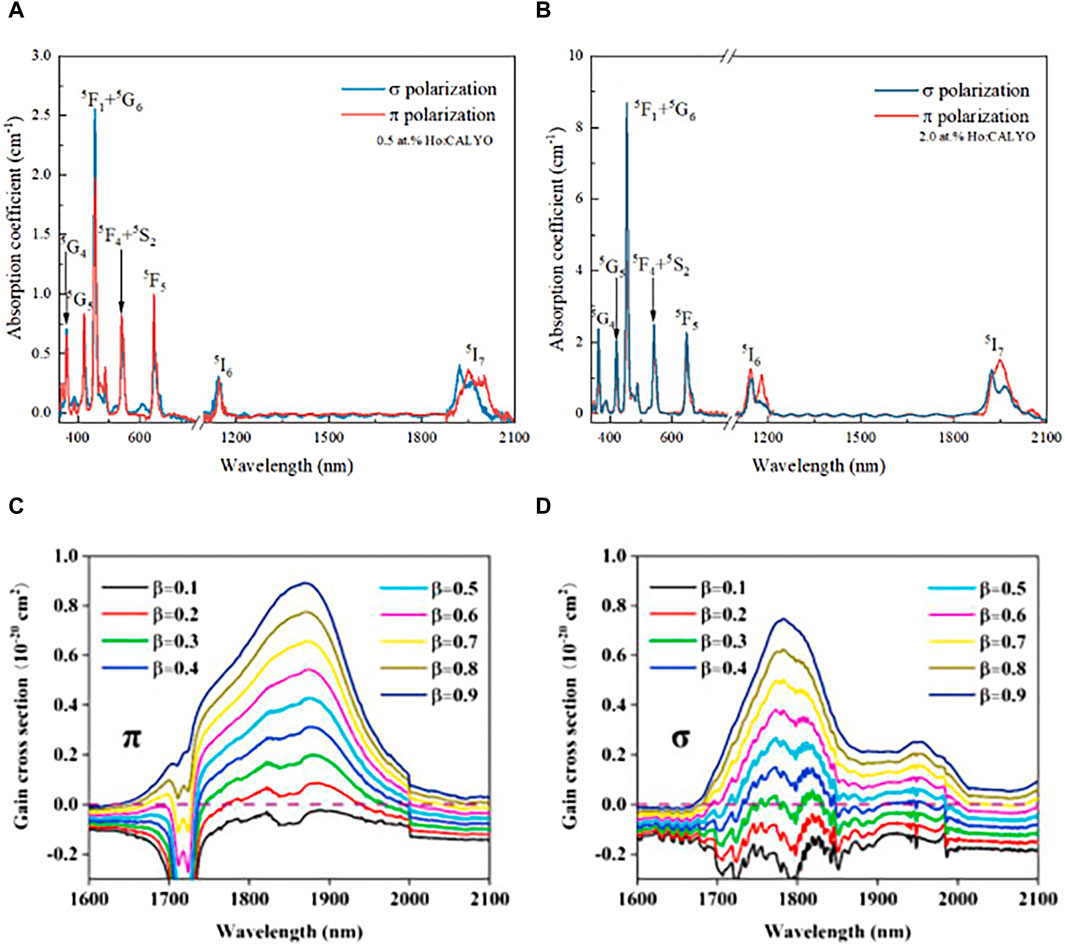
Figure 1. (A, B) Polarized absorption spectra of Ho:CALYO crystal fibers at Ho3+ concentrations of 0.5 at% and 2 at%. Reprinted with permission from ref. [6]. Copyright (2023) Elsevier. Gain cross sections of the 3F4→3H6 transition in YPO4 crystals doped with Tm3+. (C) π-polarization. (D) σ-polarization. Reprinted with permission from ref. [13]. Copyright (2023) Elsevier.
Using near-infrared waves cannot produce more than 4 μm wavelength of light. With long wavelength laser drives, high-order harmonic generation conversion efficiency is low. In order to solve these problems, Krishna Murari et al., based on a Kagome hollow photonic crystal fiber, proved that 3.3 ps at 140 μJ pulse can be compressed to 48 fs at 11 μJ pulse, and the focus intensity of 1013 W/cm2 can be reached [7]. Through the study of Ho3+-doped 2-μm band ultrafast laser amplification technology, research progress has found that due to the emission wavelength exceeding 2 μm, Ho3+-doped laser media absorbs less water vapor in the atmosphere, thus making it easier to transmit in the air, reducing the requirement for stable operation of the laser and simplifying laser design. Moreover, since it supports the broadband emission spectrum generated by ultrashort pulses and has the advantages of small quantum loss and a long upper level lifetime, it is mostly used in ultrafast laser amplifiers with large energy in the 2-μm band [8].
3 Characterization of Tm3+ doping in different substrates
Tm3+ is also a kind of rare earth ion, which is also the main doping material in the gain medium of the laser. Tm3+ can be doped in glass, for example, a high-concentration Tm3+ ion-doped fluoro-tellurate glass sample prepared using high-temperature melting technology. Using the Judd–Ofelt theory and absorption spectrum analysis, it can be seen that it has a high probability of spontaneous radiation, a large emission area and gain coefficient, and a fast inter-ion energy transfer rate. Therefore, it is concluded that fluoro-tellurate glass has a high luminous efficiency in the ∼2-µm band [9]. In addition, the Tm3+-activated tellurate glass core/borosilicate glass cladding fiber was prepared by the in-tube melting method. It has a good structure and negligible element diffusion, which can enhance the laser action of ∼2 µm [10]. In addition, Tm3+ can also be doped in semiconductors. Ozarfar Gafarov found that Tm is the material of choice for entering the mid-infrared region when doped into the II–VI semiconductor, which has wide infrared transparency, low phonon frequency, and low optical loss. However, the growth of large crystals with a good optical quality is difficult; they can be replaced by Tm ions to grow Ⅱ–Ⅵ materials and then by using the thermal diffusion doping method so as to become a more suitable gain medium [11].
In addition to the above two substrates, crystals are more commonly used in Tm3+-doped substrates. Liu et al. grew Tm3+:Ca10Li(VO4)7 single crystals using the Czochralski method and annealed them in air to eliminate oxygen vacancies and upgrade crystal transparency. By measuring its coefficient of thermal expansion and gain distribution over a certain range, it can be observed that Tm3+:Ca10Li(VO4)7 is extremely hopeful for wide tunable ultrashort pulse lasers with a 2-µm proximity [12]. Sun et al. successfully grew tetragonal Tm3+:YPO4 single crystals using the high-temperature solution method with an absorption band bandwidth near 800 nm, π-polarization of 13.1 nm, and σ-polarization of 22.5 nm. The gain cross sections of the Tm3+:YPO4 crystal with different values of β (β = 0.1, 0.2, … , 0.9) are shown in Figures 1C, D. The spectral mass factor of Tm3+:YPO4 single crystal is 1.71, and when the excited ion fraction β is ≥ 0.5, the uninterrupted positive gain range can reach 270 nm. All these denote that YPO4 crystals doped with Tm3+ are a laser medium of approximately 1.9 μm [13]. When Tm3+ is doped in the crystal, it is greatly affected by the temperature. When the temperature is 77 K, the highest slope efficiency obtained is more than five times the slope efficiency at 300 K. As the temperature drops from 300 K to 77 K, the laser threshold is reduced by a factor of two [14]. In the field of high-energy ultrafast laser amplification technology, new gain devices with a large surface area and high thermal damage threshold can effectively solve the problem of thermal management during the amplification process. By combining efficient low-temperature cooling methods, the heat dissipation efficiency of the gain medium can be improved, which is expected to become a potential technological path for achieving ultrafast lasers in the 100 mJ and kilowatt 2-μm bands [8].
4 Characterization of Er3+ doping in different substrates
Because of their complex energy-level structures, Er3+-doped laser materials have drawn a lot of attention in recent decades for application in visible and infrared solid-state lasers. Er3+-doped crystals have a quasi-three-level structure. The use of different wavelengths of pumping light makes the ground-state atom transition energy levels different, and because of the different Er3+-doped material, the excited-state photon transition from high energy levels to low energy levels is also very different [15]. Er3+ is often doped in ceramics and glass. The high-quality 5 at%Er:SrF2 transparent ceramics were prepared by chemically derived powder hot pressing at 700°C for 40 h. Its emission spectrum exhibits the mightiest emission band at 2735 nm, which implies that a laser output of approximately 2.7 μm can be achieved in 5 at% Er3+ ion-doped SrF2 transparent ceramics [16]. Wang et al. achieved efficient passive Q-switching of Er:Lu2O3 ceramic lasers with a semiconductor-saturable mirror based on a Bragg mirror. The shortest pulse duration and highest peak power generated by Q-switched Er:Lu2O3 lasers can be achieved with a passive Q-switched Er:Lu2O3 ceramic laser at 2.7 µm, proving that Er:Lu2O3 ceramics are a promising laser gain medium at 2.7 µm [17]. However, using ceramic as a substrate also has its problems. For example, Er:Y2O3 ceramics have a serious optical diffraction loss, but replacing them with Er:Y2O3 crystals can avoid this problem [18].
A more common substrate for Er3+ than ceramics is glass. Er3+-doped ZnF2-BaF2-SrF2-YF3 (ZBSY-e) glass has a strong emission of 2740 nm under the excitation of a 978-nm laser. At a fixed excitation power density, the emission intensity is higher than that of erbium-doped fluoroaluminate, fluorozirconate, and fluoroindenate glasses, which are potential gain media for 2.7-μm lasers [19]. The Ag-doped biosilicon–borotelluride glass doped with Er3+ nanoparticles prepared by melt quenching technology shows a high emission interface, and it is also a potential erbium-based gain medium, as confirmed by a detailed calculation of its laser parameters [20]. In order to obtain efficient infrared and mid-infrared luminescence properties, the new germanosilicate zinc glass with heavy Er3+ doping has caught one’s eye in the field of near- and mid-infrared applications in recent years because of its superior parameters [21]. The fluorescence of 3.5 μm was achieved in the fluoroindate glass under 635 nm excitation. By measuring the energy lifetime of Er3+ in the sample and calculating the vibrator intensity parameters using the Judd–Ofelt theory, it was found that fluorinolate-glass-doped Er3+ is a potential gain medium for 3.5-μm lasers [22]. By comparing different doping concentrations, it is found that the sample doped with 2 mol%Er (IZSB-2Er) has the longest fluorescence lifetime, the highest quantum efficiency, and the largest emission cross section. So the glass will be a suitable medium for achieving high-quality 2.7-μm fiber lasers [23]. In addition to glass, Er3+ can also be doped in halogenated glass. As the MIR spectra and energy level structure are recorded in Figure 2, when the 650-nm LD is excited, the particles in the ground state directly jump to 4F9∕2, which is in the upper level of ∼3.5 μm transition luminescence, and then down transition to the ground state stepwise. As a result, when the concentration is further increased, both the number of energy-absorbing particles and the luminous intensity increase significantly, as shown in Figure 2. In addition, the gain coefficient is often used to evaluate the effect of the matrix material on luminescence performance. The lower the coefficient, the more conducive the matrix is to luminescence. Once again, the gain coefficient of 0.5 in the gain spectra proves that the oxyhalide glass has excellent luminescence performance and high gain in the ∼3.5-μm band [24].
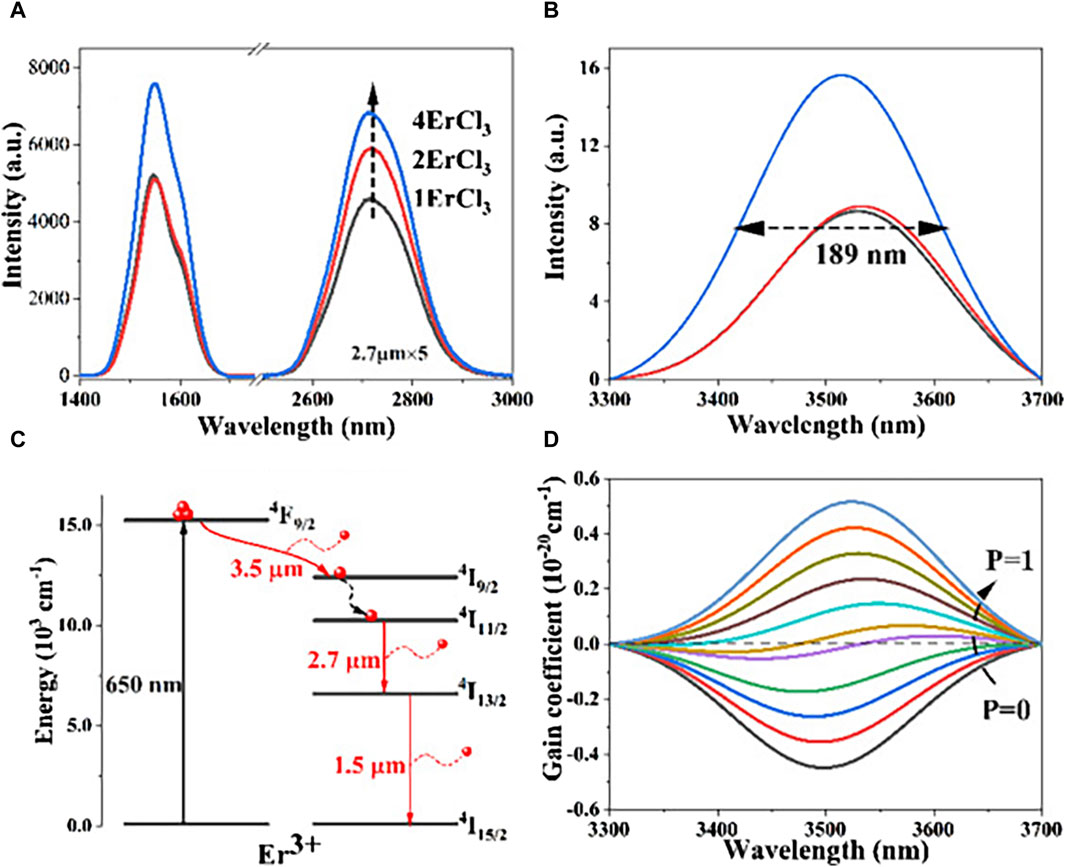
Figure 2. (A,B) MIR spectra in imparity bands of Er3+ by 650-nm LD pumped, (C) energy level structure of Er3+ by 650-nm LD pumped, and (D) gain spectra of the ∼3.5-μm band. Reprinted with permission from ref. [24]. Copyright (2022) Elsevier.
5 Characterization of co-doping of multiple rare earth ions
In rare earth ion doping, there is more multidoping than single doping. In multidoping, the co-doping of two ions is the main doping type. Compared to Dy3+ single-doped CaYAlO4 crystals, Yb3+/Dy3+ co-doped crystals have a strong 2.9-µm emission, and their upper-laser-level lifetime is longer, which is conducive to particle population inversion and 2.9-µm laser behavior [25]. NaYF4:Yb3+/Tm3+ microcrystals prepared by the hydrothermal method can achieve upconversion laser emission at 291, 346, and 364 nm, showing excellent upconversion luminescence performance [26]. Y2O3 ceramics-doped Ho and Yb prepared using the discharge plasma sintering method have a strong emission cross section and a full half-peak width of 208 nm. These consequences show that Y2O3 ceramics-doped Ho and Yb are a positive medium for a laser gain of approximately 2 μm [27]. Based on the ∼3-μm laser of Ho3+/Pr3+ co-doped AlF3-based glass single-clad fiber, by pumping the gain fiber with a single-mode 1150-nm fiber laser, the laser output wavelength of 2.87 μm can be gained [28]. There are also Er3+/Tm3+:CaLaGa3O7 crystals grown using Czochralski technology. Through detailed spectral analysis, it is known that the 980-nm excitation of the Er3+/Tm3+ co-doped CLGO crystal is the most suitable for the laser application of a 2.7-µm laser. The enrollment of Tm3+ reduces the fluorescence span of Er3+:4I13/2, so it may be a hopeful mid-infrared gain medium for intensified 2.7 µm lasers at 980-nm pumping [29]. For ZrF4-BaF2-LaF3-AlF3-YF3 zirconium fluoride glass co-doped with Tm3+ and Dy3+ ions combined by the high-temperature melting method, the addition of Tm3+ significantly improves the mid-infrared emission intensity of 2.9 μm and prolongs the fluorescence lifetime [30]. Er:Yb:glass is a commonly used 1.5-μm medium with a short length, high gain, long fluorescence life, low cost, and 95% erbium–ytterbium energy conversion efficiency. The heat accumulation process inside the Er:Yb:glass when it is used as the gain medium is carefully calculated using the finite element analysis method, and the influence of non-bonded and bonded crystals and different pumping wavelengths, power, and beam waist radius on the thermal effect of the crystal is quantitatively analyzed, which provides optimization conditions for further designing Er:Yb:glass with better thermal performance. It also provides a theoretical basis for obtaining a 1.5-μm laser with high power and high beam quality [31].
In addition to the co-doping of two ions, there are also three or even four ion co-doping cases. Liu et al. discovered that in Yb3+, Er3+, and Ho3+ triple-doped TeO2–BaF2–LaF3–La2O3 fluoro telluride glass, the glass conversion temperature is higher and the OH− absorption is lower than in Yb3+/Ho3+ co-doped. Er3+ functioned as an energy-conveying bridge between Ho3+ and Yb3+ ions, and after additional introduction, a greater 2.85-μm emission was created. As a result, the energy transfer efficiency increased to 96.2% due to the establishment of more energy transfer channels. This indicates that a better gain medium substance for 3-μm fiber lasers is anticipated to be Yb3+, Er3+, and Ho3+ triple-doped telluride glass [32]. When doping, changing just one ion can make a difference. For example, in comparison between CaLaGa3O7-doped Er3+, Yb3+, and Ho3+ and CaLaGa3O7-doped Er3+, Yb3+, and Eu3+ grown using Czochralski technology, both ions can reduce the lower laser energy level by energy spread, but for Er3+, Eu3+ is a better ineffective ion than Ho3+ when they are acting on a crystal, and therefore, Er3+/Yb3+/Ho3+:CaLaGa3O7 is a better potential medium for mid-infrared gain [33]. The Czochralski technique can be used to generate GYSGG (Gd2YSc2Ga3O12) single crystals co-doped with Yb3+, Cr3+, Ho3+, and Pr3+. Yb3+ and Cr3+ exist as sensitizers of Ho3+ ions in this co-doped crystal. Compared with the GYSGG crystals doped with Cr, Yb, and Ho ions, Pr3+ ions as passivating agents can effectively reduce the life span of low-laser level 5I7 of Ho3+ ions. These findings signify that GYSGG crystals doped with Cr, Yb, Ho, and Pr constitute a unique kind of laser gain mediator that is resistant to radiation in radiation environments. In short, there are many examples of ion co-doping. They change the properties of the medium through the interaction and influence between ions and have a great effect on practical applications [34].
6 Conclusion
Rare-earth ion doping as the gain medium of infrared lasers is a hot topic in current research. In this review, the optical properties of Ho mixed into different salt glasses or glass fibers, Tm mixed into different glasses, semiconductors, and crystals, and Er mixed into different ceramics and glass all show that they can be used as potential gain media for infrared lasers, but there are great differences in different bands. When a variety of ions are co-doped, compared with single doping, the laser emission power can be further improved, the luminous performance can be optimized, and it is better applied in practice. Although the infrared tunable laser can output high power and energy at present, the development prospect of a doped rare earth ion laser is still very large, and it can be deeply studied from the aspects of crystal preparation technology, pump source, resonator, and putting forward new solutions to further improve the performance of the laser so that it can be applied in a wider range of fields.
Author contributions
J-XZ: investigation, resources, and writing–original draft. GW: resources, supervision, and writing–review and editing. Y-FL: funding acquisition, supervision, and writing–review and editing. YY: funding acquisition, resources, and writing–review and editing. YW: resources, supervision, and writing–review and editing. ZL: resources, supervision, and writing–review and editing.
Funding
The author(s) declare financial support was received for the research, authorship, and/or publication of this article. This work was supported by the 173 Project Technical Fund [grant number JSLY-16-B16006], the Central Government Guides Local Funds for Scientific and Technological Development [grant number 236Z1813G], the Natural Science Research Foundation of Hebei University of Technology [grant number JBKYXX2203], the Funding Projects for the Introduction of Overseas Staff of Hebei Province [grant number C20210334], the National Natural Science Foundation of China [grant numbers 62075056], the Natural Science Foundation of Hebei Province [grant numbers F2022202035], and the Science and Technology Cooperation Special Project of Shijiazhuang (SJZZXA23002).
Conflict of interest
The authors declare that the research was conducted in the absence of any commercial or financial relationships that could be construed as a potential conflict of interest.
Publisher’s note
All claims expressed in this article are solely those of the authors and do not necessarily represent those of their affiliated organizations, or those of the publisher, the editors, and the reviewers. Any product that may be evaluated in this article, or claim that may be made by its manufacturer, is not guaranteed or endorsed by the publisher.
References
1. Bai Z, Hao X, Zheng H, Chen H, Qi Y, Ding J, et al. Research progress of high-power free-space Raman amplification technology (invited). Infrared Laser Eng (2023) 52(8):20230337. doi:10.3788/IRLA20230337
2. Zhu C, Kang M, Deng Y, Li W, Zhou S, Li J, et al. Research progress on directly pumping mid infrared solid state lasers. Laser and Infrared (2022) 52(7):956–62. doi:10.3969/j.issn.1001-5078.2022.07.002
3. Li N, Magden E, Su Z, Singh N, Ruocco A, Xin M, et al. Broadband 2-µm emission on silicon chips: monolithically integrated Holmium lasers. Opt Express (2018) 26(3):2220–30. doi:10.1364/OE.26.002220
4. Yang J, Zhao X, Zhang J, Lin H. 1.2μm near infrared fluorescence emission of holmium ions in thermal ion-exchanged aluminum germanate waveguide glasses(article). Faguang Xuebao/Chinese J Lumin (2018) 39(11):1519–26. doi:10.3788/fgxb20183911.1519
5. Xu C, Zhang J, Liu M, Wang S, Wang P. Midinfrared laser in Ho∼(3+)-Doped ZBYA glass fiber. Chin J Lasers (2022) 49(1):188–93. doi:10.3788/CJL202249.0101016
6. Chen P, Liu J, Xu J, Guo J, Zhang Z, Li D, et al. Ho:CaYAlO4 shaped crystal fibers grown by the micro-pulling-down method and optical properties. J Lumin (2023) 257:119705. doi:10.1016/j.jlumin.2023.119705
7. Murari K, Cirmi G, Cankaya H, Stein GJ, Debord B, Gérôme F, et al. Sub-50 fs pulses at 2050 nm from a picosecond Ho:YLF laser using a two-stage Kagome-fiber-based compressor. Photon Res (2022) 10(3):637–45. doi:10.1364/PRJ.441674
8. Mao J, Hu P, Zhou X, Wang H, Nie H, Yan B, et al. Research development on Tm3+/Ho3+ ions doped mid-infrared ultrafast lasers (Invited). Infrared Laser Eng (2021) 50(8):20210436. doi:10.3788/IRLA20210436
9. Zhang C, Han K, Zhou D, Song C, Xu P, Wu T, et al. High concentration Tm3+ doped TeO2-Al2O3-BaF2 glass for ∼ 2 µm fiber lasers. J Alloys Comp (2022) 901:163592. doi:10.1016/j.jallcom.2021.163592
10. Kang S, Tian Q, Huang X, Yang C, Liu X, Qiu J, et al. Enhanced CW lasing and Q-switched pulse generation enabled by Tm3+-doped glass ceramic fibers. Adv Opt Mater (2021) 9(3):1–8. doi:10.1002/adom.202001774
11. Ozarfar G. Optical and electrical characterization of transition metal and rare-earth metal doped II-VI semiconductors for mid-IR laser applications. The University of Alabama at Birmingham (2020).
12. Liu G, Bai Z, Yuan F, Huang Y, Zhang L, Lin Z. Growth and spectroscopic characterization of Tm3+:Ca10Li(VO4)7 crystal-a potential crystalline medium for 2 µm lasers. J Cryst Growth (2019) 520:62–7. doi:10.1016/j.jcrysgro.2019.05025
13. Sun L, Xu Q, Lu J, Su S, Zou Y, Liu R, et al. Preparation and spectroscopic characteristics of Tm:YPO4 crystal. J Lumin (2023) 257:119763. doi:10.1016/j.jlumin.2023.119763
14. Sulc J, Veselsky K, Nemec M, Jelínková H, Doroshenko ME, Konyushkin VA, et al. Temperature influence on diode-pumped Tm:SrF2-CaF2 laser properties. Proc SPIE (2017):10082. doi:10.1117/12.2251973
15. Li P, Zhang F, Li K, Cao C, Li Y, Zhang J, et al. 高重频大能量1.6 µm波段全固态激光的研究进展(特邀). Infrared Laser Eng (2023) 52(8):20230403. doi:10.3788/IRLA20230403
16. Liu J, Liu P, Wang J, Xu X, Li D, Zhang J, et al. Fabrication and sintering behavior of Er:SrF2 transparent ceramics using chemically derived powder(Article). Materials (2018) 11(4):475. doi:10.3390/ma11040475
17. Wang L, Huang H, Shen D, Zhang J, Tang D. High power and short pulse width operation of passively Q-switched Er:Lu2O3 ceramic laser at 2.7 μm. Appl Sci (2018) 8(5):801. doi:10.3390/app8050801
18. Zong M, Hou W, Zhao Y, Liu J, Zhao L, Liu J, et al. 2.7 μm laser properties research of Er:Y2O3 crystal. Infrared Phys Technol (2022) 127:104460. doi:10.1016/j.infrared.2022.104460
19. Jia S, Li C, Zhao Z, Yao C, Jia Z, Qin G, et al. Er3+-doped ZnF2-BaF2-SrF2-YF3 fluoride glasses for 2.7 μm laser applications. Mater Lett (2018) 227:97–9. doi:10.1016/j.matlet.2018.05.062
20. Halimah MK, Hamza AM, Muhammad FD, Chan KT, Umar SA, Umaru I, et al. Effect of erbium nanoparticles on structural and spectroscopic properties of bio-silica borotellurite glasses containing silver oxide. Mater Chem Phys (2019) 236:121795. doi:10.1016/j.matchemphys.2019.121795
21. Huang F, E F, Lei R, Li A, Li B, Ye R, et al. Enhancing the Er3+: infrared and mid-infrared emission performance in germanosilicate-zinc glasses(Article). J Lumin (2019) 213:370–5. doi:10.1016/j.jlumin.2019.05050
22. Wang P, Zhang J, Zhang J, Jia S, Wang L, Ning Y, et al. 3.5 μm emission in Er3+ doped fluoroindate glasses under 635 nm laser excitation. J Lumin (2021) 237:118200. doi:10.1016/j.jlumin.2021.118200
23. Liu Z, She J, Peng B. Spectroscopic properties of Er3+-doped fluoroindate glasses. J Rare Earths (2022) 40(7):1037–42. doi:10.1016/j.jre.2021.05.011
24. Xu Y, Tao C, Li Y, Li B, Huang F, Zhang J, et al. High-efficiency 3.5 μm luminescence of heavily Er3+ doped multicomponent glasses. Opt Commun (2023) 530:129146. doi:10.1016/j.optcom.2022.129146
25. Shen H, Wang Y, Zhu Z, Li J, You Z, Tu C. Intense 2.9 µm emission corresponding to Dy3+:6H13/2 → 6H15/2 transition in Yb3+/Dy3+ codoped CaYAlO4 crystal. J Lumin (2019) 208:188–92. doi:10.1016/j.jlumin.2018.12.043
26. Liang W, Xiao D, zhang W, Ni Y, Wan H, Xu X, et al. Infrared light induced deep ultraviolet internal light centers for novel cost-effective 3D printing. J Alloys Comp (2021) 863:158053. doi:10.1016/j.jallcom.2020.158053
27. Pan Y, Lin H, Liu J, Xu X, Hong R, Tao C, et al. Fabrication and spectral properties of Yb,Ho:Y2O3 transparent ceramics(Article). Opt Mater (2021) 112:110479. doi:10.1016/j.optmat.2020.110479
28. Wang P, Liu M, Zhang J, Xu N, Wang S. Watt-level ∼3 μm laser in AlF3 -based glass fiber. High Power Laser Part Beams (2021) 33(11):5–8. doi:10.11884/HPLPB202133.210311
29. Liu Y, Pan F, Wang X, Gao J. The influence of Tm3+ to enhance 2.7 μm mid-infrared emission of Er3+ in CaLaGa3O7 crystal. Laser Phys Lett (2021) 18(6):065702. doi:10.1088/1612-202X/abfa8a
30. Zhang C, Yun C, Zhang C, Zhang X, Lai S. Broadband 2.9 μm mid-infrared fluorescence behavior of Dy3+/Tm3+ co-doped zirconium fluoride glasses. Infrared Phys Technol (2022) 126:104330. doi:10.1016/j.infrared.2022.104330
31. Sun J, Wang Y, Zhang Y, Qi Y, Ding J, Yan B, et al. LD端面泵浦Er:Yb:glass/Co:MALO晶体热效应分析. Infrared Laser Eng (2023) 52(8):20230349. doi:10.3788/IRLA20230349
32. Liu C, Feng S, Xiao X, Xu Y, Guo H. Intense 2.85 μm mid-infrared emissions in Yb3+/Ho3+ codoped and Yb3+/Er3+/Ho3+ tridoped TBLL fluorotellurite glasses and their energy transfer mechanism. Ceramics Int (2022) 48(4):5267–73. doi:10.1016/j.ceramint.2021.11.068
33. Liu Y, Pan F, Tu C, Yao H. Spectroscopic properties of Er3+/Yb3+/Ho3+:CaLaGa3O7 and Er3+/Yb3+/Eu3+: CaLaGa3O7 crystals used in mid-infrared lasers(Article). Laser Phys Lett (2020) 17(6):065703. doi:10.1088/1612-202X/ab8a4e
Keywords: gain medium, doping, rare earth ions, infrared laser, optical property
Citation: Zhang J-X, Wang G, Li Y-F, Yu Y, Wang Y and Lv Z (2024) Research status of rare-earth-ion-doped infrared laser. Front. Phys. 12:1388567. doi: 10.3389/fphy.2024.1388567
Received: 20 February 2024; Accepted: 22 March 2024;
Published: 10 April 2024.
Edited by:
Quan Sheng, Tianjin University, ChinaReviewed by:
Kai Zhong, Tianjin University, ChinaRuiliang Xu, Changchun University of Science and Technology, China
Copyright © 2024 Zhang, Wang, Li, Yu, Wang and Lv. This is an open-access article distributed under the terms of the Creative Commons Attribution License (CC BY). The use, distribution or reproduction in other forums is permitted, provided the original author(s) and the copyright owner(s) are credited and that the original publication in this journal is cited, in accordance with accepted academic practice. No use, distribution or reproduction is permitted which does not comply with these terms.
*Correspondence: Gong Wang, wanggong@hebut.edu.cn; Yun-Fei Li, yfli@hebut.edu.cn; Yu Yu, yuyu1990@hebut.edu.cn