Behavioral mechanisms underlying trait-mediated survival in a coral reef fish
- 1Division of Marine Biology and Ecology, Rosenstiel School of Marine and Atmospheric Science, University of Miami, Miami, FL, United States
- 2National Oceanic and Atmospheric Administration, National Marine Fisheries Service, Office of Habitat Conservation, Silver Spring, MD, United States
- 3Department of Ecology and Evolution, University of California, Los Angeles, Los Angeles, CA, United States
- 4Department of Biological Sciences, Louisiana State University, Baton Rouge, LA, United States
- 5Department of Integrative Biology, Oregon State University, Corvallis, OR, United States
Fast growth and large size generally increase survivorship in organisms with indeterminate growth. These traits frequently covary, but where they do not, trade-offs often exist in the behavioral choices of organisms. Juvenile bicolor damselfish Stegastes partitus that settle on coral reefs at larger sizes generally experience enhanced survivorship but have slower juvenile growth rates. We hypothesized that differences in behavior may mediate this trade-off. To test whether it is trait-related behaviors or the traits themselves that enhance early survival, we combined individual behavioral observations with otolith (ear stone)-based daily growth measurements for juvenile S. partitus in the Florida Keys. Foraging, sheltering, and chasing behaviors of 256 fish were measured during 5 different months (2008–2009), and patterns of differential survival were similar to those from a 6-year (2003–2008) recruitment time series. We found a trade-off between sheltering and foraging that significantly explained patterns in size-at-settlement: damselfish that settled at larger sizes spent less time sheltered and more time feeding high in the water column. Juvenile growth rates were unrelated to any of the sheltering–foraging behaviors but instead were inversely related to adult conspecific density. Damselfish that settled near higher densities of conspecifics were subjected to increased territorial chasing. Chasing intensity interacted with settlement size such that large juveniles who were chased more frequently exhibited slower growth rates, whereas smaller settlers did not experience this energetic cost. Thus, the dominant survival strategy of S. partitus is to settle at a large size and spend more time foraging high in the water column while dodging conspecifics at an energetic cost to their growth rates. Size-at-settlement is determined during the larval period and after settlement, this trait is key to subsequent behaviors and the strength of trait-mediated survival. Understanding how somatic growth, body size, and survival are intertwined in early life is necessary to help explain population dynamics.
1 Introduction
Predation is one of the major selective forces shaping the form and behavior of animals (1). This interaction is particularly true of the minuscule early life stages of marine organisms, which frequently endure high mortality rates due to predation (2, 3). Individuals with morphologies or behaviors that facilitate avoidance of or escape from predators will have a greater probability of surviving to reproductive maturity and increasing their fitness.
The growth-mortality hypothesis (4), or as it has been recently renamed, the growth-survival paradigm [GSP; (5)], provides a theoretical framework for evaluating which traits may be selectively removed from a population by predators. Focused on the youngest life stages when all animals are most vulnerable to predation, the paradigm posits that individuals that grow faster, attain larger sizes-at-age, or have reduced early life stage durations experience enhanced survival. Much evidence has accumulated that suggests that this pattern may indeed be the case for a diversity of taxa (6), including fishes [e.g., (7–9)]. However, fast growth is also known to be associated with reduced fitness (10–14), and several potential trade-offs with accelerated growth may explain why slower growth can be beneficial. For example, rapid growth may be attained at the expense of development, tissue maintenance, and repair, as well as, for fish, swimming capabilities (6). Compromise of these functions could result in a reduced ability to escape predation.
Conflicting field and laboratory results for different fish species over the past four decades have created some debate about the generality of the GSP. A recent review reconsidered the empirical evidence for the GSP and proposed a new synthetic framework that encompasses a range of patterns of selective survival (5). While the GSP and this new framework include reference to behaviors underpinning trait-based predation, it is challenging to document behaviors that are linked directly to survival.
Behavior likely mediates the relationship between growth, size, and vulnerability to predation. For instance, increased foraging activity or selection of certain foraging habitats may result in accelerated growth as well as greater visibility to predators (15–17). These trade-offs have been proposed as drivers of the evolution of submaximal growth rates in some taxa (15, 18). Additionally, initial size may further interact with behavior to influence growth and survival. For example, smaller fish are more vulnerable to gape-limited predation and therefore may be more wary and shelter more often or for longer durations (2). However, smaller individuals also typically have lower fat reserves, higher weight-specific metabolic requirements, and larger drag coefficients and, therefore, may need to spend more time foraging than larger individuals (19, 20).
For a common western Atlantic reef fish, the bicolor damselfish, Stegastes partitus, individuals that preferentially survived the first few weeks on the reef (i.e., post-settlement) were those that were larger at settlement but had slower juvenile growth rates during the first week of life on the reef (14). A similar relationship between juvenile growth and survival was observed in older juveniles of this species in the Bahamas (21), and field experiments demonstrated that increased intraspecific chases reduced juvenile growth rates (22). Slower juvenile growth is contrary to both the current paradigm (4) and to observations of survival in other western Atlantic reef fishes [e.g., (8, 23)]. The objective of the present study was to identify the behavioral mechanisms underlying these relationships among settlement size, juvenile growth rate, and survivorship.
While we have previously documented the selective survival of large S. partitus settlers who grew more slowly as juveniles (14), as well as changes in the patterns of selective survival with water temperature and lunar phase (14, 24), we were only able to speculate on the underlying behavioral mechanisms. In the present study, to tease apart the role of the early life history traits and to test the hypothesis that survival-related behaviors underlie these selective patterns, we observed early juvenile behaviors of multiple cohorts of naturally settled juvenile S. partitus and coupled these with otolith-derived measurements of size and growth from the same individuals. Although otolith microstructure analysis is a common tool used to elucidate patterns in growth and mortality during the early life stages of fishes [e.g., (25)], it has rarely been used in conjunction with field observations to link behavior with growth [but see, for example (26, 27)]. Otolith microstructure analysis enables the estimation of daily size and growth rate at any point in the life history of an individual and the comparison of such traits at common points in the life of individuals who vary in their behavioral traits and survivorship.
2 Methods
2.1 Study species and site
The bicolor damselfish Stegastes partitus maintains benthic territories on spur and groove reefs in the Caribbean and western Atlantic (28), including dead coral rubble piles at the reef base (24). Adult and juvenile S. partitus are diurnal planktivorous that consume a range of planktonic cyanobacteria (Trichodesmium sp.) and other zooplankton along with limited amounts of benthic algae (29, 30). Territories are important for acquiring food, shelter, and eventually mates, and are defended against conspecifics and potential predators. Adults are benthic brooders, with males guarding the embryos for ~4 days until hatching (31). Larvae have a pelagic larval duration of ~1 month, after which they arrive to nearshore reefs in multiday pulses generally during the dark half of the lunar cycle (32, 33). Larvae settle onto reef and rubble areas, metamorphosing into juveniles overnight, a transition that is recorded in their otoliths (34). Daily deposition of material on otoliths provides a continuous chronological record of size and growth. S. partitus is an ideal model species for studying relationships among size, early juvenile growth, and behavior because they are abundant, easy to sample as benthic juveniles, and remain strongly site-attached following settlement. All observations and measurements for the present study were obtained from newly settled fish in the upper Florida Keys. The main predators of S. partitus juveniles are unknown, but barracuda (Sphyraena barracuda), bar jack (Caranx ruber), and cero (Scomberomorus regalis) have been observed to be preying on adults (29). Gut contents indicate that S. partitus are also preyed upon by trumpetfish (Aulostomus maculatus) but not muraenids or other nocturnal fishes (35). More recently, S. partitus juveniles and adults have been shown to be readily consumed by the invasive Indo-Pacific lionfish (36–38).
2.2 Behavioral observations
Behavioral observations of recently settled S. partitus (n = 256) were made in July, August, and September 2008 and in May and July 2009 on Sand Island Reef (25°01.09′N, 80°22.08′W) and Snapper Ledge Reef (24°58.92′N, 80°25.30′W) at an average depth of 8 m (range 5–12 m). Observations were conducted each day between 1,030 and 1,430 h, the peak period of activity for newly settled S. partitus (Rankin and Sponaugle, unpublished pilot study), and this time period is consistent with general feeding patterns of the species (39). Furthermore, as boldness and aggression have been shown to be influenced by small within-day changes in water temperature in another damselfish Pomacentrus moluccensis (40), the restriction of the time of day that observations were conducted minimized the effects of daily temperature fluctuations.
For all observations, a pair of SCUBA divers would haphazardly locate settled juveniles, position themselves ~3 m away, and allow 2 min for the subject(s) to acclimate to their presence, comparable to other in situ behavioral studies of juvenile coral reef fish (41, 42). The divers would then observe the subject for 5 min, and using a timer, stopwatch, and data sheet, record for each fish: (1) total time spent sheltered, (2) number of times shelter was sought, maximum distance travelled (3) horizontally, and (4) vertically from primary shelter. Sheltering was defined as the period when the fish was under or within the crevices of coral or rubble, often hidden from view. Maximum distance traveled horizontally and vertically was noted during the observation period and then measured using a pair of weights and a tape measure afterward. Since the number of adults surviving can be used as a proxy of habitat quality and potential aggressive pressure, we also visually estimated (5) density of adult conspecifics within a 1.5-m radius (7 m2 area) around each fish's primary shelter, which is greater than the average territory size of 1 m2 (43) but within the range of how far conspecifics will travel to each other (44). In addition to these metrics, in 2009 (May and July), divers recorded for each fish (6) the number of times an individual was chased by a conspecific (n = 168) and (7) the number of bites an individual took in the water column (n = 46). Separately, and for a limited number of fish (n = 15), divers measured (8) burst swim speed in response to a predator. For this measurement, a model of a predator was used to elicit an escape response, and this was videotaped using a stereo camera at a frame rate of 30 s−1 to estimate escape swimming speeds. Digital images were processed with VideoMach (Gromada v. 5.5.3) and analyzed in Image Pro Plus (Media Cybernetics v. 4.5). All fish were collected after observations using quinaldine and MS-222 and stored in 95% ethanol to enable the comparison of behavioral observations with otolith-derived traits.
2.3 Otolith processing
Standard procedures were used to digitally measure the standard length (SL) of each fish, dissect, and clear (in immersion oil) their otoliths and read, innumerate, and measure increments (at 400X) along the longest axis of the clearest lapillus [as per (45)]. All readings were made using an oil immersion 40X lens attached to a Leica DMLB microscope equipped with a polarized filter between the first stage and light source. Otolith images were captured by a Dage MTI video camera and analyzed using Image Pro Plus (Media Cybernetics v 4.5). Each otolith was read (increments enumerated) once blind (i.e., without sample information available) and saved as a digital file. Readings were validated as per Searcy and Sponaugle (8). Otolith microstructure analysis was used to determine juvenile age (number of concentric increments following the settlement mark), mean juvenile growth rates (average of post-settlement otolith increment widths, in μm/day), and size-at-settlement (otolith radius-at-settlement, in μm).
2.4 Data analysis
2.4.1 Comparison of traits with 6-year recruitment time series
To set the context for the study, we first re-analyzed a 6-year (2003–2008) time series of S. partitus recruitment (24). In that study, the otoliths of a representative sample of newly settled juveniles were analyzed to obtain individual age and daily growth rates. While these data were used to examine lunar cyclic patterns in larval and settlement traits (24), the present analysis focused on how average early life history traits change over time among young juveniles via differential survival. Our goal for this comparison was to ensure the data used in the present study aligned with the larger patterns evident in the 6-year dataset.
Rankin and Sponaugle (24) separated juveniles into two age groups—young fish: 2–10 days old post-settlement and older fish, or survivors 11–29 days old post-settlement—and found significant differences between the age groups with respect to size-at-settlement and juvenile growth rate (measured over the first 2 days of juvenile life). We reproduced that finding to compare to the present dataset (2008–2009 data), which has fewer fish, especially in the older juvenile group since the focus of the collection was the youngest recruits. We separated the fish into similar age groups and used the Mann-Whitney U tests to compare age groups for each dataset. Because the number of fish in the older age group in the present study was low, we also re-analyzed the time series using age as a continuous variable. To avoid data loss and maximize the relevance of the present dataset, we chose to use the entirety of the juvenile growth record (minus the last incomplete increment from the day of collection). We note that the mean juvenile growth rate during the first 2 days and mean growth rate over the entire juvenile period were significantly correlated (Pearson correlation, r = 0.87, n = 814, p < 0.001; Supplementary Figure S1); thus, thereafter, we used mean juvenile growth rate over the entire juvenile period.
We next examined whether there were individual-level trade-offs between settlement size and juvenile growth rate in both datasets. Since young fish in our dataset represent an “initial” population prior to post-settlement selective pressures, and older fish are those who have survived selective mortality, i.e., “survivors”, we expected to see a relationship between settlement size and juvenile growth rate in older fish but not necessarily in the younger fish. Thus, we included an interaction term between settlement size and juvenile age for predicting juvenile growth rate, using a linear mixed model with collection site as a random intercept.
2.4.2 Otolith-derived early life history traits and temperature
Temperature has been shown to influence growth-related early life history traits in S. partitus (14). Therefore, we controlled for the temperature that fish experienced during their juvenile period. Daily water temperature values were obtained from the NOAA Buoy MLRF1 and averaged for each fish across their actual days of life. We used average temperature experienced during the juvenile period to predict juvenile growth rate and used the residuals from this linear regression as a temperature-standardized measure of juvenile growth rate.
2.4.3 Behavioral observations and relationships to traits
To test for associations between the two main traits (settlement size and juvenile growth) and different behaviors, we planned to run a principal component analysis (PCA) on all of the observed behaviors. Unfortunately, bite count was measured for only a portion of the fish in 2009 (n = 46), so using it in the PCA would have substantially reduced our sample size. Instead, we examined those 2009 fish for a relationship between the number of bites and maximum vertical distance in the water column. Indeed, the number of bites by a fish in the water column was significantly related to the maximum vertical distance a fish traveled in the water column; thus, we assume for our purposes that the inclusion of maximum vertical distance is associated with foraging success (Supplementary Figure S2; Supplementary Table S1). We also did not include the number of times chased by conspecifics in the PCA, since being chased is a behavior not instigated by the individual itself and may be a consequence of behaviors we included in the PCA. Horn's parallel analysis for component retention (46) indicated that we should use three principal components in the analysis.
To examine the relationship between juvenile fish behavior and the two primary early life history traits (settlement size and juvenile growth rate), we constructed three different hypotheses in the form of directed acyclic graphs (DAGs) that explain how each trait of interest relates to survival (approximated by juvenile age). In the first hypothesis, settlement size affects survival directly (e.g., through gape-limited predation) and/or indirectly via behavior. Settlement size also affects juvenile growth rate directly and/or indirectly via behavior. Juvenile growth rate in turn affects survival. In the second hypothesis, adult density affects juvenile growth and survival directly (e.g., through competition, or because higher density indicates better habitat quality) and/or indirectly via the number of times an individual is chased by conspecifics. This second hypothesis was developed from observations that adult density and increased intraspecific chasing reduce growth in experimental fish (22). We factored in size-at-settlement as relative size may interact with chasing behavior to affect juvenile growth. We also included the possibility that fish behaviors (e.g., sheltering) could affect how often a fish is chased. Finally, the third hypothesis describes an energetic trade-off between swimming behavior (speed; measured for a small subset of fish) and juvenile growth that could arise if fast-growing fish invest in size over other important developmental and morphological processes, resulting in fast growers who swim more slowly. Slower growers are potentially faster swimmers and, thus, better able to escape predation; therefore, they experience higher survivorship.
To assess all direct and indirect relationships represented in the DAGs, we used confirmatory path analysis (47). We represented each path with a linear mixed (LMM) or Poisson-distributed generalized linear mixed model (GLMM; when the response variable was count data, such as number of times chased), with the collection site as a random intercept. In several cases, we were unable to fit a model with random effects due to singularity issues; in such instances, we fitted a simpler model without the random effect term. We then used psem from piecewiseSEM (48) to assess the structure of the DAG by quantifying path coefficients for all hypothesized relationships and testing the goodness of fit of the model. All data processing and statistical analyses were conducted in R version 4.2.1 (49). We used functions from the lmerTest package (50) for LMMs and the glmmTMB package (51) for GLMMs.
3 Results
3.1 Comparison of traits with 6-year recruitment time series
Comparison of the present dataset with a longer 6-year recruitment time series yielded very similar patterns (Figure 1). Older juveniles, who had survived longer, had settled larger (Figures 1A, B) and grew more slowly during their first 2 days on the reef (i.e., post-settlement; Figures 1C, D). The shorter and longer datasets had the same significant pattern of directional selection in early juvenile growth (Mann-Whitney U: p = < 0.001–0.01), and the same trend in settlement size. Likely due to low sample sizes in the present study (i.e., fewer older individuals), the pattern of larger settlers selectively surviving was not significant (Mann-Whitney U: p = 0.13). Using age as a continuous variable in generalized additive models to increase the power of the trait analysis provided a clearer view of the age-related changes in traits (settlement size in the 6-year dataset and juvenile growth in both datasets: p = < 0.001– < 0.01; Supplementary Figure S3), though the trend of larger settlement size among older survivors in the present smaller dataset remained nonsignificant (p = 0.27).
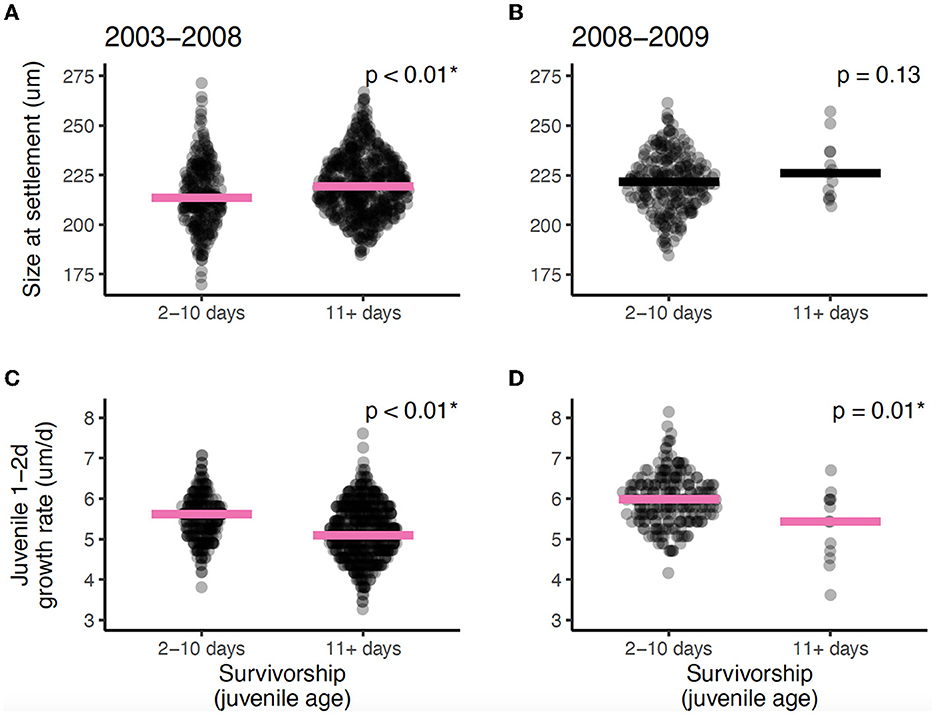
Figure 1. Comparison of early life traits between young and older juveniles for Stegastes partitus data in the (A, C) 6-year (2003–2008) recruitment time series from Rankin and Sponaugle (24), and in the (B, D) present study (2008–2009). Fish age groups were defined similarly in each data set (young fish = 2–10 days old post-settlement; older fish = 11–29 days post-settlement). Settlement size (A, B) was derived from the position of the settlement mark on otoliths of individual fish; juvenile growth (C, D) was similarly computed by averaging daily otolith increment widths for the first 2 days of life on the reef. Pink lines indicate significantly different medians; black lines are non-significant but included to facilitate visualization. *indicates significance (P < 0.05).
In both datasets, there was a significant interaction between settlement size and juvenile age for predicting juvenile growth rate: settlement size and juvenile growth rate became increasingly negatively associated with age (i.e., survival) (current dataset: conditional R2 = 0.16, marginal R2 = 0.14, pinteraction = 0.015; 6-year dataset: conditional R2 = 0.26, marginal R2 = 0.26, pinteraction = 0.011; Figure 2; Supplementary Tables S2, S3).
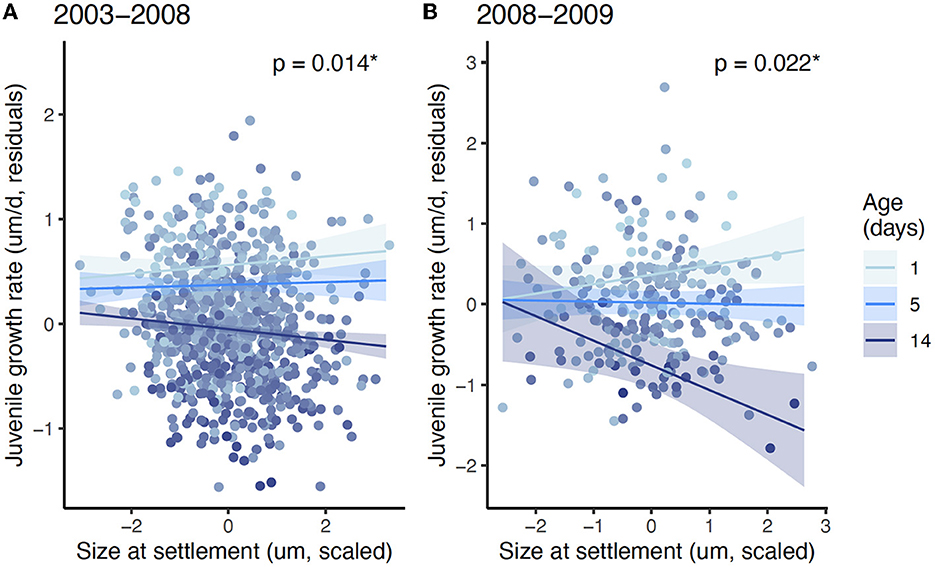
Figure 2. Mean juvenile growth rate as a function of settlement size for juvenile Stegastes partitus sampled as part of the (A) 6-year (2003–2008, n = 818) time series and in the (B) present dataset (2008–2009, n = 256). Data points are traits from individuals color-coded by juvenile age. Lines depict the significant interaction between settlement size and age (A: Linear mixed model; B: Linear model; Supplementary Tables S2, S3), with selected ages spanning the range of ages in the present dataset. *indicates significance (P < 0.05).
3.2 Behavioral observations and relationships to traits
The first axis (PC1) from the PCA of measured behaviors on the full dataset (n = 256) explained 41.9% of the variance and separated fish based on their sheltering vs. foraging and other swimming behavior (Figure 3). Positive loading on PC1 represented fish that spent more time sheltering and did not travel long distances. PC2 and PC3 explained less variance (21.8 and 20.7%, respectively) but did not capture how long fish sheltered but rather how they sheltered (Supplementary Figure S4). In both of these PCs, mean shelter time and the number of times sheltering loaded in opposite directions. Mean shelter time increases when a fish is sheltering less often, but for a long time period, as opposed to many short sheltering periods. Thus, this trade-off can be interpreted as having long, infrequent bouts of sheltering vs. short, frequent bouts of sheltering. PC2 can thus be interpreted as separating fish that travel far and shelter fewer times (but for longer) vs. fish that do not travel far and shelter for short, frequent periods. PC3 separates fish that travel far horizontally and return frequently to shelter for short periods from those that do not travel far and remain sheltered for long periods (Supplementary Figure S4).
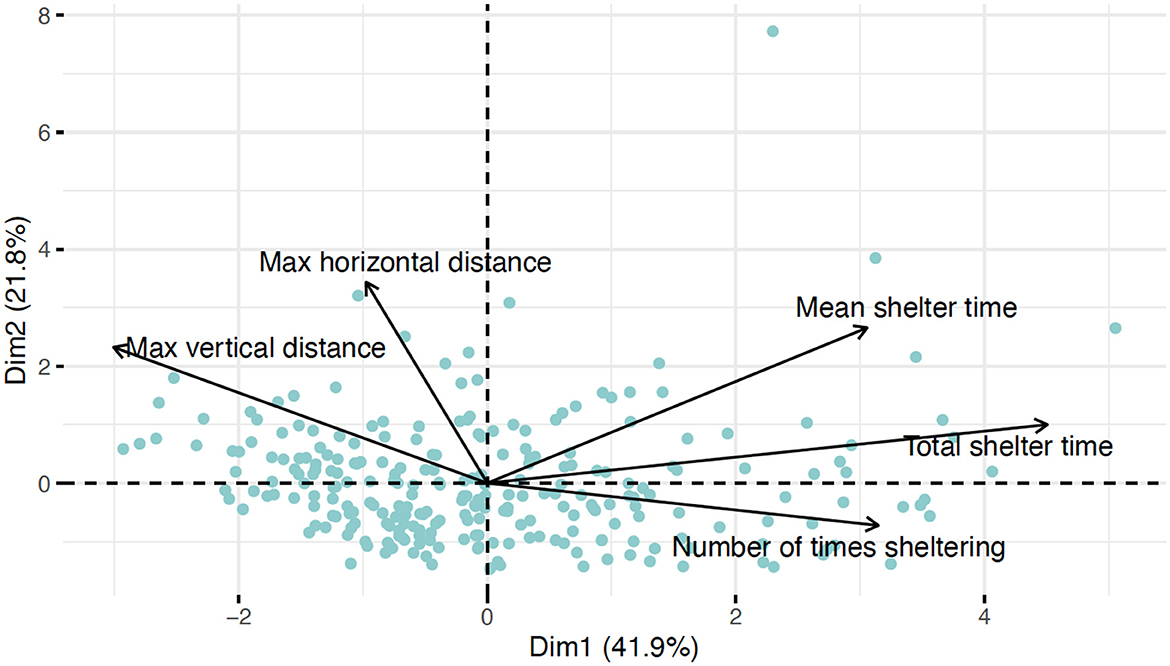
Figure 3. Primary dimensions (PC2 vs. PC1) in principal component analysis (PCA) of behaviors observed over 5 min for newly settled Stegastes partitus juveniles (n = 256). Observations were made over 5 different months in the Florida Keys. Behaviors are mean and total time spent sheltered, number of times shelter was sought (number of times sheltering), and maximum distance traveled horizontally (max horizontal distance) and vertically (max vertical distance) from the primary shelter.
3.2.1 Settlement size
PC1, the measure of time spent sheltered vs. time spent foraging, was negatively related to size-at-settlement (LM: p = 0.008; Figures 4A, B, Supplementary Table S4), with larger fish spending less time hiding and more time traveling both horizontally and vertically in the water column. Settlement size did not predict PC2 but did predict PC3 (LM: p = 0.59 and LMM: p = 0.04, respectively; Figures 4A, C, D; Supplementary Table S4). Interestingly, both PC1 and PC3 were significantly associated with survival (increasing juvenile age), and settlement size did not predict survival when controlling for these behavioral axes (Figure 4E; Supplementary Table S4). In other words, the associations between PC1 and survival and between PC3 and survival are not simply spurious correlations that arise because they are each associated with settlement size. Instead, size-at-settlement is related to survival through size-related behaviors (Figure 4A). In an analysis with a smaller dataset (n = 168), relationships between behaviors and survival were harder to distinguish (due to reduced power), and the relationship between PC3 and survival was not significant (Figure 5E). However, there was a positive relationship between PC2 (distance traveled and frequency of sheltering) and survival.
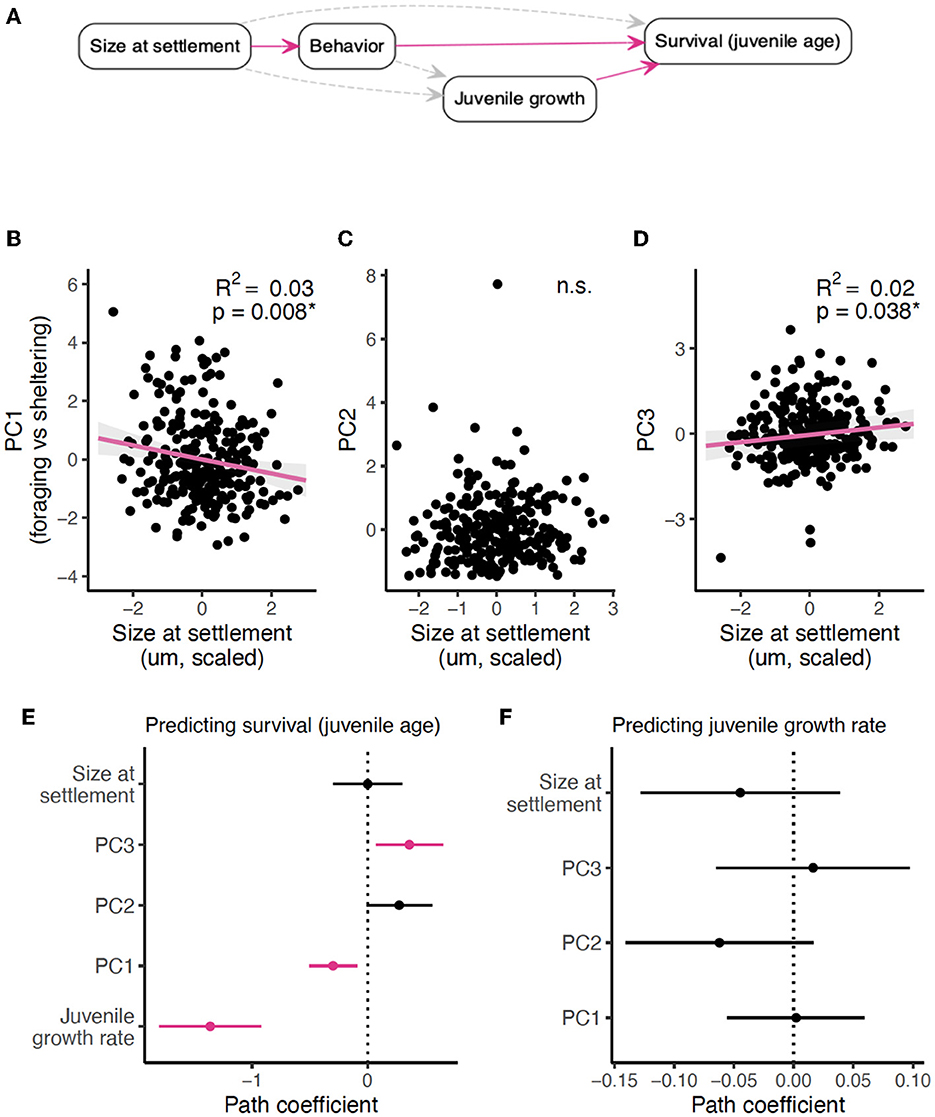
Figure 4. Confirmatory path analysis of Stegastes partitus settlement size, juvenile growth, and survival (n = 256). (A) Directed acyclic graph (DAG) outlining alternative hypotheses of how settlement size relates to survival (approximated by juvenile age) and juvenile growth rate: settlement size affects survival and juvenile growth rate directly and/or indirectly via behaviors. Solid pink arrows indicate significant relationships; gray dashed arrows indicate the relationships we investigated and found them to be non-significant. (B–D) Relationship between principal components and settlement size from a linear model (B) or from linear mixed models (C, D). Marginal R2-values are included to describe the variance captured by the main effects and interaction terms of each model. (E) Path coefficients from the component model of survivorship (juvenile age) of Stegastes partitus as a function of settlement size, juvenile growth rate, and all PCs. (F) Path coefficients from component model of juvenile growth rate as a function of settlement size and all PCs. (E, F) Pink indicates significant relationships at a p-value of <0.05; black indicates non-significance (p > 0.05). For standardized path coefficients, see Supplementary Table S4. For R2-values of all component models, see Supplementary Table S5. For PCA biplots showing how the measured behaviors load on each axis, see Figure 3 and Supplementary Figure S4. *indicates significance (P < 0.05). n.s. indicates non-significant.
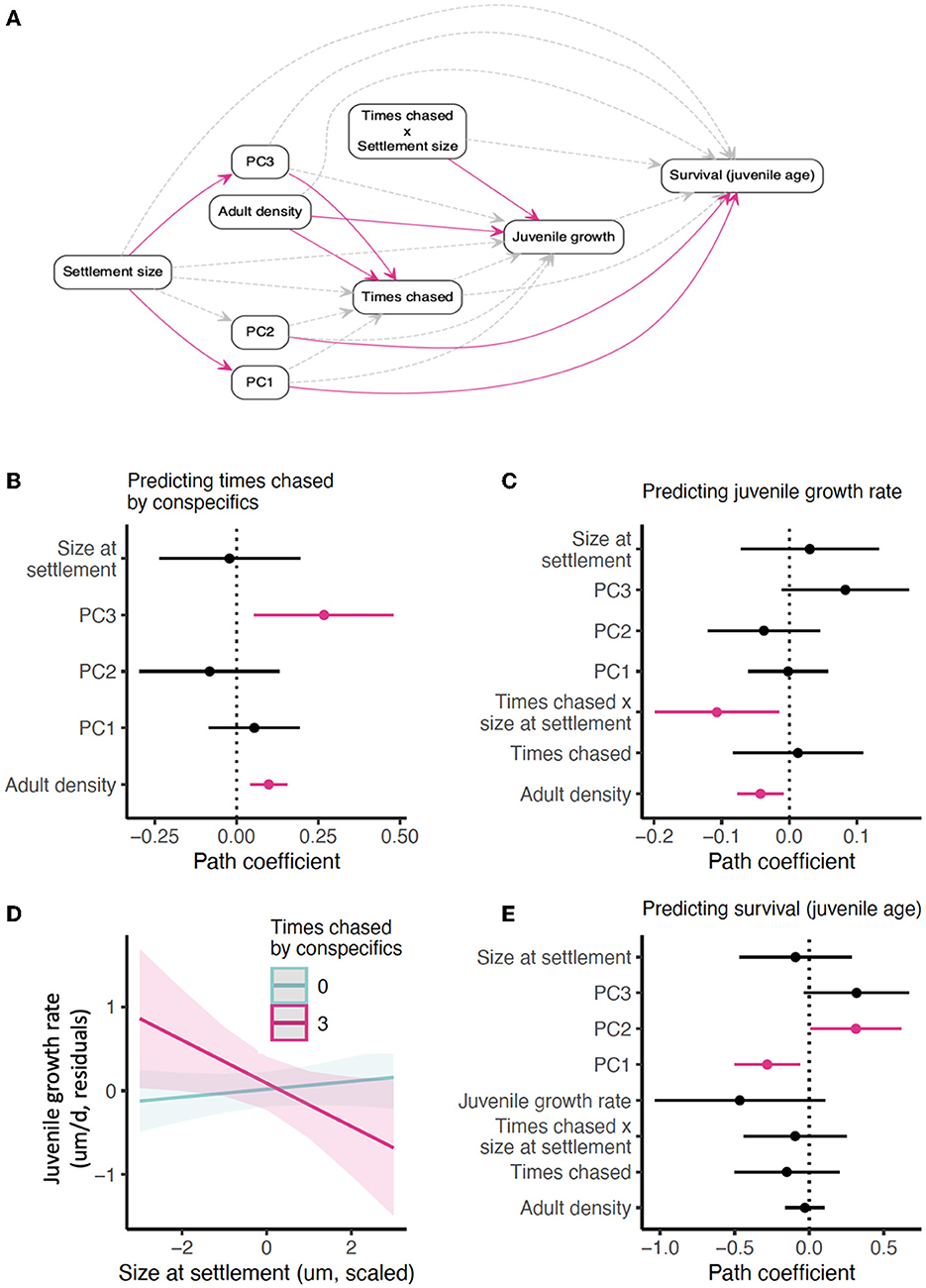
Figure 5. Confirmatory path analysis of Stegastes partitus adult density (habitat quality; aggressive pressure), chasing behaviors, and relationship to juvenile growth rate (n = 168). (A) Directed acyclic graph (DAG) outlining alternative hypotheses of how adult S. partitus density relates to juvenile growth rate and survival. Adult density could affect juvenile growth rate by impacting the frequency of conspecific chasing, or through another mechanism. Adult density may also directly impact survival, such as if the presence of adults indicates higher quality habitat, or indirectly through increased chasing. The effect of chasing on both growth and survival could depend on settlement size. Furthermore, foraging and sheltering behaviors could predict how frequently fish are chased by conspecifics. (B) Path coefficients from component model of adult density, settlement size, and behavioral axes on the number of times an individual was chased. (C) Path coefficients from the component model of adult density, behavioral axes, number of times chased, and the interaction of chased times and settlement size on juvenile growth rate. (D) Visualization of the interaction between the number of times chased and settlement size for predicting juvenile growth rate; 0 or 3 times chased were selected for simplicity of visualization. (E) Path coefficients from the component model of the same variables on survivorship (juvenile age). In the DAG, the solid pink line is a significant relationship; the gray dashed line indicates the lack of a significant relationship. (B, C, E) Pink color indicates a significant relationship from linear mixed models at p < 0.05; black color indicates non-significance (p > 0.05). For standardized path coefficients, see Supplementary Table S6. For R2-values of all component models, see Supplementary Table S7. For PCA biplots showing how the measured behaviors load on each axis, see Figure 3 and Supplementary Figure S4.
3.2.2 Juvenile growth
Although settlement size–related behaviors influenced juvenile S. partitus survival, surprisingly, the same behaviors captured by the PCA dimensions did not predict variation in juvenile growth rate (Figure 4F; Supplementary Table S4). In fact, settlement size neither directly nor indirectly (via the behaviors in the PCA) predicted juvenile growth rate (Figures 4A, E, F; Supplementary Table S4). Instead, when we considered fish for which we had data on chasing behavior (n = 168), settlement size affected juvenile growth rate through an interaction with how often fish were chased by conspecifics (Figures 5A, C, D; Supplementary Table S6). Juvenile growth was unrelated to settlement size where no chases were observed, but when fish were chased multiple times during the 5-min observation period, juvenile growth became a negative function of settlement size—larger fish at settlement experienced slower juvenile growth, the dominant emergent relationship of surviving S. partitus (Figure 2). We also found that PC3, which captured far horizontal travel with frequent, short bouts of sheltering, predicted the number of times fish were chased by conspecifics.
As shown previously for this species (22), adult density was negatively associated with juvenile growth (Figure 5C; Supplementary Table S6). Adult density was also directly related to the number of times an individual was chased (Figure 5B; Supplementary Table S6). Despite these relationships, adult density and the interaction of chasing and settlement size did not predict survival (Figure 5E; Supplementary Table S6). Furthermore, the significant relationship we found between juvenile growth and survival in the full dataset model (Figures 4A, E; Supplementary Table S4) disappeared in this model (Figures 5A, E; Supplementary Table S6). We note, however, that we had low power to detect relationships between measured variables and juvenile survival in this data subset, since this subset had fewer older fish than the full dataset (only three fish were 11+ days old and nine fish were 9+ days old, compared to 11 and 23 fish, respectively, in the full dataset).
Unfortunately, swim speed was only measured in 15 fish and since they were the same age when collected, we could not assess how swim speed related to survival. The negative correlation between swim speed and juvenile growth was not significant, though we note that we had low power for this analysis (Supplementary Figure S5B).
4 Discussion
For larval fishes to successfully recruit to adult populations, they need to survive the highly dangerous period of early life. Predators are known to target prey with particular traits [e.g., small sizes and slow growth; (4)]. Thus, settlers with particular traits may be more or less successful at navigating the hazardous first few weeks of life on the reef, potentially influencing the overall strength of recruitment to the population. The growth-survival paradigm [GSP; (5)] describes how we might expect the characteristics (or traits) of young fish to change over time due to predation pressure, but it is typically challenging to identify the mechanisms underlying observed shifts in traits. By combining otolith microstructure analysis and direct observations of behavior in individual fish, we have been able to pinpoint early life processes that create variation in the characteristics of survivors.
4.1 Consistency of behavioral dataset with a 6-year recruitment time series
Previous studies with our focal species, the bicolor damselfish, S. partitus, demonstrated that juveniles who settled at larger sizes and had slower juvenile growth experienced higher survival during the first few weeks of life on the reef (14, 21, 22, 24). While the differential survival of fish with large settlement sizes is consistent with the GSP, the success of slow growers is not. To identify the behaviors underlying this trait selection, we measured behaviors for 256 fish and combined them with otolith-derived early life history traits. This dataset exhibited the same patterns in trait distributions over time as a 6-year monthly time series of recruitment in the Florida Keys (24), justifying the use of this smaller dataset in examining behavior in the context of growth-related traits.
4.2 Relationship between settlement size and juvenile growth
For data from both the present study and the larger 6-year recruitment time series, comparison of mean juvenile growth as a function of size-at-settlement revealed clear patterns associated with juvenile age. The relationship between the two traits changed from no relationship among the youngest juveniles to a significant inverse relationship among the oldest juveniles. Thus, this relationship is not inherent to the species or individual but instead emerges as a result of selective survival. While there was variation among individuals with regard to both variables, surviving older juveniles on average were those who settled at larger sizes and then had slower early juvenile growth. Although this relationship was not examined explicitly in previous studies, the inverse relationship among the traits of survivors is consistent with previous studies (14, 21, 22, 24).
Size-at-settlement of S. partitus is determined during the larval period by larval growth rates and the number of days larvae spend growing at this rate in the plankton [i.e., pelagic larval duration, PLD; (14, 24)]. Faster-growing damselfish larvae spend less time in the plankton (shorter PLD) and vice versa, with both traits “carrying over” to affect size-at-settlement. Regardless of seasonal fluctuations in water temperature or cyclic lunar phases, the size of settling S. partitus larvae sets the stage for juvenile survival (14, 24). Similar carry-over phenomena are common in other coral reef fishes with complex life histories [e.g., (52, 53)].
4.3 Behaviors underlying selective changes in early life history traits
A PCA of the dataset in the present study (n = 256) enabled us to collapse multiple observed behaviors into a single behavioral variable. PC1 captured the variance associated with the relative time fish spent sheltering vs. the maximum distances fish swam in the vertical and horizontal dimensions. Maximum vertical distance was also positively associated with the number of bites in the water column, thus serving as an indication of foraging success. Fundamentally, PC1 reflected the trade-off between foraging (planktivory) and sheltering. When fish are sheltered under a coral or other reef rubble, they are unable to feed on their preferred diet in the water column (29, 43).
We found a significant relationship between settlement size and the sheltering–foraging axis of behavior (PC1). Bicolor damselfish settling at larger sizes had lower PC1 scores, indicating reduced sheltering behavior and increased foraging high in the water column. Furthermore, PC1 changed with juvenile age: survivors (old fish) had significantly lower PC1 scores, suggesting progressive removal of fish with high PC1 scores. Settlement size alone (i.e., controlling for PCs) did not predict survival. Together, these results point to the behaviors that underlie observed patterns of selective mortality. Larger settlers do not need to shelter as much as smaller settlers and were able to forage higher in the water column, obtaining more zooplankton prey. With previous experiences being equal, larger settlers should be less vulnerable to gape-limited predation (2, 4, 54) and should therefore have the benefit of sustaining greater activity. A similar pattern has been observed in Great Barrier Reef pomacentrid Pomacentrus aboinensis, where larger juveniles not only exhibited greater rates of foraging activity and aggression but also experienced higher survivorship (27). Larger lemon damselfish, Pomacentrus moluccensis, in Indonesia also sheltered less frequently (55). Theoretical (6, 16) and empirical (56, 57) evidence suggests that smaller settlers should spend more time foraging and less time sheltered to accelerate their growth and “catch up” to their larger conspecifics, even though this should increase their exposure to visual predators. Instead, and consistent with other damselfishes, we observed the contrary: juvenile S. partitus that were smaller at settlement spent more time sheltered and traveled less far from those shelters.
Greater activity of larger settlers suggests that they are “bolder”, a trait that has been associated with higher survival in some species (58–61). While, for some animals, bold individuals may access greater rewards (e.g., resources and mates), their increased activity may expose them to a higher probability of predation [e.g., (62, 63)]. For taxa similar to the fish species in our study, the reverse may be true. For instance, Fuiman et al. (60) found that juveniles of another pomacentrid, Pomacentrus wardi, with reduced escape swimming speeds and lower boldness were more susceptible to predation. They suggested that boldness is a form of vigilance that reduces the risk of a predator attack, therefore increasing overall survival. McCormick et al. (61) found that the selection of the white-tailed damselfish Pomacentrus chrysurus was related to a suite of behavioral traits associated with boldness. Whether boldness is a trait that reduces vulnerability to predation or simply covaries with other traits that increase survivorship is unknown.
4.4 Adult density, conspecific chasing, and juvenile growth
If larger settlers forage more than smaller settlers, why is higher survival associated with slower early juvenile growth? Johnson (22) found that S. partitus grew more slowly in areas of high adult density. We examined this in our full dataset and found the same relationship: juvenile growth decreased significantly with increasing adult density. However, surprisingly, juvenile growth was unrelated to any sheltering or foraging behaviors. Larval settlement into an area of the reef with high adult densities implies that the habitat is favorable (can successfully support higher numbers of conspecifics). While this area may be favorable for some reason (higher food supply, fewer predators, higher vigilance possible, etc.), settling in proximity to adult conspecifics also brings with it an increasing likelihood of being chased by conspecifics. Johnson (22) found that more intraspecific chasing was associated with reduced juvenile growth for S. partitus in the Bahamas. Being chased requires evasion and burst swimming by the chased, typically smaller fish, followed by quick sheltering. Such escape behavior disrupts an individual's foraging and requires energy expenditure. Agonistic behavior, such as chasing, can be physiologically costly (64) due to the build-up of lactic acid, production and release of stress hormones (64, 65), injury (66), and energy expenditure (67). Repeated chasing would likely cause reduced growth in the fish being chased.
In our dataset that included chasing measurements, the number of times an individual was chased increased significantly with adult density, but this variable alone did not directly reduce juvenile growth. Instead, the number of times chased interacted with size-at-settlement to predict juvenile growth rate. Individuals who were not chased did not experience a trade-off between size and juvenile growth. Among fish who were chased, larger fish had slower juvenile growth rates and smaller fish had faster growth rates. This result for large fish is not surprising, as evading frequent chasers would require energy expenditure. More surprising is that small settlers who were chased frequently did not experience reduced juvenile growth rates. For those fish, it is possible that chases were of shorter intensity/duration, reducing the energetic cost of this evasive behavior or evasive movement of smaller fish simply does not cost as much energetically, such that these smaller fish are able to maintain relatively high juvenile growth rates.
We also found fish that were chased more were those who traveled farther horizontally, sheltering frequently but for brief periods of time. This behavior, captured by PC3, is associated with larger settlers, suggesting that larger fish venture farther into neighboring territories, which then results in chases. That adult density and the number of times an individual was chased interacting with settlement size significantly predicted juvenile growth points to the possibility of both behavioral and energetic mechanisms underlying the preferential survival of slow-growing juveniles. However, these variables did not predict survivorship, likely due to the low number of older fish in this data subset. Thus, we cannot distinguish whether these relationships are by products of size-dependent behaviors or drivers of survivorship in themselves.
4.5 Predator evasion, condition, and juvenile growth
Another non-mutually exclusive process that may play a role in the phenomenon of reduced juvenile growth of survivors is predator evasion via escape swimming. Fast swimmers could experience higher survivorship yet pay a price in lower juvenile growth rates due to the energetic costs of swimming performance. We could not measure in situ burst swim speeds following a simulated predator attack for many S. partitus juveniles and thus could not formally test this hypothesis; our data for 15 new settlers show a negative but non-significant correlation between burst swim speed and juvenile growth rate. While we did not have the power to evaluate this hypothesis, trade-offs between growth and swimming performance have been observed in other teleosts (68–70) and amphibians (12).
Additional trade-offs can exist between condition (i.e., lipid reserves) and accelerated growth (71, 72). For instance, Johnson (22) observed that high-condition S. partitus juveniles grew more slowly than low-condition juveniles and attributed this reduction in growth to more agonistic interactions among high-condition individuals. We did not measure condition in our study, but condition often covaries with size (73–75), and we observed that larger settlers were more active, grew more slowly as juveniles, and had higher survival (14).
4.6 Conclusions
Size-at-settlement is a key trait influencing the survival of young S. partitus settlers. We found that preferential survival of large settlers is not merely a function of gape-limited predation but rather occurs via specific fish behaviors. Large settlers forage higher up in the water column, which could provide access to larger quantities of planktonic food and enhance survival. However, spending more time unsheltered likely engages more conspecific chasers. We found that being chased by conspecifics results in slower juvenile growth rates for large settlers than for small settlers, suggesting differences in the energetic costs of territorial behavior. Furthermore, the degree to which this extra energy expenditure is required depends on the reef “neighborhood” fish settle into. Favorable habitats that support high adult densities could require additional energy expenditure on the part of juveniles, as resident adults chase the new arrivals.
Thus, the size at which settlers arrive to and enter the juvenile habitat plays a critical role in determining subsequent behaviors and trait-mediated survival. Because territorial defense and maintenance are necessary for fitness and conspecific agonistic behavior is a dominant part of damselfish life history, this phenomenon is likely to apply to other damselfish species in numerous locations. Understanding the role of agonistic behaviors in survival is important as changing habitat [territory size; (76)], depth (77), and environmental conditions (78), including levels of predation pressure (79), can affect the frequency or intensity of damselfish agonistic behaviors. Such an understanding of how food availability, somatic growth, survival, and behavior are intertwined in early life is necessary to help explain population dynamics of small territorial fishes such as the bicolor damselfish.
Data availability statement
The datasets presented in this study can be found in online repositories. The names of the repository/repositories and accession number(s) can be found below: 10.5281/zenodo.10403208.
Ethics statement
The animal study was approved by University of Miami Animal Care and Use. The study was conducted in accordance with the local legislation and institutional requirements.
Author contributions
TR: Conceptualization, Formal analysis, Investigation, Writing—original draft, Writing—review & editing. MC: Data curation, Formal analysis, Investigation, Visualization, Writing—original draft, Writing—review & editing. GK: Formal analysis, Investigation, Visualization, Writing—review & editing. KS: Data curation, Formal analysis, Writing—review & editing. SS: Conceptualization, Formal analysis, Funding acquisition, Investigation, Methodology, Project administration, Resources, Supervision, Writing—original draft, Writing—review & editing.
Funding
The author(s) declare financial support was received for the research, authorship, and/or publication of this article. This project was supported by NSF OCE 0550732 and TR received and the Harding B. Michel Biological Oceanography Fellowship. During the preparation of this manuscript, SS was further supported by NSF OCE-2125407. Collections and observations were made possible with the use of a vessel provided through University of Miami Maytag Chair Endowment funds and the EPA-funded National Caribbean Coral Reef Research Center.
Acknowledgments
We thank J. Boulay, G. C. Boyton, E. Buck, E. D'Alessandro, C. Gioia, L. Havel, A. Hogarth, K. Huebert, S. Loftus, T. Murphy, R. Okazaki, L. Parsons, E. Pruitt, and K. Walter for assistance in the field. G. C. Boyton provided a stereo camera. L. Glade and F. Graham assisted with otolith dissection. An early version of the manuscript benefited from comments by R. Cowen, T. Kellison, M. Schmale, and D. Williams.
Conflict of interest
The authors declare that the research was conducted in the absence of any commercial or financial relationships that could be construed as a potential conflict of interest.
The reviewer PC declared a shared affiliation with the author(s) TR to the handling editor at the time of review.
Publisher's note
All claims expressed in this article are solely those of the authors and do not necessarily represent those of their affiliated organizations, or those of the publisher, the editors and the reviewers. Any product that may be evaluated in this article, or claim that may be made by its manufacturer, is not guaranteed or endorsed by the publisher.
Supplementary material
The Supplementary Material for this article can be found online at: https://www.frontiersin.org/articles/10.3389/frish.2023.1276343/abstract#supplementary-material
References
1. Endler JA. Interactions between predators and prey. In:Krebs JR, Davies NB, editors. Behavioural Ecology: An Evolutionary Approach. Oxford: Blackwell Scientific Publications (1991). p. 169–96.
2. Sogard SM. Size-selective mortality in the juvenile stage of teleost fishes: a review. Bull Mar Sci. (1997) 60:1129–57.
3. Houde ED. “Mortality” in fishery science: the unique contributions of early life stages. In:Fuiman LA, Werner RG, editors. Oxford: Blackwell Publishing (2002). p. 64–87.
4. Anderson JT. A review of size dependent survival during pre-recruit stages of fishes in relation to recruitment. J NW Atlantic Fish Sci. (1988) 8:55–66. doi: 10.2960/J.v8.a6
5. Robert D, Shoji J, Sirois P, Takasuka A, Catalán IA, Folkvord A, et al. Life in the fast lane: revisiting the fast growth – high survival paradigm during the early life stages of fishes. Fish Fish. (2023) 24:863–88. doi: 10.1111/faf.12774
6. Arendt JD. Adaptive intrinsic growth rates: an integration across taxa. Quart Rev Biol. (1997) 72:149–77. doi: 10.1086/419764
7. Semlitsch RD, Scott DE, Pechmann JHK. Time and size at metamorphosis related to adult fitness in Ambystoma talpoideum. Ecology. (1988) 69:184–92. doi: 10.2307/1943173
8. Searcy SP, Sponaugle S. Selective mortality during the larval-juvenile transition in two coral reef fishes. Ecology. (2001) 82:2452–70. doi: 10.1890/0012-9658(2001)082[2452:SMDTLJ]2.0.CO;2
9. Marshall DJ, Bolton TF, Keough MJ. Offspring size affects the post-metamorphic performance of a colonial marine invertebrate. Ecology. (2003) 84:3131–7. doi: 10.1890/02-0311
10. Gotthard K. Increased risk of predation as a cost of high growth rate: an experimental test in a butterfly. J Anim Ecol. (2000) 69:896–902. doi: 10.1046/j.1365-2656.2000.00432.x
11. Olsson M, Shine R. Growth to death in lizards. Evolution. (2002) 56:1867–70. doi: 10.1111/j.0014-3820.2002.tb00202.x
12. Arendt JD. Reduced burst speed is a cost of rapid growth in anuran tadpoles: problems of autocorrelation and inferences about growth rates. Funct Ecol. (2003) 17:328–34. doi: 10.1046/j.1365-2435.2003.00737.x
13. Alonso-Alvarez C, Bertrand S, Faivre B, Sorci G. Increased susceptibility to oxidative damage as a cost of accelerated somatic growth in zebra finches. Func Ecol. (2007) 21:873–9. doi: 10.1111/j.1365-2435.2007.01300.x
14. Rankin TR, Sponaugle S. Temperature influences selective mortality during the early life stages of a coral reef fish. PLoS ONE. (2011) 6:e16814. doi: 10.1371/journal.pone.0016814
15. Biro PA, Abrahams MV, Post JR, Parkinson EA. Behavioural trade-offs between growth and mortality explain evolution of submaximal growth rates. J Anim Ecol. (2006) 75:1165–71. doi: 10.1111/j.1365-2656.2006.01137.x
16. Stamps JA. Growth-mortality tradeoffs and ‘personality traits' in animals. Ecol Lett. (2007) 10:355–63. doi: 10.1111/j.1461-0248.2007.01034.x
17. Biro PA, Stamps JA. Are animal personality traits linked to life-history productivity? Trends Ecol Evol. (2008) 23:361–8. doi: 10.1016/j.tree.2008.04.003
18. Stoks R, De Block M, Van de Meutter F, Johansson F. Predation cost of rapid growth: behavioural coupling and physiological decoupling. J Anim Ecol. (2005) 74:708–15. doi: 10.1111/j.1365-2656.2005.00969.x
19. Wooton RJ. Energy allocation in the threespine stickleback. In:Bell MA, Foster SA, editors. The Evolutionary Biology of the Threespine Stickleback. Oxford: Oxford University Press (1994). p. 116–43.
20. Krause J, Loader SP, McDermott J, Ruxton GD. Refuge use by fish as a function of body length-related metabolic expenditure and predation risks. Proc Royal Soc Lond B Biol Sci. (1998) 265:2373–9. doi: 10.1098/rspb.1998.0586
21. Johnson DW, Hixon MA. Ontogenetic and spatial variation in size-selective mortality of a marine fish. J Evol Biol. (2010) 23:724–37. doi: 10.1111/j.1420-9101.2010.01938.x
22. Johnson DW. Combined effects of condition and density on post-settlement survival and growth of a marine fish. Oecologia. (2008) 155:43–52. doi: 10.1007/s00442-007-0882-0
23. Grorud-Colvert K, Sponaugle S. Variability in early life history traits affects post-settlement survival of a tropical reef fish. Oecologia. (2011) 165:675–86. doi: 10.1007/s00442-010-1748-4
24. Rankin TR, Sponaugle S. Characteristics of settling coral reef fish are related to recruitment timing and success. PLoS ONE. (2014) 9:e108871. doi: 10.1371/journal.pone.0108871
25. Sponaugle S. Otolith microstructure reveals ecological and oceanographic processes important to fisheries management. Environ Biol Fishes. (2010) 89:221–38.
26. McCormick MI, Meekan MG. The importance of attitude: the influence of behaviour on survival at an ontogenetic boundary. Mar Ecol Prog Ser. (2010) 407:173–85. doi: 10.3354/meps08583
27. Meekan MG, von Kuerthy C, McCormick MI, Radford B. Behavioural mediation of the costs and benefits of fast growth in a marine fish. Anim Behav. (2010) 79:803–9. doi: 10.1016/j.anbehav.2009.12.002
28. Emery AR. Atlantic bicolor damselfish (Pomacentridae) - taxonomic question. Copeia. (1973) 1973:590–4. doi: 10.2307/1443127
29. Emery AR. Comparative ecology of damselfishes at Alligator Reef, Florida Keys (Ph.D. thesis). University of Miami, Coral Gables, FL, USA (1968).
30. Goldstein ED, D'Alessandro E, Sponaugle S. Fitness consequences of habitat variability, trophic position, and energy allocation across the depth distribution of a coral reef fish. Coral Reefs. (2017) 36:957–68 doi: 10.1007/s00338-017-1587-4
31. Robertson DR, Green DG, Victor BC. Temporal coupling of production and recruitment of larvae of a Caribbean reef fish. Ecology. (1988) 69:370–81. doi: 10.2307/1940435
32. D'Alessandro E, Sponaugle S, Lee T. Patterns and processes of larval fish supply to the coral reefs of the upper Florida Keys. Mar Ecol Prog Ser. (2007) 331:85–100. doi: 10.3354/meps331085
33. Grorud-Colvert K, Sponaugle S. Larval supply and juvenile recruitment of coral reef fishes to marine reserves and non-reserves of the upper Florida Keys, USA. Mar Biol. (2009) 156:277–88. doi: 10.1007/s00227-008-1082-0
34. Sponaugle S, Cowen RK. Larval supply and patterns of recruitment for two Caribbean reef fishes, Stegastes partitus and Acanthurus bahianus. Mar Freshw Res. (1996) 47:433–47. doi: 10.1071/MF9960433
35. Randall JE. Food habitats of reef fishes of the West Indies. In:Bayer FM, editor. International Conference on Tropical Oceanography. Coral Gables, FL: University of Miami (1967). p. 665–840.
36. Albins MA, Hixon MA. Invasive Indo-Pacific lionfish Pterois volitans reduce recruitment of Atlantic coral-reef fishes, Mar. Ecol Prog Ser. (2008) 367:233–8. doi: 10.3354/meps07620
37. Green SJ, Akins JL, Côté IM. Foraging behavior and prey consumption in the Indo-Pacific lionfish on Bahamian coral reefs. Mar Ecol Prog Ser. (2011) 433:159–67. doi: 10.3354/meps09208
38. Palmer G, Hogan JD, Sterba-Boatwright BD, Overath RD. Invasive lionfish Pterois volitans reduce the density but not the genetic diversity of a native reef fish. Mar Ecol Prog Ser. (2016) 558:223–34. doi: 10.3354/meps,11924
39. Myrberg AA. Ethology of the bicolor damselfish, Eupomacentrus partitus (Pisces: Pomacentridae): a comparative analysis of laboratory and field behaviour. Anim Behav Monogr. (1972) 5:197–283. doi: 10.1016/0003-3472(72)90002-4
40. Biro PA, Beckmann C, Stamps JA. Small within-day increases in temperature affects boldness and alters personality in coral reef fish. Proc Royal Soc B Biol Sci. (2010) 277:71–7. doi: 10.1098/rspb.2009.1346
41. White J, Warner R. Behavioral and energetic costs of group membership in a coral reef. Oecologia. (2007) 154:423–33. doi: 10.1007/s00442-007-0838-4
42. Nedelec SL, Mills SC, Radford AN, Beldade R, Simpson SD, Nedelec B, et al. Motorboat noise disrupts co-operative interspecific interactions. Sci Rep. (2017) 7:1–8. doi: 10.1038/s41598-017-06515-2
43. Emery AR. Comparative ecology and functional osteology of fourteen species of damselfish (Pisces: Pomacentridae) at Alligator Reef, Florida Keys. Bull Mar Sci. (1973) 23:649–770.
44. Knapp RA, Warner RR. Male parental care and female choice in the bicolor damselfish, Stegastes partitus: bigger is not always better. Anim Behav. (1991) 41:747–56. doi: 10.1016/S0003-3472(05)80341-0
45. Sponaugle S, Pinkard DR. Impact of variable pelagic environments on natural larval growth and recruitment of the reef fish Thalassoma bifasciatum. J Fish Biol. (2004) 64:34–54. doi: 10.1111/j.1095-8649.2004.00279.x
46. Horn JL. A rationale and test for the number of factors in factor analysis. Psychometrika. (1965) 30:179–85. doi: 10.1007/BF02289447
47. Shipley B. Confirmatory path analysis in a generalized multilevel context. Ecology. (2009) 90:363–8. doi: 10.1890/08-1034.1
48. Lefcheck JS. PiecewiseSEM: Piecewise structural equation modelling in R for ecology, evolution, and systematics. Methods Ecol Evol. (2015) 7:573–9. doi: 10.1111/2041-210X.12512
49. R Core Team. R: A Language Environment for Statistical Computing. Vienna: R Foundation for Statistical Computing (2022). Available online at: https://www.R-project.org/ (accessed October 23, 2023).
50. Kuznetsova A, Brockhoff PB, Christensen RHB. lmerTest Package: tests in linear mixed effects models. J Stat Softw. (2017) 82:1–26. doi: 10.18637/jss.v082.i13
51. Brooks ME, Kristensen K, van Benthem KJ, Magnusson A, Berg CW, Nielsen A, et al. glmmTMB balances speed and flexibility among packages for zero-inflated generalized linear mixed modeling. R J. (2017) 9:378–400. doi: 10.32614/RJ-2017-066
52. Shima JS, Swearer SE. The legacy of dispersal: larval experience shapes persistence later in the life of a reef fish. J Anim Ecol. (2010) 79:1308–14. doi: 10.1111/j.1365-2656.2010.01733.x
53. Dingeldein AL, White JW. Larval traits carry over to affect post-settlement behaviour in a common coral reef fish. J Anim Ecol. (2016) 85:903–14. doi: 10.1111/1365-2656.12506
54. Miller TJ, Crowder LB, Rice JA, Marschall EA. Larval size and recruitment mechanisms in fishes - toward a conceptual-framework. Can J Fish Aquat Sci. (1988) 45:1657–70. doi: 10.1139/f88-197
55. Gauff RPM, Bejarano S, Madduppa HH, Subhan B, Dugeny EMA, Perdana YA, et al. Influence of predation risk on the sheltering behavior of the coral-dwelling damselfish, Pomacentrus moluccensis. Environ Biol Fish. (2018) 101:639–51. doi: 10.1007/s10641-018-0725-3
56. Damsgard B, Dill LM. Risk-taking behavior in weight-compensating coho salmon, Oncorhynchus kisutch. Behav Ecol. (1998) 9:26–32. doi: 10.1093/beheco/9.1.26
57. Hurst TP, Spencer ML, Sogard SM, Stoner AW. Compensatory growth, energy storage and behavior of juvenile Pacific halibut Hippoglossus stenolepis following thermally induced growth reduction. Mar Ecol Prog Ser. (2005) 293:233–40. doi: 10.3354/meps293233
58. Godin J-GJ, Davis SA. Who dares, benefits: predator approach behaviour in the guppy (Poecilia reticulata) deters predator pursuit. Proc R Soc Lond. (1995) B259:193–200.
59. Réale D, Festa-Blanchet M. Predator-induced natural selection on temperament in bighorn ewes. Anim Behav. (2003) 65:463–70. doi: 10.1006/anbe.2003.2100
60. Fuiman LA, Meekan MG, McCormick MI. Maladaptive behavior reinforces a recruitment bottleneck in newly settled fishes. Oecologia. (2010) 164:99–108. doi: 10.1007/s00442-010-1712-3
61. McCormick MI, Faken E, Allan BJM. Behavioural measures determine survivorship within the hierarchy of whole organism phenotypic traits. Func Ecol. (2018) 32:958–69. doi: 10.1111/1365-2435.13033
62. Biro PA, Abrahams MV, Post JR, Parkinson EA. Predators select against high growth rates and risk-taking behaviour in domestic trout populations. Proc R Soc B-Biol Sci. (2004) 271:2233–7. doi: 10.1098/rspb.2004.2861
63. Hulthén K, Chapman BB, Nilsson PA, Hansson L-A, Skov C, Brodersen J, et al. A predation cost to bold fish in the wild. Sci Rep. (2017) 7:1239. doi: 10.1038/s41598-017-01270-w
64. Briffa M, Sneddon LU. Physiological constraints on contest behaviour. Funct Ecol. (2007) 21:627–37. doi: 10.1111/j.1365-2435.2006.01188.x
65. Wilson MA, Gatten RE, Greenberg N. Glycolysis in Anolis carolinensis during agonistic encounters. Physiol Behav. (1990) 48:139–42. doi: 10.1016/0031-9384(90)90274-8
66. McDougall PT, Kramer DL. Short-term behavioral consequences of territory relocation in a Caribbean damselfish, Stegastes diencaeus. Behav Ecol. (2007) 18:53–61. doi: 10.1093/beheco/arl055
67. Neat FC, Taylor AC, Huntingford FA. Proximate costs of fighting in male cichlid fish: the role of injuries and energy metabolism. Anim Behav. (1998) 55:875–82. doi: 10.1006/anbe.1997.0668
68. Kolok AS, Oris JT. The relationship between specific growth rate and swimming performance in male fathead minnows (Pimephales promelas). Can J Zool Rev Can De Zool. (1995) 73:2165–7. doi: 10.1139/z95-254
69. Billerbeck JM, Lankford TE, Conover DO. Evolution of intrinsic growth and energy acquisition rates. I Trade-offs with swimming performance in Menidia menidia. Evolution. (2001) 55:1863–72. doi: 10.1111/j.0014-3820.2001.tb00835.x
70. Sogard SM, Olla BL. Contrasts in the capacity and underlying mechanisms for compensatory growth in two pelagic marine fishes. Mar Ecol Prog Ser. (2002) 243:165–77. doi: 10.3354/meps243165
71. Johansen SJS, Ekli M, Stangnes B, Jobling M. Weight gain and lipid deposition in Atlantic salmon, Salmo salar, during compensatory growth: evidence for lipostatic regulation? Aquac Res. (2001) 32:963–74. doi: 10.1046/j.1365-2109.2001.00632.x
72. Sogard SM, Spencer ML. Energy allocation in juvenile sablefish: effects of temperature, ration and body size. J Fish Biol. (2004) 64:726–38. doi: 10.1111/j.1095-8649.2004.00342.x
73. Henderson PA, Holmes RHA, Bamber RN. Size-selective overwintering mortality in the sand smelt, Atherina boyeri Risso, and its role in population regulation. J Fish Biol. (1988) 33:221–33. doi: 10.1111/j.1095-8649.1988.tb05465.x
74. Thompson JM, Bergersen EP, Carlson CA, Kaeding LR. Role of size, condition, and lipid-content in the overwinter survival of age-0 Colorado squawfish. Trans Amer Fish Soc. (1991) 120:346–53. doi: 10.1577/1548-8659(1991)120andlt;0346:ROSCALandgt;2.3.CO;2
75. Schultz ET, Conover DO. Latitudinal differences in somatic energy storage: adaptive responses to seasonality in an estuarine fish (Atherinidae: Menidia menidia). Oecologia. (1997) 109:516–29. doi: 10.1007/s004420050112
76. Silveira MM, Silva PF, Ferreira RG, Luchiari AC. Fighting off the intruder: context-dependent territory defence in the damselfish Stegastes fuscus. Env Biol Fish. (2020) 103:1091–104. doi: 10.1007/s10641-020-01011-5
77. Goldstein ED, D'Alessandro E, Sponaugle S. Demographic and reproductive plasticity across the depth distribution of a coral reef fish. Sci Rep. (2016) 6:34077. doi: 10.1038/srep34077
78. da Silva-Pinto T, Silveira MM, de Souza JF, Moreira ALP, Vieira EA, Longo GO, et al. Damselfish face climate change: Impact of temperature and habitat structure on agonistic behavior. PLoS ONE. (2020) 15:e0235389. doi: 10.1371/journal.pone.0235389
Keywords: early life history, selective survival, growth-mortality hypothesis, growth-survival paradigm, otolith microstructure, damselfish, foraging behavior via several trade-offs (larval growth rate and pelagic larval duration)
Citation: Rankin TL, Cowen MC, Kandlikar GS, Shulzitski K and Sponaugle S (2024) Behavioral mechanisms underlying trait-mediated survival in a coral reef fish. Front. Fish Sci. 1:1276343. doi: 10.3389/frish.2023.1276343
Received: 11 August 2023; Accepted: 29 November 2023;
Published: 05 January 2024.
Edited by:
Kevin J. Hedges, Fisheries and Oceans Canada (DFO), CanadaReviewed by:
Paul Chittaro, NOAA Fisheries, United StatesQuentin Petitjean, UMR5174 Evolution Et Diversite Biologique (EDB), France
Samuel Matchette, University of Cambridge, United Kingdom
Copyright © 2024 Rankin, Cowen, Kandlikar, Shulzitski and Sponaugle. This is an open-access article distributed under the terms of the Creative Commons Attribution License (CC BY). The use, distribution or reproduction in other forums is permitted, provided the original author(s) and the copyright owner(s) are credited and that the original publication in this journal is cited, in accordance with accepted academic practice. No use, distribution or reproduction is permitted which does not comply with these terms.
*Correspondence: Su Sponaugle, su.sponaugle@oregonstate.edu
†These authors share first authorship