Modeling the Ruminant Placenta-Pathogen Interactions in Apicomplexan Parasites: Current and Future Perspectives
- Animal Health and Zoonoses (SALUVET) Group, Animal Health Department, Faculty of Veterinary Sciences, Complutense University of Madrid, Madrid, Spain
Neospora caninum and Toxoplasma gondii are one of the main concerns of the livestock sector as they cause important economic losses in ruminants due to the reproductive failure. It is well-known that the interaction of these parasites with the placenta determines the course of infection, leading to fetal death or parasite transmission to the offspring. However, to advance the development of effective vaccines and treatments, there are still important gaps on knowledge on the placental host-parasite interactions that need to be addressed. Ruminant animal models are still an indispensable tool for providing a global view of the pathogenesis, lesions, and immune responses, but their utilization embraces important economic and ethics restrictions. Alternative in vitro systems based on caruncular and trophoblast cells, the key cellular components of placentomes, have emerged in the last years, but their use can only offer a partial view of the processes triggered after infection as they cannot mimic the complex placental architecture and neglect the activity of resident immune cells. These drawbacks could be solved using placental explants, broadly employed in human medicine, and able to preserve its cellular architecture and function. Despite the availability of such materials is constrained by their short shelf-life, the development of adequate cryopreservation protocols could expand their use for research purposes. Herein, we review and discuss existing (and potential) in vivo, in vitro, and ex vivo ruminant placental models that have proven useful to unravel the pathogenic mechanisms and the host immune responses responsible for fetal death (or protection) caused by neosporosis and toxoplasmosis.
The Ruminant Placenta: a Battlefield Between Host and Apicomplexan Parasites
The placenta plays a critical role in the pathogenesis of neosporosis and toxoplasmosis as it acts as a biological barrier that must be crossed by the parasite to reach the fetus. In addition, from an immunological point of view, it is a unique organ. On one hand, it facilitates embryo implantation promoting immunological tolerance to the conceptus. On the other hand, it mobilizes a number of defense mechanisms against pathogens potentially harmful to the developing fetus (1). Despite this, Neospora caninum and Toxoplasma gondii-the etiologic agents of bovine neosporosis and sheep (and goat) toxoplasmosis, respectively, are still able to replicate in the placental tissues, compromising pregnancy success. This outcome is triggered by two different mechanisms related to parasite replication itself and the maternal immune response developed against the replicating parasites, both potentially harmful for the placenta (2). In this review, we aim to summarize available and potential models to study effects of infection by these parasites at the maternal-fetal interface, thus encouraging future research focused on expanding the understanding of the pathogenesis of bovine neosporosis and ovine toxoplasmosis.
Structure and Function of the Placenta in Ruminants
In mammals, the growth and survival of the fetus depend on the placenta, a temporary organ formed by an intricate of maternal and fetal tissues that keep both individuals connected. The ontogeny and morphology of the ruminant placenta have been thoroughly reviewed in the last years (3–5), and thus we will focus this review on the key aspects of placental structure necessary to understand how host-pathogen interactions occur. Domestic ruminants present chorioallantoic, villous, and cotyledonary placentae. These terms refer to the fusion of the chorion and allantois in discrete areas of the fetal placenta to form villous projections named cotyledons. Considering the number of layers that act as a barrier between the fetal and maternal circulation in these species, placentae can also be classified as synepitheliochorial due to the replacement of the maternal epithelial layer opposed to the fetal chorion by a foeto-maternal syncytial layer (6) (Figures 1A, 2). In ruminants, the implantation process begins at 16 (sheep) or 20 (cow) days post coitum, when the chorionic epithelium of the embryo (formed by trophoblast cells) develops folds which fit into raised projections of the uterine wall (caruncles). These chorionic folds form villous projections (chorioallantoic villi) that branch and extend toward the caruncular wall with which interdigitates forming septa. In turn, the caruncular septa extend branching projections in the opposite direction, thus forming a network of fetal villi apposed within maternal crypts. The combination of a fetal cotyledon and a maternal caruncle is termed placentome, which is considered the functional units where the main placental functions take place (Figures 1A, 2) (3–6).
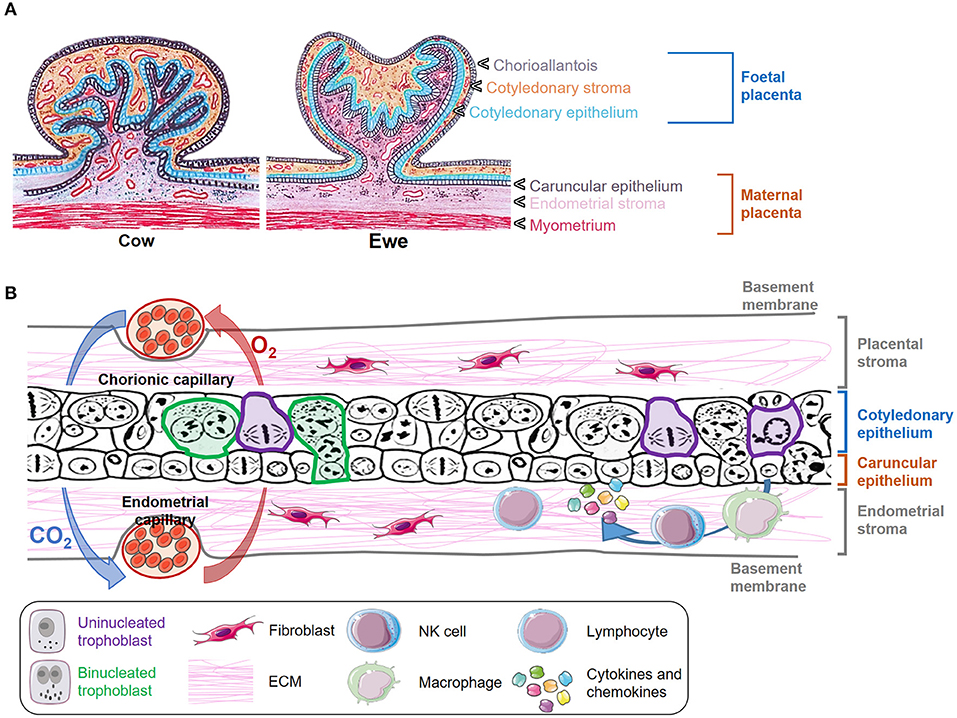
Figure 1. Morphology and structure of the placenta in ruminants. (A) Transverse section of a cow and ewe placentome indicating the different layers of the fetal and maternal placenta. (B) Schematic representation of the microscopic structure of placentomes. Some uninucleated trophoblast cells are delineated in purple, and some binucleated trophoblast cells are marked in green. Gas interchange between chorionic and endometrial capillary is represented with blue and red arrows. The presence of fibroblasts and immune cells is also depicted.
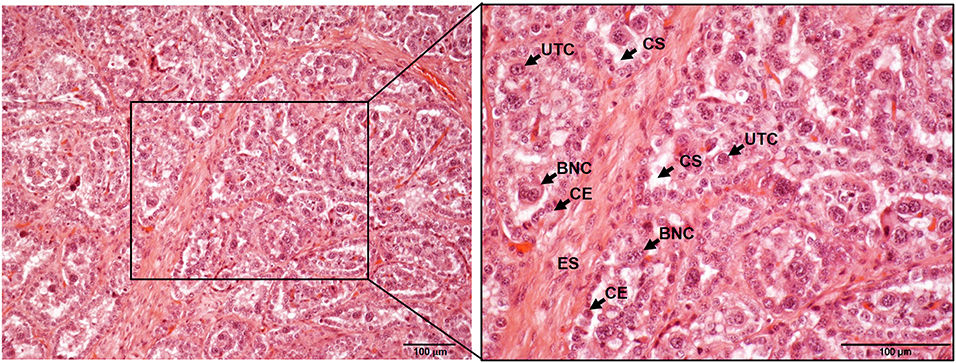
Figure 2. Histological composition of bovine placentome samples stained with hematoxylin and eosin and observed under the microscope at 10x (left) and 20x (right). ES, endometrium stroma; CE, caruncular epithelium; UTC, uninucleated trophoblast cell; BNC, bininucleated trophoblast cell; CS, cotyledonary stroma. Scale-bars: 100 μm.
Trophoblast cells are key components of the placenta, where two different populations have been described: the uninucleated trophoblast cells, which form the majority of the fetal interface and are primarily involved in nutrient exchange, and the binucleate cells (BNC), which fuse with caruncular syncytial cells and are involved in the synthesis of hormones such as placental lactogen and progesterone (7, 8) (Figures 1B, 2). The extracellular matrix (ECM) is another key component of placenta that mediates trophoblast attachment to the uterine endometrium, induces cell differentiation (9), acts as a reservoir of growth factors (10), and serves as a track on which cells can migrate along (11). ECM consists of a mixture of proteoglycans, glycosaminoglycans, and structural components such as different types of collagen, fibronectin, and laminin that are mainly synthesized by fibroblasts. These cells also produce matrix metalloproteinases (MMP), such as collagenases, gelatinases and matrilysins that degrade the ECM components. The activity of degrading enzymes is highly regulated by tissue inhibitors of MMP (TIMPs) and chemokines, cytokines, and growth factors, but the imbalance of these proteins has been associated to different pathologic conditions and with the dissemination of parasites and infected cells through the placental barrier (12, 13). Both pregnancy and delivery require a constant placenta remodeling that involves a delicate balance between ECM synthesis and degradation (4).
Regarding its functions, the placenta forms an interface between the maternal and fetal circulation, thus acting as the digestive, respiratory, excretion, metabolic and endocrine system of the fetus. Low molecular weight substances (water, electrolytes, urea, uric acid, creatine, oxygen, CO2, and some drugs) can cross the placenta by simple diffusion, other metabolites (glucose, amino acids, fatty acids) associate to specific carriers to pass the placenta, and more complex molecules (proteins, phospholipids, neutral fats) are modified to other simpler compounds before crossing the placental membrane, and then resynthesized. Gas interchange between the dam and the fetus is favored by the higher oxygen concentration and the partial CO2 pressure in the maternal circulation, but also by the higher affinity of fetal hemoglobin for oxygen (14). The placenta also secretes the hormones necessary for pregnancy maintenance, adaptation of the maternal metabolism, fetal growth, parturition and even lactation. However, the endocrine gestational profiles differ between domestic ruminant species (15).
In addition, the maternal-fetal interface constitutes a physical barrier that protects the fetus against germs and drugs that may circulate in the maternal blood stream. However, some pathogens are able to cross this barrier and infect the fetus due to their reduced size (e.g., viruses), or their ability to replicate both in maternal and fetal placental cells (e.g., T. gondii, N. caninum, Brucella abortus, Chlamydia abortus, among others), thus resulting in tissue damage and potential risk of abortion. Pregnancy success depends on a precise immune modulation able to protect the fetus from infectious agents, but also capable of providing a receptive environment for the development of a semi-allogenic conceptus. In this sense, resident immune cells, as well as trophoblast and caruncular cells, can respond to these infections by secreting cytokines and chemokines that will ultimately recruit immune cells in the damaged area (16–18). The local immune modulation that takes place during pregnancy is a complex process which has not been fully understood yet. It is known that gestation drives immune responses toward tolerance and secretion of cytokines that promote placental growth and function. This shift is characterized by reduced expression of cytokines associated with inflammation (Th1/Th17) and increased expression of cytokines that suppress inflammation (Th2) (19). Nonetheless, it has been shown that the displacement of the cytokine equilibrium toward Th1 responses under certain pathological conditions leads to an immunomediated abortion (20).
Neospora caninum and Toxoplasma gondii as Relevant Causes of Reproductive Failure in Domestic Ruminants
Neosporosis and toxoplasmosis are cosmopolitan diseases leading to reproductive failure and important economic losses in farm ruminants (21, 22). In addition, toxoplasmosis is recognized as a worldwide relevant parasitic zoonosis (23). Both diseases are caused by closely related cyst-forming apicomplexan parasites, N. caninum and T. gondii, respectively, that share many morphological and biological features (24, 25).
Neosporosis greater relevance lies in cattle, as the disease causes severe economic losses in dairy and beef industries. Abortion is the main clinical sign of bovine neosporosis. Fetuses in Neospora-infected dams may die in utero and be reabsorbed, mummified, autolysed or stillborn. Cows of any age may abort from 3 months of gestation to term, with most abortions observed at 5–7 months of gestation (21), and transplacental infections after mid-pregnancy may produce calves born alive but with clinical signs or clinically normal but persistently infected. Reproductive failure can sporadically take place in other ruminant intermediate hosts including sheep, goats, and deer (21, 26, 27). Currently, there are no effective vaccines or treatments against infection by N. caninum (28, 29) and control options are based on diagnosis, biosecurity measures and management practices (21).
Toxoplasmosis is considered a major cause of reproductive losses in sheep and goats worldwide (22, 30). Infection during pregnancy usually results in fetal death (early-gestation); stillborn or the birth of a weak lamb, sometimes accompanied by a small, mummified fetus (mid-gestation); or the birth of healthy lambs, but congenitally infected (late-gestation) (31). Toxoplasmosis has been recognized as responsible of 10–23% of ovine abortions in Europe or USA (32) and could be responsible for between 680,000 and 1,360,000 abortions annually in the European Union. In addition, small ruminants may play a major role in its transmission to humans (33). The control of ovine toxoplasmosis is primarily based on preventing its horizontal transmission via oocysts present in cat feces and on the establishment of a vaccination program with a live attenuated S48 strain (Toxovax™, MSD).
Parasite Biology
Neospora and Toxoplasma share many features in their facultative heteroxenous coccidian life cycles, including three invasive stages: (i) the rapidly replicating tachyzoites, (ii) the slowly replicating or quiescent bradyzoites harbored in tissue cysts present in brain and skeletal muscle, and (iii) the sporulated oocysts containing sporozoites. However, both parasites differ in their host range, the zoonotic potential (demonstrated only for T. gondii), and the relevance of horizontal and vertical routes of transmission in maintaining the infections in the nature (21, 34). In both cases, sexual multiplication takes place exclusively in the intestine of the definitive host, while asexual multiplication occurs in different tissues of the intermediate hosts. Infection of intermediate hosts occurs by ingestion of food or water contaminated with oocysts or tissues containing tissue cysts. Upon ingestion, sporozoites or bradyzoites are released in the small intestine and invade intestinal epithelial cells, where they transform into tachyzoites, responsible for clinical signs during the acute phase of the disease. Both parasites establish its niche inside the host cytosol by forming a parasitophorous vacuole, inside which it replicates. The lytic cycle of both parasites is a tightly regulated process which includes adhesion to the host cell, invasion, parasitophorous vacuole formation, multiplication, and egress. The succession of cycles permits the parasite to proliferate and disseminate intraorganically during the acute phase of infection and is responsible for the lesions present in the host, being a consequence of cell lysis and the immune reaction triggered locally. In fact, it is thought that this host immune pressure is required by the parasite to convert into bradyzoites and form orally infectious tissue cysts, thus favoring further transmission (21, 34).
Neospora caninum life cycle involves canids as definitive hosts (dogs, gray wolves, coyotes, and dingoes), and cattle, other ungulates and dogs as intermediate hosts. While parasite DNA and/or clinical disease have been detected in a wide range of domestic and wild animals, dogs and cattle are the most relevant hosts and maintain the domestic life cycle of the parasite (21). Despite serological evidence of parasite exposure, N. caninum is not considered to be zoonotic as presence of the parasite has not been detected in human tissues (35). Postnatal infection by ingestion of oocysts (horizontal transmission) and transplacental passage from the dam to the fetus (vertical transmission) are the only demonstrated transmission routes for cattle and other intermediate hosts. Two different forms of transplacental transmission have been described in bovine neosporosis. Reactivation of bradyzoites in persistently infected dams during gestation is responsible for endogenous transplacental transmission, considered the main transmission route in cattle. Less frequently, exogenous transplacental transmission occurs when dams are primarily infected during gestation by ingestion of oocysts. Both exogenous and endogenous fetal infection may result in abortion or stillbirth, or in most cases, in the birth of clinically healthy calves, but congenitally infected. The ingestion of bradyzoites contained in tissue cyst from infected tissues of the intermediate host is the most likely source of infection for the definitive host (21).
Domestic cats and other felids are definitive hosts of T. gondii, and only they can shed environmentally resistant oocysts with the feces upon infection. After one to few days of environmental maturation (sporulation), oocysts become infective to a large variety of warm-blooded intermediate hosts, including livestock, synanthropic species, wild animals, and humans (36). The most important route of transmission of T. gondii to small ruminants is the horizontal, occurring after oral uptake of sporulated oocysts contaminating fodder or water (36). It is generally assumed that only 2% of sheep become infected congenitally and 4% of the persistently infected sheep transmit the infection to their offspring (37). Endogenous transplacental transmission of the parasite has also been described in goats (38). Recent descriptions from Brazil seem to corroborate the relevance of this route of transmission associated to certain breeds or T. gondii strains or genotypes (39, 40).
Factors Influencing Abortion and Transmission
The pathogenesis of bovine neosporosis and ovine toxoplasmosis is complex and not yet fully understood. Both N. caninum and T. gondii tachyzoites replicate and disseminate throughout the host tissues, including the placenta. Parasites seem to first reach and infect the caruncular epithelium, then spread to the trophoblast cells within the cotyledons, and eventually reach and replicate in the fetal tissues (41). Tissue damage and altered placental functions due to parasite replication have been proposed as mechanisms triggering the abortion (2). However, considering the role of the placenta as an immune-modulating organ and the ability of the parasite replication to alter its immunological balance, an immune-mediated mechanism has been also proposed as a possible cause of abortion (42, 43). Nevertheless, clinical outcomes of both diseases are also strongly associated with the period of gestation at which primary infection occurs (44, 45). This is because the response generated against the parasites is highly dependent not only on the status of the dam's immune system, but also the immunological maturity of the fetus (41, 46, 47). In addition, susceptibility to the disease is heavily determined by the parasite strain. In N. caninum most of the isolates obtained to date have been classified as low-, moderate- and high-virulence isolates based on their in vivo (mouse models) and in vitro (non-bovine cell lines) behavior (48–51). These isolates have shown a similar behavior in pregnant bovine models, demonstrating clear variations in their ability to produce abortion and vertical transmission after experimental infections (46, 52, 53). In T. gondii, virulence is defined by the number of tachyzoites that are needed to cause mortality in the 50% of mice inoculated with the parasite (LD50). In the house mouse (Mus musculus domesticus) type I strains are very virulent (LD100 of 1 parasite), whereas type II and III strains display milder virulence (LD50 of 103 and 105 parasites, respectively) (54). However, little is known about the correlation of Toxoplasma strain virulence in mice with other animal species, including humans (55).
Models for the Study of Host-Pathogen Interactions at the Maternal-Fetal Interface
The use of animal models secures a unique opportunity to obtain a biological snapshot of the effects of infection with N. caninum or T. gondii during pregnancy, and thus, an excellent approach to analyze the interactions between the ruminant placenta and such parasites. Several pregnant cattle and sheep models have been employed so far, providing a powerful tool for the researchers working on this field. These models pose a number of disadvantages, including higher costs, longer experimental periods and reduced numbers of variables to be studied, not to mention the need to comply with the 3R principle. Consequently, different research groups have developed alternative simplified cell culture models based on the two major populations of the mature placenta: trophoblast and caruncular cells. These systems have provided useful information on the behavior of specific cell subsets when challenged with N. caninum or T. gondii, but offering a partial vision of the cellular processes that take place in the placenta, as they fail to mimic its complex structure (16, 56, 57). The use of in vitro models is mainly constrained by the lack of stimulation and activity of the immune cells resident or attracted to the placenta, the omission of host's ability to compensate for stress conditions, and the absence of the ECM, which is not only responsible for placental remodeling and function, but is also involved in the pathogenesis of some diseases, including toxoplasmosis (16, 58, 59). In this sense, and considering that explant cultures are able to retain the placental architecture and the dynamic relationship between fetal and maternal tissues, the use of ex vivo approaches seems an appealing alternative to deepen in the study of host-parasite relationships as they offer a mid-point between in vivo and classical in vitro systems (60).
In vivo Ruminant Models
The use of animal models has played an integral part of our current understanding of pathogenesis of neosporosis and toxoplasmosis, since many features of pregnancy such as the development of the uteroplacental circulation, fetal growth velocity and development, and local immune responses have no in vitro counterpart. In this section we summarize the in vivo bovine and ovine models used to study the interaction of N. caninum and T. gondii, respectively, with the placenta, both in natural and experimental animal models, and discuss their benefits and difficulties.
Natural Infection Models
Collection of placental samples from naturally infected animals would be the ideal approach to study host-parasite interactions at the maternal-fetal interface, especially at the early stages of the description of an unknown disease. However, many variables cannot be controlled in such studies, including parasite isolate and challenge dose, obstructing their interpretation.
Bovine Neosporosis
Natural infections are the only model available to study endogenous transplacental transmission of N. caninum. In naturally infected heifers, the detection of an increase in the levels of specific antibodies has been related to a reactivation on the chronic infection during gestation (61, 62). Following these findings, Rosbottom et al. (63) monitored monthly fluctuations of N. caninum-antibodies during gestation in naturally infected cows, which were euthanized when recrudescence was detected (mid-gestation) and used to study the immune response, pathological changes and parasite loads in the placenta (63). This study showed that all the fetuses were alive, but parasite had already invaded the placenta and reached the fetal tissues when recrudescence was detected. Necrosis in the fetal villi and CD4+ and CD8+ T cells infiltration was also observed, with an increase of expression of pro-inflammatory (IFN-γ, IL-12, and TNF-α) and regulatory (IL-4 and IL-10) cytokines. Authors stated that the response in the placenta was not polarized toward either a Th1 or a Th2 phenotype, which may have an important role in controlling parasite multiplication.
Ovine Toxoplasmosis
Unlike neosporosis, the horizontal transmission is the main route that T. gondii exploits to infect small ruminants in the environment. Previous works carried out with naturally infected animals focused on the study of the consequences of the infection, with little information about the interactions of the parasite with the placenta. All these studies described the presence of multifocal areas of necrosis, commonly associated with the infiltration of non-purulent lymphoid in placentomes when abortion occurred (34).
Experimental Infection Models
Most of our current knowledge of parasite-placental interactions is derived from experimental infections in animals, and fortunately these can be made in the main target species: cattle for bovine neosporosis and sheep for ovine toxoplasmosis. Despite the use of rodent-based models has been extensively reported for N. caninum, differences in the type of placentation and length of gestation prevent direct extrapolations to ruminants. Marked differences between the ovine and murine models of toxoplasmosis have been also demonstrated, both in its clinical presentation and in the immune mechanisms to control infection (64). In mice the activation of TLR-11 and TLR-12 seem essential to control the infection, but these genes do not exist in sheep, cattle and humans (65, 66). In addition, NK cells are regarded as one of the key players of the placental innate immune response of mice against T. gondii (67), but they are notably absent from the ovine placenta during normal pregnancy (68) and ovine toxoplasmosis (69). Furthermore, and contrary to what has been described in mice (70), infection with N. caninum does not cause a clear predominance of Th1 or Th2 responses in ruminants (63, 71, 72). All these differences between mice and ruminants could reflect that the first are not natural hosts for N. caninum as suggested earlier (73), thus highlighting the relevance of using ruminant models for the study of these diseases.
Knowledge on the available models is a prerequisite to reach appropriate conclusions, as interpretation of experimental findings varies between them. The Figure 3 summarizes the main ruminant models and experimental designs used to study parasite interactions with the placenta for both diseases. In all of them, experimental infection is achieved by subcutaneous or intravenous inoculation of in vitro-cultured tachyzoites (N. caninum) or by oral administration of sporulated oocyst (T. gondii) at the three terms of gestation (Figure 3A). Depiste models of oral and parental challenge have been also described for N. caninum and T. gondii, respectively, these have not been considered in this review because interactions with the placenta were scarcely analyzed and/or do not represent the main routes of transmission under natural conditions (44, 74, 75). Regardless the time and parasite used for infection, three main models can be defined (Figure 3B). Firstly, placental samples can be collected at the infection outcome, either when fetal death is detected in utero by ultrasound, or when abortion occurs. However, samples collected from abortions are often degraded with high degree of autolysis and/or display cellular and vascular changes related to placental expulsion rather than the infection. Secondly, placental samples can be obtained at a fixed time after infection, independently of the occurrence of fetal death of abortion. Lastly, a serial euthanasia at different times post-infection can be performed, allowing a thorough study of the infection dynamics, but preventing to track the progression of the parasite within the same host, which may yield relevant data. Surgical approaches such as the carunculectomy could be used to track single animals along the time, but in return, the integrity of the placental barrier could result compromised, affecting the reliability of the results.
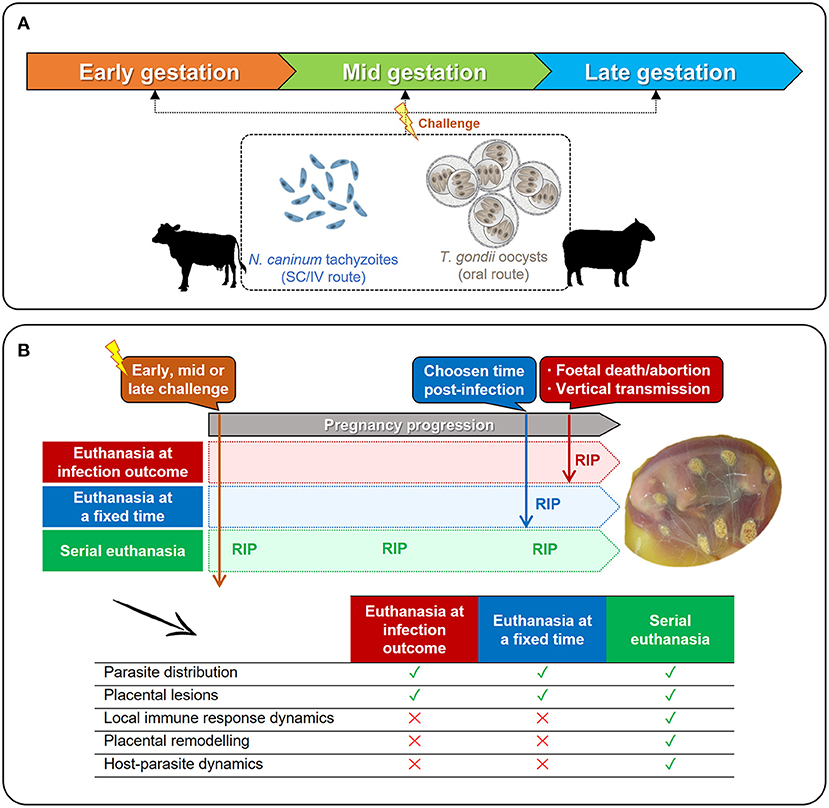
Figure 3. Available in vivo models to study the interactions between N. caninum or T. gondii and the ruminant placenta. (A) Pregnant cows or ewes can be experimentally infected at any term of gestation with N. caninum tachyzoites (subcutaneous –SC– or intravenous –IV– administration) or with sporulated oocysts of T. gondii (oral administration). (B) Regardless the time of challenge (early-, mid-, or late-gestation), pregnant females can be euthanized when clinical signs are detected (fetal death, abortion) or right after delivery; or can be serially euthanized after infection, before the appearance of clinical signs. As shown in the table, the time of euthanasia (RIP) will determine which parameters can be studied, and which cannot.
It is worth to mention that the success of these experimental models also relies on the selection of appropriate animals. Although high individual variations are expected, uniform animals in terms of breed, weight and age should be selected. In addition, animals should be preferably primiparous and free from the main reproductive infectious pathogen agents: N. caninum, Brucella abortus, bovine viral diarrhea virus, Leptospira interrogans serovar Hardjo and infectious bovine rhinotracheitis virus for cattle; and N. caninum, T. gondii, border disease virus, Schmallenberg virus, Brucella spp., Coxiella burnetii, and C. abortus for sheep. Moreover, selected animals could be vaccinated against the main abortifacient agents, and then submitted to estrus synchronization and artificial insemination or natural mating. Once pregnant, females should be housed in dog- and cat- proof facilities and handled with care until implantation occurs. At the beginning of pregnancy, pregnant females require specific handling and feeding necessities and tolerate stress worse than non-pregnant ones, which can affect negatively on the implantation process. All these requirements involve higher costs, as experienced staff and large facilities are needed, implying that only few institutions can carry out such experiments.
With regard to neosporosis, a comprehensive review of the ruminant models used for neosporosis was published in 2014 (46). This paper highlighted the need of developing a normalized model of exogenous transplacental transmission (isolates, inoculation route, inoculum size, ruminant species, breed, infection times at pregnancy, sample collection timelines) to allow comparisons between studies performed by different research groups. In the last years, we have focused our efforts on the normalization of some variables including parasite isolate, challenge dose, and route of inoculation. The Nc-Liverpool, Nc-1, Nc-BPA1, Nc-Illinois, and Nc-6 Argentina isolate have been traditionally employed to perform experimental infections, showing a broad range of clinical outcomes. However, none of these isolates have been either extensively characterized or maintained in a low number of passages to preserve their traits. For this reason, we proposed the high virulence Nc-Spain7 as a reference isolate, as it complies with all the above (48, 49, 53, 76, 77). Several bovine experimental infections using this isolate have been carried out in cattle at different times during gestation and under the same experimental design, which has allowed direct comparison of the results (53, 76–78). Specifically, intravenous inoculation of Nc-Spain7 tachyzoites showed a 100% of fetal death in a bovine model at early gestation (76, 77) and about 50% at mid-gestation (78). The intravenous route has been the most frequently used for experimental infections with N. caninum, as it simulates the hematogenous spread of the parasite after a primary infection or reactivation, likely allowing the parasite to reach the placenta quickly and in higher numbers. In contrast, parasites administered subcutaneously would target the regional lymph nodes first, and then would spread throughout the body, with lower numbers of parasites reaching the placenta. Intravenous inoculation of a high dose of tachyzoites (5 × 108) of the Nc-1 isolate on day 70 of gestation induced 100% mortality, and this was reduced by half in subcutaneous inoculation (79), highlighting the relevance of using the first to mimic the most common route infection under natural conditions. The same research group compared the effect of inoculation of 107 and 5 × 108 Nc-1 tachyzoites by subcutaneous injection at day 140 of gestation. Both inocula induced similar outcomes, although the higher dose elicited earlier and more extensive lesions in the placenta (80). More recently, we have compared the effect of different doses of the Nc-Spain7 isolate, showing a dose-dependent effect on parasite loads in placenta and fetal brain, and in the median fetal survival times (81). A dose of 107 tachyzoites intravenously administered at mid gestation was proposed to be adequate at modeling bovine pathogenesis because of its high abortion rate, placenta infection and parasite vertical transmission.
Similarly to what was described for N. caninum, experimental infections with T. gondii have been carried out under a large amount of different experimental designs (34). This prevents direct comparisons between studies and urges to the development of a normalized model of horizontal transmission. The reference type II isolates M1, M3, M4, and ME49 have been traditionally employed to perform experimental infections with T. gondii (82, 83). However, these isolates have been maintained by successive passages in cell culture and mice, in some cases for decades, and it is largely unknown if this have had any impact on their virulence traits as demonstrated for other type I isolates such as RH (84, 85). In fact, a recent study has demonstrated marked differences between the reference isolate ME49 and the recently obtained TgShSp1 isolate in terms of their in vitro behavior and virulence in mice, a model that has been historically used to classify all the T. gondii isolates into the three main clonal lineages, I, II, and III. Remarkably, susceptibility to neonatal mortality was higher in sheep than in mice, which corroborates that both the isolate and the animal model determine the outcome of infection, and as such, mice may not be a reliable indicator for disease severity (64).
Regarding the parameters that can be measured for studying parasite-placental interactions, these have been traditionally restricted to parasite detection and/or description of lesions in the placenta. Recent works have deepened in such interactions by investigating local immune responses in placentomes to elucidate its role in the clinical outcome of the disease, or in the control of parasite transmission (16, 53). However, current trends of research are moving forward the implementation of “omics” techniques (i.e., transcriptomics, proteomics, etc.) as they supply a global view of all processes altered during infection and potentially related to fetal death (58, 86).
Models for Bovine Neosporosis
Primoinfection models are the only experimental approach available to study bovine neosporosis, and they only mimic the exogenous transplacental route of transmission of N. caninum. In these models the time of gestation at which challenge is done determines the occurrence of fetal death or parasite vertical transmission, as well as the severity of pathological and immunological changes in the placenta (46).
Intravenous inoculation of heifers with tachyzoites of virulent isolates at the first term of gestation (65–70 days) is generally associated with a rapid fetal death due to the relative immunological immaturity of the fetus (46), and thus this model can be used to study pathological and immunological changes occurring in placentomes when fetal death occurs (Figure 3B). The results derived from these studies are quite homogeneous, with placentomes from aborted animals widely colonized by tachyzoites and displaying severe necrotic and extensive inflammatory lesions. With regard to cytokines expression at the maternal-fetal interface, an exacerbated pro-inflammatory response (particularly IFN-γ) and high expression of anti-inflammatory/regulatory cytokines (such as IL-10 or IL-4) were observed (76, 77, 87, 88). Moreover, a positive association between high number of T lymphocytes in placenta (CD3+, CD4+, CD8+, and γδ) and occurrence of abortion has been demonstrated by immunohistochemistry (IHC) (89). More recently, gene expression of the endosomal toll-like receptors (TLRs)-3, TLR-7, and TLR-8 by quantitative reverse transcription PCR (RT-qPCR) was described in the caruncle from cattle experimentally infected with N. caninum at day 70 of gestation and euthanized 1 month later (90), suggesting that the initial recognition of the parasite would occur in the maternal part of the placenta. Sequential euthanasia and sampling of cattle (Figure 3B) after intravenous challenge at early gestation, showed the presence of moderate to severe pathological changes and tachyzoites within areas of villous necrosis at 14 days post-infection (dpi) (79, 91). At 28 dpi, fetal death has occurred, and cotyledons were detached from caruncles, detecting N. caninum antigen in the cotyledons. When the immune response was studied by IHC, infiltration of CD4+ cells and NKp46+ cells was detected in the caruncles at 14 dpi, whereas infiltration of γδ TCR+ cells were observed from 28 dpi onwards (91). In-situ hybridization experiments demonstrated a more severe infiltration of IFN-γ expressing cells at 42 dpi, whereas the infiltrate of IL-4 expressing cells was scarce at 28 and 42 dpi, mainly in the caruncle (92). All these studies support the hypothesis that infection in early in gestation triggers a Th-1 type response in the placenta, which together with parasite replication and tissue destruction, may contribute to fetal death.
Experimental infections at mid gestation (110–140 days of gestation) can induce either foetopathy or birth of congenitally infected calves. It has been suggested that this model can be useful to understand why some dams abort and some do not. Here, the euthanasia of dams at a specific time of the experiment (Figure 3B) has been the experimental design most exploited so far. When the immune response was determined in placentomes from infected dams carrying live fetuses, Th1, Th2, and Treg cytokines were up-regulated, while TGF-β was down-regulated. This cytokine expression pattern could be beneficial to fetal survival, but could also promote transplacental transmission (93). When the immune response was determined in placentomes from aborted dams or dams carrying non-viable fetuses at the euthanasia, Th2 and Treg cytokines were down-regulated (71). Recently, cytokines expression pattern in Nc-Spain7 infected animals at mid-gestation, carrying non-viable fetuses and euthanized at 20 dpi, showed an up-regulation of IL-8, iNOS, and TNF-α, previously associated with placental inflammation, luteolysis and abortion (16). Other studies have described a reduced expression of SERPINA14 (linked to maternal immunosuppression during pregnancy) in aborting dams, and a negative relationship with IFN-γ expression in cotyledon samples (94). A recent work estimated the relative cell densities at the fetal-maternal interface from dams infected at day 110 of gestation, demonstrating lower proportions of bi- and mono-nucleate trophoblast cells after N. caninum infection at 42 dpi, and suggesting that this could be mediated by the Th1-type response of the placenta, promoting the destruction of fetal-genotyped cells (72). When infection dynamics were studied at mid gestation by serial euthanasia, the parasite was detected by PCR and IHC in the placenta as early as 10 dpi (53). Fetal death was detected from 20 dpi onwards (53, 80, 91, 95). Focal lesions and immune cell infiltration were observed in some placentomes at 14 and 28 dpi, and such lesions were resolved at later time points (80, 96). In non-aborting animals, there was no evidence of parasite antigen or infiltration of immune cells at 28, 42, and 56 dpi, suggesting that the parasite had not reached the placenta (91). In situ hybridization showed a scarce to mild infiltrate of IFN-γ, IL-4 expressing cells in the maternal caruncle at 14 and 28 dpi (92). Altogether these results suggest that infection at mid-gestation can be partially controlled by some dams, although the factors governing this remain unknown.
Experimental infection of cattle in the last third of gestation leads to the birth of healthy but congenitally infected calves. This model is useful to understand the basis of transplacental transmission and fetal survival. In placentomes obtained from animals euthanized 21 dpi, N. caninum was sporadically detected by PCR, very mild lesions with focal necrosis were found, and a modest expression of Th1 and Th2 cytokines was detected by RT-qPCR (87, 88). The presence of CD4+ and CD8+ cells was relatively low, and they were sparsely disseminated (88). Examination of placental tissues at different times post-infection evidenced the presence of parasites and focal necrotic lesions at 28 dpi, the infiltration of inflammatory cells at 42 dpi, and fibrosis at 56 dpi, which denoted lesion resolution. Animals euthanized at 14, 28, 42, and 56 dpi contained a scarce infiltrate of IFN-γ and IL-4 expressing cells, mainly in the caruncle (92). These findings indicate that infection at late gestation is less pathogenic at the maternal-fetal interface, which could partially explain the reduction on the severity of the clinical outcome after infection.
Pathological changes of N. caninum-challenged pregnant cattle at 70 days of gestation and previously immunized with live or inactivated experimental vaccines were also studied at the maternal-fetal interface. Heifers were culled at day 104 of gestation and placentomes were examined to evaluate lesions and local cellular immune responses using histopathology, IHC and RT-qPCR. Vaccination with live tachyzoites induced protective immunity, and thus, subsequent challenge only caused minimal inflammation, milder immune cell infiltration and a lower expression of cytokines. In contrast, animals vaccinated with inactivated vaccines displayed an up-regulation of Th1 and Th2 cytokines and a strong cellular immune response after challenge (97).
The influence of the N. caninum isolate on the pathological and immunological changes occurring in placentomes has been also studied in bovine experimental models. Inoculation of Nc-Spain1H and Nc-1 tachyzoites at early of gestation, resulted in death in three out of five fetuses from Nc-1 infected dams whereas that Nc-Spain1H did not have the ability to induce foetopathy. At the maternal-fetal interface, Nc-1 induced the most severe histopathological lesions and parasite DNA was also more frequently detected (52). In other studies using the same model, the high virulent isolate Nc-Spain7 induced fetal death earlier, severe placental damage and higher parasite load in caruncles than the moderate virulence Nc-1 (76) or Nc-Spain8 (77) isolates. In addition, higher IFN-γ expression was induced by Nc-Spain7, which could be detrimental for gestation, whereas a higher IL-10 upregulation was observed in Nc-Spain8 infected, which would have anti-inflammatory effects (77). More recently, we used a model of serial euthanasia for studying initial events of infection (10 and 20 dpi) at mid-gestation (53) to study the mechanisms that enable some isolates to be more effectively transmitted or cause fetal death. Infection with the low virulence Nc-Spain1H isolate triggered an early (10 dpi) innate immune recognition in the bovine placenta, characterized by upregulation of genes involved in pathogen recognition (e.g., TLR-2, TLR-3, TLR-8, TLR-9, and NOD2), inducing a solid Th1 response which were counterbalanced by a higher expression of anti-inflammatory and regulatory cytokines, minimizing placenta pathology. Conversely, signaling through pattern recognition receptors (PRRs, i.e., TLR-9, TLR-8) or cytokines (i.e., IL6, TGF-β1, IL-17A) was impaired at the placenta by the high-virulence isolate (Nc-Spain7), which may indicate the existence of an evasion mechanism that may favor an early multiplication and dissemination to the fetus. At the later stage (20 dpi), PRRs activation and a dramatically enhanced expression of the pro-inflammatory cytokines were observed. Altogether, this study demonstrated that the early activation of the innate immune response is crucial for the adequate control of N. caninum at the placenta, whereas an exacerbated pro-inflammatory immune response can lead to severe tissue damage, resulting in abortion. We went deeper in the study of the pathogenesis of the infection with these isolates of differing virulence using this model, by means of studying some components of ECM involved in maintaining placental homeostasis by IHC and RT-qPCR. Here, the low-virulence isolate promoted an ECM remodeling and tissue repair processes. However, in the placentome from animals infected by the highly virulent isolate, a profound alteration in ECM organization was observed, characterized by loss of fibronectin, vimentin and collagen in necrotic foci detected by IHC and downregulation of metalloproteases MMP-2, MMP-13, MMP-14, and their inhibitors. Recently, the lectin-binding pattern in the placentas of cows infected experimentally with N. caninum was studied by IHC techniques (98), suggesting the importance of the changes occurred in the ECM during N. caninum infection. In the last years, high-throughput technology has been used to generate relevant information on the immunological and cellular hallmarks of the host-parasite interaction at the placenta, aiming to facilitate further investigation on the key pathways influencing protection or abortion. Proteomic profiles comparison of the cotyledon and caruncle samples from previous experimental infections (53) has highlighted complement and coagulation routes, oxidation-reduction processes and ECM reorganization, among other biological processes, as key points in the pathogenic mechanism of N. caninum abortion (99). These mechanisms could play an important role and should be investigated in depth.
Models for Ovine Toxoplasmosis
Regardless the gestation term at which challenge was done (Figure 3), most of the infections documented so far have extended the length of the experiments until the occurrence of abortion, usually around 30 dpi. These studies described an extensive damage of the placenta caused by proliferating parasites, with lesions similar to those described in natural infections (multiple small foci of necrosis and non-purulent inflammation) (34, 44, 100). In these abortions, parasitic debris were observed in the maternal caruncle, whereas parasitic vacuoles were found in the trophoblasts of fetal cotyledon. This suggests that parasites are first recognized in the maternal part of the placenta where they stimulate the maternal immune response, and then spread to the fetus through infection of the fetal trophoblast villi, where they remain viable (69).
As opposed to the well-known “classical” abortion, early abortions occurring during the acute phase of the infection have been also described in some occasions, although they have not been taken into consideration until recent days (44, 64, 82, 101, 102). These works described early abortions in ewes infected at different stages of gestation, with different isolates (M4, ME49, and TgShSp1) and doses of inoculation. Although there was a correlation between the dose of infection and the rate of early abortions, a dose of just 50 sporulated oocysts was capable to cause a high rate of abortions in the acute phase of the disease and even 10 sporulated oocysts of the ME49 isolate caused early abortion in one animal (64, 82). In all cases, parasites were virtually not found in placental or fetal tissues, indicating that the pathogenic mechanism triggering early abortion is different from that observed in later abortions. Histopathological analyses revealed thrombosis and infarcts in the placentomes and ischemic lesions (periventricular leukomalacia) in the fetal brain, probably as a consequence of placental lesions (47, 82, 103). IHC analyses showed an increase of macrophages in caruncular septa, which could indicate that early abortions are immune-mediated. Nevertheless, more studies are necessary to corroborate this hypothesis and to find out the origin of the placental thrombosis observed. In this sense, experimental models of serial euthanasia at early stages post-infection along with high-throughput approaches to dissect host-parasite interactions could prove useful.
Recent attempts to reproduce the classical late abortions described in natural infections have failed, and early abortions have turned into the predominant clinical presentation of experimental toxoplasmosis despite they have been virtually neglected from the literature until now. Specifically, infection with 2,000 and 500 sporulated oocysts resulted in almost 100% of early abortions. This rate was significantly reduced with lower challenge doses but, surprisingly, this reduction was not counteracted by an increase on the rates of late abortions, and infections with 50 sporulated oocysts or less resulted in the birth of healthy lambs, stillbirths and very few late abortions (64, 82). Therefore, the development of a model able to mimic the classical late abortions described in natural infections is an urgent need. Factors such as breed, previous immunization or even individual susceptibility should be considered in new experiments.
A number of studies aimed to investigate T. gondii infection dynamics through models of serial euthanasia (44, 47). However, these works failed to describe the mechanisms underlying early abortions (infected ewes were euthanized from 10 dpi onwards once most of those abortions had occurred) and focused on the late ones. Although in very few animals, T. gondii was detected in placenta as early as 10–12 dpi; in addition, percentages of detection and parasite loads were higher as the infection progressed. Development of lesions was observed as soon as 10 dpi, but only in ewes subcutaneously inoculated with tissue cyst at 60 or 90 days of gestation (44), an uncommon way to acquire the infection in the nature. These lesions were characterized by several foci of necrosis that became larger as the infection progressed. In ewes orally infected with sporulated oocysts, typical lesions were found in placenta from 26 dpi, always associated to the presence of the parasite, and were characterized by necrotic foci in the caruncular part and infiltration of macrophages and T lymphocytes within the fetal chorionic villi (69, 82).
Similarly to bovine neosporosis, the time of gestation at which infection occurs also influences lesion severity, parasite distribution and expression of cytokines at the placenta, but very few studies have addressed these matters. As early as 1986, Buxton et al., analyzed the placental lesions developed in pregnant ewes after subcutaneously inoculation of 200 tissue cysts of the M1 isolate at 40, 60, and 90 days of gestation and at the infection outcome (44). This study described the occurrence of necrotic lesions in caruncular septa, which were more severe in ewes infected at mid-gestation and in those where fetuses were dead. More recently, similar studies were done using pregnant ewes orally inoculated at early- (40 days of gestation), mid- (90 days of gestation), and late-gestation (120 days of gestation) with sporulated oocysts of M4 isolate (17, 47, 69, 100). Infection at mid-gestation also resulted in the most severe lesions and highest parasite burdens, both in the maternal and fetal placenta. However, ewes infected at late-gestation showed the earliest development of lesions and colonization of the placenta by the parasite (47). Castaño et al. hypothesized that these variations could be due to differences in the immune modulation along the pregnancy. While pro-inflammatory cytokines (IFN-γ and TNF-α) were equally increased during the three terms of gestation, IL-4 was mainly increased at the first and second terms, and IL-10 at the last term of gestation. Besides, a regulation of IL-12 expression was not observed at any term (17). This imbalance on the Th1 and Th2 responses could play a key role on the pathogenesis of toxoplasmosis, but this issue remains controversial among the scientific community.
In vitro Models
Over the last years, a number of in vitro human placenta models have been developed and widely used for studies in different animal and human parasites (104), and similar efforts have been performed to obtain both primary and established cell lines from the bovine and ovine placenta (Table 1).
Despite the available number of such cell lines is considerable, most of them are not suitable for conducting studies on host-parasite interactions. This is because they are usually contaminated with Mycoplasma spp. and the bovine viral diarrhea virus (BVDV). Infection with BVDV is common in bovines and not routinely tested, but its presence in cell culture has demonstrated to alter host-pathogen interactions in an in vitro model of Besnoitia besnoiti infection (129). Considering this, and to ensure results reproducibility and reliability, selection of appropriate cell lines is paramount. In this section we discuss the application of available and suitable in vitro systems for the study of the pathogenesis of neosporosis and toxoplasmosis, including their advantages and disadvantages and the emerging opportunities that arise from their use.
Bovine Placenta Cell Lines
Two different cell lines, BCEC-1 and F3, both derived from bovine placentae and representing the main maternal and fetal cells that constitute the placental barrier have been successfully used to perform in vitro infections with N. caninum. The caruncular cell line BCEC-1 (bovine caruncular epithelial cell line-1) was isolated from a pregnant cow at 4 months of gestation, proved to have maternal origin, and maintained cell morphology, protein expression profile and barrier integrity for at least 32 passages (118, 119). A few years later, the bovine trophoblast cell line (F3) was isolated from cotyledons of pregnant cows at 5 months of gestation. This cell line was proved to have fetal origin, displayed epithelial morphology, supported the development of BNCs in small numbers through all passages, and maintained these features beyond 45 passages (116). The use of both cell lines has helped to unravel several aspects of host-parasite interactions in neosporosis.
Study of the Lytic Cycle in N. caninum
During the course of a natural infection, parasite survival and propagation heavily rely on successful invasion and proliferation within receptive host cells as part of the parasite's lytic cycle (130). Thus, the study of such processes in target cells appears as an ideal tool to study host-parasite interactions at the placental barrier. Our previous work demonstrated that N. caninum actively replicates in caruncular and trophoblast cells, confirming the high tropism of the parasite for the placenta observed in vivo (56). However, we found interesting differences in the behavior of N. caninum between both cell lines. Immunofluorescence assays showed that parasites displayed a lower adhesion capacity to caruncular cells, and consequently, the infection rates and percentages of cells with multi-infection were lower in BCEC-1 cells. In addition, parasite proliferation capacities, measured by RT-qPCR, were also lower in those cells. These results reflected that susceptibility to infection is higher in the fetal part of the placenta, which is widely supported by previous results obtained from experimental bovine infections (16, 76, 77). In view of these, we proposed that the maternal part of the placenta acts as a barrier for parasite infection, reducing its adhesion and subsequent intracellular multiplication.
On the other hand, pathogenesis of N. caninum infection is heavily influenced by the virulence of the isolate employed. We hypothesized that differences in lytic cycle progression among different isolates could explain the differences described in vivo using a bovine model (52, 76, 77). In an attempt to address this possibility, the lytic cycle of the high-virulence isolate Nc-Spain7 and the low-virulence isolate Nc-Spain1H was compared using the BCEC-1 and F3 cell lines (56). Differences between isolates were remarkable in trophoblast cells, where Nc-Spain7 displayed higher invasion and proliferation rates than Nc-Spain1H. By contrast, the behavior of both isolates was very similar in caruncular epithelial cells (Figure 4). This higher proliferation capacity in trophoblast cells could explain why in heifers experimentally infected with the Nc-Spain7 isolate show higher parasite burdens in fetal tissues, and consequently, more severe clinical signs and histopathological lesions, resulting in higher ratios of fetal death and abortion.
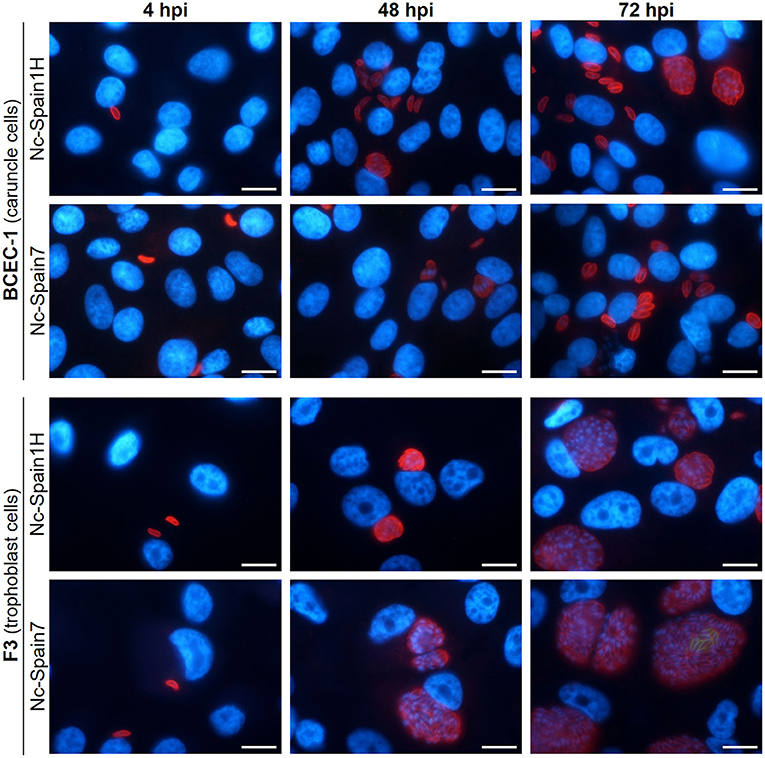
Figure 4. Tracing the lytic cycle of high- (Nc-Spain7) and low-virulence (Nc-Spain1H) isolates of N. caninum in BCEC-1 and F3 cells at 4, 48, and 72 h post-infection (hpi) through immunofluorescence assays. Host nuclei were stained in blue, while N. caninum tachyzoites were stained in red. Note the obvious differences in the parasitophorous vacuole size between BCEC-1 and F3 cells infected with any of the isolates, which is due to an early egression of the parasites in the first. While no differences were observed in parasitophorous vacuole size between BCEC-1 cells infected with Nc-Spain1H or Nc-Spain7 tachyzoites, remarkable differences were evidenced in F3 cells, with bigger vacuoles in Nc-Spain7 infected cells compared to those infected with Nc-Spain1H. Note the beginning of egress in F3 cells infected with the Nc-Spain7 isolate at 72 hpi (stained in green). Scale-bars: 10 μm.
Study of the Host-Cell Modulation by N. caninum Infection
It has been widely demonstrated that parasites and many other pathogens can manipulate host-cell machinery for their own benefit in order to perpetrate the infection by scavenging nutrients from the host, or even by inhibiting apoptosis or host immune responses. In addition, it has been shown that the pathogenesis of bovine neosporosis is not only related to parasite multiplication itself, but also to the host immune response triggered following infection (131). Therefore, the study of the host-cell modulation upon N. caninum infection could contribute to deepen on the knowledge of the disease.
The application of classical techniques such as RT-qPCR and commercial bovine cytokines ELISA tests to this in vitro infection model have proven to be very useful to study the response triggered by N. caninum in bovine target cells. This was revealed by studying the expression levels of cytokines, chemokines and other immune-related elements in F3 and BCEC-1 cell cultures infected with high- (Nc-Spain7) and low-virulence (Nc-Spain1H) isolates at early (4 h) and later (24 h) stages of infection (57). Similarly to what has been proven using in vivo models, this work described the development of a typical pro-inflammatory Th1 response by the bovine host against the parasite. Surprisingly, and despite the different phenotypes observed between BCEC-1 and F3 cells in terms of parasite adhesion, infection rates, and proliferation capacities, few differences were found in their responses against the infection. The main difference was the up-regulation of TLR2 in infected BCEC-1 cells, not found in F3 cells. This phenomenon could be in part responsible of the resistant phenotype found in caruncular cells, as TLR activation is crucial for initiating the immune responses against intracellular parasites such as N. caninum. However, the different TLR-2 activation did not influence the expression of TNF-α, IL-8, and IL-6, usually triggered by transcription factors also activated by this receptor. Regardless of TLR-2 expression levels, the pro-inflammatory cytokines TNF-α and IL-8 were in general up-regulated upon infection in both cell lines. Surprisingly, IL-6 expression levels were diminished in infected cultures of BCEC-1 and F3 cells. These results deserve further investigation, since a marked IL-6 up-regulation has been described upon N. caninum infection in vivo (16, 93, 132). On the other hand, TGF-β1 was found down-regulated in BCEC-1 and F3 cells. It is postulated that this factor might contribute to fetal death during infections, as it neutralizes the pro-inflammatory responses induced by Th1-type cytokines whose activity is detrimental for the gestation. When immune modulation was individually compared after infection with high- or low-virulence isolates, only differences on TLR-2 and TNF-α expression levels were found, with the Nc-Spain1H isolate inducing higher expression of TLR-2 and TNF-α than the Nc-Spain7. This could be translated into a better control of parasite proliferation by the cells infected with the Nc-Spain1H isolate, or the opposite in those infected with the Nc-Spain7 one (57).
Despite this model has demonstrated to be useful to unravel some aspects of N. caninum pathogenesis, its use dismisses the close interaction of caruncular and trophoblast cells with other immune cells present in the placenta. This is clearly reflected by the lack of detection or down-regulation of cytokines such as IL-17, IFN-γ, IL-4, IL-10, and IL-12, whose relevance in the response against N. caninum in bovine models has been long-established (16, 93, 132). These limitations could potentially be overcome by exogenous stimulation of F3 and BCEC-1 cultures with cytokines such as IFN-γ present at the placenta, or even by co-culturing these cell lines with other relevant bovine immune cells such as macrophages. Additional alternatives such as the use of placental explants are discussed below.
Although the use of classical methods has provided relevant information on the expression and abundance of many genes of interest upon infection with N. caninum, they fail to provide a comprehensive view of the global changes that infection might trigger on host target cells. In this regard, recent advances in high-throughput technologies have provided an opportunity to perform a global analysis of potential changes related to infection using trophoblast cells (58, 86). Infections of F3 cells with high- (Nc-Spain7) and low-virulence (Nc-Spain1H) isolates demonstrated that the parasite modulates processes such as the ECM reorganization, cholesterol biosynthesis and the transcription factor AP-1 network after just 8 hpi. Specific isolate-modulated processes were not identified, although infection with the low-virulence isolate exerted a higher modulation of the host cell (207 differentially expressed genes) compared to the high-virulence one (126 differentially expressed genes) (58). Subsequently, and in order to depict the infection dynamics throughout the tachyzoite lytic cycle, the proteome of N. caninum-infected F3 cells was studied by LC/MS-MS during early infection (8 hpi), parasite multiplication (36 hpi), and egress (56 hpi). Proteome changes revealed protein synthesis, protein turnover, and metabolism as the main host pathways disturbed by infection. Similar findings have been reported for other parasites, as manipulation of host metabolic events determines infection establishment and the initiation of host immune responses (58, 133–135). As observed in the transcriptomic study, infection with the low-virulence isolate Nc-Spain1H exerted a higher perturbation on F3 cell proteome with the exception of those profiles enriched in mitochondrial proteins, which were equally altered by high- and low-virulence isolates. This reflects the importance to govern this organelle during infection, which has been broadly demonstrated not only for N. caninum, but also for T. gondii (136–139). Nevertheless, the levels of perturbation of the host cell depending on the isolate's virulence deserves further investigation, as this could be a strategy to achieve a more successful transmission (higher perturbation by low-virulence isolates), or to avoid detection by the host's immune response (lower perturbation by high-virulence isolates). Unfortunately, there is a lack of similar studies performed in bovine caruncular cells, where the parasite has shown an uncommon behavior not described in other cells lines (49, 140). This could provide valuable information about the interactions between N. caninum and the maternal side of the placenta.
Ovine Placenta Cell Lines
Sheep are susceptible to infection by both N. caninum and T. gondii (100, 141). Even considering their limitations, the use of in vitro models of ovine placenta would provide useful information that could be extrapolated to the natural conditions of infection by both parasites. However, the number of studies that have adopted this model is strikingly scarce to date. Back in 2006, the AH-1 cell line was originated from the ovine placenta to perform in vitro infections using N. caninum tachyzoites (127). This cell line was developed from fetal cotelydon cells obtained from a near-term pregnant Suffolk ewe by transformation with the SV40 large T antigen. AH-1 cells displayed a morphology consistent with that observed in freshly obtained trophoblasts, and were able to produce IFN-τ, a cytokine nearly unique to the ruminant placenta. Haldorson et al. also demonstrated that antibodies directed against the surface protein NcSRS2 were able to inhibit N. caninum cell attachment and invasion in vitro. Nevertheless, to our knowledge this is the only study where the AH-1 cell line has been used to deepen on the pathogenesis of N. caninum. This contrasts with other works focused on the abortifacient agents C. abortus and Waddlia chondrophila, which are able to infect and grow in this cell line, which in return triggers a typical pro-inflammatory response (142, 143), demonstrating its great potential to study not only N. caninum, but also T. gondii.
Regarding T. gondii, and considering its zoonotic nature, many in vitro studies have been performed using human placenta cell lines. Among them, the BeWo cell line (human choriocarcinoma cells) has been the most widely used for studies on the parasite's pathogenic mechanisms, host immune response or drug efficacy upon T. gondii infections (144–146). Since abortion is the main consequence of T. gondii infection both in humans and ovine, studies based on BeWo cells could guide future research in ovine target cells. In this sense, the use of the AH-1 line could be a good starting point, but further efforts should be made to obtain an established cell line representing the maternal side of the ovine placenta. We have recently characterized the lytic cycle of six T. gondii isolates newly obtained from ovine tissues on infected AH-1 cells, and we can certainly corroborate their usefulness for this end (data not published).
Ex vivo Models
The term explant refers to a piece of a living tissue or organ that once removed from an animal and transferred to artificial culture conditions is able to maintain its functions for a short period of time, thus allowing to perform controlled experiments on it. During the last 80 years the use of placental explants for research in human medicine has been extensively reported, and has enabled the study of the placental physiology and certain pathologic conditions such as preeclampsia and gestational diabetes (147). In fact, it has been demonstrated that these explants are able to maintain their degree of cell differentiation and secrete hormones and cytokines under artificial culture conditions (148). This contrasts with the scarce works carried out with ruminant placental explants, which reflects the difficulties in obtaining placentas suitable to tissue culture from those farms where births take place.
Practical Applications of Placental Explants
To date, the use of placental explants has not been reported to study the effect of N. caninum and T. gondii in ruminants. However, the literature available supports their employment as a valuable approach capable of dissecting the pathogenic mechanisms and host responses triggered upon infection, thus overcoming the limitations of caruncular or trophoblast cells-based in vitro cultures. This is further backed up by several studies where human placental explants have been infected ex vivo (Table 2).
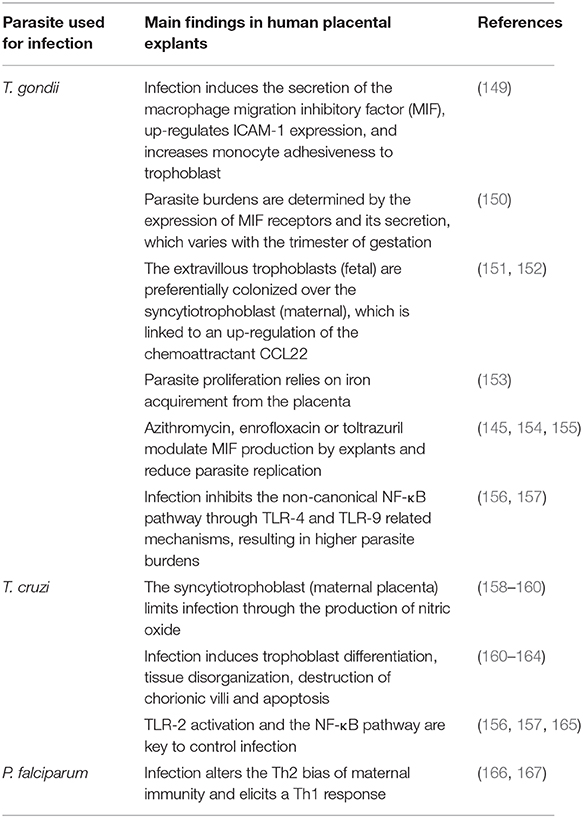
Table 2. Compilation of studies where human placental explants have been employed to study host-parasite interactions ex vivo.
Very few studies have employed cow explants to study specific aspects of the placental physiology (168). In contrast, recent endeavors to obtain inexpensive tools to study the consequences of xenobiotics exposure on the maternal-fetal interface have resulted in the development of an ex vivo model based on sections of cow placentomes (169). In fact, this model has been applied to describe the effect of environmental endocrine disruptors on the bovine placenta (170). Of more interest for this review is the use of bovine placental explants to extend what is known about the pathogenesis of B. abortus-induced placentitis and abortion in cattle. The experiments carried out with extraplacentomal chorioallantoic explants from early and late gestational stages showed that bacterial replication was significantly higher in last term tissues (171). Further studies demonstrated that infection with B. abortus suppresses the pro-inflammatory innate immune response triggered by trophoblastic cells (172, 173). Similar approaches have been followed to study the pathogenesis of Listeria monocytogenes and Listeria ivanovii, also responsible of abortion and reproductive failure in cattle. Both species displayed similar levels of invasion and intracellular multiplication of chorioallantoic explants without inducing cell lysis. Oppositely to B. abortus, no significant changes on host pro-inflammatory responses were observed after infection (174).
Regarding ovine ex vivo models, the number of works where placental explants have been employed is limited, and most of them have focused on the study of the placental physiology (175). Nevertheless, it is worth to mention a recent work in which the effect of ex vivo infection with Trypanosoma cruzi and T. gondii was compared between three different placental barriers: human, canine and ovine. This study demonstrated that the parasite burden seemed to be independent of the placenta's complexity. Besides, this burden was always higher in those explants infected with T. gondii, where the tissue damage tended to be more severe (176).
In contrast to ruminant placental explants, human extravillous explants have been extensively used during the last decades to study many aspects of placental physiology and pathology. This has been favored by the relative ease with which these tissues can be obtained during scheduled cesarean sections or pregnancy terminations. Parasites such as T. gondii, T. cruzi-the etiological agent of Chagas disease-, and Plasmodium falciparum-responsible of placental malaria- are a significant cause of placentitis, premature labor, miscarriage and low birth weight in humans. The major findings of these works are summarized in Table 2 in an effort to serve as a route map for future research that could be applied in the field of neosporosis and toxoplasmosis in ruminants.
In summary, the development of ex vivo models for the bovine and ovine placenta opens up new prospects to study in depth the intricated relationship between apicomplexan parasites and their hosts during pregnancy, and this will help to understand the consequences of such infections to the developing fetus.
Placental Explants: Pros and Cons
The employment of an ex vivo approach to model placental alterations features diverse advantages and drawbacks that have been extensively reviewed earlier (177). The explant-based models benefit from maintaining the tissue architecture and the extracellular matrix between all the cell-types that shape the placenta, thus reproducing in vivo physiologic conditions better than in vitro systems (178). Cells can keep the physical contact with the basal lamina, allowing the reception of paracrine signals from the underlying layers, and the presence of immune cells enables the study of unique immunomodulation processes that could be unnoticeable through in vivo approaches and undetectable in vitro. This makes the explants an excellent tool for studying parasitic infections and local antiparasitic mechanisms as demonstrated previously (179). The use of placental explants also offers several advantages including an increased number of variables to be tested in a single experiment, shorter experimental times, and reduced individual variability between experiments. In addition, explants could be isolated from placentas at different terms to investigate the impact of gestational age in a given parameter (148, 177). From an ethical point of view, the utilization of ex vivo models meets the principle of the “Three Rs” as the use of few animals would provide enough tissue to test a vast amount of experimental conditions.
Despite all of the above-mentioned, the utilization of placental ex vivo approaches is not exempt of some disadvantages. One of its major drawbacks is the short lifespan of explants, as they can only be used right after tissue collection, thus impeding to be tested later. This approach is also constrained by the limited availability of tissues suitable for explant cultures. Abattoirs are an ideal source to obtain placentas, but considering that breeding costs are relatively high, it is likely that pregnant females slaughtered there are suffering from underlying health conditions that would prevent their inclusion as tissue donors. Similarly to human studies, cesarean sections could constitute a practical way to obtain ruminant placental explants. Nevertheless, these surgeries require an experienced surgeon and are always an emergency procedure. As such, it is difficult to predict when and where the tissues will be available, and if blood flow has been compromised on them. On the other hand, the use of naturally expelled placentas from natural births is not even considered for this purpose, as this is a long process that in most cases results in a rapid tissue autolysis. In this context, placentomes obtention from pregnant females with known sanitary status and exclusively euthanized for this purpose emerges as an ideal alternative to overcome the mentioned drawbacks.
Tissue explants might be subjected to cryopreservation methods that would allow their isolation in a standardized way, thus reducing variability between experiments, and their usage under an on-demand basis (104). Availability of tissue biobanks from healthy donors would enable homogenous experimental designs and accelerate the obtention of reliable conclusions. The field of tissue cryopreservation has been widely expanded in human reproduction, where protocols for long-term conservation of oocytes, sperm, embryos, and even ovary slices have been long established (60). Nevertheless, cryopreservation of entire tissues is not without challenges due to their complex cell diversity and structure.
Despite that, various successful methods are now available to freeze human and canine placentas. These methods are based on controlled cooling rates and the use of cryoprotectants (60, 180). The employment of such protocols has demonstrated that cryopreservation does not significantly affect the viability of cells, their functional activity, proliferation, differentiation capacity, or their mitochondrial respiration, and only causes some damage to the mesenchyme (60, 180, 181). Consequently, the development of cryopreservation protocols for placental explants in ruminant species and their subsequent use for the study of diseases that cause reproductive failure seems feasible.
Concluding Remarks
Most of our current understanding on the pathogenesis of neosporosis and toxoplasmosis has emerged from the use of experimental models, but there are still important gaps on the placental host-parasite interactions that must be addressed to promote the development of effective control measures against both diseases in the future. In vivo models are indisputably the best available tools to secure reliable information about the mechanisms responsible of reproductive failure, but a number of factors such as the parasite isolate, challenge dose, route of inoculation, term of gestation, and the time of sampling have a substantial impact on the infection outcomes. Special efforts should be made to develop standardized models for each disease, or at least follow thoroughly previous experimental designs available in the literature, which will permit direct comparisons between works. However, the use of in vivo models incurs higher costs, longer experimental periods, and ethical constraints, making necessary the use of alternative models. In vitro models have proven useful to describe the behavior of N. caninum after infection of the main cell populations present in the bovine fetal-maternal interface, but much work remains to be done for T. gondii. Selection of appropriate cell lines is paramount to ensure reliable and reproducible results, but once obtained, they offer a cost-effective and animal-free source of experimentation material that can be easily exploited. Nevertheless, the use of in vitro approaches neglects the close interaction of trophoblast and caruncle cells with the immune populations locally present in the placenta. This problem could be solved by exogenous cytokine stimulation of responsive cells, or even with relevant immune cells, and should be explored in the future. Additional alternatives such as placental explants have the potential to overcome the limitations of the in vitro models, as they maintain the tissue architecture and preserve immune populations present in the placenta, but considering their short lifespan and limited availability, their application to study host-parasite interactions in the placenta will be limited until suitable cryopreservation methods have been developed. In all cases, complementation of classic techniques such as PCR and histology with more advanced techniques such as RT-q-PCR, IHC, transcriptomics, and proteomics, have the potential to offer a global view of all processes that result significantly altered during infection with both parasites.
Author Contributions
All authors listed have made a substantial, direct and intellectual contribution to the work, and approved it for publication.
Funding
This work was supported by the Spanish Ministry of Science and Innovation (PID2019-104713RB-C21) and the Community of Madrid (PLATESA2-CM, P2018/BAA-4370; Ayudas I+D para jóvenes investigadores UCM, PR65/19-22457). IPF was financially supported by a postdoctoral Fellowship from the Community of Madrid (Programa de Atracción del Talento, 2018T2/BIO10170). LJP was financially supported by a fellowship from the University Complutense of Madrid. The funders had no role in study design, data collection and analysis, decision to publish, or preparation of the manuscript.
Conflict of Interest
The authors declare that the research was conducted in the absence of any commercial or financial relationships that could be construed as a potential conflict of interest.
Acknowledgments
We gratefully acknowledge Dr. Julio Benavides Silván (Instituto de Ganadería de Montaña CSIC-Universidad de León) and Dr. Daniel Gutiérez Expósito (Universidad de León) for the histopathological images. We also acknowledge Dr. David Arranz Solís (School of Veterinary Medicine, University of California, Davis) for his placentome illustrations.
References
1. Rindsjö E, Holmlund U, Sverremark-Ekström E, Papadogiannakis N, Scheynius A. Toll-like receptor-2 expression in normal and pathologic human placenta. Hum Pathol. (2007) 38:468–73. doi: 10.1016/j.humpath.2006.09.009
2. Innes EA, Bartley PM, Maley SW, Wright SE, Buxton D. Comparative host-parasite relationships in ovine toxoplasmosis and bovine neosporosis and strategies for vaccination. Vaccine. (2007) 25:5495–503. doi: 10.1016/j.vaccine.2007.02.044
3. Peter AT. Bovine placenta: a review on morphology, components, and defects from terminology and clinical perspectives. Theriogenology. (2013) 80:693–705. doi: 10.1016/j.theriogenology.2013.06.004
4. Haeger J-, Hambruch N, Pfarrer C. The bovine placenta in vivo and in vitro. Theriogenology. (2016) 86:306–12. doi: 10.1016/j.theriogenology.2016.04.043
5. Fowden AL, Ward JW, Wooding FB, Forhead AJ. Developmental programming of the ovine placenta. Soc Reprod Fertil Suppl. (2010) 67:41–57 doi: 10.5661/RDR-VII-41
6. Wooding F. The synepitheliochorial placenta of ruminants: binucleate cell fusions and hormone production. Placenta. (1992) 13:101–13. doi: 10.1016/0143-4004(92)90025-O
7. Duello TM, Byatt JC, Bremel RD. Immunohistochemical localization of placental lactogen in binucleate cells of bovine placentomes. Endocrinology. (1986) 119:1351–5. doi: 10.1210/endo-119-3-1351
8. Reimers TJ, Ullmann MB, Hansel W. Progesterone and prostanoid production by bovine binucleate trophoblastic cells. Biol Reprod. (1985) 33:1227–36. doi: 10.1095/biolreprod33.5.1227
9. Nakano H, Shimada A, Imai K, Takezawa T, Takahashi T, Hashizume K. Bovine trophoblastic cell differentiation on collagen substrata: formation of binucleate cells expressing placental lactogen. Cell Tissue Res. (2002) 307:225–35. doi: 10.1007/s00441-001-0491-x
10. Taipale J, Keski-Oja J. Growth factors in the extracellular matrix. FASEB J. (1997) 11:51–9. doi: 10.1096/fasebj.11.1.9034166
11. Pfarrer C, Hirsch P, Guillomot M, Leiser R. Interaction of integrin receptors with extracellular matrix is involved in trophoblast giant cell migration in bovine placentomes. Placenta. (2003) 24:588–97. doi: 10.1016/S0143-4004(03)00059-6
12. Wang M, Lai S. Fibronectin degradation by MMP-2/MMP-9 in the serum of pregnant women and umbilical cord with Toxoplasma gondii infection. J Obstet Gynaecol. (2013) 33:370–4. doi: 10.3109/01443615.2013.769501
13. Brasil TR, Freire-de-Lima CG, Morrot A, Vetö Arnholdt AC. Host-Toxoplasma gondii coadaptation leads to fine tuning of the immune response. Front Immunol. (2017) 8:1080. doi: 10.3389/fimmu.2017.01080
14. Donnelly L, Campling G. Functions of the placenta. Anaesth Intens Care. (2016) 17:349–53. doi: 10.1016/j.mpaic.2016.04.004
15. Schuler G, Greven H, Kowalewski MP, Doring B, Ozalp GR, Hoffmann B. Placental steroids in cattle: hormones, placental growth factors or by-products of trophoblast giant cell differentiation? Exp Clin Endocrinol Diabetes. (2008) 116:429–36. doi: 10.1055/s-2008-1042408
16. Jiménez-Pelayo L, García-Sánchez M, Collantes-Fernández E, Regidor-Cerrillo J, Horcajo P, Gutiérrez-Expósito D, et al. The cross-talk at the placenta between Neospora caninum and the bovine host determines the outcome of the infection. Vet Res. (2020) 51:83. doi: 10.1186/s13567-020-00803-y
17. Castaño P, Fernandez M, Regidor-Cerrillo J, Fuertes M, Horcajo P, Ferre I, et al. Peripheral and placental immune responses in sheep after experimental infection with Toxoplasma gondii at the three terms of gestation. Vet Res. (2019) 50:66. doi: 10.1186/s13567-019-0681-8
18. Arranz-Solís D, Benavides J, Regidor-Cerrillo J, Horcajo P, Castaño P, del Carmen Ferreras M, et al. Systemic and local immune responses in sheep after Neospora caninum experimental infection at early, mid and late gestation. Vet Res. (2016) 47:1–13. doi: 10.1186/s13567-015-0290-0
19. Wegmann TG, Lin H, Guilbert L, Mosmann TR. Bidirectional cytokine interactions in the maternal-fetal relationship: is successful pregnancy a TH2 phenomenon? Immunol Today. (1993) 14:353–6. doi: 10.1016/0167-5699(93)90235-D
20. Entrican G. Immune regulation during pregnancy and host-pathogen interactions in infectious abortion. J Comp Pathol. (2002) 126:79–94. doi: 10.1053/jcpa.2001.0539
21. Dubey JP, Hemphill A, Calero-Bernal R, Schares G. Neosporosis in Animals. Boca Raton, FL: CRC Press (2017). doi: 10.1201/9781315152561
22. Stelzer S, Basso W, Silván JB, Ortega-Mora L, Maksimov P, Gethmann J, et al. Toxoplasma gondii infection and toxoplasmosis in farm animals: Risk factors and economic impact. Food Waterborne Parasitol. (2019):e00037. doi: 10.1016/j.fawpar.2019.e00037
23. Pappas G, Roussos N, Falagas ME. Toxoplasmosis snapshots: global status of Toxoplasma gondii seroprevalence and implications for pregnancy and congenital toxoplasmosis. Int J Parasitol. (2009) 39:1385–94. doi: 10.1016/j.ijpara.2009.04.003
24. Speer CA, Dubey JP, McAllister MM, Blixt JA. Comparative ultrastructure of tachyzoites, bradyzoites, and tissue cysts of Neospora caninum and Toxoplasma gondii. Int J Parasitol. (1999) 29:1509–19. doi: 10.1016/S0020-7519(99)00132-0
25. Reid AJ, Vermont SJ, Cotton JA, Harris D, Hill-Cawthorne GA, Konen-Waisman S, et al. Comparative genomics of the apicomplexan parasites Toxoplasma gondii and Neospora caninum: Coccidia differing in host range and transmission strategy. PLoS Pathog. (2012) 8:e1002567. doi: 10.1371/journal.ppat.1002567
26. González-Warleta M, Castro-Hermida JA, Calvo C, Pérez V, Gutiérrez-Expósito D, Regidor-Cerrillo J, et al. Endogenous transplacental transmission of Neospora caninum during successive pregnancies across three generations of naturally infected sheep. Vet Res. (2018) 49:106. doi: 10.1186/s13567-018-0601-3
27. Costa RC, Mesquita LP, Nunes MVL, Oliveira IM, Oliveira LFS, Souza AR, et al. Neospora caninum bioassay in gerbils using placental tissues from naturally infected goats. Vet Parasitol. (2018) 249:70–3. doi: 10.1016/j.vetpar.2017.11.009
28. Horcajo P, Regidor-Cerrillo J, Aguado-Martínez A, Hemphill A, Ortega-Mora LM. Vaccines for bovine neosporosis: current status and key aspects for development. Parasite Immunol. (2016) 38:709–23. doi: 10.1111/pim.12342
29. Sánchez-Sánchez R, Vázquez P, Ferre I, Ortega-Mora LM. Treatment of toxoplasmosis and neosporosis in farm ruminants: state of knowledge and future trends. Curr Top Med Chem. (2018). doi: 10.2174/1568026618666181002113617
30. Dubey JP, Murata FHA, Cerqueira-Cezar CK, Kwok OCH. Public health and economic importance of Toxoplasma gondii infections in goats: the last decade. Res Vet Sci. (2020) 132:292–307. doi: 10.1016/j.rvsc.2020.06.014
31. Trees AJ, Williams DJL. Endogenous and exogenous transplacental infection in Neospora caninum and Toxoplasma gondii. Trends Parasitol. (2005) 21:558–61. doi: 10.1016/j.pt.2005.09.005
32. Dubey JP, Su C. Population biology of Toxoplasma gondii: what's out and where did they come from. Mem Inst Oswaldo Cruz. (2009) 104:190–5. doi: 10.1590/S0074-02762009000200011
33. Belluco S, Mancin M, Conficoni D, Simonato G, Pietrobelli M, Ricci A. Investigating the determinants of Toxoplasma gondii prevalence in meat: a systematic review and meta-regression. PLoS ONE. (2016) 11:153856. doi: 10.1371/journal.pone.0153856
34. Dubey JP. Toxoplasmosis of Animals and Humans. Boca Raton, FL: CRC Press (2016). doi: 10.1201/9781420092370
35. Calero-Bernal R, Horcajo P, Hernández M, Ortega-Mora LM, Fuentes I. Absence of Neospora caninum DNA in Human Clinical Samples, Spain. Emerg Infect Dis. (2019) 25:1226–7. doi: 10.3201/eid2506.181431
36. Elmore SA, Jones JL, Conrad PA, Patton S, Lindsay DS, Dubey JP. Toxoplasma gondii: epidemiology, feline clinical aspects, and prevention. Trends Parasitol. (2010) 26:190–6. doi: 10.1016/j.pt.2010.01.009
37. Innes EA, Bartley PM, Buxton D, Katzer F. Ovine toxoplasmosis. Parasitology. (2009) 136:1887–94. doi: 10.1017/S0031182009991636
38. Dubey JP. Repeat transplacental transfer of Toxoplasma gondii in dairy goats. J Am Vet Med Assoc. (1982) 180:1220–1.
39. Dos Santos TR, Faria Gabriela da SM, Guerreiro BM, Lopes WDZ, da Silva HM, Garcia JL, et al. Congenital toxoplasmosis in chronically infected and subsequently challenged ewes. PLoS ONE. (2016) 11:e0165124. doi: 10.1371/journal.pone.0165124
40. Klauck V, Pazinato R, Radavelli WM, Custodio E, Bianchi AE, Camillo G, et al. Toxoplasma gondii infection in dairy ewes: vertical transmission and influence on milk production and reproductive performance. Microb Pathog. (2016) 99:101–5. doi: 10.1016/j.micpath.2016.08.012
41. Dubey JP, Lindsay DS. Neosporosis, toxoplasmosis, and sarcocystosis in ruminants. Vet Clin North Am Food Anim Pract. (2006) 22:645–71. doi: 10.1016/j.cvfa.2006.08.001
42. Innes EA. The host-parasite relationship in pregnant cattle infected with Neospora caninum. Parasitology. (2007) 134:1903–10. doi: 10.1017/S0031182007000194
43. Quinn HE, Ellis JT, Smith NC. Neospora caninum: a cause of immune-mediated failure of pregnancy? Trends Parasitol. (2002) 18:391–4. doi: 10.1016/S1471-4922(02)02324-3
44. Buxton D, Finlayson J. Experimental infection of pregnant sheep with Toxoplasma gondii: pathological and immunological observations on the placenta and fetus. J Comp Pathol. (1986) 96:319–33. doi: 10.1016/0021-9975(86)90052-6
45. Collantes-Fernández E, Arnaiz-Seco I, Burgos BM, Rodríguez-Bertos A, Aduriz G, Fernández-García A, et al. Comparison of Neospora caninum distribution, parasite loads and lesions between epidemic and endemic bovine abortion cases. Vet Parasitol. (2006) 142:187–91. doi: 10.1016/j.vetpar.2006.05.030
46. Benavides J, Collantes-Fernández E, Ferre I, Perez V, Campero C, Mota R, et al. Experimental ruminant models for bovine neosporosis: what is known and what is needed. Parasitology. (2014) 141:1471–88. doi: 10.1017/S0031182014000638
47. Castaño P, Fuertes M, Regidor-Cerrillo J, Ferre I, Fernández M, Ferreras MC, et al. Experimental ovine toxoplasmosis: influence of the gestational stage on the clinical course, lesion development and parasite distribution. Vet Res. (2016) 47:1. doi: 10.1186/s13567-016-0327-z
48. Regidor-Cerrillo J, Álvarez-García G, Pastor-Fernández I, Marugán-Hernández V, Gómez-Bautista M, Ortega-Mora LM. Proteome expression changes among virulent and attenuated Neospora caninum isolates. J Proteomics. (2012) 75:2306–18 doi: 10.1016/j.jprot.2012.01.039
49. Regidor-Cerrillo J, Gómez-Bautista M, Sodupe I, Aduriz G, Álvarez-García G, Del Pozo I, et al. In vitro invasion efficiency and intracellular proliferation rate comprise virulence-related phenotypic traits of Neospora caninum. Vet Res. (2011) 42:41. doi: 10.1186/1297-9716-42-41
50. Dellarupe A, Regidor-Cerrillo J, Jiménez-Ruiz E, Schares G, Unzaga JM, Venturini MC, et al. Comparison of host cell invasion and proliferation among Neospora caninum isolates obtained from oocysts and from clinical cases of naturally infected dogs. Exp Parasitol. (2014) 145:22–8. doi: 10.1016/j.exppara.2014.07.003
51. Dellarupe A, Regidor-Cerrillo J, Jiménez-Ruiz E, Schares G, Unzaga JM, Venturini MC, et al. Clinical outcome and vertical transmission variability among canine Neospora caninum isolates in a pregnant mouse model of infection. Parasitology. (2014) 141:356–66. doi: 10.1017/S0031182013001479
52. Rojo-Montejo S, Collantes-Fernández E, Blanco-Murcia J, Rodríguez-Bertos A, Risco-Castillo V, Ortega-Mora LM. Experimental infection with a low virulence isolate of Neospora caninum at 70 days gestation in cattle did not result in foetopathy. Vet Res. (2009) 40:49. doi: 10.1051/vetres/2009032
53. Jiménez-Pelayo L, García-Sánchez M, Vázquez P, Regidor-Cerrillo J, Horcajo P, Collantes-Fernández E, et al. Early Neospora caninum infection dynamics in cattle after inoculation at mid-gestation with high (Nc-Spain7)- or low (Nc-Spain1H)-virulence isolates. Vet Res. (2019) 50:72,019-0691-6. doi: 10.1186/s13567-019-0691-6
54. Sibley LD, Boothroyd JC. Virulent strains of Toxoplasma gondii comprise a single clonal lineage. Nature. (1992) 359:82. doi: 10.1038/359082a0
55. Saeij JP, Boyle JP, Boothroyd JC. Differences among the three major strains of Toxoplasma gondii and their specific interactions with the infected host. Trends Parasitol. (2005) 21:476–81. doi: 10.1016/j.pt.2005.08.001
56. Jiménez-Pelayo L, García-Sánchez M, Regidor-Cerrillo J, Horcajo P, Collantes-Fernández E, Gómez-Bautista M, et al. Differential susceptibility of bovine caruncular and trophoblast cell lines to infection with high and low virulence isolates of Neospora caninum. Parasit Vectors. (2017) 10:463. doi: 10.1186/s13071-017-2409-9
57. Jiménez-Pelayo L, García-Sánchez M, Regidor-Cerrillo J, Horcajo P, Collantes-Fernández E, Gómez-Bautista M, et al. Immune response profile of caruncular and trophoblast cell lines infected by high- (Nc-Spain7) and low virulente (Nc-Spain1H) isolates of Neospora caninum. Parasit Vectors. (2019) 12(1):218. doi: 10.1186/s13071-019-3466-z
58. Horcajo P, Jiménez-Pelayo L, García-Sánchez M, Regidor-Cerrillo J, Collantes-Fernández E, Rozas D, et al. Transcriptome modulation of bovine trophoblast cells in vitro by Neospora caninum. Int J Parasitol. (2017) 47:791–9. doi: 10.1016/j.ijpara.2017.08.007
59. Geurts N, Opdenakker G, Van den Steen PE. Matrix metalloproteinases as therapeutic targets in protozoan parasitic infections. Pharmacol Ther. (2012) 133:257–79. doi: 10.1016/j.pharmthera.2011.11.008
60. Huppertz B, Kivity V, Sammar M, Grimpel Y, Leepaz N, Orendi K, et al. Cryogenic and low temperature preservation of human placental villous explants - a new way to explore drugs in pregnancy disorders. Placenta. (2011) 32(Suppl.):S65–76. doi: 10.1016/j.placenta.2010.11.020
61. Quintanilla-Gozalo A, Pereira-Bueno J, Seijas-Carballedo A, Costas E, Ortega Mora LM. Observational studies in Neospora caninum infected dairy cattle: relationship infection-abortion and gestational antibody fluctuations. In: Hemphill A, Gottstein B. A European perspective on Neospora caninum. Int J Parasitol. (2000) 30:877–924. doi: 10.1016/S0020-7519(00)00072-2
62. Guy CS, Williams DJL, Kelly DF, McGarry JW, Guy F, Bjorkman C, et al. Neospora caninum in persistently infected, pregnant cows: spontaneous transplacental infection is associated with an acute increase in maternal antibody. Vet Rec. (2001) 149:443–9. doi: 10.1136/vr.149.15.443
63. Rosbottom A, Gibney H, Kaiser P, Hartley C, Smith RF, Robinson R, et al. Up regulation of the maternal immune response in the placenta of cattle naturally infected with Neospora caninum. PLoS ONE. (2011) 6:e15799. doi: 10.1371/journal.pone.0015799
64. Sánchez-Sánchez R, Ferre I, Regidor-Cerrillo J, Gutiérrez-Expósito D, Ferrer LM, Arteche-Villasol N, et al. Virulence in mice of a Toxoplasma gondii type II isolate does not correlate with the outcome of experimental infection in pregnant sheep. Front Cell Infect Microbiol. (2019) 8:436. doi: 10.3389/fcimb.2018.00436
65. Yarovinsky F, Sher A. Toll-like receptor recognition of Toxoplasma gondii. Int J Parasitol. (2006) 36:255–9. doi: 10.1016/j.ijpara.2005.12.003
66. Koblansky AA, Jankovic D, Oh H, Hieny S, Sungnak W, Mathur R, et al. Recognition of profilin by Toll-like receptor 12 is critical for host resistance to Toxoplasma gondii. Immunity. (2013) 38:119–30. doi: 10.1016/j.immuni.2012.09.016
67. Ivanova DL, Fatima R, Gigley JP. Comparative analysis of conventional natural killer cell responses to acute infection with Toxoplasma gondii strains of different virulence. Front Immunol. (2016) 7:347. doi: 10.3389/fimmu.2016.00347
68. Wattegedera SR, Doull LE, Goncheva MI, Wheelhouse NM, Watson DM, Pearce J, et al. Immunological homeostasis at the ovine placenta may reflect the degree of maternal fetal interaction. Front Immunol. (2019) 9:3025. doi: 10.3389/fimmu.2018.03025
69. Castaño P, Fuertes M, Fernández M, Ferreras MC, Ferre I, Ortega-Mora LM, et al. Macrophages and T Lymphocytes in the ovine placenta after experimental infection with Toxoplasma gondii. Vet Pathol. (2020) 57:545–9. doi: 10.1177/0300985820923987
70. Quinn HE, Miller CM, Ellis JT. The cell-mediated immune response to Neospora caninum during pregnancy in the mouse is associated with a bias toward production of interleukin-4. Int J Parasitol. (2004) 34:723–32. doi: 10.1016/j.ijpara.2004.01.007
71. Almería S, Serrano-Pérez B, Darwich L, Mur-Novales R, Garcia-Ispierto I, Cabezón O, et al. Cytokine gene expression in aborting and non-aborting dams and in their fetuses after experimental infection with Neospora caninum at 110 days of gestation. Vet Parasitol. (2016) 227:138–42. doi: 10.1016/j.vetpar.2016.08.006
72. Mur-Novales R, Serrano-Pérez B, García-Ispierto I, de Sousa NM, Beckers JF, Almería S, et al. Experimental Neospora caninum infection modifies trophoblast cell populations and plasma pregnancy-associated glycoprotein 1 and 2 dynamics in pregnant dairy heifers. Vet Parasitol. (2016) 216:7–12. doi: 10.1016/j.vetpar.2015.12.001
73. Fernández-Escobar M, Millán J, Chirife AD, Ortega-Mora LM, Calero-Bernal R. Molecular survey for cyst-forming coccidia (Toxoplasma gondii, Neospora caninum, Sarcocystis spp.) in Mediterranean periurban micromammals. Parasitol Res. (2020) 119:2679–86. doi: 10.1007/s00436-020-06777-2
74. Mévélec M, Ducournau C, Ismael AB, Olivier M, Sèche É, Lebrun M, et al. Mic1-3 Knockout Toxoplasma gondii is a good candidate for a vaccine against T. gondii-induced abortion in sheep. Vet Res. (2010) 41:49. doi: 10.1051/vetres/2010021
75. McCann CM, McAllister MM, Gondim LF, Smith RF, Cripps PJ, Kipar A, et al. Neospora caninum in cattle: Experimental infection with oocysts can result in exogenous transplacental infection, but not endogenous transplacental infection in the subsequent pregnancy. Int J Parasitol. (2007) 37:1631–9. doi: 10.1016/j.ijpara.2007.05.012
76. Caspe SG, Moore DP, Leunda MR, Cano DB, Lischinsky L, Regidor-Cerrillo J, et al. The Neospora caninum-Spain 7 isolate induces placental damage, fetal death and abortion in cattle when inoculated in early gestation. Vet Parasitol. (2012) 189:171–81. doi: 10.1016/j.vetpar.2012.04.034
77. Regidor-Cerrillo J, Arranz-Solís D, Benavides J, Gómez-Bautista M, Castro-Hermida JA, Mezo M, et al. Neospora caninum infection during early pregnancy in cattle: how the isolate influences infection dynamics, clinical outcome and peripheral and local immune responses. Vet Res. (2014) 45:10. doi: 10.1186/1297-9716-45-10
78. Almería S, Serrano-Perez B, Darwich L, Domingo M, Mur-Novales R, Regidor-Cerrillo J, et al. Fetal death in naive heifers inoculated with Neospora caninum isolate Nc-Spain7 at 110 days of pregnancy. Exp Parasitol. (2016) 168:62–9. doi: 10.1016/j.exppara.2016.06.009
79. Macaldowie C, Maley SW, Wright S, Bartley P, Esteban-Redondo I, Buxton D, et al. Placental pathology associated with fetal death in cattle inoculated with Neospora caninum by two different routes in early pregnancy. J Comp Pathol. (2004) 131:142–56. doi: 10.1016/j.jcpa.2004.02.005
80. Maley SW, Buxton D, Rae AG, Wright SE, Schock A, Bartley PM, et al. The pathogenesis of neosporosis in pregnant cattle: inoculation at mid-gestation. J Comp Pathol. (2003) 129:186–95. doi: 10.1016/S0021-9975(03)00032-X
81. Vázquez P, Osoro K, Fernández M, Román-Trufero A, Regidor-Cerrillo J, Jiménez-Pelayo L, et al. Effects of challenge dose and inoculation route of the virulent Neospora caninum Nc-Spain7 isolate in pregnant cattle at mid-gestation. Vet Res. (2019) 50:68. doi: 10.1186/s13567-019-0700-9
82. Castaño P, Fuertes M, Ferre I, Fernández M, del Carmen Ferreras M, Moreno-Gonzalo J, et al. Placental thrombosis in acute phase abortions during experimental Toxoplasma gondii infection in sheep. Vet Res. (2014) 45:9. doi: 10.1186/1297-9716-45-9
83. Dubey JP, Murata FHA, Cerqueira-Cezar CK, Kwok OCH, Su C. Economic and public health importance of Toxoplasma gondii infections in sheep: 2009–2020. Vet Parasitol. (2020) 286:109195. doi: 10.1016/j.vetpar.2020.109195
84. Mavin S, Joss AW, Ball J, Ho-Yen DO. Do Toxoplasma gondii RH strain tachyzoites evolve during continuous passage? J Clin Pathol. (2004) 57:609–11. doi: 10.1136/jcp.2003.013763
85. Khan A, Behnke MS, Dunay IR, White MW, Sibley LD. Phenotypic and gene expression changes among clonal type I strains of Toxoplasma gondii. Eukaryot Cell. (2009) 8:1828–36. doi: 10.1128/EC.00150-09
86. Regidor-Cerrillo J, Xia D, Jiménez-Pelayo L, García-Sánchez M, Collantes-Fernández E, Randle N, et al. Proteomic characterization of host-pathogen interactions during bovine trophoblast cell line infection by Neospora caninum. Pathogens. (2020) 9:749. doi: 10.3390/pathogens9090749
87. Rosbottom A, Gibney EH, Guy CS, Kipar A, Smith RF, Kaiser P, et al. Upregulation of cytokines is detected in the placentas of cattle infected with Neospora caninum and is more marked early in gestation when fetal death is observed. Infect Immun. (2008) 76:2352–61. doi: 10.1128/IAI.01780-06
88. Gibney EH, Kipar A, Rosbottom A, Guy CS, Smith RF, Hetzel U, et al. The extent of parasite-associated necrosis in the placenta and fetal tissues of cattle following Neospora caninum infection in early and late gestation correlates with fetal death. Int J Parasitol. (2008) 38:579–88. doi: 10.1016/j.ijpara.2007.09.015
89. Canton GJ, Katzer F, Maley SW, Bartley PM, Benavides-Silvan J, Palarea-Albaladejo J, et al. Inflammatory infiltration into placentas of Neospora caninum challenged cattle correlates with clinical outcome of pregnancy. Vet Res. (2014) 45:11. doi: 10.1186/1297-9716-45-11
90. Marin MS, Hecker YP, Quintana S, Pérez S, Leunda MR, Cantón G, et al. Immunization with inactivated antigens of Neospora caninum induces toll-like receptors 3, 7, 8 and 9 in maternal-fetal interface of infected pregnant heifers. Vet Parasitol. (2017) 243:12–7. doi: 10.1016/j.vetpar.2017.06.005
91. Maley SW, Buxton D, Macaldowie CN, Anderson IE, Wright SE, Bartley PM, et al. Characterization of the immune response in the placenta of cattle experimentally infected with Neospora caninum in early gestation. J Comp Pathol. (2006) 135:130–41. doi: 10.1016/j.jcpa.2006.07.001
92. Cantón GJ, Katzer F, Maley SW, Bartley PM, Benavides-Silván J, Palarea-Albaladejo J, et al. Cytokine expression in the placenta of pregnant cattle after inoculation with Neospora caninum. Vet Immunol Immunopathol. (2014) 161:77–89. doi: 10.1016/j.vetimm.2014.07.004
93. Almería S, Araujo RN, Darwich L, Dubey JP, Gasbarre LC. Cytokine gene expression at the materno-fetal interface after experimental Neospora caninum infection of heifers at 110 days of gestation. Parasite Immunol. (2011) 33:517–23. doi: 10.1111/j.1365-3024.2011.01307.x
94. Serrano-Pérez B, Hansen PJ, Mur-Novales R, Garcia-Ispierto I, de Sousa NM, Beckers JF, et al. Crosstalk between uterine serpin (SERPINA14) and pregnancy-associated glycoproteins at the fetal-maternal interface in pregnant dairy heifers experimentally infected with Neospora caninum. Theriogenology. (2016) 86:824–30. doi: 10.1016/j.theriogenology.2016.03.003
95. Almería S, Araujo R, Tuo W, López-Gatius F, Dubey JP, Gasbarre LC. Fetal death in cows experimentally infected with Neospora caninum at 110 days of gestation. Vet Parasitol. (2010) 169(3-4):304–11. doi: 10.1016/j.vetpar.2009.12.044
96. Almería S, Serrano-Pérez B, Darwich L, Araujo R, López-Gatius F, Dubey J, et al. Maternal and fetal immune response patterns in heifers experimentally infected with Neospora caninum in the second trimester of pregnancy–a descriptive study. Vet Parasitol. (2014) 204:146–52. doi: 10.1016/j.vetpar.2014.05.006
97. Hecker YP, Cantón G, Regidor-Cerrillo J, Chianini F, Morrell E, Lischinsky L, et al. Cell mediated immune responses in the placenta following challenge of vaccinated pregnant heifers with Neospora caninum. Vet Parasitol. (2015) 214:247–54. doi: 10.1016/j.vetpar.2015.10.015
98. Dorsch M, de Yaniz M, Fiorani F, Hecker Y, Odeón A, Morrell E, et al. A descriptive study of lectin histochemistry of the placenta in cattle following inoculation of Neospora caninum. J Comp Pathol. (2019) 166:45–53. doi: 10.1016/j.jcpa.2018.10.172
99. Horcajo P, Pitarch A, Jiménez-Pelayo L, García-Sánchez M, Collantes-Fernández E, Ortega-Mora LM. Comparative proteomic analysis of bovine placentas infected with high and low virulence Neospora caninum isolates. in Proceedings of the 5th International Meeting on Apicowplexan parasites in Farm Animals. Berlin. (2019).
100. Benavides J, Fernández M, Castaño P, Ferreras M, Ortega-Mora L, Pérez V. Ovine toxoplasmosis: a new look at its pathogenesis. J Comp Pathol. (2017) 157:34–8. doi: 10.1016/j.jcpa.2017.04.003
101. Trees AJ, Crozier SJ, Buxton D, Blewett DA. Serodiagnosis of ovine toxoplasmosis: an assessment of the latex agglutination test and the value of IgM specific titres after experimental oocyst-induced infections. Res Vet Sci. (1989) 46:67–72. doi: 10.1016/S0034-5288(18)31120-2
102. Owen MR, Clarkson MJ, Trees AJ. Acute phase Toxoplasma abortions in sheep. Vet Rec. (1998) 142:480–2. doi: 10.1136/vr.142.18.480
103. Gutiérrez-Expósito D, Arteche-Villasol N, Vallejo-Garcia R, Ferreras-Estrada MC, Ferre I, Sánchez-Sánchez R, et al. Characterization of fetal brain damage in early abortions of ovine toxoplasmosis. Vet Pathol. (2020) 57:535–44. doi: 10.1177/0300985820921539
104. Orendi K, Kivity V, Sammar M, Grimpel Y, Gonen R, Meiri H, et al. Placental and trophoblastic in vitro models to study preventive and therapeutic agents for preeclampsia. Placenta. (2011) 32(Suppl.):S49–54. doi: 10.1016/j.placenta.2010.11.023
105. Stringfellow D, Gray B, Lauerman L, Thomson M, Rhodes P, Bird R. Monolayer culture of cells originating from apreimplantation bovine embryo. In vitro Cell Dev Biol. (1987) 23:750–4. doi: 10.1007/BF02623675
106. Talbot NC, Caperna TJ, Edwards JL, Garrett W, Wells KD, Ealy AD. Bovine blastocyst-derived trophectoderm and endoderm cell cultures: interferon tau and transferrin expression as respective in vitro markers. Biol Reprod. (2000) 62:235–47. doi: 10.1095/biolreprod62.2.235
107. Talbot NC, Powell AM, Ocon OM, Caperna TJ, Camp M, Garrett WM, et al. Comparison of the interferon-tau expression from primary trophectoderm outgrowths derived from IVP, NT, and parthenogenote bovine blastocysts. Mol Reprod Dev. (2008) 75:299–308. doi: 10.1002/mrd.20741
108. Shimada A, Nakano H, Takahashi T, Imai K, Hashizume K. Isolation and characterization of a bovine blastocyst-derived trophoblastic cell line, BT-1: development of a culture system in the absence of feeder cell. Placenta. (2001) 22:652–62. doi: 10.1053/plac.2001.0702
109. Ramos-Ibeas P, Calle A, Pericuesta E, Laguna-Barraza R, Moros-Mora R, Lopera-Vásquez R, et al. An efficient system to establish biopsy-derived trophoblastic cell lines from bovine embryos. Biol Reprod. (2014) 91:15. doi: 10.1095/biolreprod.114.118430
110. Suzuki Y, Koshi K, Imai K, Takahashi T, Kizaki K, Hashizume K. Bone morphogenetic protein 4 accelerates the establishment of bovine trophoblastic cell lines. Reproduction. (2011) 142:733–43. doi: 10.1530/REP-11-0275
111. Pillai VV, Siqueira LG, Das M, Kei TG, Tu LN, Herren AW, et al. Physiological profile of undifferentiated bovine blastocyst-derived trophoblasts. Biol Open. (2019) 8:10.1242/bio.037937. doi: 10.1242/bio.037937
112. Saadeldin IM, Abdelfattah-Hassan A, Swelum AA. Feeder cell type affects the growth of in vitro cultured bovine trophoblast cells. Biomed Res Int. (2017) 2017:1061589. doi: 10.1155/2017/1061589
113. Kawaguchi T, Cho D, Hayashi M, Tsukiyama T, Kimura K, Matsuyama S, et al. Derivation of induced trophoblast cell lines in cattle by doxycycline-inducible piggyBac vectors. PLoS ONE. (2016) 11:e0167550. doi: 10.1371/journal.pone.0167550
114. Munson L, Chandler SK, Schlafer DH. Long-term culture of bovine trophoblastic cells. J Tissue Cult Methods. (1988) 11:123–8. doi: 10.1007/BF01404264
115. Vanselow J, Fürbass R, Tiemann U. Cultured bovine trophoblast cells differentially express genes encoding key steroid synthesis enzymes. Placenta. (2008) 29:531–8. doi: 10.1016/j.placenta.2008.03.004
116. Hambruch N, Haeger JD, Dilly M, Pfarrer C. EGF stimulates proliferation in the bovine placental trophoblast cell line F3 via Ras and MAPK. Placenta. (2010) 31:67–74. doi: 10.1016/j.placenta.2009.10.011
117. Martino N, Rizzo A, Pizzi F, Dell'Aquila M, Sciorsci R. Effects of kisspeptin-10 on in vitro proliferation and kisspeptin receptor expression in primary epithelial cell cultures isolated from bovine placental cotyledons of fetuses at the first trimester of pregnancy. Theriogenology. (2015) 83:978–987. doi: 10.1016/j.theriogenology.2014.11.033
118. Bridger P, Haupt S, Klisch K, Leiser R, Tinneberg H, Pfarrer C. Validation of primary epitheloid cell cultures isolated from bovine placental caruncles and cotyledons. Theriogenology. (2007) 68:592–603. doi: 10.1016/j.theriogenology.2007.05.046
119. Bridger PS, Menge C, Leiser R, Tinneberg HR, Pfarrer CD. Bovine caruncular epithelial cell line (BCEC-1) isolated from the placenta forms a functional epithelial barrier in a polarized cell culture model. Placenta. (2007) 28:1110–7. doi: 10.1016/j.placenta.2007.07.002
120. Wango EO, Heap RB, Wooding FB. Progesterone and 5 beta-pregnanediol production by isolated fetal placental binucleate cells from sheep and goats. J Endocrinol. (1991) 129:283–9. doi: 10.1677/joe.0.1290283
121. Morgan G, Whyte A, Wooding FB. Characterization of the synthetic capacities of isolated placental binucleate cells from sheep and goats. Anat Rec. (1990) 226:27–36. doi: 10.1002/ar.1092260105
122. Soares MC, Servely JL, Puissant C, Bolifraud P, Lacroix MC, Schaeffer B, et al. Ovine chorionic somatomammotrophin (oCS) production by isolated cotyledon cells from sheep in early and mid-gestation: auto-regulation by recombinant oCS. J Endocrinol. (1999) 161:289–98. doi: 10.1677/joe.0.1610289
123. Steven DH, Mallon KA, Nathanielsz PW. Sheep trophoblast in monolayer cell culture. Placenta. (1980) 1:209–21. doi: 10.1016/S0143-4004(80)80003-8
124. Farmer JL, Burghardt RC, Jousan FD, Hansen PJ, Bazer FW, Spencer TE. Galectin 15 (LGALS15) functions in trophectoderm migration and attachment. FASEB J. (2008) 22:548–60. doi: 10.1096/fj.07-9308com
125. Ralph MM, Lee CS, Thorburn GD. Identification and characterization of monolayer cultures of sheep trophoblast cells maintained in bicameral culture chambers. Biol Reprod. (1989) 41:481–9. doi: 10.1095/biolreprod41.3.481
126. Kim J, Song G, Gao H, Farmer JL, Satterfield MC, Burghardt RC, et al. Insulin-like growth factor II activates phosphatidylinositol 3-kinase-protooncogenic protein kinase 1 and mitogen-activated protein kinase cell Signaling pathways, and stimulates migration of ovine trophectoderm cells. Endocrinology. (2008) 149:3085–94. doi: 10.1210/en.2007-1367
127. Haldorson GJ, Stanton JB, Mathison BA, Suarez CE, Baszler TV. Neospora caninum: antibodies directed against tachyzoite surface protein NcSRS2 inhibit parasite attachment and invasion of placental trophoblasts in vitro. Exp Parasitol. (2006) 112:172–8. doi: 10.1016/j.exppara.2005.11.004
128. Zhang Y, Shi J, Liu S. Establishment and characterization of a telomerase-immortalized sheep trophoblast cell line. Biomed Res Int. (2016) 2016:5808575. doi: 10.1155/2016/5808575
129. Jimenéz-Meléndez A, Fernández-Álvarez M, Calle A, Ramírez MA, Diezma-Diaz C, Vázquez-Arbaizar P, et al. Lytic cycle of Besnoitia besnoiti tachyzoites displays similar features in primary bovine endothelial cells and fibroblasts. Parasit Vectors. (2019) 12:517. doi: 10.1186/s13071-019-3777-0
130. Hemphill A, Gottstein B. Neospora caninum and neosporosis - recent achievements in host and parasite cell biology and treatment. Acta Parasitol. (2006) 51:15–25. doi: 10.2478/s11686-006-0002-z
131. Almería S, Serrano-Pérez B, López-Gatius F. Immune response in bovine neosporosis: protection or contribution to the pathogenesis of abortion. Microbial Pathogenesis. (2017) 109:177–82. doi: 10.1016/j.micpath.2017.05.042
132. Pinheiro AM, Costa SL, Freire SM, Ribeiro CS, Tardy M, El-Bacha RS, et al. Neospora caninum: early immune response of rat mixed glial cultures after tachyzoites infection. Exp Parasitol. (2010) 124:442–7. doi: 10.1016/j.exppara.2009.12.018
133. Xu T, Ping J, Yu Y, Yu F, Yu Y, Hao P, et al. Revealing parasite influence in metabolic pathways in Apicomplexa infected patients. BMC Bioinformatics. (2010) 11(Suppl.11):S13. doi: 10.1186/1471-2105-11-S11-S13
134. Remmerie A, Scott CL. Macrophages and lipid metabolism. Cell Immunol. (2018) 330:27–42. doi: 10.1016/j.cellimm.2018.01.020
135. Hargrave KE, Woods S, Millington O, Chalmers S, Westrop GD, Roberts CW. Multi-omics tudies demonstrate Toxoplasma gondii-induced metabolic reprogramming of murine dendritic cells. Front Cell Infect Microbiol. (2019) 9:309 doi: 10.3389/fcimb.2019.00309
136. Nelson MM, Jones AR, Carmen JC, Sinai AP, Burchmore R, Wastling JM. Modulation of the host cell proteome by the intracellular apicomplexan parasite Toxoplasma gondii. Infect Immun. (2008) 76:828–44. doi: 10.1128/IAI.01115-07
137. Pernas L, Adomako-Ankomah Y, Shastri AJ, Ewald SE, Treeck M, Boyle JP, et al. Toxoplasma effector MAF1 mediates recruitment of host mitochondria and impacts the host response. PLoS Biol. (2014) 12:e1001845. doi: 10.1371/journal.pbio.1001845
138. Nolan SJ, Romano JD, Luechtefeld T, Coppens I. Neospora caninum recruits host cell structures to its parasitophorous vacuole and salvages lipids from organelles. Eukaryot Cell. (2015) 14:454–73. doi: 10.1128/EC.00262-14
139. Syn G, Anderson D, Blackwell JM, Jamieson SE. Toxoplasma gondii infection is associated with mitochondrial dysfunction in-vitro. Front Cell Infect Microbiol. (2017) 7:512. doi: 10.3389/fcimb.2017.00512
140. García-Sánchez M, Jiménez-Pelayo L, Horcajo P, Regidor-Cerrillo J, Ólafsson EB, Bhandage AK, et al. Differential responses of bovine monocyte-derived macrophages to infection by Neospora caninum isolates of high and low virulence. Front Immunol. (2019) 10:915. doi: 10.3389/fimmu.2019.00915
141. Moreno B, Collantes-Fernández E, Villa A, Navarro A, Regidor-Cerrillo J, Ortega-Mora LM. Occurrence of Neospora caninum and Toxoplasma gondii infections in ovine and caprine abortions. Vet Parasitol. (2012) 187:312–8. doi: 10.1016/j.vetpar.2011.12.034
142. Wheelhouse N, Wattegedera S, Stanton J, Maley S, Watson D, Jepson C, et al. Ovine trophoblast is a primary source of TNFα during Chlamydophila abortus infection. J Reprod Immunol. (2009) 80:49–56. doi: 10.1016/j.jri.2008.12.003
143. Wheelhouse N, Coyle C, Barlow PG, Mitchell S, Greub G, Baszler T, et al. Waddlia chondrophila infects and multiplies in ovine trophoblast cells stimulating an inflammatory immune response. PLoS ONE. (2014) 9:e102386. doi: 10.1371/journal.pone.0102386
144. Castro AS, Alves CM, Angeloni MB, Gomes AO, Barbosa BF, Franco PS, et al. Trophoblast cells are able to regulate monocyte activity to control Toxoplasma gondii infection. Placenta. (2013) 34:240–7. doi: 10.1016/j.placenta.2012.12.006
145. da Silva RJ, Gomes AO, Franco PS, Pereira AS, Milian ICB, Ribeiro M, et al. Enrofloxacin and toltrazuril are able to reduce Toxoplasma gondii growth in human BeWo trophoblastic cells and villous explants from human third trimester pregnancy. Front Cell Infect Microbiol. (2017) 7:340. doi: 10.3389/fcimb.2017.00340
146. Angeloni MB, Guirelli PM, Franco PS, Barbosa BF, Gomes AO, Castro AS, et al. Differential apoptosis in BeWo cells after infection with highly (RH) or moderately (ME49) virulent strains of Toxoplasma gondii is related to the cytokine profile secreted, the death receptor Fas expression and phosphorylated ERK1/2 expression. Placenta. (2013) 34:973–82. doi: 10.1016/j.placenta.2013.09.005
147. Huckle W. Cell-and tissue-based models for study of placental development. Prog Mol Biol Transl Sci. (2017) 145:29–37. doi: 10.1016/bs.pmbts.2016.12.002
148. Miller RK, Genbacev O, Turner MA, Aplin JD, Caniggia I, Huppertz B. Human placental explants in culture: approaches and assessments. Placenta. (2005) 26:439–48. doi: 10.1016/j.placenta.2004.10.002
149. Ferro EAV, Mineo JR, Ietta F, Bechi N, Romagnoli R, Silva DAO, et al. Macrophage migration inhibitory factor is up-regulated in human first-trimester placenta stimulated by soluble antigen of Toxoplasma gondii, resulting in increased monocyte adhesion on villous explants. Am J Pathol. (2008) 72:50–8. doi: 10.2353/ajpath.2008.070432
150. de Oliveira Gomes A, de Oliveira Silva, Deise Aparecida, Silva NM, de Freitas Barbosa B, Franco PS, Angeloni MB, et al. Effect of macrophage migration inhibitory factor (MIF) in human placental explants infected with Toxoplasma gondii depends on gestational age. Am J Pathol. (2011) 178:2792–801. doi: 10.1016/j.ajpath.2011.02.005
151. Robbins JR, Zeldovich VB, Poukchanski A, Boothroyd JC, Bakardjiev AI. Tissue barriers of the human placenta to infection with Toxoplasma gondii. Infect Immun. (2012) 80:418–28. doi: 10.1128/IAI.05899-11
152. Ander SE, Rudzki EN, Arora N, Sadovsky Y, Coyne CB, Boyle JP. Human placental syncytiotrophoblasts restrict Toxoplasma gondii attachment and replication and respond to infection by producing immunomodulatory chemokines. mBio. (2018) 9:e01678–17. doi: 10.1128/mBio.01678-17
153. Almeida MPO, Ferro EAV, Briceno MPP, Oliveira MC, Barbosa BF, Silva NM. Susceptibility of human villous (BeWo) and extravillous (HTR-8/SVneo) trophoblast cells to Toxoplasma gondii infection is modulated by intracellular iron availability. Parasitol Res. (2019) 118:1559–72. doi: 10.1007/s00436-019-06257-2
154. Castro-Filice LS, Barbosa BF, Angeloni MB, Silva NM, Gomes AO, Alves CM, et al. Azithromycin is able to control Toxoplasma gondii infection in human villous explants. J Transl Med. (2014) 12:132. doi: 10.1186/1479-5876-12-132
155. Franco PS, Gois PSG, de Araujo TE, da Silva RJ, de Freitas Barbosa B, de Oliveira Gomes A, et al. Brazilian strains of Toxoplasma gondii are controlled by azithromycin and modulate cytokine production in human placental explants. J Biomed Sci. (2019) 26:10. doi: 10.1186/s12929-019-0503-3
156. Castillo C, Muñoz L, Carrillo I, Liempi A, Gallardo C, Galanti N, et al. Ex vivo infection of human placental chorionic villi explants with Trypanosoma cruzi and Toxoplasma gondii induces different Toll-like receptor expression and cytokine/chemokine profiles. Am J Reprod Immunol. (2017) 78:12660. doi: 10.1111/aji.12660
157. Liempi A, Castillo C, Medina L, Rojas M, Maya JD, Parraguez VH, et al. Ex vivo infection of human placental explants with Trypanosoma cruzi and Toxoplasma gondii: differential activation of NF kappa B signaling pathways. Acta Trop. (2019) 199:105153. doi: 10.1016/j.actatropica.2019.105153
158. Luján CD, Triquell MF, Sembaj A, Guerrero CE, Fretes RE. Trypanosoma cruzi: productive infection is not allowed by chorionic villous explant from normal human placenta in vitro. Exp Parasitol. (2004) 108:176–81. doi: 10.1016/j.exppara.2004.07.013
159. Triquell MF, Díaz-Luján C, Freilij H, Paglini P, Fretes RE. Placental infection by two subpopulations of Trypanosoma cruzi is conditioned by differential survival of the parasite in a deleterious placental medium and not by tissue reproduction. Trans R Soc Trop Med Hyg. (2009) 103:1011–8. doi: 10.1016/j.trstmh.2009.03.004
160. Díaz-Luján C, Triquell MF, Castillo C, Hardisson D, Kemmerling U, Fretes RE. Role of placental barrier integrity in infection by Trypanosoma cruzi. Acta Trop. (2016) 164:360–8. doi: 10.1016/j.actatropica.2016.09.021
161. Sartori MJ, Pons P, Mezzano L, Lin S, de Fabro SP. Trypanosoma cruzi infection induces microfilament depletion in human placenta syncytiotrophoblast. Placenta. (2003) 24:767–71. doi: 10.1016/S0143-4004(03)00111-5
162. Duaso J, Rojo G, Cabrera G, Galanti N, Bosco C, Maya JD, et al. Trypanosoma cruzi induces tissue disorganization and destruction of chorionic villi in an ex vivo infection model of human placenta. Placenta. (2010) 31:705–11. doi: 10.1016/j.placenta.2010.05.007
163. Duaso J, Rojo G, Jana F, Galanti N, Cabrera G, Bosco C, et al. Trypanosoma cruzi induces apoptosis in ex vivo infected human chorionic villi. Placenta. (2011) 32:356–61. doi: 10.1016/j.placenta.2011.02.005
164. Liempi A, Castillo C, Duaso J, Droguett D, Sandoval A, Barahona K, et al. Trypanosoma cruzi induces trophoblast differentiation: a potential local antiparasitic mechanism of the human placenta? Placenta. (2014) 35:1035–42. doi: 10.1016/j.placenta.2014.09.017
165. Castillo C, Muñoz L, Carrillo I, Liempi A, Medina L, Galanti N, et al. Toll-like receptor-2 mediates local innate immune response against Trypanosoma cruzi in ex vivo infected human placental chorionic villi explants. Placenta. (2017) 60:40–6. doi: 10.1016/j.placenta.2017.10.005
166. Fievet N, Moussa M, Tami G, Maubert B, Cot M, Deloron P, et al. Plasmodium falciparum induces a Th1/Th2 disequilibrium, favoring the Th1-type pathway, in the human placenta. J Infect Dis. (2001) 183:1530–4. doi: 10.1086/320201
167. Suguitan AL, Jr, Leke RG, Fouda G, Zhou A, Thuita L, Metenou S, et al. Changes in the levels of chemokines and cytokines in the placentas of women with Plasmodium falciparum malaria. J Infect Dis. (2003) 188:1074–82. doi: 10.1086/378500
168. Patel OV, Takahashi T, Imai K, Hashizume K. Characterization of native and recombinant bovine pregnancy-associated glycoproteins. Res Vet Sci. (2004) 77:203–10. doi: 10.1016/j.rvsc.2004.05.003
169. Wojciechowska A, Mlynarczuk J, Kotwica J. Short-term incubation of bovine placentome sections as a tool to study xenobiotic mechanism of action. Reprod Biol. (2015) 15:238–46. doi: 10.1016/j.repbio.2015.10.002
170. Wojciechowska A, Mlynarczuk J, Kotwica J. The protein expression disorders of connexins (Cx26, Cx32 and Cx43) and keratin 8 in bovine placenta under the influence of DDT, DDE and PCBs. Pol J Vet Sci. (2018) 21:721–9. doi: 10.24425/124311
171. Samartino LE, Enright FM. Brucella abortus differs in the multiplication within bovine chorioallantoic membrane explants from early and late gestation. Comp Immunol Microbiol Infect Dis. (1996) 19:55–63. doi: 10.1016/0147-9571(95)00018-6
172. Dos Santos LS, da Silva Mol JP, de Macedo AA, Silva AP, Dos Santos Ribeiro DL, Santos RL, et al. Transcription of non-classic major histocompatibility complex (MHC) class I in the bovine placenta throughout gestation and after Brucella abortus infection. Vet Immunol Immunopathol. (2015) 167:166–70. doi: 10.1016/j.vetimm.2015.06.014
173. Mol JP, Pires SF, Chapeaurouge AD, Perales J, Santos RL, Andrade HM, et al. Proteomic profile of Brucella abortus-infected bovine chorioallantoic membrane explants. PLoS ONE. (2016) 11:e0154209. doi: 10.1371/journal.pone.0154209
174. Rocha CE, Mol JPS, Garcia LNN, Costa LF, Santos RL, Paixao TA. Comparative experimental infection of Listeria monocytogenes and Listeria ivanovii in bovine trophoblasts. PLoS ONE. (2017) 12:e0176911. doi: 10.1371/journal.pone.0176911
175. Zheng J, Li Y, Weiss AR, Bird IM, Magness RR. Expression of endothelial and inducible nitric oxide synthases and nitric oxide production in ovine placental and uterine tissues during late pregnancy. Placenta. (2000) 21:516–24. doi: 10.1053/plac.1999.0504
176. Liempi A, Castillo C, Medina L, Galanti N, Maya JD, Parraguez VH, et al. Comparative ex vivo infection with Trypanosoma cruzi and Toxoplasma gondii of human, canine and ovine placenta: analysis of tissue damage and infection efficiency. Parasitol Int. (2020) 76:102065. doi: 10.1016/j.parint.2020.102065
177. Fry RC, Bangma J, Szilagyi J, Rager JE. Developing novel in vitro methods for the risk assessment of developmental and placental toxicants in the environment. Toxicol Appl Pharmacol. (2019) 378:114635. doi: 10.1016/j.taap.2019.114635
178. Balan A, Szaingurten-Solodkin I, Swissa SS, Feinshtein V, Huleihel M, Holcberg G, et al. The effects of pravastatin on the normal human placenta: lessons from ex-vivo models. PLoS ONE. (2017) 12:e0172174. doi: 10.1371/journal.pone.0172174
179. Fretes RE, Kemmerling U. Mechanism of Trypanosoma cruzi placenta invasion and infection: the use of human chorionic villi explants. J Trop Med. (2012) 2012:614820. doi: 10.1155/2012/614820
180. Sahlfeld L, Hazzard T, Kutzler M. Cellular characteristics of cultured canine trophoblasts. Reprod Domest Anim. (2012) 47(Suppl.6):161–4. doi: 10.1111/rda.12060
Keywords: ruminants, placenta, neosporosis, toxoplasmosis, in vitro models, in vivo models, ex vivo models
Citation: Pastor-Fernández I, Collantes-Fernández E, Jiménez-Pelayo L, Ortega-Mora LM and Horcajo P (2021) Modeling the Ruminant Placenta-Pathogen Interactions in Apicomplexan Parasites: Current and Future Perspectives. Front. Vet. Sci. 7:634458. doi: 10.3389/fvets.2020.634458
Received: 27 November 2020; Accepted: 23 December 2020;
Published: 21 January 2021.
Edited by:
Maria Rosa Caro, University of Murcia, SpainReviewed by:
Ulrike Kemmerling, University of Chile, ChileAngelica Oliveira Gomes, Universidade Federal do Triângulo Mineiro, Brazil
Copyright © 2021 Pastor-Fernández, Collantes-Fernández, Jiménez-Pelayo, Ortega-Mora and Horcajo. This is an open-access article distributed under the terms of the Creative Commons Attribution License (CC BY). The use, distribution or reproduction in other forums is permitted, provided the original author(s) and the copyright owner(s) are credited and that the original publication in this journal is cited, in accordance with accepted academic practice. No use, distribution or reproduction is permitted which does not comply with these terms.
*Correspondence: Pilar Horcajo, phorcajo@ucm.es