The potential for senotherapy as a novel approach to extend life quality in veterinary medicine
- 1Department of Clinical Sciences, College of Veterinary Medicine and Biomedical Sciences, Colorado State University, Fort Collins, CO, United States
- 2Department of Microbiology, Immunology and Pathology, College of Veterinary Medicine and Biomedical Sciences, Colorado State University, Fort Collins, CO, United States
Cellular senescence, a condition where cells undergo arrest and can assume an inflammatory phenotype, has been associated with initiation and perpetuation of inflammation driving multiple disease processes in rodent models and humans. Senescent cells secrete inflammatory cytokines, proteins, and matrix metalloproteinases, termed the senescence associated secretory phenotype (SASP), which accelerates the aging processes. In preclinical models, drug interventions termed “senotherapeutics” selectively clear senescent cells and represent a promising strategy to prevent or treat multiple age-related conditions in humans and veterinary species. In this review, we summarize the current available literature describing in vitro evidence for senotheraputic activity, preclinical models of disease, ongoing human clinical trials, and potential clinical applications in veterinary medicine. These promising data to date provide further justification for future studies identifying the most active senotherapeutic combinations, dosages, and routes of administration for use in veterinary medicine.
Introduction
Cellular senescence refers to the physiological mechanism by which proliferating cells undergo stable cell cycle arrest upon stress or damage and secrete an array of factors, termed the senescence associated secretory phenotype (SASP) (1–5) (Figure 1). Cellular senescence is a hallmark of aging (6, 7), first documented in 1961 by Hayflick and Moorehead (8). In health, senescent cells (SC) are cleared from tissues by innate immune and natural killer cells (9, 10). In disease, these cells can accumulate beyond the capacity of immune surveillance mechanisms to fully clear. This massive expansion of senescent cells is driven by paracrine and endocrine signaling mechanisms that induces a senescence state in neighboring cells. A portion of senescent cells (between 30 and 70%) are termed deleterious senescent cells and can secrete inflammatory, pro-apoptotic, insulin resistance-inducing cytokines (e.g., TNF-α, IL-6,) and chemokines (MCP-1, IL-8) that attract and activate innate immune cells. Other effects include activation of matrix metalloproteinases that cause tissue destruction, bioactive lipids that contribute to inflammation (e.g., prostaglandins, bradykinins), microRNAs that contribute to stem and progenitor cell dysfunction, and extracellular vesicles (EVs) carrying cytotoxic cargo to neighboring cells (3, 11–13).
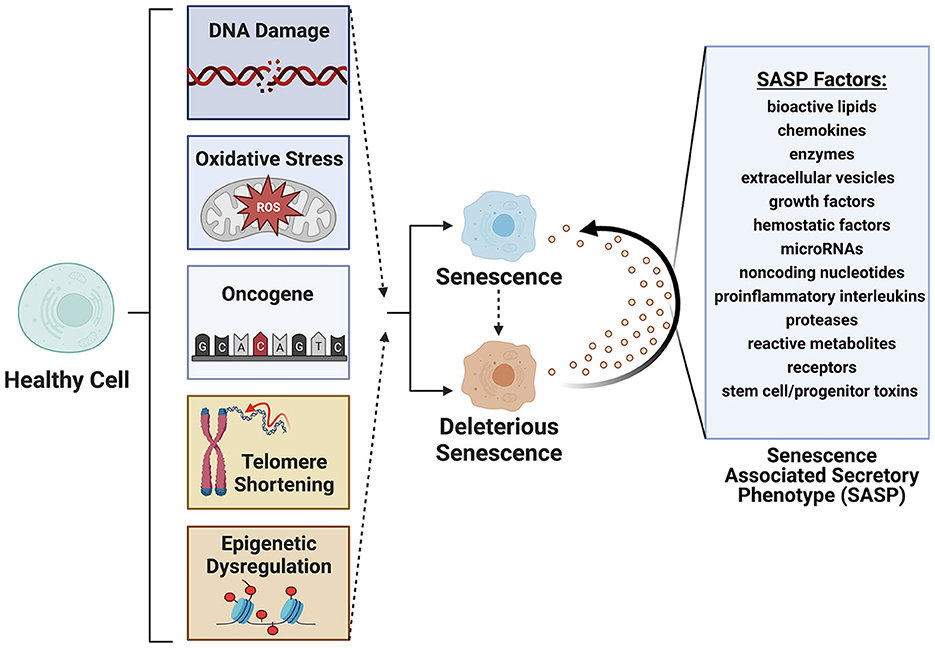
Figure 1. Senescence is induced by factors such as DNA damage, oxidative stress, oncogene formation, telomere shortening and epigenetic modifications. Deleterious senescent cells produce a senescence associated secretory phenotype (SASP) with paracrine or juxtacrine signaling of factors that promote deleterious senescent states in adjacent cells. Created with BioRender.com.
Cellular senescence underlies numerous age-related diseases (e.g., congestive heart failure, strokes, Alzheimer's, cancer, metabolic dysregulation, renal dysfunction, chronic lung diseases, osteoporosis, osteoarthritis) (3, 14) as the SASP phenotype initiates immune clearance driving chronic inflammation (15, 16). Consequently, a popular rationale for senotherapy is to reduce the overall senescent cell burden. This in turn would lead to reduced inflammation, decreased macromolecular dysregulation and improved function of stem and progenitor cells (2, 17, 18). These approaches, including the various senotherapeutics under development, their mechanisms of action, and early preclinical or clinical evidence of activity are the subject of this review.
Detection and induction of cellular senescence in vitro
While general hallmarks of cellular senescence have been established, including structural, epigenetic and signaling alterations, a universal set of sensitive and specific markers has yet to be agreed upon, which complicates senescence studies (3, 4, 19). Understanding the mechanisms leading to senescence allows for the detection and experimental induction of cellular senescence. SC exhibit a complex gene expression profile, marked by increased levels of p16Ink4a and p21Cip1, along with the activation of pathways that help them resist apoptosis, known as senescent cell antiapoptotic pathways (1, 20). Detection of cellular senescence may be performed through detection of primary markers of cell cycle arrest. These markers are characterized by upregulated expression of protein markers p16 (p16INK4a), p21 (p21Cip1), p53, decreased phosphorylated Retinoblastoma protein (pRB), and structural changes associated with increased lysosomal content and senescence-associated ß-galactosidase (SA-ß-Gal) (21, 22). Two separate, yet interconnected, pathways that drive cell cycle arrest are p53/p21 and p16/pRb (16, 23, 24). The p53 regulated gene product p21, plays a role in arresting the cell cycle through inhibition of cyclin-dependent kinase-2 (CDK2) inactivation retinoblastoma (Rb) protein necessary for cell cycle progression (23). Through the inhibition of cyclin-dependent kinase 4 (CDK4) and CDK6, p16INK4a prevents the phosphorylation of the Rb protein (23, 24). The expression of markers such as p53, p16INK4a, and p21Cip1 increase during senescence, making them commonly used indicators for senescence (25, 26).
Secondary senescence biomarkers include flattened or enlarged cellular morphology and SASP expression (e.g., IL-6, IL-8, IL-1a, GRO-a, MCP-1, and IFN-γ, among others) (4). Detecting SASP markers does not verify senescent cell states outright, as many factors other than senescence can trigger SASP cytokine secretion such as infections, immune-mediated diseases, and cancer. Profiling senescent cells with gene and protein expression analyses has demonstrated upregulation of antiapoptotic pathways in certain types of neoplastic processes such as lymphocytic leukemia and B-cell lymphoma (27, 28). Efforts toward defining the pleiotropic nature of senescent cells and SASP signatures are ongoing and have recently been published for specific cell lines (29), which warrants further investigation utilizing cell lines isolated from veterinary species of interest.
In vitro studies evaluating senotherapeutics have integrated various cell lines including WI-38, IMR-90, mouse embryonic fibroblasts (MEFs), human preadipocytes, human umbilical vein endothelial cells (HUEVCs), and bone marrow-derived mesenchymal stem cells (BMD-MSCs) with artificially induced senescence (30–33). Fibroblasts, in particular, are commonly used in studying overall aging mechanisms (34). Multiple methods to induce senescence have been reported including a single dose of gamma-radiation (e.g., 10 Gray), exposure to bleomycin, chemotherapy drugs, hydrogen peroxide, TGF-β1, oncogene induction, or replicative exhaustion (8, 33, 35, 36). The optimal method to induce senescence depends on the cell line evaluated (37). Specific considerations regarding in vitro evaluation of senotherapeutics include concentration, duration of exposure, and solubility. To identify and quantify senescent cells (SCs), several techniques have been described, including SA-ß-Gal staining, gamma-histone 2AX (γ-H2AX), senescence associated heterochromatin foci markers, immunoblotting for senescence-associated proteins, mRNA level analysis, CDKN2A/p16, and detection of senescence-associated secretory phenotype proteins (38, 39) (Figure 2). Of note, cells can adopt a senescent phenotype simply under regular culture conditions, which is indicated by elevated levels of SA-β-Gal, the DNA damage marker γ-H2AX in the nucleus, and a rise in the expression of p21 mRNA (40).
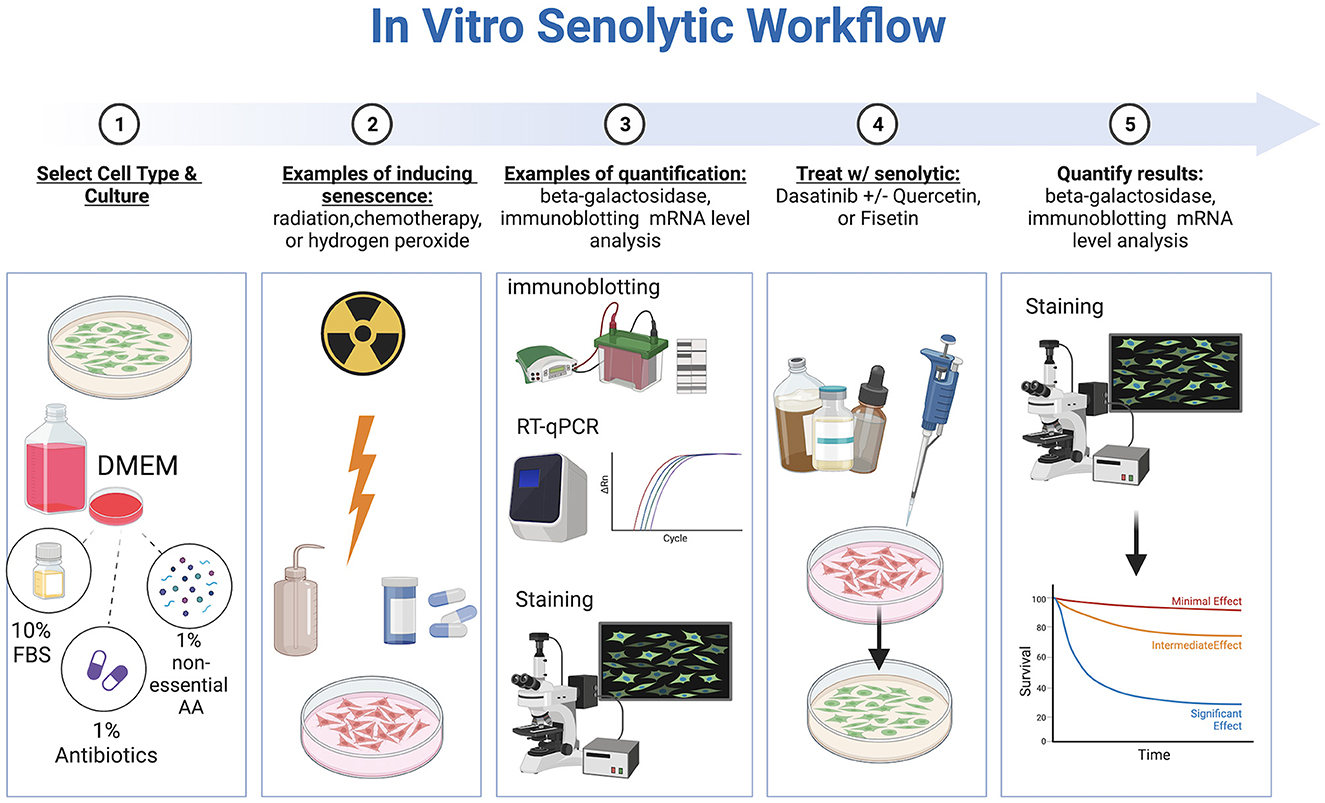
Figure 2. Workflow of in vitro senotherapeutic drug studies comprised of cell culture, induction of cell senescence, senotherapeutic treatment, and final quantification with statistical analyses. Created with BioRender.com.
Preluding gene expression, epigenetic regulators modulate transcription senescent markers and SASP factors (41, 42). This was shown in human stromal cells when histone H3-specific demethylase KDM4 was found to potentiate senescent markers such as p16INK4a, p21CIP1, and CXCL8 (43). Another study evaluated the epigenetic modifications of preadipocytes in first degree relatives of people with type 2 diabetes (44). When compared with controls, those individuals had increased senescent marker expression and hypomethylation of a gene called ZMAT3. Increased protein expression due to hypomethylation, led to premature senescence. This suggests an epigenetic influence on development of senescence and potential predisposition to type 2 diabetes (44). In summary, while general indicators of cellular senescence have been established, a complete set of sensitive and specific markers has yet to be agreed upon, which complicates detection of senescence and monitoring response to treatment with senotherapeutics, representing an area for further investigation.
Preclinical evidence for senotherapeutics
Multiple different therapeutic approaches to remove senescent cells have been investigated following the initial reports that caloric restriction or mutations that decreased growth hormone signaling significantly extended lifespan, presumably in part through reduction of the senescent cell burden (3, 45). Since then, over 40 compounds have been identified that target senescent cell associated anti-apoptotic pathways (SCAPs) with the potential for use as senotherapeutics (30). From these screens, priority was given to drugs that targeted multiple pathways, could be administered orally, and were natural products with either known safety profiles or already approved by the US Food and Drug Administration (FDA) for use in humans (1). Three such drugs, including the tyrosine kinase inhibitor dasatinib and the natural flavonoids quercetin and fisetin, were identified as promising (1). These senotherapeutics have consequently been investigated in multiple recent and ongoing human clinical trials for treatment of diseases such as osteoarthritis, skeletal health, mobility, and frailty, among others (1). Here we present a summary of the currently described senotherapeutic drugs for potential application in veterinary medicine.
First generation senotherapeutic drugs
First-generation senotherapeutics and their activities are summarized in Table 1 and described in further detail here.
Dasatinib
Dasatinib is an FDA approved tyrosine kinase inhibitor used to treat chronic myeloid leukemia and acute lymphoblastic leukemia, as well as refractory non-Hodgkin lymphoma, metastatic gastrointestinal stromal tumors, and metastatic prostate cancer in humans (64, 65). Dasatinib functions as a senotherapeutic by interfering with ephrin ligand dependent suppression of apoptosis (30–32). When administered, dasatinib decreases viability of senescent human preadipocytes by 30–40% (30) at concentrations of 50 nM and murine embryonic fibroblasts (MEFs) at concentrations of 250 nM (30). However, with an apparent cell line specific effect, dasatinib has not demonstrated efficacy against senescent human umbilical vein cells (HUVECs) nor senescent bone marrow-derived mesenchymal cells (30–32), which prompted evaluation of co-administration with other senotherapeutics, such as quercetin, to enhance efficacy.
Quercetin
Quercetin is a naturally occurring flavonoid found in certain fruits, vegetables, onions, and kale. Quercetin inhibits kinases and serpines integral to modulating cell growth and arrest such as phosphoinositide 3-kinases (PI3K) (46, 47). Clinically, quercetin alone has cardioprotective effects (66, 67) by reducing obesity, restoring plasma thyroid hormone levels, mitigating cardiac oxidative stress (66, 68), and promoting angiogenesis (69). ApoE-/- hypercholesterolemic mice that were treated with quercetin had a significant reduction in left ventricular (LV) hypertrophy (70). Quercetin enhanced antioxidant defenses and improved cardiac bioenergetics in rats that were fed a high fat diet (67). Likewise, quercetin attenuated oxidant-induced endothelial dysfunction in high cholesterol mice fed a high fat diet by enhancing nitric oxide bioavailability (71). Mouse atherosclerosis studies have shown variable pro-inflammatory and non-specific systemic effects of both genetic and pharmacological senolysis (72). While quercetin has demonstrated efficacy to induce senolysis on senescent HUVECs, MEFs, and BM-MSC in vitro, but with limited significant therapeutic effect on preadipocytes (30, 46, 47), demonstrating an efficacy profile opposite that of dasatinib. Therefore, quercetin has been investigated further in combination with dasatinib (3, 14, 30, 73–80).
Dasatinib and quercetin
The combination of dasatinib and quercetin has proven effective in mice at a dosage of 5 mg/kg of dasatinib and 50 mg/kg for quercetin (30, 81), and clears from systemic circulation within 48 h after the last dose (82, 83).
Relevant to cardiovascular disease, dasatinib and quercetin has demonstrated significant improvements in vascular smooth muscle sensitivity to nitroprusside and an enhanced ventricular left ejection fraction in mouse models of cardiac disease (84). This senotherapeutic combination also activates cardiac progenitor cells following treatment in a mouse model of cardiovascular disease (85). Both aged mice and hypercholesterolemic mice treated with dasatinib and quercetin had significant reductions in telomere associated foci, staining of aortic calcification, and osteogenic gene and protein expression (86). Another study demonstrated that dasatinib and quercetin treatment not only reduced SC numbers, but also promoted cardiomyocyte regeneration in aged mice (85). In an arteriovenous fistula chronic kidney disease mouse model, dasatinib and quercetin treatment significantly reduced p16Ink4a venous expression, indicating reduction in SC (81). Pre-treatment with this senotherapeutic combination has also been shown to reduce the quantity of SC in arterial walls and lessened the severity of abdominal aortic aneurysms in mice given angiotensin II (87).
Relevant to obesity, evaluation in mouse models have indicated that the combination of dasatinib and quercetin reduces inflammation, alleviates metabolic dysfunction pre-adiposity, and facilitates the differentiation of adipose cells into mature, insulin-responsive ones (88). Aged mice treated with dasatinib and quercetin had decreased SA-ß-Gal, p16 and p21 expression in white adipose tissue. Dasatinib and quercetin also suppressed age-related increases in pro-inflammatory SASP genes (MCP-1, TNA-α, IL-1α, IL-1β, IL-6, CXCL-2, and CXCL-10) (76). That study also demonstrated improved fasting blood glucose, glucose tolerance, reduced plasma triglycerides, and improved systemic lipid tolerance in old mice treated with dasatinib and quercetin (76). Human adipocytes treated with dasatinib and quercetin demonstrated reduced insulin resistance following xenotransplanation. Because insulin resistance is a risk factor of type 2 diabetes, these findings indicate that senotherapeutics may offer a new treatment avenue to target adipocytes expressing elevated p21Cip (89).
With respect to neurologic disease, mouse models of Alzheimer's disease have found that Tau-containing neurofibrillary tangles display senescent phenotypes with increased CDKN2A expression and brain atrophy (90). When 23-month-old tau transgenic mice were treated with dasatinib and quercetin, neurofibrillary tangle burden, ventricular enlargement, and neurodegeneration was significantly reduced (90). Furthermore, treatment with dasatinib and quercetin in a mouse model of Alzheimer's showed reduction of SC in amyloid-β plaques, reduced neuroinflammation (IL1-β, IFN-γ and TNFα), and significantly improved cognitive function (91). Another study investigating dasatinib and quercetin to treat age-related cognitive decline in male Wistar rats found that this therapeutic combination alleviated learning deficits, memory impairment, and markers of peripheral inflammation (IL-1α, IL-β, IL-4, IL-2, IL-10, MCP-1 and TNF-α) (92). Furthermore, these differences were associated with cellular changes to dendritic spine morphology, specifically hippocampal CA1 neurons and molecular alterations of histone H3 trimethylation at lysine 9 and 27 (92). While quercetin alone was found to ameliorate the progression of intervertebral disc disease in mice via the Nrf2/NF-κB axis (93), the combination of dasatinib and quercetin have shown decreases in p16INK4a, p19ARF, IL-6, and MMP13 expression while preserving cell viability (94). The neuroprotective effects of dasatinib and quercetin have also been demonstrated in a controlled cortical impact mouse model of traumatic brain injury over the time of 4 months (95). Treatment with dasatinib and quercetin significantly reduced SASP pro-inflammatory factors, IL-1β, IL-6, and attenuated neurodegeneration in mice with cortical impact traumatic brain injury (95). Furthermore, clinical behavior testing of treated mice revealed significantly improved spatial reference memory and improvement in depression-like behavior (95). Following treatment with dasatinib and quercetin, obese mice showed improved neurogenesis and a significant decrease in anxiety-like behavior (96). Specifically, dasatinib and quercetin treatment increased Nestin-positive neuronal precursor cells, double-courtin positive immature neurons, and CD133 positive ependymal cells (96).
Relevant to pulmonary disease, in a mouse model of idiopathic pulmonary fibrosis, dasatinib and quercetin treatment significantly improved lung compliance (97). This was further supported by a study evaluating dasatinib, quercetin, or dasatinib and quercetin in a mouse model of hyperoxia induced airway smooth muscle senescence (98). Mice treated with quercetin (25 mg/kg) had improved pulmonary compliance but not resistance, while dasatinib (1 mg/kg dasatinib) improved both compliance and resistance (98). To model premature neonatal pulmonary disease, fetal smooth muscle explants exposed to moderate hyperoxia (40% O2) for 7 days display significant increases in senescent markers such as SA-ß-Gal, p16, p21, p53, and the DNA damage marker gamma-H2AX (99). Following treatment with dasatinib and quercetin, there was a reduction in cells expressing SA-ß-Gal, p21, p16, and phosphorylated γ-histone family member X (99).
With respect to renal disease, treatment with dasatinib and/or quercetin reduced renal tubular senescence and ameliorated renal fibrosis in multiple mouse models of acute kidney injury (100). In models involving a high-fat diet and renal fibrosis, treatment quercetin alone decreased SC burden, reduced protein secretome markers (p16, p19, and p53), and improved renal function shown by a decreased plasma creatinine (101).
Relevant to orthopedic disease, one study found that treatment with dasatinib and quercetin decreased osteoclastic activity while promoting osteoblastic differentiation in mouse models of osteoporosis (102). Conversely, in another mouse model (accelerated aging Z24–/– model) dasatinib and quercetin treatment did not mitigate trabecular bone loss (103) while another study indicated dasatinib and quercetin treated mice may actually improve bone production of aged bone marrow derived mesenchymal stem cells (104). Dasatinib and quercetin treatment have facilitated growth of aged muscles through SC clearance and altered Igf1, Ddit4, Mmp14 gene expression in a mouse model of blunted muscle hypertrophy (105).
Finally, dasatinib and quercetin have been investigated in several other unrelated disease processes in rodent models. In a murine model of sclerodermatous graft vs. host disease, the administration of a combination of dasatinib and quercetin led to a reduction in peripheral senescence-associated secretory phenotype (SASP) cytokines, specifically IL-4, IL-6, and IL-8Rα (106). In a mouse model of doxorubicin-induced ovarian injury, both dasatinib and quercetin and fisetin treatments reduced SC presence, however treatment was unable to restore normal ovarian function (107). A study conducted in aged mice revealed that dasatinib and quercetin treatment significantly decreased SC markers p16 and p21 expression, as well as the expression of inflammatory markers Cxcl1, Il1β, Il6, Mcp1, and Tnfα in the small and large intestine (108). Notably, dasatinib and quercetin treatment also induced alterations in specific microbial signatures across ileal, cecal, and colonic regions, and within feces.
Fisetin
Fisetin is a natural flavonoid with several reported modes of action that target the anti-apoptotic pathways of senescent cells, including BCL-2/BCL-xL inhibition (48, 109) and induction of hypoxia–inducible factor−1α (HIF-1α) (3, 110). Promoting apoptosis through increased caspase activity (48, 49), fisetin successfully targeted senescent HUVECs (48). Studies have shown that treatment with 500 nM fisetin induces apoptosis in senescent cells derived from subcutaneous adipose tissue (48) and that the effect is larger in adipose cells expressing p16Ink4a and lower in p21CIP1-expressing cells (49).
In vivo, fisetin has been studied at dosages ranging from 60 to 100 mg/kg, administered orally for up to five consecutive days, exhibiting efficacy in reducing the number of senescent cells in white adipose tissue. One study evaluated the impact of fisetin on neuronal cellular senescence in a randomized controlled trial using aged sheep (111). There was a significant decrease in senescent neurons, astrocytes, and microglia in the neural tissue of sheep treated with fisetin (111). The treatment group also exhibited decreased senescent mRNA expression of SA-ß-Gal in the lung, heart, and spleen. Furthermore, the senescent marker p21 was also decreased in the liver and lung, and inflammatory markers in the lung, liver, heart and spleen following treatment (111).
A mouse model of systemic lupus erythematosus found that senescent neural cells accumulate in a hippocampal region of the brains of mice with depressive behavior (112). Oral administration of fisetin successfully reduced the number of senescent neural cells, reduced depressive behavior and limited SASP factors in the hippocampal region (112). Mouse models of chronic wounds also support a potential benefit to the senotherapeutic fisetin in chronic wound management and elimination of dermal SC (113). Targeting SC, treatment with fisetin improved muscle function and myogenic phenotypes in a mouse model of muscular dystrophy (114). Conversely, in a mouse model of chronic inflammatory myopathy, a benefit to senescent fibro-adipogenic-progenitor cells was demonstrated in mitigating exercise-induced muscle degeneration through pro-inflammatory regenerative mediators while mice without senescent adipogenic-progenitor cells exhibited muscle degeneration, which warrants further investigation (115).
Navitoclax
Navitoclax (ABT263) is a BCL-2 inhibitor with demonstrated efficacy to reduce the number of senescent bone marrow hematopoietic stem cells and muscle stem cells (50). Navitoclax reduced the senescent cell burden in HUVECs, IMR90 human lung fibroblasts, and MEFs by targeting BCL-2 proteins (116). However, navitoclax has reported side effects of neutrophil toxicity and thrombocytopenia (50, 117, 118). To mitigate these adverse effects, galacto-conjugation of navitoclax has shown a significant reduction in platelet toxicity and increased senotherapeutic specificity in both human blood samples and a murine lung cancer model (119). Explant studies have also shown that human pulmonary endothelial cells from patients with pulmonary arterial hypertension have a significant increase in SC burden and these cells undergo apoptosis when exposed to navitoclax in vitro (120).
Relevant to cardiovascular disease, mice with angiotensin II or doxorubicin induced heart failure had decreased cardiac fibrosis, hypertrophy, improved cardiac function and decreased inflammation following treatment with navitoclax (121, 122). Aged mice treated with navitoclax had a significantly reduced number of telomere associated foci, fibrosis and reduced SC, but there was no difference in cardiac ejection fraction or left ventricle mass compared with controls (123). These findings were also supported in a mouse model of ischemia-reperfusion cardiac injury, where mice treated with navitoclax had significant reduction in pro-inflammatory, profibrotic, and anti-angiogenic cytokines (interferon gamma-induced protein-10, TGF-β3, interleukin-11, interleukin-16, and fractalkine) (124). Senotherapeutic clearance of β-cells in obese metabolically dysregulated transgenic mice treated with navitoclax had decreased SASP factors, increased glucose tolerance and increased β-cell metabolism. This study suggests that pancreatic β-cell senescence may also play a role in peripheral insulin intolerance and predisposition to type 2 diabetes (125).
With relation to age related neurodegenerative disorders, navitoclax treated mice had increases in neurogenesis of hippocampal neuronal precursors and increases spatial memory (126). Cellular senescence also plays a role in chronic skin diseases like scleroderma (127–130) and psoriasis (131, 132) by promoting inflammation, fibrosis, and tissue dysfunction. One study used young and old hairless mice treated with navitoclax showed selective clearance of senescent dermal fibroblasts (133). Furthermore, aged mouse skin treated with navitoclax had increased collagen density, epidermal thickness, proliferation of keratinocytes, and decreased SASP factors such as MMP-1 and IL-6 (133).
Relevant to pulmonary disease, a mouse model of idiopathic pulmonary fibrosis found that radiation induced pulmonary fibrosis could be reversed by the clearance of senescent type II pneumocytes with Navitoclax treatment (134). However, secondary effects following clearance of heavy SC burden in chronic disease have been described, such as vessel remodeling, increased right ventricular systolic pressure, and increased cardiac hypertrophy index in rodents following navitoclax treatment (24). These findings indicate that consideration should be given to the disease severity and potential for systemic reaction following senotherapeutic treatment, particularly in more chronic disease processes. With respect to renal disease, navitoclax has been shown to specifically target senescent proximal tubular epithelial cells and resulted in improved renal function with decreased renal fibrosis demonstrated in mice (135).
The effects of navitoclax on clinical bone health are mixed when it comes to osteoporosis and bone loss with age. One study indicated that navitoclax decreased the senescent cell burden and decreased the trabecular bone in aged mice by up 60.1% in females (136). With increased cytotoxicity, use of navitoclax in vivo should be carefully considered.
Luteolin
Luteolin is a flavonoid found in celery, broccoli, dandelion, carrots, and olive oil (137) that exerts senotherapeutic properties through modulation of sirtuin 1 (SIRT1) and p53. Luteolin has largely been studied in mouse auditory cells (House Ear Institute-Organ of Corti 1) with 50 % efficacy in reversing senescence induced with hydrogen peroxide, although it appears less effective in reducing senescence in MEF (49, 51, 52). Through the upregulation of SIRT1, luteolin effectively protects against senescence induced by hydrogen peroxide (52). This mode of action was confirmed when the knockout of SIRT1 resulted in induced senescence. Luteolin also protected cells from peroxide induced senescence by decreasing p53 phosphorylation and p21 expression (52).
Curcumin
Curcumin is a senotherapeutic that selectively targets apoptotic pathways such as nuclear factor NF-kB, mitogen-activated protein kinases (MAP-kinase), p53, nuclear factor erythroid 2-related factor 2 (NRF2), AKT, COX-2 and EGFR (49, 53, 54), although its effect is relatively weak relative to other senotherapeutics due to limited bioavailability (54, 138). When attempting to concentrate curcumin above 10 μM, it exhibits genotoxic and cytotoxic effects. To mitigate these toxicity risks, a curcumin analog EF24 has shown promise against SC through the proteasomal degradation of Bcl-2 family proteins and production of reactive oxygen species (ROS), although further investigation is indicated (55).
A1331852 and A1155463
The compounds A1331852 and A1155463 are both BCL-XL inhibitors, but with a lower relative risk of BCL-2 mediated neutrophil toxicity compared to navitoclax (48, 117). Treatment with A1331852 and A1155463 resulted in apoptosis, shown through enhanced caspase3/7 activity, of senescent HUVECs and IMR90 cells, but not preadipocytes (48). Further mechanistic insights of A1331852 have shown caspase-dependent apoptosis of senescent chondrocytes and increased expression of a pro-apoptotic Bcl-2 family member called BAK (139). Utilizing live cell fluorescence resonance imaging, A1331852 was shown to interfere with binding of BCL-XL (139). In vivo, treatment of genetically modified mice with A1331852 resulted in clearance of 80% of senescent cholangiocytes, reduced expression of fibrosis-inducing growth factors, and subsequent reduction in liver fibrosis (140).
Heat shock protein 90 inhibitors
Heat shock protein 90 (HSP90) inhibitors influence protein stability and function, impacting p53′s ability to regulate apoptosis and DNA repair (141). Geldanamycin and tanespimycin (17-AAG) reduce SC viability, although geldanamycin in not particularly water soluble, while alvespimycin (17-DMAG) is (53). The targeted effects of geldanamycin and Tanespimycin are specific to HSP90. All HSP90 inhibitors have a dose-dependent senotherapeutic effect that is not cell type-specific (53). The in vivo effect of alvespimycin treatment in age related symptoms in mice, resulted in a significant reduction in kyphosis, dystonia, tremor, loss of forelimb grip strength, coat condition, ataxia, gait disorder, and overall body condition when compared to sex matched untreated mice (53). Similarly, azythromycin has been briefly studied for its ability to reduce senescent human fibroblasts by 97% (142). However, many of the senotheraputics in the HSP90 inhibitor class were initially developed and FDA approved for their antimicrobial action. Therefore, selection of these senotherapeutics must be made with antimicrobial stewardship in mind.
Piperlongumine
Piperlongumine is a therapeutic agent often paired with chemotherapeutics because of its established pro-apoptotic properties (143). The precise mechanism of piperlongumine in unknown (56). It was previously thought that piperlongumine promoted the production of reactive oxygen species; however, it has since been proven to be an ROS-independent mechanism (56). Piperlongumine has been shown to promote caspase activity and kill senescent human WI-38 fibroblasts (56). Piperlongumine, has been assessed in an ex vivo goat osteoarthritis model, demonstrating decreased p53 and p16 gene and protein expression in senescent chondrocytes in a concentration-dependent manner following treatment (57). Furthermore, piperlongumine treatment rescued the oxidative stress cause by IL-1β in cartilage explants, indicating that it has potential benefit to rescue senescent chondrocytes in OA (57).
FOXO-related peptide
The FOXO-related peptide was engineered to be a permeable peptide in p53-interaction domain in FOXO4. Treatment with this synthetic peptide induces apoptosis in senescent fibroblasts through the nuclear exclusion of p53 (58). In a concentration-dependent manner, FOXO-related peptide reduced the viability of senescent by 11.73-fold compared with control IMR90 cells (58). Importantly, the targeted design of FOXO-related peptide allows it to be safe to normal cells. In vivo, the FOXO-related peptide restored fitness, hair density, and renal function in aged mice (58).
Nutlin-3a
Nutlin-3a acts as an inhibitor of MDM2, a ubiquitin ligase responsible for downregulating p53 (59). Interestingly, Nutlin-3a has been described as both a senotherapeutic (60, 144) and a senescence inducing agent (59, 145, 146). Originally studied for its anti-cancer properties, Nutlin-3a has proven effective in inducing apoptosis in carcinomas (147, 148), melanomas, T-cell lymphoma (149) and adult T-cell leukemia (145). The senescence inducing properties of Nutlin-3a have been described in normal human and mouse fibroblasts (146, 150), non-small cell lung cancers (151), adult T-cell leukemia cells (145), cutaneous T-cell lymphoma (149), glioblastomas (152), renal carcinoma (153). It is important to note that a dose dependent cytotoxic effect has been described in both senescent and non-senescent melanoma cells at concentrations ranging from 2.5 to 10 μmol/L in vitro (60). Further studies are needed to evaluate the pharmacodynamics and pharmacokinetics of Nutlin-3a in different cellular phenotypes. In vivo, The senotherapeutic nutlin-3a has been investigated as a treatment for age-related macular degeneration (144). Treatment of a mouse model with nutlin-3a showed a significant recovery of visual function (144) and ameliorated retinal degeneration (154).
Cardiac glycosides
Another previously established drug class, cardiac glycosides has recently been described for their senotherapeutic properties (61, 62, 155). This class of drug is FDA approved for the treatment of heart failure and arrythmias such as atrial fibrillation (156, 157). Acting with Na+/K+ ATPase pump inhibition, cardiac glycosides target SCs with higher H+ concentrations and slightly depolarized membranes (62, 155). On the in vitro scale, the cardiac glycoside digoxin had significant senotherapeutic activity against A549 tumor cells, primary human BJ fibroblasts, and osteoarthritic chondrocytes (62). However, efficacy in mouse embryo fibroblasts was not seen (62). Treatment with Digoxin had significant senotherapeutic activity in a mouse model of IPF (62). However, research on the senotherapeutic uses of cardiac glycosides is still in its infancy and further investigation is needed to determine efficacy against different senescent phenotypes in vivo.
Aspirin
The non-steroidal anti-inflammatory, aspirin, has an established repertoire in reducing endothelial senescence (158–160). Recently, aspirin has been investigated for its senotherapeutic abilities to ameliorate the long term effects on patients that have received chemotherapy and radiation (63). This study found that aspirin suppresses p53 and p21 accumulation in doxorubicin induced senescent human fibroblasts and murine embryonic fibroblasts (63). Cyclooxygenase 2 (COX2) knockout mouse embryonic fibroblasts that underwent the same treatment had a significant reduction in p53 accumulation (63). This data suggests that aspirin's senotherapeutic activity is through the inhibition of COX2. In vivo, aspirin treatment significantly reduce amyloid-β42 induced senescent neuronal cells by upregulating sirtuin-1 (SIRT1), a key regulator in cell aging (161). Aspirin has also demonstrated senotherapeutic potential in doxorubicin-treated mouse models through the reduction of SA-ß-Gal staining in liver, spleen, pancreas, and lung tissues when compared to controls (63).
Second generation senotherapeutics
Following the identification of readily available “first generation” senotherapeutics, the so-called “second generation” of these drugs have been more recently identified and engineered compounds (Table 2). The information available pertaining to preclinical evidence for their application in veterinary medicine is summarized below.
Oligosaccharide coated nanoparticles
The development of oligosaccharide coated nanoparticles containing drug encapsulated beads such as doxorubicin or navitoclax are capable of inducing apoptosis in senescent cells (162). Direct targeting through endocytosis allows these drugs to be delivered intracellularly with minimal reported systemic side effects to date (162).
Senotherapeutic vaccines
Senotherapeutic vaccinations have evolved at the intersection of oncology and immunotherapy. Chemotherapy-induced tumor senescence has been demonstrated to limit further tumor growth and can allow for immunomodulation through vaccination against the static tumor cell type, leading to the development of senotherapeutic vaccination (163–166). A murine model of senescence-related aging implemented a CD153 vaccine to effectively clear senescent T-cells (164). In another mouse model, transcriptomic analyses were used to evaluate vascular endothelial cells for senescent cell markers which identified transmembrane glycoprotein nonmetastatic melanoma protein B (GPNMB) as a sero-antigen candidate (165). Following vaccination with GPNMB, improvement in aging phenotypes and male mouse lifespans were noted (165). While these targeted approaches are promising, additional preclinical modeling and Phase 1 clinical testing are needed to ensure their safety and efficacy (164–168).
CAR-T targeting senescence
Recent attention has focused on chimeric antigen receptor T cell (CAR T cell) modulated treatments targeting SC accumulation. The urokinase-type plasminogen activator receptor (uPAR) was identified as an expressed SC surface antigen, making it a potential target for immunomodulatory senotherapeutics (169). Treatment with uPAR-specific CAR T cell therapy demonstrated efficacy in reducing liver fibrosis and improving treatment outcomes in mouse models of lung adenocarcinoma, which warrants further investigation (169).
Human clinical trials evaluating senotherapeutics
At present, human clinical trials are underway to assess the safety and effectiveness of senotherapeutic agents. These trials cover a diverse spectrum of medical conditions, including idiopathic pulmonary fibrosis, hematopoietic stem cell transplants, chronic diabetic kidney disease, childhood cancer survivors, age related osteoporosis, Alzheimer's disease, frailty, macular degeneration, osteoarthritis, and viral infections such as COVID-19. While initial findings indicate promising results in terms of medication safety and patient tolerance, many of these clinical trials are advancing to phase two and necessitate a randomized, blinded, placebo-controlled study design. Current trials are summarized in Table 3.
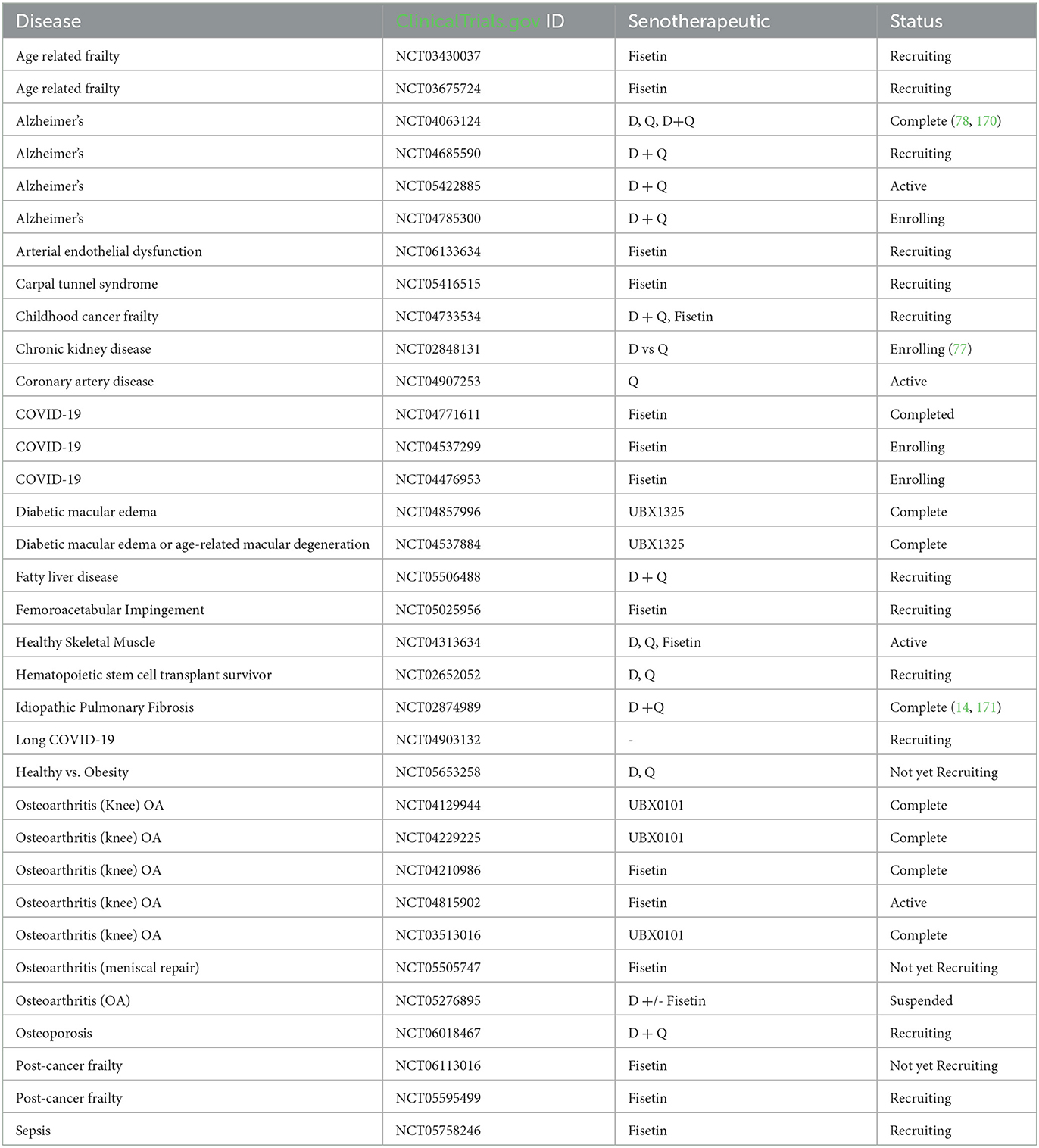
Table 3. Clinical trials involving senotherapeutic, as listed on clinicaltrials.gov (as of December 2023).
Senotherapeutic potential in veterinary species
The use of first generation senotherapeutics (e.g., dasatinib) in veterinary medicine has largely been studied in the context of neoplasia (31, 32, 172–174). Moving forward, evaluation of senotherapeutics to specifically target SC in the context of naturally occurring veterinary diseases presents a translational opportunity to explore the long-term safety and efficacy of senotherapeutics in spontaneous disease models (Figure 3). Examples of potential applications of senotherapeutics in veterinary medicine include treatment of osteoarthritis which is prevalent in dogs, horses and cats (175, 176), or more specific disease processes such as idiopathic pulmonary fibrosis in West Highland Terriers (177, 178), canine cardiomyopathies (179–181), or renal disease and sarcopenia in cats (182–185) (Figure 4). These collaborative prospects offer a new avenue to bridge the gap between in vivo rodent models and clinical trials in people, while simultaneously benefiting veterinary species suffering from similar disease processes.
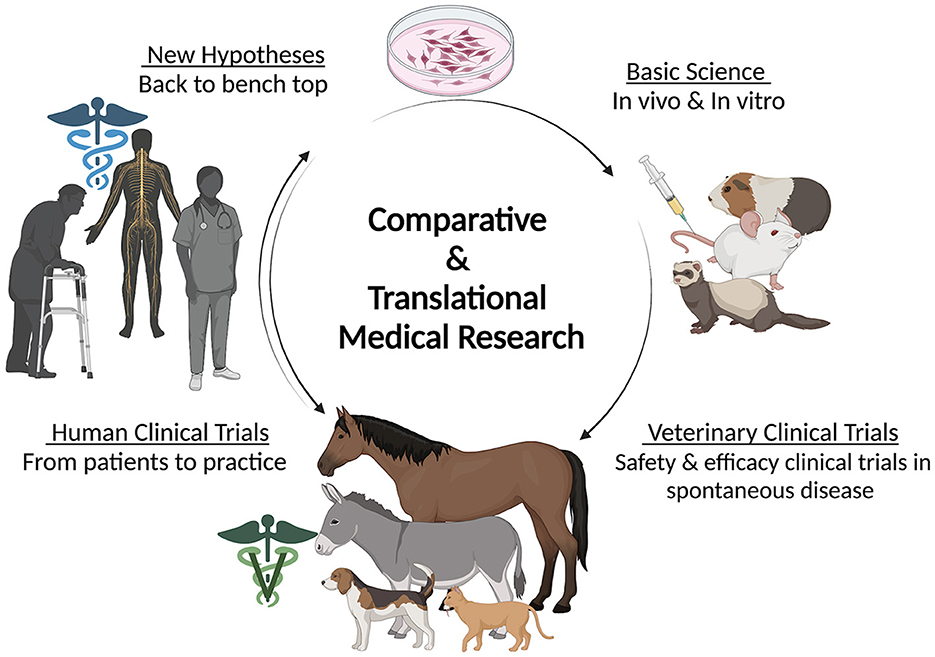
Figure 3. Diagram depicting the circular flow of comparative and translational medical research including the contribution of veterinary species in clinical trials. Created with BioRender.com.
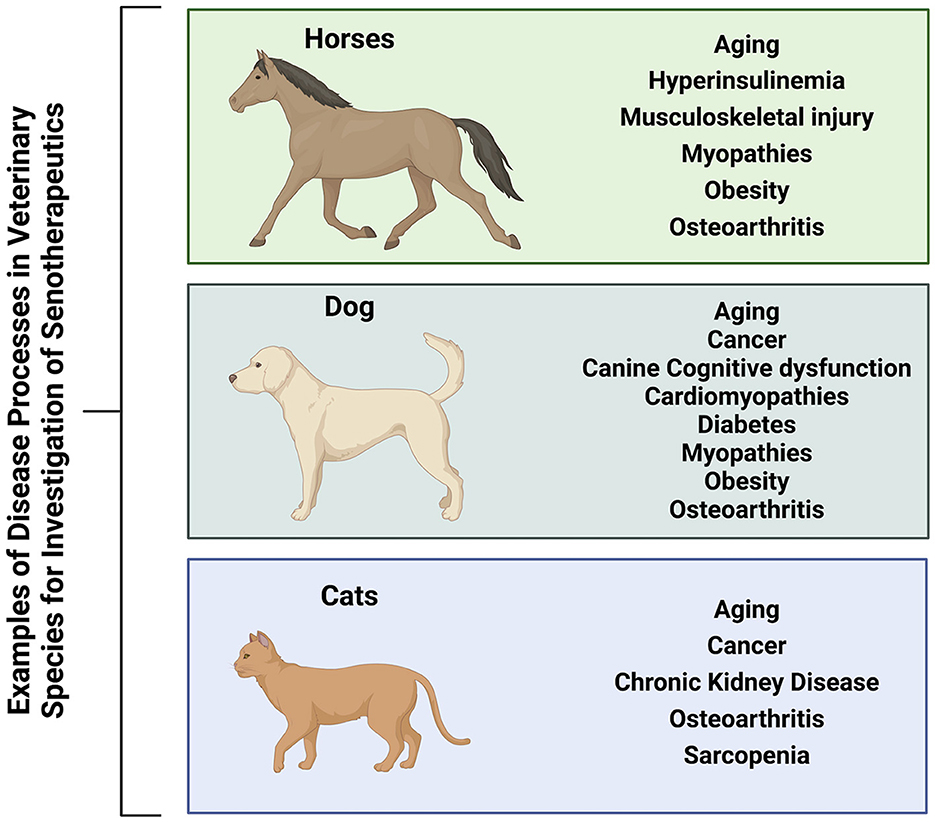
Figure 4. Veterinary species (horses, dogs, and cats) suffer from many similar disease processes to humans that may benefit from treatment with senotherapeutics, serving as preclinical naturally occurring disease models to also provide translational data for future human clinical trials. Created with BioRender.com.
Osteoarthritis in veterinary species
To highlight a few disease processes for example, osteoarthritis (OA) is found to be a common cause of pain and lameness in horses (186, 187). Approximately 33% of the equine population overall in the US is estimated to be affected by OA (188), and that prevalence climbs to 50% at 15 years of age and 80 to 90% in horses over 30 years of age (189–191). Therefore, OA risk is highly associated with aging, which has been similarly reported in humans (175, 176). In conditions such as osteoarthritis, rheumatoid arthritis, and age-related frailty, senescent cells can accumulate in musculoskeletal tissues. Transplantation of SC has precipitated an OA-like phenotype in mice when compared to transplantation of non-SC (192). A recent study evaluating synovial fluid in healthy horses and horses with OA found an increased number of senescent mesenchymal stem cells (193). The senescent cells displayed impaired chondrogenic differentiation (193) when compared with a non-senescent cell population, making them potential targets for senotherapeutics. Murine models of rheumatoid arthritis (RA) found that dasatinib was protective against RA by inhibiting osteoclastogenesis through immunomodulatory effects (194). Additional murine studies have shown that SC interact with synovial cells and that dasatinib and/or quercetin have been effective in ameliorating cartilage damage and pain due to OA, as well as alleviating post-menopausal osteoporosis (73, 75, 195, 196). These findings collectively support further investigation of senotherapeutics as a potential disease modifying treatment in OA.
Obesity in veterinary species
Senescent cells have been found to accumulate in adipose tissue which is associated with chronic low-grade inflammation and insulin resistance (17, 76, 197–200). Senotherapeutics have been shown to reduce senescent cell burden in diet-induced obesity, to alleviate metabolic dysfunction, and to restore the capacity of preadipocytes to differentiate into functional insulin-responsive fat cells (88). Obesity in companion animals is prevalent in the US (e.g., 20–45% of riding horses) and has increasingly been recognized as a factor associated with insulin resistance and OA progression. Implementation of senotherapeutics in the population of horses commonly treated for OA has potential benefit to simultaneously alleviate multiple co-morbidities (2, 201–203). Displaying an age-related phenotype, metabolic dysregulations may precipitate insulin resistance (204, 205). Hyperinsulinemia induces human hepatocyte senescence (206), which has been shown to be attenuated by dasatinib and quercetin. Interestingly, increased cellular senescence has also been observed in equine adipose-derived stem cells in horses with equine metabolic syndrome and is associated with impaired antigen stability and clonogenic potential (207). SC have altered metabolism compared to non-SC (208) and likely contribute to impaired fat metabolism and insulin resistance. Elimination of these cells may aid in healthy weight loss and return to metabolic homeostasis, potentially improving treatment of insulin resistance and associated disease processes (76).
Cardiac disease in veterinary species
Senescent cells have been found to contribute to the progression of atherosclerosis, arterial stiffness, and heart failure in human cardiovascular disease (120, 122, 209–211). The risk of heart disease increases with age and precipitates hemodynamic instability. Senotherapeutics have been investigated in canine myxomatous mitral valve disease (212). Valve interstitial cells that were treated with quercetin or quercetin plus dasatinib showed a decrease in SC and p53 expression (212). The decrease in SC is achieved through PI3K/AKT/mTOR antagonism which facilitates the reversal of myofibroblast senescence and promoting autophagy (213). Therefore, current therapeutic approaches for mitral valve disease could be expanded to include senotherapeutics which may slow disease progression (212).
Renal disease in veterinary species
Senescent cells can accumulate in renal tissue, contributing to inflammation, fibrosis, and impaired function (100, 135, 214). Eliminating dysfunctional cells may reduce inflammation, decrease fibrosis, slow down the progression of kidney damage, and preserve renal function (100, 214). Spontaneous chronic kidney disease (CKD) is well-described in cats (182–185). Feline patients suffering from CKD have renal senescence, telomere shortening, and nitrosative stress in renal cells (215). With similar pathologic findings to humans (216, 217), they represent a naturally occurring model for study of senotherapeutics (215). In vitro studies utilizing feline renal cells have shown a dose dependent correlation with radiation and SC (218). Further studies can utilize this data to understand the etiopathology of radiotoxicity induced renal senescence and investigation of senotherapeutics in vivo in this context (Figure 4).
Limitations of senotherapy in veterinary species
Despite the potential benefits, there are prominent limitations that call for collaborative research to fully understand the breadth and depth of senotherapy in veterinary species. Studies in laboratory species and human clinical trials are still examining the full extent of side effects and long-term effects of senotherapeutics. It remains crucial to also examine the impact this drug class has on healthy cells and to not lose focus on the unknown effects on cell and tissue homeostasis. This raises concerns for off-target effects and toxicity in veterinary species since there are metabolic nuances depending on which species is studied. SC have different markers and characteristics depending on the tissue and the cause of senescence. The heterogeneity in SC makes it difficult to develop universally effective protocol, especially when considering effective dosing across species. Lastly, it is important to recognize that senescent cells could become resistant to these therapies. Similar with anthelmintic use and antibiotic stewardship, judicious use of medication relies on accurate diagnostics, clinical monitoring, and continued research.
Discussion
Senotherapeutics attenuate tissue inflammation and restore progenitor cell function to delay, prevent or alleviate symptoms in multiple age-related diseases (3). The first generation senotherapeutics have been largely deemed safe and with varying degrees of efficacy (1, 135, 219, 220), with the exception of navitoclax and curcumin that have demonstrated cytotoxicity (28, 31, 39, 40). Optimal dosage and duration for various disease processes have not been fully explored. Although short intermittent dosing regimens appear effective and offer clinical translation with fewer negative off-target effects compared to sustained administration with currently available drugs. Administration for extensive periods may consequently deplete cell types necessary for remodeling (1, 3, 49, 221, 222). The majority of senotherapeutic pre-clinical trials have been conducted in induced murine models (49, 53, 83, 101, 114, 135). Further development of senotherapeutics for use in naturally occurring companion animal models of disease may inform future human clinical trials regarding pharmacokinetic, pharmacodynamic and interactions with other drugs that may be administered concurrently (223–226).
Treatments targeting senescent cells and the SASP have broad potential implications in the field of veterinary medicine as the hallmarks of aging (6) are highly conserved across species (227, 228). These hallmarks include DNA damage (229), telomere shortening (230, 231), aberrant proteostasis (232), epigenetic modifications (233), altered nutritional signaling (234, 235), cell senescence (193, 213, 215, 231, 236), stem cell depletion (237), mitochondrial dysfunction (238, 239), and abnormal inflammatory signaling (240, 241). Treatment of age-related diseases in veterinary species may further serve to bolster preclinical evidence for use in humans. To date, the majority of preclinical or in vitro studies have largely focused on investigating dasatinib, quercetin, or fisetin (1, 171). Dasatinib is well known in the oncology realm and has been used to treat a variety of human, canine, and feline cancers (64, 65, 172–174, 242). Additionally, quercetin has also been beneficial in treating canine neoplasia including osteosarcoma cells (243, 244). Similar to humans, sarcopenia (185, 245–247) and decreased bone density (248, 249) is commonly associated with age in both felines and canines. The use of senotherapeutics for Alzheimer's disease is being evaluated (74, 78, 170, 250) and canine or feline cognitive dysfunction may serve as a comparative naturally occurring model (249, 251–253). Osteoarthritis is also significantly linked with age and cellular senescence (193, 196). Due to similarities in cartilage thickness and joint volume, equine models are well suited comparisons of human osteoarthritis (175, 176, 254). Greater recognition of similarities in animal preclinical models to human aging related disorders has the potential to advance the field of senotherapeutics to the benefit of both humans and veterinary species.
The current state-of-play in the field of senotherapeutics presents multiple avenues for future research. Further development of species- and tissue-specific biomarkers for senescent cell abundance, SASP mediators, and senescent phenotypes (i.e., senotype), a field termed “gerodiagnostics” will be critical to fully understand the effects of senotherapeutics and reduce off-target effects with their administration (1, 3, 15). Generation of comprehensive atlases of senescent cells that arise during aging and disease states across multiple tissue types and specific to the target species of interest are warranted (221). Toward this goal, the National Institute of Aging (NIA) has established a common fund's cellular senescence network (SenNet) program to generate atlases for humans and mice (221), to facilitate identification of senotype specific biomarkers that will help to identify the therapeutic window for senotherapeutic interventions and to guide dosage, timing, and duration of senotherapeuthic treatments in the aging population. Future directions for new drug development may include evaluation of clearance of senescent cells using genetic and epigenetic approaches or interventions that modulate SASP (1, 3, 15). Correlation of interventions with more specifically defined veterinary gerodiagnostics and development of therapeutic strategies targeting fundamental aging processes such as dietary changes and exercise will further the field. Expanded clinical trials to ensure safety, benefit, and target engagement first in serious disease processes followed by other senescence associated disorders are indicated. Results of ongoing clinical trials will yield insights and informative data into the role of cellular senescence as a therapeutic target for age-related disorders (1, 3, 15). Evaluation of senotherapeutics in different age groups will identify limits of biomarkers and therapeutic benefit in different species and signalments. Future clinical trials could facilitate identification of systemic markers that could be associated with senotherapeutic responsive individuals given inter-individual variability in aging (e.g., circulating SASP factors, cytokines such as TGF-ß). Finally, more comprehensive investigation of the mechanisms of action of senotherapeutics is indicated as proposed mechanisms have other functions outside of addressing senescence, thus confounding the contributions of each response during tissue repair process and aging. Addressing these scientific and regulatory challenges will be critical if senotherapeutics are to be used outside of clinical trials and in veterinary medicine.
Conclusions
Cellular senescence is considered a “double-edged” sword in the balance of disease and health states (4), and addressing states of immunosenescence, both systemically and locally, represents a novel treatment of age-related diseases in veterinary medicine (255). Senotherapeutic drugs identified via bioinformatic analyses present a novel therapeutic strategy to selectively clear senescent cells with broad implications to aging related disorders. Dasatinib, quercetin and fisetin represent the most studied compounds to date and are currently under investigation in human clinical trials. Further exploration of senotherapeutic applications in companion animals, including enhanced understanding of mechanism of action and investigation of route of delivery, bioabsorption, and potential off-target effects represents a new frontier to extend healthspan in veterinary patients.
Author contributions
ZW: Data curation, Investigation, Methodology, Writing—original draft, Writing—review & editing. LC: Conceptualization, Data curation, Methodology, Writing—original draft, Writing—review & editing. SD: Conceptualization, Data curation, Formal analysis, Funding acquisition, Investigation, Project administration, Resources, Software, Supervision, Visualization, Writing—original draft, Writing—review & editing. LP: Conceptualization, Data curation, Formal analysis, Funding acquisition, Investigation, Methodology, Project administration, Resources, Software, Supervision, Validation, Visualization, Writing—original draft, Writing—review & editing.
Funding
The author(s) declare that financial support was received for the research, authorship, and/or publication of this article. This work was supported by Animal Health and Disease Grant Project Accession No. COLV 2023-01 from the USDA National Institute of Food and Agriculture. Stipend support for ZW was provided by the NIH/NCATS Colorado CTSA Grant Number T32TR004366.
Conflict of interest
The authors declare that the research was conducted in the absence of any commercial or financial relationships that could be construed as a potential conflict of interest.
Publisher's note
All claims expressed in this article are solely those of the authors and do not necessarily represent those of their affiliated organizations, or those of the publisher, the editors and the reviewers. Any product that may be evaluated in this article, or claim that may be made by its manufacturer, is not guaranteed or endorsed by the publisher.
Author disclaimer
Contents are the authors' sole responsibility and do not necessarily represent official NIH views.
References
1. Chaib S, Tchkonia T, Kirkland JL. Cellular senescence and senolytics: the path to the clinic. Nat Med. (2022) 28:1556–68. doi: 10.1038/s41591-022-01923-y
2. Kirkland JL, Tchkonia T, Zhu Y, Niedernhofer LJ, Robbins PD. The clinical potential of senolytic drugs. J Am Geriatr Soc. (2017) 65:2297–301. doi: 10.1111/jgs.14969
3. Kirkland JL, Tchkonia T. Senolytic drugs: from discovery to translation. J Intern Med. (2020) 288:518–36. doi: 10.1111/joim.13141
4. González-Gualda E, Baker AG, Fruk L, Muñoz-Espín D, A. guide to assessing cellular senescence in vitro and in vivo. FEBS J. (2021) 288:56–80. doi: 10.1111/febs.15570
5. Birch J, Gil J. Senescence and the SASP: many therapeutic avenues. Genes Dev. (2020) 34:1565–76. doi: 10.1101/gad.343129.120
6. López-Otín C, Blasco MA, Partridge L, Serrano M, Kroemer G. The hallmarks of aging. Cell. (2013) 153:1194–217. doi: 10.1016/j.cell.2013.05.039
7. Kennedy BK, Berger SL, Brunet A, Campisi J, Cuervo AM, Epel ES, et al. Geroscience: linking aging to chronic disease. Cell. (2014) 159:709–13. doi: 10.1016/j.cell.2014.10.039
8. Hayflick L, Moorhead PS. The serial cultivation of human diploid cell strains. Exp Cell Res. (1961) 25:585–621. doi: 10.1016/0014-4827(61)90192-6
9. Prata LGPL, Ovsyannikova IG, Tchkonia T, Kirkland JL. Senescent cell clearance by the immune system: emerging therapeutic opportunities. Semin Immunol. (2018) 40:101275. doi: 10.1016/j.smim.2019.04.003
10. Pereira BI, Devine OP, Vukmanovic-Stejic M, Chambers ES, Subramanian P, Patel N, et al. Senescent cells evade immune clearance via HLA-E-mediated NK and CD8+ T cell inhibition. Nat Commun. (2019) 10:2387. doi: 10.1038/s41467-019-10335-5
11. De Cecco M, Ito T, Petrashen AP, Elias AE, Skvir NJ, Criscione SW, et al. Author Correction: L1 drives IFN in senescent cells and promotes age-associated inflammation. Nature. (2019) 572:E5. doi: 10.1038/s41586-018-0784-9
12. Xu D, Tahara H. The role of exosomes and microRNAs in senescence and aging. Adv Drug Deliv Rev. (2013) 65:368–75. doi: 10.1016/j.addr.2012.07.010
13. Victoria B, Nunez Lopez YO, Masternak MM. MicroRNAs and the metabolic hallmarks of aging. Mol Cell Endocrinol. (2017) 455:131–47. doi: 10.1016/j.mce.2016.12.021
14. Justice JN, Nambiar AM, Tchkonia T, LeBrasseur NK, Pascual R, Hashmi SK, et al. Senolytics in idiopathic pulmonary fibrosis: results from a first-in-human, open-label, pilot study. EBioMedicine. (2019) 40:554–63. doi: 10.1016/j.ebiom.2018.12.052
15. Robbins PD, Jurk D, Khosla S, Kirkland JL, LeBrasseur NK, Miller JD, et al. Senolytic drugs: reducing senescent cell viability to extend health span. Annu Rev Pharmacol Toxicol. (2021) 61:779–803. doi: 10.1146/annurev-pharmtox-050120-105018
16. Tchkonia T, Zhu Y, van Deursen J, Campisi J, Kirkland JL. Cellular senescence and the senescent secretory phenotype: therapeutic opportunities. J Clin Invest. (2013) 123:966–72. doi: 10.1172/JCI64098
17. Xu M, Palmer AK, Ding H, Weivoda MM, Pirtskhalava T, White TA, et al. Targeting senescent cells enhances adipogenesis and metabolic function in old age. Elife. (2015) 4:e12997. doi: 10.7554/eLife.12997.028
18. Zhu Y, Armstrong JL, Tchkonia T, Kirkland JL. Cellular senescence and the senescent secretory phenotype in age-related chronic diseases. Curr Opin Clin Nutr Metab Care. (2014) 17:324–8. doi: 10.1097/MCO.0000000000000065
19. Frescas D, Hall BM, Strom E, Virtuoso LP, Gupta M, Gleiberman AS, et al. Murine mesenchymal cells that express elevated levels of the CDK inhibitor p16(Ink4a) in vivo are not necessarily senescent. Cell Cycle. (2017) 16:1526–33. doi: 10.1080/15384101.2017.1339850
20. Kaur J, Farr JN. Cellular senescence in age-related disorders. Transl Res. (2020) 226:96–104. doi: 10.1016/j.trsl.2020.06.007
21. Dimri GP, Lee X, Basile G, Acosta M, Scott G, Roskelley C, et al. biomarker that identifies senescent human cells in culture and in aging skin in vivo. Proc Natl Acad Sci U S A. (1995) 92:9363–7. doi: 10.1073/pnas.92.20.9363
22. Ressler S, Bartkova J, Niederegger H, Bartek J, Scharffetter-Kochanek K, Jansen-Dürr P, et al. p16INK4A is a robust in vivo biomarker of cellular aging in human skin. Aging Cell. (2006) 5:379–89. doi: 10.1111/j.1474-9726.2006.00231.x
23. Childs BG, Durik M, Baker DJ, van Deursen JM. Cellular senescence in aging and age-related disease: from mechanisms to therapy. Nat Med. (2015) 21:1424–35. doi: 10.1038/nm.4000
24. Di Micco R, Krizhanovsky V, Baker D, d'Adda di Fagagna F. Cellular senescence in ageing: from mechanisms to therapeutic opportunities. Nat Rev Mol Cell Biol. (2021) 22:75–95. doi: 10.1038/s41580-020-00314-w
25. Safwan-Zaiter H, Wagner N, Wagner K-D. P16INK4A—more than a senescence marker. Life. (2022) 12:1332. doi: 10.3390/life12091332
26. Chen J, Huang X, Halicka D, Brodsky S, Avram A, Eskander J, et al. Contribution of p16INK4a and p21CIP1 pathways to induction of premature senescence of human endothelial cells: permissive role of p53. Am J Physiol Heart Circ Physiol. (2006) 290:H1575–86. doi: 10.1152/ajpheart.00364.2005
27. Chen LS, Balakrishnan K, Gandhi V. Inflammation and survival pathways: chronic lymphocytic leukemia as a model system. Biochem Pharmacol. (2010) 80:1936–45. doi: 10.1016/j.bcp.2010.07.039
28. Bessler H, Bergman M, Salman H, Cohen AM, Fenig E, Djaldetti M. Factor(s) released from irradiated B-CLL cells induce apoptosis in leukemic lymphocytes. Cancer Lett. (2002) 179:103–8. doi: 10.1016/S0304-3835(01)00868-0
29. Basisty N, Kale A, Jeon OH, Kuehnemann C, Payne T, Rao C, et al. A proteomic atlas of senescence-associated secretomes for aging biomarker development. PLoS Biol. (2020) 18:e3000599. doi: 10.1371/journal.pbio.3000599
30. Zhu Y, Tchkonia T, Pirtskhalava T, Gower AC, Ding H, Giorgadze N, et al. The Achilles' heel of senescent cells: from transcriptome to senolytic drugs. Aging Cell. (2015) 14:644–58. doi: 10.1111/acel.12344
31. Chan CM, Jing X, Pike LA, Zhou Q, Lim D-J, Sams SB, et al. Targeted inhibition of Src kinase with dasatinib blocks thyroid cancer growth and metastasis. Clin Cancer Res. (2012) 18:3580–91. doi: 10.1158/1078-0432.CCR-11-3359
32. Breccia M, Alimena G. Activity and safety of dasatinib as second-line treatment or in newly diagnosed chronic phase chronic myeloid leukemia patients. BioDrugs. (2011) 25:147–57. doi: 10.2165/11591840-000000000-00000
33. Noren Hooten N, Evans MK. Techniques to induce and quantify cellular senescence. J Vis Exp. (2017) 1:55533. doi: 10.3791/55533
34. Schneider EL. Aging and cultured human skin in fibroblasts. J Invest Dermatol. (1979) 73:15–8. doi: 10.1111/1523-1747.ep12532753
35. Serrano M, Lin AW, McCurrach ME, Beach D, Lowe SW. Oncogenic ras provokes premature cell senescence associated with accumulation of p53 and p16INK4a. Cell. (1997) 88:593–602. doi: 10.1016/S0092-8674(00)81902-9
36. Sati S, Bonev B, Szabo Q, Jost D, Bensadoun P, Serra F, et al. 4D Genome rewiring during oncogene-induced and replicative senescence. Mol Cell. (2020) 78:522–38.e9. doi: 10.1016/j.molcel.2020.03.007
37. Copp ME, Flanders MC, Gagliardi R, Gilbertie JM, Sessions GA, Chubinskaya S, et al. The combination of mitogenic stimulation and DNA damage induces chondrocyte senescence. Osteoarthritis Cartilage. (2021) 29:402–12. doi: 10.1016/j.joca.2020.11.004
38. Fuhrmann-Stroissnigg H, Santiago FE, Grassi D, Ling Y, Niedernhofer LJ, Robbins PD. SA-β-galactosidase-based screening assay for the identification of senotherapeutic drugs. J Vis Exp. (2019) 28:148. doi: 10.3791/58133
39. Aird KM, Zhang R. Detection of senescence-associated heterochromatin foci (SAHF). Methods Mol Biol. (2013) 965:185–96. doi: 10.1007/978-1-62703-239-1_12
40. Meuter A, Rogmann L-M, Winterhoff BJ, Tchkonia T, Kirkland JL, Morbeck DE. Markers of cellular senescence are elevated in murine blastocysts cultured in vitro: molecular consequences of culture in atmospheric oxygen. J Assist Reprod Genet. (2014) 31:1259–67. doi: 10.1007/s10815-014-0299-8
41. Desbats MA, Zumerle S, Alimonti A. Epiregulation of the SASP makes good neighbors. Nat Aging. (2021) 1:420–1. doi: 10.1038/s43587-021-00068-w
42. Rodríguez S, Bermúdez LG, González D, Bernal C, Cañas A, Morales-Ruíz T, Henríquez B, Rojas A. Transcriptional regulation of CDKN2A/p16 by sirtuin 7 in senescence. Mol Med Rep. (2022) 26:12861. doi: 10.3892/mmr.2022.12861
43. Zhang B, Long Q, Wu S, Xu Q, Song S, Han L, et al. KDM4 orchestrates epigenomic remodeling of senescent cells and potentiates the senescence-associated secretory phenotype. Nat Aging. (2021) 1:454–72. doi: 10.1038/s43587-021-00063-1
44. Spinelli R, Florese P, Parrillo L, Zatterale F, Longo M, D'Esposito V, et al. ZMAT3 hypomethylation contributes to early senescence of preadipocytes from healthy first-degree relatives of type 2 diabetics. Aging Cell. (2022) 21:e13557. doi: 10.1111/acel.13557
45. Krishnamurthy J, Torrice C, Ramsey MR, Kovalev GI, Al-Regaiey K, Su L, et al. Ink4a/Arf expression is a biomarker of aging. J Clin Invest. (2004) 114:1299–307. doi: 10.1172/JCI22475
46. Olave NC, Grenett MH, Cadeiras M, Grenett HE, Higgins PJ. Upstream stimulatory factor-2 mediates quercetin-induced suppression of PAI-1 gene expression in human endothelial cells. J Cell Biochem. (2010) 111:720–6. doi: 10.1002/jcb.22760
47. Bruning A. Inhibition of mTOR signaling by quercetin in cancer treatment and prevention. Anticancer Agents Med Chem. (2013) 13:1025–31. doi: 10.2174/18715206113139990114
48. Zhu Y, Doornebal EJ, Pirtskhalava T, Giorgadze N, Wentworth M, Fuhrmann-Stroissnigg H, et al. New agents that target senescent cells: the flavone, fisetin, and the BCL-XL inhibitors, A1331852 and A1155463. Aging. (2017) 9:955–63. doi: 10.18632/aging.101202
49. Yousefzadeh MJ, Zhu Y, McGowan SJ, Angelini L, Fuhrmann-Stroissnigg H, Xu M, et al. Fisetin is a senotherapeutic that extends health and lifespan. EBioMedicine. (2018) 36:18–28. doi: 10.1016/j.ebiom.2018.09.015
50. Chang J, Wang Y, Shao L, Laberge R-M, Demaria M, Campisi J, et al. Clearance of senescent cells by ABT263 rejuvenates aged hematopoietic stem cells in mice. Nat Med. (2016) 22:78–83. doi: 10.1038/nm.4010
51. Aziz N, Kim M-Y, Cho JY. Anti-inflammatory effects of luteolin: a review of in vitro, in vivo, and in silico studies. J Ethnopharmacol. (2018) 225:342–58. doi: 10.1016/j.jep.2018.05.019
52. Zhu RZ Li BS, Gao SS, Seo JH, Choi B-M. Luteolin inhibits H2O2-induced cellular senescence via modulation of SIRT1 and p53. Korean J Physiol Pharmacol. (2021) 25:297–305. doi: 10.4196/kjpp.2021.25.4.297
53. Fuhrmann-Stroissnigg H, Ling YY, Zhao J, McGowan SJ, Zhu Y, Brooks RW, et al. Identification of HSP90 inhibitors as a novel class of senolytics. Nat Commun. (2017) 8:422. doi: 10.1038/s41467-017-00314-z
54. Beltzig L, Frumkina A, Schwarzenbach C, Kaina B. Cytotoxic, genotoxic and senolytic potential of native and micellar curcumin. Nutrients. (2021) 13:2385. doi: 10.3390/nu13072385
55. Li W, He Y, Zhang R, Zheng G, Zhou D. The curcumin analog EF24 is a novel senolytic agent. Aging. (2019) 11:771–82. doi: 10.18632/aging.101787
56. Wang Y, Chang J, Liu X, Zhang X, Zhang S, Zhang X, et al. Discovery of piperlongumine as a potential novel lead for the development of senolytic agents. Aging. (2016) 8:2915–26. doi: 10.18632/aging.101100
57. Kapoor N, Bhattacharjee A, Chakraborty S, Katti DS. Piperlongumine mediates amelioration of osteoarthritis via inhibition of chondrocyte senescence and inflammation in a goat ex vivo model. Eur J Pharmacol. (2023) 961:176136. doi: 10.1016/j.ejphar.2023.176136
58. Baar MP, Brandt RMC, Putavet DA, Klein JDD, Derks KWJ, Bourgeois BRM, et al. Targeted apoptosis of senescent cells restores tissue homeostasis in response to chemotoxicity and aging. Cell. (2017) 169:132–47.e16. doi: 10.1016/j.cell.2017.02.031
59. Xu X, Liu Q, Zhang C, Ren S, Xu L, Zhao Z, et al. Inhibition of DYRK1A-EGFR axis by p53-MDM2 cascade mediates the induction of cellular senescence. Cell Death Dis. (2019) 10:282. doi: 10.1038/s41419-019-1521-5
60. Vilgelm AE, Pawlikowski JS, Liu Y, Hawkins OE, Davis TA, Smith J, et al. Mdm2 and aurora kinase a inhibitors synergize to block melanoma growth by driving apoptosis and immune clearance of tumor cells. Cancer Res. (2015) 75:181–93. doi: 10.1158/0008-5472.CAN-14-2405
61. Guerrero A, Herranz N, Sun B, Wagner V, Gallage S, Guiho R, et al. Cardiac glycosides are broad-spectrum senolytics. Nat Metab. (2019) 1:1074–88. doi: 10.1038/s42255-019-0122-z
62. Triana-Martínez F, Picallos-Rabina P, Da Silva-Álvarez S, Pietrocola F, Llanos S, Rodilla V, et al. Identification and characterization of Cardiac Glycosides as senolytic compounds. Nat Commun. (2019) 10:4731. doi: 10.1038/s41467-019-12888-x
63. Feng M, Kim J, Field K, Reid C, Chatzistamou I, Shim M. Aspirin ameliorates the long-term adverse effects of doxorubicin through suppression of cellular senescence. FASEB Bioadv. (2019) 1:579–90. doi: 10.1096/fba.2019-00041
64. Yoshimura S, Panetta JC, Hu J, Li L, Gocho Y, Du G, et al. Preclinical pharmacokinetic and pharmacodynamic evaluation of dasatinib and ponatinib for the treatment of T-cell acute lymphoblastic leukemia. Leukemia. (2023) 37:1194–203. doi: 10.1038/s41375-023-01900-5
65. Samad A, Huq MA, Rahman MS. Bioinformatics approaches identified dasatinib and bortezomib inhibit the activity of MCM7 protein as a potential treatment against human cancer. Sci Rep. (2022) 12:1539. doi: 10.1038/s41598-022-05621-0
66. Liu H, Guo X, Chu Y, Lu S. Heart protective effects and mechanism of quercetin preconditioning on anti-myocardial ischemia reperfusion (IR) injuries in rats. Gene. (2014) 545:149–55. doi: 10.1016/j.gene.2014.04.043
67. Castillo RL, Herrera EA, Gonzalez-Candia A, Reyes-Farias M, de la Jara N, Peña JP, Carrasco-Pozo C. Quercetin prevents diastolic dysfunction induced by a high-cholesterol diet: role of oxidative stress and bioenergetics in hyperglycemic rats. Oxid Med Cell Longev. (2018) 2018:7239123. doi: 10.1155/2018/7239123
68. Cheserek MJ, Wu G, Li L, Li L, Karangwa E, Shi Y, et al. Cardioprotective effects of lipoic acid, quercetin and resveratrol on oxidative stress related to thyroid hormone alterations in long-term obesity. J Nutr Biochem. (2016) 33:36–44. doi: 10.1016/j.jnutbio.2016.02.008
69. Yu S, Kim SR, Jiang K, Ogrodnik M, Zhu XY, Ferguson CM, et al. Quercetin reverses cardiac systolic dysfunction in mice fed with a high-fat diet: role of angiogenesis. Oxid Med Cell Longev. (2021) 2021:8875729. doi: 10.1155/2021/8875729
70. Ulasova E, Perez J, Hill BG, Bradley WE, Garber DW, Landar A, et al. Quercetin prevents left ventricular hypertrophy in the Apo E knockout mouse. Redox Biol. (2013) 1:381–6. doi: 10.1016/j.redox.2013.07.001
71. Shen Y, Ward NC, Hodgson JM, Puddey IB, Wang Y, Zhang D, et al. Dietary quercetin attenuates oxidant-induced endothelial dysfunction and atherosclerosis in apolipoprotein E knockout mice fed a high-fat diet: a critical role for heme oxygenase-1. Free Radi Biol Med. (2013) 65:908–15. doi: 10.1016/j.freeradbiomed.2013.08.185
72. Garrido AM, Kaistha A, Uryga AK, Oc S, Foote K, Shah A, et al. Efficacy and limitations of senolysis in atherosclerosis. Cardiovasc Res. (2022) 118:1713–27. doi: 10.1093/cvr/cvab208
73. Gil T-H, Zheng H, Lee HG, Shin J-W, Hwang SW, Jang K-M, et al. Senolytic drugs relieve pain by reducing peripheral nociceptive signaling without modifying joint tissue damage in spontaneous osteoarthritis. Aging. (2022) 14:6006–27. doi: 10.18632/aging.204204
74. Riessland M, Orr ME. Translating the biology of aging into new therapeutics for Alzheimer's disease: senolytics. J Prev Alzheimers Dis. (2023) 10:633–46. doi: 10.14283/jpad.2023.104
75. Wang Y, Che L, Chen X, He Z, Song D, Yuan Y, et al. Repurpose dasatinib and quercetin: targeting senescent cells ameliorates postmenopausal osteoporosis and rejuvenates bone regeneration. Bioact Mater. (2023) 25:13–28. doi: 10.1016/j.bioactmat.2023.01.009
76. Islam MT, Tuday E, Allen S, Kim J, Trott DW, Holland WL, et al. Senolytic drugs, dasatinib and quercetin, attenuate adipose tissue inflammation, and ameliorate metabolic function in old age. Aging Cell. (2023) 22:e13767. doi: 10.1111/acel.13767
77. Hickson LJ, Langhi Prata LGP, Bobart SA, Evans TK, Giorgadze N, Hashmi SK, et al. Corrigendum to ‘Senolytics decrease senescent cells in humans: preliminary report from a clinical trial of Dasatinib plus Quercetin in individuals with diabetic kidney disease' EBioMedicine 47 (2019) 446–456. eBioMedicine. (2020) 52:4. doi: 10.1016/j.ebiom.2019.12.004
78. Gonzales MM, Garbarino VR, Marques Zilli E, Petersen RC, Kirkland JL, Tchkonia T, et al. Senolytic therapy to modulate the progression of Alzheimer's disease (SToMP-AD): a pilot clinical trial. J Prev Alzheimers Dis. (2022) 9:22–9. doi: 10.14283/jpad.2021.62
79. Xu M, Pirtskhalava T, Farr JN, Weigand BM, Palmer AK, Weivoda MM, et al. Senolytics improve physical function and increase lifespan in old age. Nat Med. (2018) 24:1246–56. doi: 10.1038/s41591-018-0092-9
80. Alharbi KS, Afzal O, Altamimi ASA, Almalki WH, Kazmi I, Al-Abbasi FA, et al. study of the molecular mechanism of quercetin and dasatinib combination as senolytic in alleviating age-related and kidney diseases. J Food Biochem. (2022) 46:e14471. doi: 10.1111/jfbc.14471
81. Nath KA, O'Brien DR, Croatt AJ, Grande JP, Ackerman AW, Nath MC, et al. The murine dialysis fistula model exhibits a senescence phenotype: pathobiological mechanisms and therapeutic potential. Am J Physiol Renal Physiol. (2018) 315:F1493–9. doi: 10.1152/ajprenal.00308.2018
82. Luo FR, Yang Z, Camuso A, Smykla R, McGlinchey K, Fager K, et al. Dasatinib (BMS-354825) pharmacokinetics and pharmacodynamic biomarkers in animal models predict optimal clinical exposure. Clin Cancer Res. (2006) 12:7180–6. doi: 10.1158/1078-0432.CCR-06-1112
83. Piantelli M, Rossi C, Iezzi M, La Sorda R, Iacobelli S, Alberti S, et al. Flavonoids inhibit melanoma lung metastasis by impairing tumor cells endothelium interactions. J Cell Physiol. (2006) 207:23–9. doi: 10.1002/jcp.20510
84. Roos CM, Hagler M, Zhang B, Oehler EA, Arghami A, Miller JD. Transcriptional and phenotypic changes in aorta and aortic valve with aging and MnSOD deficiency in mice. Am J Physiol Heart Circ Physiol. (2013) 305:H1428–39. doi: 10.1152/ajpheart.00735.2012
85. Lewis-McDougall FC, Ruchaya PJ, Domenjo-Vila E, Shin Teoh T, Prata L, Cottle BJ, et al. Aged-senescent cells contribute to impaired heart regeneration. Aging Cell. (2019) 18:e12931. doi: 10.1111/acel.12931
86. Roos CM, Zhang B, Palmer AK, Ogrodnik MB, Pirtskhalava T, Thalji NM, et al. Chronic senolytic treatment alleviates established vasomotor dysfunction in aged or atherosclerotic mice. Aging Cell. (2016) 15:973–7. doi: 10.1111/acel.12458
87. Parvizi M, Franchi F, Arendt BK, Ebtehaj S, Rodriguez-Porcel M, Lanza IR. Senolytic agents lessen the severity of abdominal aortic aneurysm in aged mice. Exp Gerontol. (2021) 151:111416. doi: 10.1016/j.exger.2021.111416
88. Palmer AK, Xu M, Zhu Y, Pirtskhalava T, Weivoda MM, Hachfeld CM, et al. Targeting senescent cells alleviates obesity-induced metabolic dysfunction. Aging Cell. (2019) 18:e12950. doi: 10.1111/acel.12950
89. Wang L, Wang B, Gasek NS, Zhou Y, Cohn RL, Martin DE, et al. Targeting p21Cip1 highly expressing cells in adipose tissue alleviates insulin resistance in obesity. Cell Metab. (2022) 34:186. doi: 10.1016/j.cmet.2021.12.014
90. Musi N, Valentine JM, Sickora KR, Baeuerle E, Thompson CS, Shen Q, et al. Tau protein aggregation is associated with cellular senescence in the brain. Aging Cell. (2018) 17:e12840. doi: 10.1111/acel.12840
91. Zhang P, Kishimoto Y, Grammatikakis I, Gottimukkala K, Cutler RG, Zhang S, et al. Senolytic therapy alleviates Aβ-associated oligodendrocyte progenitor cell senescence and cognitive deficits in an Alzheimer's disease model. Nat Neurosci. (2019) 22:719–28. doi: 10.1038/s41593-019-0372-9
92. Krzystyniak A, Wesierska M, Petrazzo G, Gadecka A, Dudkowska M, Bielak-Zmijewska A, et al. Combination of dasatinib and quercetin improves cognitive abilities in aged male Wistar rats, alleviates inflammation and changes hippocampal synaptic plasticity and histone H3 methylation profile. Aging. (2022) 14:572–95. doi: 10.18632/aging.203835
93. Shao Z, Wang B, Shi Y, Xie C, Huang C, Chen B, et al. Senolytic agent Quercetin ameliorates intervertebral disc degeneration via the Nrf2/NF-κB axis. Osteoarthritis Cartilage. (2021) 29:413–22. doi: 10.1016/j.joca.2020.11.006
94. Novais EJ, Tran VA, Johnston SN, Darris KR, Roupas AJ, Sessions GA, et al. Long-term treatment with senolytic drugs Dasatinib and Quercetin ameliorates age-dependent intervertebral disc degeneration in mice. Nat Commun. (2021) 12:5213. doi: 10.1038/s41467-021-25453-2
95. Wang J, Lu Y, Carr C, Dhandapani KM, Brann DW. Senolytic therapy is neuroprotective and improves functional outcome long-term after traumatic brain injury in mice. Front Neurosci. (2023) 17:1227705. doi: 10.3389/fnins.2023.1227705
96. Ogrodnik M, Zhu Y, Langhi LGP, Tchkonia T, Krüger P, Fielder E, et al. Obesity-induced cellular senescence drives anxiety and impairs neurogenesis. Cell Metab. (2019) 29:1233. doi: 10.1016/j.cmet.2018.12.008
97. Schafer MJ, White TA, Iijima K, Haak AJ, Ligresti G, Atkinson EJ, et al. Cellular senescence mediates fibrotic pulmonary disease. Nat Commun. (2017) 8:14532. doi: 10.1038/ncomms14532
98. Parikh P, Britt RD, Wicher SA, Roesler AM, Roos B, Manlove L, et al. Dasatinib and Quercetin reverse hyperoxia-induced airway hyperreactivity in a murine model of premature airways disease. In: B29. Mechanisms for Airway Hyperresponsiveness: From Cell to Organism. Washington, DC: American Thoracic Society International Conference Abstracts. American Thoracic Society (2019). p. A2858–A2858.
99. Parikh P, Britt RD Jr, Manlove LJ, Wicher SA, Roesler A, Ravix J, et al. Hyperoxia-induced cellular senescence in fetal airway smooth muscle cells. Am J Respir Cell Mol Biol. (2019) 61:51–60. doi: 10.1165/rcmb.2018-0176OC
100. Li C, Shen Y, Huang L, Liu C, Wang J. Senolytic therapy ameliorates renal fibrosis postacute kidney injury by alleviating renal senescence. FASEB J. (2021) 35:e21229. doi: 10.1096/fj.202001855RR
101. Kim SR, Jiang K, Ogrodnik M, Chen X, Zhu X-Y, Lohmeier H, et al. Increased renal cellular senescence in murine high-fat diet: effect of the senolytic drug quercetin. Transl Res. (2019) 213:112–23. doi: 10.1016/j.trsl.2019.07.005
102. Farr JN, Xu M, Weivoda MM, Monroe DG, Fraser DG, Onken JL, et al. Corrigendum: Targeting cellular senescence prevents age-related bone loss in mice. Nat Med. (2017) 23:1384. doi: 10.1038/nm1117-1384c
103. Hambright WS, Mu X, Gao X, Guo P, Kawakami Y, Mitchell J, et al. The senolytic drug fisetin attenuates bone degeneration in the Zmpste24 -/- progeria mouse model. J Osteoporos. (2023) 2023:5572754. doi: 10.1155/2023/5572754
104. Zhou Y, Xin X, Wang L, Wang B, Chen L, Liu O, et al. Senolytics improve bone forming potential of bone marrow mesenchymal stem cells from aged mice. NPJ Regen Med. (2021) 6:34. doi: 10.1038/s41536-021-00145-z
105. Dungan CM, Figueiredo VC, Wen Y, VonLehmden GL, Zdunek CJ, Thomas NT, et al. Senolytic treatment rescues blunted muscle hypertrophy in old mice. Geroscience. (2022) 44:1925–40. doi: 10.1007/s11357-022-00542-2
106. Raman D, Chêne C, Nicco C, Jeljeli M, Eu JQ, Clément M-V, et al. Therapeutic potential of a senolytic approach in a murine model of chronic GVHD. Biology. (2023) 12:647. doi: 10.3390/biology12050647
107. Gao Y, Wu T, Tang X, Wen J, Zhang Y, Zhang J, et al. Increased cellular senescence in doxorubicin-induced murine ovarian injury: effect of senolytics. Geroscience. (2023) 45:1775–90. doi: 10.1007/s11357-023-00728-2
108. Saccon TD, Nagpal R, Yadav H, Cavalcante MB, Nunes AD de C, Schneider A, et al. Senolytic combination of dasatinib and quercetin alleviates intestinal senescence and inflammation and modulates the gut microbiome in aged mice. J Gerontol A Biol Sci Med Sci. (2021) 76:1895–905. doi: 10.1093/gerona/glab002
109. Pal HC, Sharma S, Elmets CA, Athar M, Afaq F. Fisetin inhibits growth, induces G2/M arrest and apoptosis of human epidermoid carcinoma A431 cells: role of mitochondrial membrane potential disruption and consequent caspases activation. Exp Dermatol. (2013) 22:470–5. doi: 10.1111/exd.12181
110. Triantafyllou A, Mylonis I, Simos G, Bonanou S, Tsakalof A. Flavonoids induce HIF-1α but impair its nuclear accumulation and activity. Free Radi Biol Med. (2008) 44:657–70. doi: 10.1016/j.freeradbiomed.2007.10.050
111. Huard CA, Gao X, Dey Hazra ME, Dey Hazra R-O, Lebsock K, Easley JT, et al. Effects of fisetin treatment on cellular senescence of various tissues and organs of old sheep. Antioxidants. (2023) 12:1646. doi: 10.3390/antiox12081646
112. Saito Y, Miyajima M, Yamamoto S, Sato T, Miura N, Fujimiya M, et al. Accumulation of senescent neural cells in murine lupus with depression-like behavior. Front Immunol. (2021) 12:692321. doi: 10.3389/fimmu.2021.692321
113. Takaya K, Asou T, Kishi K. Fisetin, a potential skin rejuvenation drug that eliminates senescent cells in the dermis. Biogerontology. (2023) 25:1–15. doi: 10.1007/s10522-023-10064-9
114. Liu L, Yue X, Sun Z, Hambright WS, Feng Q, Cui Y, et al. Senolytic elimination of senescent macrophages restores muscle stem cell function in severely dystrophic muscle. Aging. (2022) 14:7650–61. doi: 10.18632/aging.204275
115. Saito Y, Chikenji TS, Matsumura T, Nakano M, Fujimiya M. Exercise enhances skeletal muscle regeneration by promoting senescence in fibro-adipogenic progenitors. Nat Commun. (2020) 11:889. doi: 10.1038/s41467-020-14734-x
116. Zhu Y, Tchkonia T, Fuhrmann-Stroissnigg H, Dai HM, Ling YY, Stout MB, et al. Identification of a novel senolytic agent, navitoclax, targeting the Bcl-2 family of anti-apoptotic factors. Aging Cell. (2016) 15:428–35. doi: 10.1111/acel.12445
117. Leverson JD, Phillips DC, Mitten MJ, Boghaert ER, Diaz D, Tahir SK, et al. Exploiting selective BCL-2 family inhibitors to dissect cell survival dependencies and define improved strategies for cancer therapy. Sci Transl Med. (2015) 7:279ra40. doi: 10.1126/scitranslmed.aaa4642
118. Kaefer A, Yang J, Noertersheuser P, Mensing S, Humerickhouse R, Awni W, et al. Mechanism-based pharmacokinetic/pharmacodynamic meta-analysis of navitoclax (ABT-263) induced thrombocytopenia. Cancer Chemother Pharmacol. (2014) 74:593–602. doi: 10.1007/s00280-014-2530-9
119. González-Gualda E, Pàez-Ribes M, Lozano-Torres B, Macias D, Wilson JR 3rd, González-López C, et al. Galacto-conjugation of Navitoclax as an efficient strategy to increase senolytic specificity and reduce platelet toxicity. Aging Cell. (2020) 19:e13142. doi: 10.1111/acel.13142
120. van der Feen DE, Bossers GPL, Hagdorn QAJ, Moonen J-R, Kurakula K, Szulcek R, et al. Cellular senescence impairs the reversibility of pulmonary arterial hypertension. Sci Transl Med. (2020) 12:aaw4974. doi: 10.1126/scitranslmed.aaw4974
121. Jia K, Dai Y, Liu A, Li X, Wu L, Lu L, et al. Senolytic agent navitoclax inhibits angiotensin ii-induced heart failure in mice. J Cardiovasc Pharmacol. (2020) 76:452–60. doi: 10.1097/FJC.0000000000000878
122. Lérida-Viso A, Estepa- Fernández A, Morellá-Aucejo Á, Lozano-Torres B, Alfonso M, Blandez JF, et al. Pharmacological senolysis reduces doxorubicin-induced cardiotoxicity and improves cardiac function in mice. Pharmacol Res. (2022) 183:106356. doi: 10.1016/j.phrs.2022.106356
123. Anderson R, Lagnado A, Maggiorani D, Walaszczyk A, Dookun E, Chapman J, et al. Length-independent telomere damage drives post-mitotic cardiomyocyte senescence. EMBO J. (2019) 38:e100492. doi: 10.15252/embj.2018100492
124. Dookun E, Walaszczyk A, Redgrave R, Palmowski P, Tual-Chalot S, Suwana A, et al. Clearance of senescent cells during cardiac ischemia-reperfusion injury improves recovery. Aging Cell. (2020) 19:e13249. doi: 10.1111/acel.13249
125. Aguayo-Mazzucato C, Andle J, Lee TB Jr, Midha A, Talemal L, Chipashvili V, et al. Acceleration of β cell aging determines diabetes and senolysis improves disease outcomes. Cell Metab. (2019) 30:129–42.e4. doi: 10.1016/j.cmet.2019.05.006
126. Fatt MP, Tran LM, Vetere G, Storer MA, Simonetta JV, Miller FD, et al. Restoration of hippocampal neural precursor function by ablation of senescent cells in the aging stem cell niche. Stem Cell Reports. (2022) 17:259–75. doi: 10.1016/j.stemcr.2021.12.010
127. Bueno M, Papazoglou A, Valenzi E, Rojas M, Lafyatis R, Mora AL. Mitochondria, aging, and cellular senescence: implications for scleroderma. Curr Rheumatol Rep. (2020) 22:37. doi: 10.1007/s11926-020-00920-9
128. Shi B, Tsou P-S, Ma F, Mariani MP, Mattichak MN, LeBrasseur NK, et al. Senescent cells accumulate in systemic sclerosis skin. J Invest Dermatol. (2023) 143:661–4.e5. doi: 10.1016/j.jid.2022.09.652
129. Kizilay Mancini O, Acevedo M, Fazez N, Cuillerier A, Fernandez Ruiz A, Huynh DN, et al. Oxidative stress-induced senescence mediates inflammatory and fibrotic phenotypes in fibroblasts from systemic sclerosis patients. Rheumatology. (2022) 61:1265–75. doi: 10.1093/rheumatology/keab477
130. Tsou P-S, Shi B, Varga J. Role of cellular senescence in the pathogenesis of systemic sclerosis. Curr Opin Rheumatol. (2022) 34:343–50. doi: 10.1097/BOR.0000000000000898
131. Šahmatova L, Sügis E, Šunina M, Hermann H, Prans E, Pihlap M, et al. Signs of innate immune activation and premature immunosenescence in psoriasis patients. Sci Rep. (2017) 7:7553. doi: 10.1038/s41598-017-07975-2
132. Albanesi C, Mercurio L, Bailey J, Glick A, Dellambra E, Scarponi C, Pallotta S, Madonna S. RAS-activated PI3K/AKT signaling sustains cellular senescence in experimental models of psoriasis via P53/P21 axis. Res. Square. (2023). doi: 10.21203/rs.3.rs-3209194/v1
133. Kim H, Jang J, Song MJ, Kim G, Park C-H, Lee DH, et al. Attenuation of intrinsic ageing of the skin via elimination of senescent dermal fibroblasts with senolytic drugs. J Eur Acad Dermatol Venereol. (2022) 36:1125–35. doi: 10.1111/jdv.18051
134. Pan J, Li D, Xu Y, Zhang J, Wang Y, Chen M, et al. Inhibition of Bcl-2/xl with ABT-263 selectively kills senescent type II pneumocytes and reverses persistent pulmonary fibrosis induced by ionizing radiation in mice. Int J Radiat Oncol Biol Phys. (2017) 99:353–61. doi: 10.1016/j.ijrobp.2017.02.216
135. Mylonas KJ, O'Sullivan ED, Humphries D, Baird DP, Docherty M-H, Neely SA, et al. Cellular senescence inhibits renal regeneration after injury in mice, with senolytic treatment promoting repair. Sci Transl Med. (2021) 13:abb0203. doi: 10.1126/scitranslmed.abb0203
136. Sharma AK, Roberts RL, Benson RD Jr, Pierce JL Yu K, Hamrick MW, McGee-Lawrence ME. The senolytic drug navitoclax (ABT-263) causes trabecular bone loss and impaired osteoprogenitor function in aged mice. Front Cell Dev Biol. (2020) 8:354. doi: 10.3389/fcell.2020.00354
137. López-Lázaro M. Distribution and biological activities of the flavonoid luteolin. Mini Rev Med Chem. (2009) 9:31–59. doi: 10.2174/138955709787001712
138. Bielak-Zmijewska A, Grabowska W, Ciolko A, Bojko A, Mosieniak G, Bijoch Ł, et al. The role of curcumin in the modulation of ageing. Int J Mol Sci. (2019) 20:1239. doi: 10.3390/ijms20051239
139. Wu G, Zhang C, Xu L, Chen H, Fan X, Sun B, et al. plays a key role in A-1331852-induced apoptosis in senescent chondrocytes. Biochem Biophys Res Commun. (2022) 609:93–9. doi: 10.1016/j.bbrc.2022.03.155
140. Moncsek A, Al-Suraih MS, Trussoni CE, O'Hara SP, Splinter PL, Zuber C, et al. Targeting senescent cholangiocytes and activated fibroblasts with B-cell lymphoma-extra large inhibitors ameliorates fibrosis in multidrug resistance 2 gene knockout (Mdr2-/-) mice. Hepatology. (2018) 67:247–59. doi: 10.1002/hep.29464
141. Fuhrmann-Stroissnigg H, Niedernhofer LJ, Robbins PD. Hsp90 inhibitors as senolytic drugs to extend healthy aging. Cell Cycle. (2018) 17:1048–55. doi: 10.1080/15384101.2018.1475828
142. Ozsvari B, Nuttall JR, Sotgia F, Lisanti MP. Azithromycin and Roxithromycin define a new family of “senolytic” drugs that target senescent human fibroblasts. Aging. (2018) 10:3294–307. doi: 10.18632/aging.101633
143. Piska K, Gunia-Krzyżak A, Koczurkiewicz P, Wójcik-Pszczoła K, Pekala E. Piperlongumine (piplartine) as a lead compound for anticancer agents - Synthesis and properties of analogues: a mini-review. Eur J Med Chem. (2018) 156:13–20. doi: 10.1016/j.ejmech.2018.06.057
144. Chung H, Kim C. Nutlin-3a for age-related macular degeneration. Aging. (2022) 14:5614–6. doi: 10.18632/aging.204187
145. Hasegawa H, Yamada Y, Iha H, Tsukasaki K, Nagai K, Atogami S, et al. Activation of p53 by Nutlin-3a, an antagonist of MDM2, induces apoptosis and cellular senescence in adult T-cell leukemia cells. Leukemia. (2009) 23:2090–101. doi: 10.1038/leu.2009.171
146. Kumamoto K, Spillare EA, Fujita K, Horikawa I, Yamashita T, Appella E, et al. Nutlin-3a activates p53 to both down-regulate inhibitor of growth 2 and up-regulate mir-34a, mir-34b, and mir-34c expression, and induce senescence. Cancer Res. (2008) 68:3193–203. doi: 10.1158/0008-5472.CAN-07-2780
147. Crane EK, Kwan S-Y, Izaguirre DI, Tsang YTM, Mullany LK, Zu Z, et al. Nutlin-3a: a potential therapeutic opportunity for TP53 wild-type ovarian carcinomas. PLoS ONE. (2015) 10:e0135101. doi: 10.1371/journal.pone.0135101
148. Yee-Lin V, Pooi-Fong W, Soo-Beng AK. Nutlin-3, A p53-Mdm2 antagonist for nasopharyngeal carcinoma treatment. Mini Rev Med Chem. (2018) 18:173–83. doi: 10.2174/1389557517666170717125821
149. Manfé V, Biskup E, Johansen P, Kamstrup MR, Krejsgaard TF, Morling N, et al. MDM2 inhibitor nutlin-3a induces apoptosis and senescence in cutaneous T-cell lymphoma: role of p53. J Invest Dermatol. (2012) 132:1487–96. doi: 10.1038/jid.2012.10
150. Efeyan A, Ortega-Molina A, Velasco-Miguel S, Herranz D, Vassilev LT, Serrano M. Induction of p53-dependent senescence by the MDM2 antagonist nutlin-3a in mouse cells of fibroblast origin. Cancer Res. (2007) 67:7350–7. doi: 10.1158/0008-5472.CAN-07-0200
151. Luo H, Yount C, Lang H, Yang A, Riemer EC, Lyons K, et al. Activation of p53 with Nutlin-3a radiosensitizes lung cancer cells via enhancing radiation-induced premature senescence. Lung Cancer. (2013) 81:167–73. doi: 10.1016/j.lungcan.2013.04.017
152. Villalonga-Planells R, Coll-Mulet L, Martínez-Soler F, Castaño E, Acebes J-J, Giménez-Bonafé P, et al. Activation of p53 by nutlin-3a induces apoptosis and cellular senescence in human glioblastoma multiforme. PLoS ONE. (2011) 6:e18588. doi: 10.1371/journal.pone.0018588
153. Polański R, Noon AP, Blaydes J, Phillips A, Rubbi CP. Senescence induction in renal carcinoma cells by Nutlin-3: a potential therapeutic strategy based on MDM2 antagonism. Cancer Lett. (2014) 353:211–9. doi: 10.1016/j.canlet.2014.07.024
154. Chae J-B, Jang H, Son C, Park C-W, Choi H, Jin S, et al. Correction to: Targeting senescent retinal pigment epithelial cells facilitates retinal regeneration in mouse models of age-related macular degeneration. Geroscience. (2022) 44:1885. doi: 10.1007/s11357-022-00523-5
155. Lee H, Wilson D, Bunting KV, Kotecha D, Jackson T. Repurposing digoxin for geroprotection in patients with frailty and multimorbidity. Ageing Res Rev. (2023) 86:101860. doi: 10.1016/j.arr.2023.101860
157. Prassas I, Diamandis EP. Novel therapeutic applications of cardiac glycosides. Nat Rev Drug Discov. (2008) 7:926–35. doi: 10.1038/nrd2682
158. Bode-Böger SM, Martens-Lobenhoffer J, Täger M, Schröder H, Scalera F. Aspirin reduces endothelial cell senescence. Biochem Biophys Res Commun. (2005) 334:1226–32. doi: 10.1016/j.bbrc.2005.07.014
159. Yi T-N, Zhao H-Y, Zhang J-S, Shan H-Y, Meng X, Zhang J. Effect of aspirin on high glucose-induced senescence of endothelial cells. Chin Med J. (2009) 122:3055–61. doi: 10.3760/cma.j.issn.0366-6999.2009.24.027
160. Hu Z, Zhang F, Yang Z, Zhang J, Zhang D, Yang N, et al. Low-dose aspirin promotes endothelial progenitor cell migration and adhesion and prevents senescence. Cell Biol Int. (2008) 32:761–8. doi: 10.1016/j.cellbi.2008.03.004
161. Li Y, Lu J, Hou Y, Huang S, Pei G. Alzheimer's amyloid-β accelerates human neuronal cell senescence which could be rescued by sirtuin-1 and aspirin. Front Cell Neurosci. (2022) 16:906270. doi: 10.3389/fncel.2022.906270
162. Muñoz-Espín D, Rovira M, Galiana I, Giménez C, Lozano-Torres B, Paez-Ribes M, et al. A versatile drug delivery system targeting senescent cells. EMBO Mol Med. (2018) 10:9355. doi: 10.15252/emmm.201809355
163. Mendelsohn AR, Larrick JW. Antiaging vaccines targeting senescent cells. Rejuvenation Res. (2022) 25:39–45. doi: 10.1089/rej.2022.0008
164. Yoshida S, Nakagami H, Hayashi H, Ikeda Y, Sun J, Tenma A, et al. The CD153 vaccine is a senotherapeutic option for preventing the accumulation of senescent T cells in mice. Nat Commun. (2020) 11:2482. doi: 10.1038/s41467-020-16347-w
165. Suda M, Shimizu I, Katsuumi G, Yoshida Y, Hayashi Y, Ikegami R, et al. Senolytic vaccination improves normal and pathological age-related phenotypes and increases lifespan in progeroid mice. Nat Aging. (2021) 1:1117–26. doi: 10.1038/s43587-021-00151-2
166. Weiland T, Lampe J, Essmann F, Venturelli S, Berger A, Bossow S, et al. Enhanced killing of therapy-induced senescent tumor cells by oncolytic measles vaccine viruses. Int J Cancer. (2014) 134:235–43. doi: 10.1002/ijc.28350
167. Chen Z, Hu K, Feng L, Su R, Lai N, Yang Z, et al. Senescent cells re-engineered to express soluble programmed death receptor-1 for inhibiting programmed death receptor-1/programmed death ligand-1 as a vaccination approach against breast cancer. Cancer Sci. (2018) 109:1753–63. doi: 10.1111/cas.13618
168. Nakagami H. Cellular senescence and senescence-associated T cells as a potential therapeutic target. Geriatr Gerontol Int. (2020) 20:97–100. doi: 10.1111/ggi.13851
169. Amor C, Feucht J, Leibold J, Ho Y-J, Zhu C, Alonso-Curbelo D, et al. Senolytic CAR T cells reverse senescence-associated pathologies. Nature. (2020) 583:127–32. doi: 10.1038/s41586-020-2403-9
170. Gonzales MM, Krishnamurthy S, Garbarino V, Daeihagh AS, Gillispie GJ, Deep G, et al. geroscience motivated approach to treat Alzheimer's disease: Senolytics move to clinical trials. Mech Ageing Dev. (2021) 200:111589. doi: 10.1016/j.mad.2021.111589
171. Nambiar A, Kellogg D 3rd, Justice J, Goros M, Gelfond J, Pascual R, et al. Senolytics dasatinib and quercetin in idiopathic pulmonary fibrosis: results of a phase I, single-blind, single-center, randomized, placebo-controlled pilot trial on feasibility and tolerability. EBioMedicine. (2023) 90:104481. doi: 10.1016/j.ebiom.2023.104481
172. Ito K, Kuroki S, Kobayashi M, Ono K, Washizu T, Bonkobara M. Identification of dasatinib as an in vitro potent growth inhibitor of canine histiocytic sarcoma cells. Vet J. (2013) 196:536–40. doi: 10.1016/j.tvjl.2012.12.016
173. Timmermans-Sprang EPM, Mestemaker HM, Steenlage RR, Mol JA. Dasatinib inhibition of cSRC prevents the migration and metastasis of canine mammary cancer cells with enhanced Wnt and HER signalling. Vet Comp Oncol. (2019) 17:413–26. doi: 10.1111/vco.12490
174. Hadzijusufovic E, Peter B, Rebuzzi L, Baumgartner C, Gleixner KV, Gruze A, et al. Growth-inhibitory effects of four tyrosine kinase inhibitors on neoplastic feline mast cells exhibiting a Kit exon 8 ITD mutation. Vet Immunol Immunopathol. (2009) 132:243–50. doi: 10.1016/j.vetimm.2009.05.007
175. Malda J, Benders KEM, Klein TJ, de Grauw JC, Kik MJL, Hutmacher DW, et al. Comparative study of depth-dependent characteristics of equine and human osteochondral tissue from the medial and lateral femoral condyles. Osteoarthritis Cartilage. (2012) 20:1147–51. doi: 10.1016/j.joca.2012.06.005
176. McIlwraith CW, Frisbie DD, Kawcak CE. The horse as a model of naturally occurring osteoarthritis. Bone Joint Res. (2012) 1:297–309. doi: 10.1302/2046-3758.111.2000132
177. Heikkilä-Laurila HP, Rajamäki MM. Idiopathic pulmonary fibrosis in West Highland white terriers. Vet Clin North Am Small Anim Pract. (2014) 44:129–42. doi: 10.1016/j.cvsm.2013.08.003
178. Laurila HP, Rajamäki MM. Update on canine idiopathic pulmonary fibrosis in west highland white terriers. Vet Clin North Am Small Anim Pract. (2020) 50:431–46. doi: 10.1016/j.cvsm.2019.11.004
179. Wess G. Screening for dilated cardiomyopathy in dogs. J Vet Cardiol. (2022) 40:51–68. doi: 10.1016/j.jvc.2021.09.004
180. Meurs KM, Friedenberg SG, Kolb J, Saripalli C, Tonino P, Woodruff K, et al. A missense variant in the titin gene in Doberman pinscher dogs with familial dilated cardiomyopathy and sudden cardiac death. Hum Genet. (2019) 138:515–24. doi: 10.1007/s00439-019-01973-2
181. Friederich J, Seuß AC, Wess G. The role of atrial fibrillation as a prognostic factor in doberman pinschers with dilated cardiomyopathy and congestive heart failure. Vet J. (2020) 264:105535. doi: 10.1016/j.tvjl.2020.105535
182. Geddes R, Aguiar J. Feline Comorbidities: Balancing hyperthyroidism and concurrent chronic kidney disease. J Feline Med Surg. (2022) 24:641–50. doi: 10.1177/1098612X221090390
183. Ray M, Carney HC, Boynton B, Quimby J, Robertson S, St Denis K, et al. 2021 AAFP feline senior care guidelines. J Feline Med Surg. (2021) 23:613–38. doi: 10.1177/1098612X211021538
184. Lutchman A, Shanker N, Comerford E, German AJ, Leung YB, Maddox T, Dowgray N. Ultrasonographic monitoring of feline epaxial muscle height as part of an annual wellness examination to assess for the development of sarcopenia. J Feline Med Surg. (2023) 25:1098612X221140081. doi: 10.1177/1098612X221140081
185. Dowgray N, Comerford E. Feline musculoskeletal ageing: How are we diagnosing and treating musculoskeletal impairment? J Feline Med Surg. (2020) 22:1069–83. doi: 10.1177/1098612X20965832
186. Ross MW, Dyson SJ. Diagnosis and Management of Lameness in the Horse. London: Elsevier Health Sciences (2010). 1424 p.
187. USDA. Lameness and Laminitis in U.S. Horses. Fort Collins, CO: USDA; APHIS; VS; CEAH; National Animal Health Monitoring System #N318.0400 (2000).
188. Neundorf RH, Lowerison MB, Cruz AM, Thomason JJ, McEwen BJ, Hurtig MB. Determination of the prevalence and severity of metacarpophalangeal joint osteoarthritis in Thoroughbred racehorses via quantitative macroscopic evaluation. Am J Vet Res. (2010) 71:1284–93. doi: 10.2460/ajvr.71.11.1284
189. Ireland JL, Clegg PD, McGowan CM, Platt L, Pinchbeck GL. Factors associated with mortality of geriatric horses in the United Kingdom. Prev Vet Med. (2011) 101:204–18. doi: 10.1016/j.prevetmed.2011.06.002
190. Ireland JL, Clegg PD, McGowan CM, McKane SA, Chandler KJ, Pinchbeck GL. Disease prevalence in geriatric horses in the United Kingdom: veterinary clinical assessment of 200 cases. Equine Vet J. (2012) 44:101–6. doi: 10.1111/j.2042-3306.2010.00361.x
191. van Weeren PR, Back W. Musculoskeletal disease in aged horses and its management. Vet Clin North Am Equine Pract. (2016) 32:229–47. doi: 10.1016/j.cveq.2016.04.003
192. Xu M, Bradley EW, Weivoda MM, Hwang SM, Pirtskhalava T, Decklever T, et al. Transplanted senescent cells induce an osteoarthritis-like condition in mice. J Gerontol A Biol Sci Med Sci. (2017) 72:780–5. doi: 10.1093/gerona/glw154
193. Teti G, Mazzotti E, Gatta V, Chiarini F, Alfieri ML, Falconi M. Implication of cellular senescence in osteoarthritis: a study on equine synovial fluid mesenchymal stromal cells. Int J Mol Sci. (2023) 24:3109. doi: 10.3390/ijms24043109
194. Min HK, Kim SH, Won J-Y, Kim K-W, Lee J-Y, Lee S-H, et al. Dasatinib, a selective tyrosine kinase inhibitor, prevents joint destruction in rheumatoid arthritis animal model. Int J Rheum Dis. (2023) 26:718–26. doi: 10.1111/1756-185X.14627
195. Gilmer G, Iijima H, Jackson N, Hettinger Z, Bean AC, Bergmann J, et al. A network medicine approach to elucidate mechanisms underlying menopause-induced knee osteoarthritis. bioRxiv. (2023). doi: 10.1101/2023.03.02.530756
196. Wu C-J, Liu R-X, Huan S-W, Tang W, Zeng Y-K, Zhang J-C, et al. Senescent skeletal cells cross-talk with synovial cells plays a key role in the pathogenesis of osteoarthritis. Arthritis Res Ther. (2022) 24:59. doi: 10.1186/s13075-022-02747-4
197. Minamino T, Orimo M, Shimizu I, Kunieda T, Yokoyama M, Ito T, et al. A crucial role for adipose tissue p53 in the regulation of insulin resistance. Nat Med. (2009) 15:1082–7. doi: 10.1038/nm.2014
198. Palmer AK, Gustafson B, Kirkland JL, Smith U. Cellular senescence: at the nexus between ageing and diabetes. Diabetologia. (2019) 62:1835–41. doi: 10.1007/s00125-019-4934-x
199. Tchkonia T, Morbeck DE, Von Zglinicki T, Van Deursen J, Lustgarten J, Scrable H, et al. Fat tissue, aging, and cellular senescence. Aging Cell. (2010) 9:667–84. doi: 10.1111/j.1474-9726.2010.00608.x
200. Palmer AK, Tchkonia T, LeBrasseur NK, Chini EN, Xu M, Kirkland JL. Cellular senescence in type 2 diabetes: a therapeutic opportunity. Diabetes. (2015) 64:2289–98. doi: 10.2337/db14-1820
201. Wyse CA, McNie KA, Tannahill VJ, Murray JK, Love S. Prevalence of obesity in riding horses in Scotland. Vet Rec. (2008) 162:590–1. doi: 10.1136/vr.162.18.590
202. Stephenson HM, Green MJ, Freeman SL. Prevalence of obesity in a population of horses in the UK. Vet Rec. (2011) 168:131. doi: 10.1136/vr.c6281
203. Johnson PJ, Wiedmeyer CE, Messer NT, Ganjam VK. Medical implications of obesity in horses—lessons for human obesity. J Diabetes Sci Technol. (2009) 3:163–74. doi: 10.1177/193229680900300119
204. Frank N, Geor RJ, Bailey SR, Durham AE, Johnson PJ. American College of Veterinary Internal Medicine. Equine metabolic syndrome. J Vet Intern Med. (2010) 24:467–75. doi: 10.1111/j.1939-1676.2010.0503.x
205. Taylor R. Insulin resistance and type 2 diabetes. Diabetes. (2012) 61:778–9. doi: 10.2337/db12-0073
206. Baboota RK, Spinelli R, Erlandsson MC, Brandao BB, Lino M, Yang H, et al. Chronic hyperinsulinemia promotes human hepatocyte senescence. Mol Metab. (2022) 64:101558. doi: 10.1016/j.molmet.2022.101558
207. Marycz K, Kornicka K, Basinska K, Czyrek A. Equine metabolic syndrome affects viability, senescence, and stress factors of equine adipose-derived mesenchymal stromal stem cells: new insight into EqASCs isolated from EMS horses in the context of their aging. Oxid Med Cell Longev. (2015) 2016:4710326. doi: 10.1155/2016/4710326
208. Shmulevich R, Krizhanovsky V. Cell senescence, DNA damage, and metabolism. Antioxid Redox Signal. (2021) 34:324–34. doi: 10.1089/ars.2020.8043
209. Clayton ZS, Rossman MJ, Mahoney SA, Venkatasubramanian R, Maurer GS, Hutton DA, et al. Cellular senescence contributes to large elastic artery stiffening and endothelial dysfunction with aging: amelioration with senolytic treatment. Hypertension. (2023) 80:2072–87. doi: 10.1161/HYPERTENSIONAHA.123.21392
210. Cianflone E, Torella M, Biamonte F, De Angelis A, Urbanek K, Costanzo FS, et al. Targeting cardiac stem cell senescence to treat cardiac aging and disease. Cells. (2020) 9:1558. doi: 10.3390/cells9061558
211. Childs BG, Baker DJ, Wijshake T, Conover CA, Campisi J, van Deursen JM. Senescent intimal foam cells are deleterious at all stages of atherosclerosis. Science. (2016) 354:472–7. doi: 10.1126/science.aaf6659
212. Tkacz M, Tang Q, Corcoran B. Investigating the Potential Therapeutic Effects of Senolytic Drugs in Canine Myxomatous Mitral Valve Disease (MMVD). (2023). Available online at: https://www.bsavalibrary.com/content/chapter/10.22233/9781913859152.Ch220
213. Tang Q, Markby GR, MacNair AJ, Tang K, Tkacz M, Parys M, et al. TGF-β-induced PI3K/AKT/mTOR pathway controls myofibroblast differentiation and secretory phenotype of valvular interstitial cells through the modulation of cellular senescence in a naturally occurring in vitro canine model of myxomatous mitral valve disease. Cell Prolif. (2023) 56:e13435. doi: 10.1111/cpr.13435
214. Kim SR, Puranik AS, Jiang K, Chen X, Zhu X-Y, Taylor I, et al. Progressive cellular senescence mediates renal dysfunction in ischemic nephropathy. J Am Soc Nephrol. (2021) 32:1987–2004. doi: 10.1681/ASN.2020091373
215. Quimby J, Erickson A, Mcleland S, Cianciolo R, Maranon D, Lunn K, et al. Renal senescence, telomere shortening and nitrosative stress in feline chronic kidney disease. Vet Sci China. (2021) 8:314. doi: 10.3390/vetsci8120314
216. Levstek T, Trebušak Podkrajšek K. Telomere attrition in chronic kidney diseases. Antioxidants. (2023) 12:579. doi: 10.3390/antiox12030579
217. Dai L, Qureshi AR, Witasp A, Lindholm B, Stenvinkel P. Early vascular ageing and cellular senescence in chronic kidney disease. Comput Struct Biotechnol J. (2019) 17:721–9. doi: 10.1016/j.csbj.2019.06.015
218. Koike M, Yutoku Y, Koike A. Inhibition of Crandell-Rees Feline Kidney cell proliferation by X-ray-induced senescence. J Vet Med Sci. (2021) 83:798–804. doi: 10.1292/jvms.20-0679
219. Hsu B, Visich J, Lane NE Li L, Mittal J, An M, Laberge R-M, et al. Safety, tolerability, pharmacokinetics, and clinical outcomes following treatment of painful knee osteoarthritis with senolytic molecule UBX0101. Osteoarthritis Cartilage. (2020) 28:S479–80. doi: 10.1016/j.joca.2020.02.752
220. Wissler Gerdes EO, Zhu Y, Tchkonia T, Kirkland JL. Discovery, development, and future application of senolytics: theories and predictions. FEBS J. (2020) 287:2418–27. doi: 10.1111/febs.15264
221. Beerman I, Basisty N, de Cabo R. Short-term senolytic treatment: a paradigm to promote fracture repair during aging. J Clin Invest. (2022) 132:158871. doi: 10.1172/JCI158871
222. Kirkland JL, Tchkonia T. Cellular senescence: a translational perspective. EBioMedicine. (2017) 21:21–8. doi: 10.1016/j.ebiom.2017.04.013
223. Vail DM, MacEwen EG. Spontaneously occurring tumors of companion animals as models for human cancer. Cancer Invest. (2000) 18:781–92. doi: 10.3109/07357900009012210
224. Fox PR, Basso C, Thiene G, Maron BJ. Spontaneous animal models. In: Markus FI, Nava A, Thiene G, , editors. Arrhythmogenic RV Cardiomyopathy/Dysplasia: Recent Advances. Milano: Springer Milan (2007). p. 69–78.
225. Freeman LM, Rush JE, Stern JA, Huggins GS, Maron MS. Feline hypertrophic cardiomyopathy: a spontaneous large animal model of human HCM. Cardiol Res Pract. (2017) 8:139–42. doi: 10.14740/cr578w
226. Löscher W. Dogs as a natural animal model of epilepsy. Front Vet Sci. (2022) 9:928009. doi: 10.3389/fvets.2022.928009
227. McKenzie BA. Comparative veterinary geroscience: mechanism of molecular, cellular, and tissue aging in humans, laboratory animal models, and companion dogs and cats. Am J Vet Res. (2022) 83:27. doi: 10.2460/ajvr.22.02.0027
228. Sándor S, Kubinyi E. Genetic pathways of aging and their relevance in the dog as a natural model of human aging. Front Genet. (2019) 10:948. doi: 10.3389/fgene.2019.00948
229. Grosse N, van Loon B, Rohrer Bley C. DNA damage response and DNA repair – dog as a model? BMC Cancer. (2014) 14:203. doi: 10.1186/1471-2407-14-203
230. Fick LJ, Fick GH Li Z, Cao E, Bao B, Heffelfinger D, Parker HG, et al. Telomere length correlates with life span of dog breeds. Cell Rep. (2012) 2:1530–6. doi: 10.1016/j.celrep.2012.11.021
231. Quimby JM, Maranon DG, Battaglia CLR, McLeland SM, Brock WT, Bailey SM. Feline chronic kidney disease is associated with shortened telomeres and increased cellular senescence. Am J Physiol Renal Physiol. (2013) 305:F295–303. doi: 10.1152/ajprenal.00527.2012
232. Vite CH, Head E. Aging in the canine and feline brain. Vet Clin North Am Small Anim Pract. (2014) 44:1113–29. doi: 10.1016/j.cvsm.2014.07.008
233. Thompson MJ, vonHoldt B, Horvath S, Pellegrini M. An epigenetic aging clock for dogs and wolves. Aging. (2017) 9:1055–68. doi: 10.18632/aging.101211
234. Laflamme DP. Nutrition for aging cats and dogs and the importance of body condition. Vet Clin North Am Small Anim Pract. (2005) 35:713–42. doi: 10.1016/j.cvsm.2004.12.011
235. Mizorogi T, Kobayashi M, Ohara K, Okada Y, Yamamoto I, Arai T, et al. Effects of age on inflammatory profiles and nutrition/energy metabolism in domestic cats. Vet Med (Auckl). (2020) 11:131–7. doi: 10.2147/VMRR.S277208
236. Zayed M, Iohara K. Age related senescence, apoptosis, and inflammation profiles in periodontal ligament cells from canine teeth. Curr Mol Med. (2023) 23:808–14. doi: 10.2174/1566524022666220520124630
237. Voga M, Adamic N, Vengust M, Majdic G. Stem cells in veterinary medicine—current state and treatment options. Front Vet Sci. (2020) 7:278 doi: 10.3389/fvets.2020.00278
238. Nicholatos JW, Robinette TM, Tata SVP, Yordy JD, Francisco AB, Platov M, et al. Cellular energetics and mitochondrial uncoupling in canine aging. Geroscience. (2019) 41:229–42. doi: 10.1007/s11357-019-00062-6
239. Christiansen LB, Dela F, Koch J, Hansen CN, Leifsson PS, Yokota T. Impaired cardiac mitochondrial oxidative phosphorylation and enhanced mitochondrial oxidative stress in feline hypertrophic cardiomyopathy. Am J Physiol Heart Circ Physiol. (2015) 308:H1237–47. doi: 10.1152/ajpheart.00727.2014
240. Alexander JE, Colyer A, Haydock RM, Hayek MG, Park J. Understanding how dogs age: longitudinal analysis of markers of inflammation, immune function, and oxidative stress. J Gerontol A Biol Sci Med Sci. (2018) 73:720–8. doi: 10.1093/gerona/glx182
241. Day MJ. Ageing, immunosenescence and inflammageing in the dog and cat. J Comp Pathol. (2010) 142 Suppl 1:S60–9. doi: 10.1016/j.jcpa.2009.10.011
242. Wu K, Rodrigues L, Post G, Harvey G, White M, Miller A, et al. Analyses of canine cancer mutations and treatment outcomes using real-world clinico-genomics data of 2119 dogs. NPJ Precis Oncol. (2023) 7:8. doi: 10.1038/s41698-023-00346-3
243. Asgharian P, Tazekand AP, Hosseini K, Forouhandeh H, Ghasemnejad T, Ranjbar M, et al. Potential mechanisms of quercetin in cancer prevention: focus on cellular and molecular targets. Cancer Cell Int. (2022) 22:257. doi: 10.1186/s12935-022-02677-w
244. Biswas P, Dey D, Biswas PK, Rahaman TI, Saha S, Parvez A, et al. A comprehensive analysis and anti-cancer activities of quercetin in ROS-mediated cancer and cancer stem cells. Int J Mol Sci. (2022) 23:1746 doi: 10.3390/ijms231911746
245. Pagano TB, Wojcik S, Costagliola A, De Biase D, Iovino S, Iovane V, et al. Age related skeletal muscle atrophy and upregulation of autophagy in dogs. Vet J. (2015) 206:54–60. doi: 10.1016/j.tvjl.2015.07.005
246. Freeman LM. Cachexia and sarcopenia in companion animals: an under-utilized natural animal model of human disease. JCSM Rapid Commun. (2018) 1:1–17. doi: 10.1002/j.2617-1619.2018.tb00006.x
247. Peterson ME, Little SE. Cachexia, Sarcopenia and Other Forms of Muscle Wasting: Common Problems of Senior and Geriatric Cats and of Cats with Endocrine Disease. Available online at: https://www.purinainstitute.com/sites/g/files/2018-05/Peterson%20-%20Cachexia%2C%20Sarcopenia%20and%20Other%20Forms%20of%20Muscle%20Wasting.pdf (accessed November 17, 2023).
248. Williams EA, Kelly PJ. Age-related changes in bone in the dog: calcium homeostasis. J Orthop Res. (1984) 2:8–14. doi: 10.1002/jor.1100020103
249. Cheon H, Choi W, Lee Y, Lee D, Kim J, Kang J-H, et al. Assessment of trabecular bone mineral density using quantitative computed tomography in normal cats. J Vet Med Sci. (2012) 74:1461–7. doi: 10.1292/jvms.11-0579
250. Gonzales MM, Garbarino VR, Kautz TF, Palavicini JP, Lopez-Cruzan M, Dehkordi SK, et al. Senolytic therapy in mild Alzheimer's disease: a phase 1 feasibility trial. Nat Med. (2023) 29:2481–8. doi: 10.1038/s41591-023-02543-w
251. Tapp PD, Siwak CT. The canine model of human brain aging: cognition, behavior, and neuropathology. In: Handbook of Models for Human Aging. Cambridge MA: Academic Press (2006) p. 415–434.
252. Sikora E, Bielak-Zmijewska A, Dudkowska M, Krzystyniak A, Mosieniak G, Wesierska M, et al. Cellular senescence in brain aging. Front Aging Neurosci. (2021) 13:646924. doi: 10.3389/fnagi.2021.646924
253. Landsberg GM, Nichol J, Araujo JA. Cognitive dysfunction syndrome: a disease of canine and feline brain aging. Vet Clin North Am Small Anim Pract. (2012) 42:749–68. doi: 10.1016/j.cvsm.2012.04.003
254. Chu CR, Szczodry M, Bruno S. Animal models for cartilage regeneration and repair. Tissue Eng Part B Rev. (2010) 16:105–15. doi: 10.1089/ten.teb.2009.0452
Keywords: senotherapeutics, senescence-associated secretory phenotype, dasatinib, quercetin, fisetin
Citation: Williams ZJ, Chow L, Dow S and Pezzanite LM (2024) The potential for senotherapy as a novel approach to extend life quality in veterinary medicine. Front. Vet. Sci. 11:1369153. doi: 10.3389/fvets.2024.1369153
Received: 11 January 2024; Accepted: 30 April 2024;
Published: 15 May 2024.
Edited by:
Cristina Esteves, University of Edinburgh, United KingdomReviewed by:
Cristin Coman, “Cantacuzino”, National Institute of Medical-Military Research and Development, RomaniaOgnian Neytchev, University of Glasgow, United Kingdom
Copyright © 2024 Williams, Chow, Dow and Pezzanite. This is an open-access article distributed under the terms of the Creative Commons Attribution License (CC BY). The use, distribution or reproduction in other forums is permitted, provided the original author(s) and the copyright owner(s) are credited and that the original publication in this journal is cited, in accordance with accepted academic practice. No use, distribution or reproduction is permitted which does not comply with these terms.
*Correspondence: Lynn M. Pezzanite, lynn.pezzanite@colostate.edu