Archaea membranes in response to extreme acidic environments
- Department of Medical Genetics and Molecular Biochemistry, Lewis Katz School of Medicine, Temple University, Philadelphia, PA, United States
Bipolar tetraether lipids (BTL), such as glycerol dialkyl calditol tetraether (GDNT) and glycerol dialkyl glycerol tetraether (GDGT), are the dominating lipid species in thermoacidophiles that inhabit at pH ≤ 4 and temperatures ≥65°C. BTL containing archaea membranes respond to environmental pH changes by varying the number of cyclopentane rings in the isoprenoids, the amount of GDNT relative to GDGT, the ratio of tetraethers to diethers, and the level of glycosylation in polar headgroups. These structural and compositional adjustments can alter the hydrogen bond networks in the membrane polar headgroup regions and the packing tightness and rigidity in the membrane hydrophobic core. It is likely that these changes in non-covalent interactions among archaea lipids are made to retain low membrane volume fluctuations and their low sensitivity to temperature, as illustrated in the case of liposomes made of the polar lipid fraction E (PLFE) of Sulfolobus acidocaldarius. As such, a low passive proton permeability and a near neutral intracellular pH can be maintained, and, as a result, optimal activities of soluble and membrane-bound proteins in thermoacidophiles can be retained in acidic growth conditions at elevated growth temperatures.
Introduction
Certain microorganisms can thrive in extreme acidic (pH 1–4) (Lund et al., 2020) or alkaline (∼pH 9–13) (Koga et al., 1982; Preiss et al., 2015) environments while the pH of their intracellular compartments is near neutral. Alkaliphiles are bacteria. Acidophiles can be bacteria or archaea and many of them are thermophiles (≥60°C) (Auernik et al., 2008). This article reviews the research progress in biophysical characterization and understanding of membranes in thermoacidophilic archaea (Auernik et al., 2008).
Structure features of archaea lipids and their roles in archaea membranes
Archaea lipids have structural features that are distinctly different from those in bacteria and eukaryotes. Lipids in archaea contain isoprenoids linked to either glycerol or calditol via ether bonds (Figure 1A), forming an sn-2,3-glycerol stereo-configuration. In contrast, naturally occurring non-archaea lipids are in an sn-1,2 stereo-configuration and most lipids synthesized in bacteria and eukaryotes have fatty acyl chains linked to glycerol via ester bonds. Compared to ester bonds, ether linkages are chemically and thermally more stable. Phytanyl (20C) and biphytanyl (40C) are the most common isoprenoids found in archaea lipids and they contain branched methyl groups separated by 2−3 carbons (Figure 1A and Supplementary Figures S1, S2). Branched methyl group increases the cross-sectional area and hinders close packing of the hydrocarbon chains (Dote et al., 1990; Chugunov et al., 2014).
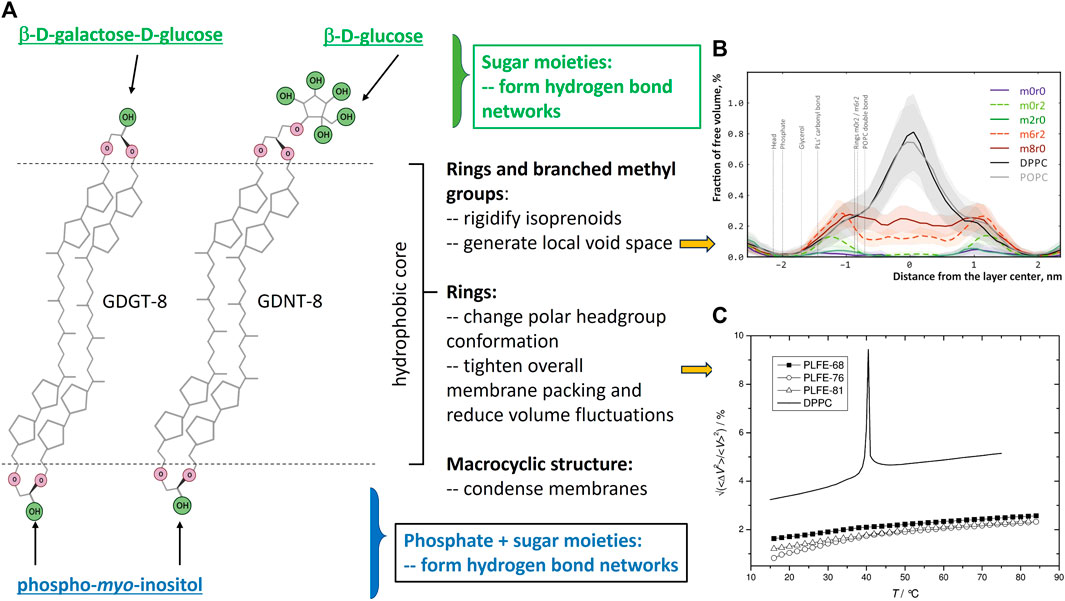
FIGURE 1. (A) shows the structure features of bipolar tetraether lipids (created with Biorender.com) and their contributions to archaea membrane properties, particularly, membrane free volumes and free volume fluctuations as they are closely related to solute (e.g., proton) permeation through membranes. The polar headgroups that are attached to the glycerol or calditol backbone can vary. This panel shows the polar headgroups associated with PLFE lipids (Chang and Lo, 1991). (B) is taken from Figure 6 of the molecular dynamics’ simulations study by Chugunov et al. (Chugunov et al., 2014) (with permission). This study shows the existence of local free volume in the isoprenoid areas of BTL membranes with 6 branched methyl groups and 2 cyclopentane rings (m6r2) or 8 branched methyl groups (m8r0) or 2 cyclopentane rings (m0r2). (C) is taken from Figure 6 of Zai et al. (Zhai et al., 2012), with permission. This figure shows the temperature dependence of experimentally obtained relative volume fluctuations (⟨ΔV2⟩/V2)1/2 of PLFE liposomes derived from S. acidocaldarius cells grown at three different temperatures: 68 °C (dark squares), 76 °C (open circles), 81 °C (open triangles). Solid line: DPPC liposomes for comparison.
Archaea lipids can be diethers or tetraethers. Certain archaea contain only diether lipids (e.g., in Methanococcus jannaschii and M. burtonii) or only tetraether lipids (e.g., in Pyrococcus woesei and P. islandicum) whereas many others have both diethers and tetraethers (Ulrih et al., 2009).
Archaea diether lipids typically have two isoprenoid chains attached to the glycerol moiety (Supplementary Figure S1). In rare cases, the two isoprenoid chains in a diether are covalently linked at the end to form a macrocyclic compound (Sprott et al., 1991) (Supplementary Figure S1). While most archaea diether lipids are saturated, unsaturated diethers (e.g., 2,3-di-O-geranylgeranyl-sn-glycerol) are present in some methanogens and halophiles (Nichols and Franzmann, 1992; Hafenbradl et al., 1996). Diether archaea lipids form bilayer membranes in aqueous solution; however, they can also form monolayers on a solid support. Membrane properties of archaea diethers can be modulated by apolar isoprenoid molecules such as squalene, which are abundant in archaea. Squalene tightens monolayer packing of diethers and alters monolayer membrane lateral organization creating bowl-like domains (Gilmore et al., 2013). Squalane (a hydrogenation product of squalene) can insert into the mid-plane space of diether bilayers reducing proton permeation (Salvador-Castell et al., 2019).
Most tetraethers in archaea are macrocyclic having two biphytanyl chains with one end of each of the biphytanyl chains attached to a glycerol and another end to either the second glycerol (called glycerol dialkyl glycerol tetraether, GDGT) or a calditol (called glycerol dialkyl calditol tetraether, GDNT) (Figure 1A). Some tetraether lipids are semi-macrocyclic, e.g., glycerol trialkyl glycerol tetraether (GTGT) (Rosa et al., 1983) (Supplementary Figure S2). Macrocyclic conformation per se has a condensation effect on membranes (Bulacu et al., 2012). In both GDGT and GDNT, up to four cyclopentane rings can be synthesized in each biphytanyl chain (Rao et al., 2023). The average number and distribution of cyclopentane rings in the isoprenoid chains depend upon the growth temperature (De Rosa et al., 1980), pH (Shimada et al., 2008; Boyd et al., 2011; Chiu et al., 2023), growth rate (Quehenberger et al., 2020) and growth phase (Jensen et al., 2015; Chiu et al., 2023). For example, the number of cyclopentane rings per tetraether molecule from the thermoacidophile Sulfolobus acidocaldarius increases from 3.4 to 4.8 when the growth temperature increases from 65°C to 82°C (De Rosa et al., 1980) and decreases from 5.1 to 4.6 when the growth rate increases from 0.011 to 0.035 h−1 at 75°C and pH 3.1 (Quehenberger et al., 2020). In the cases of S. islandicus and S. tokodaii, the number of cyclopentane rings per tetraether molecule in the exponential growth phase is slightly less than that in the lag and stationary phase (Jensen et al., 2015). In addition to cyclopentane, one cyclohexane ring can be present in a biphytanyl chain in certain crenarchaea (Damsté et al., 2002; Pearson et al., 2004). Cyclopentane ring hinders hydrocarbon chain rotation, shortens membrane thickness, and tightens membrane packing (Gabriel and Chong, 2000). While cyclopentane rings and branched methyl groups in the tetraether isoprenoids bring about membrane rigidity, they also generate some local free volume in the hydrophobic core (Chugunov et al., 2014) (Figure 1B). Various polar groups, such as phosphoinositol and carbohydrates, are attached to the two glycerol moieties in GDGT or to the glycerol and calditol ends of GDNT, generating asymmetric bipolar tetraether lipids (BTL) (Figure 1A).
In archaea cell membranes, BTL span the entire membrane forming a monomolecular structure, with anionic groups, such as the phosphoinositol-containing polar end, facing the intracellular compartment and the glycosyl polar end residing at the outer surface of the cell (Morii and Koga, 1994). Since the BTL polar headgroups are rich in hydroxyl groups, extensive hydrogen bond networks are formed on both the outer and the inner surface of BTL membranes. Compared to glycerol, calditol has five more OH groups. Thus, GDNT can, in principle, form more hydrogen bonds with neighboring molecules than GDGT; however, the overall hydrogen bonding is dependent upon the actual polar headgroups attached to the calditol and glycerol. In addition to the upright configuration, BTL may adopt a U-shaped disposition in membranes (Gulik et al., 1985; Bakowsky et al., 2000; Jeworrek et al., 2011), which is energetically less favorable than the upright conformation (Bulacu et al., 2012). Additional structure features of archaeal lipids in membranes are discussed in several previous reviews (Albers et al., 2000; Koga and Morii, 2005; Chong, 2010; Oger and Cario, 2013; Schouten et al., 2013; Caforio and Driessen, 2017; Siliakus et al., 2017).
Effects of growth pH on lipid structures, membrane compositions, and the physicochemical properties of thermoacidophile membranes
Thermoacidophiles, mainly those belonging to the archaeal orders of Sulfolobales and Thermoplasmatales, are rich in bipolar tetraether lipids (BTL). Membranes composed of archaea BTL have unusual physicochemical properties. For example, the relative membrane volume fluctuations of the polar lipid fraction E (PLFE, exclusively BTL) from S. acidocaldarius are extraordinarily low and temperature insensitive, changing only from 1% at 20°C to 2% at 75°C, as opposed to 3.2% below, 9% during, and 5% above the main phase transition of dipalmitoylphosphatidylcholine (DPPC) diester bilayers (Zhai et al., 2012) (Figure 1C). As another illustration, membrane packing of PLFE is so tight and rigid that the membrane probe 6-dodecanoyl-2-dimethylaminonaphthalene (Laurdan) can only partially insert into PLFE vesicular membranes, leaving the long axis of the naphthalene chromophore of Laurdan exposed to the outside and aligned parallel to the membrane surface, in sharp contrast to the disposition of Laurdan in diester membranes (Bagatolli et al., 2000). Atomistic molecular dynamics simulations further revealed that BTL membranes are tightly packed but there is local void space near the branched methyl groups and the cyclopentane rings in the isoprenoid chains (Chugunov et al., 2014) (Figure 1B). Therefore, BTL membranes are “durable” (rigid and tight) yet “liquid” (Chugunov et al., 2014). In short, BTL membranes exhibit tight and rigid packing in both the hydrophobic core and the polar headgroup regions, yet, they have certain fluidity for membrane functions which together contribute significantly to thermoacidophilic archaea’s capability to thrive in extreme environments such as low pHs [recently reviewed in (Rao et al., 2023; Řezanka et al., 2023)].
Under the optimal growth conditions (pH ≤ 4), the intracellular pH of thermoacidophiles falls within a narrow range, 5.4–6.5 (Slonczewski et al., 2009), with the exception from the extreme acidophiles such as Picrophilus torridus and Picrophilus oshimae, which have an intracellular pH value 4.6 (Table 1). This near neutral or slightly acidic intracellular pH range (4.6–6.5) is essential for the optimal activities of DNA and intracellular proteins (Auernik et al., 2008). Maintaining pH homeostasis inside the cell, while under acidic stress, requires a fine balance between proton inflow and outflow, which can come from several membrane transport systems or mechanisms including membrane channels, proton pumps, ATPase, and passive proton permeation (Michels and Bakker, 1985; Guan and Liu, 2020).
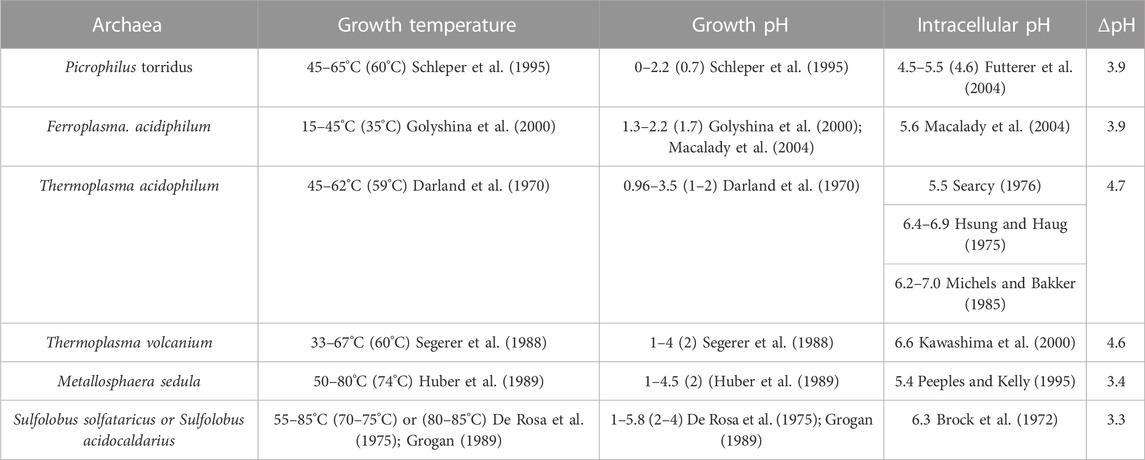
TABLE 1. Illustrations of intracellular pHs and growth temperatures/pHs of acidophilic archaea. Optimum values are within the parentheses. ΔpH, intracellular pH—growth pH.
Passive proton permeability in archaea tetraether lipid model membranes has been studied by using pH sensitive fluorescent probes (e.g., 6-carboxyfluorescein and pyranine). Liposomal membranes made of asymmetric BTL isolated from the thermoacidophile S. acidocaldarius exhibit exceedingly low proton permeability (Chang, 1994; Elferink et al., 1994; Komatsu and Chong, 1998), such as (0.3–0.5) x10−8 cm s−1 at 65–82°C for PLFE liposomes versus (3–9) x 10−8 cm s−1 at the same temperatures for liposomes made of egg yolk phosphatidylcholine (Komatsu and Chong, 1998). Furthermore, proton permeability in PLFE liposomes increases by less than 2 x 10−10 cm s−1 from 25 to 82°C whereas liposomes made of egg yolk phosphatidylcholine changes by 8 x 10−8 cm s−1 from 25 to 82°C (Komatsu and Chong, 1998). This remarkably low proton permeability and temperature insensitivity for BTL liposomes has been attributed to the strong hydrogen bond networks in the membrane polar headgroup regions (Elferink et al., 1994) and the tight and rigid packing in the hydrophobic core (Komatsu and Chong, 1998). This proposition is in line with not only the theory that proton permeation across liposomal membranes occurs through transient defects produced by thermal fluctuations (Nichols and Deamer, 1980; Lawaczeck, 1988), but also the more recent experimental finding that membrane volume fluctuations in PLFE liposomes are exceedingly low and temperature insensitive (Chong et al., 2010; Zhai et al., 2012) (Figure 1C). The branched methyl groups can also contribute to the low proton permeability of BTL membranes (Yamauchi et al., 1993), but to a much lesser extent (Komatsu and Chong, 1998). The negative charge on the membrane surface is not the contributing factor for the low proton permeability in PLFE liposomes (Komatsu and Chong, 1998). It is believed that such a low proton permeability and temperature insensitivity are essential for the plasma membrane of S. acidocaldarius to maintain a sharp pH gradient between the extracellular environment (pH 2.5) and the intracellular space (pH 6.5) over a wide range of growth temperatures (65–90°C) (van de Vossenberg et al., 1998).
For thermoacidophiles under optimal growth conditions, the extracellular environment can be 3–5 pH units more acidic than the intracellular compartment (Table 1). When the growth pH is further lowered, an even larger proton gradient across the archaea membrane will be formed and the rate of proton permeation will be significantly enhanced, which could lead to a lower and physiologically less favorable pH inside the cell. To counteract the lowered growth pH and the enlarged pH gradient across the membrane, archaea cells can increase the number of cyclopentane rings in the isoprenoids. Molecular dynamics simulation (Gabriel and Chong, 2000) shows that archaea tetraether lipid membranes made of GDNT containing eight cyclopentane rings in the molecule are packed much tighter, with membrane volume reduced by 4.9% and the interaction energy increased by 35 kcal/mol, when compared to GDNT membranes with no rings in the isoprenoids. Furthermore, the polar headgroup of GDNT runs almost parallel to the membrane surface when containing eight cyclopentane rings, whereas the headgroup is oriented perpendicular to the membrane surface when containing no rings. As such, cyclization of isoprenoids affects the packing of both the hydrophobic and hydrophilic regions of the membrane. These ring-induced condensing effects and structural changes have been attributed to increased hydrogen-bonding, harmonic bond stretching, theta expansion bond angle bending, and dihedral angle torsion. It is well known that solute permeability (including proton permeability) is decreased when membrane packing is tighter and more rigid (Falck et al., 2004; Zhai et al., 2012; Chugunov et al., 2014). Thus, increasing the number of isoprenoid cyclization is an effective adaptation strategy employed by archaea cells to fight against the acid stress. To this end, it is worthy of mentioning that archaea membrane tightness may not vary with the number of cyclopentane rings in BTL isoprenoids in a monotonic manner. Membrane compressibility data showed that membrane packing in PLFE liposomes reaches maximal tightness when the lipids are derived from cells grown at optimal temperatures (Zhai et al., 2012).
In addition to isoprenoid cyclization, archaea can synthesize more sugar moieties for the BTL to cope with the increased acid stress. The additional sugars can bring about more OH groups to the BTL polar headgroups and consequently strengthen the hydrogen bond networks in BTL membranes. Protons in the extracellular environment need to overcome three physical barriers, namely, the polar headgroups facing the extracellular environment, the hydrophobic core, and the polar headgroups facing the intracellular side, to reach the cytoplasm of the cell. A more extensive hydrogen bonding network in the lipid polar headgroup regions would hinder proton permeation in the membranes. This proton shelter concept was proven correct by a biomimetic study, which showed that a 10-nm-thick polymer layer rich in OH on a quartz crystal microbalance chip was able to raise the pH of the coated chip from 1.0 to >5.0 (Wang et al., 2012).
The above-described lipid structural changes with growth pH are, for the most part, consistent with the data obtained from the molecular biology and geochemistry studies. Lipid analyses from cultivated archaea cells (De Rosa et al., 1980; De Rosa and Gambacorta, 1988) and the archaea cells taken from geothermal springs (Boyd et al., 2013) showed that the number of cyclopentane rings in archaea tetraether lipids increases with decreasing growth pH (Boyd et al., 2011). The enzyme that generates cyclopentane rings in the isoprenoid chains of GDGT (ring synthase, Grs) has been discovered (Zeng et al., 2019). Sulfolobus Grs has two isoforms. GrsA catalyzes the synthesis of the first four cyclopentane rings, namely, GDGT-1, GDGT-2, GDGT-3, and GDGT-4 (Zeng et al., 2019). GrsB prefers to act on the products resulting from GrsA and generates additional cyclopentane rings (i.e., GDGT-5-8) (Zeng et al., 2019). Like the case of lipid analyses, higher grs abundance and more grs gene copies were found in the archaea grown in more acidic environments (Blum et al., 2023).
While a few studies showed a negative correlation between the number of cyclopentane rings and the environmental pH, a couple of studies lead to an opposite conclusion. For example, the average number of cyclopentane rings in GDGT isolated from the thermoacidophile Thermoplasma acidophilum HO-62 was found to change from 5.1 at pH 3 to 4.1 at pH 1.8 (Shimada et al., 2008) and the average number of GDGT cyclization in the thermoacidophilic archaeon Saccharolobus islandicus changed from 3.7 at pH 3.4 to 1.6 at pH 2.4 during the mid-log growth phase at 76°C (Chiu et al., 2023). Interestingly, the study of Shimada et al. also reported that the archaea BTL carried more sugar moieties in the polar headgroups when the growth pH decreased (Shimada et al., 2008). An increase in sugar moieties could provide more of the proton shelter effect (Wang et al., 2012), resulting in a decrease in proton permeability in archaea membranes, as discussed earlier. Thus, it appears that the assessment of the pH effect on archaea membranes should consider both the number of cyclopentane rings in the isoprenoids and the amount of sugar moieties in the lipid polar headgroups. In other words, to evaluate whether membrane modification is the main mechanism for thermoacidophiles to cope with acid stress, lipid analysis (or molecular dynamics simulations) should be conducted on the intact BTL molecules, rather than just the core structure (Chiu et al., 2023) or BTL without sugar moieties (Chugunov et al., 2014).
The amount of GDNT relative to GDGT is also an important factor governing proton permeability and other archaea cell membrane properties. Membrane behaviors of GDNT can be quite different from those of GDGT. For instance, the surface potential of monolayer made of hydrolyzed GDNT (with the phosphoinositols and carbohydrates removed) increases by 13% when the pH in the aqueous subphase is changed from 5.5 to pH 7.4, whereas that of hydrolyzed GDGT remains virtually unchanged with the same pH changes (Dote et al., 1990). Such a difference can be understood by the fact that calditol in hydrolyzed GDNT has 5 free hydroxyl groups whereas glycerol in hydrolyzed GDGT has only one free hydroxyl. Thus, compared to GDGT, GDNT can have more hydrogen bonds with neighboring molecules. This property can partially explain why calditol-linked membrane lipids are required for acid tolerance in S. acidocaldarius (Zeng et al., 2018). Since low pH is known to disrupt hydrogen bonding and GDNT has more hydrogen bonds, it is expected that membranes made of GDNT-containing BTL can tolerate more acidic stress than GDGT-containing lipid membranes.
Lipid analyses, proteomics, and transcriptomics are useful for assessing the changes in archaea membranes under environmental stresses. However, due to the discrepancy between the observation of grsB upregulation and the detection of a lower number of cyclopentane rings per BTL in S. islandicus upon acid stress, Chiu et al. (2023) pointed out that transcript data alone may not be used to predict GDGT cyclization because significant post-transcriptional regulation may occur. Nevertheless, the transcriptomic data indicated that there was an upregulation of proton pumping ATPase when the growth pH of S. islandicus changed from 3.4 to 2.4 (Chiu et al., 2023). Thermoacidophiles have other active proton pumping proteins such as NADH dehydrogenase (Futterer et al., 2004). How their protein expression levels respond to acidic stress are not well documented.
Lipid structure changes induced by an acidic environment (e.g., more cyclopentane rings, additional sugar moieties, increased GDNT-to-GDGT ratio), as revealed by lipid analyses, transcriptomics, and proteomics, should affect the overall membrane packing, which consequently can alter not only passive proton permeability but also the activities of proton pumps embedded in the membrane. However, to date, the quantitative determinations of proton permeability, membrane fluidity, and the activities of active proton pumps as a function of cyclopentane rings plus sugar moieties in live archaea cells or well-defined model membrane systems are largely missing. This is the major research gap that needs to be filled before claiming that thermoacidophilic archaea follow the principles of pH and membrane viscosity homeostasis in response to environmental acidic stress. The concept of homeoviscous adaptation was previously established with substantial support from biophysical measurements of membrane properties in bacteria and eukaryotes (Sinensky, 1974; Ernst et al., 2016); but controversy still existed (Hazel, 1995). When animal cells are grown in higher or lower temperatures, optimal cell membrane viscosity, as measured by fluorescent probes, is retained for normal cell functions by changing the length and degree of unsaturation in diester lipid acyl chains and by changing the content of membrane regulators, such as cholesterol and ergosterol in eukaryotes and hopanoids in bacteria. In thermoacidophilic archaea membranes, sterols are not present, and their cyclopentane rings in the isoprenoids may serve as the modulator of membrane viscosity, which affects membrane-bound proteins and proton permeation. This proposition needs to be supported by some sorts of membrane “viscosity” measurements, in addition to molecular dynamics simulations.
Conclusion
Thermoacidophilic archaea have multiple mechanisms to biochemically adjust themselves in response to an increase in environmental acidic stress. They can synthesize more cyclopentane rings in the isoprenoids and more sugar moieties in the polar headgroups. They can also synthesize more GDNT relative to GDGT and more tetraether lipids relative to dieters, in conjunction with upregulation of active proton pumping proteins. These structural and compositional adjustments can alter the hydrogen bond networks in the membrane polar headgroup regions and the packing tightness and rigidity in the membrane hydrophobic core.
It is likely that these changes in non-covalent interactions among archaea lipids are made to retain low membrane volume fluctuations and their low sensitivity to temperature, as illustrated in the case of PLFE liposomes mentioned above. As such, a low passive proton permeability and a near neutral intracellular pH can be maintained, and, as a result, optimal activities of soluble and membrane-bound proteins in thermoacidophiles can be retained in acidic growth conditions at elevated growth temperatures.
The growth conditions of thermoacidophiles may resemble, to some extent, the conditions for life on earth billion years ago (di Giulio, 2005). Thus, our knowledge gained in this research area could be useful for shedding light on early life’s biological adaptation and for designing artificial cells that can sustain life in harsh physical conditions.
Author contributions
P-GC: Conceptualization, Validation, Writing–original draft, Writing–review and editing.
Funding
The author(s) declare that no financial support was received for the research, authorship, and/or publication of this article.
Acknowledgments
The author would like to thank Michelle Tanujaya for making the drawings and for editing the manuscript.
Conflict of interest
The author declares that the research was conducted in the absence of any commercial or financial relationships that could be construed as a potential conflict of interest.
Publisher’s note
All claims expressed in this article are solely those of the authors and do not necessarily represent those of their affiliated organizations, or those of the publisher, the editors and the reviewers. Any product that may be evaluated in this article, or claim that may be made by its manufacturer, is not guaranteed or endorsed by the publisher.
Supplementary material
The Supplementary Material for this article can be found online at: https://www.frontiersin.org/articles/10.3389/frbis.2023.1338019/full#supplementary-material
References
Albers, S. V., van de Vossenberg, J. L., Driessen, A. J. M., and Konings, W. N. (2000). Adaptations of the archaeal cell membrane to heat stress. Front. Biosci. 5, D813–D820. doi:10.2741/albers
Auernik, K. S., Cooper, C. R., and Kelly, R. M. (2008). Life in hot acid: pathway analyses in extremely thermoacidophilic archaea. Curr. Opin. Biotechnol. 19, 445–453. doi:10.1016/j.copbio.2008.08.001
Bagatolli, L., Gratton, E., Khan, T. K., and Chong, P.L.-G. (2000). Two-photon fluorescence microscopy studies of bipolar tetraether giant liposomes from thermoacidophilic archaebacteria Sulfolobus acidocaldarius. Biophysical J. 79, 416–425. doi:10.1016/s0006-3495(00)76303-x
Bakowsky, U., Rothe, U., Antonopoulos, E., Martini, T., Henkel, L., and Freisleben, H. J. (2000). Monomolecular organization of the main tetraether lipid from Thermoplasma acidophilum at the water-air interface. Chem. Phys. Lipids 105, 31–42. doi:10.1016/s0009-3084(99)00131-0
Blum, L. N., Colman, D. R., Eloe-Fadrosh, E. A., Kellom, M., Boyd, E. S., Zhaxybayeva, O., et al. (2023). Distribution and abundance of tetraether lipid cyclization genes in terrestial hot springs reflect pH. Environ. Microbiol. 25, 1644–1658. doi:10.1111/1462-2920.16375
Boyd, E. S., Hamilton, T. L., Wang, J., He, L., and Zhang, C. L. (2013). The role of tetraether lipid composition in the adaptation of thermophilic archaea to acidity. Front. Microbiol. 4, 62–15. doi:10.3389/fmicb.2013.00062
Boyd, E. S., Pearson, A., Pi, Y., Li, W. J., Zhang, Y. G., He, L., et al. (2011). Temperature and pH controls on glycerol dibiphytanyl glycerol tetraether lipid composition in the hyperthermophilic crenarchaeon Acidilobus sulfurireducens. Extremophiles 15, 59–65. doi:10.1007/s00792-010-0339-y
Brock, T. D., BrocK, K. M., Belly, R. T., and Weiss, R. L. (1972). Sulfolobus: a new genus of sulfur-oxidizing bacteria living at low pH and high temperature. Arch. fur Mikrobiol. 84, 54–68. doi:10.1007/bf00408082
Bulacu, M., Periole, X., and Marrink, S. J. (2012). In silico design of robust bolalipid membranes. Biomacromolecules 13, 196–205. doi:10.1021/bm201454j
Caforio, A., and Driessen, A. J. M. (2017). Archaeal phospholipids: structural properties and biosynthesis. Biochimica Biophysica Acta (BBA) - Mol. Cell Biol. Lipids 1862, 1325–1339. doi:10.1016/j.bbalip.2016.12.006
Chang, E. L. (1994). Unusual thermal stability of liposomes made from bipolar tetraether lipids. Biochem. biophysical Res. Commun. 202, 673–679. doi:10.1006/bbrc.1994.1983
Chang, E. L., and Lo, S. L. (1991). Extraction and purification of tetraether lipids from Sulfolobus acidocaldarius, protocols for archaebacterial research. Baltimore, MD: Maryland Biotechnology Institute, 2.3.1–2.3.14.
Chiu, B. K., Waldbauer, J., Elling, F. J., Mete, Ö. Z., Zhang, L., Pearson, A., et al. (2023). Membrane lipid and expression responses of Saccharolobus islandicus REY15A to acid and cold stress. Front. Microbiol. 14, 1219779. doi:10.3389/fmicb.2023.1219779
Chong, P.L.-G. (2010). Archaebacterial bipolar tetraether lipids: physico-chemical and membrane properties. Chem. Phys. Lipids 163, 253–265. doi:10.1016/j.chemphyslip.2009.12.006
Chong, P.L.-G., Sulc, M., and Winter, R. (2010). Compressibilities and volume fluctuations of archaeal tetraether liposomes. Biophysical J. 99, 3319–3326. doi:10.1016/j.bpj.2010.09.061
Chugunov, A. O., Volynsky, P. E., Krylov, N. A., Boldyrev, I. A., and Efremov, R. G. (2014). Liquid but durable: molecular dynamics simulations explain the unique properties of archaeal-like membranes. Sci. Rep. 4, 7462. doi:10.1038/srep07462
Damsté, J. S., Schouten, S., Hopmans, E. C., van Duin, A. C. T., and Geenevasen, J. A. J. (2002). Crenarchaeol: the characteristic core glycerol dibiphytanyl glycerol tetraether membrane lipid of cosmopolitan pelagic Crenarchaeota. J. Lipid Res. 43, 1641–1651. doi:10.1194/jlr.m200148-jlr200
Darland, G., Brock, T. D., Samsonoff, W., and Conti, S. F. (1970). A thermophilic, acidophilic mycoplasma isolated from a coal refuse pile. Science 170, 1416–1418. doi:10.1126/science.170.3965.1416
De Rosa, M., Esposito, E., Gambacorta, A., Nicolaus, B., and Bu’Lock, J. D. (1980). Effects of temperature on ether lipid composition of Caldariella acidophila. Phytochemistry 19, 827–831. doi:10.1016/0031-9422(80)85120-x
De Rosa, M., and Gambacorta, A. (1988). The lipids of archaebacteria. Prog. Lipid Res. 27, 153–175. doi:10.1016/0163-7827(88)90011-2
De Rosa, M., Gambacorta, A., and Bu’Lock, J. D. (1975). Extremely thermophilic acidophilic bacteria convergent with Sulfolobus acidocaldarius. J. General Microbiol. 86, 156–164. doi:10.1099/00221287-86-1-156
di Giulio, M. (2005). Structuring of the genetic code took place at acidic pH. J. Theor. Biol. 237, 219–226. doi:10.1016/j.jtbi.2005.04.009
Dote, J. L., Barger, W. R., Behroozi, F., Chang, E. L., Lo, S. L., Montague, C. E., et al. (1990). Monomolecular film behavior of tetraether lipids from a thermoacidophilic archaebacterium at the air/water interface. Langmuir 6, 1017–1023. doi:10.1021/la00095a023
Elferink, M. G., de Wit, J. G., Driessen, A. J., and Konings, W. N. (1994). Stability and proton-permeability of liposomes composed of archaeal tetraether lipids. Biochimica Biophysica Acta (BBA) - Biomembr. 1193, 247–254. doi:10.1016/0005-2736(94)90160-0
Ernst, R., Ejsing, C. S., and Antonny, B. (2016). Homeoviscous adaptation and the regulation of membrane lipids. J. Mol. Biol. 428, 4776–4791. doi:10.1016/j.jmb.2016.08.013
Falck, E., Patra, M., Karttunen, M., Hyvonen, M. T., and Vattulainen, I. (2004). Impact of cholesterol on voids in phospholipid membranes. J. Chem. Phys. 121, 12676–12689. doi:10.1063/1.1824033
Futterer, O., Angelov, A., Liesegang, H., Gottschalk, G., Schleper, C., Schepers, B., et al. (2004). Genome sequence of Picrophilus torridus and its implications for life around pH 0. Proc. Natl. Acad. Sci. U. S. A. 101, 9091–9096. doi:10.1073/pnas.0401356101
Gabriel, J. L., and Chong, P. L.-G. (2000). Molecular modeling of archaebacterial bipolar tetraether lipid membranes. Chem. Phys. Lipids 105, 193–200. doi:10.1016/s0009-3084(00)00126-2
Gilmore, S. F., Yao, A. I., Tietel, Z., Kind, T., Facciotti, M. T., and Parikh, A. N. (2013). The role of squalene in the organization of monolayers derived from lipid extracts of Halobacterium salinarum. Langmuir 29, 7922–7930. doi:10.1021/la401412t
Golyshina, O. V., Pivovarova, T. A., Karavaiko, G. I., Kondrat’eva, T. F., Moore, E. R. B., Abraham, W.-R., et al. (2000). Ferroplasma acidiphilum gen. nov., sp. nov., an acidophilic, autotrophic, ferrous-iron-oxidizing, cell-wall-lacking, mesophilic member of the Ferroplasmaceae fam. nov., comprising a distinct lineage of the Archaea. Int. J. Syst. Evol. Microbiol. 50, 997–1006. doi:10.1099/00207713-50-3-997
Grogan, D. W. (1989). Phenotypic characterization of the archaebacterial genus Sulfolobus: comparison of five wild-type strains. J. Bacteriol. 171, 6710–6719. doi:10.1128/jb.171.12.6710-6719.1989
Guan, N., and Liu, L. (2020). Microbial response to acid stress: mechanisms and applications. Appl. Microbiol. Biotechnol. 104, 51–65. doi:10.1007/s00253-019-10226-1
Gulik, A., Luzzati, V., De Rosa, M., and Gambacorta, A. (1985). Structure and polymorphism of bipolar isopranyl ether lipids from archaebacteria. J. Mol. Biol. 182, 131–149. doi:10.1016/0022-2836(85)90032-4
Hafenbradl, D., Keller, M., and Stetter, K. O. (1996). Lipid analysis of Methanopyrus kandleri. FEMS Microbiol. Lett. 136, 199–202. doi:10.1111/j.1574-6968.1996.tb08049.x
Hazel, J. R. (1995). Thermal adaptation in biological membranes: is homeoviscous adaptation the explanation? Annu. Rev. Physiology 57, 19–42. doi:10.1146/annurev.ph.57.030195.000315
Hsung, J. C., and Haug, A. (1975). Intracelullar pH of thermoplasma acidophila. Biochimica Biophysica Acta (BBA) - Biomembr. 389, 477–482. doi:10.1016/0005-2736(75)90158-3
Huber, G., Spinnler, C., Gambacorta, A., and Stetter, K. (1989). Metallosphaera sedula gen, and sp. nov. Represents a new genus of aerobic, metal-mobilizing, thermoacidophilic archaebacteria. Syst. Appl. Microbiol. 12, 38–47. doi:10.1016/s0723-2020(89)80038-4
Jensen, S. M., Neesgaard, V. L., Skjoldbjerg, S. L. N., Brandl, M., Ejsing, C. S., and Treusch, A. H. (2015). The effects of temperature and growth phase on the lipidomes of Sulfolobus islandicus and Sulfolobus tokodaii. Life 5, 1539–1566. doi:10.3390/life5031539
Jeworrek, C., Evers, F., Erlkamp, M., Grobelny, S., Tolan, M., Chong, P.L.-G., et al. (2011). Structure and phase behavior of archaeal lipid monolayers. Langmuir 27, 13113–13121. doi:10.1021/la202027s
Kawashima, T., Amano, N., Koike, H., Makino, S., Higuchi, S., Kawashima-Ohya, Y., et al. (2000). Archaeal adaptation to higher temperatures revealedby genomic sequence of Thermoplasma volcanium. Proc. Natl. Acad. Sci. U. S. A. 97, 14257–14262. doi:10.1073/pnas.97.26.14257
Koga, Y., and Morii, H. (2005). Recent advances in structural research on ether lipids from archaea including comparative and physiological aspects. Biosci. Biotechnol. Biochem. 69, 2019–2034. doi:10.1271/bbb.69.2019
Koga, Y., Nishihara, M., and Morii, H. (1982). Lipids of alkalophilic bacteria: identification, composition and metabolism. J. UOEH 4, 227–240. doi:10.7888/juoeh.4.227
Komatsu, H., and Chong, P.L.-G. (1998). Low permeability of liposomal membranes composed of bipolar tetraether lipids from thermoacidophilic archaebacterium Sulfolobus acidocaldarius. Biochemistry 37, 107–115. doi:10.1021/bi972163e
Lawaczeck, R. (1988). Defect structures in membranes: routes for the permeation of small molecules. Ber. Bun. Senges Phys. Chem. 92, 961–963. doi:10.1002/bbpc.198800241
Lund, P. A., De Biase, D., Liran, O., Scheler, O., Mira, N. P., Cetecioglu, Z., et al. (2020). Understanding how microorganisms respond to acid pH is central to their control and successful exploitation. Front. Microbiol. 11, 556140. doi:10.3389/fmicb.2020.556140
Macalady, J. L., Vestling, M. M., Baumler, D., Boekelheide, N., Kaspar, C. W., and Banfield, J. F. (2004). Tetraether-linked membrane monolayers in Ferroplasma spp: a key to survival in acid. Extremophiles 8, 411–419. doi:10.1007/s00792-004-0404-5
Michels, M., and Bakker, E. P. (1985). Generation of a large, protonophore-sensitive proton motive force and pH difference in the acidophilic bacteria Thermoplasma acidophilum and Bacillus acidocaldarius. J. Bacteriol. 161, 231–237. doi:10.1128/jb.161.1.231-237.1985
Morii, H., and Koga, Y. (1994). Asymmetrical topology of diether- and tetraether-type polar lipids in membranes of Methanobacterium thermoautotrophicum cells. J. Biol. Chem. 269, 10492–10497. doi:10.1016/s0021-9258(17)34086-3
Nichols, J. W., and Deamer, D. W. (1980). Net proton-hydroxyl permeability of large unilamellar liposomes measured by an acid-base titration technique. Proc. Natl. Acad. Sci. U. S. A. 77, 2038–2042. doi:10.1073/pnas.77.4.2038
Nichols, P. D., and Franzmann, P. D. (1992). Unsaturated diether phospholipids in the antarctic methanogen Methanococcoides burtonii. FEMS Microbiol. Lett. 98, 205–208. doi:10.1111/j.1574-6968.1992.tb05515.x
Oger, P. M., and Cario, A. (2013). Adaptation of the membrane in archaea. Biophys. Chem. 183, 42–56. doi:10.1016/j.bpc.2013.06.020
Pearson, A., Huang, Z., Ingalls, A. E., Romanek, C. S., Wiegel, J., Freeman, K. H., et al. (2004). Nonmarine crenarchaeol in Nevada hot springs. Appl. Environ. Microbiol. 70, 5229–5237. doi:10.1128/aem.70.9.5229-5237.2004
Peeples, T., and Kelly, R. M. (1995). Bioenergetic response of the extreme thermoacidophile metallosphaera sedula to thermal and nutritional stresses. Appl. Environ. Microbiol. 61, 2314–2321. doi:10.1128/aem.61.6.2314-2321.1995
Preiss, L., Hicks, D. B., Suzuki, S., Meier, T., and Krulwich, T. A. (2015). Alkaliphilic bacteria with impact on industrial applications, concepts of early life forms, and bioenergetics of ATP synthesis. Front. Bioeng. Biotechnol. 3, 75. doi:10.3389/fbioe.2015.00075
Quehenberger, J., Pittenauer, E., Allmaier, G., and Spadiut, O. (2020). The influence of the specific growth rate on the lipid composition of Sulfolobus acidocaldarius. Extremophiles 24, 413–420. doi:10.1007/s00792-020-01165-1
Rao, A., de Kok, N. A. W., and Driessen, A. J. M. (2023). Membrane adaptations and cellular responses of Sulfolobus acidocaldarius to the allylamine terbinafine. Int. J. Mol. Sci. 24, 7328. doi:10.3390/ijms24087328
Řezanka, T., Kyselová, L., and Murphy, D. J. (2023). Archaeal lipids. Prog. Lipid Res. 91, 101237. doi:10.1016/j.plipres.2023.101237
Rosa, M. D., Gambacorta, A., Nicolaus, B., Chappe, B., and Albrecht, P. (1983). Isoprenoid ethers; backbone of complex lipids of the archaebacterium Sulfolobus solfataricus. Biochimica Biophysica Acta (BBA) - Lipids Lipid Metabolism 753, 249–256. doi:10.1016/0005-2760(83)90014-0
Salvador-Castell, M., Tourte, M., and Oger, P. M. (2019). In search for the membrane regulators of archaea. Int. J. Mol. Sci. 20, 4434. doi:10.3390/ijms20184434
Schleper, C., Puehler, G., Holz, I., Gambacorta, A., Janekovic, D., Santarius, U., et al. (1995). Picrophilus gen. nov., fam. nov.: a novel aerobic, heterotrophic, thermoacidophilic genus and family comprising archaea capable of growth around pH 0. J. Bacteriol. 177, 7050–7059. doi:10.1128/jb.177.24.7050-7059.1995
Schouten, S., Hopmans, E. C., and Sinninghe Damste, J. S. (2013). The organic geochemistry of glycerol dialkyl glycerol tetraether lipids: a review. Org. Geochem. 54, 19–61. doi:10.1016/j.orggeochem.2012.09.006
Searcy, D. G. (1976). Thermoplasma acidophilum: intracellular pH and potassium concentration. Biochimica Biophysica Acta (BBA) - General Subj. 451, 278–286. doi:10.1016/0304-4165(76)90278-6
Segerer, A., Langworthy, T. A., and Stetter, K. O. (1988). Thermoplasma acidophilum and Thermoplasma volcanium sp. nov. from Solfatara fields. Syst. Appl. Microbiol. 10, 161–171. doi:10.1016/s0723-2020(88)80031-6
Shimada, H., Nemoto, N., Shida, Y., Oshima, T., and Yamagishi, A. (2008). Effects of pH and temperature on the composition of polar lipids in Thermoplasma acidophilum HO-62. J. Bacteriol. 190, 5404–5411. doi:10.1128/jb.00415-08
Siliakus, M. F., van der Oost, J., and Kengen, S. W. M. (2017). Adaptations of archaeal and bacterial membranes to variations in temperature, pH and pressure. Extremophiles 21, 651–670. doi:10.1007/s00792-017-0939-x
Sinensky, M. (1974). Homeoviscous adaptation—a homeostatic process that regulates the viscosity of membrane lipids in Escherichia coli. Proc. Natl. Acad. Sci. U. S. A. 71, 522–525. doi:10.1073/pnas.71.2.522
Slonczewski, J. L., Fujisawa, M., Dopson, M., and Krulwich, T. A. (2009). Cytoplasmic pH measurement and homeostasis in bacteria and archaea. Adv. Microb. Physiology 55, 1–79. doi:10.1016/S0065-2911(09)05501-5
Sprott, G. D., Meloche, M., and Richards, J. C. (1991). Proportions of diether, macrocyclic diether, and tetraether lipids in Methanococcus jannaschii grown at different temperatures. J. Bacteriol. 173, 3907–3910. doi:10.1128/jb.173.12.3907-3910.1991
Ulrih, N. P., Gmajner, D., and Raspor, P. (2009). Structural and physicochemical properties of polar lipids from thermophilic archaea. Appl. Microbiol. Biotechnol. 84, 249–260. doi:10.1007/s00253-009-2102-9
van de Vossenberg, J. L. C. M., Driessen, A. J. M., and Konings, W. N. (1998). The essence of being extremophilic: the role of the unique archaeal membrane lipids. Extremophiles 2, 163–170. doi:10.1007/s007920050056
Wang, X., Lv, B., Cai, G., Fu, L., Wu, Y., Wang, X., et al. (2012). A proton shelter inspired by the sugar coating of acidophilic archaea. Sci. Rep. 2, 892. doi:10.1038/srep00892
Yamauchi, K., Doi, K., Yoshida, Y., and Kinoshita, M. (1993). Archaebacterial lipids: highly proton-impermeable membranes from 1,2-diphytanyl-sn-glycero-3-phosphocoline. Biochimica Biophysica Acta (BBA) - Biomembr. 1146, 178–182. doi:10.1016/0005-2736(93)90353-2
Zeng, Z., Liu, X.-L., Farley, K. R., Wei, J. H., Metcalf, W. W., Summons, R. E., et al. (2019). GDGT cyclization proteins identify the dominant archaeal sources of tetraether lipids in the ocean. Proc. Natl. Acad. Sci. U. S. A. 116, 22505–22511. doi:10.1073/pnas.1909306116
Zeng, Z., Liu, X.-L., Wei, J. H., Summons, R. E., and Welander, P. V. (2018). Calditol-linked membrane lipids are required for acid tolerance in Sulfolobus acidocaldarius. Proc. Natl. Acad. Sci. U. S. A. 115, 12932–12937. doi:10.1073/pnas.1814048115
Zhai, Y., Chong, P. L.-G., Taylor, L. J. A., Erlkamp, M., Grobelny, S., Czeslik, C., et al. (2012). Physical properties of archaeal tetraether lipid membranes as revealed by differential scanning and pressure perturbation calorimetry, molecular acoustics, and neutron reflectometry: effects of pressure and cell growth temperature. Langmuir 28, 5211–5217. doi:10.1021/la300142r
Keywords: proton permeation, thermoacidophiles, bipolar tetraether lipids, membrane volume fluctuations, adaptation
Citation: Chong PL-G (2024) Archaea membranes in response to extreme acidic environments. Front. Biophys. 1:1338019. doi: 10.3389/frbis.2023.1338019
Received: 14 November 2023; Accepted: 13 December 2023;
Published: 04 January 2024.
Edited by:
Olga Vinogradova, University of Connecticut, United StatesReviewed by:
Helgi I. Ingolfsson, Lawrence Livermore National Laboratory (DOE), United StatesCopyright © 2024 Chong. This is an open-access article distributed under the terms of the Creative Commons Attribution License (CC BY). The use, distribution or reproduction in other forums is permitted, provided the original author(s) and the copyright owner(s) are credited and that the original publication in this journal is cited, in accordance with accepted academic practice. No use, distribution or reproduction is permitted which does not comply with these terms.
*Correspondence: Parkson Lee-Gau Chong, pchong02@temple.edu