Microbiome as a Key Player in Sustainable Agriculture and Human Health
- 1Department of Soil Science and Agricultural Chemistry, Banaras Hindu University, Varanasi, India
- 2Department of Plant Pathology, Professor Jayashankar Telangana State Agricultural University, Hyderabad, India
The aggregate of microorganisms in the soil environment is a microbiome that emerged as a vital component of sustainable agriculture in the recent past. These beneficial microorganisms perform multiple plant growth-promoting activities including fixation, mineralization, solubilization, and mobilization of nutrients, production of siderophores, antagonistic substances, antibiotics, and release of plant growth-promoting substances, such as auxin and gibberellin hormones, mediated by interactions between host plant roots and microbes in the rhizosphere. Numerous plant species forms symbiotic association with microbes and draw the benefit of mineral nutrient supply with the expense of minimal energy, and their distribution is governed by nature and the number of root exudates, crop species, and cultivars. On the other hand, microorganisms with critical roles in the microbiome can be isolated, formulated, and developed as a new biological product called biofertilizers. Agriculturally, important microbes with Fe- and Zn-solubilizing attributes can be used for the biofortification of micronutrients in different cereal crops. Regardless of the approach to be used, innovations with the use of microbiomes represent the future of sustainable agriculture. Probiotic microbes, such as Lactobacillus, etc., are increasingly being used as dietary supplements in functional food products. Effective utilization of microbiome aids in promoting sustainable agriculture that accomplishes a safe environment, which in turn manifests positively on human health.
Introduction
The world population is expected to reach 9.7 billion in 2050, with a subsequent increase in demand for food and water. In this scenario, food production needs to increase by 70% by 2050 (1). Intensive cropping and exhaustive use of mineral fertilizers, agrochemicals, and water to meet global food demand led to land degradation, environmental pollution, and depletion of natural resources. Food production with minimal environmental impact and residual effect of chemicals on the food chain is the way toward sustainable agriculture. Plants' bodies can be considered a complex interplay of ecological niches that harbor in their rhizosphere, surface tissues (rhizoplane and phylloplane), and inner tissues (endosphere), a great diversity of microorganisms with which they establish a broad range of beneficial, neutral, and harmful interactions. The typical relationship of plants with their surrounding environment for a long time has been the topic of research in the scientific community. Many studies have been conducted to answer the complicated questions about their interaction, but in real life, plant–microbe interaction is much more complex. Harnessing the potential use of soil microbiota in agriculture is considered as a most fruitful and long-term solution to environmental pollution, food security, and human health (2).
The term microbiome was first coined by Lederberg and McCray (3) defined as the “Set of resident micro-organisms that inhabit a given host/environment.” In agriculture, microbes are used as biocontrol agents (for controlling pests and diseases), biostimulants (mobilizing locally available nutrients for plant uptake), and biofertilizers (4). These microorganisms are dynamic, as they have self-sustaining as well as self-replicating capabilities and do not need repeated inoculation. The efficiency and durability of microbial inoculants in promoting plant growth and toward soil resilience depend on the environment, type of plant, and microbial community (5). Plant microbiomes are agriculturally important bioresources for agriculture as beneficial microbes may enhance plant growth and improve plant nutrition uptake through solubilization of P, K, and Zn, nitrogen fixation, and other mechanisms including siderophore production [microbe-mediated biofortification of different crops (6)]. Beneficial microbes may increase crop yields, remove contaminants, inhibit pathogens, and produce fixed nitrogen or novel substances. The growth stimulation by plant microbiomes can be a consequence of biological nitrogen fixation; production of plant growth regulators, such as IAA, gibberellic acids, and cytokines; biocontrol of phytopathogens through the production of antibiotic, antifungal, or antibacterial agents; Fe-chelating compound production; nutrient competition; induction of acquired host resistance; and enhancing the bioavailability of minerals. The microbiota comprises all living members forming the microbiome. Microbiota is usually defined as the assemblage of living microorganisms present in a defined environment. Soil microbes help regulate our emotions and immune response, and they also play a key role in determining the nutrient content of our food. The hotspot for microbiota in the human body is the gut, and their composition is majorly affected by food and dietary intake (7). In general, the concentrations of micronutrients in different crops are not adequate for human nutrition in diets. Hence, consumption of such cereals and cereal products are staple food in most human diets in both developed and developing countries, providing a major proportion of dietary energy and nutrients, which may result in micronutrient malnutrition and related severe health complications (8). More recently, an understanding of the complex interactions between plant roots and microbial communities (plant growth-promoting rhizobacteria and plant growth-promoting fungi) in the rhizosphere has fueled research into the role and application of soil microbes in the biofortification (“Biofortification is the process of adding essential micronutrients and other health-promoting compounds to crops or foods to improve their nutritional value”) of crops. The biofortification approach is getting much attention to increase the availability of micronutrients, especially Se, Fe, and Zn in the major food crops (9, 10). Sundaria et al. (11) reported that it is possible to prime seeds before sowing by bombarding them with iron oxide nanoparticles. This method would trigger more iron acquisition in the wheat plants and, thus, increase the nutritive value of the grains. The beneficial microbes can also be used as probiotics as functional foods for human health. Probiotic microbes, such as Bifidobacterium, Lactobacillus, Methanobrevibacter, Methanosphaera, and Saccharomyces are increasingly being used as dietary supplements in functional food products. Microbes with beneficial properties could be utilized for sustainable agriculture and human health. Currently, there has been an increased curiosity in the development of new functional foods and their assimilation into a healthy diet. Such products, and especially probiotics, exert a beneficial effect on host-gut microbiota after consumption and may be proficient to prevent several diseases. Probiotics are defined as live microbiomes, which when administered in ample amounts confer a health benefit on the host. In this review, the importance of the way and impact of the microbiome and biotechnological intervention on sustainable agriculture following its manifestation on soil, and environmental and human health.
Sustainable Agriculture for Soil and Environmental Health
To meet the global population demand, countries follow intensive farming practices that enhance production but leave detrimental effects on soil and adverse impacts on environment, animal, and human health (12). The high dependence on modern agricultural practices and application of mineral fertilizers and agrochemicals hinders internal nutrient cycling in soil (13). Adoption of various mineral fertilizers and agrochemicals causing harm to beneficial insects, soil microbiome, and plants, generate contaminated runoff and pollute water systems, also leading to climate change in terms of fluctuating temperature and rainfall (14). Uneven distribution of rainfall caused severe soil erosion and degradation showing a severely detrimental effect on soil structure and productivity. The soil microbiome plays an important role in plant growth development and soil fertility for sustainable agriculture (4). The soil microbiome plays a role in nutrient cycling through organic matter decomposition and ecosystem functioning (nutrient recycling and resistance to biotic and abiotic stress) (15). In sustainable agriculture, diverse groups of the microbiome are used for nutrient acquisition through rhizospheric or endophytic, or phyllosphere interaction in symbiotic/free living mechanisms and plant protection through the use of biopesticide/biofungicide. Conservation and integrated use of natural resources, such as soil and water, which are the main sources of mineral nutrients are crucial for a safe environment, crop productivity, and human health. Sustainable agricultural practices conserve natural resources against deterioration of soil and water, minimize environmental pollution, and maintain soil health and biodiversity. Sustainable agriculture can be defined as an integration of plant and animal products, such as farmyard manure (FYM), crop residue, etc., that will meet human food and fiber demand through efficient use of on-farm and non-renewable resources that can enhance economic viability; the environment also improves the quality of the farmer's life as well as environmental quality (16). Sustainability can be achieved through proper waste management at the farm and using animal manure, so that adoption of off-farm resources, such as agrochemicals can be minimized. For example, crop rotation with a variety of crops, e.g., cereals–oilseed–pulse crop pattern (rice–soybean–green gram) based on climatic condition and source of water availability benefits the farmers, reduces production risk and uncertainty, and enhances soil ecological sustainability. Sustainable agriculture practices comprise practices, such as agroforestry, intercropping, crop rotation, green manuring, conservation tillage, cover crops, and adopting biofertilizers (17) (Figure 1).
Crop Rotation
Crop rotation is a critical feature of sustainable cropping systems because it provides numerous benefits to crop production, building healthy soils, a major way to control pests, and a variety of other benefits including nutrient cycling and decomposition of organic residue, etc. They can help conserve, maintain, or replenish soil resources, including organic matter, nitrogen, and other nutrient inputs, and physical and chemical properties. Crop rotation means changing the type of crop grown on a particular piece of land from year to year. It has an important influence on its microbial properties (18). Unsuitable land management can lead to a loss of soil fertility and a reduction in the abundance and diversity of soil microorganisms (19). The appropriate choice of crops within the rotation and their sequence is crucial if nutrient cycling within the field system is to be optimized and losses minimized over the short and long term. Each crop species has slightly different characteristics, e.g., N demanding or N2 fixing (20), shallow or deep rooting (21), as well as amount and quality of crop residue returned (22). Soil microbes modify soil structure by aggregating both mineral and organic constituents via the production of extracellular compounds with adhesive properties (17).
Veneklaas et al. (23) indicated that several legume crops, such as chickpea and white lupine, can mobilize soil and fertilizer P through the exudation of organic acid anions, such as citrate and malate and other compounds from their roots. Hocking and Randall (24) reported an improvement in growth and P nutrition of less P-efficient crops following organic-anion exuding legumes. Khadr et al. (25) indicated that application of organic manure to crops grown in 3-year rotation implemented in low soil fertility increases soil organic matter and increases available soil N, P, and K, compared with no manure application. Crop rotations can increase total soil carbon (C) and nitrogen (N) concentrations over time, which may further improve soil productivity. Kelley et al. (26) found that the replacement of dry season rice by maize in rice–rice rotation caused a reduction in soil C and N due to a 33–41% increase in the estimated amount of mineralized C and N during the dry season. As a result, there was 11–12% more C sequestration and 5–12% more N accumulation in soils continuously cropped with rice than in the maize–rice rotation with the greater amounts sequestered in N-fertilized treatments.
Intercropping
Intercropping involves cultivating two or more crops in a field simultaneously and is mainly practiced in regions where soils are relatively degraded. Intercropping is receiving increasing global interest as an agricultural practice as farmers strive to be more sustainable and maintain soil health (27). This intercropping system reduces land and water demand, and offers an inexpensive management strategy in environments projected to face water scarcity. In sustainable agriculture, intercropping plays a major role in the main economic yield balance to farmers and maintains the yield stability of the crops. Intercropping practices have a complementary effect for plants used regarding resource use, which effectively utilizes solar radiation, water, and nutrient elements compared with pure cropping (28).
Therefore, the positive effects of intercropping include the maintenance of soil water and utilization of the available environmental resources; it is also facilitated through the activity of microorganisms and nitrogen fixation (29). Therefore, intercropping can lead to improvements in the physiological and biochemical characteristics of the plant rhizosphere, which in turn increases productivity. The beneficial effects of intercropping can also encourage the proliferation of natural enemies, reduce disease and insect injury, and inhibit weed growth all of which undoubtedly lead to positive effects for the final products of the intercropping unit area under sustainable agriculture. Multiple benefits can be obtained from the application of different intercropping systems, which can improve soil conditions and the environment in which the plant grows and, thus, increase crop productivity.
Conservation Tillage
Using plow and maintaining 30% crop residue on the soil surface after harvest is called conventional tillage practice (CT). In sustainable agricultural production systems, conservation tillage practices have been widely used to alleviate the negative effects of conventional cropland management practices. Typical conservation tillage practices include no-tillage (NT) or reduced-tillage (RT) methods (30) and crop residue retention. Conservation tillage practices alter the spatial distribution of soil microbial populations and soil organic matter in the soil profile (31). No-tillage practices lead to higher C and N concentrations and water content in the surface soil, resulting in increased enzyme activity levels and microbial resource use efficiencies relative to conventional tillage (CT) practices (32, 33). Conservation tillage can also alter soil pH, thereby influencing soil microbial diversity and soil suitability for the growth of crops (34), promoting the formation of fungal hyphal networks, and leading to higher soil fungal population sizes (35). Additionally, microbial population size and diversity in agricultural soils can be affected by either tillage (36, 37) or crop residue retention (38) as well as the combined effects of tillage and crop residue retention (39).
Recent meta-analyses have quantified the effects of conservation tillage practices, including decreases in crop yield (30), increases in soil enzyme activity levels (33), increases in soil microbial biomass (31), and improvements in soil physical properties (40). Many conservation tillage systems can improve soil quality over time and reduce the soil erosion risk, improve soil properties, and reduce tillage costs. Adoption of conservation tillage can cause an increase in organic matter at the soil surface, relative to conventional tillage, as a result of maintaining a greater proportion of crop residue near the soil surface. This stratification with the depth of soil organic matter has important implications for soil quality and the environment because the soil surface receives much of the fertilizer and organic amendments and rainfall impact, and serves as an interface for gaseous exchange.
Green Manuring
Today's intensive agricultural practices, crop rotation, and green manuring (GM) offer technology to achieve sustainable production efficiently. Green manuring is the practice of incorporating undecomposed green plants into the soil to maintain the nutrient supply to the succeeding crop. Green manuring is also known as the process of incorporating green plants into the soil, which are raised in the same field or another field at the green stage before flowering (41). Since continuous and conventional cultivation leads to a decline in soil organic matter (SOM) content, soil nutrients holding capacity are affected severely, resulting in a loss of soil sustainability. One of the options to maintain sustainability in agriculture by restoring soil quality and reclaiming degraded soil is to increase soil OM content by green manure crops because this practice is eco-friendly, non-polluting, and non-hazardous to the soil, water, and air (42, 43). Green manure crops are mainly grown for the benefit of the soil and are very commonly referred to as soil fertility-building crops. Besides, green manure crops increase microbial growth and their dynamics in soil by releasing nutrients and energy materials as root exudates and eventually enhance soil fertility and soil health (44, 45). Additionally, microorganisms synthesize polysaccharide gums that bind the soil particles together to form soil aggregates and, thus, soil structure is maintained properly. Soil microbes decompose organic substances present in soil and become instrumental to transform unavailable forms of nutrients to their available form for crops. During the decomposition of plant residues in soil, the distribution and population dynamics of soil microorganisms are significantly influenced. Ghosh et al. (46) reported that the GM with dhaincha and sunn hemp supplemented with urea at different levels exhibited an increase in sugarcane yield up to 57% along with the significant increase in SOM, total N, available P and S in the soil. N loss from soil amended with GM is considered significantly low compared with chemical nitrogenous fertilizers. It has been observed that microbial population, growth, and diversity are significantly influenced by GM and soil admixture. This might be attributed to the fact that easily available energy and nutrient source through GM was delivered to the soil microbial community, which stimulate their activity and growth. More microbial proliferation leads to more extracellular enzymes, and their favorable abundance causes major transformations of nutrients from plant unavailable forms to their available forms.
Biofertilizers
Meeting the projected demand for healthy and sustainable food production is a crucial challenge. Increasing crop productivity by mitigating climate change and preserving agroecosystems is one of the significant goals of sustainable agriculture. Sustainable agriculture has been defined as an alternative integrated approach that could be used to solve fundamental and applied issues ecologically related to food production. It integrates biological, physical, chemical, and ecological principles to develop new practices that are not harmful to the environment. Successful application of microbes helps in maintaining soil health, improving water holding capacity, carbon storage, root growth, availability and cycling of essential nutrients, filtering pollutants, and also in conservation of biodiversity (47). Microbes in soil and overall soil health can be depleted by common agricultural practices; nevertheless, but this can be prevented by various ways of improving soil quality (48).
Integrated pest management (IPM), as one of the effective methods used in modern agriculture, takes into account all plant protection methods available in the application. IPM defines the management of pests by reducing pest numbers to acceptable levels, taking into consideration protecting the environment, non-target organisms, and human health (49). IPM implies the integration of appropriate measures that minimize the risks for human health and the environment by preventing the development of pest populations and by ensuring the use of plant protection products and other forms of intervention at economic and ecologically justified and reduced levels. In insect pest management, several plant products derived from neem, custard apple, tobacco, pyrethrum, etc., have been used as safer insecticides (50). Compounds, such as limonene, pyrethrum/pyrethrins, rotenone, sabadilla, and ryania, are widely used across the globe to control fleas, aphids, mites, ants, roaches, ticks, beetles, caterpillars and thrips, squash bugs, harlequin bugs, thrips, etc. (51, 52).
Agroforestry
Agroforestry is multiple land-use systems in which crops and woody perennials are grown on the same land management unit. Agroforestry system is practiced all over the world, and it has major importance in reducing the impact of climate change. Nowadays, climate change is the problem of developed and developing countries, thus, meeting to find out a solution to reduce the impact of climate change on agriculture, biodiversity, and food security. Thus, agroforestry is now receiving increasing attention as a sustainable land management option the world over because of its ecological, economic, and social attributes. Agroforestry, an ecologically and environmentally sustainable land use, offers great promise toward mitigating the_rising atmospheric CO2 levels through carbon sequestration. Synergies between climate change adaptation and mitigation actions are particularly likely in situations involving income diversification with tree and forest products. These options also reduce the susceptibility of land-use systems to extreme weather events, enhance soil fertility, and favor the conservation and restoration of forest and riparian corridors. Sequestration of carbon in soils and in the biota, along with payments to resource-poor farmers for the ecosystem services rendered, would be a timely win–win strategy in the fight against food insecurity and global warming.
The importance of agroforestry cannot be overemphasized, as it has several advantages in the provision of food and other basic needs (i.e., fuel wood, staking materials, fibers, timber, medicinal concentrates, oils, fruits, and fodder for animals) for a large proportion of the rural population as well as its role in soil fertility restoration and the control of weeds in addition to amelioration of environmental degradation. Advocates have contended that soil conservation is one of its primary benefits. The presence of woody perennials in agroforestry systems may affect several biophysical and biochemical processes that determine the health of the soil substrate (53). The less disputed of the effects of trees on soil include amelioration of erosion, primarily through surface litter cover and understory vegetation; maintenance or increase in organic matter and diversity, through continuous degeneration of roots and decomposition of litter; nitrogen fixation; enhancement of physical properties, such as soil structure, porosity, and moisture retention through the extensive root system and canopy cover; and enhanced efficiency of nutrient use because the tree–root system can intercept, absorb and recycle nutrients in the soil that would otherwise be lost through leaching (54).
Soil Beneficial Microorganisms as a Link to Sustainable Agriculture
Soil is a natural medium for plant growth and development. Soil is the most diverse and complex habitat that consists of the smallest organisms in the soil and includes bacteria, actinomycetes, fungi, algae, etc. The plant microbiome is a key determinant of plant health and productivity (55) and has received substantial attention in recent years (56, 57). Microbes in the rhizosphere can establish beneficial, neutral, or detrimental associations of varying intimacies with their host plants (Figure 2). Specific interactions between microbes and model plants, such as in Rhizobium–legume symbioses (41), are well understood. A significant amount, 5–20%, of the products of photosynthesis (the photosynthate) is released, mainly into the rhizosphere (the soil–root interface) through roots (12). These photosynthates include rhizodeposit exudates, mucilage, and sloughed cells. Root exudates contain a variety of compounds, predominately organic acids and sugars, and also amino acids, fatty acids, vitamins, growth factors, hormones, and antimicrobial compounds (58). Root exudates are key determinants of rhizosphere microbiome structure (59–62). The composition of root exudates can vary between plant species and cultivars (63, 64) and with plant age and developmental stage (65–67).
Among the various advantages of the microbiome for plants, symbiotic associations between nitrogen-fixing bacteria, mainly rhizobia, arbuscular mycorrhizal fungi, and phosphate-solubilizing bacteria, are typical examples of the microbiome for how plants obtain nitrogen and phosphorus, respectively (53). The interaction between nitrogen-fixing bacteria and legume crops, and arbuscular mycorrhizal fungi and phosphate-solubilizing bacteria increases the availability of nitrogen and phosphorus for plants since the bacteria fix the nitrogen and solubilize phosphorus ions, while the fungi translocate them to the plant (54). Bacillus, Azotobacter, Microbacterium, Erwinia, Beijerinckia, Enterobacter, Flavobacterium, Pseudomonas, and Rhizobium bacteria are known as phosphate solubilizers (54). The microbiome containing rhizobia, the legume nodule microbiome, consists of other endophytic bacteria, both of which are responsible for direct and indirect growth promotion mechanisms in plants (55). Some nitrogen-fixing endophytes are cyanobacteria (Anabaena, Nostoc, Calothrix), Azotobacter, Azospirillum, and Gluconacetobacter (54). However, fixators can also be free living and establish themselves in non-leguminous plants, as is the case, for example, with the genera Beijerinckia, Klebsiella, and Bacillus (54).
Another method is phytostimulation or biostimulation intrinsically linked to plant growth and made up of the production of phytohormones by the microbiome (52), such as indole-3-acetic acid (IAA), auxin, gibberellins, cytokinins, and salicylic acid (SA) (56). Besides, some bacteria can secrete an enzyme, 1-carboxylic acid-1-aminocyclopropane (ACC) deaminase, which reduces the level of ethylene in the plant (57, 68). Aguiar-Pulido et al. (58) reported that bacteria from the tomato rhizospheric microbiome produced the hormone IAA, and promoted plant growth. Duran et al. (59) found, in the roots of cereals (wheat) and oilseed (soybeans) crops, rhizospheric bacteria producing IAA and ACC deaminase, which were Pseudomonas spp. The microbiome also plays an essential role in plant tolerance to extreme conditions, such as salinity, drought, and exposure to heavy metals (60). Soil salinity has hindered the growth rates of plants and reduced their yield. However, the negative impact of high levels of salt in the soil can be minimized through the production of phytohormones by the microbiome, with a consequent increase in plant resistance to these extreme environments (60). In a study in Ref. (61), the rhizosphere microbiome was able to promote germination and growth of Hibiscus hamabo under salinity conditions. Recently, in Refs. (62, 63) a model was proposed to explain the establishment and maintenance of the beneficial and degrading microbiome in the rhizosphere of contaminated soil plants. Four strategies were identified, including plant selection based on the microbiome, interference from root exudates, disturbance, and feeding of supply lines, to ensure that the microbial community is kept under control in polluted environments. Plants that live in oil-contaminated soils depend on their microbiome for survival, growth optimization, and biomass production (64). At the same time, as the contamination of these areas increases, there are changes in the composition of the microbiome, favoring hydrocarbon-degrading microorganisms associated with plant growth (59). The plant–microbiome interaction will not always be efficient for phytoremediation; therefore, human interventions are necessary to optimize this interaction and promote the degradation of pollutants (63). Understanding the potential of the microbiome for agriculture can lead to its use as an inoculant or its manipulation, to select more efficient microbial groups for plant development (66). Besides that, reducing the use of pesticides and chemical fertilizers based on an understanding of the potential of the plant microbiome is of paramount importance in advancing sustainable agricultural practices (Table 1).
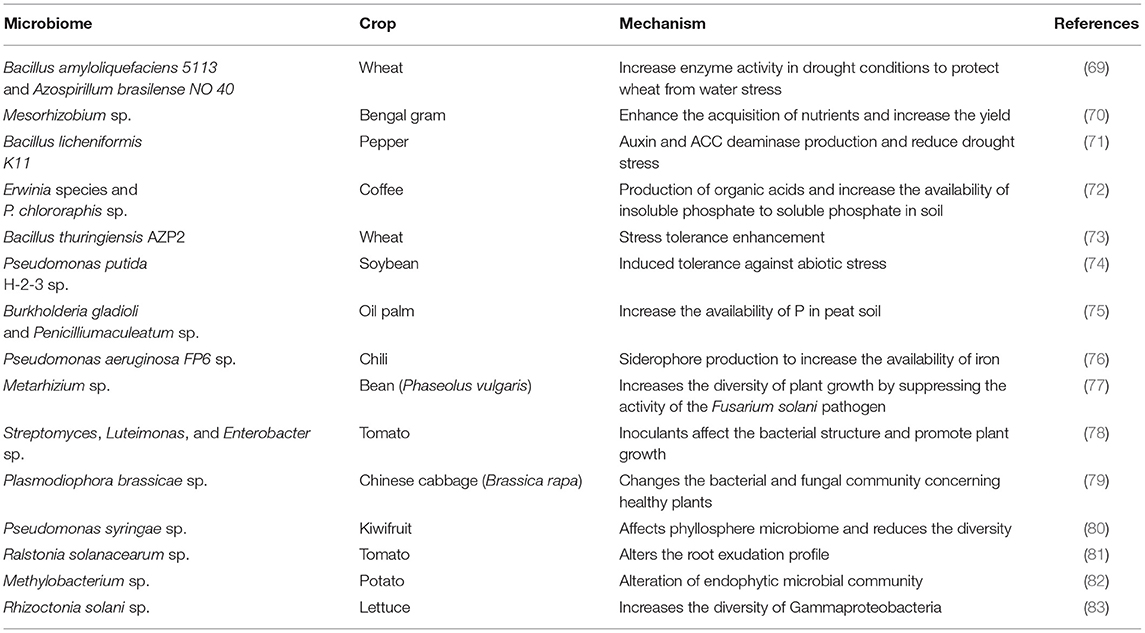
Table 1. Essential contributions of soil microorganisms toward sustainable agriculture in different crops.
The microbiome can also be used as a biocontrol agent. There are a few examples of biocontrol by the plant-associated microbiota (84) through the production of siderophores, volatile compounds, enzymes, and antibiotics, in addition to phytohormone levels being modulated (85, 86). The plant microbiome also inhibits the growth and activity of pathogens through competition for nutrients and microenvironments through parasitism, antibiosis (17), and the resistance they confer to the plants' immune system (87). Some soil bacteria are capable of protecting the plant from pathogens belonging to the genera Pseudomonas, Streptomyces, Bacillus, Paenibacillus, Enterobacter, Pantoea, Burkholderia, and Paraburkholderia (88, 89). In the soil microbiome, the phyla Acidobacteria, Actinobacteria, and Firmicutes were able to control the wilt caused by Fusarium oxysporum (90), while the endophytes Serratia and Enterobacter were able to control the pathogen Gaeumannomyces graminis, which caused take-all of wheat (91). Soil microbiome promotes plant growth through the biocontrol mechanism. The microbiome stimulates growth through bio-fertilization, that is, by controlling the availability and acquisition of nutrients by plants (92). Manipulation of the plant microbiome has the potential to reduce the incidence of plant disease (93), increase agricultural production (94), reduce chemical inputs (95), and reduce emissions of greenhouse gases (96), resulting in more sustainable agricultural practices. This goal is seen as vital in sustaining the world's growing population.
Although both soil and plant microbiomes have important roles in crop performance and yield, the greater chance of success in using plant microbiomes is driven by eco-evolutionary interactions between plant species and their microbiomes. However, to realize the full potential of the crop microbiome to support productive and sustainable croplands would require a better understanding of the structure and function of the crop microbiomes, and their interactions with host species across the globe soil types at a level similar to the Human Microbiome Project.
Role of Microbial Biotechnology in Agriculture
Agricultural technologies ensured a green revolution in the middle of the 20th century but caused high ecological costs and contributed to global pollution, unfavorable climate change, and loss of biodiversity (97). Biotechnology and microbiology together offer a broad field of research for the improvement of crop quality, crop productivity, and sustainability of existing systems to produce more and better quality agricultural products through genetically modified organisms (GMO) and transgenic crops. Microbial biotechnology played a sustainable role in sustainable agriculture in various ways, i.e., biofertilizers, biopesticides, bioherbicides, bioinsecticides, etc. Microbial biotechnology reduces the dependence on agrochemicals in sustainable agriculture by the management of biotic and abiotic stresses. This management contains several steps from the selection of microorganisms, selection of targets to the selection of genes from related or unrelated genetic resources. Microbial biotechnology assisted with genetic engineering will lead to the advancement in modifications of plant and animal pathogens for lesser virulence, disease-diagnostic tools, and improvement in microbial agents for biological control of plant and animal pests, and development of new and better microbial agents for bioremediation, etc. (98). Soil microbiome is considered as one of the major components for the microbial biotechnology tools to sustainable agriculture. In that, rhizosphere acts as a microhabitat and are rich in diversity of microorganisms (99). Microbial biotechnology assisted with genetic engineering will lead to the advancement in modifications of plant and animal pathogens for lesser virulence, disease-diagnostic tools, and improvement in microbial agents for biological control of plant and animal pests, and development of new and better microbial agents for bioremediation, etc. (100). The tools of molecular biotechnology have augmented preciseness in plant breeding by distinguishing, DNA isolation, gene cloning, and transferring of genes desired from one species to another species (101).
Soil microbes are thought of jointly as the most important parts for the sustainable production of sustainable and healthy food. Microhabitat for microorganisms in the rhizosphere is enriched with a huge diversity of microorganisms (102, 103). In biotechnology, rhizospheric microbes play a vital role in creating agricultural sustainability by varied means (104), such as increasing N2 fixation; increasing the availability of P, S, Zn, and Fe; managing abiotic and biotic stresses; increasing productivity, quality of crops, and bioremediation (105). The major benefits of microbial biotechnology are many. It helps to produce a crop without much application of chemical fertilizers, pesticides, herbicides, etc. It also keeps our environment safe and clean for the use of future generations. The benefit of microbial biotechnology helps us to avoid the use of hazardous pollutants and wastes that affect natural resources and the environment.
The development of society should be done in such a way that it helps to protect our environment and also helps us to develop it. Biotechnology and microbiology together offer a broad field of research for the improvement of crop quality, crop productivity, and sustainability of existing systems to produce more and better quality agricultural products through genetically modified organisms (GMO) and transgenic crops. Some of the examples of genetically manipulated crops for the global benefit are as follows: control ripening improves shelf life and quality (e.g., tomatoes, peas, peppers, tropical fruits, broccoli, raspberries, and melons), insect resistance to reduce insecticide use (e.g., tomatoes, potatoes, corn, rice, lettuce, coffee, cabbage family, and apples), fungal resistance to reduce fungicide use (e.g., peppers, tomatoes, and cucumbers), viral resistance to reduce diseases caused by plant viruses and, since insects carry viruses, reduce the use of insecticides (e.g., potatoes, tomatoes, cantaloupe, squash, cucumbers, corn, oilseed rape canola, soybeans, and grapes), and herbicide tolerance improves weed control (e.g., soybeans, tomatoes, corn, cotton, oilseed rape canola, and wheat) (101, 102, 106).
Biotechnological intervention plays a pivotal role in this context regarding the identification of the microbiome. In the recent past, genetic material sequencing and increment power of computation is extensively used for the detection of microbes in the microbiomes of different agro-ecologies, which makes it easy to identify microbiome in every feasible environment bypassing the necessity to isolate and culture microbes. Extrapolating such diverse knowledge is greatly worthwhile to develop approaches in the manipulation of the microbiomes for the higher acquisition of nutrients and enhanced biotic and abiotic stress tolerances causing better productivity of crops (107). As a matter of fact, the technology of sequencing, synchronized with bioinformatic tools, has not only discovered speedy microbiome identification (108) but also made it feasible to rapid gene microbiome annotation within a lesser period (109). Next-generation sequencing (NGS), a recently emerged technology, is now being used because of its remarkable advantages over the conventional method of Sanger sequencing. The advancement in this technology has caused high-throughput sequencing, which has supplementarily enhanced the various outcomes, which previously appeared to be hard and arduous by traditional techniques (110). The completed genome sequences are of great quality by characterization, represent further precise genomic data, and can be implemented for model organisms and agriculturally important microorganisms (111). Marching ahead in this direction, the recently advanced sequencing tool metagenomics reveals the study of genetic matter of microbes that exists in environmental samples, by employing high-throughput sequencing tools. In studying the aboveground and belowground microbiomes, metagenomic analysis has a huge potential to provide sufficient information about the detection of microbial composition and diversity, novel genes, microbial pathways, and interactions.
Due to the cheap cost of sequencing, an amazing number of microbiomes have been discovered and identified in the earlier contemporary period using studies of metagenomics (112) through direct assessment of the sequence of the 16S/18S rDNA amplicons. Additionally, the third-generation sequencing technique, SMRT (single-molecule real-time sequencing), does not require a prior PCR process that relies upon a single molecule of DNA. The pyrosequencing of 16S/18S rDNA amplicons is now in use to inspect the taxonomical identification (from the phylum to the genus level) of the microbiome in various soils (91).
Agricultural practices are, as of now, implemented on a worldwide scale, and diverse methodologies are being routed to meet sustainable environmental and economical developments, with the final aims of maintaining yield while safeguarding the biosphere. Agricultural microbiology is introduced as a synthetic research field responsible for the exchange of knowledge from general microbiology and microbial ecology to agricultural biotechnology. Analysis of the regular circulation of microorganisms between plants, animal, and soil-borne niches is required to reconstruct the arrangement of the microbiota in natural and agricultural ecosystems (113).
Microbiota for Human Health
As a major source of food, fiber, and biodiversity, the soil is considered an important resource not only for agriculture but also for human health and the environment. Plants require nutrients and clean water to generate nutritious food, and healthy soils provide them. Humans are becoming increasingly conscious of how their nutrition affects their overall health. However, we frequently overlook the complete food life cycle, focusing solely on the quality of the last processing phases and ignoring the soil's health, which is essential to sustain the 95% of food derived from the soil (114). Healthy soil generates a healthy society. Soil microbes play a key role in determining the nutrient content of our food through the mineralization of degradable organic compounds to inorganic forms that are readily available to crops. The large diversity of microbiome in soil affects its microbial ecology, including its primary productivity and nutrient cycling. Healthy soils are the foundation of sustainable and productive agroecosystems, and they can be maintained by following basic soil health principles, such as minimal soil disturbance, protecting surface soils by growing cover crops, increasing plant diversity through crop rotation, and organic manure enhancing soil microbiome, etc. (115). Agroecosystem/natural ecosystem (“agroecosystem that has been modified for the production of food and fiber”) functioning has been isolated from the internal cycle of important plant nutrients, such as nitrogen and phosphorus, due to a heavy reliance on artificial chemical fertilizers and pesticides in the soil rhizosphere. Successful application of beneficial microbes helps in maintaining soil health, improving water holding capacity, carbon storage, root growth, availability and cycling of essential nutrients, filtering pollutants, and also in conservation of biodiversity (110, 116, 117). The maintenance and development of soil health and fertility are important for our agricultural productivity and human health considered as mostly dependent on plant-based products (118, 119).
At present, the biofortification approach is getting much attention in increasing the availability of micronutrients, especially Fe and Zn, in the major food crops. Biofortification is a sustainable process that can increase the vitamin or mineral nutrient contents in cultivated plants or animals via agronomic practices, plant breeding, or transgenic techniques (120). The production of biofortified staple crops mainly depends on the content of available soil nutrients for plants and then human health and nutrition. Promising results have been obtained using the biofortification of different nutrients or vitamins for many crops under different climatic conditions. Van Der Heijden et al. (121) reported that combined application with native plant growth-promoting rhizobacteria (PGPR) combined with arbuscular mycorrhizal fungi (AM) showed the best effect on yield and biofortification of micronutrients (Fe and Zn) in wheat grain. The Food Safety Authority of Ireland (122) reported that the use of microorganisms possessing multifarious nutrient sequestering and plant growth-promoting traits, which can load high levels of minerals in plant roots and translocate to the edible parts, can be another promising biofortification strategy. Bouis and Saltzman (123) reported that the iron concentration doubled in lentil seeds when grown in association with pseudomonas species.
Bioprocessing engineering has developed this further, with the particular production of food. The genus Lactobacillus and other species of Lactobacillus are beneficial microbes of particular interest because of their long history of use (123). The term “probiotic” is used to describe food supplements, specifically designed to improve health, and this concludes the probiotic as a “live microbial feed supplement which beneficially affects the host by improving its microbial balance.” The procedure of microorganisms by which they act as probiotics or do their effects are not properly unknown, but the many studies revealed that they may be involved in modifying the pH value, may neutralize the pathogens through the production of compounds that exerts the property of antimicrobial (probiotics produce a wide range of antimicrobial metabolites, i.e., organic acids, diacetyl, acetoin, hydrogen peroxide, and bacteriocins). These activities contribute to microbiological safety by controlling the growth of other microorganisms, and inhibit pathogenic bacteria, and may occupy the receptor sites of the pathogens as well as chase them for an available nutrient (124). In human health, apart from their application, phytase-producing microbes provide great promises for nutritional applications in human food and animal feed. Exogenous phytase addition has been used to enhance the mineral bioavailability (125), and dephytinization, by the addition of exogenous phytase in porridges of different cereal crops, such as maize, oats, rice, and wheat, has been shown to improve iron bioavailability in humans (126). It has been demonstrated that phytic acid significantly reduces (about 86%) bioavailability of Fe from infant cereal diets in an in vitro digestion study in a Caco-2 cell model (127), and dephytinization markedly improved Fe and Zn bioavailability in these diets (128). The present review revealed microbes reported from different sources including plant microbiome, human microbiomes, and its biotechnological application in agriculture, and human health. Microbiomes having multifunctional plant growth-promoting attributes can be utilized as bio-inoculants for sustainable agriculture.
Conclusion
This review examined the role of the microbiome in sustainable agriculture and human health. In sustainable agriculture, soil health is mainly determined by the presence and diversity of microbes present in the soil rhizosphere. The diversity and abundance of soil and rhizosphere microorganisms influence plant composition, productivity, and sustainability. Deploying microbes to improve agriculture productivity is an extremely attractive approach that is non-transgenic and can be viewed collectively as the extended plant genome. Because these same microbes can contribute to restoring soil health and productivity, they have a bright future in low-input, sustainable agriculture. Improved assessment of soil health indicators is necessary to further enhance our understanding of how production strategies and environmental factors affect the physical, biological, and chemical stability and dynamics of the soil–rhizosphere–plant systems and their impact on short- or long-term sustainability.
Future Prospects
One of the challenges for future research work includes the protection and conservation of rhizosphere biodiversity and its potential application in agricultural soils. The current use of microbial inoculants has proven useful to address some agronomic challenges; however, large-scale adoption remains low mainly owing to inconsistency in the efficacy under different environmental conditions. The increasing demand for safe food and better nutrition, advancing research technologies, and interest in sustainable agriculture have further renewed global interest in the microbiome. More knowledge and deeper understanding are needed on how agronomic practices under changing climatic conditions affect the composition, abundance, and biofunctionality of microbes in delivering multiple agroecosystem services. Sustainable agriculture is important for today's agricultural practices because policymakers, conservationists, ecologists, scientists, farmers, and biologists are all interested in debating the topic of sustainable agriculture (129). Presently, the agricultural soil has been polluted due to extensive use of mineral fertilizers and agrochemicals. The use of the microbiome offers an assured distinct advantage over many other control agents and methods. The use of microbial technology would be fruitful for the development of ecological and sustainable agriculture (130–133).
Author Contributions
JS and AR were made substantial contributions to conception and design, drafting the manuscript. SO, SS, and CG were to be accountable for all aspects of the work. All authors listed have made a substantial, direct, and intellectual contribution to the work and approved it for publication.
Conflict of Interest
The authors declare that the research was conducted in the absence of any commercial or financial relationships that could be construed as a potential conflict of interest.
Publisher's Note
All claims expressed in this article are solely those of the authors and do not necessarily represent those of their affiliated organizations, or those of the publisher, the editors and the reviewers. Any product that may be evaluated in this article, or claim that may be made by its manufacturer, is not guaranteed or endorsed by the publisher.
References
1. Poveda J. Insect frass in the development of sustainable agriculture. A review. Agron Sustain Dev. (2021) 41:1–10. doi: 10.1007/s13593-020-00656-x
2. Schlaeppi K, Bulgarelli D. The plant microbiome at work. Mol Plant Microbe Interact. (2015) 212:212–7. doi: 10.1094/MPMI-10-14-0334-FI
3. Lederberg BJ, McCray AT. ‘Ome sweet' omics - a genealogical treasury of words. Science. (2001) 15:8.
4. Santos LF, Olivares FL. Plant microbiome structure and benefits for sustainable agriculture. Curr Plant Biol. (2021) 26:100198. doi: 10.1016/j.cpb.2021.100198
5. Trivedi P, Mattupalli C, Eversole K, Leach JE. Enabling sustainable agriculture through understanding and enhancement of microbiomes. Transley Review. New Phytol. (2021) 230:2129–47. doi: 10.1111/nph.17319
6. Prasanna R, Nain L, Rana A, Shivay YS. Biofortification with microorganisms: present status and future challenges. Biofortif Food Crops. (2016) 249–62. doi: 10.1007/978-81-322-2716-8_19
7. Molino S, Lerma Aguilera A, Jimenez Hernandez, N Gosalbes MJ, Rufian Henares JÁ, Francino MP. Enrichment of food with tannin extracts promotes healthy changes in the human gut microbiota. Front Microbiol. (2021) 12:570. doi: 10.3389/fmicb.2021.625782
8. Yadav AN, Kumar R, Kumar S, Kumar V, Sugitha TC, Singh B, et al. Beneficial microbiomes: biodiversity and potential biotechnological applications for sustainable agriculture and human health. J Appl Biol Biotechnol. (2017) 5:45–57. doi: 10.7324/JABB.2017.50607
9. Wu Z, Bañuelos GS, Lin ZQ, Liu Y, Yuan L, Yin X, et al. Biofortification and phytoremediation of selenium in China. Front Plant Sci. (2015) 6:136. doi: 10.3389/fpls.2015.00136
10. Berutti A, Lumini E, Balestrini R, Bianciotto V. Arbuscular mycorrhizal fungi as natural biofertilizers: let's benefit from past successes. Front Microbiol. (2016) 6:1559. doi: 10.3389/fmicb.2015.01559
11. Sundaria N, Singh M, Upreti P, Chauhan RP, Jaiswal JP, Kumar A. Seed priming with iron oxide nanoparticles triggers acquisistion and biofortification in wheat (Triticum aestivum L.). J Plant Growth Regulat. (2019) 38:122–31. doi: 10.1007/s00344-018-9818-7
12. Ray P, Lakshmanan V, Labbe JL Craven KD. Microbe to microbiome: a paradigm shift in the application of microorganisms for sustainable agriculture. Front Microbiol. (2020) 11:622926. doi: 10.3389/fmicb.2020.622926
13. Alexandridis TK, Andrianopoulos A, Galanis G, Kalopesa E, Dimitrakos A, Katsogiannos F, et al. An Integrated approach to promote precision farming as a measure toward reduced-input agriculture in Northern Greece using a spatial decision support system. Book chapter. In: Huang B, editor. Comprehensive Geographic Information Systems. 1st ed. (2017). p. 315–52.
14. Kughur PG, Otene V, Audu OC. Effects of intensive agricultural production on the environment in benue state, Nigeria. IOSR J Agric Vet Sci. (2015) 8:7–11. doi: 10.9790/2380-08810711
15. Kumar A, Verma JP. The role of microbes to improve crop productivity and soil health. In: Yarenyam A, Abhijit M, editors. Ecological Wisdom Inspired Restoration Engineering, EcoWISE. Singapore: Springer (2019).
16. Farms and Land in Farms (USDA National Agricultural Statistics Service Feb. 1999). Available online at: http://usda.mannlib.cornell.edu/usda/nass/FarmLandIn/1990s/1999/FarmLandIn-02-26-1999.pdf (accessed August 23, 2007).
17. White PJ, Crawford JW, Diaz Alvarez MC, Garcia Moreno R. Soil Management for Sustainable Agriculture. Editorial. Hindawi Publishing Corporation (2012). doi: 10.1155/2012/850739
18. Machado S. Does intercropping have a role in modern agriculture? J Soil Water Conserv. (2009) 64:233–9 doi: 10.2489/jswc.64.2.55A
19. Hauggaard-Nielsen H, Andersen MK, Joernsgaard B, Jensen ES. Density and relative frequency effects on competitive interactions and resource use in pea–barley intercrops. Field Crops Res. (2006) 95:256–67 doi: 10.1016/j.fcr.2005.03.003
20. Glaze-Corcoran S, Hashemi M, Sadeghpour A, Jahanzad E, Afshar RK, Liu X. Understanding intercropping to improve agricultural resiliency and environmental sustainability. Adv Agron. (2020) 162:199–256. doi: 10.1016/bs.agron.2020.02.004
21. Eskandari H. Intercropping of wheat (Triticum aestivum) and bean (Vicia faba): effects of complementarity and competition of intercrop components in resource consumption on dry matter production and weed growth. Afr J Biotechnol. (2011) 10:17755–62. doi: 10.5897/AJB11.2250
22. Devi P, Kumar P. Enhancement effect of biofertilizers on germination percentage and plant height in maize grown under chromium toxic soil. J Pharmacognosy Phytochem. (2020) 9:702–7. doi: 10.13140/RG.2.2.13660.31367
23. Veneklaas EJ, Stevens J, Cawthray GR, Turner S, Grigg AM, Lambers H. Chickpea and white lupine rhizosphere carboxylates vary with soil properties and enhance phosphorus uptake. Plant Soil. (2003) 248:187–97. doi: 10.1023/A:1022367312851
24. Hocking PJ, Randall PJ. Better growth and phosphorus nutrition of sorghum and wheat following organic acid secreting crops. In: Wittenmayer L, editor, Plant Nutrition. Food Security and Sustainability of Agro-Ecosystems Through Basic and Applied Research (2001).
25. Khadr MS, Abou-El-Enwin R, El-Shafy A, Zahran F, Zohry A. Sustainability of soil fertility status after 3-year crop rotation in sandy soils in Egypt. Egypt J Agric Res. (2004) 82:475–91.
26. Kelley KW, Long JH, Todd TC. Long-term crop rotations affect soybean yield, seed weight, and soil chemical properties. Field Crops Res. (2003) 83:41–50. doi: 10.1016/S0378-4290(03)00055-8
27. Nawaz M, Mabubu JI, Hua H. Current status and advancement of biopesticides: microbial and botanical pesticides. J Entomolo Zoo Stud. (2016) 4:241246
28. Koul O. Plant biodiversity as a resource for natural products for insect pest management. In: Gurr GM, Wratten SD, Snyder WE, Read DMY, editors. Biodiversity and Insect Pests: Key Issues for Sustainable Management. Sussex: Wiley (2012). p. 85–105.
29. Awaad H, El-Naggar N. Potential role of intercropping in maintaining and cilitating environmental sustainability. sustainability of agricultural environment in egypt: part I - soil-water-food nexus. Hdb Env Chem. (2018) 76:81–100. doi: 10.1007/698_2017_163
30. Pittelkow CM, Liang X, Linquist BA, van Groenigen KJ, Lee J, Lundy ME, et al. Productivity limits and potentials of the principles of conservation agriculture. Nature. (2014) 517:365. doi: 10.1038/nature13809
31. Li Y, Chang SX, Tian LH, Zhang QP. Conservation agriculture practices increase soil microbial biomass carbon and nitrogen in agricultural soils: a global meta-analysis. Soil Biol Biochem. (2018) 121:50–8. doi: 10.1016/j.soilbio.2018.02.024
32. Somenahally A, DuPont JI, Brady J, McLawrence J, Northup B, Gowda P. Microbial communities in soil profile are more responsive to legacy effects of wheat-cover crop rotations than tillage systems. Soil Biol Biochem. (2018) 123:126–35. doi: 10.1016/j.soilbio.2018.04.025
33. Zuber SM, Villamil MB. Meta-analysis approach to assessing the effect of tillage on microbial biomass and enzyme activities. Soil Biol. Biochem. (2016) 97:176–87. doi: 10.1016/j.soilbio.2016.03.011
34. Hewins DB, Sinsabaugh RL, Archer SR, Throop HL. Soil-litter mixing and microbial activity mediate decomposition and soil aggregate formation in a sandy shrub-invaded Chihuahuan Desert grassland. Plant Ecol. (2017) 218:459–74. doi: 10.1007/s11258-017-0703-4
35. Gottshall CB, Cooper M, Emery SM. Activity, diversity and function of arbuscular mycorrhizae vary with changes in agricultural management intensity. Agric Ecosyst Environ. (2017) 241:142–9. doi: 10.1016/j.agee.2017.03.011
36. Helgason BL, Walley FL, Germida JJ. Long-term no-till management affects microbial biomass but not community composition in Canadian prairie agroecosytems. Soil Biol Biochem. (2010) 42:2192–202. doi: 10.1016/j.soilbio.2010.08.015
37. Schmidt R, Gravuer K, Bossange AV, Mitchell J, Scow K. Long-term use of cover crops and no-till shift soil microbial community life strategies in agricultural soil. PLoS ONE. (2018) 13:e0192953. doi: 10.1371/journal.pone.0192953
38. Lupwayi NZ, May WE, Kanashiro DA, Petri RM. Soil bacterial community responses to black medic cover crop and fertilizer N under no-till. Appl Soil Ecol. (2018) 124:95–103. doi: 10.1016/j.apsoil.2017.11.003
39. Li Y, Li Z, Cui S, Jagadamma S, Zhang Q. Residue retention and minimum tillage improve physical environment of the soil in croplands: a global meta-analysis. Soil Tillage Res. (2019) 194:104292. doi: 10.1016/j.still.2019.06.009
40. Wang Y, Li C, Tu C, Hoyt GD, DeForest JL, Hu S. Long-term no-tillage and organic input management enhanced the diversity and stability of soil microbial community. Sci Total Environ. (2017) 609:341–7. doi: 10.1016/j.scitotenv.2017.07.053
41. Nair P, K R. An Introduction to Agroforestry. Dordrecht: Kluwer Academic Publishers Netherlands. (1993). p. 499.
42. Kaul A, Kaur R, Choudhary AK, Sepat S, Dass A. Importance of green manuring in sustainable agriculture. Popular Kheti. (2018) 3:2361–6.
43. Komatsuzaki M, Ohta H. Soil management practices for sustainable agro-ecosystems. Sustain Sci. (2007) 2:103–20. doi: 10.1007/s11625-006-0014-5
44. Hasan ZA, Khan A, Jha AK, Razzaq A, Sajjad MR, Muhammad G. Green manuring for improved wheat yield through moisture conservation in rainfed areas of Pakistan. J Agri Food Appl Sci. (2004) 2:171–3.
45. Singh S, Singh RJ, Kumar K, Singh B, Shukla L. Biofertilizers and Green Manuring for Sustainable Agriculture, 1st ed. New Delhi: New India Publishing Agency (2013). p. 129–50.
46. Ghosh PK, Bandyopadhyay KK, Wanjari RH, Manna MC, Misra AK, Mohanty M, et al. Legume effect for enhancing productivity and nutrient use-efficiency in major cropping systems– an indian perspective: a review. J Sustain Agr. (2007) 30:59–86. doi: 10.1300/J064v30n01_07
47. Salas-Marina MA, Silva-Flores MA, Uresti-Rivera EE, Castro-Longoria E, Herrera-Estrella A, Casas-Flores S. Colonization of Arabidopsis roots by Trichoderma atroviride promotes growth and enhances systemic disease resistance through jasmonic acid/ethylene and salicylic acid pathways. Eur J Plant Pathol. (2011) 131:15–26. doi: 10.1007/s10658-011-9782-6
48. Praveen Kumar G, Mir Hassan Ahmed SK, Desai S, Leo Daniel Amalraj E, Rasul A. In vitro screening for abiotic stress tolerance in potent biocontrol and plant growth promoting strains of Pseudomonas and Bacillus spp. Int J Bacteriol. (2014) 2014:195946. doi: 10.1155/2014/195946
49. Romeh AA. Integrated pest management for sustainable agriculture. Sustainability of agricultural environment. Egypt: part II - soil-water-plant nexus. Hdb Env Chem. (2018). doi: 10.1007/698_2018_267
50. Srinivasan R, Tamo M, Lee ST, Lin MY, Huang CC, Hsu YC. Towards developing a biological control program for legume pod borer, Maruca vitrata. In: Gupta S, Ali M, Singh BB, editors. Grain Legumes: Genetic Improvement, Management and Trade. Kanpur: Indian Society of Pulses Research and Development (2009). p. 183–96.
51. Sanchez PA. Soil Productivity and Sustainability in Agroforestry Systems. Raleigh, NC: North Carolina Agricultural Research Service (1987).
52. Hashem A, Abd_Allah EF, Alqarawi AA, Radhakrishnan R, Kumar A. Plant defense approach of bacillus subtilis (Bera 71) against Macrophomina phaseolina (tassi) Goid in mung bean. J Plant Interact. (2017) 12:390–401. doi: 10.1080/17429145.2017.1373871
53. Kohl L, Oehl F, Van Der Heijden MGA. Agricultural practices indirectly influence plant productivity and ecosystem services through effects on soil biota. Ecol Appl. (2014) 24:1842–53. doi: 10.1890/13-1821.1
54. Kumar A, Sharma S, Mishra S, Dames JF. Arbuscular mycorrhizal inoculation improves growth and antioxidative response of Jatropha curcas (L.) under Na2SO4 salt stress. Plant Biosyst. (2015) 149:260–9. doi: 10.1080/11263504.2013.845268
55. Hunter P. Plant microbiomes and sustainable agriculture. EMBO Rep. (2016) 17:1696–9. doi: 10.15252/embr.201643476
56. Trivedi P, Delgado-Baquerizo M, Trivedi C, Hamonts K, Anderson IC, Singh BK. Keystone microbial taxa regulate the invasion of a fungal pathogen in agro-ecosystems. Soil Biol Biochem. (2017) 111:10–14. doi: 10.1016/j.soilbio.2017.03.013
57. Pylro VS, Roesch LFW, Ortega JM, do Amaral AM, Totola MR, Hirsch PR, et al. Brazilian microbiome project: revealing the unexplored microbial diversity-challenges and prospects. Microb Ecol. (2014) 67:237–41. doi: 10.1007/s00248-013-0302-4
58. Aguiar-Pulido V, Huang W, Suarez-Ulloa V, Cickovski T, Mathee K, Narasimhan G. Metagenomics, metatranscriptomics, and metabolomics approaches for microbiome analysis. Evol Bioinforma. (2016) 12:5–16. doi: 10.4137/EBO.S36436
59. Duran P, Tortella G, Viscardi S, Barra PJ, Carrion VJ, De La Luz Mora M, et al. Microbial community composition in take-All suppressive soils. Front Microbiol. (2018) 9:1–15. doi: 10.3389/fmicb.2018.02198
60. Gaiero JR, McCall CA, Thompson KA, Day NJ, Best AS, Dunfield KE. Inside the root microbiome: bacterial root endophytes and plant growth promotion. Am J Bot. (2013) 100:1738–50. doi: 10.3732/ajb.1200572
61. Müller DB, Vogel C, Bai Y, Vorholt JA. The plant microbiota: systems-level insights and perspectives. Annu Rev Genet. (2016) 50:211–34. doi: 10.1146/annurev-genet-120215-034952
62. Kumar I, Mondal M, Gurusamy R, Balakrishnan S, Natarajan S. Plant-microbiome interaction and the effects of biotic and abiotic components in agroecosystem. Microb Interv Agric Environ. (2019) 2:517–46. doi: 10.1007/978-981-13-8383-0_18
63. Velazquez E, Carro L, FloresFelix JD. The legume nodule microbiome: a source of plant growth-promoting Bacteria. Probiotics Plant Heal. (2017) 41–70. doi: 10.1007/978-981-10-3473-2_3
64. Stringlis IA, Zhang H, Pieterse CM, Bolton MD, de Jonge R. Microbial small molecules-weapons of plant subversion. Nat Prod Rep. (2018) 35:410–33. doi: 10.1039/C7NP00062F
65. Ellis JG. Can plant microbiome studies lead to effective biocontrol of plant diseases? Mol Plant Microbe Interact. (2017) 30:190–3. doi: 10.1094/MPMI-12-16-0252-CR
66. Hopkins SR, Wojdak JM, Belden LK. Defensive symbionts mediate host–Parasite interactions at multiple scales. Trends Parasitol. (2017) 33:53–64. doi: 10.1016/j.pt.2016.10.003
67. S Compant, A Samad, H Faist Sessitsch A. A review on the plant microbiome: ecology, functions, and emerging trends in microbial application. J Adv Res. (2019) 19:29–37. doi: 10.1016/j.jare.2019.03.004
68. Mohler CL. Enhancing the competitive ability of crops. In: Liebman M, Mohler CL, Staver CP, editors. Ecological Management of Agricultural Weeds. New York, NY: Cambridge University Press. (2001). p. 269–321.
69. Yergeau E, Bell TH, Champagne J, Maynard C, Tardif S, Tremblay J, et al. Transplanting soil microbiomes leads to lasting effects on willow growth, but not on the rhizosphere microbiome. Front Microbiol. (2015) 6:1436. doi: 10.3389/fmicb.2015.01436
70. Tardif S, Yergeau É, Tremblay J, Legendre P, Whyte LG, Greer CW. The willow microbiome is influenced by soil petroleum-hydrocarbon concentration with plant compartment-specific effects. Front Microbiol. (2016) 7:1363. doi: 10.3389/fmicb.2016.01363
71. Johns NI, Blazejewski T, Gomes AL, Wang HH. Principles for designing synthetic microbial communities. Curr Opin Microbiol. (2016) 31:146–53. doi: 10.1016/j.mib.2016.03.010
72. Bar On YM, Phillips R, Milo R. The biomass distribution on Earth. Proc Natl Acad Sci USA. (2018) 115:6506–11. doi: 10.1073/pnas.1711842115
73. Thies JE, Grossman JM. The soil habitat and soil ecology. In: Uphoff N, editor. Biological Approaches to Sustainable Soil Systems. Boca Raton, FL: CRC Press (2006). p. 59–78.
74. Kasim WA, Osman ME, Omar MN, Abd El-Daim IA, Bejai S, Meijer J. Control of drought stress in wheat using plant-growth-promoting bacteria. J Plant Growth Regul. (2013) 32:1–9. doi: 10.1007/s00344-012-9283-7
75. Verma JP, Yadav J, Tiwari KN, Kumar A. Effect of indigenous Mesorhizobium spp. and plant growth promoting rhizobacteria on yields and nutrients uptake of chickpea (Cicer arietinum L.) under sustainable agriculture. Ecol Eng. (2013) 51:282–6. doi: 10.1016/j.ecoleng.2012.12.022
76. Lim JH, Kim SD. Induction of drought stress resistance by multi-functional PGPR Bacillus licheniformis K11 in pepper. Plant Patho J. (2013) 29:201–8. doi: 10.5423/PPJ.SI.02.2013.0021
77. Muleta D, Assefa F, Börjesson E, Granhall U. Phosphate-solubilising rhizobacteria associated with Coffea arabica L. in natural coffee forests of southwestern Ethiopia. J Saudi Soc Agric Sci. (2013) 12:73–84. doi: 10.1016/j.jssas.2012.07.002
78. Timmusk S, Islam A, Abd El D, Lucian C, Tanilas T, Kännaste A, et al. Drought-tolerance of wheat improved by rhizosphere bacteria from harsh environments: enhanced biomass production and reduced emissions of stress volatiles. PLoS ONE. (2014) 9:e96086 doi: 10.1371/journal.pone.0096086
79. Kang SM, Radhakrishnan R, Khan AL, Kim MJ, Park JM, Kim BR, et al. Gibberellin secreting rhizobacterium, Pseudomonas putida H-2-3 modulates the hormonal and stress physiology of soybean to improve the plant growth under saline and drought conditions. Plant Physiol Biochem. (2014) 84:115–24. doi: 10.1016/j.plaphy.2014.09.001
80. Barelli L, Waller AS, Behie SW, Bidochka MJ. Plant microbiome analysis after Metarhizium amendment reveals increases in abundance of plant growth-promoting organisms and maintenance of disease-suppressive soil. PLoS ONE. (2020) 15:e0231150. doi: 10.1371/journal.pone.0231150
81. Gu Y, Dong K, Geisen S, Yang W, Yan Y, Gu D, et al. The effect of microbial inoculant origin on the rhizosphere bacterial community composition and plant growth-promotion. Plant Soil. (2020) 452:105–17. doi: 10.1007/s11104-020-04545-w
82. Lebreton L, Guillerm-Erckelboudt AY, Gazengel K, Linglin J, Ourry M, Glory P, et al. Temporal dynamics of bacterial and fungal communities during the infection of Brassica rapa roots by the protist Plasmodiophora brassicae. PLoS ONE. (2019) 14:e0204195. doi: 10.1371/journal.pone.0204195
83. Purahong W, Orrù L, Donati I, Perpetuini G, Cellini A, Lamontanara A, et al. Plant microbiome and its link to plant health: host species, organs and Pseudomonas syringae pv. Actinidiae infection shaping bacterial phyllosphere communities of kiwifruit plants. Front Plant Sci. (2018) 871:1563. doi: 10.3389/fpls.2018.01563
84. Kumar P, Thakur S, Dhingra GK, Singh A, Pal MK, Harshvardhan K, et al. Inoculation of siderophore producing rhizobacteria and their consortium for growth enhancement of wheat plant. Biocatal Agric Biotechnol. (2018) 15:264–9. doi: 10.1016/j.bcab.2018.06.019
85. Kumar PA, Dubey RC, Maheshwari DK, Bajpai V. ACC deaminase producing Rhizobium leguminosarum RPN5 isolated from root nodules of Phaseolus vulgaris L. Bangladesh J Bot. (2016) 45:477–84.
86. Tian B, Zhang C, Ye Y, Wen J, Wu Y, Wang H, et al. Beneficial traits of bacterial endophytes belonging to the core communities of the tomato root microbiome. Agric Ecosyst Environ. (2017) 247:149–56. doi: 10.1016/j.agee.2017.06.041
87. Rascovan N, Carbonetto B, Perrig D, Díaz M, Canciani W, Abalo M, et al. Integrated analysis of root microbiomes of soybean and wheat from agricultural fields. Sci Rep. (2016) 6:1–12. doi: 10.1038/srep28084
88. Shahzad R, Khan AL, Bilal S, Waqas M, Kang SM, Lee IJ. Inoculation of abscisic acid-producing endophytic bacteria enhances salinity stress tolerance in Oryza sativa. Environ Exp Bot. (2017) 136:68–77. doi: 10.1016/j.envexpbot.2017.01.010
89. Yang J, Kloepper JW, Ryu CM. Rhizosphere bacteria help plants tolerate abiotic stress. Trends Plant Sci. (2009) 14:1–4. doi: 10.1016/j.tplants.2008.10.004
90. Anderson J, Gipmans A, Hurst M, Layton S, Nehra R, Pickett NJ, et al. Emerging agricultural biotechnologies for sustainable agriculture and food security. J Agric Food Chem. (2016) 64:383–93. doi: 10.1021/acs.jafc.5b04543
91. Barea JM. Future challenges and perspectives for applying microbial biotechnology in sustainable agriculture based on a better understanding of plant-microbiome interactions. J Soil Sci Plant Nutr. (2015) 15:261–82. doi: 10.4067/S0718-95162015005000021
92. Mosttafiz S, Rahman M, Rahman M. Biotechnology: role of microbes in sustainable agriculture and environmental health. Inter J Microbiol. (2002) 10:1–6.
93. Tripathi N. Agroecology and sustainability of agriculture in India: an overview. EC Agriculture. (2015) 2:241–8.
94. Yuan Y, Brunel C, van Kleunen M, Li J, Jin Z. Salinity-induced changes in the rhizosphere microbiome improve salt tolerance of Hibiscus hamabo. Plant Soil. (2019) 443:525–37. doi: 10.1007/s11104-019-04258-9
95. Thijs S, Sillen W, Rineau F, Weyens N, Vangronsveld J. Towards an enhanced understanding of plant-microbiome interactions to improve phytoremediation: engineering the metaorganism. Front Microbiol. (2016) 7:341. doi: 10.3389/fmicb.2016.00341
96. Thijs S, Sillen W, Weyens N, Vangronsveld J. Phytoremediation: state-of-the-art and a key role for the plant microbiome in future trends and research prospects. Int J Phytoremediat. (2016) 19:23–38. doi: 10.1080/15226514.2016.1216076
97. Gu Y, Wei Z, Wang X, Friman VP, Huang J, Wang X, et al. Pathogen invasion indirectly changes the composition of soil microbiome via shifts in root exudation profile. Biol Fertil Soils. (2016) 52:997–1005. doi: 10.1007/s00374-016-1136-2
98. Ardanov P, Lyastchenko S, Karppinen K, Häggman H, Kozyrovska N, Pirttilä AM. Effects of Methylobacterium sp. on emergence, yield, and disease prevalence in three cultivars of potato (Solanum tuberosum L.) were associated with the shift in endophytic microbial community. Plant Soil. (2016) 405:299–310. doi: 10.1007/s11104-015-2500-y
99. Erlacher A, Cardinale M, Grosch R, Grube M, Berg G. The impact of the pathogen Rhizoctonia solani and its beneficial counterpart Bacillus amyloliquefaciens on the indigenous lettuce microbiome. Front Microbiol. (2014) 5:175. doi: 10.3389/fmicb.2014.00175
100. Vance CP. Legume Symbiotic Nitrogen Fixation: Agronomic Aspects. In: Spaink HP, Kondorosi A, and Hooykaas PJJ, editors. The Rhizobiaceae. Dordrecht: Springer (1998). p. 509–30.
101. Lugtenberg B. Life of microbes in the rhizosphere. In: Lugtenberg B, editor. Principles of Plant-Microbe Interactions. Heidelberg: Springer International Publishing Switzerland (2015). p. 7–15.
102. Hirsch PR, Miller AJ, Dennis PG. Do root exudates exert more influence on rhizosphere bacterial community structure than other rhizodeposits? Book Chapter. Mol Microb Ecol Rhizosphere. (2013) 1:229–42. doi: 10.1002/9781118297674.ch22
103. Istina IN, Widiastuti H, Joy B, Antralina M. Phosphate-solubilizing microbe from saprists peat soil and their potency to enhance oil palm growth and p uptake. Proc Food Sci. (2015) 3:426–35. doi: 10.1016/j.profoo.2015.01.047
104. Sasirekha B, Srividya S. Siderophore production by Pseudomonas aeruginosa FP6, abiocontrol strain for Rhizoctonia solani and Colletotrichum gloeosporioides causing diseases in chilli. Agric Nat Resour. (2016) 50:250–6. doi: 10.1016/j.anres.2016.02.003
105. Noble AD, Ruaysoongnern S. The nature of sustainable agriculture. Book Chapter. In: Geoffrey RD, Emma LT, editors. Soil Microbiology and Sustainable Crop Production. Dordrecht: Springer (2010). p. 1–25.
106. Umesha S, Singh PK, Singh RP. Microbial biotechnology and sustainable agriculture. Book chapter. In: Biotechnology for Sustainable Agriculture. (2018). p. 185–205. Woodhead Publishing. Available online at: http://eprints.uni-mysore.ac.in/id/eprint/11322 (accessed March 06, 2020).
107. Prasad R, Gill SS, Tuteja N. New and Future Developments in Microbial Biotechnology and Bioengineering: Crop Improvement Through Microbial Biotechnology. Amsterdam: Elsevier (2018).
108. Marshall IP, Karst SM, Nielsen PH, Jorgensen BB. Metagenomes from deep Baltic Sea sediments reveal how past and present environmental conditions determine microbial community composition. Marine Genom. (2018) 37:58–68. doi: 10.1016/j.margen.2017.08.004
109. Logue JB, Burgmann H, Robinson CT. Progress in the ecological genetics and biodiversity of freshwater bacteria. BioScience. (2008) 58:103–13. doi: 10.1641/B580205
110. Bharati AP, Kumar A, Kumar S, Maurya DK, Kumari S, Agarwal DK, et al. Role of biotechnology in the exploration of soil and plant microbiomes. In: Manoj KS, Pream LK, Baby K, editors. Phytobiomes: Current Insights and Future Vistas. Singapore: Springer (2020). p. 335–55.
111. Singh A, Kumar M, Verma S, Choudhary P, Chakdar H. Plant microbiome: trends and prospects for sustainable agriculture. In: Plant-Microbe Symbiosis. Cham: Springer (2020). p. 129–51.
112. Moronta-Barrios F, Gionechetti F, Pallavicini A, Marys E, Venturi V. Bacterial microbiota of rice roots: 16S-based taxonomic profiling of endophytic and rhizospheric diversity, endophytes isolation and simplified endophytic community. Microorganisms. (2018) 6:14. doi: 10.3390/microorganisms6010014
113. Spence C, Bais H. Probiotics for plants: rhizospheric microbiome and plant fitness. Book Chapter. Mol Microb Ecol Rhizosphere. (2013) 1:713–21. doi: 10.1002/9781118297674.ch67
114. Singh R. Microbial biotechnology: a promising implement for sustainable agriculture. In: Singh JS, Singh DP, editors. New and Future Developments in Microbial Biotechnology and Bioengineering. Elsevier (2019). p. 107–14. doi: 10.1016/C2017-0-03932-0
115. Adekambi T, Drancourt M. Dissection of phylogenetic relationships among 19 rapidly growing Mycobacterium species by 16S rRNA, hsp65, sodA, recA and rpoB gene sequencing. Int J Syst Evolut Microbiol. (2004) 54:2095–105. doi: 10.1099/ijs.0.63094-0
116. Peplies J, Glockner FO, Amann R. Optimization strategies for DNA microarray-based detection of bacteria with 16S rRNA-targeting oligonucleotide probes. Appl Environ Microbiol. (2003) 69:1397–407. doi: 10.1128/AEM.69.3.1397-1407.2003
117. Chen YC, Eisner JD, Kattar MM, Rassoulian Barrett SL, LaFe K, Yarfitz SL, et al. Identification of medically important yeasts using PCR-based detection of DNA sequence polymorphisms in the internal transcribed spacer 2 region of the rRNA genes. J Clin Microbiol. (2000) 38:2302–10. doi: 10.1128/JCM.38.6.2302-2310.2000
118. Brussaard L, de Ruiter PC, Brown GG. Soil biodiversity for agricultural sustainability. Agric Ecosyst Environ. (2007) 121:233–44. doi: 10.1016/j.agee.2006.12.013
119. FAO. Tracking Progress on Food and Agriculture-Related SDG Indicators A Report on the Indicators Under FAO Custodianship. (2018). Available online at: http://www.fao.org/sdg-progress-report/en/ (accessed December 21, 2021).
120. Kibblewhite MG, Ritz K, Swift MJ. Soil health in agricultural systems. Philos Trans R Soc B Biol Sci. (2008) 363:685–701. doi: 10.1098/rstb.2007.2178
121. Van Der Heijden MGA, Bardgett RD, Van Straalen NM. The unseen majority: soil microbes as drivers of plant diversity and productivity in terrestrial ecosystems. Ecol Lett. (2008) 11:296–310. doi: 10.1111/j.1461-0248.2007.01139.x
122. Food Safety Authority of Ireland. Probiotic Health Claims. Dublin: Food Safety Authority of Ireland (2013).
123. Bouis HE, Saltzman A. Improving nutrition through biofortification: a review of evidence from HarvestPlus, 2003 through 2016. Glob Food Secur. (2017) 12:49–58. doi: 10.1016/j.gfs.2017.01.009
124. Yadav R, Ror P, Rathore P, Ramakrishna W. Bacteria from native soil in combination with arbuscular mycorrhizal fungi augment wheat yield and biofortification. Plant Physiol Biochem. (2020) 150:222–33. doi: 10.1016/j.plaphy.2020.02.039
125. Holzapfel WH. Appropriate starter culture technologies for small-scale fermentation in developing countries. Int J Food Microbiol. (2002) 75:197–212. doi: 10.1016/S0168-1605(01)00707-3
126. Lee IC, Caggianiello G, van Swam II, Taverne N, Meijerink M, Bron PA. Strain-specific features of extracellular polysaccharides and their impact on Lactobacillus plantarum-host interactions. Appl Environ Microbiol. (2016) 82:3959–70. doi: 10.1128/AEM.00306-16
127. Penella JS, Collar C, Haros M. Effect of wheat bran and enzyme addition on dough functional performance and phytic acid levels in bread. J Cereal Sci. (2008) 48:715–21. doi: 10.1016/j.jcs.2008.03.006
128. Hurrell RF, Reddy MB, Juillerat MA, Cook JD. Degradation of phytic acid in cereal porridges improves iron absorption by human subjects. Am J Clin Nutr. (2003) 77:1213–9. doi: 10.1093/ajcn/77.5.1213
129. Jin F, Frohman C, Thannhauser TW, Welch RM, Glahn RP. Effects of ascorbic acid, phytic acid and tannic acid on iron bioavailability from reconstituted ferritin measured by an in vitro digestion-Caco-2 cell model. Br J Nutr. (2009) 101:972–81. doi: 10.1017/S0007114508055621
130. Frontela C, Scarino ML, Ferruzza S, Ros G, Martínez C. Effect of dephytinization on bioavailability of iron, calcium and zinc from infant cereals assessed in the Caco-2 cell model. World J Gastroenterol. (2009) 15:1977–84. doi: 10.3748/wjg.15.1977
131. Abubakar MS, Attanda ML. The concept sustainable agriculture: challenges and prospects. Int Conf Mechatronics Materials Sci Engg. (2013) 53:012001. doi: 10.1088/1757-899X/53/1/012001
132. Garcia-Orenes F, Morugan-Coronado A, Zornoza R, Scow K. Changes in soil microbial community structure influenced by agricultural management practices in a Mediterranean agro-ecosystem. PLoS ONE. (2013) 8:e80522 doi: 10.1371/journal.pone.0080522
Keywords: symbiotic association, microbiome, rhizosphere, solubilization, human health
Citation: Suman J, Rakshit A, Ogireddy SD, Singh S, Gupta C and Chandrakala J (2022) Microbiome as a Key Player in Sustainable Agriculture and Human Health. Front. Soil Sci. 2:821589. doi: 10.3389/fsoil.2022.821589
Received: 24 November 2021; Accepted: 14 February 2022;
Published: 11 April 2022.
Edited by:
Saurav Das, University of Nebraska-Lincoln, United StatesReviewed by:
Avishesh Neupane, The University of Tennessee, Knoxville, United StatesOjas Natarajan, University of South Florida, United States
Bijit Bhowmik, Croda, United Kingdom
Copyright © 2022 Suman, Rakshit, Ogireddy, Singh, Gupta and Chandrakala. This is an open-access article distributed under the terms of the Creative Commons Attribution License (CC BY). The use, distribution or reproduction in other forums is permitted, provided the original author(s) and the copyright owner(s) are credited and that the original publication in this journal is cited, in accordance with accepted academic practice. No use, distribution or reproduction is permitted which does not comply with these terms.
*Correspondence: Jarupula Suman, jarupulajnkvv@gmail.com; Amitava Rakshit, amitavar@bhu.ac.in