Opportunities for membrane technology in controlled environment agriculture
- Chemical Engineering, University of Toledo, Toledo, OH, United States
Controlled environment agriculture has the potential to enhance agriculture sustainability, a United Nations sustainable development goal. Enclosed agricultural facilities can be used in locations that cannot support field agriculture while reducing water usage and increasing productivity relative to open field agriculture. The primary challenges with operation arise from energy consumption to maintain the proper growth conditions. Membrane processes can reduce energy consumption by controlling temperature, humidity, and carbon dioxide concentration. Membrane processes also can minimize water consumption by enabling the use of non-conventional water resources and reducing wastewater production. The literature describing these applications is reviewed and opportunities for future innovation are discussed.
1 Introduction
Global food supply chains are under stress as the population surges past 8 billion, climate change reduces farm productivity, and agricultural land is repurposed or lost (Tilman et al., 2011). This stress is exacerbated by attempts to intensify traditional field cultivation through increased use of fertilizer that in turn leads to increased greenhouse gas emissions (Menegat et al., 2022), greater eutrophication of adjacent bodies of water (Liu et al., 2021), and loss of soil health (Krasilnikov et al., 2022).
Controlled environment agriculture (CEA) offers the potential to dramatically intensify agricultural operations while simultaneously enhancing sustainability and resiliency (McClements et al., 2020). CEA encompasses a spectrum of technologies that allow food production in facilities isolated from the external environment including traditional soil-based greenhouses, vertical farms, hydroponics, aeroponics, and aquaponics (Cowan et al., 2022).
The potential benefits of indoor food production are reduced water consumption through precision application and recycle, optimization of growth conditions, increased productivity through year-round growth, increased arable area through vertical farming, reduced transportation costs through farm placement closer to markets (especially near food deserts), and reduced exposure to pests and invasive plants (Benke and Tomkins, 2017). However, these benefits come at the cost of increased capital expenses for the production infrastructure, greater energy consumption for environmental control and lighting, and lack of ecosystem services such as pollination (Cowan et al., 2022).
Currently, food production with CEA systems is more expensive than field production. An analysis of landed costs for field-produced lettuce sent from California to New York and Chicago indicated field lettuce costs were less than one-half of CEA lettuce costs. Moreover, energy usage and greenhouse gas emissions were higher (Nicholson et al., 2020). Despite these cost differences, sales of CEA produced crops have risen to $600 million in 2019 (corrected to 2012 dollars) with production coming from nearly 3000 facilities (Dohlman et al., 2024). The top three sales crops were tomatoes, lettuce, and herbs.
To improve economics, energy consumption in CEA must be reduced (Engler and Krarti, 2021). A study of energy consumption in leafy green production indicated 70% was for lighting and 28% for HVAC (Zeidler and Vrakking, 2015). Membrane technology has the potential to reduce the HVAC contribution. Additionally, membrane technology has the potential to reduce water consumption, increase productivity, and improve resilience.
This review summarizes past work on the use of membrane technology in CEA. It emphasizes how membranes can improve sustainability. Finally, opportunities for future innovation are suggested.
Figure 1 illustrates the opportunities that exist. CEA facilities can be envisioned as biochemical reactors that transform feed streams into food products. Plant DNA (seeds or seedlings), carbon dioxide (CO2), water, and nutrients are needed to produce the hydrocarbons constituting the food product. Continuous flows of CO2 and water are required to provide carbon, hydrogen, and oxygen for photosynthesis and plant growth. Additional inputs include natural light for photosynthesis and energy for temperature control, equipment operation, and supplemental artificial lighting.
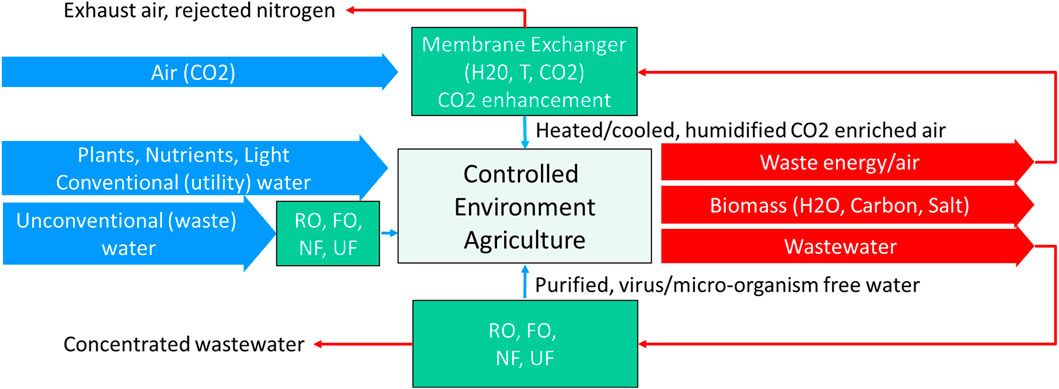
Figure 1. Primary opportunities for application of membrane technology to increase sustainability of CEA operations. Feed streams are indicated by blue arrows, product streams by red arrows and membrane applications by green boxes.
Three primary streams exit the bioreactor: 1) the desired food product and other biomass that accompanies its production such as roots, stems, and leaves, 2) a waste air stream from displacement of air in the facility by the incoming air stream, and 3) a wastewater stream to remove contaminants that build-up in the water supply system. The waste streams are valuable due to their water and energy content. Membrane processes offer the potential to recover both. Membranes also can be used to control humidity and CO2 concentration within the facility. Additionally, membranes allow use of unconventional water sources when conventional water sources are lacking or insufficient. Past work in these areas is reviewed here.
2 Water management
Global water scarcity and rising food demand necessitate innovation in water management for the entire agriculture industry (Greenlee et al., 2009). By 2025, half the global population will inhabit water-stressed regions (WHO, 2024) underscoring the importance of identifying unconventional sources and enhancing conservation. Agriculture currently utilizes −70% of freshwater withdrawals globally, often unsustainably (Organization et al., 2012). Greenhouses, with their controlled environments, offer a viable solution to manage water more efficiently in agricultural areas (Al-Ismaili and Jayasuriya, 2016). Incorporation of membrane technology in CEA has the potential to lower agriculture’s water demand relative to field cultivation.
2.1 Source water management
Greenhouses typically rely on municipal water, which can be costly and scarce in some regions. This has led to the use of groundwater instead. However, approximately 50% of global groundwater resources exhibit salinity levels (0.5 ≤ S ≤ 5 g/kg) too high for agricultural use (Gleick, 2012). Therefore, there is a critical need for desalination to ensure sustainable and efficient crop cultivation (Pimentel et al., 1997).
Reverse osmosis (RO) offers a solution to water salinity control. RO, with its reduced energy consumption relative to traditional thermal desalination methods, is especially effective for treating brackish water, due to its low osmotic pressure (Fikana and Raafi u, 2023). Efforts from the large body of literature on membranes for desalination that focus on CEA applications are discussed here.
While RO effectively removes 99% of ions, resulting in a low concentration, it also eliminates essential nutrients for crop growth (Ukraine et al., 2020) which must be replaced either by adding fertilizers (increasing costs (Ben Gal et al., 2009)) or blending with brackish water; blending can have the detrimental effect of raising soil salinity and requiring extra water to flush out salts (Cohen et al., 2018). Fouling and scaling, common problems of RO systems, increase costs but can be controlled through pretreatment. Many pilot studies have shown that that MF, UF, and FO are effective pre-treatment steps for RO, enhancing biofouling prevention and water quality while potentially lowering energy use (Zhang et al., 2006; Shaffer et al., 2012; Coday et al., 2014; Lew et al., 2020).
Recent studies highlight the advantages of integrating nanofiltration (NF) as a pretreatment for reverse osmosis (RO) in desalination. Hassan et al., 1998 and others (Uhlinger, 2001) have demonstrated that NF reduces divalent ions, lowers water production costs by 27%, and improves RO efficiency, despite higher complexity and initial costs. These findings suggest NF pretreatment can double RO recovery rates, reduce energy use by 40%, and cut water costs by 20%. Furthermore, the RO-NF process can lead to reduced investment costs and electricity usage compared to a two-stage RO process (Kurihara et al., 2001). Studies of ultrafiltration (UF) and forward osmosis (FO) with RO as an alternative pretreatment suggest potential for further efficiency gains (Pazouki et al., 2022). The key challenge is balancing the benefits of NF, UF, and FO pretreatments against their added complexity and costs for commercial-scale viability.
Monovalent selective electrodialysis (MSED) offers a tailored approach to water management, selectively separating beneficial divalent ions (Ca2+, Mg2+, SO42-) from harmful monovalent ions (Na+, Cl−) providing an effective alternative to RO. Although not yet commercialized for brackish water, research shows the potential for MSED cost savings by minimizing fertilizer usage. Rehman et al. (Rehman et al., 2021) modeled MSED for U.S. greenhouse irrigation, estimating $4,991 per hectare savings in fertilizer costs by retaining nutrients. Kishor et al. (Nayar and V, 2020) found switching from RO to MSED saved $15,000 annually in fertilizer for a 10-ha farm. The viability of MSED desalination for greenhouses depends on nutrient and water savings outweighing higher capital and operating costs than RO. Yvana et al. (Ahdab et al., 2021a) found SW-MSED 30% more expensive than SW-RO at current prices. However, SW-MSED matches SW-RO cost-effectiveness if electricity is under $0.08/kWh.
Membrane distillation (MD) utilizes a membrane contactor for thermal separation by evaporation and shows promise for hypersaline water when combined with use of waste heat or solar energy (Lee et al., 2020). Studies have demonstrated the potential for MD in desalinating seawater and hypersaline water, especially when used alongside conventional desalination systems like ED, RO, and FO (Yadav et al., 2021). However, the limited water flux of MD has hindered scale-up (Hussain et al., 2022a).
While ED, Reverse ED and MD have been successfully piloted for agricultural projects (Chafidz et al., 2014), they presently lack the reliability and cost-effectiveness for widespread adoption. Further R&D focused on enhancing membrane performance and integration with renewable energy is needed to realize the potential of these technologies.
The use of concentrated aqueous fertilizer as a draw solution in FO has also been proposed to produce fertilized irrigation water (fertigation) from brackish water, desalination brine, and other non-potable water sources. Since the concept was first introduced in 2011 (Phuntsho et al., 2011), numerous investigators have studied stand-alone fertilizer-driven FO processes as well as hybrids with other water purification processes. Pourmovahed et al., 2022 summarize the literature and provide an analysis of the thermodynamic limits to the amount of water that can be recovered, relative to water irrigation requirements, as well as limitations imposed by maintaining sufficiently high-water fluxes to ensure economic viability.
2.2 Wastewater management
Fertigation, the addition of fertilizer including nitrogen as nitrate or ammonium to irrigation water, is used to enhance crop growth. However, excess fertilizer not used by crops leads to nutrient-rich wastewater. Due to stricter EU and US regulations on nitrate disposal, two main wastewater treatment methods have emerged: biological denitrification in large basins or artificial wetlands, which is land-intensive and offers limited control (Gruyer et al., 2013), and wastewater reuse for irrigation after disinfection to mitigate disease and biofilm growth in irrigation systems. Reusing wastewater for irrigation reduces source water demand but leads to challenges like sodium buildup which can damage crops. Operators must choose between discharging this water or using energy-intensive reverse osmosis (RO) that also removes beneficial minerals, necessitating extra fertilizer.
MSED technology has been demonstrated to outperform RO in greenhouse wastewater treatment for irrigation with selective ion removal with over 90% water recovery, less waste, and longer-lasting, fouling-resistant membranes (Strathmann, 2010) enhancing cost-effectiveness and efficiency. Additional studies by Ahdab et al. (Ahdab et al., 2021b) and Schücking (Schücking, 2020) have demonstrated MSED can treat greenhouse wastewater by removing sodium and nitrates. Despite this potential, MSED technology has not yet been adopted commercially for greenhouse wastewater treatment.
Additional challenges exist in separating potassium and sodium in sustainable greenhouse farming. Potassium (K+) is vital for plant health, enhancing water uptake, enzyme activity, photosynthesis, nutrient transport, growth, and stress resilience (Ragel et al., 2019; Rawat et al., 2022). However, the K+ and Na+ separation is difficult with conventional MSED due to the similarity in ion exchange equilibrium coefficients and physicochemical characteristics (Zhang et al., 2021). B et al., 2022 investigate the development of sustainable K+/Na+ monovalent-selective membranes using a hot-pressed polyelectrolyte complex method. The resulting membrane demonstrates superior selectivity for K+ over Na+. Moreover, Qian et al., 2022 examine sodium ion removal from greenhouse water using electrodialysis with a supported liquid membrane (SLM) and a cation-selective membrane (CIMS), achieving up to 96% potassium recovery and 80% for other ions. This method shows potential for improving water quality in greenhouse farming.
3 Air management
CEA systems offer the ability to produce food in geographical locations where field agriculture is not viable due to climatic conditions, most notably extreme temperature and humidity conditions. For example, CEA can enable food production in desert areas or other hot climates and be integrated with energy production via thermal solar or photovoltaics (Ghoulem et al., 2019; Lefers et al., 2020). Membrane processes offer alternatives to systems being considered for temperature (e.g., evaporative cooling (Cuce and Riffat, 2016) and humidity (e.g., heat pump (Cámara-Zapata et al., 2019)) control. Additionally, membranes offer the potential for CO2 management.
3.1 Humidity management
Humidity control is critical for optimization of plant growth and prevention of physiological disorders. Traditional methods for humidity control include condensation, use of solid and liquid desiccants, and electrochemical techniques (Huang and Zhang, 2013; Lefers et al., 2016; Ali et al., 2017; Amani et al., 2020; Hussain et al., 2022b; Soussi et al., 2022).
Liquid desiccants can capture water vapor either directly or via a membrane contactor. The use of membrane contactors in liquid desiccant-based systems has been studied extensively (Abdel-Salam et al., 2013; Das and Jain, 2013; Zhang and Zhang, 2014; Zhang et al., 2016). Dehumidification utilizes a semi-permeable membrane barrier to facilitate contacting the process air with the liquid desiccant. The membrane allows water vapor transport across it but prevents desiccant flow; the direction and rate of water permeation determined by the water vapor chemical potential difference across the membrane (Qu et al., 2018). A membrane contactor allows use of smaller dehumidification systems and can provide more efficient performance.
System performance is controlled by the type of membrane used. Triethylene glycol (TEG) supported liquid membranes were used in early studies for indoor humidity control (Abdul-Wahab et al., 2004; Li and Ito, 2008; Li et al., 2011). Kneifel et al. (Kneifel et al., 2006) developed a coated polyetherimide hollow fiber membrane and evaluated the effect of the PDMS coating thickness on water vapor permeation using LiCl as the absorbent. Bergero et al. (Bergero and Chiari, 2010) demonstrated that a hybrid air-conditioning system combining vapor-compression with LiCl-based membrane dehumidification could reduce energy costs by 50% compared to conventional systems. Zhang, 2011 reported a hollow fiber membrane contactor model and its use in dehumidification process optimization. They noted the importance of preventing liquid desiccant crossover. Bettahalli et al., 2016 developed a dehumidification device using a calcium chloride desiccant with a PVDF triple-bore hollow fiber membrane that offered enhanced efficiency. They examined how the device could be used for energy-efficient humidity control in CEA systems located in Jeddah. Lefers et al., 2019 explored the use of magnesium chloride with PVDF membrane contactors and demonstrated its potential to maintain desired humidity levels. Pasqualin and Davies, 2022 examined the use of multi-stage nanofiltration for liquid desiccant regeneration in air conditioning, focusing on how salt rejection in NF membranes is influenced by desiccant concentration and pressure. They tested four commercial NF membranes with LiCl, LiBr, and MgCl2, finding that LiCl shows lower rejection but higher permeate flux. The study revealed the need for membranes capable of operation at higher pressures for effective water removal from used desiccant solutions (i.e., desalination where the desired product is the concentrated brine) and improved coefficient of performance.
Moussaddy et al., 2024 proposed using concentrated fertilizer solution as the desiccant in a membrane-based process. Using dense polydimethylsiloxane hollow fiber membranes, they demonstrated the ability to reduce humidity to −40% with diverse fertilizer mixtures. Energy efficiencies as high as 0.24 Wh/g were observed which compare well with other technologies. Control of desiccant temperature was critical to optimizing dehumidification performance.
3.2 Thermal management
Temperature control is critical for CEA operations in areas with extreme ambient temperatures. Both cooling and heating may be required depending on the location and time of year.
The need for cooling in warm climates has motivated significant efforts to evaluate the technological options for maintenance, ease of use, and cost (Rabbi et al., 2019). Membrane-based systems using liquid desiccant air conditioning (LDAC) have attracted attention recently. Pasqualin and Davies, 2022 proposed a LDAC system using multi-stage nanofiltration (NF) for desiccant regeneration. Their system enabled closed air recirculation and a closed water cycle for both cooling and irrigation while keeping temperatures below 32°C with greater efficiency than conventional cooling. Future advancements could reduce complexity and cost. Lefers et al., 2016 explored a greenhouse cooling system using liquid desiccation and solar-powered MD with PVDF membranes. The system was designed for hot, humid climates and integrated dehumidification with freshwater generation. The study addressed challenges with efficient desiccant regeneration and managing thermal inputs through use of solar energy. Pilot systems are being developed to refine the technology.
Membrane enthalpy exchangers offer the potential for simultaneous temperature and humidity management. A relatively new concept (Zhang and Jiang, 1999; Zhang, 2012), these air-to-air heat exchangers used to contact building exhaust air with incoming air can exchange both enthalpy and humidity. Such systems have been studied extensively to improve efficiency of building HVAC systems (Li et al., 2022). However, studies of their application in CEA are limited.
3.3 CO2 control
CO2 concentration is a critical variable, along with temperature and humidity, in the optimization of CEA environment for plant growth (Pasgianos et al., 2003; Panwar et al., 2011). Increased CO2 levels can enhance photosynthesis efficiency thereby leading to enhanced energy and water utilization (Thongbai et al., 2010; Zhang et al., 2014). Five primary sources of CO2 enrichment have been used in CEA: compost, exhaust gas from fossil fuels, exhaust gas from renewable energy, natural or forced ventilation, and pure liquefied CO2 (Jin et al., 2009; Dion et al., 2011; Kuroyanagi et al., 2014; Roy et al., 2014). Membrane gas separation is a highly promising technology to concentrate CO2 for use in CEA that offers reduced capture cost, smaller process footprints, and reduced process complexity relative to other processes (Yang et al., 2008; Brunetti et al., 2010; Dion et al., 2011; Khalilpour et al., 2015; Norahim et al., 2018).
Wang et al., 2014 proposed the use of an anion exchange membrane in a moisture swing adsorption process for direct air capture (DAC) and greenhouse agriculture. The membrane they evaluated had a capacity of 0.83 mol CO2 per kilogram and adsorption was well described by a Langmuir isotherm model. Desorption studies indicated nearly 80% of the adsorbed CO2 could be recovered and the impact on greenhouse energy consumption was evaluated.
Stacey et al., 2018 investigated the use of CO2 enrichment to reduce water losses in CEA. Water is lost in the humid air displaced when outside air is circulated into a CEA facility to provide CO2 for plant growth. Humidity losses were estimated to be 90% of overall water usage. Simulations of mass and energy flows indicated water losses could be reduced by 95% using a pressure-driven membrane gas separation process for CO2 enrichment in the entering air. Changes in process energy requirements were evaluated, including compression for the membrane process and changes in greenhouse heating or cooling arising from use of CO2 enriched air, but the impact on plant growth was not considered.
Shin et al., 2019 focused on repurposing CO2 from biogas (a mixture of carbon dioxide and methane) for industrial and agricultural use. They evaluated a commercial hollow-fiber membrane module, made of polysulfone (PSf) coated with polydimethylsiloxane (PDMS). At the optimal operating conditions of 40°C and 7 bar, a 95.6% CO2 product was produced with minimal CH4 loss demonstrating the viability of CO2 recovery from biogas.
Hu et al., 2022 prepared composite membranes consisting of polydimethylsiloxane (PDMS), polyethylene oxide (PEO) and polyether block amide copolymer (Pebax) supported by a porous polysulfone (PSf) layer on a nonwoven polyester fabric. The PDMS/PSf membranes possessed the highest CO2 permeance and produced the most concentrated permeate. Optimum crosslinker concentration, UV/ozone treatment time (to produce a silica-like surface layer), and final heat treatment time were determined to achieve the desired CO2 permeance and selectivity. Growth of Glebionis coronaria with the CO2 enriched air led to increases in plant mass and height of 458% and 61%, respectively, after 4 weeks. Similar increases were observed for bok choy. Membrane performance was stable over an 80-day period demonstrating the potential of the process to enhance CEA productivity.
Yoo et al., 2023 studied a membrane-based system for indoor CO2 management and utilization, featuring a commercial hollow fiber membrane module. Designed to maintain CO2 levels below 300 ppm to ensure healthy indoor environments, the system also produced a CO2 enriched permeate for enhancement of indoor plant growth. Such findings indicate the synergy that can exist by combining CO2 capture with CEA utilization in urban environments.
4 Conclusion
Applications of membrane technology to increase sustainable CEA operation are reviewed. Membranes offer unique approaches to control water, air, and carbon dioxide flows to reduce energy consumption. The primary applications have been in source water management, wastewater management, humidity control, thermal management, and CO2 enrichment.
Opportunities for further innovation are numerous. Continued improvement in membrane material properties and module design is expected to increase process efficiency, especially for use in water recycling and utilization of non-potable feed waters. Water management also would benefit significantly from improved monovalent selective electrodialysis membranes. Additionally, new materials for use in liquid desiccant air conditioning have the potential to reduce energy consumption and simultaneously control CEA facility humidity and temperature.
CO2 enrichment strategies may benefit from integration of membrane technology with adsorption and absorption technology being developed for point source and direct air carbon capture. Co-location of CEA facilities with these carbon dioxide capture operations, including both fossil fuel power plants and enclosed environments such as office buildings (Sinha et al., 2018), would further improve economics. Furthermore, opportunities exist for hybrid processes that integrate membrane enthalpy exchanger technology with non-membrane technologies being developed to reduce energy consumption of HVAC systems.
Author contributions
PS: Investigation, Writing–original draft, Writing–review and editing. HR: Investigation, Writing–original draft, Writing–review and editing. GL: Conceptualization, Supervision, Visualization, Writing–original draft, Writing–review and editing.
Funding
The author(s) declare that no financial support was received for the research, authorship, and/or publication of this article.
Conflict of interest
The authors declare that the research was conducted in the absence of any commercial or financial relationships that could be construed as a potential conflict of interest.
Publisher’s note
All claims expressed in this article are solely those of the authors and do not necessarily represent those of their affiliated organizations, or those of the publisher, the editors and the reviewers. Any product that may be evaluated in this article, or claim that may be made by its manufacturer, is not guaranteed or endorsed by the publisher.
References
Abdel-Salam, A. H., Ge, G., and Simonson, C. J. (2013). Performance analysis of a membrane liquid desiccant air-conditioning system. Energy Build. 62, 559–569. doi:10.1016/j.enbuild.2013.03.028
Abdul-Wahab, S. A., Zurigat, Y. H., and Abu-Arabi, M. K. (2004). Predictions of moisture removal rate and dehumidification effectiveness for structured liquid desiccant air dehumidifier. Energy 29, 19–34. doi:10.1016/j.energy.2003.08.001
Ahdab, Y. D., Schücking, G., Rehman, D., and Lienhard, J. H. (2021a). Cost effectiveness of conventionally and solar powered monovalent selective electrodialysis for seawater desalination in greenhouses. Appl. Energy. 301, 117425. doi:10.1016/j.apenergy.2021.117425
Ahdab, Y. D., Schücking, G., Rehman, D., and Lienhard, J. H. (2021b). Treatment of greenhouse wastewater for reuse or disposal using monovalent selective electrodialysis. Desalination 507, 115037. doi:10.1016/j.desal.2021.115037
Ali, A., Ishaque, K., Lashin, A., and Arifi, N. A. (2017). Modeling of a liquid desiccant dehumidification system for close type greenhouse cultivation. Energy 118, 578–589. doi:10.1016/j.energy.2016.10.069
Al-Ismaili, A. M., and Jayasuriya, H. (2016). Seawater greenhouse in Oman: a sustainable technique for freshwater conservation and production. Renew. Sustain. Energy Rev. 54, 653–664. doi:10.1016/j.rser.2015.10.016
Amani, M., Foroushani, S., Sultan, M., and Bahrami, M. (2020). Comprehensive review on dehumidification strategies for agricultural greenhouse applications. Appl. Therm. Eng. 181, 115979. doi:10.1016/j.applthermaleng.2020.115979
B, A. K., Zwijnenberg, H. J., Lindhoud, S., and Vos, W.M. de (2022). Sustainable K+/Na+ monovalent-selective membranes with hot-pressed PSS-PVA saloplastics. J. Membr. Sci. 652, 120463. doi:10.1016/j.memsci.2022.120463
Ben Gal, A., Yermiyahu, U., and Cohen, S. (2009). Fertilization and blending alternatives for irrigation with desalinated water. J. Environ. Qual. 38, 529–536. doi:10.2134/jeq2008.0199
Benke, K., and Tomkins, B. (2017). Future food-production systems: vertical farming and controlled-environment agriculture. Sustain. Sci. Pr. Polic. 13, 13–26. doi:10.1080/15487733.2017.1394054
Bergero, S., and Chiari, A. (2010). Performance analysis of a liquid desiccant and membrane contactor hybrid air-conditioning system. Energy Build. 42, 1976–1986. doi:10.1016/j.enbuild.2010.06.003
Bettahalli, N. M. S., Lefers, R., Fedoroff, N., Leiknes, T., and Nunes, S. P. (2016). Triple-bore hollow fiber membrane contactor for liquid desiccant based air dehumidification. J. Membr. Sci. 514, 135–142. doi:10.1016/j.memsci.2016.04.059
Brunetti, A., Scura, F., Barbieri, G., and Drioli, E. (2010). Membrane technologies for CO2 separation. J. Membr. Sci. 359, 115–125. doi:10.1016/j.memsci.2009.11.040
Cámara-Zapata, J. M., Sánchez-Molina, J. A., Rodríguez, F., and López, J. C. (2019). Evaluation of a dehumidifier in a mild weather greenhouse. Appl. Therm. Eng. 146, 92–103. doi:10.1016/j.applthermaleng.2018.09.107
Chafidz, A., Al-Zahrani, S., Al-Otaibi, M. N., Hoong, C. F., Lai, T. F., and Prabu, M. (2014). Portable and integrated solar-driven desalination system using membrane distillation for arid remote areas in Saudi Arabia. Desalination 345, 36–49. doi:10.1016/j.desal.2014.04.017
Coday, B. D., Yaffe, B. G. M., Xu, P., and Cath, T. Y. (2014). Rejection of trace organic compounds by forward osmosis membranes: a literature review. Environ. Sci. Technol. 48, 3612–3624. doi:10.1021/es4038676
Cohen, B., Lazarovitch, N., and Gilron, J. (2018). Upgrading groundwater for irrigation using monovalent selective electrodialysis. Desalination 431, 126–139. doi:10.1016/j.desal.2017.10.030
Cowan, N., Ferrier, L., Spears, B., Drewer, J., Reay, D., and Skiba, U. (2022). CEA systems: the means to achieve future food security and environmental sustainability? Front. Sustain. Food Syst. 6, 891256. doi:10.3389/fsufs.2022.891256
Cuce, P. M., and Riffat, S. (2016). A state of the art review of evaporative cooling systems for building applications. Renew. Sustain. Energy Rev. 54, 1240–1249. doi:10.1016/j.rser.2015.10.066
Das, R. S., and Jain, S. (2013). Experimental performance of indirect air–liquid membrane contactors for liquid desiccant cooling systems. Energy 57, 319–325. doi:10.1016/j.energy.2013.05.013
Dion, L.-M., Lefsrud, M., and Orsat, V. (2011). Review of CO2 recovery methods from the exhaust gas of biomass heating systems for safe enrichment in greenhouses. Biomass Bioenergy 35, 3422–3432. doi:10.1016/j.biombioe.2011.06.013
Dohlman, M. E., Davis, K., Husby, W. V., Bovay, M., Weber, J., and Lee, C. (2024). Yoonjung: trends, insights, and future prospects for production in controlled environment agriculture and agrivoltaics systems. U S Dep. Agric. Econ. Res. Serv.
Engler, N., and Krarti, M. (2021). Review of energy efficiency in controlled environment agriculture. Renew. Sustain. Energy Rev. 141, 110786. doi:10.1016/j.rser.2021.110786
Fikana, E. R., and Raafi u, B. (2023). Design of water treatment system to change Brackish water into fresh water using Reverse Osmosis method. AIP Conf. Proc. 2580, 040008. doi:10.1063/5.0124367
Ghoulem, M., Moueddeb, K. E., Nehdi, E., Boukhanouf, R., and Calautit, J. K. (2019). Greenhouse design and cooling technologies for sustainable food cultivation in hot climates: review of current practice and future status. Biosyst. Eng. 183, 121–150. doi:10.1016/j.biosystemseng.2019.04.016
Gleick (2012). Gleick: the world’s water volume 7: the biennial report on freshwater resources. China: Island press.
Greenlee, L. F., Lawler, D. F., Freeman, B. D., Marrot, B., and Moulin, P. (2009). Reverse osmosis desalination: water sources, technology, and today’s challenges. Water Res. 43, 2317–2348. doi:10.1016/j.watres.2009.03.010
Gruyer, N., Dorais, M., Alsanius, B. W., and Zagury, G. J. (2013). Simultaneous removal of nitrate and sulfate from greenhouse wastewater by constructed wetlands. J. Environ. Qual. 42, 1256–1266. doi:10.2134/jeq2012.0306
Hassan, A. M., Al-Sofi, M. A. K., Al-Amoudi, A. S., Jamaluddin, A. T. M., Farooque, A. M., Rowaili, A., et al. (1998). A new approach to membrane and thermal seawater desalination processes using nanofiltration membranes (Part 1). Desalination 118, 35–51. doi:10.1016/s0011-9164(98)00079-4
Hu, C.-C., Lin, C.-W., Hu, C.-P., Keshebo, D. L., Huang, S.-H., Hung, W.-S., et al. (2022). Carbon dioxide enrichment of PDMS/PSf composite membranes for solving the greenhouse effect and food crisis. J. CO2 Util. 61, 102011. doi:10.1016/j.jcou.2022.102011
Huang, S.-M., and Zhang, L.-Z. (2013). Researches and trends in membrane-based liquid desiccant air dehumidification. Renew. Sustain. Energy Rev. 28, 425–440. doi:10.1016/j.rser.2013.08.005
Hussain, A., Janson, A., Matar, J. M., and Adham, S. (2022a). Membrane distillation: recent technological developments and advancements in membrane materials. Emergent Mater 5, 347–367. doi:10.1007/s42247-020-00152-8
Hussain, G., Aleem, M., Sultan, M., Sajjad, U., Ibrahim, S. M., Shamshiri, R. R., et al. (2022b). Evaluating evaporative cooling assisted solid desiccant dehumidification system for agricultural storage application. Sustainability 14, 1479. doi:10.3390/su14031479
Jin, C., Du, S., Wang, Y., Condon, J., Lin, X., and Zhang, Y. (2009). Carbon dioxide enrichment by composting in greenhouses and its effect on vegetable production. J. Plant Nutr. Soil Sci. 172, 418–424. doi:10.1002/jpln.200700220
Khalilpour, R., Mumford, K., Zhai, H., Abbas, A., Stevens, G., and Rubin, E. S. (2015). Membrane-based carbon capture from flue gas: a review. J. Clean. Prod. 103, 286–300. doi:10.1016/j.jclepro.2014.10.050
Kneifel, K., Nowak, S., Albrecht, W., Hilke, R., Just, R., and Peinemann, K.-V. (2006). Hollow fiber membrane contactor for air humidity control: Modules and membranes. J. Membr. Sci. 276, 241–251. doi:10.1016/j.memsci.2005.09.052
Krasilnikov, P., Taboada, M. A., and Amanullah, H. (2022). Amanullah: fertilizer use, soil health and agricultural sustainability. Agriculture 12, 462. doi:10.3390/agriculture12040462
Kurihara, M., Yamamura, H., Nakanishi, T., and Jinno, S. (2001). Operation and reliability of very high-recovery seawater desalination technologies by brine conversion two-stage RO desalination system. Desalination 138, 191–199. doi:10.1016/s0011-9164(01)00264-8
Kuroyanagi, T., Yasuba, K., Higashide, T., Iwasaki, Y., and Takaichi, M. (2014). Efficiency of carbon dioxide enrichment in an unventilated greenhouse. Biosyst. Eng. 119, 58–68. doi:10.1016/j.biosystemseng.2014.01.007
Lee, W. J., Ng, Z. C., Hubadillah, S. K., Goh, P. S., Lau, W. J., Othman, M. H. D., et al. (2020). Fouling mitigation in forward osmosis and membrane distillation for desalination. Desalination 480, 114338. doi:10.1016/j.desal.2020.114338
Lefers, R., Bettahalli, N. M. S., Nunes, S. P., Fedoroff, N., Davies, P. A., and Leiknes, T. (2016). Liquid desiccant dehumidification and regeneration process to meet cooling and freshwater needs of desert greenhouses. Desalination Water Treat. 57, 23430–23442. doi:10.1080/19443994.2016.1173383
Lefers, R. M., Bettahalli, N. M. S., Fedoroff, N. V., Ghaffour, N., Davies, P. A., Nunes, S. P., et al. (2019). Hollow fibre membrane-based liquid desiccant humidity control for controlled environment agriculture. Biosyst. Eng. 183, 47–57. doi:10.1016/j.biosystemseng.2019.04.010
Lefers, R. M., Tester, M., and Lauersen, K. J. (2020). Emerging technologies to enable sustainable controlled environment agriculture in the extreme environments of Middle East-north africa coastal regions. Front. Plant Sci. 11, 801. doi:10.3389/fpls.2020.00801
Lew, B., Tarnapolski, O., Afgin, Y., Portal, Y., Ignat, T., Yudachev, V., et al. (2020). Exploratory ranking analysis of brackish groundwater desalination for sustainable agricultural production: a case study of the Arava Valley in Israel. J. Arid. Environ. 174, 104078. doi:10.1016/j.jaridenv.2019.104078
Li, J., and Ito, A. (2008). Dehumidification and humidification of air by surface-soaked liquid membrane module with triethylene glycol. J. Membr. Sci. 325, 1007–1012. doi:10.1016/j.memsci.2008.09.034
Li, J., Shao, S., Wang, Z., Xie, G., Wang, Q., Xu, Z., et al. (2022). A review of air-to-air membrane energy recovery technology for building ventilation. Energy Build. 265, 112097. doi:10.1016/j.enbuild.2022.112097
Li, J., Sui, G., Zuo, C., and Deng, Q. (2011). Air humidification by a liquid membrane of triethylene glycol. Int. Conf. Remote Sens. Environ. Transp. Eng., 7357–7359. doi:10.1109/rsete.2011.5966068
Liu, L., Zheng, X., Wei, X., Kai, Z., and Xu, Y. (2021). Excessive application of chemical fertilizer and organophosphorus pesticides induced total phosphorus loss from planting causing surface water eutrophication. Sci. Rep. 11, 23015. doi:10.1038/s41598-021-02521-7
McClements, D. J., Barrangou, R., Hill, C., Kokini, J. L., Lila, M. A., Meyer, A. S., et al. (2020). Building a resilient, sustainable, and healthier food supply through innovation and technology. Annu. Rev. Food Sci. Technol. 12, 1–28. doi:10.1146/annurev-food-092220-030824
Menegat, S., Ledo, A., and Tirado, R. (2022). Greenhouse gas emissions from global production and use of nitrogen synthetic fertilisers in agriculture. Sci. Rep. 12, 14490. doi:10.1038/s41598-022-18773-w
Moussaddy, S., Aryal, S., and Maisonneuve, J. (2024). Specific energy analysis of using fertilizer-based liquid desiccants to dehumidify indoor plant environments. Appl. Therm. Eng. 238, 121849. doi:10.1016/j.applthermaleng.2023.121849
Nayar, K. G., and V, J. H. L. (2020). Brackish water desalination for greenhouse agriculture: comparing the costs of RO, CCRO, EDR, and monovalent-selective EDR. Desalination 475, 114188. doi:10.1016/j.desal.2019.114188
Nicholson, C. F., Harbick, K., Gómez, M. I., and Mattson, N. S.: An economic and environmental comparison of conventional and controlled environment agriculture (CEA) supply chains for leaf lettuce to US cities. 33–68. (2020). doi:10.1007/978-3-030-34065-0
Norahim, N., Yaisanga, P., Faungnawakij, K., Charinpanitkul, T., and Klaysom, C. (2018). Recent membrane developments for CO2 separation and capture. Chem. Eng. Technol. 41, 211–223. doi:10.1002/ceat.201700406
Organization, U. N. E., Balaji, R., Connor, R., Glennie, P., Gun, J. van der, Lloyd, G. J., et al. (2012). The united Nations world water development report 2012. U. N. World Water Dev. Rep. doi:10.18356/bd022534-en
Panwar, N. L., Kaushik, S. C., and Kothari, S. (2011). Solar greenhouse an option for renewable and sustainable farming. Renew. Sustain. Energy Rev. 15, 3934–3945. doi:10.1016/j.rser.2011.07.030
Pasgianos, G. D., Arvanitis, K. G., Polycarpou, P., and Sigrimis, N. (2003). A nonlinear feedback technique for greenhouse environmental control. Comput. Electron. Agric. 40, 153–177. doi:10.1016/s0168-1699(03)00018-8
Pasqualin, P., and Davies, P. A. (2022). Concept design of a greenhouse cooling system using multi-stage nanofiltration for liquid desiccant regeneration. Appl. Therm. Eng. 216, 119057. doi:10.1016/j.applthermaleng.2022.119057
Pazouki, P., Sidhu, J. P. S., Ipe, D. S., Pype, M. L., Wohlsen, T. D., Helfer, F., et al. (2022). Seawater dilution desalination with hybrid FO-RO and UF-RO: characterisation and assessment of pathogen removal efficacy. Desalination 525, 115509. doi:10.1016/j.desal.2021.115509
Phuntsho, S., Shon, H. K., Hong, S., Lee, S., and Vigneswaran, S. (2011). A novel low energy fertilizer driven forward osmosis desalination for direct fertigation: evaluating the performance of fertilizer draw solutions. J. Membr. Sci. 375, 172–181. doi:10.1016/j.memsci.2011.03.038
Pimentel, D., Houser, J., Preiss, E., White, O., Fang, H., Mesnick, L., et al. (1997). Water resources: agriculture, the environment, and society. BioScience 47, 97–106. doi:10.2307/1313020
Pourmovahed, P., Lefsrud, M., and Maisonneuve, J. (2022). Thermodynamic limits of using fertilizer to produce clean fertigation solution from wastewater via forward osmosis. J. Membr. Sci. 647, 120168. doi:10.1016/j.memsci.2021.120168
Qian, Z., Miedema, H., Pintossi, D., Ouma, M., and Sudhölter, E. J. R. (2022). Selective removal of sodium ions from greenhouse drainage water – a combined experimental and theoretical approach. Desalination 536, 115844. doi:10.1016/j.desal.2022.115844
Qu, M., Abdelaziz, O., Gao, Z., and Yin, H. (2018). Isothermal membrane-based air dehumidification: a comprehensive review. Renew. Sustain. Energy Rev. 82, 4060–4069. doi:10.1016/j.rser.2017.10.067
Rabbi, B., Chen, Z.-H., and Sethuvenkatraman, S. (2019). Protected cropping in warm climates: a review of humidity control and cooling methods. Energies 12, 2737. doi:10.3390/en12142737
Ragel, P., Raddatz, N., Leidi, E. O., Quintero, F. J., and Pardo, J. M. (2019). Regulation of K+ nutrition in plants. Front. Plant Sci. 10, 281. doi:10.3389/fpls.2019.00281
Rawat, J., Pandey, N., and Saxena, J. (2022). “Role of potassium in plant photosynthesis, transport, growth, and yield,” in Role of potassium in abiotic stress. Editors N. Iqbal, and S. Umar (Singapore: Springer), 1–14. doi:10.1007/978-981-16-4461-0_1
Rehman, D., Ahdab, Y. D., and Lienhard, J. H. (2021). Monovalent selective electrodialysis: modelling multi-ionic transport across selective membranes. Water Res. 199, 117171. doi:10.1016/j.watres.2021.117171
Roy, Y., Lefsrud, M., Orsat, V., Filion, F., Bouchard, J., Nguyen, Q., et al. (2014). Biomass combustion for greenhouse carbon dioxide enrichment. Biomass Bioenergy 66, 186–196. doi:10.1016/j.biombioe.2014.03.001
Schücking, G. (2020). Monovalent selective electrodialysis in greenhouses. Master Thesis: Lund University.
Shaffer, D. L., Yip, N. Y., Gilron, J., and Elimelech, M. (2012). Seawater desalination for agriculture by integrated forward and reverse osmosis: improved product water quality for potentially less energy. J. Membr. Sci. 415, 1–8. doi:10.1016/j.memsci.2012.05.016
Shin, M. S., Jung, K.-H., Kwag, J.-H., and Jeon, Y.-W. (2019). Biogas separation using a membrane gas separator: focus on CO2 upgrading without CH4 loss. Process Saf. Environ. Prot. 129, 348–358. doi:10.1016/j.psep.2019.07.020
Sinha, A., Thakkar, H., Rezaei, F., Kawajiri, Y., and Realff, M. J. (2018). Direct air capture of CO2 in enclosed environments: design under uncertainty and techno-economic analysis. Comput. Aided Chem. Eng. 44, 2179–2184. doi:10.1016/b978-0-444-64241-7.50358-x
Soussi, M., Chaibi, M. T., Buchholz, M., and Saghrouni, Z. (2022). Comprehensive review on climate control and cooling systems in greenhouses under hot and arid conditions. Agronomy 12, 626. doi:10.3390/agronomy12030626
Stacey, N., Fox, J., and Hildebrandt, D. (2018). Reduction in greenhouse water usage through inlet CO2 enrichment. AIChE J. 64, 2324–2328. doi:10.1002/aic.16120
Strathmann, H. (2010). Electrodialysis, a mature technology with a multitude of new applications. Desalination 264, 268–288. doi:10.1016/j.desal.2010.04.069
Thongbai, P., Kozai, T., and Ohyama, K. (2010). CO2 and air circulation effects on photosynthesis and transpiration of tomato seedlings. Sci. Hortic. 126, 338–344. doi:10.1016/j.scienta.2010.07.018
Tilman, D., Balzer, C., Hill, J., and Befort, B. L. (2011). Global food demand and the sustainable intensification of agriculture. Proc. Natl. Acad. Sci. 108, 20260–20264. doi:10.1073/pnas.1116437108
Uhlinger, A. R. (2001). Desalination method and apparatus utilizing nanofiltration and reverse osmosis membranes.
Ukraine, U. N. U. of H., Hospodarenko, H. M., Prokopchuk, I. V., and Boiko, V. P. (2020). Soybean uptake of essential nutrients from soil and fertilizers. Agrochem. Soil Sci., 63–70. doi:10.31073/acss89-07
Wang, T., Huang, J., He, X., Wu, J., Fang, M., and Cheng, J. (2014). CO2 fertilization system integrated with a low-cost direct air capture technology. Energy Procedia 63, 6842–6851. doi:10.1016/j.egypro.2014.11.718
Yadav, A., Labhasetwar, P. K., and Shahi, V. K. (2021). Membrane distillation using low-grade energy for desalination: a review. J. Environ. Chem. Eng. 9, 105818. doi:10.1016/j.jece.2021.105818
Yang, H., Xu, Z., Fan, M., Gupta, R., Slimane, R. B., Bland, A. E., et al. (2008). Progress in carbon dioxide separation and capture: a review. J. Environ. Sci. 20, 14–27. doi:10.1016/s1001-0742(08)60002-9
Yoo, S. Y., Kim, Y. J., Lee, T. H., Lee, B. K., Kim, M. J., Han, S. H., et al. (2023). Membrane system for management and utilization of indoor CO2. J. Ind. Eng. Chem. 122, 161–168. doi:10.1016/j.jiec.2023.02.018
Zeidler, S. C., and Vrakking, D. and: Vertical farm 2.0: designing an economically feasible vertical farm - a combined European endeavor for sustainable urban agriculture. (2015)
Zhang, J. D., Liu, Y. W., Gao, S. M., Li, C. Z., Zhang, F., Zen, H. M., et al. (2006). Pilot testing of outside-in UF pretreatment prior to RO for high turbidity seawater desalination. Desalination 189, 269–277. doi:10.1016/j.desal.2005.07.009
Zhang, L.-Z. (2011). An analytical solution to heat and mass transfer in hollow fiber membrane contactors for liquid desiccant air dehumidification. J. Heat. Transf. 133, 092001. doi:10.1115/1.4003900
Zhang, L.-Z. (2012). Progress on heat and moisture recovery with membranes: from fundamentals to engineering applications. Energy Convers. Manag. 63, 173–195. doi:10.1016/j.enconman.2011.11.033
Zhang, L. Z., and Jiang, Y. (1999). Heat and mass transfer in a membrane-based energy recovery ventilator. J. Membr. Sci. 163, 29–38. doi:10.1016/s0376-7388(99)00150-7
Zhang, L.-Z., and Zhang, N. (2014). A heat pump driven and hollow fiber membrane-based liquid desiccant air dehumidification system: modeling and experimental validation. Energy 65, 441–451. doi:10.1016/j.energy.2013.10.014
Zhang, N., Yin, S.-Y., and Zhang, L.-Z. (2016). Performance study of a heat pump driven and hollow fiber membrane-based two-stage liquid desiccant air dehumidification system. Appl. Energy. 179, 727–737. doi:10.1016/j.apenergy.2016.07.037
Zhang, S., Ou, R., Ma, H., Lu, J., Holl, M. M. B., and Wang, H. (2021). Thermally regenerable metal-organic framework with high monovalent metal ion selectivity. Chem. Eng. J. 405, 127037. doi:10.1016/j.cej.2020.127037
Keywords: membrane gas separator, reverse osmosis, controlled environment agriculture (CEA), Dehumidication, membranes
Citation: Safari P, Rahnema H and Lipscomb G (2024) Opportunities for membrane technology in controlled environment agriculture. Front. Membr. Sci. Technol. 3:1406326. doi: 10.3389/frmst.2024.1406326
Received: 24 March 2024; Accepted: 11 April 2024;
Published: 29 April 2024.
Edited by:
Nalan Kabay, Ege University, TürkiyeReviewed by:
Marek Bryjak, Wrocław University of Technology, PolandSacide Alsoy Altinkaya, Izmir Institute of Technology, Türkiye
Copyright © 2024 Safari, Rahnema and Lipscomb. This is an open-access article distributed under the terms of the Creative Commons Attribution License (CC BY). The use, distribution or reproduction in other forums is permitted, provided the original author(s) and the copyright owner(s) are credited and that the original publication in this journal is cited, in accordance with accepted academic practice. No use, distribution or reproduction is permitted which does not comply with these terms.
*Correspondence: Glenn Lipscomb, parisa.safari@rockets.utoledo.edu