Effects of Fluoxetine on Human Embryo Development
- 1Department of Women's and Children's Health, Uppsala University, Uppsala, Sweden
- 2Centre of Reproduction, Uppsala University Hospital, Uppsala, Sweden
- 3IVF-Clinic Falun, Falun, Sweden
- 4Analytical Chemistry, Department of Chemistry – BMC and Science for Life Laboratory, Uppsala University, Uppsala, Sweden
- 5Unit Behavioural Neuroscience, Department of Neurobiology, Groningen Institute for Evolutionary Life Sciences, University of Groningen, Groningen, Netherlands
The use of antidepressant treatment during pregnancy is increasing, and selective serotonin reuptake inhibitors (SSRIs) are the most widely prescribed antidepressants in pregnant women. Serotonin plays a role in embryogenesis, and serotonin transporters are expressed in two-cell mouse embryos. Thus, the aim of the present study was to evaluate whether fluoxetine, one of the most prescribed SSRI antidepressant world-wide, exposure influences the timing of different embryo developmental stages, and furthermore, to analyze what protein, and protein networks, are affected by fluoxetine in the early embryo development. Human embryos (n = 48) were randomly assigned to treatment with 0.25 or 0.5 μM fluoxetine in culture medium. Embryo development was evaluated by time-lapse monitoring. The fluoxetine-induced human embryo proteome was analyzed by shotgun mass spectrometry. Protein secretion from fluoxetine-exposed human embryos was analyzed by use of high-multiplex immunoassay. The lower dose of fluoxetine had no influence on embryo development. A trend toward reduced time between thawing and start of cavitation was noted in embryos treated with 0.5 μM fluoxetine (p = 0.065). Protein analysis by shotgun mass spectrometry detected 45 proteins that were uniquely expressed in fluoxetine-treated embryos. These proteins are involved in cell growth, survival, proliferation, and inflammatory response. Culturing with 0.5 μM, but not 0.25 μM fluoxetine, caused a significant increase in urokinase-type plasminogen activator (uPA) in the culture medium. In conclusion, fluoxetine has marginal effects on the timing of developmental stages in embryos, but induces expression and secretion of several proteins in a manner that depends on dose. For these reasons, and in line with current guidelines, the lowest possible dose of SSRI should be used in pregnant women who need to continue treatment.
Introduction
The prevalence of major depression during pregnancy is approximately 3–5% (Andersson et al., 2003; Gavin et al., 2005). Nowadays, selective serotonin reuptake inhibitors (SSRIs) are the most widely prescribed antidepressants in pregnant women as these drugs, besides proven efficacy, are associated with fairly few side-effects. While generally also considered safe to use during pregnancy (Barbey and Roose, 1998; Gentile, 2005), SSRI treatment has been associated with increased risk of pregnancy complications such as preterm birth and preeclampsia (Qiu et al., 2009; Wisner et al., 2009). With regards to the offspring, reduced fetal head growth and low birth weight have been reported (Kallen, 2004; Wen et al., 2006; El Marroun et al., 2012). SSRIs have also been associated with cardiac malformations (Olivier et al., 2013; El Marroun et al., 2014; however, see also Furu et al., 2015) and increased risk of persistent pulmonary hypertension, although these conditions are rare (Wogelius et al., 2006; Kieler et al., 2012). Behavioral defects caused by antenatal SSRI exposure include increased risk for autism spectrum disorders and externalizing behaviors (Oberlander et al., 2007; Croen et al., 2011). However, other studies suggest that these malformations and behavioral defects may be caused by the underlying depression and not by the treatment itself (reviewed in Olivier et al., 2013, 2015a; Waters et al., 2014).
SSRIs inhibit the reuptake of released serotonin (5-HT) by blocking the serotonin transporter, which results in increased levels of 5-HT in the synaptic cleft. Serotonin is important during brain development, where it acts as a neurotrophic factor (Lauder et al., 1981; Gaspar et al., 2003; Ansorge et al., 2007). Lauder and Krebs (1978) found that serotonin is involved in early neurogenesis in chicken embryo and it has been shown in mice that 5-HT produced by the placenta is accumulated in the fetal forebrain during critical periods of brain development (Bonnin and Levitt, 2012). 5-HT is also important for the regulation of embryogenesis in such diverse species like sea urchins and mice (reviewed in Buznikov et al., 2001), where high and low levels of serotonin can have both positive and negative effects, depending on the stage of development (Khozhai et al., 1995; Il'kova et al., 2004). Of relevance for the research question at hand, serotonin transporters are expressed as early as in two-cell mouse embryos (Amireault and Dube, 2005). In addition, serotonin plays a crucial role during craniofacial development (Moiseiwitsch and Lauder, 1995) and cardiac morphogenesis in mice (Yavarone et al., 1993). SSRIs are found in cord blood and amniotic fluid of SSRI-treated pregnant women (Hendrick et al., 2003; Loughhead et al., 2006), indicating that the fetus is exposed to SSRIs during pregnancy.
In previous studies, we have shown that SSRI treatment affects gene expression and protein levels in human placenta (Kaihola et al., 2015; Olivier et al., 2015b). While these effects were minor (at least in terms of fold change) in comparison to what is typically seen in preeclampsia or other placental disorders (Winn et al., 2009), it may be speculated that the changes we see, for instance in nerve growth factor signaling (Kaihola et al., 2015), may influence placental function, and thus, the intrauterine development of the fetus. To further investigate the impact of SSRIs on fetal development, studies focusing on embryonic development before implantation are needed. The aim of the present study was to evaluate whether fluoxetine, one of the most widely prescribed SSRI antidepressants, exposure influences the timing of different embryo developmental stages, and furthermore, to analyze what protein, and protein networks, are affected by fluoxetine in the early embryo development.
Materials and Methods
All the couples who were asked to participate attended the Center for Reproduction, Uppsala University Hospital, Uppsala, Sweden, and written informed consent was obtained from all couples donating embryos for this study. No reimbursement was given to couples participating.
Embryo Collection, Culturing, and Developmental Scoring
Couples who had undergone in vitro fertilization (IVF) treatment at the Center for Reproduction, Uppsala, Sweden, were asked to donate surplus cryopreserved embryos that otherwise, according to Swedish law, had to be destroyed following 5 years of cryopreservation.
Before freezing the oocytes were inseminated with sperm in IVF medium (G-IVF Plus™) (catalog no. 10136, Vitrolife, Sweden) and transferred to G1 medium (G1 Plus™) (catalog no. 10128, Vitrolife, Sweden) after evaluation of fertilization. Only zygotes with two pro-nuclei were selected for further culture. Embryos were cultured in 25 μL droplets of medium under an oil overlay, in a humidified incubator at 37°C and 6% CO2/6% O2.
All in all, 48 human embryos were used and no embryos were obtained from the same couple. The experiments were performed in two parts: (1) six embryos in each group were used for mass spectrometry analysis (see below) and (2) 10 embryos in each group were used for protein detection in culture medium and for immunofluorescent staining (see below). Cryopreserved 2-day embryos were thawed using a thawing kit (Sydney IVF Thawing Kit) (catalog no. G19014 Cook Medical Inc., US) and transferred to equilibrated culture medium used for human cleavage stage embryos (CCM) (catalog no. 10093, Vitrolife, Sweden) (Hambiliki et al., 2013). An independent embryologist scored the embryos and ensured that the three treatment groups would be as similar as possible at baseline. Following this group allocation, each group was randomly assigned to treatment with 0.25 or 0.5 μM fluoxetine or control. The fluoxetine doses were chosen based on the literature, information on maternal SSRI concentrations from one of our studies, and a pilot study. The literature suggested that 0.25 and 0.5 μM fluoxetine are within the ranges found in amniotic fluid of women treated with fluoxetine during pregnancy (Hendrick et al., 2003; Rampono et al., 2004; Loughhead et al., 2006). According to yet unpublished findings, fluoxetine concentrations in late pregnant women vary between 0.1 and 0.7 μmol/L, which should approximate the concentration to which the embryo is exposed. Finally, in a pilot study we found that embryos treated with 1.0 μM fluoxetine (n = 3) tended to develop faster but also die to a higher degree than control embryos.
For treatment with fluoxetine, fluoxetine was added to the culture medium to reach the concentrations of 0.25 or 0.5 μM fluoxetine (catalog no. F132, Sigma-Aldrich Corp., US), while control embryos were cultured in CCM medium without additives. All embryos were treated using the same batch of culturing medium, and each experiment included a blank media. The embryos were then cultured using time-lapse monitoring in an EmbryoScope® (Unisense FertiliTech, Denmark) for 4 days (i.e., until day six after insemination) in 6% CO2 and 6% O2 at 37°C (Hambiliki et al., 2013). Images of the embryos were recorded at 15 min intervals. The embryo quality was evaluated retrospectively using standard morphological criteria for cleavage stage embryos, according to Alpha Scientists in Reproductive Medicine and ESHRE Special Interest Group of Embryology (2011). The scoring was performed by an independent observer. Timing of different embryo developmental stages was determined as time after thawing, e.g., time to first division after thawing of the embryo. Embryos from both experiments were used for evaluation (n = 16 in each group). The cut off value for a high-quality embryo was the same as for blastocysts selected for transfer to the women, according to Alpha Scientists in Reproductive Medicine and ESHRE Special Interest Group of Embryology (2011).
Shotgun Mass Spectrometry of Embryos
After the embryos had been cultured, the medium was collected and saved for further analyses. The embryos (six embryos per condition) were snap frozen and run individually in a shotgun mass spectrometry analysis, for the detection of proteins expressed in the embryos. One embryo per condition was used for a pilot study and the data presented in the results are based on the five remaining embryos.
Embryonic samples were dissolved in four times the sample volume of 2 M thiourea (catalog no. 107979, Merck KGaA, Germany) /6 M urea (catalog no. 108488, Merck KGaA, Germany) in 50 mM ammonium bicarbonate (catalog no. 09830, Fluka, Germany). Cell lysis and protein extraction was performed by vigorous vortexing followed by 2 h incubation at −20°C and a final step of vigorous vortexing. The proteins were reduced with dithiothreitol (DTT) (catalog no. D5545, Sigma-Aldrich Corp., US) and alkylated with iodoacetamide (IAA) (catalog no. I1149, Sigma-Aldrich Corp., US) prior to enzymatic digestion. The digestion was performed using two different enzymes. First, the proteins were digested with Lys-C (catalog no. 129-02541, Wako Chemicals GmbH, Germany) for 2 h at room temperature (RT). Thereafter the urea concentration was diluted to < 2 M using 50 mM ammonium bicarbonate, and trypsin (catalog no. V5111, Promega, US) was added to the samples. The tryptic digestion was carried out at 37°C overnight. Prior to the analysis by mass spectrometry the peptides were purified by ZipTips® (catalog no. ZTC18SO96, Merck Millipore, US) (Bergquist et al., 2002) and dried in a SpeedVac® system.
All analyses were performed using a QExactive Plus Orbitrap mass spectrometer (Thermo Fisher Scientific, Germany) equipped with a nano electrospray ion source. Samples were dissolved in water/ formic acid (0.1%) (catalog no. 100264, Merck KGaA, Germany), and peptides were separated by reversed phase liquid chromatography using an EASY-nLC 1000 system (Thermo Fisher Scientific, Germany). A set-up of pre-column and analytical column was used. The pre-column was a 2 cm EASY-column (1D 100 μm, 5 μm C18) (catalog no. SC001, Thermo Fisher Scientific, Germany) and the analytical column was a 10 cm EASY-column (ID 75 μm, 3 μm, C18) (catalog no. SC2003, Thermo Fisher Scientific, Germany). Peptides were eluted with a 150 min linear gradient from 4 to 100% acetonitrile at 250 nL/min. The mass spectrometer was operated in positive ion mode, acquiring a survey mass spectrum with resolving power 70,000 and consecutive high collision dissociation (HCD) fragmentation spectra of the 10 most abundant ions.
The acquired data (RAW-files) were submitted to the Mascot™ search algorithm (Matrix Science, UK) embedded in Proteome Discoverer software (Version 1.4.0.288, Thermo Fisher Scientific, Germany) and searched against human proteins in the UniProtKB/Swiss-Prot database. The search parameters included: maximum 10 ppm and 0.02 Da error tolerance for the survey scan and MS/MS analysis, respectively; enzyme specificity was trypsin; maximum two missed cleavage sites were allowed; cysteine carbamidomethylation was set as static modification; oxidation (M) and deamidation (N,Q) were set as variable modifications. The protein identifications were based on at least two matching peptides of 95% confidence per protein.
Immunofluorescent Staining of Embryos
After culturing, two high-quality embryos from each group were briefly washed in sterile phosphate-buffered saline (PBS) containing 0.8 mg/mL polyvinylpyrrolidon (PVP) Clinical Grade (catalog no. 1090500, MediCult, Origio, Denmark), fixated in 2.5% paraformaldehyde in PBS for 15 min at RT and then stored in PBS/PVP at 4°C. The embryos were permeabilized in 0.25% Triton X-100 in PBS/PVP for 30 min at RT, blocked in blocking solution containing 0.1% bovine serum albumin (BSA) and 0.01% Tween20 in PBS for 15 min at RT, and incubated with primary antibody (diluted 1:100 in blocking solution) overnight at 4°C. Primary antibodies against urokinase-type plasminogen activator (uPA) (catalog no. sc-6830) and nerve growth factor (NGF) (catalog no. sc-548) were used, both from SantaCruz Biotechnology Inc., US. After incubation, the embryos were washed 3 × 15 min in blocking solution, incubated with secondary antibodies (diluted 1:100 in blocking solution) for 1 h at RT and washed again 3 × 15 min in blocking solution. The secondary antibodies were labeled with fluorophores AlexaFluor488 and AlexaFluor594 (catalog no. A-21206 and A-11058, Life Technologies, Thermo Scientific Inc., US). After washing, the embryos were mounted on a slide in 5 μL of VectaShield (Vector Laboratories Inc., US) surrounded by Vaseline. A cover slip was lowered onto the slide, gently pressed against the Vaseline, sealed with nail varnish and allowed to dry. Pictures were taken using a fluorescence microscope (20x objective; Axio Observer.Z1, Carl Zeiss AG Corp. Germany).
Protein Detection in Embryo Culture Medium
After embryo culturing, 20 μL of the culture medium was snap frozen (n = 10 in each group) and protein analysis was run on each individual sample by using Proseek Multiplex Immunoassay analysis based on Proximity Extension Assay Technology (Olink Bioscience, Sweden) (Lundberg et al., 2011; Assarsson et al., 2014). The Proseek Multiplex Inflammation I96×96 panel was chosen for this study and the analysis was run at Olink Bioscience facilities in Uppsala, Sweden.
Statistical Analyses
For analysis of proteins detected in the embryo, a Venn diagram was used to elucidate which proteins were uniquely expressed in each fluoxetine dose group and which proteins were detected in both fluoxetine groups (http://bioinfogp.cnb.csic.es/tools/venny/index.html). QIAGEN's Ingenuity Pathway Analysis (IPA®) (QIAGEN, Redwood City, US; www.qiagen.com/ingenuity) was used to determine which pathways the proteins of interest were involved in. IPA computes a score for each network according to the fit of that network to the user-defined set of focus proteins. The score, derived from a p-value, indicates the likelihood of the association between the focus proteins and a given pathway.
The timing of developmental stages, the ability to form a blastocyst, the quality of the embryos, as well as protein levels were compared by use of Mann-Whitney U-test. All statistical analyses were performed by the IBM Statistical Package for the Social Sciences 20.0 (IBM Corp., Armonk, US) for Windows software package.
Ethical Approval
The study was approved by the Regional Ethical Review Board in Uppsala, Sweden (2014/298).
Results
Embryo Development
The impact of fluoxetine on embryo development was evaluated. Human embryos exposed to 0.25 or 0.5 μM fluoxetine were compared to embryos cultured in control medium (n = 16 in each group). The embryos were cultured for 4 days under conditions similar to those used in assisted reproduction and were monitored by a time-lapse system. No differences between treatment groups were noted at baseline (Table 1). Embryos treated with 0.25 μM fluoxetine reached the individual developmental stages after thawing at almost the same time as control embryos. The embryos treated with 0.5 μM fluoxetine tended to develop more quickly, with a shorter time for development to start of cavitation after thawing (p = 0.065; Table 1). No significant differences in the ability to form a blastocyst or the proportion of high-quality embryos were noted between fluoxetine supplement and the control conditions (Table 1).
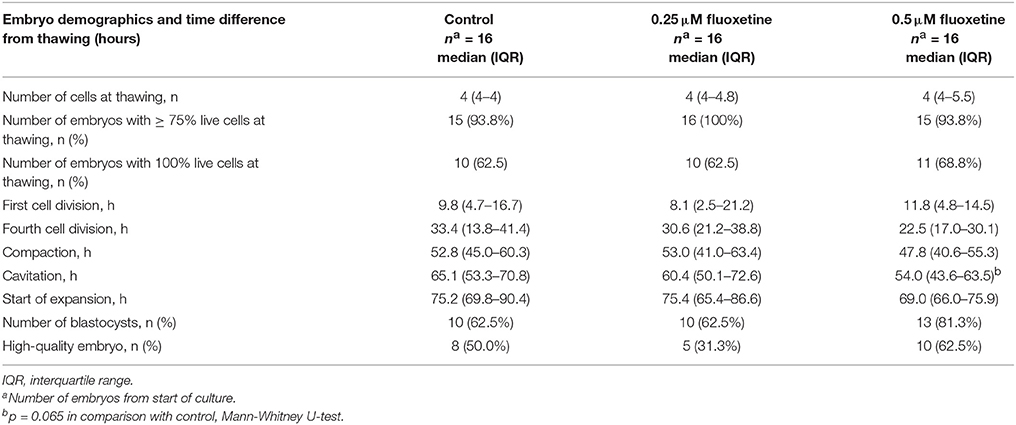
Table 1. Embryo demographics at thawing, timing of different developmental stages after thawing, the number of embryos that developed into blastocysts, and the number of high-quality embryos following treatment with 0.25 or 0.5 μM fluoxetine.
Proteomics and Secretomics
A proteome analysis on the human embryos was performed to investigate unique protein expression patterns induced by fluoxetine. Total protein lysate from single embryos, cultured in ordinary medium supplemented with 0.25 or 0.5 μM fluoxetine, were analyzed by use of shotgun mass spectrometry and compared to embryos cultured in ordinary medium. As presented in Table 2 and Figure 1, a number of differentiated proteins were detected in the embryos. In total, 45 proteins were uniquely expressed in fluoxetine-treated embryos, among which 24 were detected in both fluoxetine-dose groups (Table 2). Nine proteins were uniquely expressed by embryos treated with 0.25 μM fluoxetine and 12 unique proteins were detected in embryos treated with the higher fluoxetine dose (Table 2). Only proteins that were detected in at least three out of five embryos in one group are presented. In order to determine the biological relevance of these proteins, an IPA analysis was performed, focusing on proteins that were expressed in embryos treated with 0.5 μM fluoxetine (in total 36 proteins). Three protein networks of relevance were detected in 0.5 μM fluoxetine-treated embryos (Table 3 and Figure S1): (1) Cell Death and Survival, Cellular Growth and Proliferation, Hematological Disease with an IPA score of 23; (2) Cancer, Organismal Injury and Abnormalities, Reproductive System Disease with an IPA score of 18; and (3) Inflammatory Response, Cell Death and Survival, Digestive System Development and Function with an IPA score of 14.
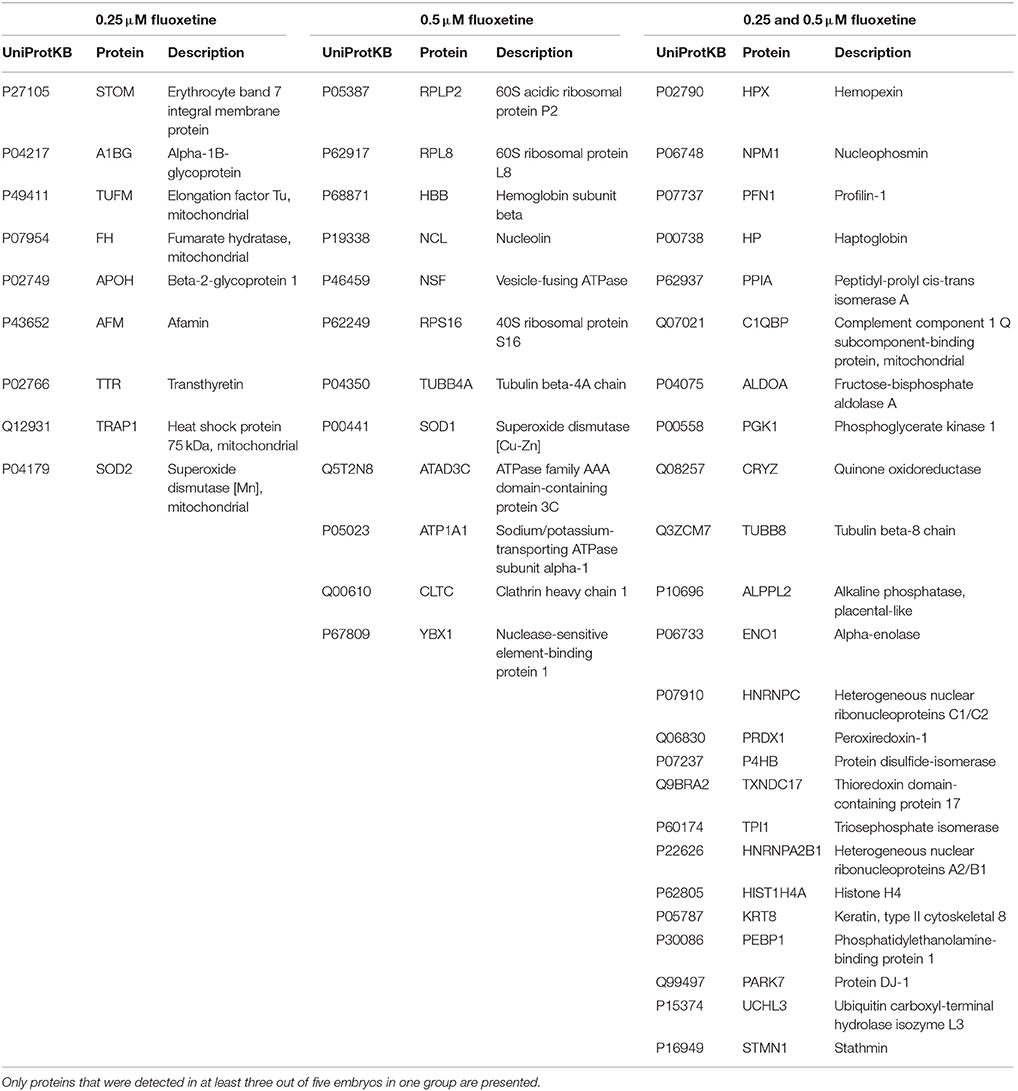
Table 2. Proteins detected by mass spectrometry that were found uniquely in 0.25 μM fluoxetine- and 0.5 μM fluoxetine-treated embryos.
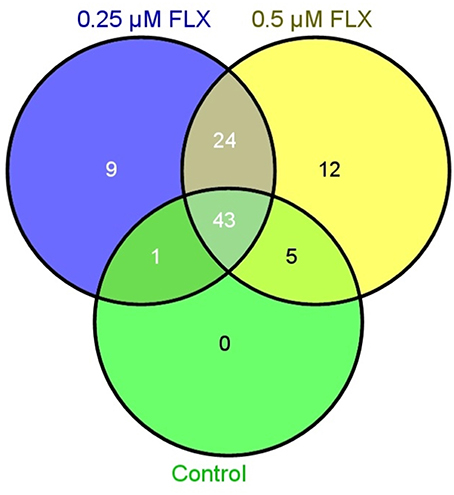
Figure 1. Venn diagram illustrating the number of proteins detected by mass spectrometry in embryos treated with 0.25 μM fluoxetine (FLX), 0.5 μM fluoxetine and controls.

Table 3. Networks identified by Ingenuity Pathway Analysis (IPA), focusing on the proteins detected in 0.5 μM fluoxetine-treated embryos.
Finally, protein secretion from fluoxetine-treated embryos was compared with control embryos. Culture medium in which the different embryos had been cultured was analyzed by use of the Olink Proseek Multiplex Inflammation I96×96 Immunoassay. After normalization against culture medium without fluoxetine supplement, a number of proteins were detected above the limit of detection (LOD) (Table 4 and Table S1). Urokinase-type plasminogen activator (uPA) levels were significantly higher in 0.5 μM-treated embryos than in 0.25 μM-treated embryos. For the other proteins, there were either no significant differences between the groups or the number of embryos where the protein could be detected was too limited for statistical analyses (Table 4 and Table S1).
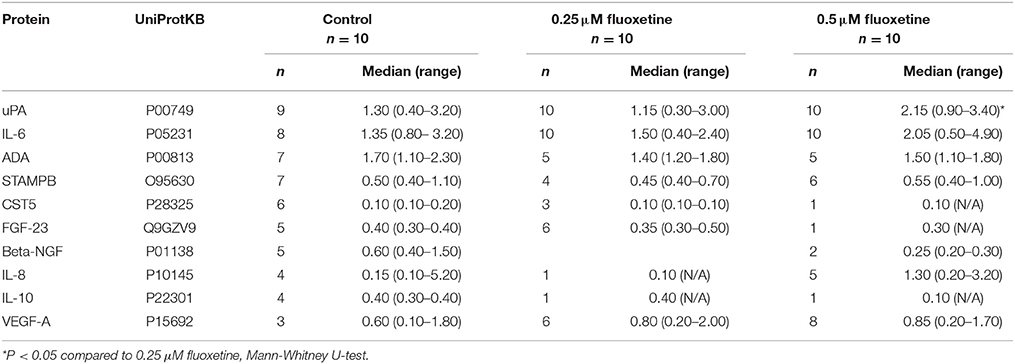
Table 4. Top 10 proteins detected by Multiplex Immunoassay analysis in medium from 0.25 μM fluoxetine-, 0.5 μM fluoxetine-treated and control embryos.
Immunohistochemical Staining of Cultured Embryos
To validate true presence in human embryos for some of the proteins of interest, immunohistochemistry was performed. uPA and nerve growth factor (NGF) were chosen because of their high ranking in the Multiplex Immunoassay analysis. Furthermore, we have previously found differences in NGF signaling in SSRI-exposed placenta (Kaihola et al., 2015). Staining of uPA and NGF was found in the cytosol and cellular membrane of the trophectoderm of the embryos. NGF was also found in the inner cell mass, whereas uPA had a weak staining in the inner cell mass (Figure 2)
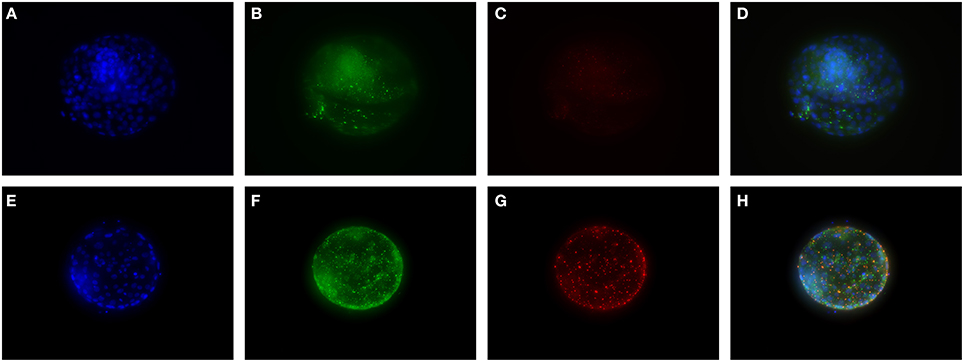
Figure 2. Immunohistochemical staining of cultured human embryos. (A–D) are embryos cultured in control medium, (E–H) are embryos cultured in 0.5 μM FLX. DAPI staining (blue) shows cell nuclei. Staining for NGF is shown in green and staining for uPA in red. (D,H) are overlay pictures.
Discussion
We have previously shown that SSRI treatment during pregnancy affects gene expression and protein levels in the placenta, and pathways of interest for placental function (Kaihola et al., 2015; Olivier et al., 2015b). Based on the established role for 5-HT in embryogenesis (Lauder and Krebs, 1978; Lauder et al., 1981; reviewed in Buznikov et al., 2001), and the fact that serotonin transporters are expressed in early mouse embryos (Amireault and Dube, 2005), we hypothesized that the fetus may also be affected though direct exposure to SSRIs. In this study we demonstrate that fluoxetine has a marginal influence on early human embryo development in culture. Whereas the lower fluoxetine dose had no influence on embryo development compared to controls, embryos treated with 0.5 μM fluoxetine tended to need a shorter time between thawing and start of cavitation. Furthermore, a small dose-response pilot study was conducted before the trial started, where even higher concentrations of fluoxetine (1.0 μM) increased the number of dead embryos. These findings are in line with prior research in mouse embryos (Kim et al., 2012), where it was shown that short-term exposure of 2-cell mouse embryos to fluoxetine increased the percentage of blastocysts via activation of Ca2+/calmodulin-dependent protein kinase II (CaMKII)-dependent signal transduction pathways. Furthermore, fluoxetine enhanced mouse embryonic development into blastocysts up to a certain dose, followed by an inhibition of blastocyst formation at higher doses (Kim et al., 2012). In the study by Kim et al. (2012), short-term exposure (6 h) of 2-cell mouse embryos to doses up to 5–10 μM fluoxetine increased the number of blastocysts. However, longer exposure to 5 μM fluoxetine (up to 72 h) resulted in a reduction of embryos that developed into blastocysts. Notably, the doses used in the present study corresponded to one tenth of the doses used in mice, but were chosen to correspond with human physiological umbilical cord and amniotic fluid concentrations of fluoxetine-exposed fetuses (Hendrick et al., 2003; Rampono et al., 2004; Loughhead et al., 2006). From research within the field of IVF, it is known that timing to different developmental stages correlate with embryo quality and with implantation rate after assisted reproduction (Wong et al., 2010; Meseguer et al., 2011; Machtinger and Racowsky, 2013; Kaser and Racowsky, 2014). Although the optimal embryo morphokinetics remains to be settled, i.e., whether accelerated development is good or bad for the implantation rate, the lower fluoxetine dose had less impact than the higher dose on embryo development in our experiments, and this was also true for the embryo protein expression and secretion. For this reason, clinicians should adhere to recent National Institute for Health and Care Excellence (NICE) guidelines (National Institute for Health and Care Excellence, 2014) on SSRI treatment in pregnancy, and prescribe the lowest possible dose in women who need to continue antidepressant therapy when pregnant. However, while the lower dose had less impact, according to what could be detected with the present methods, this finding does not indicate that it is harmless.
The exact mechanism by which SSRI influence early embryo development is not known, although alteration in serotonin levels (Lauder et al., 1981) or activation of CaMKII-dependent signal transduction pathways would be plausible (Kim et al., 2012). Based on our results from the mass spectrometry and proteomics analysis, we hypothesize that pathways of relevance for regulation of cellular growth, proliferation and survival are affected by fluoxetine. The Multiplex Immunoassay analysis revealed significantly increased levels of uPA in culture medium from 0.5 μM fluoxetine-treated embryos. uPA has been shown to be involved in cell proliferation, cell migration (reviewed in Noh et al. (2013) and cellular differentiation. Lino et al. (2014) showed that uPA in complex with its receptor, urokinase-type plasminogen activator receptor (uPAR), is involved in cell signaling during neuronal migration and neuritogenesis in explants from chick embryos. In addition, fluoxetine could be acting through other, hitherto unknown, mechanism(s).
The inconsistency between the Multiplex Immunoassay and mass spectrometry as regards uPA may be due to two different factors. First, the multiplex immunoassay was performed in the culture medium and the mass spectrometry in the embryo, whereby the former method would detect proteins secreted by the embryo and the latter proteins within the embryo. Thus, high levels of uPA in the secretome do not necessarily correspond to the levels detected in the embryo. Potentially, if secretion is high, the embryonic pool of a specific protein could be depleted. Secondly, the Multiplex Immunoassay analysis includes ready-made panels with antibodies directed against target of interest and even though proteins detected by this method were not detected by mass spectrometry, they may very well be expressed in the embryo although not at levels detected by mass spectrometry. However, the detection of uPA by Multiplex Immunoassay analysis was verified by immunohistochemistry of the embryos, where uPA was found mainly in the trophectoderm. The staining for uPA in the trophectoderm goes well with the findings of Khamsi et al. (1996), where uPA was detected in human preimplantation embryos. Both Hofmann et al. (1994) and Teesalu et al. (1996) showed that uPA is expressed in the trophoblast at the maternal-fetal interface in human and mouse implantation sites. Later on in development the trophectoderm will become the placenta, therefore any alterations in placental protein levels may ultimately affect the function of the placenta and then, consequently, also the development of the fetus. Indeed, uPA seems to play a role in trophoblast invasion and in the pathophysiology of preeclampsia (Strickland and Richards, 1992; Zhang et al., 1994; Uszynski and Uszynski, 2011), and may contribute to the increased risk of preeclampsia previously noted in SSRI users (Toh et al., 2009; De Vera and Berard, 2012; Palmsten et al., 2012, 2013). Also, NGF was detected in the embryo trophectoderm which is in line with our previous studies (Kaihola et al., 2015), where the placental NGF levels were increased in placenta from SSRI-treated women.
Our aim with this study was to investigate the effects of pharmacologically relevant levels of fluoxetine on human early embryonic development. For obvious reasons, the number of human embryos that can be used for research is not unlimited. The relatively low number of embryos in this study could have an impact on the results, and this is particularly true for the blastocyst formation and the number of high-quality embryos in each treatment group. However, we noted more than 40 proteins uniquely expressed in fluoxetine-exposed embryo, and also changes in protein expression in the embryo culture medium. Obviously, with a greater sample size, more fluoxetine-induced differences could have been uncovered. The analysis method used for the proteomics has been used in several previous studies within reproductive medicine (Nilsson et al., 2004; Naessen et al., 2010; Hambiliki et al., 2013), but the complexity of the method should be taken into consideration when interpreting and analyzing the results. The embryos used were at a very early stage of development and would in the normal course of events not have implanted in the uterus yet. This means that the placenta has not yet been developed and there is no amniotic fluid or umbilical cord through which fluoxetine could reach and affect the embryo. However, both the oocyte and the embryo could be exposed to fluoxetine, both by the follicular fluid in the ovaries or during the pre-implantation period by secretions in the tuba. Indeed, previous research has indicated that serotonin is found in the follicular fluid and fallopian tubes, at least in female rats (Amenta et al., 1992; Bodis et al., 1993).
Another limitation is that our study cannot elucidate whether the proteins expressed is due to fluoxetine exposure, or the slightly enhanced embryo development, or both. At present, the embryo development proteomics is not known to its full extent, and for that reason, we cannot single out the proteins that would be normally expressed and the ones that are due to toxic effects.
In conclusion, we have studied the effects of fluoxetine on human early embryonic development. We found that fluoxetine has marginal effects on the timing of developmental stages in embryos, but induces expression and secretion of several proteins in a manner that depends on dose. For these reasons, and in line with current guidelines, the lowest possible dose of SSRI should be used in pregnant women who need to continue treatment.
Author Contributions
Conceived and designed the experiments: HK, JO, HÅ, IS. Performed the experiments: HK, FG, JH, KH. Analyzed the data: HK, KH, HÅ, IS. Contributed with reagents/materials/analysis tools: JB, HÅ, IS. Drafting and/or revising the paper, including final approval to publish: HK, FG, JH, KH, JB, JO, HÅ, IS.
Funding
This research was supported by the Swedish Research Council (grant VR 621-2011-4423) and the Family Planning Foundation, Uppsala.
Conflict of Interest Statement
The authors declare that the research was conducted in the absence of any commercial or financial relationships that could be construed as a potential conflict of interest.
Acknowledgments
This work was supported by the Mass Spectrometry-based Proteomics Facility at the Science for Life Laboratory in Uppsala. Data storage was obtained and supported by BILS (Bioinformatics Infrastructure for Life Sciences).
Supplementary Material
The Supplementary Material for this article can be found online at: https://www.frontiersin.org/article/10.3389/fncel.2016.00160
References
Alpha Scientists in Reproductive Medicine and ESHRE Special Interest Group of Embryology (2011). The Istanbul consensus workshop on embryo assessment: proceedings of an expert meeting. Hum. Reprod. 26, 1270–1283. doi: 10.1093/humrep/der037
Amenta, F., Vega, J. A., Ricci, A., and Collier, W. L. (1992). Localization of 5-hydroxytryptamine-like immunoreactive cells and nerve fibers in the rat female reproductive system. Anat. Rec. 233, 478–484. doi: 10.1002/ar.1092330315
Amireault, P., and Dube, F. (2005). Serotonin and its antidepressant-sensitive transport in mouse cumulus-oocyte complexes and early embryos. Biol. Reprod. 73, 358–365. doi: 10.1095/biolreprod.104.039313
Andersson, L., Sundstrom-Poromaa, I., Bixo, M., Wulff, M., Bondestam, K., and aStrom, M. (2003). Point prevalence of psychiatric disorders during the second trimester of pregnancy: a population-based study. Am. J. Obstet. Gynecol. 189, 148–154. doi: 10.1067/mob.2003.336
Ansorge, M. S., Hen, R., and Gingrich, J. A. (2007). Neurodevelopmental origins of depressive disorders. Curr. Opin. Pharmacol. 7, 8–17. doi: 10.1016/j.coph.2006.11.006
Assarsson, E., Lundberg, M., Holmquist, G., Björkesten, J., Bucht Thorsen, S., Ekman, D., et al. (2014). Homogenous 96-Plex PEA immunoassay exhibiting high sensitivity, specificity, and excellent scalability. PLoS ONE 9:e95192. doi: 10.1371/journal.pone.0095192
Barbey, J. T., and Roose, S. P. (1998). SSRI safety in overdose. J. Clin. Psychiatry 59(suppl. 15), 42–48.
Bergquist, J., Palmblad, M., Wetterhall, M., Hakansson, P., and Markides, K. E. (2002). Peptide mapping of proteins in human body fluids using electrospray ionization Fourier transform ion cyclotron resonance mass spectrometry. Mass Spectrom. Rev. 21, 2–15. doi: 10.1002/mas.10016
Bodis, J., Hartmann, G., Tinneberg, H. R., Torok, A., Hanf, V., Papenfuss, F., et al. (1993). Relationship between the monoamine, progesterone and estradiol content in follicular fluid of preovulatory graafian follicles after superovulation treatment. Gynecol. Obstet. Invest. 35, 232–235. doi: 10.1159/000292706
Bonnin, A., and Levitt, P. (2012). Placental source for 5-HT that tunes fetal brain development. Neuropsychopharmacology 37, 299–300. doi: 10.1038/npp.2011.194
Buznikov, G. A., Lambert, H. W., and Lauder, J. M. (2001). Serotonin and serotonin-like substances as regulators of early embryogenesis and morphogenesis. Cell Tissue Res. 305, 177–186. doi: 10.1007/s004410100408
Croen, L. A., Grether, J. K., Yoshida, C. K., Odouli, R., and Hendrick, V. (2011). Antidepressant use during pregnancy and childhood autism spectrum disorders. Arch. Gen. Psychiatry 68, 1104–1112. doi: 10.1001/archgenpsychiatry.2011.73
De Vera, M. A., and Berard, A. (2012). Antidepressant use during pregnancy and the risk of pregnancy-induced hypertension. Br. J. Clin. Pharmacol. 74, 362–369. doi: 10.1111/j.1365-2125.2012.04196.x
El Marroun, H., Jaddoe, V. W., Hudziak, J. J., Roza, S. J., Steegers, E. A., Hofman, A., et al. (2012). Maternal use of selective serotonin reuptake inhibitors, fetal growth, and risk of adverse birth outcomes. Arch. Gen. Psychiatry 69, 706–714. doi: 10.1001/archgenpsychiatry.2011.2333
El Marroun, H., White, T., Verhulst, F. C., and Tiemeier, H. (2014). Maternal use of antidepressant or anxiolytic medication during pregnancy and childhood neurodevelopmental outcomes: a systematic review. Eur. Child Adolesc. Psychiatry 23, 973–992. doi: 10.1007/s00787-014-0558-3
Furu, K., Kieler, H., Haglund, B., Engeland, A., Selmer, R., Stephansson, O., et al. (2015). Selective serotonin reuptake inhibitors and venlafaxine in early pregnancy and risk of birth defects: population based cohort study and sibling design. BMJ 350:h1798. doi: 10.1136/bmj.h1798
Gaspar, P., Cases, O., and Maroteaux, L. (2003). The developmental role of serotonin: news from mouse molecular genetics. Nat. Rev. Neurosci. 4, 1002–1012. doi: 10.1038/nrn1256
Gavin, N. I., Gaynes, B. N., Lohr, K. N., Meltzer-Brody, S., Gartlehner, G., and Swinson, T. (2005). Perinatal depression: a systematic review of prevalence and incidence. Obstet. Gynecol. 106, 1071–1083. doi: 10.1097/01.AOG.0000183597.31630.db
Gentile, S. (2005). SSRIs in pregnancy and lactation: emphasis on neurodevelopmental outcome. CNS Drugs 19, 623–633. doi: 10.2165/00023210-200519070-00004
Hambiliki, F., Hanrieder, J., Bergquist, J., Hreinsson, J., Stavreus-Evers, A., and Wanggren, K. (2013). Glycoprotein 130 promotes human blastocyst development in vitro. Fertil. Steril. 99, 1592–1599. doi: 10.1016/j.fertnstert.2012.12.041
Hendrick, V., Stowe, Z. N., Altshuler, L. L., Hwang, S., Lee, E., and Haynes, D. (2003). Placental passage of antidepressant medications. Am. J. Psychiatry 160, 993–996. doi: 10.1176/appi.ajp.160.5.993
Hofmann, G. E., Glatstein, I., Schatz, F., Heller, D., and Deligdisch, L. (1994). Immunohistochemical localization of urokinase-type plasminogen activator and the plasminogen activator inhibitors 1 and 2 in early human implantation sites. Am. J. Obstet. Gynecol. 170, 671–676. doi: 10.1016/S0002-9378(94)70246-2
Il'kova, G., Rehak, P., Vesela, J., Cikos, S., Fabian, D., Czikkova, S., et al. (2004). Serotonin localization and its functional significance during mouse preimplantation embryo development. Zygote 12, 205–213. doi: 10.1017/S0967199404002862
Kaihola, H., Olivier, J., Poromaa, I. S., and Åkerud, H. (2015). The effect of antenatal depression and selective serotonin reuptake inhibitor treatment on nerve growth factor signaling in human placenta. PLoS ONE 10:e0116459. doi: 10.1371/journal.pone.0116459
Kallen, B. (2004). Neonate characteristics after maternal use of antidepressants in late pregnancy. Arch. Pediatr. Adolesc. Med. 158, 312–316. doi: 10.1001/archpedi.158.4.312
Kaser, D. J., and Racowsky, C. (2014). Clinical outcomes following selection of human preimplantation embryos with time-lapse monitoring: a systematic review. Hum. Reprod. Update 20, 617–631. doi: 10.1093/humupd/dmu023
Khamsi, F., Armstrong, D. T., and Zhang, X. (1996). Expression of urokinase-type plasminogen activator in human preimplantation embryos. Mol. Hum. Reprod. 2, 273–276. doi: 10.1093/molehr/2.4.273
Khozhai, L. I., Puchkov, V. F., and Otellin, V. A. (1995). The effect of a serotonin deficiency on mammalian embryonic development. Ontogenez 26, 350–355.
Kieler, H., Artama, M., Engeland, A., Ericsson, O., Furu, K., Gissler, M., et al. (2012). Selective serotonin reuptake inhibitors during pregnancy and risk of persistent pulmonary hypertension in the newborn: population based cohort study from the five Nordic countries. BMJ 344:d8012. doi: 10.1136/bmj.d8012
Kim, C. W., Choe, C., Kim, E. J., Lee, J. I., Yoon, S. Y., Cho, Y. W., et al. (2012). Dual effects of fluoxetine on mouse early embryonic development. Toxicol. Appl. Pharmacol. 265, 61–72. doi: 10.1016/j.taap.2012.09.020
Lauder, J. M., and Krebs, H. (1978). Serotonin as a differentiation signal in early neurogenesis. Dev. Neurosci. 1, 15–30. doi: 10.1159/000112549
Lauder, J. M., Wallace, J. A., and Krebs, H. (1981). Roles for serotonin in neuroembryogenesis. Adv. Exp. Med. Biol. 133, 477–506. doi: 10.1007/978-1-4684-3860-4_28
Lino, N., Fiore, L., Rapacioli, M., Teruel, L., Flores, V., Scicolone, G., et al. (2014). uPA-uPAR molecular complex is involved in cell signaling during neuronal migration and neuritogenesis. Dev. Dyn. 243, 676–689. doi: 10.1002/dvdy.24114
Loughhead, A. M., Fisher, A. D., Newport, D. J., Ritchie, J. C., Owens, M. J., DeVane, C. L., et al. (2006). Antidepressants in amniotic fluid: another route of fetal exposure. Am. J. Psychiatry 163, 145–147. doi: 10.1176/appi.ajp.163.1.145
Lundberg, M., Eriksson, A., Tran, B., Assarsson, E., and Fredriksson, S. (2011). Homogeneous antibody-based proximity extension assays provide sensitive and specific detection of low-abundant proteins in human blood. Nucleic Acids Res. 39, e102. doi: 10.1093/nar/gkr424
Machtinger, R., and Racowsky, C. (2013). Morphological systems of human embryo assessment and clinical evidence. Reprod. Biomed. Online 26, 210–221. doi: 10.1016/j.rbmo.2012.10.021
Meseguer, M., Herrero, J., Tejera, A., Hilligsoe, K. M., Ramsing, N. B., and Remohi, J. (2011). The use of morphokinetics as a predictor of embryo implantation. Hum. Reprod. 26, 2658–2671. doi: 10.1093/humrep/der256
Moiseiwitsch, J. R., and Lauder, J. M. (1995). Serotonin regulates mouse cranial neural crest migration. Proc. Natl. Acad. Sci. U.S.A. 92, 7182–7186. doi: 10.1073/pnas.92.16.7182
Naessen, T., Kushnir, M. M., Chaika, A., Nosenko, J., Mogilevkina, I., Rockwood, A. L., et al. (2010). Steroid profiles in ovarian follicular fluid in women with and without polycystic ovary syndrome, analyzed by liquid chromatography-tandem mass spectrometry. Fertil. Steril. 94, 2228–2233. doi: 10.1016/j.fertnstert.2009.12.081
National Institute for Health and Care Excellence (2014). Antenatal and Postnatal Mental Health: Clinical Management and Service Guidance (CG192). Clinical Guideline 123. Available online at: nice.org.uk/guidance/cg192
Nilsson, S., Ramstrom, M., Palmblad, M., Axelsson, O., and Bergquist, J. (2004). Explorative study of the protein composition of amniotic fluid by liquid chromatography electrospray ionization Fourier transform ion cyclotron resonance mass spectrometry. J. Proteome Res. 3, 884–889. doi: 10.1021/pr0499545
Noh, H., Hong, S., and Huang, S. (2013). Role of urokinase receptor in tumor progression and development. Theranostics 3, 487–495. doi: 10.7150/thno.4218
Oberlander, T. F., Reebye, P., Misri, S., Papsdorf, M., Kim, J., and Grunau, R. E. (2007). Externalizing and attentional behaviors in children of depressed mothers treated with a selective serotonin reuptake inhibitor antidepressant during pregnancy. Arch. Pediatr. Adolesc. Med. 161, 22–29. doi: 10.1001/archpedi.161.1.22
Olivier, J. D., Akerud, H., Kaihola, H., Pawluski, J. L., Skalkidou, A., Hogberg, U., et al. (2013). The effects of maternal depression and maternal selective serotonin reuptake inhibitor exposure on offspring. Front. Cell. Neurosci. 7:73. doi: 10.3389/fncel.2013.00073
Olivier, J. D. A., Åkerud, H., and Sundström Poromaa, I. (2015a). Antenatal depression and antidepressants during pregnancy: unraveling the complex interactions for the offspring. Eur. J. Pharmacol. 753, 257–262. doi: 10.1016/j.ejphar.2014.07.049
Olivier, J. D. A., Åkerud, H., Skalkidou, A., Kaihola, H., and Sundström Poromaa, I. (2015b). The effects of antenatal depression and antidepressant treatment on placental gene expression. Front. Cell. Neurosci. 8:465. doi: 10.3389/fncel.2014.00465
Palmsten, K., Huybrechts, K. F., Michels, K. B., Williams, P. L., Mogun, H., Setoguchi, S., et al. (2013). Antidepressant use and risk for preeclampsia. Epidemiology 24, 682–691. doi: 10.1097/EDE.0b013e31829e0aaa
Palmsten, K., Setoguchi, S., Margulis, A. V., Patrick, A. R., and Hernandez-Diaz, S. (2012). Elevated risk of preeclampsia in pregnant women with depression: depression or antidepressants? Am. J. Epidemiol. 175, 988–997. doi: 10.1093/aje/kwr394
Qiu, C., Williams, M. A., Calderon-Margalit, R., Cripe, S. M., and Sorensen, T. K. (2009). Preeclampsia risk in relation to maternal mood and anxiety disorders diagnosed before or during early pregnancy. Am. J. Hypertens. 22, 397–402. doi: 10.1038/ajh.2008.366
Rampono, J., Proud, S., Hackett, L. P., Kristensen, J. H., and Ilett, K. F. (2004). A pilot study of newer antidepressant concentrations in cord and maternal serum and possible effects in the neonate. Int. J. Neuropsychopharmacol. 7, 329–334. doi: 10.1017/S1461145704004286
Strickland, S., and Richards, W. G. (1992). Invasion of the trophoblasts. Cell 71, 355–357. doi: 10.1016/0092-8674(92)90503-5
Teesalu, T., Blasi, F., and Talarico, D. (1996). Embryo implantation in mouse: fetomaternal coordination in the pattern of expression of uPA, uPAR, PAI-1 and alpha 2MR/LRP genes. Mech. Dev. 56, 103–116. doi: 10.1016/0925-4773(96)00515-1
Toh, S., Mitchell, A. A., Louik, C., Werler, M. M., Chambers, C. D., and Hernandez-Diaz, S. (2009). Selective serotonin reuptake inhibitor use and risk of gestational hypertension. Am. J. Psychiatry 166, 320–328. doi: 10.1176/appi.ajp.2008.08060817
Uszynski, M., and Uszynski, W. (2011). Coagulation and fibrinolysis in amniotic fluid: physiology and observations on amniotic fluid embolism, preterm fetal membrane rupture, and pre-eclampsia. Semin. Thromb. Hemost. 37, 165–174. doi: 10.1055/s-0030-1270345
Waters, C. S., Hay, D. F., Simmonds, J. R., and van Goozen, S. H. (2014). Antenatal depression and children's developmental outcomes: potential mechanisms and treatment options. Eur. Child Adolesc. Psychiatry 23, 957–971. doi: 10.1007/s00787-014-0582-3
Wen, S. W., Yang, Q., Garner, P., Fraser, W., Olatunbosun, O., Nimrod, C., et al. (2006). Selective serotonin reuptake inhibitors and adverse pregnancy outcomes. Am. J. Obstet. Gynecol. 194, 961–966. doi: 10.1016/j.ajog.2006.02.019
Winn, V. D., Gormley, M., Paquet, A. C., Kjaer-Sorensen, K., Kramer, A., Rumer, K. K., et al. (2009). Severe preeclampsia-related changes in gene expression at the maternal-fetal interface include sialic acid-binding immunoglobulin-like lectin-6 and pappalysin-2. Endocrinology 150, 452–462. doi: 10.1210/en.2008-0990
Wisner, K. L., Sit, D. K., Hanusa, B. H., Moses-Kolko, E. L., Bogen, D. L., Hunker, D. F., et al. (2009). Major depression and antidepressant treatment: impact on pregnancy and neonatal outcomes. Am. J. Psychiatry 166, 557–566. doi: 10.1176/appi.ajp.2008.08081170
Wogelius, P., Norgaard, M., Gislum, M., Pedersen, L., Munk, E., Mortensen, P. B., et al. (2006). Maternal use of selective serotonin reuptake inhibitors and risk of congenital malformations. Epidemiology 17, 701–704. doi: 10.1097/01.ede.0000239581.76793.ae
Wong, C. C., Loewke, K. E., Bossert, N. L., Behr, B., De Jonge, C. J., Baer, T. M., et al. (2010). Non-invasive imaging of human embryos before embryonic genome activation predicts development to the blastocyst stage. Nat. Biotechnol. 28, 1115–1121. doi: 10.1038/nbt.1686
Yavarone, M. S., Shuey, D. L., Tamir, H., Sadler, T. W., and Lauder, J. M. (1993). Serotonin and cardiac morphogenesis in the mouse embryo. Teratology 47, 573–584. doi: 10.1002/tera.1420470609
Keywords: embryo development, selective serotonin reuptake inhibitors, serotonin, human, time-lapse monitoring, proteomics, secretomics, shotgun mass spectrometry
Citation: Kaihola H, Yaldir FG, Hreinsson J, Hörnaeus K, Bergquist J, Olivier JDA, Åkerud H and Sundström-Poromaa I (2016) Effects of Fluoxetine on Human Embryo Development. Front. Cell. Neurosci. 10:160. doi: 10.3389/fncel.2016.00160
Received: 16 March 2016; Accepted: 01 June 2016;
Published: 16 June 2016.
Edited by:
Tommaso Pizzorusso, University of Florence and National Research Council, ItalyReviewed by:
Gonzalo Alvarez-Bolado, University of Heidelberg, GermanyMichele Studer, Institut national de la santé et de la recherche médicale, France
Copyright © 2016 Kaihola, Yaldir, Hreinsson, Hörnaeus, Bergquist, Olivier, Åkerud and Sundström-Poromaa. This is an open-access article distributed under the terms of the Creative Commons Attribution License (CC BY). The use, distribution or reproduction in other forums is permitted, provided the original author(s) or licensor are credited and that the original publication in this journal is cited, in accordance with accepted academic practice. No use, distribution or reproduction is permitted which does not comply with these terms.
*Correspondence: Helena Kaihola, helena.kaihola@kbh.uu.se