Fundamental physics studies in time domain and multi-messenger astronomy
- Los Alamos National Laboratory, Center for Theoretical Astrophysics, Los Alamos, NM, United States
The era of ime domain and multi-messenger astronomy is not only leading to the development of a much broader set of detectors and instruments for astrophysical observations, but is also providing the means for astronomy to tie directly to cutting-edge studies in physics. In this manner, fundamental physics (theory and experiment) coupled with a strong theoretical understanding of astrophysical phenomena (guided by high-performance computing simulations) can tie directly to the amazing new observations in astronomy. This paper discusses how physics, astrophysical models, and observations can not only help astronomy probe fundamental physics but guide the needs for next-generation astrophysical missions.
1 Introduction
Time Domain and Multi-Messenger (TDAMM) astronomy is one of the fastest-evolving fields in astronomy, with new discoveries occurring annually both through the discovery of new phenomena (e.g., new classes of transients) and the availability of new observational regimes (different photon-energy band or new messengers like dust, gravitational waves, and neutrinos). With the rapid growth of data, astronomers can now leverage an abundant and diverse set of data. But to take advantage of this data, astronomers must understand the uncertainties and biases of each diagnostic.
The uncertainties are driven by uncertainties in the observations (e.g., instrumentation techniques) and the process by which we interpret them which includes uncertainties in the analysis procedures, our understanding of the fundamental physics behind the phenomenon, and the numerical issues in the modeling. The biases can only truly be understood if we have a comprehensive model that connects the different diagnostics. This requires detailed multi-physics models, often requiring the coupling of simulations from multiple codes running on the latest advances in supercomputers. It also requires improving the fidelity of the physics models used in these codes. As such, TDAMM astronomy provides an ideal conduit between the latest advances fundamental physics and astrophysical phenomena.
As an example of one such phenomena, we consider the growing set of diagnostics that constrain the supernova engine (Fryer et al., 2023a). Type Ib, Ic and all II supernovae are believed to be driven by the energy released when the core of a massive star collapses down to a proto-neutron star. The gravitational potential energy released is
But a number of observations could help us constrain the growth and nature of this convection. The outward mixing of the 56Ni synthesized in the engine, observed in both the gamma-rays and infra-red lines of SN 1987A (for a review, see Hungerford et al., 2003), provided the first clues of this engine. Observations of the 44Ti distribution in Cassiopeia A confirmed this convective engine (Grefenstette et al., 2017). But these observations were limited to single events and, as we obtain observations of more systems, we can better understand both the characteristics of this engine and its dominance in the population of observed supernova explosions. Astronomers can tap a diagnostics to probe the supernova engine (Fryer et al., 2023a). Photons across a broad wavelength range provide a myriad of constraints, providing indirect (e.g., supernova light-curves) and direct (e.g., gamma-rays) clues into the nature of the engine. Additional constraints, such as the compact remnant mass distribution also improve our understanding. The most direct observations are rare, e.g., gravitational waves and neutrinos, but a Milky Way supernova with these observables will provide the foundation for our understanding of this engine. Tying these results with the large number of supernova light-curves and spectral observations will allow us to study the core-collapse engine as a broad population.
Connecting all these observations requires a series of cutting edge simulations, as well as a deep understanding of the theory behind these simulations, the fundamental physics required in these simulations, and of the observations providing insight into the problem. At this time, much work needs to be done to improve our physics understanding, the codes that implement this physics to study complex phenomena, and our interpretation of the simulations from these calculations so that we can best connect them to the observations. Understanding the observations also requires characterizing the uncertainties in both instrumentation and analysis tools. Most scientists focus on one narrow aspect of this problem and, to move forward, we must train scientists to break out of their stove-pipes and understand a more holistic picture of how we will use the upcoming flood of TDAMM data to understand astrophysical transients. In turn, our improved understanding of the process from fundamental physics to observed phenomena will help guide the priorities for next-generation detectors (both ground- and space-based) to maximize the science gain from these missions.
Instead of focusing on a single problem as we did here with the supernova engine, this paper will focus on a series of fundamental physics studies and their ties to observations: nuclear physics and nucleosynthetic yields (Section 2), radiation hydrodynamics and its effect on shocked emission (Section 3), and plasma physics and its importance in spectra (Section 4). With these three examples, we demonstrate just how interconnected and important fundamental physics studies (both theory and experiment) are to the upcoming TDAMM era. We discuss that future in Section 5.
2 Nuclear physics and nucleosynthesis
A broad range of nuclear physics is important to TDAMM phenomena including nuclear cross-sections, neutrino physics and the behavior of matter at extreme conditions (nuclear densities, ∼ 1014 g cm−3, and temperatures above 10 MeV). In this section, we discuss the role of nuclear physics on observations constraining the formation of elements in the universe. The coupling between physics experiment to astrophysics observation is not direct. In most cases, it requires implementing physical models into a series of astrophysical studies (each requiring both detailed simulations and a theoretical understanding) that then tie to observations. This complex path means that experts across multiple fields must work together to do a complete study. Figure 1 diagrams this complex path. In the rest of this section we will discuss, for a variety of astrophysical observations, how these studies tie together to produce a full system study.
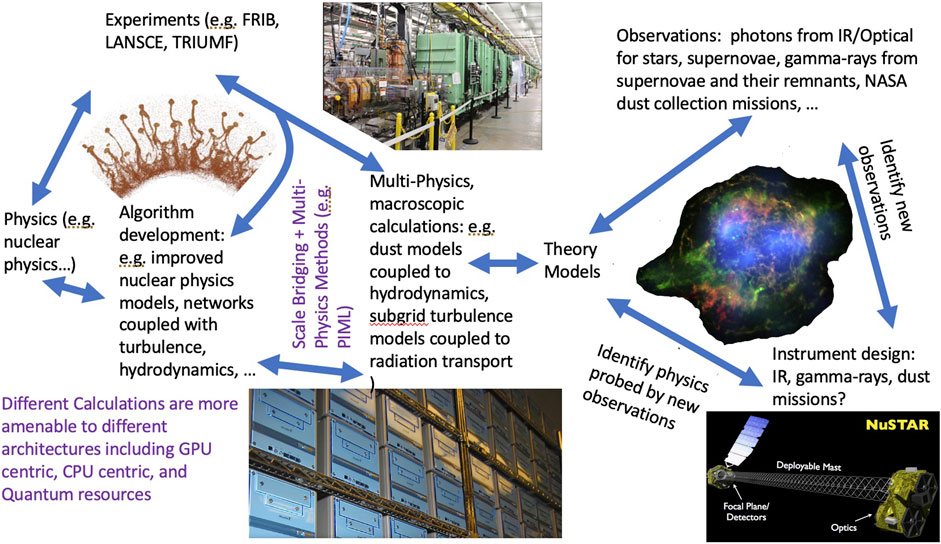
Figure 1. Nuclear Physics: This diagram shows the broad connection between fundamental physics fields and astrophysical phenomena studying nucleosynthesis and nuclear physics. To tie to observations, a great deal more physics must be included in the simulations, requiring the development of multi-physics algorithms. For example, dust grain studies require coupling both materials physics (e.g., density functional theory) and nuclear physics. Scientist leverage these fundamental studies to develop algorithms used in physics simulation that ultimately get implemented into macroscopic high-performance computing calculations used to compare to experiment. These macroscopic calculations are often needed to model the experiments successfully. The cycle from fundamental physics, algorithm development, macroscopic codes and experiment helps to fine-tune the codes. But there is another cycle from macroscopic simulations to astrophysical simulations to instrument design for observatories. Astrophysical observations, through macroscopic simulations of astrophysical phenomena can then feed back into the fundamental physics just as experiment does. The figures from bottom left moving clockwise are: the space simulator (Warren et al., 2003) Beowulf cluster (precursor for modern commodity computing) where the first 3-dimensional supernova simulations were run (Fryer and Warren, 2002), simulations of turbulent mixing (Ellinger et al., 2012), an image of the Facility for Rare Isotope Beams (FRIB https://frib.msu.edu/index.php), an image of Cassiopeia A including both Chandra observation (Hwang et al., 2004) the 44Ti decay emission from NuSTAR (Grefenstette et al., 2017), and an image of the NuSTAR satellite (Harrison et al., 2013). The FRIB image is adapted from in wikipedia (FRIBcomm) ∼ https://en.wikipedia.org/wiki/Creative_Commons.
Before we do specific examples, lets discuss some sample science goals. For instance, science goals include: studying the neutrino signal and probing neutrino physics, studying properties of neutron stars, and understanding the origin of the heavy elements. For this discussion, we will focus on nucleosynthetic yields and, in particular, the study of the production of elements up to the iron peak (although we will discuss heavy element production, rapid and slow neutron capture elements briefly at the end of this section). We can also focus on a single observed constraint: e.g., dust grains formed in supernova explosions, yields in supernova remnants, and stellar abundances measuring multiple yields. With these observations, we identify the properties of the phenomena and the fundamental physics probed through these observations. In this section, we will limit ourselves to the three observations: dust grains, supernova remnants, and stellar abundances. We will discuss the full system studies needed for each of these observations separately and then show how they all tie together at the end of this section.
2.1 Supernova remnants
Supernova remnants provide an ideal window nucleosynthetic yields which, in turn, will improve our understanding of stellar evolution, supernova explosions and, ultimately, nuclear physics. But the connection between supernova-remnant observation to fundamental physics requires many steps and modeling everything from the stellar evolution through collapse to remnant evolution multiple modeling steps including 3 calculations require high-performance computing. Figure 2 highlights the high-perfomance computing steps.
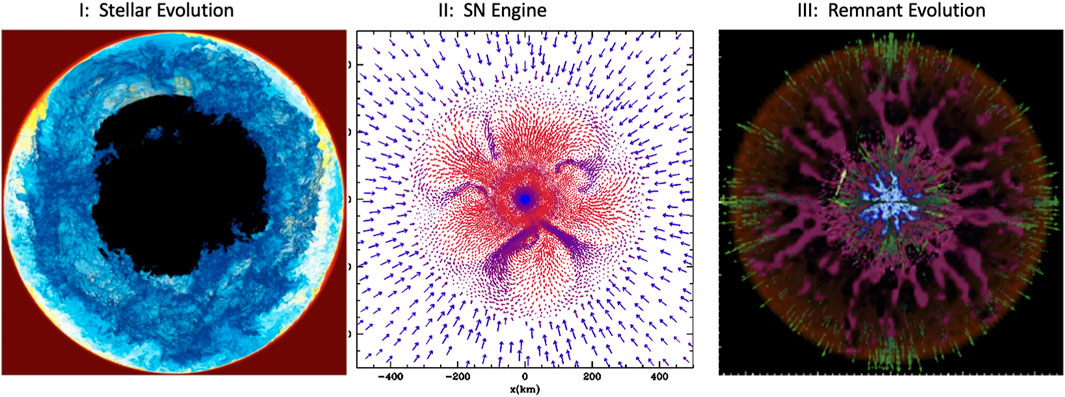
Figure 2. Nucleosynthetic yield simulations from stellar evolution to ejection typically require the combination of a series of high-performance calculations including (from left to right) detailed studies of stellar convection (Herwig et al., 2014), multi-dimensional models of the collapse, convection and explosion of supernovae (Fryer and Warren, 2002) and stellar explosions (Ellinger et al., 2013). Ideally, these simulations are connected not simply by taking the output of one calculation to connect to the other. Instead, the most impactful studies use these calculations to understand the basic physics behind these processes and then use this understanding to guide the next phase of study. This is required of the stellar convection simulations, the results of which must be distilled into a prescription for convection into stellar evolution codes capable of modeling stellar evolution through the evolution of the star which is many orders of magnitude longer than a typical eddy turnover time.
2.1.1 Stellar evolution simulations
Massive stars produce many of the elements up through the iron peak. Stars are powered through a series of nuclear burning phases where the ashes of one phase becomes the fuel of the next. For most stars above
Because of the large timescales in stellar evolution, these stars are modeled implicitly, meaning that the hydrodynamics is not followed in first principles. Instead, much of the physics is modeled either using a sub-grid or approximate method including: sub-grid models to mimic convection, simplified (gray) transport schemes with approximate opacity implementations. But these implementations are guided by high performance computing calculations as shown in Figure 2. Nuclear cross-sections can make a difference in these models. In particular, astronomers have focused on the 12C (α, γ) cross section (Woosley and Heger, 2021). We may be able to probe this physics directly through stellar observations, but most of the constraints arise from then following the collapse of these stars to study their remnants (both the supernova ejecta studied here and the compact remnants—black holes and neutron stars).
2.1.2 Collapse and explosion simulations
As the iron core grows, it contracts in a balance between the thermal and electron supporting the core and gravitational forces compressing it. At some point, the core becomes so dense that electrons begin to capture onto protons (producing a neutron and a neutrino), reducing the electron degeneracy pressure, causing the core to compress further and, ultimately, resulting in a runaway collapse (). This collapse proceeds until the core reaches nuclear densities where neutron degeneracy pressure and nuclear forces halt the collapse, forming a proto-neutron star causing the core to bounce. After the bounce shock fails, a region above the proto-neutron becomes convectively unstable. Neutrinos from the hot proto-neutron star (which continues to grow with convection), deposit energy into the convective region. When this energy is sufficient to overcome the ram pressure of the infalling star, an explosion is launched. The innermost ejecta is heated to extreme temperatures, driving further nuclear burning that produces many of the iron peak elements (as well as intermediate elements such as 44Ti) in the supernovae.
Simulations of this explosion depend detailed hydrodynamics, neutrino transport and neutrino cross-sections, the behavior of matter at nuclear densities and, potentially, magnetic fields (e.g., middle panel of Figure 2). Because the explosion depends sensitively on the strength of the convection, its growth rate is critical. Unfortunately, numerical viscosity (dependent on the resolution of the simulations) is far too high in current simulations to accurately capture this growth rate (Fryer and Young, 2007; Fryer et al., 2021). Indeed, the resolution of all current models are many orders of magnitude too low to capture this growth rate. Without a better physical understanding of the convection, no simulation can make quantitative predictions to compare to data. The computing power needed for such simulations is not obtainable over next century unless some new method (e.g., quantum computing) provides viable. An alternate approach is to better understand the growth of convection and implement this physics using sub-grid models (e.g., Reynolds Averaged Navier Stokes solutions). But, at this time, the current state of these models leads to large uncertainties in the nature of the convection (Fryer et al., 2018; Couch et al., 2020; Fryer et al., 2021). The exact yields in these calculations also rely on the nuclear masses.
2.1.3 Remnant phase and emission simulations
Once the explosion is launched, the supernova blastwave moves out through the star and into the circumstellar medium. As the shock moves through the star, it drives further nuclear burning, further changing the composition of the ejecta. The characterstics of the circumstellar medium (also set by the stellar evolution models) set the deceleration and shocks in the expansion of the supernova blastwave. The reverse shock can then reheat the expanding material, causing it to emit. It is this emission that we observe in the X-ray when observing supernova remnants (Chevalier, 1974).
Our ability to model hydrodynamical effects is critical to tying to the observations. Both the mixing and the propagation of the reverse shock will determine what we observe (right panel of Figure 2). But the observations also depend on the emission properties of the atoms. In supernova remnants, local thermodynamic equilibrium (LTE, a simplifying assumption made in many opacity calculations) is not valid. Inferring abundances from supernova remnants will require detailed models of non-LTE calculations for the emission (Reynolds, 2008). We will discuss this further in Section 4.
The exception to this additional complexity is the observation of radioactive isotopes (e.g., 44Ti). Radioactive isotopes emit through the decay of the isotope [e.g., 44Ti → (Electron Capture) 44Sc → (Electron Capture, β+ decay) 44Ca.]. The decays produce excited nuclear states that produce gamma-rays independent of the whether the material has been shock-heated by the reverse shock. The NuSTAR satellite was able to map the 44Ti distribution by observing 2 of its decay photons (at the edge of NuSTAR’s sensitivity) and this map confirmed that the convective engine was responsible for the explosion producing this remnant (Grefenstette et al., 2017). But, as we can see from the 3 sets of simulations needed to calculate yields (Figure 2, even tying remnant observations of the relatively simple 44Ti emission requires advances in multiple physics (nuclear matter, neutrinos, nuclar cross-sections, turbulence, atomic physics) and modeling methods (sub-grid convection models, transport schemes). This doesn’t even include the fact that additional models are needed to tie the fundamental physics experiments to the theory.
Figure 1 shows how these all fit together: fundamental physics theory models help code-developers derive better nuclear physics calculations and new computational physics algorithms for macroscopic, multi-physics codes. For many nuclear physics experiments, the multi-physics codes are not needed to interpret the data. The experiments both constrain the nuclear physics models (e.g., Hartree-Fock solutions) and can guide the development of nuclear network calculations. In some experiments (e.g., NIF studies of cross-sections for isomeric states), multi-physics codes are needed to analyze the data. Code-developers work with computer scientists/hardware experts to design new algorithms to develop codes both to model these experiments and to model astrophysical observations (often-times, the same code can do both). But these computationally expensive multi-dimensional models are limited in the accuracy of their physics (low resolution, incomplete physics) and the number of calculations. To tie to observations, we must develop a theoretical understanding of the simulations to derive predictions for observations.
For example, based on an understanding of the supernova explosion using multi-dimensional models run on supercomputers, Magkotsios et al. (2010) simulated a series of simplified trajectories to determine how details of the explosion and the uncertainties in nuclear cross-sections can affect the 44Ti yield. With this work, we can study observations, inferring both the asymmetries and strength of the shock from the yields. Further, this work demonstrated that the ratio of 44Ti to 56Ni (which varies dramatically with the details of the explosion shock and mass ejection) could help shed light into the supernova explosion mechanism and, ultimately, the nuclear physics.
Although we learn a great deal 44Ti distribution alone, the ratio with respect to 56Ni can be even further constraints. Unfortunately, we observe 56Fe (the decay product of 56Ni), not 56Ni itself, in supernova remnants and it can be confused with the iron in the star when it first formed (Ellinger et al., 2013): a solar metallicity, 20 M⊙ has
The amount of work needed to do accurate models of the 44Ti production is daunting. Fortunately, if we work with the physics community, astronomy can leverage the work of these physics fields. For example, the fluid dynamics community is actively improving sub-grid models for turbulence, testing their models against a growing list of experiments [e.g., Livescu et al. (2009); Duraisamy et al. (2019)]. In addition, the nuclear physics community is continuously improving the nuclear cross-sections combining nuclear theory and experiment (e.g., for 44Ti Vockenhuber et al., 2008), reducing the uncertainties from nuclear physics.
As complex as it is to study 44Ti, other yields are even more complex. It is difficult to observe stable isotopes unless they are shock heated. And it is even more difficult to get exact abundances without understanding the out-of-equilibrium setting the atomic level-states that determine the observed line-strengths. However, these yields have the potential to provide even greater insight into the explosion and the stellar progenitor (Braun et al., 2023).
2.2 Dust grains
Nucleosynthetic yields, particularly specific isotopes and isotope ratios, can be probed by studying dust grains formed in the supernova ejecta. These grains can be incorporated into meteorites that are then analyzed to determine detailed yields [e.g., Nittler and Ciesla (2016)]. Isotopic ratios are ideally suited to direct comparisons of nuclear cross-sections and dust grains provide a powerful messenger to probe nuclear physics and the supernova engine.
Many studies of dust grains assume the composition of the dust grain can be tied directly to the nucleosynthetic yields in the explosion. But to truly study dust grains, scientists must understand the formation of these grains to better determine the subset of exploding material that is likely to form dust. Although modeling of dust grains is starting to include results of supernova models (Sarangi and Cherchneff, 2013; Sarangi and Cherchneff, 2015; Brooker et al., 2022), advances in both the incorporation of supernova mixing and dust grain production and destruction are needed to tie these observations to the supernova engine.
Not only do these studies rely on much of the physics needed to study yields in supernova remnants, but it also requires a detailed understanding of the materials physics behind dust grain production and formation. This includes detailed density function theory (DFT) models calibrated by detailed molecular dynamics (MD) and, where possible, quantum molecular dynamics (QMD) calculations. Again, fortuanately for astronomy, there have been great advances in this field both in improving the these models (Hickel et al., 2012; Afzalian et al., 2021) leveraging everything from AI to quantum computing. As with supernova remnants, the study of dust is a Herculean effort, but astronomers can make great strides if they work with the materials physics community to improve their models.
2.3 Stellar abundances
The Sloan Digital Sky Survey and the Sloan Extension for Galactic Understanding and Exploration have dramatically increased the spectral observations of stars (Ahumada et al., 2020). With intensive follow-up observations, astronomers have begun to amass a large number of stellar abundance patterns. Combined with models, we can use these patterns to study nucleosynthetic yields and the explosions and fundamental physics that make them.
Unfortunately, these are some of the most indirect observations we will discuss in this paper. Not only do we need to capture all of the physics from a single supernova explosion, but we will need to combine it with stellar populations and galaxy models to determine how these yields mix (over multiple supernovae) into the observed stars. Although these observations and the theory development behind them are important and could provide hints, much more work must be done to infer properties of supernovae, stars or nuclear physics from these observations.
2.4 Benefit of tying it together
All of this data has its limitations: e.g., detailed supernova remnant data or dust grains are limited to a handful of events. Stellar abundances measurements rely on uncertain calculations, but there is much more data. Combining all of this data will help provide a more complete picture of the nature of supernova explosions and the nuclear physics behind nucleosynthetic yields.
2.5 Heavy element production
In this section, we did not discuss the potential to probe slow- and rapid-neutron-capture elements. Tying the observations of these yields to nuclear physics also requires a much better understanding of the processes behind their production, distribution and detection and most studies to date either underestimate or outright ignore the uncertainties in these processes. The first step in moving these studies forward is to identify the physics processes and, as we have done above for supernova yields, identify the dominant uncertainties that must be addressed to advance this field to a more quantitative state. But this discussion is beyond the scope of this paper.
3 Radiation hydrodynamics and shocked emission
Core-collapse supernovae are the observed phenomena from the explosion of a massive star, powered by the potential energy release in the collapse of the core. 99% of this energy (
The evidence that shock heating can be an important component in the observed UV, Optical and IR (UVOIR) emission arises from a number of events that must be understood. For many decades, type II supernova light-curves have been believed to be powered by shock heating that occurs when the supernova propagates through the star. But recent observations of both shock breakout (Alp and Larsson, 2020) and early-time Swift UV emission (Brown et al., 2014) have shown that shock interactions occur as the blast wave pushes through the circumstellar medium. For example, if XMM has truly detected prompt X-rays from type II supernovae (Alp and Larsson, 2020), then it is extremely likely that shock interactions are pumping up the ejecta temperature. In addition, Swift UV observations of the pre-peak supernova emission (Brown et al., 2014) demonstrate that shocks continue to occur driving bright UV long after we’d expect from typical adiabatic cooling. Coupled with the fact that a large fraction of superluminous supernovae are believed to be produced by shock heating and not through the onset of an additional engine (Chatzopoulos et al., 2013). For these supernova, shock interactions must be strong enough to convert up to 10% of the supernova’s kinetic energy into emission.
Most supernova light-curve calculations assume a homologous outflow (without hydrodynamic effects), focusing on the details of radiation transport (e.g., detailed transport methods, atomic physics and out-of-equilibrium effects). Following the format of Figures 1, 3 shows the additional physics studies required to understand shock heating effects in supernovae. The key physics missing in most astrophysics calculations is the coupling of the radiation to the matter and the effects of the matter interactions (i.e., shocks) on the radiation source term.
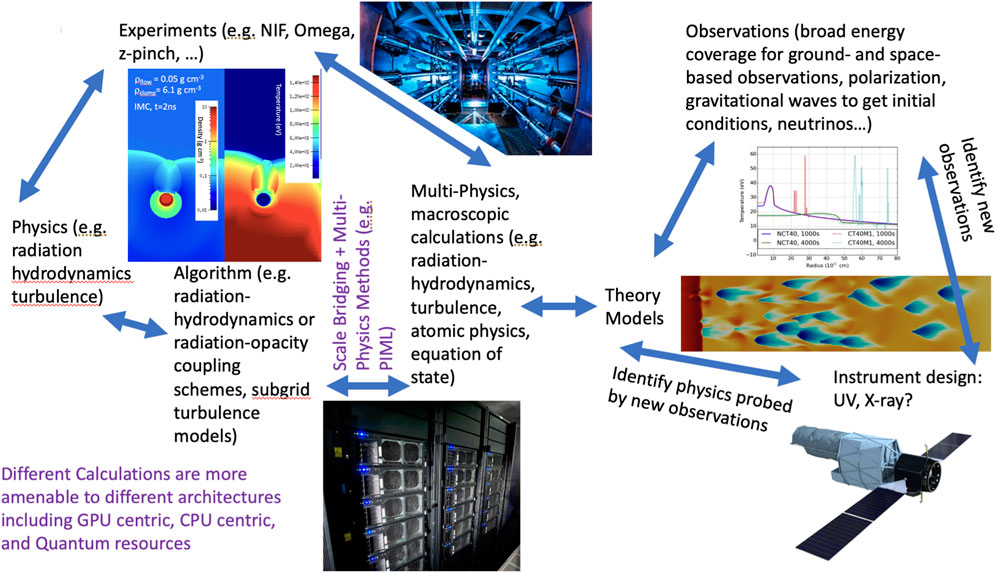
Figure 3. Radiation Hydrodynamics: This diagram shows the physics involved in understanding astrophysical phenomena driven by radiation hydrodynamics using the same format as Figure 1. Radiation-hydrodynamics is a robust field in the high-energy/density physics community and considerable work has been done to both develop improved algorithms and macroscopic models to test these models against laboratory experiment. In astrophysics, this field has been driven both by current astrophysical observations and upcoming NASA missions. This is a case where the instruments are really driving the theoretical and modeling work and advances typically occur to keep up with new observations or experiments. For this problem, the multi-physics models are critical both to interpret the experiments and the observations. Oftentimes this means that the same code tested on a laboratory experiment can be used on the astrophysical phenomenon. The bottom image shows the Roadrunner machine developed at LANL. This machine relied heavily on heterogeneous compute nodes (cell processors), requiring computational scientists to rework the nature of their codes. This heterogeneous hardware persists today where many modern computers combine computational and graphical processing units. Moving clockwise, we show microscopic studies of radiation flow across a clumpy medium that ultimately will be used in interpreting upcoming laboratory experiments (Fryer et al., 2023b) that will be run on the National Ignition Facility (Haynam et al., 2007). The macroscopic codes developed for these experiments have been used for shock breakout calculations in the next two images (Fryer et al., 2020) that will help guide the development of satellties such as UVEX (Kulkarni et al., 2021). The NIF image is adapted from wikipedia (LLNL) ∼ https://en.wikipedia.org/wiki/Creative_Commons. The Roadrunner image is adapted from wikipedia (LANL) ∼ https://commons.wikimedia.org/wiki/File:Roadrunner_supercomputer_HiRes.jpg?uselang=en#Licensing. Unless otherwise indicated, this information has been authored by an employee or employees of the Los Alamos National Security, LLC (LANS), operator of the Los Alamos National Laboratory under Contract No. DE-AC52-06NA25396 with the U.S. Department of Energy. The U.S. Government has rights to use, reproduce, and distribute this information. The public may copy and use this information without charge, provided that this Notice and any statement of authorship are reproduced on all copies. Neither the Government nor LANS makes any warranty, express or implied, or assumes any liability or responsibility for the use of this information.
Radiation-hydrodynamics, including turbulence effects, is an active area of research. For example, the XFlows experiment program at LANL (Johns et al., 2023) is currently running experiments at the National Ignition Facility to study the flow of radiation around an inhomogeneous medium. These experiments are used to validate existing codes and ultimately design subgrid algorithms that can be used in problems where we can not resolve the inhomogeneities (e.g., turbulent flows in shock breakout or early-time supernovae). Here, fundamental physics works tightly with algorithm development to produce codes that simulate the experiments. With the guidance from experimental data, we fine-tune our numerical models that can then be used to model supernova emission. The results of these calculations can, in turn, can be theoretically understood to conduct comparisons with astrophysical observations, further validating the codes (feeding back into the fundamental and algorithmic models of this physics). Ultimately, these calculations can be used to both design and maximize the science for upcoming telescopes and satellite missions (e.g., https://www.ipac.caltech.edu/project/uvex).
4 Plasma physics and spectra
In Section 3 we ignored many of the details of the emission processes. For many calculations, it is assumed that both the electrons and atomic energy states are in thermal equilibrium. Although this is a reasonable assumption at early times in a supernova explosion, the thermalization timescale increases as the ejecta expands and its density, and hence the particle collision rate, decreases. Collisions are what allow particles to reach an equilibrium state and, as the collision rate decreases, the equilibrium assumptions used in many astrophysical studies no longer hold. Unfortunately, Out of equilibrium physics plays an important role in many astrophysical observations. For example, particle acceleration in astrophysical shocks can produce a subset of highly energetic particles that either produce energetic (X-ray, gamma-ray) photons and/or are observed as cosmic rays or high-energy neutrinos. As atoms and their excitation levels fall out of equilibrium, it also becomes increasingly difficult to infer material properties from spectral features. This physics is studied by a broad range of scientists including laboratory experimentalists and space weather physicists. Figure 4 shows the interplay of these fields and how they might improve the astronomy and high-energy astrophysics.
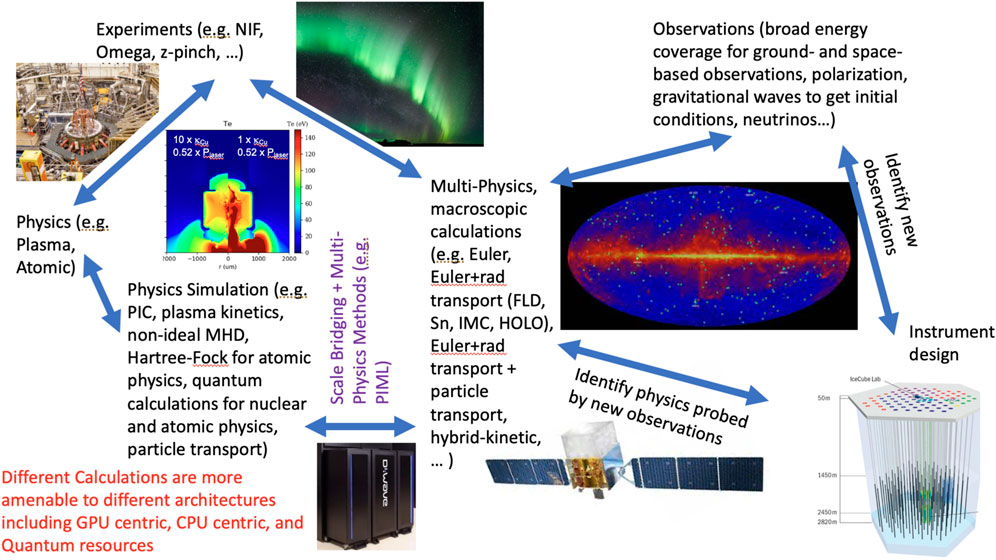
Figure 4. Plasma Physics: This diagram shows how plasma physics affects astrophysical observations following the format of Figure 1. There exist a wide range of problems where the emission arises from nonthermal emission from shock-accelerated electrons including astrophysical jets and supernova remnants, particle acceleration in extreme magnetic fields or particles emitted in the decay of radioactive isotopes. Nonthermal electrons are also produced in solar flairs and there is a broad community of plasma physicists working in heliophysics and space weather. Nonthermal electrons are also produced in the laboratory; for example, in laser-driven experiments. Astronomy can leverage the research in laboratory experiments, fusion science and heliophysics to both better understand the physics and take advantage code developments in plasma physics (as well as an understanding of their numerical limitations). Plasma physics and atomic physics tie together closely and understanding both is essential for astrophysics (for instance late-time spectral features in kilonovae and supernovae). Although much progress has been made to bridge scales between the fundamental plasma and atomic physics and macroscopic calculations, these studies stress computing. It may be that quantum computing can eventually play a role in these studies, but this will require an entirely new style of coding, again testing the expertise of computational scientists. In the bottom left, we show the D-Wave machine (one such quantum computer). Moving clockwise, we show a simulation of a laser-driven hohlraum for experiments at the Rochester Omega facility or the National Ignition Facility, the Fusion Pilot Plant at the Princeton Plasma Physics Laboratory https://www.pppl.gov/ and the aurora borealis (produced from nonthermal electrons from the solar wind interacting with the earth’s magnetosphere—NOAA). Astrophysics sources include pulsar winds, hot stars, and jetted outflows as seen in the Fermi map of the sky (NASA/DOE/Fermi LAT Collaboration). Finally we show images of both the Fermi (Atwood et al., 2009) and the high-energy neutrino IceCube (IceCube Collaboration et al., 2006) telescopes. The D-wave image is adapted from wikipedia (Oleg Alexandrov) ∼ https://creativecommons.org/licenses/by-sa/4.0/.
High-energy astrophysics has suffered the most from not working closely with the physics experts. For example, the cosmic ray field relied too heavily on diffusive particle transport methods, not realizing that particle transport can be strongly affected by magnetic fields (Giacinti and Sigl, 2012; Ahlers, 2014; Fitz Axen et al., 2021). Unfortunately, this lack of understanding led this field to drastically mis-characterize the nature of their observations. Similarly, the simple models used to produce gamma-rays in GRB jets could lead to misleading interpretations of the jet properties. Fortunately, there are an increasing number of particle-in-cell and other plasma kinetic calculations of particle acceleration and astronomers can leverage these codes to model this physics at higher fidelity. But we also need to understand the limitations of these numerical approximations and this is where working closely with the plasma physics community is essential. For instance, many of the early models reduced the ratio of the proton to electron mass to make the simulation more tractable on limited computing resources, but this is known to alter the final electron energy distribution and astrophysics studies are beginning to study these uncertainties in their applications (Rassel et al., 2023).
Further problems lie in simply understanding the origin of the accelerated electrons. Astronomers still rely on simple diffusive shock acceleration models (Reynolds, 2008) despite both plasma theory (Fan et al., 2010) and observations (Grefenstette et al., 2015) suggested this model is insufficient to explain all of the energetic electrons in remnants. Active work is involved to both improve diffusive shock acceleration models. But implementing more sophisticated models is essential to pushing this field forward and working closely with plasma physicists can accelerate this progress.
Fortunately, considerable progress has been made in both the plasma physics and high-energy density/physics fields as well as those of heliophysics and space weather. A growing set of plasma physics codes have been developed and reviews already exist discussing the advantages and disadvantages of the different techniques. Astronomers can leverage this knowledge in their calculations, improving the fidelity of their simulations that will improve the accuracy of the interpretation of astrophysical phenomena.
5 The future of TDAMM
Many of the physics models used in astronomy are derived from an era where simplifications were essential under the current calculational power. But just as computing resources have advanced considerably, so too has the ability to model the fundamental physics. TDAMM astrophysics is pushing astronomy to a regime where including these physics advances is essential to understanding the phenomena we are facing. In some aspects of some problems, astrophysics has advanced more than the field itself, and building these ties will lead to advances on both sides. TDAMM can be the conduit to make these ties.
But the breadth of the connections that must be made to build these ties is enormous. Bringing together these fields is not as simple as bringing together an expert in a particular physics discipline and an astronomer. It requires bringing together experimental, theory and simulation experts in that physics discipline and tying it together with simulation, theory, observation and instrumentation experts in astronomy. And, on the simulation side, you also have to bring in algorithm and hardware experts. Ultimately, we must train scientists that can span all these fields to work with these experts.
Especially in computational physics, astronomy already leads the training of such broad scientists which is why you see astronomers in leading roles in the computer facilities and software development at national laboratories and in industry. Astronomy also has developed scientists that have a broad understanding of physics and experimental/observational uncertainties. But achieving the fidelity needed to drive forward many of the TDAMM goals requires much more continuous connections and will not only require focused funds at NASA for this work but better interagency collaboration.
Probably the most neglected connection lies in Astronomy’s ties to experimental physics. Laboratory astrophysics can not only strive to reproduce astrophysical conditions studying turbulence, radiation flow, dust production, plasma properties and nuclear physics, but because a lot of the observed diagnostics are similar, close collaboration between experimental scientists and astronomy observers could lead to advances in our analysis techniques. For example, experimental scientists are now using spectral diagnostics with increasing frequency and they could definitely benefit from the intense effort astronomers have committed in inferring properties from spectra.
Astronomy has one additional strength to help advance physics: public support. No facility developed in physics has claimed the attention of the public like the launch of JWST, the release of a new Chandra image or the image of the event horizon for the Event Horizon Telescope. The public doesn’t get excited about PREX (Reed et al., 2021) probing neutron skins until they realize that this experiment allows us to study the properties of neutron stars. Astronomy is well known to be the gateway drug into physics, attracting the next-generation of scientists and the public into physics, astronomy and computational modeling. And TDAMM science is at the forefront of this effort.
Author contributions
CF: Conceptualization, Writing–original draft, Writing–review and editing.
Funding
The author(s) declare that financial support was received for the research, authorship, and/or publication of this article. The work by CF was supported by the US Department of Energy through the Los Alamos National Laboratory. Los Alamos National Laboratory is operated by Triad National Security, LLC, for the National Nuclear Security Administration of U.S. Department of Energy (Contract No. 89233218CNA000001).
Acknowledgments
This work is guided by discussions with Eric Burns in organizing the 3rd TDAMM workshop to be held at Louisiana State University September 23-26.
Conflict of interest
The author declares that the research was conducted in the absence of any commercial or financial relationships that could be construed as a potential conflict of interest.
Publisher’s note
All claims expressed in this article are solely those of the authors and do not necessarily represent those of their affiliated organizations, or those of the publisher, the editors and the reviewers. Any product that may be evaluated in this article, or claim that may be made by its manufacturer, is not guaranteed or endorsed by the publisher.
References
Afzalian, A., Akhoundi, E., Gaddemane, G., Duflou, R., and Houssa, M. (2021). Advanced DFT-NEGF transport techniques for novel 2-D material and device exploration including HfS2/WSe2 van der Waals heterojunction TFET and WTe2/WS2 metal/semiconductor contact. IEEE Trans. Electron Devices 68, 5372–5379. doi:10.1109/TED.2021.3078412
Ahlers, M. (2014). Anomalous anisotropies of cosmic rays from turbulent magnetic fields. Phys. Rev. Lett. 112, 021101. doi:10.1103/PhysRevLett.112.021101
Ahumada, R., Allende Prieto, C., Almeida, A., Anders, F., Anderson, S. F., Andrews, B. H., et al. (2020). The 16th data release of the sloan digital sky surveys: first release from the APOGEE-2 southern Survey and full release of eBOSS spectra. Astrophysical J. Suppl. 249, 3. doi:10.3847/1538-4365/ab929e
Alp, D., and Larsson, J. (2020). Blasts from the past: supernova shock breakouts among X-ray transients in the XMM-Newton archive. Astrophysical J. 896, 39. doi:10.3847/1538-4357/ab91ba
Atwood, W. B., Abdo, A. A., Ackermann, M., Althouse, W., Anderson, B., Axelsson, M., et al. (2009). The large area telescope on the Fermi gamma-ray space telescope mission. Astrophysical J. 697, 1071–1102. doi:10.1088/0004-637X/697/2/1071
Braun, C., Safi-Harb, S., Fryer, C. L., and Zhou, P. (2023). Progenitors and explosion properties of supernova remnants hosting central compact objects: II. A global systematic study with a comparison to nucleosynthesis models. Mon. Notices R. Astronomy Soc. 525, 6257–6284. doi:10.1093/mnras/stad2592
Brooker, E. S., Stangl, S. M., Mauney, C. M., and Fryer, C. L. (2022). Dependence of dust formation on the supernova explosion. Astrophysical J. 931, 85. doi:10.3847/1538-4357/ac57c3
Brown, P. J., Breeveld, A. A., Holland, S., Kuin, P., and Pritchard, T. (2014). SOUSA: the Swift optical/ultraviolet supernova archive. Astrophysics Space Sci. 354, 89–96. doi:10.1007/s10509-014-2059-8
Chatzopoulos, E., Wheeler, J. C., Vinko, J., Horvath, Z. L., and Nagy, A. (2013). Analytical light curve models of superluminous supernovae: χ2-minimization of parameter fits. Astrophysical J. 773, 76. doi:10.1088/0004-637X/773/1/76
Chevalier, R. A. (1974). The evolution of supernova remnants. Spherically symmetric models. Astrophysical J. 188, 501–516. doi:10.1086/152740
Couch, S. M., Warren, M. L., and O’Connor, E. P. (2020). Simulating turbulence-aided neutrino-driven core-collapse supernova explosions in one dimension. Astrophysical J. 890, 127. doi:10.3847/1538-4357/ab609e
Duraisamy, K., Iaccarino, G., and Xiao, H. (2019). Turbulence modeling in the age of data. Annu. Rev. Fluid Mech. 51, 357–377. doi:10.1146/annurev-fluid-010518-040547
Ellinger, C. I., Rockefeller, G., Fryer, C. L., Young, P. A., and Park, S. (2013). First simulations of core- collapse supernovae to supernova remnants with SNSPH. arXiv e-prints , arXiv:1305.4137. doi:10.48550/arXiv.1305.4137
Ellinger, C. I., Young, P. A., Fryer, C. L., and Rockefeller, G. (2012). A case study of small-scale structure formation in three-dimensional supernova simulations. Astrophysical J. 755, 160. doi:10.1088/0004-637X/755/2/160
Fan, Z., Liu, S., and Fryer, C. L. (2010). Stochastic electron acceleration in the TeV supernova remnant RX J1713.7-3946: the high-energy cut-off. Mon. Notices R. Astronomy Soc. 406, 1337–1349. doi:10.1111/j.1365-2966.2010.16767.x
Fitz Axen, M., Speicher, J., Hungerford, A., and Fryer, C. L. (2021). Cosmic ray transport in mixed magnetic fields and their role on the observed anisotropies. Mon. Notices R. Astronomy Soc. 500, 3497–3510. doi:10.1093/mnras/staa3500
Fryer, C. L., Andrews, S., Even, W., Heger, A., and Safi-Harb, S. (2018). Parameterizing the supernova engine and its effect on remnants and basic yields. Astrophysical J. 856, 63. doi:10.3847/1538-4357/aaaf6f
Fryer, C. L., Burns, E., Hungerford, A., Safi-Harb, S., Wollaeger, R. T., Miller, R. S., et al. (2023a). Multimessenger diagnostics of the engine behind core-collapse supernovae. Astrophysical J. 956, 19. doi:10.3847/1538-4357/ace0c3
Fryer, C. L., Fontes, C. J., Warsa, J. S., Roming, P. W. A., Coffing, S. X., and Wood, S. R. (2020). The role of inhomogeneities in supernova shock breakout emission. Astrophysical J. 898, 123. doi:10.3847/1538-4357/ab99a7
Fryer, C. L., Karpov, P., and Livescu, D. (2021). Understanding convection in the core-collapse supernovae engine. Astron. Rep. 65, 937–941. doi:10.1134/S1063772921100103
Fryer, C. L., Keiter, P. A., Sharma, V., Leveillee, J., Meyerhofer, D. D., Barnak, D. H., et al. (2023b). Understanding radiation flow in a stochastic medium. arXiv e-prints , arXiv:2312.16677. doi:10.48550/arXiv.2312.16677
Fryer, C. L., and Warren, M. S. (2002). Modeling core-collapse supernovae in three dimensions. Astrophysical J. Lett. 574, L65–L68. doi:10.1086/342258
Fryer, C. L., and Young, P. A. (2007). Late-time convection in the collapse of a 23 msolar star. Astrophysical J. 659, 1438–1448. doi:10.1086/513003
Giacinti, G., and Sigl, G. (2012). Local magnetic turbulence and TeV-PeV cosmic ray anisotropies. Phys. Rev. Lett. 109, 071101. doi:10.1103/PhysRevLett.109.071101
Grefenstette, B. W., Fryer, C. L., Harrison, F. A., Boggs, S. E., DeLaney, T., Laming, J. M., et al. (2017). The distribution of radioactive 44Ti in Cassiopeia A. Astrophysical J. 834, 19. doi:10.3847/1538-4357/834/1/19
Grefenstette, B. W., Reynolds, S. P., Harrison, F. A., Humensky, T. B., Boggs, S. E., Fryer, C. L., et al. (2015). Locating the most energetic electrons in Cassiopeia A. Astrophysical J. 802, 15. doi:10.1088/0004-637X/802/1/15
Harrison, F. A., Craig, W. W., Christensen, F. E., Hailey, C. J., Zhang, W. W., Boggs, S. E., et al. (2013). The nuclear spectroscopic telescope array (NuSTAR) high-energy X-ray mission. Astrophysical J. 770, 103. doi:10.1088/0004-637X/770/2/103
Haynam, C. A., Wegner, P. J., Auerbach, J. M., Bowers, M. W., Dixit, S. N., Erbert, G. V., et al. (2007). National Ignition Facility laser performance status. Astrophys. Opt. 46, 3276–3303. doi:10.1364/AO.46.003276
Herant, M., Benz, W., Hix, W. R., Fryer, C. L., and Colgate, S. A. (1994). Inside the supernova: a powerful convective engine. Astrophysical J. 435, 339. doi:10.1086/174817
Herwig, F., Woodward, P. R., Lin, P.-H., Knox, M., and Fryer, C. (2014). Global non-spherical oscillations in three-dimensional 4π simulations of the H-ingestion flash. Astrophysical J. Lett. 792, L3. doi:10.1088/2041-8205/792/1/L3
Hickel, T., Grabowski, B., Körmann, F., and Neugebauer, J. (2012). Advancing density functional theory to finite temperatures: methods and applications in steel design. J. Phys. Condens. Matter 24, 053202. doi:10.1088/0953-8984/24/5/053202
Hungerford, A. L., Fryer, C. L., and Warren, M. S. (2003). Gamma-ray lines from asymmetric supernovae. Astrophysical J. 594, 390–403. doi:10.1086/376776
Hwang, U., Laming, J. M., Badenes, C., Berendse, F., Blondin, J., Cioffi, D., et al. (2004). A million second Chandra view of Cassiopeia A. Astrophysical J. Lett. 615, L117–L120. doi:10.1086/426186
IceCube Collaboration Achterberg, A., Ackermann, M., Adams, J., Ahrens, J., Andeen, K., et al. (2006). First year performance of the IceCube neutrino telescope. Astropart. Phys. 26, 155–173. doi:10.1016/j.astropartphys.2006.06.007
Johns, H. M., Byvank, T., Robey, H., Urbatsch, T., Coffing, S., Fryer, C. L., et al. (2023). Roadmap for the exposé of radiation flows (Xflows) experiment on NIF. Rev. Sci. Instrum. 94, 023502. doi:10.1063/5.0101421
Kulkarni, S. R., Harrison, F. A., Grefenstette, B. W., Earnshaw, H. P., Andreoni, I., Berg, D. A., et al. (2021). Science with the ultraviolet explorer (UVEX). arXiv e-prints , arXiv:2111.15608. doi:10.48550/arXiv.2111.15608
Livescu, D., Ristorcelli, J. R., Gore, R. A., Dean, S. H., Cabot, W. H., and Cook, A. W. (2009). High-Reynolds number Rayleigh-Taylor turbulence. J. Turbul. 10, 13. doi:10.1080/14685240902870448
Magkotsios, G., Timmes, F. X., Hungerford, A. L., Fryer, C. L., Young, P. A., and Wiescher, M. (2010). Trends in 44Ti and 56Ni from core-collapse supernovae. Astrophysical J. Suppl. 191, 66–95. doi:10.1088/0067-0049/191/1/66
Milisavljevic, D., Temim, T., De Looze, I., Dickinson, D., Laming, J. M., Fesen, R., et al. (2024). A JWST Survey of the supernova remnant Cassiopeia A. arXiv e-prints , arXiv:2401.02477. doi:10.48550/arXiv.2401.02477
Nittler, L. R., and Ciesla, F. (2016). Astrophysics with extraterrestrial materials. ARA&A 54, 53–93. doi:10.1146/annurev-astro-082214-122505
Rassel, M., Kilian, P., Aberham, V., Spanier, F., Lloyd-Ronning, N., and Fryer, C. L. (2023). Particle-in-cell simulations of mildly relativistic outflows in kilonova emissions. Astrophysical J. 952, 165. doi:10.3847/1538-4357/acd76a
Reed, B. T., Fattoyev, F. J., Horowitz, C. J., and Piekarewicz, J. (2021). Implications of PREX-2 on the equation of state of neutron-rich matter. Phys. Rev. Lett. 126, 172503. doi:10.1103/PhysRevLett.126.172503
Reynolds, S. P. (2008). Supernova remnants at high energy. ARA&A 46, 89–126. doi:10.1146/annurev.astro.46.060407.145237
Sarangi, A., and Cherchneff, I. (2013). The chemically controlled synthesis of dust in type II-P supernovae. Astrophysical J. 776, 107. doi:10.1088/0004-637X/776/2/107
Sarangi, A., and Cherchneff, I. (2015). Condensation of dust in the ejecta of Type II-P supernovae. Astronomy Astrophysics 575, A95. doi:10.1051/0004-6361/201424969
Vockenhuber, C., Ouellet, C. O., The, L. S., Buchmann, L., Caggiano, J., Chen, A. A., et al. (2008). 40Ca(α,γ)44Ti and the production of 44Ti in supernovae. J. Phys. G Nucl. Phys. 35, 014034. doi:10.1088/0954-3899/35/1/014034
Warren, M. S., Fryer, C. L., and Goda, M. P. (2003). “The space simulator: modeling the universe from supernovae to cosmology,” in ACM/IEEE SC 2003 Conference (SC’03), 30.
Woosley, S. E., and Heger, A. (2021). The pair-instability mass gap for black holes. Astrophysical J. Lett. 912, L31. doi:10.3847/2041-8213/abf2c4
Keywords: supernova, nuclear physics, plasma physics, radiation transport, atomic physics
Citation: Fryer C (2024) Fundamental physics studies in time domain and multi-messenger astronomy. Front. Astron. Space Sci. 11:1384587. doi: 10.3389/fspas.2024.1384587
Received: 09 February 2024; Accepted: 02 April 2024;
Published: 16 April 2024.
Edited by:
Rita Sambruna, National Aeronautics and Space Administration, United StatesReviewed by:
Chris Reynolds, University of Maryland, United StatesCopyright © 2024 Fryer. This is an open-access article distributed under the terms of the Creative Commons Attribution License (CC BY). The use, distribution or reproduction in other forums is permitted, provided the original author(s) and the copyright owner(s) are credited and that the original publication in this journal is cited, in accordance with accepted academic practice. No use, distribution or reproduction is permitted which does not comply with these terms.
*Correspondence: Chris Fryer, fryer@lanl.gov