Molecular approaches and challenges for monitoring marine harmful algal blooms in a changing world
- 1Molecular and Algal Ecology Group, Cawthron Institute, Nelson, New Zealand
- 2School of Biological Sciences, Victoria University of Wellington, Wellington, New Zealand
Harmful algal blooms (HABs) of marine microalgae are a growing concern globally and many studies predict that their occurrence, range, and toxicity may increase with climate change, highlighting the need for responsive and adaptable monitoring techniques. Monitoring programmes for HABs require appropriate sampling techniques, accurate and quantitative identification of potentially toxic taxa, and the ability to respond to novel species or HAB events. These challenges have made it difficult to move past traditional techniques, but research tools are continuing to be developed to bring new opportunities for both monitoring programmes and fundamental understanding of these globally significant organisms within a changing landscape.
1 Introduction
Eukaryotic microalgae occur throughout the world’s aquatic environments and are crucial components of our ecosystem. They form the base of aquatic food webs, drive biogeochemical processes, and influence the global climate. The biodiversity of all algae is enormous and has been estimated that about 200,000-800,000 species in many different genera exist of which approximately 40,000 are microalgal species (Guiry, 2012). A small percentage (approximately 300 species) can form harmful algal blooms (HABs) (Hallegraeff, 2003) and these species can produce a wide variety of toxins or bioactive compounds. Eukaryotic marine HABs can have detrimental impacts on other marine life (Rolton et al., 2022) and/or on humans through recreational activities or contaminated seafood (Berdalet et al., 2016; Hallegraeff et al., 2021b; Yan et al., 2022), with most of these belonging to the dinoflagellates. Other eukaryotic marine HAB taxa belong to the diatom, haptophyte, raphidophyte, and dictyochocales groups (Figure 1) (Lundholm et al., 2009 onwards). Toxins produced by HAB species that accumulate in seafood through trophic transfer in the marine food web are relatively well characterized (Anderson et al., 2021; Zingone et al., 2021; Hallegraeff et al., 2021b) while the mechanism of toxicity for those species that are associated with mortality or reduced health of marine fauna, are less understood (Rolton et al., 2022).
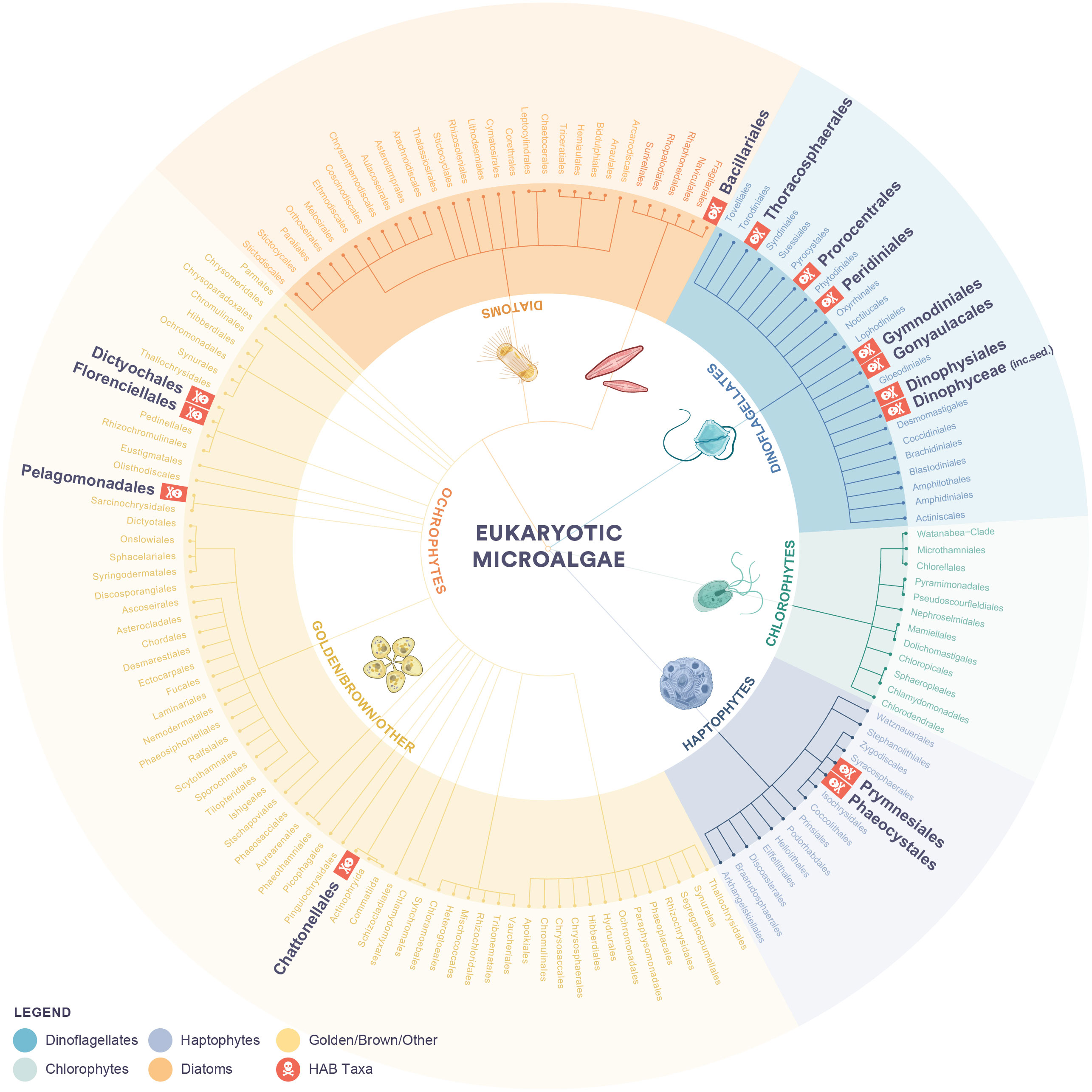
Figure 1 The taxonomic groups of eukaryotic marine microalgae with orders that contain harmful algal bloom species highlighted. Taxonomy was sourced from the PR2 database (Guillou et al., 2012) and the IOC-UNESCO Taxonomic Reference List of Harmful Microalgae (Lundholm et al., 2009 onwards).
Many of these HAB species have been thoroughly researched to allow the development of risk assessments. Such assessments are critical for the development of suitable monitoring systems to enable some form of management, prevention, or mitigation of these events (Anderson, 2009). Determining accurate taxonomy of toxic species, understanding their associated toxin production, and predicting the spatial patterns and occurrences of HABs, have been long-standing challenges in the field. Recently, these challenges have intensified due to the implications of oceanic responses to environmental changes. HAB dynamics are likely to change with warming seas, and to investigate bloom-environment-climate interactions meaningfully, baseline data is needed. To this end, for example, the microalgae found in subtropical areas of Aotearoa New Zealand have been studied for many years and the potential arrival of warmer water dinoflagellates, such as Gambierdiscus polynesiensis (the cause of Ciguatera Poisoning in the Pacific) is being monitored through an Aotearoa New Zealand government funded research programme (Rhodes et al., 2020; Rhodes et al., 2023).
Toxic species have often been determined through investigations following illnesses or deaths of humans and/or marine fauna. An example is the discovery of the producer of the neurotoxin, domoic acid, found in mussels in Canada, and which caused serious illnesses, and even deaths of, consumers. A concerted effort by Canadian scientists from many fields led to the rapid tracking of the diatom Pseudo-nitzschia multiseries (then classified as Nitzschia pungens) as the causative microalga (Bates et al., 1989). There are currently 63 species of Pseudo-nitzschia listed on the taxonomy browser AlgaeBase and monitoring of risk is facilitated by targeted molecular assays, for example, Kim et al. (2017) and Andree et al. (2011).
The dinoflagellate genus Karenia is another example of intensive research leading to the pinpointing of K. brevis (previously Gymnodinium breve) as the cause of Florida red tides, which cause neurotoxic shellfish poisoning (Steidinger, 2009). In Aotearoa New Zealand massive mortalities of marine biota, and respiratory problems in humans, lead to the description of K. brevisulcata (Chang, 2011) and the characterizing of K. brevisulcata toxins (KBTs) and brevisulcatic acids (BSXs) (Holland et al., 2012). Mass cultures of isolates for toxin testing and toxicity tests have led to a considerable amount of information being generated for risk assessments following analysis of monitoring data.
2 Monitoring techniques for harmful algal bloom species
The diversity of marine microalgae present at any one site can be difficult to determine. Even the first, seemingly simple, step of obtaining a representative seawater sample requires some thought. In deep oceans and coastal areas plankton hauls (Figure 2A) using nets may be used but need to have a fine enough mesh to capture small harmful species, which in some cases can be less than 10 µm. Plankton net hauls are a qualitative approach but have the benefit of capturing microalgae from a much larger water sample and concentrating the biomass. This may be useful for locations with low biomass of microalgae in the water column or if the target species is rare. Plankton net hauls are also useful for collecting samples to isolate specimens for laboratory culture. Bottle type, Van Dorn (Figures 2B, C) or tube samplers (Figure 2D) allow the quantification of cells per litre (Franks and Keafer, 2003), a requirement for many HAB monitoring programmes. In Aotearoa New Zealand the Marine Biotoxin Monitoring Programme samples sites around the coast weekly using bottle, Van Dorn or tube samplers for depth integrated samples. Tube samplers allow a depth integrated sample to be collected. A weighted tube is lowered slowly through the water column to the required depth and the sample is retrieves by placing a bung securely in the top of tube and then pulling the tube out of the water. The sample is then emptied into a sampling bottle. Microalgae from samples are identified and enumerated using Utermöhl chambers (Utermöhl, 1958) and inverted light microscopes for potentially toxic taxa and if they are over a certain species-specific threshold, a warning to seafood managers and public health officials is triggered. These data are used to inform commercial and wild seafood harvest as well as alert for potential health risks for shellfish and finfish farms and public safety (Rolton et al., 2022).
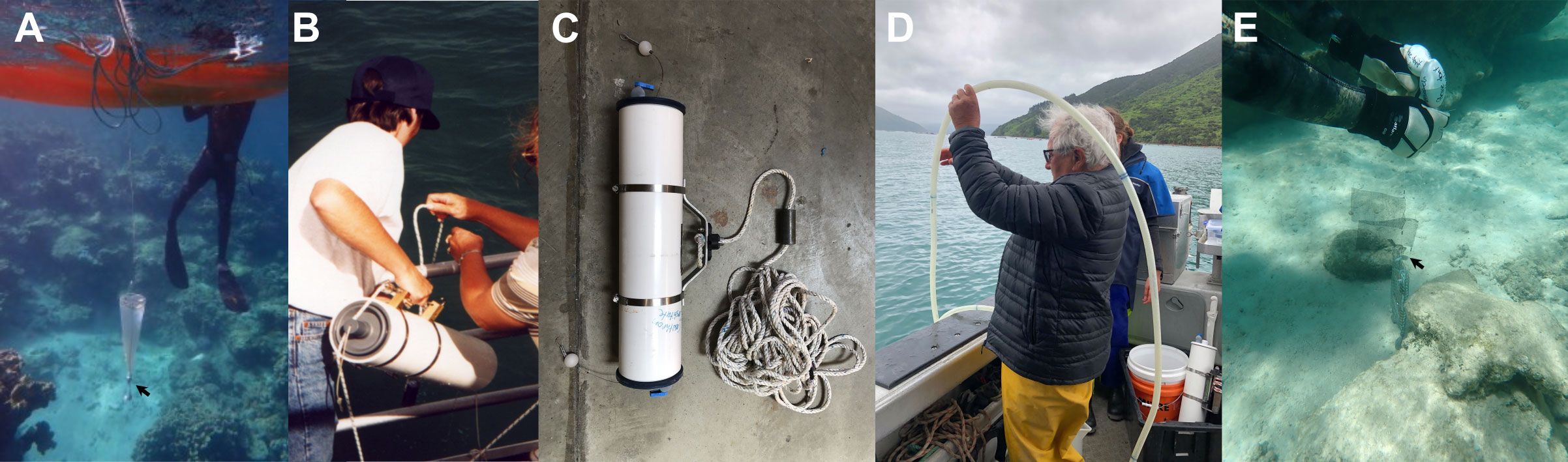
Figure 2 Examples of sampling techniques used for monitoring harmful algal bloom species, for both planktonic and benthic/epiphytic taxa. (A) plankton net (black arrow indicates the bottom of the net), (B, C) Van Dorn, (D) tube sampler, (E) artificial substrate sampler of fiberglass mesh (black arrow indicates the artificial substrate). Approaches (A-D) are for planktonic species, and approach (E) is for benthic species.
However, most monitoring systems only sample the water column, and these approaches will not include epiphytic and benthic microflora, which are an increasing HAB risk in many geographic areas. Different approaches are required to capture those taxa that live associated with macroalgae, corals and other biotic and abiotic substrates (Reguera et al., 2016). Currently there is no standardized approach for a quantitative method of sampling benthic dinoflagellates (Tester et al., 2022). Historically, a common method has been the collection of macroalgae from a site which are then shaken to dislodge the epiphytic microalgae and the cells either assessed as presence-absence or normalized to cells per gram wet weight of the macroalgae (Chinain et al., 1999; Reguera et al., 2016). This approach has several weaknesses including variation in macroalgal presence across sites, different surface areas of macroalgal species, the destructive sampling methodology, and potentially, macroalgal host preferences by microalgae, as discussed by Tester et al. (2022).
In recent years, several sampling devices have been developed to address these challenges and allow the quantification of cell abundances in relation to a standardized surface area. Examples include the Benthic Dinoflagellate Integrator (BEDI) (Mangialajo et al., 2017) and artificial substrates or screens (Figure 2E) (Tester et al., 2022). The artificial substrate technique is rapidly being adopted by the scientific community. It is being widely tested and has proved useful for embayments and coral reef lagoons (Fernández-Zabala et al., 2019; Tester et al., 2022). However, the type of artificial substrate to be deployed needs to be evaluated at each location or environment to establish the relationship between cell densities detected from macroalgal sampling versus substrate samplers (Parsons et al., 2017). These sampling devices also have the benefit of samples being relatively clean of sediment and therefore easily integrated into other downstream techniques including molecular identification tools (see below).
Enumeration of cells from harmful algal blooms and microalgal communities using manual counting and light microscopy with the Utermöhl cell counting method (Utermöhl, 1958) is still commonly used (Andersen and Throndsen, 2003). This can be physically taxing and time consuming, requires highly skilled taxonomic expertise and thus long training periods, and may require high frequency sampling. Additionally, harmful taxa can be nearly impossible to identify accurately to species level, and many cryptic species exist. New approaches are being developed that resolve some of these issues or offer alternative methods to detecting HAB events, such as methods based on artificial neural networks, automated flowcytometry, autonomous underwater vehicles and satellite imaging (Seltenrich, 2014; Agarwal et al., 2023; Gernez et al., 2023; Otálora et al., 2023).
3 Molecular approaches to taxonomy and monitoring programmes
To fully characterize the taxonomic diversity of microalgae, culturing, and maintenance of unialgal strains is critical. Microalgal culture collections provide a repository of diversity, albeit only those strains that can be cultured, protection against the loss of genetic and functional variation, and provision of material for research, development, training, and auditing monitoring programmes (Rhodes et al., 2016). To ensure live microalgae can be isolated and cultured for DNA sequencing and testing for toxin production, samples need maintenance at a suitable temperature, light and nutrients conditions. Culturing has its own difficulties as each species will have its own characteristics and therefore requirements (Guillard and Morton, 2003). Molecular tools for HAB detection have increased rapidly in recent decades but their develop relies on understanding diversity of species and strains at a local scale. Assay will continue to be refined as new species are described and so isolation of mono-algal strains to aid in the description of novel species continue to be critical.
Polymerase chain reaction (PCR) in various formats (e.g., quantitative PCR (qPCR); digital PCR (dPCR)) has been increasingly utilized for marine phytoplankton studies world-wide (e.g., Wood et al., 2013; Zaiko et al., 2022). It is cheap (<$10 USD per sample for consumables; Karlson et al., 2010), rapid, highly sensitive and has the potential to be adapted into field deployable methods (Yamahara et al., 2015; Pearson et al., 2021). However, molecular expertise and expensive equipment is required as start-up costs. Thorough optimization and specificity testing on local strains and sample types is also required before an assays can be implemented in a monitoring programme (Karlson et al., 2010). Currently qPCR/dPCR are the most likely molecular-based techniques to be implemented into HAB monitoring programmes, with various options for multiplexing and gene targets including toxin biosynthesis genes, such as those underlying saxitoxin production (Murray et al., 2011). However, it can be difficult to obtain accurate cell concentrations using molecular methods due to variation at even the strain level in gene copy number per cell. Only a few examples of molecular-based HAB monitoring programmes exist. A good example comes from Ireland with the use of qPCR assays for the molecular detection of Azadinium spinosum, Az. obesum and Az. poporum as part of the National Monitoring Programme of Ireland (McGirr et al., 2021. In New Zealand, fluorescent in situ hybridization (FISH) assays have been used since the late 1990s to identify toxic Pseudo-nitzschia spp. and provides a relative abundance result (Rhodes et al., 1998). qPCR assays are also currently implemented in support of the microscopy-based monitoring to confirm identifications (Smith et al., 2014).
Other approaches are constantly being developed in this fast-moving field, for example, the use of CRISPR/Cas (Clustered Regularly Interspaced Short Palindromic Repeats/their associated proteins Cas) for next generation biosensing (Pal et al., 2023). The main drawback to specific assays is that genomic or gene sequence data is required to develop the assays and the target species need to be known before the sample is analyzed. In response to this issue the application of environmental DNA amplicon sequencing has been rapidly adapted as a research tool for HAB investigations. Also termed metabarcoding, this technique utilizes high-throughput sequencing coupled with curated sequence databases. Metabarcoding is especially useful when flexibility is required, for example, when surveying relatively uncharacterized ecosystems or species distributions are changing (Smith et al., 2017a; Smith et al., 2017b; Cui et al., 2021). Much thought is needed for the primers used and gene targets, depending on the research question or aim. Fragment length and nucleotide polymorphism can also impact on the taxonomic resolution that can be achieved, and this can vary depending on the species. Additionally, a reliable link between gene copy number and cell concentration in water samples still needs to be established for each gene/species target (Ruvindy et al., 2023). The use of multiple gene regions to fully capture diversity and reduce bias is often recommended. Long read sequencing capabilities (e.g., Oxford Nanopore technologies) may offer a solution to these issues with potentially lower cost and more portable formats. If multiplexing samples, long-read sequencing cost per sample can be very economical (approximately $20–30 USD not including DNA extraction) (Hatfield et al., 2020), however, current turnaround times are generally longer than required by biotoxin regulators and seafood managers (Rolton et al., 2022).
Functional genomics is providing huge understanding of the genetic basis underlying the important traits related to ecological, socioeconomic, and ecosystem health impacts of HAB species (Murray et al., 2016). New tools for omic approaches including genomics, transcriptomics, proteomics and metabolomics, are driving a rapid increase in the understanding of HAB dynamics (Hennon and Dyhrman, 2020). Microalgal genomes can vary significantly in size and include some of the largest haploid genomes known with many unique features relating to structure and sequence (e.g., dinoflagellates) (Ishida et al., 2023), making sequencing of whole genome very difficult. However, de novo transcriptomic studies have enabled gene discovery for many species and ongoing research will continue to add crucial information (Murray et al., 2016). Omic datasets are also complementary to each other and allow the characterization of genes and proteins via multiple datasets, demonstrating the strength of meta-analyses (Hennon and Dyhrman, 2020). Systems biology and the connections between genomes, gene products and the environment, both abiotic and biotic factors, will be key to understanding the role and function of HAB species including their response to global climatic changes.
4 Global change impacts
Global change is being expressed in the world’s oceans by rising sea temperatures, changes in ocean currents and salinity, anthropogenically caused coastal and ocean acidification, and increases in nutrient and trace metal runoff. It is likely that these changes will alter the dynamics of HAB formation, including range expansion, increased frequency and occurrence, and potentially increased toxicity (Gobler, 2020; Rhodes et al., 2023). There is strong evidence that phytoplankton blooms are increasing worldwide (Dai et al., 2023). However, trends can be difficult to resolve due to availability of HAB datasets, lack of spatial sampling and a bias towards reporting of blooms only when seafood becomes sufficiently toxic to generate human health hazards or cause economic damage (Hallegraeff et al., 2021a) Predicting how HABs will respond to global change is difficult due to variation at the species/strain level, interactive effects of multi-stressor factors, limitations to species that are culturable, and adaptative responses (Hennon and Dyhrman, 2020). On-going research is critical both to monitor changes and to enable warnings of potential risk. Monitoring programmes need to be flexible to detect new incursions and adaptable to changing risk profiles. Targeted or automated approaches for detecting HABs could potentially miss novel blooms or species that have been rare in the past. Taxonomists also need consistent awareness and upskilling. Monitoring of sentinel sites or seafood species that can accumulate toxins are a good approach for reducing this risk (Rhodes et al., 2023). The development of models using predicted climate scenarios, particularly in coastal zones, will aid in identifying areas to prioritize monitoring and for which species.
5 Conclusions
Research tools for HABs and the causative species are consistently being developed and refined, ranging from molecular techniques to satellite imaging and include many opportunities for automation and in situ monitoring. Due to requirements for both highly specific and adaptable techniques to ensure that monitoring programmes are future-proofed for any geographic changes in species distributions (e.g., the spread of new HAB species into new areas), many of these tools have not been transferred to official monitoring programmes, and most countries still rely heavily on taxonomic identification using light microscopy. New omic techniques show great promise encompassing taxonomic resolution and functional understanding of HABs, but still lack the rapid turnaround time required for routine application. With continued development to reduce the time lag, understanding of gene copy number vs cells concentrations and the reference databases available metabarcoding offers many solutions. Modeling of predicted HAB sceneries will be crucial tools for environmental managers, public health organizations and seafood industries to prepare for the future. With global change already impacting on our microalgal communities, both planktonic and benthic, and the occurrence of HABs, we need to be prepared with responsive and adaptable monitoring techniques that will reduce the impact of HABs on coastal ecosystems and human health.
Ethics statement
Written informed consent was obtained from Dr Lincoln Mackenzie for the publication of any potentially identifiable images or data included in this article.
Author contributions
KS: Conceptualization, Writing – original draft. JS: Writing – original draft. LR: Conceptualization, Writing – original draft.
Funding
The author(s) declare financial support was received for the research, authorship, and/or publication of this article. The research was funded by the New Zealand Ministry for Business, Innovation and Employment (Seafood Safety Platform, Contract No. CAWX1801; National Databases Contract No. CAWX0902).
Conflict of interest
The authors declare that the research was conducted in the absence of any commercial or financial relationships that could be construed as a potential conflict of interest.
The author(s) declared that they were an editorial board member of Frontiers, at the time of submission. This had no impact on the peer review process and the final decision.
Publisher’s note
All claims expressed in this article are solely those of the authors and do not necessarily represent those of their affiliated organizations, or those of the publisher, the editors and the reviewers. Any product that may be evaluated in this article, or claim that may be made by its manufacturer, is not guaranteed or endorsed by the publisher.
References
Agarwal V., Chávez-Casillas J., Mouw C. B. (2023). Sub-monthly prediction of harmful algal blooms based on automated cell imaging. Harmful Algae 122, 102386. doi: 10.1016/j.hal.2023.102386
Andersen P., Throndsen J. (2003). “Estimating cell numbers,” in Manual on harmful marine microalgae. Eds. Hallegraeff G. M., Anderson D. M., Cembella A. D., Envoldsen H. O. (IOC, UNESCO Publishing), 99–129.
Anderson D. M. (2009). Approaches to monitoring, control and management of harmful algal blooms (HABs). Ocean Coast. Manage. 52, 342. doi: 10.1016/j.ocecoaman.2009.04.006
Anderson D. M., Fensin E., Gobler C. J., Hoeglund A. E., Hubbard K. A., Kulis D. M., et al. (2021). Marine harmful algal blooms (HABs) in the United States: History, current status and future trends. Harmful Algae 102, 101975. doi: 10.1016/j.hal.2021.101975
Andree K. B., Fernández-Tejedor M., Elandaloussi L. M., Quijano-Scheggia S., Sampedro N., Garcés E., et al. (2011). Quantitative PCR coupled with melt curve analysis for detection of selected Pseudo-nitzschia spp. (Bacillariophyceae) from the Northwestern Mediterranean Sea. Appl. Environ. Microbiol. 77, 1651–1659. doi: 10.1128/AEM.01978-10
Bates S. S., Bird C. J., de Freitas A. S. W., Foxall R., Gilgan M., Hanic L. A., et al. (1989). Pennate diatom Nitzschia pungens as the primary source of domoic acid, a toxin in shellfish from eastern Prince Edward Island, Canada. Can. J. Fisheries Aquat. Sci. 46, 1203–1215. doi: 10.1139/f89-156
Berdalet E., Fleming L. E., Gowen R., Davidson K., Hess P., Backer L. C., et al. (2016). Marine harmful algal blooms, human health and wellbeing: challenges and opportunities in the 21st century. J. Mar. Biol. Assoc. United Kingdom 96, 61–91. doi: 10.1017/S0025315415001733
Chang F. H. (2011). Toxic effects of three closely-related dinoflagellates, Karenia concordia, K. brevisulcata and K. mikimotoi (Gymnodiniales, Dinophyceae) on other microalgal species. Harmful Algae 10, 181–187. doi: 10.1016/j.hal.2010.09.004
Chinain M., Germain M., Deparis X., Pauillac S., Legrand A. M. (1999). Seasonal abundance and toxicity of the dinoflagellate Gambierdiscus spp. (Dinophyceae), the causative agent of ciguatera in Tahiti, French Polynesia. Mar. Biol. 135, 259–267. doi: 10.1007/s002270050623
Cui Z., Xu Q., Gibson K., Liu S., Chen N. (2021). Metabarcoding analysis of harmful algal bloom species in the Changjiang Estuary, China. Sci. Total Environ. 782, 146823. doi: 10.1016/j.scitotenv.2021.146823
Dai Y., Yang S., Zhao D., Hu C., Xu W., Anderson D. M. (2023). Coastal phytoplankton blooms expand and intensify in the 21st century. Nature 615, 280–284. doi: 10.1038/s41586-023-05760-y
Fernández-Zabala J., Tuya F., Amorim A., Soler-Onís E. (2019). Benthic dinoflagellates: Testing the reliability of the artificial substrate method in the Macaronesian region. Harmful Algae 87, 101634. doi: 10.1016/j.hal.2019.101634
Franks P. J. S., Keafer B. A. (2003). “Sampling techniques and strategies for coastal phytoplankton blooms,” in Manual on harmful marine microalgae. Eds. Hallegraeff G. M., Anderson D. M., Cembella A. D., Envoldsen H. O. (IOC, UNESCO Publishing), 51–76.
Gernez P., Zoffoli M. L., Lacour T., Fariñas T. H., Navarro G., Caballero I. (2023). The many shades of red tides: Sentinel-2 optical types of highly concentrated harmful algal blooms. Remote Sens. Environ. 287, 113486. doi: 10.1016/j.rse.2023.113486
Gobler C. J. (2020). Climate change and harmful algal blooms: insights and perspective. Harmful Algae 91, 101731. doi: 10.1016/j.hal.2019.101731
Guillard R. R. L., Morton S. L. (2003). “Culture methods,” in Manual on harmful marine microalgae. Eds. Hallegraeff G. M., Anderson D. M., Cembella A. D., Envoldsen H. O. (IOC, UNESCO publishing), 77–98.
Guillou L., Bachar D., Audic S., Bass D., Berney C., Bittner L., et al (2012). The Protist Ribosomal Reference database (PR2): a catalog of unicellular eukaryote small sub-unit rRNA sequences with curated taxonomy. Nucleic Acids Res 41, D597–D604.
Guiry M. D. (2012). How many species of algae are there? J. Phycol. 48, 1057–1063. doi: 10.1111/j.1529-8817.2012.01222.x
Hallegraeff G. M. (2003). “Harmful algal blooms: a global overview,” in Manual on harmful marine microalgae. Eds. Hallegraeff G. M., Anderson D. M., Cembella A. D., Envoldsen H. O. (IOC, UNESCO Publishing), 25–50.
Hallegraeff G. M., Anderson D. M., Belin C., Bottein M. Y. D., Bresnan E., Chinain M., et al. (2021a). Perceived global increase in algal blooms is attributable to intensified monitoring and emerging bloom impacts. Commun. Earth Environ. 2, 117. doi: 10.1038/s43247-021-00178-8
Hallegraeff G. M., Schweibold L., Jaffrezic E., Rhodes L., MacKenzie L., Hay B., et al. (2021b). Overview of Australian and New Zealand harmful algal species occurrences and their societal impacts in the period 1985 to 2018, including a compilation of historic records. Harmful Algae 102, 101848. doi: 10.1016/j.hal.2020.101848
Hatfield R. G., Batista F. M., Bean T. P., Fonseca V. G., Santos A., Turner A. D., et al. (2020). The application of nanopore sequencing technology to the study of dinoflagellates: a proof-of-concept study for rapid sequence-based discrimination of potentially harmful algae. Front. Microbiol. 11, 844. doi: 10.3389/fmicb.2020.00844
Hennon G. M., Dyhrman S. T. (2020). Progress and promise of omics for predicting the impacts of climate change on harmful algal blooms. Harmful Algae 91, 101587. doi: 10.1016/j.hal.2019.03.005
Holland P. T., Shi F., Satake M., Hamamoto Y., Ito E., Beuzenberg V., et al. (2012). Novel toxins produced by the dinoflagellate Karenia brevisulcata. Harmful Algae 13, 47–57. doi: 10.1016/j.hal.2011.10.002
Ishida H., John U., Murray S. A., Bhattacharya D., Chan C. X. (2023). Developing model systems for dinoflagellates in the post-genomic era. J. Phycol. 59, 799–808. doi: 10.1111/jpy.13386
Karlson B., Cusack C., Bresnan E. (2010). “Intergovernmental oceanographic commission of ©UNESCO. Microscopic and molecular methods for quantitative phytoplankton analysis,” in IOC Manuals and Guides, no. 55 (Paris: UNESCO).
Kim J. H., Kim J.-H., Park B. S., Wang P., Patidar S. K., Han M.-S. (2017). Development of a QPCR assay for tracking the ecological niches of genetic sub-populations within Pseudo-nitzschia pungens (Bacillariophyceae). Harmful Algae 63, 68–78. doi: 10.1016/j.hal.2016.12.002
Lundholm N., Churro C., Escalera L., Fraga S., Hoppenrath M., Iwataki M., et al. (2009 onwards) IOC-UNESCO Taxonomic Reference List of Harmful Micro Algae. Available at: https://www.marinespecies.org/hab (Accessed 2023-09-27).
Mangialajo L., Fricke A., Perez-Gutierrez G., Catania D., Jauzein C., Lemee R. (2017). Benthic dinoflagellate integrator (BEDI): a new method for the quantification of benthic harmful algal blooms. Harmful Algae 64, 1–10. doi: 10.1016/j.hal.2017.03.002
McGirr S., Clarke D., Kilcoyne J., Salas R., Koehler H., Silke J., et al. (2021). Insights into the discrepancy between Azadinium spp. and azaspiracid toxins near strategically important aquaculture operations in the west and southwest of Ireland. Estuar. Coast. Shelf Sci. 262, 107622. doi: 10.1016/j.ecss.2021.107622
Murray S. A., Suggett D. J., Doblin M. A., Kohli G. S., Seymour J. R., Fabris M., et al. (2016). Unravelling the functional genetics of dinoflagellates: a review of approaches and opportunities. Perspect. Phycol. 3, 37–52. doi: 10.1127/pip/2016/0039
Murray S. A., Wiese M., Stüken A., Brett S., Kellmann R., Hallegraeff G., et al. (2011). sxtA-based quantitative molecular assay to identify saxitoxin-producing harmful algal blooms in marine waters. Appl. Anvironmental Microbiol. 77, 7050–7057. doi: 10.1128/AEM.05308-11
Otálora P., Guzmán J. L., Acién F. G., Berenguel M., Reul A. (2023). An artificial intelligence approach for identification of microalgae cultures. New Biotechnol. 77, 58–67. doi: 10.1016/j.nbt.2023.07.003
Pal P., Anand U., Saha S. C., Sundaramurthy S., Okeke E. S., Kumar M., et al. (2023). Novel CRISPR/Cas technology in the realm of algal bloom biomonitoring: Recent trends and future perspectives. Environ. Res. 231, 115989. doi: 10.1016/j.envres.2023.115989
Parsons M. L., Brandt A. L., Ellsworth A., Leynse A. K., Rains L. K., Anderson D. M. (2017). Assessing the use of artificial substrates to monitor Gambierdiscus populations in the Florida Keys. Harmful Algae 68, 52–66. doi: 10.1016/j.hal.2017.07.007
Pearson L. A., D’Agostino P. M., Neilan B. A. (2021). Recent developments in quantitative PCR for monitoring harmful marine microalgae. Harmful Algae 108, 102096. doi: 10.1016/j.hal.2021.102096
Reguera B., Alonso R., Moreira A., Méndez S., Dechraoui-Bottein M.-Y. (Eds.) (2016). “Guide for designing and implementing a plan to monitor toxin-producing microalgae,” in IOC Manuals and Guides, no. 59, 2nd Ed (Paris and Vienna: Intergovernmental Oceanographic Commission (IOC) of UNESCO and International Atomic Energy Agency (IAEA), 66 pages.
Rhodes L. L., Scholin C., Garthwaite I. (1998). Pseudo-nitzschia in New Zealand and the role of DNA probes and immunoassays in refining marine biotoxin monitoring programmes. Natural Toxins 6, 105–111. doi: 10.1002/(SICI)1522-7189(199805/08)6:3/4<105::AID-NT13>3.0.CO;2-9
Rhodes L. L., Smith K. F., MacKenzie L., Wood S. A., Ponikla K., Harwood D. T., et al. (2016). The Cawthron Institute Culture Collection of Micro-algae: a significant national collection. New Z. J. Mar. Freshw. Res. 50, 291–316. doi: 10.1080/00288330.2015.1116450
Rhodes L. L., Smith K. F., Murray J. S., Nishimura T., Finch S. C. (2020). Ciguatera fish poisoning: the risk from an Aotearoa/New Zealand perspective. Toxins 12, 50. doi: 10.3390/toxins12010050
Rhodes L. L., Smith K. F., Murray J. S., Passfield E. M. F., D’Archino R., Nelson W., et al. (2023). The benthic and epiphytic dinoflagellates of sub-tropical Aotearoa New Zealand and Rangitāhua Kermadec Islands. Harmful Algae 128, 102494. doi: 10.1016/j.hal.2023.102494
Rolton A., Rhodes L., Nishimura T., Biessy L., Bui T., MacKenzie L., et al. (2022). Effects of Harmful Algal Blooms on cultured bivalve and finfish species: relevance for New Zealand in a changing environment. Toxins 14, 341. doi: 10.3390/toxins14050341
Ruvindy R., Barua A., Bolch C. J., Sarowar C., Savela H., Murray S. A. (2023). Genomic copy number variability at the genus, species and population levels impacts in situ ecological analyses of dinoflagellates and harmful algal blooms. ISME Commun. 3, 70. doi: 10.1038/s43705-023-00274-0
Seltenrich N. (2014). Keeping tabs on HABs: new tools for detecting, monitoring, and preventing harmful algal blooms. Environ. Health Perspect. 122, 8. doi: 10.1289/ehp.122-A206
Smith K. F., Biessy L., Argyle P. A., Trnski T., Halafihi T., Rhodes L. L. (2017a). Molecular identification of Gambierdiscus and Fukuyoa (Dinophyceae) from environmental samples. Mar. Drugs 15, 243. doi: 10.3390/md15080243
Smith K. F., De Salas M., Adamson J., Rhodes L. L. (2014). Rapid and accurate identification by real-time PCR of biotoxin-producing dinoflagellates from the family Gymnodiniaceae. Mar. Drugs 12, 1361–1376. doi: 10.3390/md12031361
Smith K. F., Kohli G. S., Murray S. A., Rhodes L. L. (2017b). Assessment of the metabarcoding approach for community analysis of benthic-epiphytic dinoflagellates using mock communities. New Z. J. Mar. Freshw. Res. 51, 555–576. doi: 10.1080/00288330.2017.1298632
Steidinger K. A. (2009). Historical perspective on Karenia brevis red tide research in the Gulf of Mexico. Harmful Algae 8, 549–561. doi: 10.1016/j.hal.2008.11.009
Tester P. A., Litaker R. W., Soler-Onís E., Fernández-Zabala J., Berdalet E. (2022). Using artificial substrates to quantify Gambierdiscus and other toxic benthic dinoflagellates for monitoring purposes. Harmful Algae 120, 102351. doi: 10.1016/j.hal.2022.102351
Utermöhl H. (1958). Zur vervollkomnung der quantitativen phytoplankton metodik. Internationale Vereinigung für theoretische und angewandte Limnologie: Mitt. 9, 1–38. doi: 10.1080/05384680.1958.11904091
Wood S. A., Smith K. F., Banks J. C., Tremblay L. A., Rhodes L. L., Mountfort D., et al. (2013). Molecular genetic tools for environmental monitoring of New Zealand’s aquatic habitats, past, present and the future. New Z. J. Mar. Freshw. Res. 47, 90–119. doi: 10.1080/00288330.2012.745885
Yamahara K. M., Demir-Hilton E., Preston C. M., Marin R. III, Pargett D., Roman B., et al. (2015). Simultaneous monitoring of faecal indicators and harmful algae using an in-situ autonomous sensor. Lett. Appl. Microbiol. 61, 130–138. doi: 10.1111/lam.12432
Yan T., Li X. D., Tan Z. J., Yu R. C., Zou J. Z. (2022). Toxic effects, mechanisms, and ecological impacts of harmful algal blooms in China. Harmful Algae 111, 102148. doi: 10.1016/j.hal.2021.102148
Zaiko A., von Ammon U., Stuart J., Smith K. F., Yao R., Welsh M., et al. (2022). Assessing the performance and efficiency of environmental DNA/RNA capture methodologies under controlled experimental conditions. Methods Ecol. Evol. 13, 1581–1594. doi: 10.1111/2041-210X.13879
Keywords: HABs, climate change, sampling techniques, molecular tools, functional genomics, metabarcoding, quantitative PCR
Citation: Smith KF, Stuart J and Rhodes LL (2024) Molecular approaches and challenges for monitoring marine harmful algal blooms in a changing world. Front. Protistol. 1:1305634. doi: 10.3389/frpro.2023.1305634
Received: 02 October 2023; Accepted: 29 December 2023;
Published: 16 January 2024.
Edited by:
Panagiota Katikou, Veterinary Research Institute Greek Agricultural Organization Demeter, GreeceReviewed by:
Elisa Berdalet, Spanish National Research Council (CSIC), SpainGustaaf Marinus Hallegraeff, University of Tasmania, Australia
Copyright © 2024 Smith, Stuart and Rhodes. This is an open-access article distributed under the terms of the Creative Commons Attribution License (CC BY). The use, distribution or reproduction in other forums is permitted, provided the original author(s) and the copyright owner(s) are credited and that the original publication in this journal is cited, in accordance with accepted academic practice. No use, distribution or reproduction is permitted which does not comply with these terms.
*Correspondence: Kirsty F. Smith, kirsty.smith@cawthron.org.nz