Solar energy for societal decarbonisation: a perspective on emerging opportunities in utility-scale solar electricity and solar in the built environment
- Department of Physics, Center for Integrative Semiconductor Materials, Swansea University, Swansea, United Kingdom
The solar resource presents almost limitless opportunities for creating clean energy – not just in the form of solar-derived electricity, but also to drive heating and cooling cycles, create solar fuels, or indeed to promote chemical and biochemical pathways for the production of food, clean water and raw materials. There is an ever-expanding plethora of applications for solar energy as a critical contributor to global decarbonization. This short Perspective is focused on two of the largest opportunities for solar energy to make major emissions reduction and deliver societal fairness and economic impacts: notably utility-scale electricity generation and the more local opportunities of the built environment. We examine the two dominant forms of solar energy – the direct generation of electrical power via the photovoltaic effect, and the indirect generation of electricity (and indeed heating and cooling energy) through solar thermal cycles. As a Perspective, it provides necessary background for the general reader, but also seeks to emphasise the specific challenges and opportunities for the interested specialist.
BOX 1 1 Introduction
The global solar resource is immense with an estimated average of 173,000 TW of instantaneous power continuously reaching the earth’s surface – that represents 10,000 times the peak energy consumption of the current human population. Of course, the sun’s energy is central to life on earth, and the human species has been actively harvesting the solar resource throughout its entire existence – to heat, light and grow food. However, the urgent imperatives of human-driven climate change require a completely different scale of thinking and innovation in the way that we utilize solar energy – for “what”, “how” and importantly “where”.
Solar insolation (a measure of the solar energy incident per unit area per unit time) varies dramatically by geography, time and season (Besharat et al., 2013; World Bank Group, 2023). This variability in some senses defines what we can usefully “do” with the energy at any particular location, and thus requires both site specific and more regional approaches to harvesting and utilising the sun as a source of clean energy. This of course equally applies to other renewables leading to the obvious conclusion that multi-vector approaches (such as hybrid energy systems with storage) are ultimately the most efficient way to increase generating capacity factor (see Box 1) plus address intermittency of supply versus demand [International Renewable Energy Agency (IRENA), 2022]. That said, the World Bank has estimated that 86% of the global population live in some 150 countries where the maximum and minimum seasonal variance of the solar resource is less than a factor of two, and where an optimised photovoltaic system (see §2) could produce an average daily output of 3.5 kWh/kWp equivalent to a capacity factor of 14.5% (World Bank Group, 2020 Management Assistance Program (ESMAP)). Solar energy can be used (and is increasingly so) in a diversity of ways – for heating and cooling, to generate local and utility electricity, to drive chemical and biochemical processes. This fact, coupled with the immensity of the global resource is driving huge investment and innovation in solar technology as a key driver of societal decarbonisation alongside other renewable energies such as wind and hydro (Nijsse et al., 2023). The innovation is not limited to science and engineering, and the last decade has seen substantial progress on policy to promote solar energy deployment (Tay and Konovessis, 2023), techno-economics (Nijsse et al., 2023), and an increasing realization that societal acceptance through – a just transition in which no one is left behind – away from fossil fuels, is absolutely central to global decarbonisation (IRENA, 2023).
It is within this dynamic, multi-disciplinary, exciting and technologically frontier landscape that this short Perspective is framed. It is part of a series of Perspectives on the expanding plethora of applications for solar energy as a critical contributor to societal decarbonization. This Perspective is focused on the big-ticket items: the generation of electricity at the utility-scale; and the more local challenges and opportunities of the built environment. It is primarily a technology and techno-economic Perspective, but with reference to the important social science challenges, especially in relation to the afore-mentioned just transition. The Perspective is not all-encompassing but does seek to highlight areas of significant opportunity particularly suited to the utilization of solar energy.
Capacity Factor (CF) is a measure of the utilization of an energy generating system relative to its thermodynamic and technological constraints. The US Energy Information Administration defines CF as: the ratio of the electrical energy produced by a generating unit for the period of time considered to the electrical energy that could have been produced at continuous full power operation during the same period. It is an important comparator metric between energy generating systems and reflects input resource availability particularly in relation to electrical power plants. In theory, fossil-fuel power plants could have a CF of 100% (as does nuclear fission) but in reality are operated at ~80% for commercial reasons. Solar power plants without storage are typically in the range 10–30%, likewise wind 10–40% (Bolsona et al., 2022).
2 Solar energy for utility-scale stationary electrical power
The two dominant technologies for generating electricity from solar energy are solar photovoltaics (PV) and the lesser known concentrating solar thermal (CST). PV is a direct method of generation whereby solar photons create electron–hole pairs (i.e., photocurrent) and an associated photovoltage across a semiconductor junction. The so-called photovoltaic effect was demonstrated in 1839 by Edmond Becquerel with the first practical “solar cells” developed using silicon at the Bell Labs in the 1950s. Solar PV panels consist of individual cells (Figure 1A) in strings engineered for efficiency and particular output power profiles. Panels can be likewise connected to create “strings” suitable for deployment at small scale (for example a 10 kW system on a commercial residence), at commercial-industrial-scale (100 s of kWs – Figure 1B), or indeed utility-scale (> 10s of MW). The output from a solar cell, panel or string is Direct Current (DC) and must be converted to AC using an inverter. There are now numerous types of solar PV – silicon still dominates with >95% of the global market, but chalcogenide (CdTe in particular) technology has a growing presence. The performance of a PV system is defined by the power conversion efficiency (PCE) of the solar panel itself (the efficiency at which solar photon power is converted to electrical DC power) multiplied by the AC conversion efficiency. A typical silicon PV system would have an overall efficiency of 20–22% (CdTe slightly lower), with the highest efficiencies delivered by multi-junction III-V semiconductor solar cells at >40% (National Renewable Energy Laboratory (NREL), 2023)—the latter only used in very selected applications such as space solar power due to their very high cost and technological complexity, although this opportunity is rapidly emerging as the cube-sat revolution takes-off. For perspective, although a module PCE of ~20% may seem modest, it is really a remarkable technical achievement given the relatively inefficient photovoltaic process and very simple architecture of a commercial solar cell.
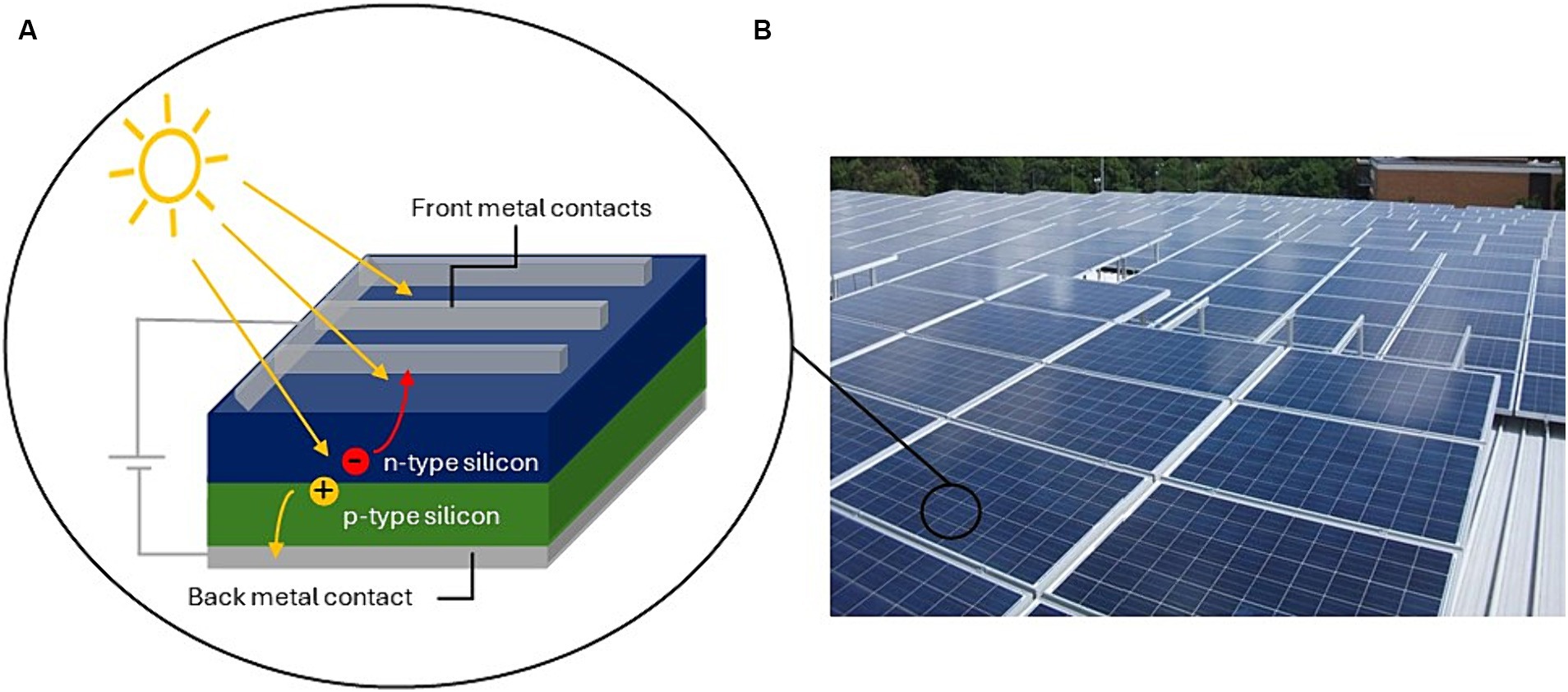
Figure 1. Solar photovoltaic cells and modules (A) schematic of a silicon solar cell showing basic construction and the photovoltaic effect. (B) a roof top array of silicon solar modules [University of Queensland, Australia ©UQ-Solar (Yan et al., 2016)].
Solar PV is now a mature, commoditized technology and systems last in excess of 20 years. These factors combine to make PV the second cheapest form of renewables with a global average levelised cost of energy (LCOE) of US$0.049 (2022 figures) behind on-shore wind (US$0.033) (IRENA, 2023). The solar PV LCOE has reduced by ~ x10 since 2010 – the largest decrease of any form of electrical generation and in 2022 it was 29% cheaper than the average fossil fuel electricity cost. These truly remarkable cost reduction metrics have been driven by rapid advances (mainly in China and mainly in silicon PV) in advanced robotic manufacturing. In 2022 the cumulative installed solar PV global capacity topped 1TW (IRENA, 2023) of which 40% is distributed (building integrated or applied [BIPV, BAPV], or commercial-industrial) with the balance being utility-scale (International Energy Agency, 2022). It’s important to note that efficiency improvements at the module, inverter and balance of systems levels (framing, installation, wiring, etc.) have also contributed to per kW cost and LCOE reductions. These efficiency improvements have also driven increased capacity factors (Box 1) from 13.8% in 2010 to 16.9% in 2022 at utility-scale. For perspective, the module plus inverter (i.e., the critical components of a PV system), now represent only 37% (utility-scale average) of the per kW installed cost with the remainder being BOS (IRENA, 2023). Capacity Factors can be also increased by a few percent by “tracking” the PV panels (i.e., following the sun over the course of a day either in a single or dual axis – single axis becoming common-place at the utility-scale) or with system-level combination of solar PV with battery storage. This system-level strategy is increasingly attractive at all scales as battery technology improves and costs decrease, although it is still by no means ubiquitous due to the considerable technical challenges of smart-grid integration and control (Bolsona et al., 2022; Nijsse et al., 2023). What is very clear though, is that solar PV development in the last decade is an incredible story of technical, techno-economic and policy innovation – efforts which continue at pace to both drive down LCOE and increase PCE. There are a small number of promising areas in this regard, notably: the development of silicon-perovskite tandem PV (OxfordPV, 2023) with PCEs >30%; cell-and-module-level architectural innovations such as the TOPcon (tunnel oxide passivated contact) and IBC interdigitated back contact silicon technologies (IRENA, 2023); accelerated deployment of so-called bifacial crystalline silicon modules at utility-scale (IRENA, 2023); and the development of more efficient power electronics (for inversion) based upon wide band gap semiconductors such as SiC and GaN (Alatise et al., 2023).
CST is a non-direct method of electricity generation whereby solar energy is concentrated using optical elements (usually mirrors although lenses were used especially in early systems) onto a receiver which contains a working fluid. The working fluid is heated (often superheated under pressure) to several hundred °C and via a suitable heat exchange element used to create pressurized super-heated steam to drive a conventional turbine and thus create AC electricity. There are numerous concentrating mirror–receiver architectures: mirror dish central receiver; tower heliostat mirror field; and trough mirror or Fresnel reflector trough receiver pipe (Alami et al., 2023). The origins of CST can be traced back to 1866 when Auguste Mouchout drove the first solar steam engine with a parabolic trough. The first CST plant was built near Genoa by Giovanni Francia in 1968 – it consisted of a central receiver atop a tower at the focus of a field of tracking heliostatic mirrors and could produce 1 MW of power with superheated steam (100 bar, 500°C). The oil crisis of the 1970s and 80s saw an acceleration of utility-scale CST especially in the USA: for example, the 10 MW Solar One in California in 1981 followed by Solar Two in 1995 which utilized molten salts both as a working fluid and thermal storage medium for the first time. The largest CST plant in the world is the Ivanpah Solar Energy Generating System (on the California-Nevada border) completed in 2014 and capable of generating 392 MW (Figure 2). It is an astonishing piece of engineering.
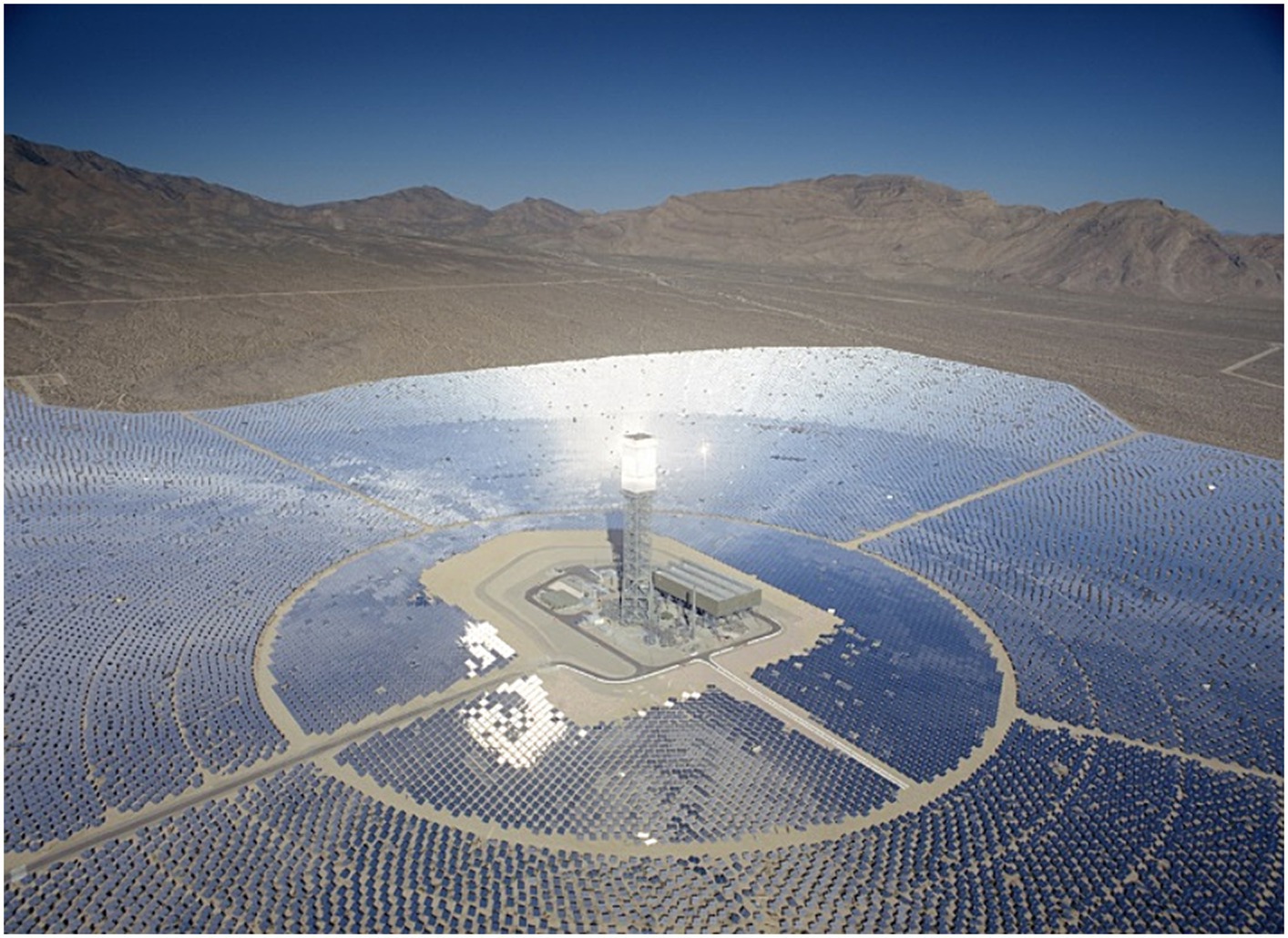
Figure 2. One of the three Ivanpah “power towers” (140 m tall) receiver systems capable of generating in total 392 MW and with a staggering 173,500 tracking heliostats. Ivanpah cost US$2.1bn and was enabled by a $1.6bn US-Government backed loan guarantee. It remains one of the most ambitious renewable energy projects ever conceived, although has not been replicated (©American Society of Civil Engineers).
The global average LCOE of utility-scale CST electricity was US$0.118 in 2022 down by a factor of three relative to 2010 (not quite the trajectory of PV) but only on a total installed capacity of only 7.5GW. These are relatively modest figures when compared with solar PV. It is somewhat surprising, given the obvious advantages of CST for utility-scale electrical power, that there has not been wider adoption especially in desert regions of high insolation. These advantages include: (i) CFs of >40% can be achieved and a recent study indicated that 17.5 h of thermal storage could yield fossil fuel plant-like CFs of >80% i.e., baseload power, in a power-tower molten salt architecture (Gasa et al., 2022); (ii) CST uses conventional turbine technology and this means it can be used as a spinning reserve connected directly to the grid, and be hybridized with conventional fossil fuel plant; and (iii) the means to increase CF in CST is via thermal storage – heat energy being considerably simpler and cheaper to store than electrical energy (c.f. batteries required for solar PV). Maybe the answer to this limited deployment lies in the fact that CST only makes commercial and technical sense at the utility-scale – the efficiency and cost of all major systems components (concentrators, receivers, storage elements, turbines) do not scale down. PV does have an economy of scale, but it is much less pronounced than CST. That said, CST must play an increasing role in grid-connected electricity generation – both as a pseudo base-load source, and as a stabilizing spinning reserve to compensate for intermittency of other renewables. There is also considerable room for improvements at virtually every level of a CST system – materials with increased solar absorption and heat conversion, innovative approaches to storage media, new working fluids with higher specific heat capacities such as supercritical CO2, AI and machine learning solar tracking, scalable turbines, etc. CST hybridization is another area of significant opportunity – either to reduce emissions from fossil fuel plants, or in combinatorial approaches using biofuel or modular nuclear reactors.
3 Solar energy in the built environment
The scalable nature of PV described in §2 means that it can be integrated into the built environment (designed-in or as an add-on) without major economy of scale negatives. Building-integrated (or building applied) PV (BIPV, BAPV on roofs, facades, etc.) is common-place even in locations with modest solar capacity factors such as Northern Europe (CF ~ 10% in the UK for example). The electrical power generated is invariably used locally within a single building or precinct – indeed, many electrical network operators prohibit the export of locally generated power to safeguard against system instabilities or contractual supply issues. Network disruption caused by increased intermittent generation, both at local and grid-scale, remains arguably the most significant challenge to accelerated deployment of PV. Ensuring PV installations do not lead to reduced electrical supply stability, and increased mismatch of peak supply and demand often requires detailed network planning and costly network augmentation. Connecting commercial-industrial PV installation in the built environment (particularly in historically mature networks such as those across Europe) can take several years and can even take in excess of 12 months for a simple household system.
To overcome some of these network issues, local integration of PV with batteries, combined with deployment of micro-grid controllers or distributed energy resource management systems to interface with existing building energy management systems is an attractive proposition for commercial or industrial sites. In addition, the idea of measuring, monitoring and optimizing electrical power flows (and plant / building operations) with AI or machine learning control systems is emerging within the context of “Smart Buildings” – the underlying rational being to understand emissions intensity of a facility, and to automatically optimise its operation to reduce overall energy consumption (Vahidinasab et al., 2021; Alanne and Sierla, 2022). PV plus batteries (or indeed other storage vectors such as heating and cooling) allows for “load shifting”, i.e., the production of solar electricity during the day for use at night, or indeed the charging of batteries at night (on lower tariffs) and use during the day to augment the solar. These are still frontier concepts, expensive to implement and with limited providers and supply chains but will become standard practice in the next decade.
The predominant form of BIPV is silicon-based – the same technology that dominates at utility-scale, and for the same reasons: cost, reliability, performance, lifetime. However, the built environment has many added dimensions compared to a solar plant in the desert – aesthetics, human comfort, urban design, as well as impacts on building fabric integrity (Sailor et al., 2021). This is opening new opportunities for both modified forms of silicon-PV (semi-transparent window integrated for example), and alternative, less developed technology such as lightweight, flexible form factor “plastic” PV based upon organic semiconductors which can be bandgap engineered for different colors and truly integrated into the building or structure fabric (Li et al., 2023). There is also a growing realization that the Internet of Things, whilst delivering ubiquitous connectivity will challenge building energy infrastructure. What will power the billions of sensors deployed across every building by the late 2020s? Burwell (Burwell et al., 2022) has suggested that modified solar PV can be bandgap engineered for indoor light harvesting and when combined with super capacitors or batteries provide non-hard-wired local power in the μW-to-mW regime – which is enough to drive most sensor systems. Indoor lighting conditions are highly variable with mixed artificial and natural sources (Seunarine et al., 2023). Recognizing this, Kay et al. (2023) have developed predictive simulation tools to help design PV for different indoor environments. They have determined that PCEs >40% are theoretically possible with current light emitting diode (LED) artificial lighting and suggest the optimum indoor light harvesting semiconductor should have a bandgap of between 1.7–1.9 eV (as opposed to silicon at 1.1 eV or CdTe at 1.44 eV). The potential opportunity for indoor PV has recently been recognized by the international standards bodies. With learnings from the well-established AM1.5 solar PV standards, a new IEC standard for measuring indoor PV has just been published (IEC TS 62607–7-2). This is a crucial step for technology development and benchmarking, and hence the creation of a commercial product.
Outside of individual buildings, the concept of solar technology integrated “street-scapes” is emerging in frontier Solar Urban Planning (Akrofi and Okitasari, 2022). This is a socio-technical endeavour with considerations (for example) as to how solar facades enhance the street-scape aesthetics, solar-driven electric vehicle charging infrastructure can be integrated into roads and pavements, precincts can be laid out with commercial-industrial scale solar PV, storage, heating and cooling (see below). Again, the concept of the just transition is a central theme since one could easily see the widening of socio-economic-demographic gaps if investment flows to “high-end solar suburbs” rather than disadvantaged dense neighborhoods with poor and worsening infrastructure.
Whilst the factors that favour CST for utility-scale electricity production were outlined in §2, that’s not to say that solar thermal as a concept is not valuable within the built environment at much smaller scale. Thermal energy consumption of the built environment (i.e., heating and cooling) has been estimated to consume up to 40% of worldwide energy. This is a staggering figure and set to increase – the IEA predicting that 2/3 of the world’s buildings will have air conditioning by 2050 with China, India and Indonesia accounting for half (International Energy Agency, 2018). Local solar PV can be used to drive conventional electrical compressor air conditioning systems, but one should not overlook the potential of solar thermal systems with low concentration (<100) to drive heating and cooling cycles, or to boost the Coefficient of Performance (COP) of a hybrid heat pump. In a recent review on solar cooling and air-conditioning systems (SCACSs) by Al-Yasiri et al. (2022) absorption, adsorption and desiccant-based technologies are compared and contrasted. What emerges from this and other surveys of solar thermal driven heating and cooling is the clear opportunity and relatively nascent status of the technology: only a few thousand systems at any scale are deployed worldwide; and most with very modest COPs in the range 0.5–1.5. This application of solar energy is arguably the most untapped and highest priority in the built environment – requiring a PV-like innovation explosion as noted in §2.
4 Discussion and conclusion
In this short Perspective we have looked at the current status of, and emerging opportunities in, two “big ticket” areas for the deployment of solar energy: utility scale electricity, and the more local considerations of the built environment. Solar PV and solar thermal are the two dominant families for both electrical and thermal energy generation – in both cases we have proven, reliable technology. Whist global solar energy deployment has been impressive and accelerated past the 1TW benchmark in 2023 (PV alone), this has to be viewed in the context of the estimated 50TW needed by 2050 (Haegel et al., 2019, 2023). That is a completely different scale of challenge requiring a step-change in urgency. If there were ever any doubt, the need for rationale systems thinking is now also a high priority, as is the need for a holistic understanding of priorities in hard to decarbonize sectors of society and the global economy such as the large emissions industries and transport. This holistic thinking must include (for example) strategies such as:
– Global approaches to generating and using solar energy where the necessary resources are abundant (including feedstocks), e.g., semiconductor component manufacturing where there is plentiful solar energy, water and raw materials, large-scale manufacturing of solar system components (necessarily outside of China or where the solar plant is to be deployed), and even “data centers in the desert”;
– Long distance DC transport of utility solar electricity, e.g., North Africa to Europe, North Australia to the Indonesian Archipelago, the Chilean Andes to South American population centers;
– The re-prioritization of both utility-scale and local solar thermal technology for electricity generation and grid support, plus local heating and cooling;
– Consideration of more esoteric concepts such as solar-driven factories in space serviced by cube sat payloads, or a wider deployment of “float-voltaics” (PV on bodies of water), or “agri-voltaics” (the combined use of agricultural land for food and solar energy production and / or the powering of agriculture with local solar electricity) to increase the real estate available for PV.
These are definitely short-to-medium term strategies as we accelerate the now mission critical transition to net zero. However, a sustainable longer-term will require a complete re-imaging of our electricity network and grid philosophy and major global societal re-balance. For example, a new global framework for solar system component disposal and recycling (of the sort adopted in Europe for CdTe thin film technology) is desperately needed, as is the full implementation of circular economy principles in the design and manufacture of (in particular) PV modules and inverters as we accelerate towards multi-TW supply chains. A just transition also means more globally distributed manufacturing, sovereign energy security and independence for all countries, and a breaking of the exploitation of labor and critical raw materials from developing nations. These are all massive challenges, but also opportunities for economic growth, and a healthier, cleaner, happier human global society.
Data availability statement
The original contributions presented in the study are included in the article/supplementary material, further inquiries can be directed to the corresponding author.
Author contributions
PM: Writing – original draft.
Funding
The author(s) declare financial support was received for the research, authorship, and/or publication of this article. This work was supported by the Welsh Government’s Sêr Cymru II Programme (European Regional Development Fund, Welsh European Funding Office, and Swansea University Strategic Initiative in Sustainable Advanced Materials). The Centre for Integrative Semiconductor Materials (CISM) is a UKRI Research Partnership Investment Fund project. The work was also supported through the CSconnected Strength in Places Fund programme (UKRI and Industrial Strategy Challenge Fund) and the Engineering and Physical Sciences Research Council (EPSRC-UKRI) through its Programme Grant Application Targeted Integrated Photovoltaics (EP/T028513/1).
Acknowledgments
PM would like to thank Dr. Ardalan Armin and Dr. Greg Burwell of Swansea University, and Professor Tapan Saha at the University of Queensland for fruitful discussions.
Conflict of interest
The author declares that the research was conducted in the absence of any commercial or financial relationships that could be construed as a potential conflict of interest.
Publisher’s note
All claims expressed in this article are solely those of the authors and do not necessarily represent those of their affiliated organizations, or those of the publisher, the editors and the reviewers. Any product that may be evaluated in this article, or claim that may be made by its manufacturer, is not guaranteed or endorsed by the publisher.
References
Akrofi, M. M., and Okitasari, M. (2022). Integrating solar energy considerations into urban planning for low carbon cities: a systematic review of the state-of-the-art. Urban Govern. 2, 157–172. doi: 10.1016/j.ugj.2022.04.002
Alami, A. H., Olabi, A. G., Mdallal, A., Rezk, A., Radwan, A., Rahman, S. M. A., et al. (2023). Concentrating solar power (CSP) technologies: status and analysis. Int. J. Thermofl. 18:100340. doi: 10.1016/j.ijft.2023.100340
Alanne, K., and Sierla, S. (2022). An overview of machine learning applications for smart buildings. Sustain. Cities Soc. 76:103445. doi: 10.1016/j.scs.2021.103445
Alatise, O., Deb, A., Bashar, E., Gonzalez, J. O., Jahdi, S., and Issa, W. (2023). A review of power electronic devices for heavy goods vehicles electrification: performance and reliability. Energies 16:4380. doi: 10.3390/en16114380
Al-Yasiri, Q., Szabó, M., and Arıcı, M. (2022). A review on solar-powered cooling and air-conditioning systems for building applications. Energy Rep. 8, 2888–2907. doi: 10.1016/j.egyr.2022.01.172
Besharat, F., Dehghan Ahmad, A. A., and Faghih, R. (2013). Empirical models for estimating global solar radiation: a review and case study. Renew. Sust. Energ. Rev. 21, 798–821. doi: 10.1016/j.rser.2012.12.043
Bolsona, N., Prieto, P., and Patzek, T. (2022). Capacity factors for electrical power generation from renewable and nonrenewable sources. Proc. Nat. Acad. Sci. 119:e2205429119. doi: 10.1073/pnas.2205429119
Burwell, G., Sandberg, O. J., Li, W., Meredith, P., Carnie, M. J., and Armin, A. (2022). Scaling considerations for organic photovoltaics for indoor applications. Solar RRL 7:2200315. doi: 10.1002/solr.202200315
Gasa, G., Prieto, C., Lopez-Roman, A., and Cabeza, L. F. (2022). Life cycle assessment (LCA) of a concentrating solar power (CSP) plant in tower configuration with different storage capacity in molten salts. J. Energy Storage 53:105219. doi: 10.1016/j.est.2022.105219
Haegel, N. M., Atwater, H. Jr., Barnes, T., Breyer, C., Burrell, A., Chiang, Y. M., et al. (2019). Photovoltaics at multi-terawatt scale: waiting is not an option. Science 364, 836–838. doi: 10.1126/science.aaw1845
Haegel, N. M., Verlinden, P., Victoria, M., Altermatt, P., Atwater, H., Barnes, T., et al. (2023). Terawatt-scale photovoltaics: transform global energy. Science 380, 39–42. doi: 10.1126/science.adf6957
International Energy Agency. (2018). The future of cooling. Available at: https://www.iea.org/reports/the-future-of-cooling
International Energy Agency. (2022). Technology and innovation pathways for zero-carbon-ready buildings by 2030. Available at: https://www.iea.org/reports/approximately-100-million-households-rely-on-rooftop-solar-pv-by-2030
International Renewable Energy Agency (IRENA). (2022). Available at: https://www.irena.org/Publications/2023/Aug/Renewable-Power-Generation-Costs-in-2022
IRENA. (2023). Finding Common Ground for a Just Energy Transition: Labour and Employers Perspectives. Available at: https://www.irena.org/Publications/2023/Aug/Finding-common-ground-for-a-just-energy-transition-Labour-and-employer-perspectives
Kay, A. M., Fitzsimons, M., Burwell, G., Meredith, P., Armin, A., and Sandberg, O. J. (2023). The thermodynamic limit of indoor photovoltaics based on energetically disordered semiconductors. Solar RRL 7:2300277. doi: 10.1002/solr.202300277
Li, Y., Huang, X., Hafiz, K., Sheriff, M. Jr., and Forrest, S. R. (2023). Semitransparent organic photovoltaics for building-integrated photovoltaic applications. Nat. Rev. Mat. 8, 86–201.
Management Assistance Program (ESMAP) Washington, D.C. Available at: http://documents.worldbank.org/curated/en/466331592817725242/Global-Photovoltaic-Power-Potential-by-Country
National Renewable Energy Laboratory (NREL). (2023). Photovoltaic Cell Efficiency Tables. https://www.nrel.gov/pv/assets/pdfs/best-research-cell-efficiencies.pdf
Nijsse, F. J. M. M., Mercure, J. F., Ameli, N., Larosa, F., Kothari, S., Rickman, J., et al. (2023). The momentum of the solar energy transition. Nat. Commun. 14:6542. doi: 10.1038/s41467-023-41971-7
OxfordPV. (2023). The perovskite-on-silicon tandem solar cell. Available at: https://www.oxfordpv.com/perovskite-silicon-tandem-cell
Sailor, D. J., Anand, J., and King, R. R. (2021). Photovoltaics in the built environment: a critical review. Energ. Buildings 253:111479. doi: 10.1016/j.enbuild.2021.111479
Seunarine, K., Haymoor, Z., Spence, M. A., Burwell, G., Kay, A. M., Meredith, P., et al. (2023). Light power resource variability for energy harvesting photovoltaics for self-powered IoT. J. Phys. Energy. 6:01508. doi: 10.1088/2515-7655/ad1764 (In Press).
Tay, Z. Y., and Konovessis, D. (2023). Sustainable energy propulsion system for sea transport to achieve United Nations sustainable development goals: a review. Discov. Sustain. 4:20. doi: 10.1007/s43621-023-00132-y
Vahidinasab, V., Chenour, A., Mohammadi-Ivatloo, B., Giaouris, D., and Walker, S. L. (2021). Active building as an energy system: concept, challenges, and outlook. IEEE Access 9, 58009–58024. doi: 10.1109/ACCESS.2021.3073087
World Bank Group (2020). Global photovoltaic power potential by country (English) Washington D.C., USA: Energy Sector.
World Bank Group. (2023). Global Solar Atlas: Available at: https://globalsolaratlas.info/map
Keywords: solar photovoltaics (PV), solar thermal energy, utility scale and built environment, indoor PV, just transition to net zero
Citation: Meredith P (2024) Solar energy for societal decarbonisation: a perspective on emerging opportunities in utility-scale solar electricity and solar in the built environment. Front. Sustain. Cities. 6:1344771. doi: 10.3389/frsc.2024.1344771
Edited by:
Sebastian Bonilla, University of Oxford, United KingdomReviewed by:
Robert L. Z. Hoye, University of Oxford, United KingdomTasmiat Rahman, University of Southampton, United Kingdom
Nicholas Grant, University of Warwick, United Kingdom
Copyright © 2024 Meredith. This is an open-access article distributed under the terms of the Creative Commons Attribution License (CC BY). The use, distribution or reproduction in other forums is permitted, provided the original author(s) and the copyright owner(s) are credited and that the original publication in this journal is cited, in accordance with accepted academic practice. No use, distribution or reproduction is permitted which does not comply with these terms.
*Correspondence: Paul Meredith, paul.meredith@swansea.ac.uk