Remediation of heavy metal(loid) contaminated soil through green nanotechnology
- Plant Biotechnology Lab, Department of Botany, Faculty of Science, Dayalbagh Educational Institute, Agra, India
Modern industrialization is progressively degrading soil quality due to heavy metal contamination. Heavy metal (HM) contamination of agricultural soil has gained considerable attention due to its rapidly increasing levels. Nanoparticles (NPs) have unique physicochemical properties that make them effective stress relievers. Material science has recently been emphasizing “green” synthesis as a reliable, environmentally friendly, and sustainable method of synthesizing different kinds of materials, such as alloys, metal oxides, hybrids, and bioinspired materials. Therefore, green synthesis can be viewed as an effective tool to reduce the detrimental effects of the traditional nanoparticle synthesis methods commonly used in laboratories and industries. The review briefly describes the biosynthesis of NPs, the use of nanobiotechnology to remediate heavy metal-contaminated soil, the effect that NPs have on growth and development of plants, the behavior of NPs within plants when exposed to pollutants and the mechanisms used to alleviate HM stress. In addition, a broad overview of the major types of nanomaterials used so far in bioremediation of toxic heavy materials, recent advances regarding HM stress and the possible mechanisms by which NPs and HM interact in the agricultural system are also discussed.
Introduction
Soil is an indispensable part of our ecosystem supporting all life forms, aquatic as well as terrestrial. It is a pre requisite for overall development of plant and crop cultivation. Often, “soil” and “land” are used interchangeably but it is important to understand that land is much more than just soil and soil is a significant as well as fundamental unit of land (Bakshi and Abhilash, 2020). Soil health plays a key role in regulating planetary processes and safeguarding crop productions. However, past few decades have witnessed degradation in soil health due to accelerated industrialization, extensive use of chemical fertilizers in agricultural practices in order to meet the growing population demand, anthropogenic activities, and environmental pollution (Lal, 2015). The contaminants that are associated with public and ecological health interest are pesticides, greenhouse gases, toxic heavy metal(loid) (HM), hydrocarbons, and nuclear residues. The major concern regarding these contaminants is that they are persistent, non-biodegradable and toxic in nature (Amin et al., 2021). Among these the notable contaminant rising exponentially all across the globe are heavy metal (loid), which negatively affect human health. Therefore, the emerging challenge around the planet is the food security and crop productivity especially in the countries with huge population (Rai et al., 2019).
Elements with density higher than 5 g/cm3 and atomic mass greater than (>20) are grouped as heavy metals. They are the natural constituent of the soil that originate from the weathering of parent material of soil, called “Paedogenesis.” While occurring naturally, the concentration of these HM in the soil are low which increases gradually (Bakshi and Abhilash, 2020). This increase is attributed to various anthropogenic activities such as dumping the waste of textile mills, tanneries, metal mining industries, pulp and paper industries in landfills and releasing the industrial effluents in the water bodies which further leaches and accumulate in soil (Fayiga and Saha, 2016; Rai et al., 2019). Due to the combustion of the fossil fuels aerosols are formed which play a major role in the HM accumulation (Bhuiyan et al., 2021). HM such as Pb (lead), Cr (chromium), As (arsenic), Zn (zinc), Cd (cadmium), Cu (copper), Hg (mercury) and Ni (nickel) are considered highly toxic, whereas Ba (barium), Sb (Antimony), Mo (molybdenum), Tl (Thallium), Sn (tin) are considered as less toxic than others (Bakshi and Abhilash, 2020).
The presence of HM in the soil has a negative impact on the plant as well as the microbiome associated with it. The stress generated by them lead to the formation of ROS (Reactive Oxygen Species) which in turn damages the essential macro-molecules inside the organism, affecting the nutrition quality and ecology of the vegetation as well as crops. For example, by amending Cu-contaminated soil with natural soil, it can disturb normal growth processes in plants by adversely affecting biochemical reactions and physiological processes (Saleem et al., 2020a). The damage caused by HM varies according to the plant species, type of heavy metal and physio-chemical properties (Chaplygin et al., 2021). Some studies also suggest that the HM contaminated soil hampers various biological process of plants and microflora including their diversity, abundance and genetic makeup (Rajput et al., 2022). The enzymatic activities, biomass, crop yield is reduced whereas oxidative stress caused by ROS is elevated (Huang et al., 2019). Stabilization of HMs at a specific site using site stabilization can help reduce their mobility, availability, and leaching at distant sites, thereby halting the spread of HMs (Suman et al., 2018). Nano-remediation has gained much attention in recent times, with a major potential to protect the planet from pollution and clean up contaminated sites. Enhancement of the microbial activities by involving the use of nanoparticles to eradicate the toxic contaminants is termed as “Nano-bioremediation” (Amin et al., 2021). One of the major advantages of this technology is that it is effective in deep soils and could work well along with other techniques like bioremediation. In addition to decreasing the costs of cleaning up polluted sites, nanotechnology also manages to decrease the progress time (Benjamin et al., 2019). The current review emphasized on the role of green nanotechnology in remediating heavy metal(loid) contaminated soil, biosynthesis of bio nanoparticles and different biogenic remediation techniques with their impact on environment.
Bio nanoparticles and their synthesis
Recent decades have witnessed the development of novel approaches for nanomaterial synthesis. Two primary principles are followed for the formation of the nanomaterial of desired shape, size, and function, namely bottom-up and top-down approaches (Figure 1). In bottom-up method nanoparticles are developed from smaller molecules. It also includes approaches like sol-gel processes, laser pyrolysis, chemical vapor deposition etc. Moreover, the top-down method includes the formation of nanoparticles from wide range of techniques such as sputtering, lithographic technique etc. (Kandiah and Chandrasekaran, 2021).
Although in the last few years number of nanoparticles were developed using conventional top- down and bottom-up approaches but only few of them are currently used as they are produced from toxic chemicals and their by-products becomes a matter of serious concern to mankind. Moreover, the insufficient knowledge about their toxicity and issues related to human health does not allow the use of these nanoparticles in agricultural fields. To overcome this limitation “green synthesis” approach is being used extensively nowadays. In green synthesis the biologically originated extracts such as that of plants and microbes are used in the formation of nanoparticles (Andrade-Zavaleta et al., 2022). Synthesis of nanoparticles from plant derived extract is cost effective, and favorable as compared to microorganisms. In addition to being challenging to isolate, microbes can pose bio-hazards and are more susceptible to contamination. The following steps shows the green synthesis of nanoparticle (Singh et al., 2018).
a) Preparation of plant or microbe derived extracts and mixing it with metal salt solution.
b) Fabrication and biochemical reduction of the metal solution. In this process, the monovalent/divalent metal ions are converted into zero-valent states that are then grown and nucleated into nanoparticles.
c) Analysis of the formed nanoparticles through UV-VIS Spectroscopy.
d) Characterization of nanoparticles through TEM, SEM, XRD etc. and application in different sectors.
Fundamentally, the green synthesis of materials/ nanomaterials, produced through cleanup, regulation, remediation, and control will directly enhance their environmental affability. In essence, “green synthesis” can be summarized by several basic principles, such as waste prevention/minimization, lowering of derivatives/pollution, and using safer (or nontoxic) solvents and auxiliaries, along with renewable feedstocks. By implementing eco-friendly and environmentally-responsible synthesis procedures, it is possible to avoid the generation of unwanted byproducts (Singh et al., 2018; Kandiah and Chandrasekaran, 2021).
Mechanism of action of nanoparticles to alleviate the heavy metal stress
Several studies have been made to understand the underlying mechanism of action of nanoparticles in order to alleviate the HM stress. The effects of HM stress have been found to influence plant physiology, morphology, and biochemistry. Certain reports suggested that the NPs that regulates the plant growth could be harmful to them at higher concentrations (Vishwakarma et al., 2020). In order to overcome HM stress in plants, various approaches have been used, such as lowering the bioavailable HM concentration in the soil (Moharem et al., 2019), improving the plant defense system, enhancing the physiological functions, development of protective agents and heavy metal transport gene expression regulation (Cao et al., 2020a). NPs enters the cells either through channels of the plasma membrane or through penetration. These NPs acts as signal molecules which induces the genes of stress signaling thus activating the plant defense system. NPs transforms or absorbs the HMs present in the soil therefore minimizing their bioavailability as well as their mobility. For instance, the mobility of cadmium was reported to be decreased with the application of Fe3O4 NPs (Sebastian et al., 2019). Cadmium can be converted into much stable forms under the influence of mercapto Si NPs (Wang et al., 2020).
In the cell wall of the plant nanoparticles makes complexes after binding with the heavy metals, thus, making them biologically unavailable. The complex is then adsorbed on the plant cell surface which hinders their migration all across the plant therefore minimizing their biological activities (Wang et al., 2021). Additionally, the damage caused by the HM stress in plants can be reduced to some degree by chelating organic acids accumulated in their cell walls. NPs have been shown to increase the production of protective agents (Zhou et al., 2020).
Plant oxidation defenses can also be activated to alleviate HM stress (Figure 2). Biochemical reactions in plants produce ROS as a by-product (Wu et al., 2017). For instance, ROS are continuously formed in chloroplast or during photosynthesis and respiration. These reactive species, at lower concentrations acts as signaling molecules for growth, defense, and development. However, in stressed conditions ROS accumulates in higher concentration which in turn damages the proteins, cell membranes and other components of the cell (Wu et al., 2017). Antioxidant enzymes such as peroxidase (POD), superoxide dismutase (SOD), ascorbate peroxidase (APX), catalase (CAT), glutathione peroxidase (GPX), and glutathione reductase (GR) are mainly responsible for maintaining the ROS levels in the cells and hence they scavenge these reactive species when they are present in high level. Low molecular weight, non-enzymatic metabolites (polyphenols, Vitamin E, and Vitamin C) also possess the ability to degrade the ROS (Ullah et al., 2020; Zhao et al., 2020). In response to stress, ROS clearance pathways are triggered. Taking into consideration the biosynthesis of shikimate-phenylpropanoid and alanine, galactose, ascorbate, and aspartic acid metabolism can help plants recover from oxidative stress (Hussain et al., 2020). Plants using antioxidant enzyme-activated NPs (CeO2 NPs and Mn3O4 NPs and Fe3O4 NPs) can reduce ROS, which will ultimately reduce the effects of crop production losses (Konate et al., 2017; Yao et al., 2018).
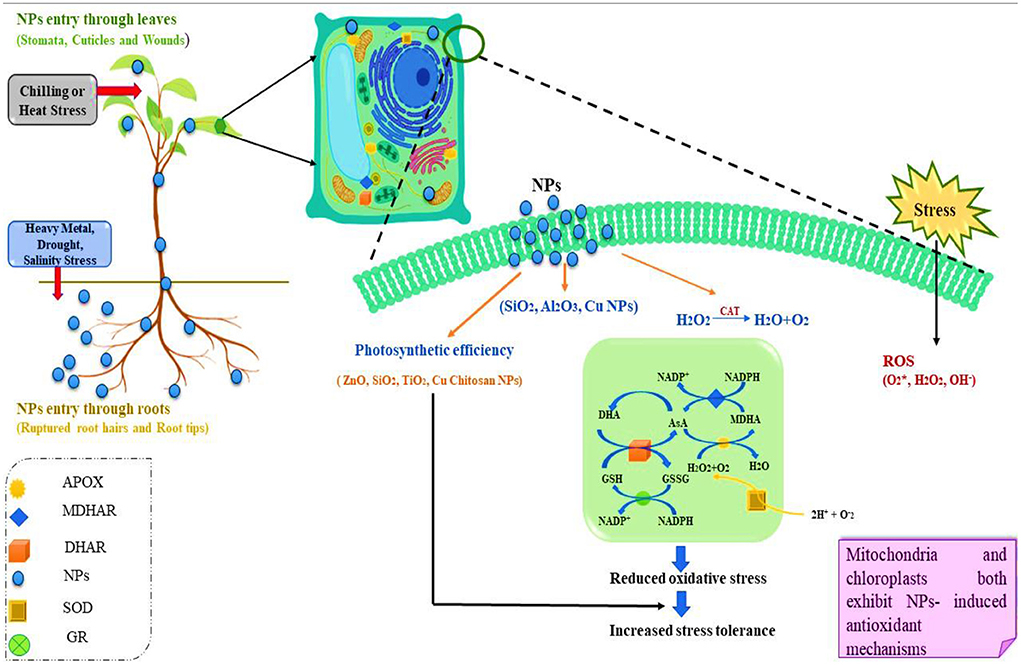
Figure 2. Pictorial illustration of the mechanism involved in remediating HM contaminated soil. The presence of NPs enhances the adsorption of HM by rising the soil pH reducing the bioavailability and mobility of HM in soil. Nanoparticles can help in stimulating the defense cascade in plants by synthesizing the ROS which promote the ability of the plant for increased nutritional uptake and improving plant growth (APOX, ascorbate peroxidase; MDHAR, monodehydroascorbate reductase; DHAR, dehydro-ascorbate reductase; NPs, nanoparticles; SOD, superoxide dismutase; GR, glutathione reductase; ROS, reactive oxygen species).
Contribution of nanotechnology for remediating heavy metal contaminated soil
Nano-bioremediation is the process of removing contaminants by greenly synthesized nanomaterials/nanoparticles (Amin et al., 2021). It is a low-cost method of breaking down toxic chemicals using plants and microbes for reducing pollution and improving soil quality. The procedure can eliminate, reduce, or retain the number of pollutants existing in the soil by contaminants breakdown (Ashraf et al., 2019). Bioremediation effectiveness has been examined and improved using biotechnology (Gerhardt et al., 2017) however, nanotechnology has added a new dimension to the process (Gong et al., 2018). For instance, nanotechnology-based phytoremediation boosts the effectiveness of phytoremediation by allowing nanoparticles to be used to clean soil, water that contains heavy metals, and organic matter contaminated with pollutants (Pillai and Kottekottil, 2016; Awasthi et al., 2022). NPs have distinct physico-chemical properties that serve as catalyst and help to reduce the activation energy necessary for breaking down the compounds, causing the targeted pollutants to be degraded, adsorbated, or altered. There have been many studies and examinations of nano-bioremediation, using metal- and carbon-based nanoparticles as the most common materials (Chen et al., 2017a). Polymeric nanoparticles as nano capsules or nanospheres can also effectively remove hydrocarbons and pesticides (Chauhan et al., 2020). However, HM provide a unique challenge since they are non-biodegradable and very susceptible to infiltrating food chains and biological systems that are still required to be understood for their effective removal (Briffa et al., 2020). Nanobioremediation covers various techniques and devices such as nanobiosensors, nanosorbents, nanobiosurfactants, and nanophytoremediation which are discussed below in detail. However, the role of different nanomaterials in heavy metal stress alleviation of contaminated soil is discussed in Table 1.
Nanobiosensors
Nanobiosensors are modified biosensors that consist of biological elements which are linked with a transducer, usually made on nanoscale for the acquire, process and scrutinize data at atomic level (Rai et al., 2012; Ghaffar et al., 2020). The analytes are detected at very low concentrations through a physicochemical transducer that is more compact and linked to sensitized elements (Scognamiglio et al., 2013). Nano biosensors have several intriguing features, such as fictionalization, immobilization, and miniaturization, which increase the analytical performance of NMs by integrating bio components of a system into complex structures (Arduini et al., 2016; Usman et al., 2020). Nanobiosensors have shown great advantage over traditional biosensors as they exhibit increased specificity of detection and have applications in various fields such as soil nutrient monitoring, bioprocessing, environment control, and agriculture (Mandal et al., 2020). By managing water, land, fertilizers, and pesticides in a rational way, nano-biosensor technology can boost crop yields through early detection and rapid decision-making.
Heavy metals (such as mercury, cadmium, lead, arsenic,) are considered as one of the most hazardous substance that is disturbing the environment. They may accumulate in the crops which will further affect the plants and the human population consuming these plants. They can be detected using nanomaterials synthesized by biochemical and green synthesis techniques. A variety of nanomaterials (nanoclusters, antibodies, aptamers) and nanodevices (FRET or fluorescence resonance energy transfer, quantum dots and cantilever biosensors) are recently being used for the detection of heavy metals in water and soil (Saha and Bhaduri, 2021). By using nanobiosensors heavy metal phytotoxicity can be detected by measuring the chlorophyll content, plant water status etc.
Arsenic is one of the heavy metal contaminants posing major health issues to creatures residing the ecosystem. Lew and hi coworkers have studied the natural arsenic accumulation ability of the plant Pteris cretica with the use of nanobionic sensors that were embedded in the plant tissue (Lew et al., 2021). The nanobionic sensor can detect the real time changes occurring inside the plant during the uptake of the heavy metal. The study showed that the nanobionic sensors were able to detect the arsenic levels from 0.6 to 0.2 ppb after 2 weeks. Although the role of nanobiosensors in remediating the heavy metal contaminated soil is not well known but their role in detecting the heavy metal in water and soil are reported in several recent studies (Rigo et al., 2020a,b; Ballen et al., 2021).
Nanobiosorbents
The elimination of organic and inorganic matter with the help of biologically derived material is known as “biosorption” (Velkova et al., 2018). Whereas, the degradation of the pollutants through biosorption in combination with nanotechnology is referred to as “Nanobiosorbents.” It is one of the recent techniques that majorly focus on the HM removal from soil and water. Nanobiosorbents are sustainable, eco-friendly, greener, economical, and renewable materials that are widely exploited for their unique pollutant removal properties. The presence of -COOH (carboxyl) and -OH (hydroxyl) groups is a pre requisite for a biosorbent for adsorption of pollutants, heavy metals, radionuclides and dyes (Rizwan et al., 2022).
Waste products such as rice husk, bagasse, fly ash, and spent microorganisms have a high affinity to act as nanosorbents or biosorbents. For example, the formation of graphene Quantum Dots with rice husk as biosorbent for the removal of La (III) and Pb (II) (Mahmoud et al., 2020). Moreover, cellulosic material, starch, alginates, gums, pectin, chitin, and others can also act as a potent material for the formation of nanobiosorbents (Khan et al., 2022). Although several studies are reported till now on the role of nanobiosorbents in eliminating heavy metals from the contaminated soils, but they found a great application in the removal of contaminants from wastewater (Yadav et al., 2020).
Nanobiosurfactants
Biosurfactants are molecules produced either on living spaces or secreted by microbes extracellularly. These molecules exhibit lipophilic as well as hydrophilic activities and reduces the surface tension. In sustainable chemistry, biosurfactants emerged as ecofriendly and promising source for synthesis of nanoparticles (Debnath et al., 2021). Production of biosurfactants from microbes are often used for the heavy metal remediation like zinc, copper and nickel. The interaction between nano zirconia and rhamnolipid (glycolipid biosurfactant) was studied by Biswas and Raichur (2008). The study reveals the presence of high electronegativity interaction on the surface of nano zirconia with rhamnolipid. The ability of rhamnolipid to act as foaming agent, dispersant, and its ability to reduce toxicity made it a potent nano biosurfactant for alleviation of heavy metal stress. Soil with HM and hydrocarbon contamination was effectively remediated with the use of lipopeptide biosurfactant formed from Bacillus subtilis (Reddy et al., 2009).
Nanoparticles (NP) capped with biosurfactant stabilizes the complex and play a key role in heavy metal bioremediation. Sophorolipids (glycolipids) are produced by several non-pathogenic yeasts. NP capped with sophorolipids are synthesized by Starmerella bombicola which has a diameter of about 50 nm (Kasture et al., 2008; Sarubbo et al., 2022). The nanoparticles of zinc sulfide when capped with Rhamnolipid (synthesized by Pseudomonas aeruginosa) resulted in the formation of 4.5 nm of NPs. Stabilization of the NP and bioremediating heavy metals can be achieved with capping. The rhamnolipid (Biosurfactant) when capped with ZnS then NPs have the potential to escalate the decolorization and degradation of dyes used in textile industry. The rhamnolipids produced by P. aeruginosa BS01 are also used in the synthesis of ZnS nanoparticles to act as photocatalyst (Hazra et al., 2013).
Nanophytoremediation
Biotechnological technique that utilizes the ability of the plant to improve the environment is referred as “phytoremediation.” Recent studies suggest that plants have potential to degrade and eliminate HM contaminants such as sediment, soil, water and beyond. Phytoremediation is considered more expedient than conventional technologies due to its simplicity, sustainability, and compatibility with the surroundings (Awasthi et al., 2022). In addition to plants, the rhizospheric microbiome also plays an essential role in cleaning up these contaminated resources. Plants and microbes utilize the same phytoremediation principles found in nature for reducing organic and inorganic pollutants (Bakshi and Abhilash, 2020). Many phytoremediation techniques for alleviating soil pollution includes phytodegradation, phytovolatilization, rhizodegradation, and phytoextraction. Bioremediation is anticipated to provide a variety of benefits, including production of fiber or biofuel, soil organic carbon sequestration, soil stabilization, and microbial activity stimulation (Abhilash et al., 2009; Ali et al., 2013). The major drawback of phytoremediation is that it typically takes years, or sometimes even decades, to produce positive results. Furthermore, the application of this technique is restricted by soil quality, pollutant phytotoxicity, and weather conditions (Song et al., 2019). In recent years, phytoremediation has gained popularity due to its enhanced efficiency and cost-effectiveness when combined with other technologies like nanotechnology and microbial bioremediation (Gerhardt et al., 2017).
The rapid action and on-site remediation of nanotechnology are gaining acceptance as an effective and reasonable way to remove soil pollutants. As part of nano-phytoremediation, phytotechnology, and nanotechnology are used to remove contaminants. Most research on nanomaterials has been conducted on carbon-based materials, followed by metal oxides and metal nanomaterials (Chen et al., 2017b). Considering wheat plants grown in cadmium-contaminated soil, Hussain et al. (2019) investigated the possible role of Fe NPs (iron oxide nanoparticles). Addition of Fe nanoparticles at 20 ppm reduced the adverse effects of Cd by increasing Fe uptake, plant growth rate, antioxidant enzymes, and photosynthetic activity thereby lowering the plant cadmium concentration. Exogenous approach for applying Fe NPs has improved wheat plant morphology such as dry biomass of roots, shoots, grains and spike husks as well as photosynthetic pigments. Wang et al. (2014) reported chromium contaminated soil remediation using CMC-stabilized nZVI and observed that it significantly improved chromium immobilization by lowering the bio accessibility and leachability by 58 and 82%, respectively.
Nanomaterials (NMs) can considerably alleviate heavy metal stress through phytoremediation. Flax (Linum usitatissimum) is capable of removing large quantities of Cu from soils and can be grown as flax seed and is therefore a possible candidate for phytoremediation of Cu (Saleem et al., 2020b). The effect of TiO2 (nano-titanium dioxide) on Glycine max plant Cd accumulation from the soil was reported (Singh and Lee, 2016; Usman et al., 2020). By shielding plants from oxidative damage and scavenging free radicals generated by Cd toxicity, TiO2 decreased Cd toxicity, and increased Cd absorption in soybean plants. In a field experiment, Liang and his coworkers investigated the combined influence of NHAP (nano-hydroxyapatite) and NCB (nano-carbon black) on phytoextraction of lead by Lolium temulentum L. (ryegrass) from the soil as an improved technique of remediation. NCB or NHAP considerably reduced Pb phytotoxicity in ryegrass and boosted the ryegrass phytoextraction capacity. NHAP was identified as much suited than NCB for in situ treatment of Pb-contaminated soils (Liang et al., 2017). Plant metal toxicity can be reduced by using nanomaterials to regulate related gene expressions linked to water homeostasis, metal stress, cell wall production, oxidative stress, cell division, and photosynthetic processes (Gopalakrishnan Nair and Chung, 2014). According to a study, NMs can promote heavy metal accumulation in plants by regulating cell wall permeability, heavy metal co-transportation and transporter gene expression with NMs. Consequently, unfavorable effects of NMs on efficiency of phytoremediation have been found (Hu et al., 2014). For instance, the integrated effects of Cd (cadmium) and GO (graphene oxide) exacerbated Cd toxicity by increasing ROS production in Microcystis aeruginosa (Tang et al., 2015). It can also be concluded that various NMs affect heavy metal absorption or toxicity in various plant species in different ways (Gong et al., 2018).
Biogenic remediation
Plant and microbe derived
Phyto nanotechnology is considered as an eco-friendly, simple, quick, stable, and cost-effective approach for the production of nanoparticles and has recently opened up new paths for alleviating the HM stress associated with the soil. Biocompatibility, adaptability and the pharmaceutical use of integrating nanoparticles using water, as a reducing medium are all included in the benefits of phyto nanotechnology. In this approach, plant-derived nanoparticles are made from readily available plant components and the non-toxic nature of plants made them suitable for meeting all rising demands for nanoparticles with biomedical and environmental applications. Using leaf and root extracts from the therapeutic herbal plant Panax ginseng, gold and silver nanoparticles were successfully combined recently (Singh et al., 2016). In addition, many other plants have been employed to create metal nanoparticles.
The use of microorganisms such as fungus, yeast, actinomycetes, and bacteria for the production of nanoparticles has been explained in Figure 3 (Huang et al., 2019). Fungi have recently emerged as the most important source for the production of nanoparticles. FeO nanoparticles coated with polyvinylpyrrolidone (PVP) have been extensively applied by Gram-negative bacteria, Halomonas sp., to improve bioremediation of land polluted with Pb and Cd. Compared to bacteria or just NPs, this method eliminated almost 100% of Pb after 24 h and ~100% of Cd after 48 h (Alabresm et al., 2018). For the removal of Cu, Ni, and Pb, a biosorbent of magnetic Fe3O4 NPs treated with S. aureus with a surface coated with phthalic acid (nFe3O4PhthS) was utilized with adsorptive removal of 795, 1,355, and 985 mol g1 for Cu, Pb, and Ni, respectively. Cu2+ recovery was 83.0–89.5 per cent, Pb2+ recovery rates were 99.4–100 per cent, and Ni2+ recovery rates were 92.6–7.5 per cent. The nFe3O4PhthS core of the NPs and the functional groups present on the microbial surface played a crucial role in the elimination of HMs in a comparison investigation with dried S. aureus and nFe3O4PhthS (Mahmoud et al., 2016). As a result of this research, it was discovered that the core of the NPs and functional groups found on the microbial membrane had a significant influence on the pollutants removal. Recent research on the reduction of Cu, Cd, Cr, and Pb using HM resistant bacteria such as B. cereus (PMBL3) and L. macrolides (PMBL7) confirmed that ZnO NPs at 5 mg L−1 synergistically remove the Cr by 60%, the Cu by 70%, and the Pb by 85%, as compared to B. cereus (80 and 60%) and L. macroides (55 and 50%) at neutral pH, respectively (Baragaño et al., 2020).
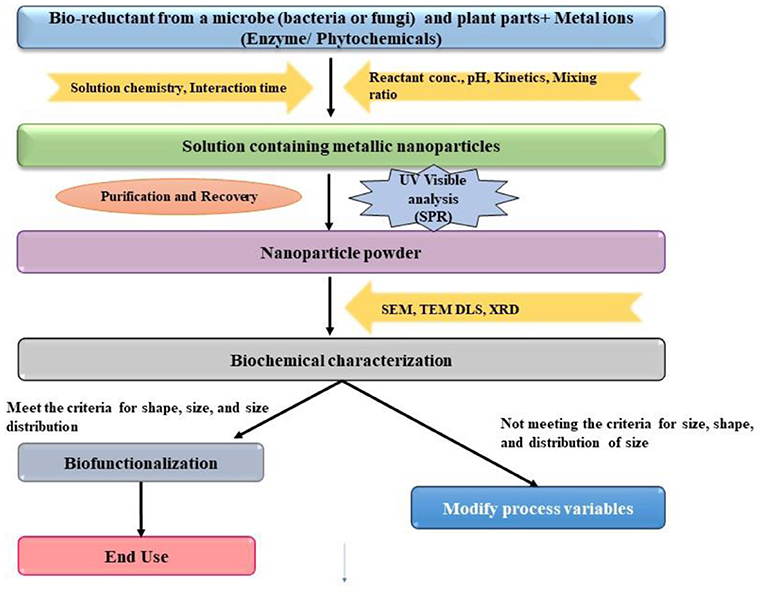
Figure 3. Biosynthesis of microbe-based nanoparticles. SPR, surface plasmon resonance; SEM, scanning electron microscopy; TEM, transmission electron microscopy; DLS, dynamic light scattering; XRD, X-Ray diffraction spectroscopy.
At neutral pH, the negative charges on the surface of ZnO NPs encourage electrostatic interactions with metal cations; however, at lower pH, the HMs precipitate as hydroxides, and hydrogen ions compete with adsorbents for binding. The B. cereus strain XMCr-6 has also decreased Cr6+ via an enzyme-mediated mechanism. The reduced Cr3+ was shown to have a high affinity for cells, forming coordination interactions with the functional group on the bacterial cell wall's surface. Cr2O3 NPs were discovered on the cell surface (Laslo et al., 2022).
Utilization of HM contaminants by selected microorganisms to synthesize biogenic NPs (resource recovery) eliminates them from the environment (remediation) and enhances the value of the waste (effective waste utilization) (Ru et al., 2020). The formation of intracellular and extracellular (membrane-bound) Pd NPs was studied utilizing Enterococcus faecalis to recover Pd as Pd NPs. Transmission electron microscopy revealed a size range of 10 nm for Pd NPs, although the size of the Pd NPs depended on environmental factors such as temperature, pH, and biomass. The Pd NPs developed to have a lot of potential as nano catalysts since they have a high catalytic efficiency (6.3 mg Pd NPs reduced 5.0 mol Cr6+ in 12 h) and may be used to remediate industrial effluents (Cao et al., 2020b).
A similar work was done using riboflavin supplementation to create Te NPs from anaerobic sludge. It used pollutant tellurite Te4+ oxyanions to create insoluble elemental tellurium (Te0 NPs). It has been found that 2-Hydroxy 1,4 naphthoquinone stimulates Te4+ reduction and the formation of Te0NPs (Ramos-Ruiz et al., 2017). The process is aided by Rhodobacter capsulatus, which uses malate as an electron donor (Borghese et al., 2014), and riboflavin helps anaerobic methanogenic granular sludge reduce Te4+ faster.
Environmental concerns and fate of nanotechnology in soil remediation
Nanotechnology plays numerous roles and has made many advancements but still there is a concern about the implications of its presence on the environment, its fate, and its toxicological impact (Biswas et al., 2019). Because of their enhanced use, especially in remediation processes, the main aim of this section is to comprehensively summarize the fate and the environmental challenges associated with the deliberate emission of NMs and NPs. Based on statistics provided by, global production of engineered NMs in 2010 was estimated to be 260,000–309,000 tons, with 9–17% ending up in soils, 2% in waterbodies, and 0.1–1.5% in the atmosphere (Jośko et al., 2014). Environmental hazards are caused by NMs that persist in the environment for a long time by biodegrading or bioaccumulating in food chains and spread by organisms across trophic levels, causing them to pose ecotoxicological risks (Ameen et al., 2021). NM accumulation originates from the environment and from the plants. Plants act both as sinks as well as entry points for NMs for their accumulation in the food chain. The relevance of NMs to phytoremediation, including their effect on biomass production and plant responses, has led recent studies to focus more on their general effects on plant uptake of NMs. In spite of the fact that nanotechnology has the ability to solve problems that cannot be addressed by full-scale products, the safety of nanomaterials is still one of the biggest obstacles to their full application and prohibition to their wide use; hence, intensive studies must be conducted prior to their use.
It has recently been reported that NPs are posing serious problems for agriculture. NPs, when applied deliberately, may cause their accumulation or increase in their concentration in the soil, thereby altering their properties. Soils containing NPs have been reported to have altered pH, which is one of the most important parameters affecting soil nutrient availability, soil microbiology, soil health, and plant growth and development (Shi et al., 2018). As reported in a study carried out by Cullen, nanoscale zero-valent iron (nZVI) modification in the soil can cause a substantial rise in pH. Researchers reported similar results in a study on the effects of CuO nanoparticles on soil pH, where CuO nanoparticles consumed H+ from the soil to produce Cu ions and Cu(OH)+ complexes. Additionally, there is evidence that this process is more efficient in acidic soil. A number of NPs of Ag, Au, Ti, and Zn have been reported to be altering the soil pH and adversely affect soil microbiota and nematode populations (García-Gómez et al., 2018). There are three main factors influencing the detrimental effects of the presence of NPs in soil: their type and concentration, the type of soil, and the enzymatic activity of the soil.
The elevated concentration of NPs can lead to decreased dehydrogenase activity, which affects the balance of soil nutrients and fertility. Additionally, absorption and internalization of such nanoparticles can significantly impact mycelium and damage the normal functioning of microbes (Ameen et al., 2021). To bind and transport NPs in soil, three main mechanisms are involved, including surface complexation, hydrophobic partitioning, and ion exchange, which are also involved in the coupling of pollutants to colloid surfaces (Gao et al., 2017). In the presence of continuous NP emissions into the environment, they become ubiquitous. NPs are quite likely to penetrate into the food chain at various levels of trophic hierarchy and exert toxicological effects on a variety of aquatic and terrestrial animals as well as on humans (Cedervall et al., 2012).
NPs act as pollutants once they enter into the water bodies, where they ingested by lower aquatic organisms. Three species of animals representing different trophic levels were tested for metal NPs toxicity, including Danio rero (zebrafish), Daphnia pulex (Daphnia), and the microalgae Pseudokirchneriella subcapitata. Daphnia and microalgae were found to be more susceptible to NP exposure than zebrafish due to their particle filter-feeding habits. Therefore, once NPs enter the biological system, they influence biochemical processes at the molecular and tissue level (Yang et al., 2021). A number of toxicological effects, including DNA damage and oxidative stress induced by ROS, protein folding disruption, and cell death, have been documented because of the NPs (Olaru et al., 2019). Hence, before implementations of NPs for nano-bioremediation, these environmental concerns ought to be viewed as of the utmost importance. This would also enable NPs to be developed in a way that promotes sustainable applications.
Conclusion
The rapid release of pollutants into the environment in the modern era poses a substantial threat to both human health and the environment. Phytoremediation is one of the most promising technologies for treating HM-contaminated soil and has many advantages. In spite of this, the slow remediation process and variable weather conditions, as well as phytotoxic effects resulting from excessive contaminants (HMs), preclude its widespread use. Therefore, novel approaches are required to eliminate pollutants as much as possible because many conventional techniques are ineffective in removing different contaminants. The utilization of NPs in phytoremediation has shown great potential for enhancing phytoremediation efficiency in recent years. A major aim of this review is to provide an overview of the use of nanoparticles in solving potential issues, including the treatment of soil contaminated with heavy metals. Nano remediation has the potential to clean up large, contaminated sites, reducing clean-up time and eliminating the need for removal of contaminants and hence reducing the contaminant concentration to near zero. The success of the technique under field conditions is a key factor in the interdisciplinary work that is involved. Collaboration between chemistry and materials science is one of the main challenges of this research. Manipulating nanoparticle size and geometry can also enhance the remediation potential of nanoparticles. Future research is needed to address the constraints associated with the use of biologically derived nanoparticles in HM remediation, including studies on their toxicity to flora and fauna, bionanomaterials regeneration, regulation, degradation, and combined approaches for harnessing the potentials of bionanomaterials for phytoremediation, synergistic and antagonistic effects among microorganisms and bionanomaterials, and their stability in the bioremediation of the heavy metals at commercial and industrial levels.
Author contributions
SM designed the study. SM and DS wrote the manuscript. RR edited the manuscript. All authors read and approved the manuscript.
Acknowledgments
We are grateful to Director, Dayalbagh Educational Institute, Dayalbagh, Agra for encouragement and kind support.
Conflict of interest
The authors declare that the research was conducted in the absence of any commercial or financial relationships that could be construed as a potential conflict of interest.
Publisher's note
All claims expressed in this article are solely those of the authors and do not necessarily represent those of their affiliated organizations, or those of the publisher, the editors and the reviewers. Any product that may be evaluated in this article, or claim that may be made by its manufacturer, is not guaranteed or endorsed by the publisher.
References
Abhilash, P. C., Jamil, S., and Singh, N. (2009). Transgenic plants for enhanced biodegradation and phytoremediation of organic xenobiotics. Biotechnol. Adv. 27, 474–488. doi: 10.1016/j.biotechadv.2009.04.002
Alabresm, A., Chen, Y. P., Decho, A. W., and Lead, J. (2018). A novel method for the synergistic remediation of oil-water mixtures using nanoparticles and oil-degrading bacteria. Sci. Total Environ. 630, 1292–1297. doi: 10.1016/j.scitotenv.2018.02.277
Ali, H., Khan, E., and Sajad, M. A. (2013). Phytoremediation of heavy metals—concepts and applications. Chemosphere 91, 869–881. doi: 10.1016/j.chemosphere.2013.01.075
Ameen, F., Alsamhary, K., Alabdullatif, J. A., and AL-Nadhari, S. (2021). A review on metal-based nanoparticles and their toxicity to beneficial soil bacteria and fungi. Ecotoxicol. Environ. Saf. 213, 112027. doi: 10.1016/j.ecoenv.2021.112027
Amin, I., Nazir, R., and Rather, M. A. (2021). Nano-bioremediation: an innovative approach for remedying heavy metals using fungi. J. Bioremediat. Biodegrad. 12, 2.
Andrade-Zavaleta, K., Chacon-Laiza, Y., Asmat-Campos, D., and Raquel-Checca, N. (2022). Green synthesis of superparamagnetic iron oxide nanoparticles with eucalyptus globulus extract and their application in the removal of heavy metals from agricultural soil. Molecules 27, 1367. doi: 10.3390/molecules27041367
Arduini, F., Micheli, L., Moscone, D., Palleschi, G., Piermarini, S., Ricci, F., et al. (2016). Electrochemical biosensors based on nanomodified screen-printed electrodes: recent applications in clinical analysis. Trends Anal. Chem. 79, 114–126. doi: 10.1016/j.trac.2016.01.032
Ashraf, S., Siddiqa, A., Shahida, S., and Qaisar, S. (2019). Titanium-based nanocomposite materials for arsenic removal from water: a review. Heliyon 5, e01577. doi: 10.1016/j.heliyon.2019.e01577
Awasthi, G., Nagar, V., Mandzhieva, S., Minkina, T., Sankhla, M. S., Pandit, P. P., et al. (2022). Sustainable amelioration of heavy metals in soil ecosystem: existing developments to emerging trends. Minerals 12, 85. doi: 10.3390/min12010085
Bakshi, M., and Abhilash, P. C. (2020). “Nanotechnology for soil remediation: revitalizing the tarnished resource,” in Nano-Materials as Photocatalysts for Degradation of Environmental Pollutants (Varanasi: Elsevier), 345–370. doi: 10.1016/B978-0-12-818598-8.00017-1
Ballen, S. C., Steffens, J., and Steffens, C. (2021). Stability characteristics of cantilever nanobiosensors with simple and complex molecules for determination of cadmium. Sens. Actuator. Phys. 324, 112686. doi: 10.1016/j.sna.2021.112686
Baragaño, D., Forján, R., Welte, L., and Gallego, J. L. R. (2020). Nanoremediation of as and metals polluted soils by means of graphene oxide nanoparticles. Sci. Rep. 10, 1896. doi: 10.1038/s41598-020-58852-4
Benjamin, S. R., Lima, F. D., Florean, E. O. P. T., and Guedes, M. I. F. (2019). Current trends in nanotechnology for bioremediation. Int. J. Environ. Pollut. 66, 19–40. doi: 10.1504/IJEP.2019.104526
Bhuiyan, M. A. H., Karmaker, S. C., Bodrud-Doza, M., Rakib, M. A., and Saha, B. B. (2021). Enrichment, sources and ecological risk mapping of heavy metals in agricultural soils of dhaka district employing SOM, PMF and GIS methods. Chemosphere 263, 128339. doi: 10.1016/j.chemosphere.2020.128339
Biswas, B., Warr, L. N., Hilder, E. F., Goswami, N., Rahman, M. M., Churchman, J. G., et al. (2019). Biocompatible functionalisation of nanoclays for improved environmental remediation. Chem. Soc. Rev. 48, 3740–3770. doi: 10.1039/C8CS01019F
Biswas, M., and Raichur, A. M. (2008). Electrokinetic and rheological properties of nano zirconia in the presence of rhamnolipid biosurfactant. J. Am. Ceram. Soc. 91, 3197–3201. doi: 10.1111/j.1551-2916.2008.02617.x
Borghese, R., Baccolini, C., Francia, F., Sabatino, P., Turner, R. J., and Zannoni, D. (2014). Reduction of chalcogen oxyanions and generation of nanoprecipitates by the photosynthetic bacterium Rhodobacter capsulatus. J. Hazard. Mater. 269, 24–30. doi: 10.1016/j.jhazmat.2013.12.028
Briffa, J., Sinagra, E., and Blundell, R. (2020). Heavy metal pollution in the environment and their toxicological effects on humans. Heliyon 6, e04691. doi: 10.1016/j.heliyon.2020.e04691
Cao, F., Dai, H., Hao, P. F., and Wu, F. (2020a). Silicon regulates the expression of vacuolar H+-pyrophosphatase 1 and decreases cadmium accumulation in rice (Oryza sativa L.). Chemosphere 240, 124907. doi: 10.1016/j.chemosphere.2019.124907
Cao, X., Alabresm, A., Chen, Y. P., Decho, A. W., and Lead, J. (2020b). Improved metal remediation using a combined bacterial and nanoscience approach. Sci. Total Environ. 704, 135378. doi: 10.1016/j.scitotenv.2019.135378
Cedervall, T., Hansson, L. A., Lard, M., Frohm, B., and Linse, S. (2012). Food chain transport of nanoparticles affects behaviour and fat metabolism in fish. PLoS ONE 7, e32254. doi: 10.1371/journal.pone.0032254
Chaplygin, V. A., Minkina, T. M., Mandzhieva, S. S., Nazarenko, O. G., Zimulina, I. V., Bauer, T. V., et al. (2021). “Heavy metals in agricultural crops of Rostov region through the example of soft wheat (Triticum aestivum),” in IOP Conference Series: Earth and Environmental Science, Vol. 624 (Rostov-on-Don: IOP Publishing), 012204. doi: 10.1088/1755-1315/624/1/012204
Chauhan, R., Yadav, H. O., and Sehrawat, N. (2020). Nanobioremediation: a new and a versatile tool for sustainable environmental clean up-overview. J. Mater. Environ. Sci. 11, 564–573.
Chellaiah, E. R. (2018). Cadmium (heavy metals) bioremediation by Pseudomonas aeruginosa: a minireview. Appl. Water Sci. 8, 1–10. doi: 10.1007/s13201-018-0796-5
Chen, C., Tsyusko, O. V., McNear, D. H. Jr., Judy, J., Lewis, R. W., and Unrine, J. M. (2017a). Effects of biosolids from a wastewater treatment plant receiving manufactured nanomaterials on Medicago truncatula and associated soil microbial communities at low nanomaterial concentrations. Sci. Total Environ. 609, 799–806. doi: 10.1016/j.scitotenv.2017.07.188
Chen, M., Zeng, G., Xu, P., Zhang, Y., Jiang, D., and Zhou, S. (2017b). Understanding enzymatic degradation of single-walled carbon nanotubes triggered by functionalization using molecular dynamics simulation. Environ. Sci. Nano R. Soc. Chem. 4, 720–727. doi: 10.1039/C7EN00050B
Das, P. K., Das, B. P., and Dash, P. (2022). A super-tolerant bacteria strain improves phytoremediation of Cr (VI) contaminated soil with Pongamia pinnata. Rhizosphere 22, 100543. doi: 10.1016/j.rhisph.2022.100543
De Rossi, A., Rigueto, C. V., Dettmer, A., Colla, L. M., and Piccin, J. S. (2020). Synthesis, characterization, and application of Saccharomyces cerevisiae/alginate composites beads for adsorption of heavy metals. J. Environ. Chem. Eng. 8, 104009. doi: 10.1016/j.jece.2020.104009
Debnath, M., Chauhan, N., Sharma, P., and Tomar, I. (2021). “Potential of nano biosurfactants as an ecofriendly green technology for bioremediation,” in Handbook of Nanomaterials for Wastewater Treatment (Jaipur: Elsevier), 1039–1055. doi: 10.1016/B978-0-12-821496-1.00013-1
Fayiga, A. O., and Saha, U. K. (2016). Arsenic hyperaccumulating fern: implications for remediation of arsenic contaminated soils. Geoderma 284, 132–143. doi: 10.1016/j.geoderma.2016.09.003
Fosso-Kankeu, E., Mulaba-Bafubiandi, A. F., and Mishra, A. K. (2014). Prospects for Immobilization of Microbial Sorbents on Carbon Nanotubes for Biosorption: Bioremediation of Heavy Metals Polluted Water, Vol. 1 (Hoboken, NJ: John Wiley & Sons, Inc), 213–235. doi: 10.1002/9781118939314.ch3
Gao, J., Wang, Y., Du, Y., Zhou, L., He, Y., Ma, L., et al. (2017). Construction of biocatalytic colloidosome using lipase-containing dendritic mesoporous silica nanospheres for enhanced enzyme catalysis. Chem. Eng. J. 317, 175–186. doi: 10.1016/j.cej.2017.02.012
García-Gómez, C., Fernández, M. D., García, S., Obrador, A. F., Letón, M., and Babín, M. (2018). Soil pH effects on the toxicity of zinc oxide nanoparticles to soil microbial community. Environ. Sci. Pollut. Res. 25, 28140–28152. doi: 10.1007/s11356-018-2833-1
Gerhardt, K. E., Gerwing, P. D., and Greenberg, B. M. (2017). Opinion: taking phytoremediation from proven technology to accepted practice. Plant Sci. 256, 170–185. doi: 10.1016/j.plantsci.2016.11.016
Ghaffar, N., Farrukh, M. A., and Naz, S. (2020). “Applications of nanobiosensors in agriculture,” in Nanoagronomy, ed S. Javad (Cham: Springer), 179–196. doi: 10.1007/978-3-030-41275-3_10
Gong, X., Huang, D., Liu, Y., Peng, Z., Zeng, G., Xu, P., et al. (2018). Remediation of contaminated soils by biotechnology with nanomaterials: bio-behavior, applications, and perspectives. Crit. Rev. Biotechnol. 38, 455–468. doi: 10.1080/07388551.2017.1368446
Gopalakrishnan Nair, P. M., and Chung, I. M. (2014). Cell cycle and mismatch repair genes as potential biomarkers in Arabidopsis thaliana seedlings exposed to silver nanoparticles. Bull. Environ. Contam. Toxicol. 92, 719–725. doi: 10.1007/s00128-014-1254-1
Hazra, C., Kundu, D., Chaudhari, A., and Jana, T. (2013). Biogenic synthesis, characterization, toxicity and photocatalysis of zinc sulfide nanoparticles using rhamnolipids from Pseudomonas aeruginosa BS01 as capping and stabilizing agent. J. Chem. Technol. Biotechnol. 88, 1039–1048. doi: 10.1002/jctb.3934
Hu, X., Kang, J., Lu, K., Zhou, R., Mu, L., and Zhou, Q. (2014). Graphene oxide amplifies the phytotoxicity of arsenic in wheat. Sci. Rep. 4, 6122. doi: 10.1038/srep06122
Huang, H., Ullah, F., Zhou, D. X., Yi, M., and Zhao, Y. (2019). Mechanisms of ROS regulation of plant development and stress responses. Front. Plant Sci. 10, 800. doi: 10.3389/fpls.2019.00800
Hussain, A., Ali, S., Rizwan, M., ur Rehman, M. Z., Qayyum, M. F., Wang, H., et al. (2019). Responses of wheat (Triticum aestivum) plants grown in a Cd contaminated soil to the application of iron oxide nanoparticles. Ecotoxicol. Environ. Saf. 173, 156–164. doi: 10.1016/j.ecoenv.2019.01.118
Hussain, B., Lin, Q., Hamid, Y., Sanaullah, M., Di, L., Khan, M. B., et al. (2020). Foliage application of selenium and silicon nanoparticles alleviates Cd and Pb toxicity in rice (Oryza sativa L.). Sci. Total Environ. 712, 136497. doi: 10.1016/j.scitotenv.2020.136497
Ibrahim, M. L., Ijah, U. J. J., Manga, S. B., Bilbis, L. S., and Umar, S. (2013). Production and partial characterization of biosurfactant produced by crude oil degrading bacteria. Int. Biodeteriorat. Biodegrad. 81, 28–34. doi: 10.1016/j.ibiod.2012.11.012
Jaafar, R., Al-Sulami, A., and Al-Taee, A. (2016). The biosorption ability of shewanella oneidensis for cadmium and lead isolated from soil in Basra Governorate, Iraq. Afr. J. Microbiol. Res. 38, 267–270.
Jośko, I., Oleszczuk, P., and Futa, B. (2014). The effect of inorganic nanoparticles (ZnO, Cr2O3, CuO and Ni) and their bulk counterparts on enzyme activities in different soils. Geoderma 232, 528–537. doi: 10.1016/j.geoderma.2014.06.012
Kandiah, M., and Chandrasekaran, K. N. (2021). Green synthesis of silver nanoparticles using Catharanthus roseus flower extracts and the determination of their antioxidant, antimicrobial, and photocatalytic activity. J. Nanotechnol. 2021, 5512786. doi: 10.1155/2021/5512786
Kasture, M. B., Patel, P., Prabhune, A. A., Ramana, C. V., Kulkarni, A. A., and Prasad, B. L. V. (2008). Synthesis of silver nanoparticles by sophorolipids: effect of temperature and sophorolipid structure on the size of particles. J. Chem. Sci. 120, 515–520. doi: 10.1007/s12039-008-0080-6
Khan, A., Malik, S., Ali, N., Bilal, M., Yang, Y., Akhter, M. S., et al. (2022). “Nanobiosorbents: basic principles, synthesis, and application for contaminants removal,” in Nano-Biosorbents for Decontamination of Water, Air, and Soil Pollution (Peshawar: Elsevier), 45–59. doi: 10.1016/B978-0-323-90912-9.00003-4
Konate, A., He, X., Zhang, Z., Ma, Y., Zhang, P., Alugongo, G. M., et al. (2017). Magnetic (Fe3O4) nanoparticles reduce heavy metals uptake and mitigate their toxicity in wheat seedling. Sustainability 9, 790. doi: 10.3390/su9050790
Lal, R. (2015). Restoring soil quality to mitigate soil degradation. Sustainability 7, 5875–5895. doi: 10.3390/su7055875
Laslo, V., Pinzaru, S. C., Zaguła, G., Kluz, M., Vicas, S. I., and Cavalu, S. (2022). Synergic effect of selenium nanoparticles and lactic acid bacteria in reduction cadmium toxicity. J. Mol. Struct. 1247, 131325. doi: 10.1016/j.molstruc.2021.131325
Lew, T. T. S., Park, M., Cui, J., and Strano, M. S. (2021). Plant nanobionic sensors for arsenic detection. Adv. Mater. 33, 2005683. doi: 10.1002/adma.202005683
Liang, S. X., Jin, Y., Liu, W., Li, X., Shen, S. G., and Ding, L. (2017). Feasibility of Pb phytoextraction using nano-materials assisted ryegrass: Results of a one-year field-scale experiment. J. Environ. Manage. 190, 170–175. doi: 10.1016/j.jenvman.2016.12.064
Ma, X., and Wang, C. (2010). Fullerene nanoparticles affect the fate and uptake of trichloroethylene in phytoremediation systems. Environ. Eng. Sci. 27, 989–992 doi: 10.1089/ees.2010.0141
Mahmoud, M. E., Abdou, A. E., Mohamed, S. M., and Osman, M. M. (2016). Engineered Staphylococcus aureus via immobilization on magnetic Fe3O4-phthalate nanoparticles for biosorption of divalent ions from aqueous solutions. J. Environ. Chem. Eng. 4, 3810–3824. doi: 10.1016/j.jece.2016.08.022
Mahmoud, M. E., Fekry, N. A., and Abdelfattah, A. M. (2020). A novel nanobiosorbent of functionalized graphene quantum dots from rice husk with barium hydroxide for microwave enhanced removal of lead (II) and lanthanum (III). Bioresour. Technol. 298, 122514. doi: 10.1016/j.biortech.2019.122514
Mandal, N., Adhikary, S., and Rakshit, R. (2020). “Nanobiosensors: recent developments in soil health assessment,” in Soil Analysis: Recent Trends and Applications, eds A. Rakshit, S. Ghosh, S. Chakraborty, V. Philip, and A. Datta (Bhagalpur: Springer), 285–304. doi: 10.1007/978-981-15-2039-6_15
Mechrez, G., Krepker, M. A., Harel, Y., Lellouche, J. P., and Segal, E. (2014). Biocatalytic carbon nanotube paper: a ‘one-pot'route for fabrication of enzyme-immobilized membranes for organophosphate bioremediation. J. Mater. Chem. B 2, 915–922. doi: 10.1039/C3TB21439G
Mitra, S., Pramanik, K., Sarkar, A., Ghosh, P. K., Soren, T., and Maiti, T. K. (2018). Bioaccumulation of cadmium by Enterobacter sp. and enhancement of rice seedling growth under cadmium stress. Ecotoxicol. Environ. Saf. 156, 183–196. doi: 10.1016/j.ecoenv.2018.03.001
Moharem, M., Elkhatib, E., and Mesalem, M. (2019). Remediation of chromium and mercury polluted calcareous soils using nanoparticles: sorption–desorption kinetics, speciation and fractionation. Environ. Res. 170, 366–373. doi: 10.1016/j.envres.2018.12.054
Mohsenzadeh, F., and Rad, C. A. (2011). International Conference Nanotechnology Biosensoors. Singapore: IACSIT Press.
Mosbah, R., and Sahmoune, M. (2013). Biosorption of heavy metals by Streptomyces species—an overview. Open Chem. 11, 1412–1422. doi: 10.2478/s11532-013-0268-6
Olaru, D. G., Olaru, A., Kassem, G. H., Popescu-Driga, M. V., Pinosanu, L. R., Dumitrascu, D. I., et al. (2019). Toxicity and health impact of nanoparticles. Basic biology and clinical perspective. Rom. J. Morphol. Embryol. 60, 787–792.
Peng, H., Li, D., Ye, J., Xu, H., Xie, W., Zhang, Y., et al. (2019). Biosorption behavior of the Ochrobactrum MT180101 on ionic copper and chelate copper. J. Environ. Manage. 235, 224–230. doi: 10.1016/j.jenvman.2019.01.060
Pillai, H. P., and Kottekottil, J. (2016). Nano-phytotechnological remediation of endosulfan using zero valent iron nanoparticles. J. Environ. Protect. 7, 734. doi: 10.4236/jep.2016.75066
Qiao, W., Zhang, Y., Xia, H., Luo, Y., Liu, S., Wang, S., et al. (2019). Bioimmobilization of lead by Bacillus subtilis X3 biomass isolated from lead mine soil under promotion of multiple adsorption mechanisms. R. Soc. Open Sci. 6, 181701. doi: 10.1098/rsos.181701
Rai, P. K., Lee, S. S., Zhang, M., Tsang, Y. F., and Kim, K. H. (2019). Heavy metals in food crops: health risks, fate, mechanisms, and management. Environ. Int. 125, 365–385. doi: 10.1016/j.envint.2019.01.067
Rai, V., Acharya, S., and Dey, N. (2012). Implications of nanobiosensors in agriculture. J. Biomater. Nanobiotechnol. 3, 315–324. doi: 10.4236/jbnb.2012.322039
Rajput, V. D., Minkina, T., Upadhyay, S. K., Kumari, A., Ranjan, A., Mandzhieva, S., et al. (2022). Nanotechnology in the restoration of polluted soil. Nanomaterials 12, 769. doi: 10.3390/nano12050769
Ramírez, V., Baez, A., López, P., Bustillos, R., Villalobos, M. Á., Carreño, R., et al. (2019). Chromium hyper-tolerant Bacillus sp. MH778713 assists phytoremediation of heavy metals by mesquite trees (Prosopis laevigata). Front. Microbiol. 10, 1833. doi: 10.3389/fmicb.2019.01833
Ramos-Ruiz, A., Sesma-Martin, J., Sierra-Alvarez, R., and Field, J. A. (2017). Continuous reduction of tellurite to recoverable tellurium nanoparticles using an upflow anaerobic sludge bed (UASB) reactor. Water Res. 108, 189–196. doi: 10.1016/j.watres.2016.10.074
Ravindran, A., Sajayan, A., Priyadharshini, G. B., Selvin, J., and Kiran, G. S. (2020). Revealing the efficacy of thermostable biosurfactant in heavy metal bioremediation and surface treatment in vegetables. Front. Microbiol. 11, 222. doi: 10.3389/fmicb.2020.00222
Reddy, A. S., Chen, C. Y., Chen, C. C., Jean, J. S., Fan, C. W., Chen, H. R., et al. (2009). Synthesis of gold nanoparticles via an environmentally benign route using a biosurfactant. J. Nanosci. Nanotechnol. 9, 6693–6699. doi: 10.1166/jnn.2009.1347
Rigo, A. A., Cezaro, A. M. D., Muenchen, D. K., Martinazzo, J., Brezolin, A. N., Hoehne, L., et al. (2020a). Cantilever nanobiosensor based on the enzyme urease for detection of heavy metals. Braz. J. Chem. Eng. 36, 1429–1437. doi: 10.1590/0104-6632.20190364s20190035
Rigo, A. A., Cezaro, A. M. D., Muenchen, D. K., Martinazzo, J., Manzoli, A., Steffens, J., et al. (2020b). Heavy metals detection in river water with cantilever nanobiosensor. J. Environ. Sci. Health Part B 55, 239–249. doi: 10.1080/03601234.2019.1685318
Rizwan, K., Rasheed, T., and Bilal, M. (2022). “Alginate-based nanobiosorbents for bioremediation of environmental pollutants,” in Nano-Biosorbents for Decontamination of Water, Air, and Soil Pollution (Sahiwal: Elsevier), 479–502. doi: 10.1016/B978-0-323-90912-9.00021-6
Ru, J., Huo, Y., and Yang, Y. (2020). Microbial degradation and valorization of plastic wastes. Front. Microbiol. 11, 442. doi: 10.3389/fmicb.2020.00442
Saha, A., and Bhaduri, D. (2021). Nano-Biosensors: applications in agriculture and allied fields. Biotica Res. Today 3, 1106–1109.
Saleem, M. H., Fahad, S., Adnan, M., Ali, M., Rana, M. S., Kamran, M., et al. (2020a). Foliar application of gibberellic acid endorsed phytoextraction of copper and alleviates oxidative stress in jute (Corchorus capsularis L.) plant grown in highly copper-contaminated soil of China. Environ. Sci. Pollut. Res. 27, 37121–37133. doi: 10.1007/s11356-020-09764-3
Saleem, M. H., Fahad, S., Khan, S. U., Din, M., Ullah, A., Sabagh, A. E., et al. (2020b). Copper-induced oxidative stress, initiation of antioxidants and phytoremediation potential of flax (Linum usitatissimum L.) seedlings grown under the mixing of two different soils of China. Environ. Sci. Pollut. Res. 27, 5211–5221. doi: 10.1007/s11356-019-07264-7
Sarubbo, L. A., Maria da Gloria, C. S., Durval, I. J. B., Bezerra, K. G. O., Ribeiro, B. G., Silva, I. A., et al. (2022). Biosurfactants: production, properties, applications, trends, and general perspectives. Biochem. Eng. J. 181, 108377. doi: 10.1016/j.bej.2022.108377
Scognamiglio, V., Pezzotti, I., Pezzotti, G., Cano, J., Manfredonia, I., Buonasera, K., et al. (2013). A new embedded biosensor platform based on micro-electrodes array (MEA) technology. Sens. Actuat. B Chem. 176, 275–283. doi: 10.1016/j.snb.2012.09.101
Sebastian, A., Nangia, A., and Prasad, M. N. V. (2019). Cadmium and sodium adsorption properties of magnetite nanoparticles synthesized from Hevea brasiliensis Muell. Arg. bark: relevance in amelioration of metal stress in rice. J. Haz. Mater. 371, 261–272. doi: 10.1016/j.jhazmat.2019.03.021
Shi, J., Ye, J., Fang, H., Zhang, S., and Xu, C. (2018). Effects of copper oxide nanoparticles on paddy soil properties and components. Nanomaterials 8, 839. doi: 10.3390/nano8100839
Singh, J., Dutta, T., Kim, K. H., Rawat, M., Samddar, P., and Kumar, P. (2018). ‘Green'synthesis of metals and their oxide nanoparticles: applications for environmental remediation. J. Nanobiotechnol. 16, 1–24. doi: 10.1186/s12951-018-0408-4
Singh, J., and Lee, B. K. (2016). Influence of nano-TiO2 particles on the bioaccumulation of Cd in soybean plants (Glycine max): a possible mechanism for the removal of Cd from the contaminated soil. J. Environ. Manage. 170, 88–96. doi: 10.1016/j.jenvman.2016.01.015
Singh, P., Kim, Y. J., Wang, C., Mathiyalagan, R., and Yang, D. C. (2016). The development of a green approach for the biosynthesis of silver and gold nanoparticles by using Panax ginseng root extract, and their biological applications. Artif. Cells Nanomed. Biotechnol. 44, 1150–1157.
Song, B., Xu, P., Chen, M., Tang, W., Zeng, G., Gong, J., et al. (2019). Using nanomaterials to facilitate the phytoremediation of contaminated soil. Crit. Rev. Environ. Sci. Technol. 49, 791–824. doi: 10.1080/10643389.2018.1558891
Souri, Z., Karimi, N., Sarmadi, M., and Rostami, E. (2017). Salicylic acid nanoparticle (SANPs) improves growth and phytoremediation efficiency of Isatis cappadocica Desv. under arsenic stress. IET Nanobiotechnol. 11, 650–655. doi: 10.1049/iet-nbt.2016.0202
Suman, J., Uhlik, O., Viktorova, J., and Macek, T. (2018). Phytoextraction of heavy metals: a promising tool for clean-up of polluted environment? Front. Plant Sci. 9, 1476. doi: 10.3389/fpls.2018.01476
Tang, Y., Tian, J., Li, S., Xue, C., Xue, Z., Yin, D., et al. (2015). Combined effects of graphene oxide and Cd on the photosynthetic capacity and survival of Microcystis aeruginosa. Sci. Total Environ. 532, 154–161. doi: 10.1016/j.scitotenv.2015.05.081
Taran, M., Fateh, R., Rezaei, S., and Gholi, M. K. (2019). Isolation of arsenic accumulating bacteria from garbage leachates for possible application in bioremediation. Iran. J. Microbiol. 11, 60. doi: 10.18502/ijm.v11i1.707
Torres, E. (2020). Biosorption: a review of the latest advances. Processes 8, 1584. doi: 10.3390/pr8121584
Ullah, S., Adeel, M., Zain, M., Rizwan, M., Irshad, M. K., Jilani, G., et al. (2020). Physiological and biochemical response of wheat (Triticum aestivum) to TiO2 nanoparticles in phosphorous amended soil: a full life cycle study. J. Environ. Manage. 263, 110365. doi: 10.1016/j.jenvman.2020.110365
Upadhyay, N., Vishwakarma, K., Singh, J., Mishra, M., Kumar, V., Rani, R., et al. (2017). Tolerance and reduction of chromium (VI) by Bacillus sp. MNU16 isolated from contaminated coal mining soil. Front. Plant Sci. 8, 778. doi: 10.3389/fpls.2017.00778
Usman, M., Farooq, M., Wakeel, A., Nawaz, A., Cheema, S. A., ur Rehman, H., et al. (2020). Nanotechnology in agriculture: current status, challenges and future opportunities. Sci. Total Environ. 721, 137778. doi: 10.1016/j.scitotenv.2020.137778
Velkova, Z., Kirova, G., Stoytcheva, M., Kostadinova, S., Todorova, K., and Gochev, V. (2018). Immobilized microbial biosorbents for heavy metals removal. Eng. Life Sci. 18, 871–881. doi: 10.1002/elsc.201800017
Vishwakarma, K., Singh, V. P., Prasad, S. M., Chauhan, D. K., Tripathi, D. K., and Sharma, S. (2020). Silicon and plant growth promoting rhizobacteria differentially regulate AgNP-induced toxicity in Brassica juncea: implication of nitric oxide. J. Haz. Mater. 390, 121806. doi: 10.1016/j.jhazmat.2019.121806
Waghmode, S., Suryavanshi, M., Dama, L., Kansara, S., Ghattargi, V., Das, P., et al. (2019). Genomic insights of halophilic Planococcus maritimus SAMP MCC 3013 and detail investigation of its biosurfactant production. Front. Microbiol. 10, 235. doi: 10.3389/fmicb.2019.00235
Wang, K., Wang, Y., Wan, Y., Mi, Z., Wang, Q., Wang, Q., et al. (2021). The fate of arsenic in rice plants (Oryza sativa L.): influence of different forms of selenium. Chemosphere 264, 128417. doi: 10.1016/j.chemosphere.2020.128417
Wang, Y., Fang, Z., Kang, Y., and Tsang, E. P. (2014). Immobilization and phytotoxicity of chromium in contaminated soil remediated by CMC-stabilized nZVI. J. Haz. Mater. 275, 230–237. doi: 10.1016/j.jhazmat.2014.04.056
Wang, Y., Liu, Y., Zhan, W., Zheng, K., Lian, M., Zhang, C., et al. (2020). Long-term stabilization of Cd in agricultural soil using mercapto-functionalized nano-silica (MPTS/nano-silica): a three-year field study. Ecotoxicol. Environ. Saf. 197, 110600. doi: 10.1016/j.ecoenv.2020.110600
Wu, H., Tito, N., and Giraldo, J. P. (2017). Anionic cerium oxide nanoparticles protect plant photosynthesis from abiotic stress by scavenging reactive oxygen species. ACS Nano 11, 11283–11297. doi: 10.1021/acsnano.7b05723
Xu, S., Xing, Y., Liu, S., Hao, X., Chen, W., and Huang, Q. (2020). Characterization of Cd2+ biosorption by Pseudomonas sp. strain 375, a novel biosorbent isolated from soil polluted with heavy metals in Southern China. Chemosphere 240, 124893 doi: 10.1016/j.chemosphere.2019.124893
Yadav, V. K., Choudhary, N., Khan, S. H., Malik, P., Inwati, G. K., Suriyaprabha, R., et al. (2020). “Synthesis and characterisation of nano-biosorbents and their applications for waste water treatment,” in Handbook of Research on Emerging Developments and Environmental Impacts of Ecological Chemistry (Visnagar: IGI Global), 252–290. doi: 10.4018/978-1-7998-1241-8.ch012
Yang, W., Wang, L., Mettenbrink, E. M., DeAngelis, P. L., and Wilhelm, S. (2021). Nanoparticle toxicology. Ann. Rev. Pharmacol. Toxicol. 61, 269–289. doi: 10.1146/annurev-pharmtox-032320-110338
Yao, J., Cheng, Y., Zhou, M., Zhao, S., Lin, S., Wang, X., et al. (2018). ROS scavenging Mn 3 O 4 nanozymes for in vivo anti-inflammation. Chem. Sci. 9, 2927–2933. doi: 10.1039/C7SC05476A
Zhao, L., Lu, L., Wang, A., Zhang, H., Huang, M., Wu, H., et al. (2020). Nano-biotechnology in agriculture: use of nanomaterials to promote plant growth and stress tolerance. J. Agric. Food Chem. 68, 1935–1947. doi: 10.1021/acs.jafc.9b06615
Keywords: green nanotechnology, heavy metal(loid), nanomaterial, nanobiosensors, biogenic remediation, biosurfactants
Citation: Mathur S, Singh D and Ranjan R (2022) Remediation of heavy metal(loid) contaminated soil through green nanotechnology. Front. Sustain. Food Syst. 6:932424. doi: 10.3389/fsufs.2022.932424
Received: 29 April 2022; Accepted: 29 June 2022;
Published: 05 August 2022.
Edited by:
Khurram Shahzad, Lasbela University of Agriculture, Water and Marine Sciences, PakistanReviewed by:
Christine Jeyaseelan, Amity University, IndiaShah Fahad, The University of Haripur, Pakistan
Copyright © 2022 Mathur, Singh and Ranjan. This is an open-access article distributed under the terms of the Creative Commons Attribution License (CC BY). The use, distribution or reproduction in other forums is permitted, provided the original author(s) and the copyright owner(s) are credited and that the original publication in this journal is cited, in accordance with accepted academic practice. No use, distribution or reproduction is permitted which does not comply with these terms.
*Correspondence: Rajiv Ranjan, rajivranjanbt@gmail.com