Breakthrough applications of porous organic materials for membrane-based CO2 separation: a review
- 1School of Computer Science and Engineering, Xi’an Technological University, Xi’an, China
- 2Department of Petroleum and Chemical Engineering, Science and Research Branch, Islamic Azad University, Tehran, Iran
- 3Institute of Research and Development, Duy Tan University, Da Nang, Vietnam
- 4The Faculty of Environment and Chemical Engineering, Duy Tan University, Da Nang, Vietnam
Over the last decades, porous organic materials (POMs) have been extensively employed in various industrial approaches including gas separation, catalysis and energy production due to possessing indisputable advantages like great surface area, high permeability, controllable pore size, appropriate functionalization and excellent processability compared to traditional substances like zeolites, Alumina and polymers. This review presents the recent breakthroughs in the multifunctional POMs for potential use in the membrane-based CO2 separation. Some examples of highly-selective membranes using multifunctional POMs are described. Moreover, various classifications of POMs following with their advantages and disadvantages in CO2 separation processes are explained. Apart from reviewing the state-of-the-art POMs in CO2 separation, the challenges/limitations of POMs with tailored structures for reasonable application are discussed.
1 Introduction
Over the last decades, significant increment in the anthropogenic industrial-based release of carbon dioxide (CO2) greenhouse gas has exacerbated the risk of serious air pollution and unfavorable climate changes like global warming, unbalanced pattern of precipitation and sea-level rise, which not only endanger the humans’ health but also negatively affect economic systems (Nakhjiri et al., 2018/11; Carnicer et al., 2022; Huhe et al., 2023). The maximum permittable value of CO2 in natural gas for commercial natural gas is 2.5% (Mazzetti et al., 2014). Higher value of this water-soluble chemical compound in natural gas can result is different detrimental effects like corrosion of pipe lines and reduction of gas heating value (Nakhjiri et al., 2020). Therefore, development of cutting-edge, environmentally-friendly, cost-effective and breakthrough technologies to increase the separation rate of CO2 from gaseous mixtures is of prime importance in industry. Table 1 aims to present detailed information about CO2.
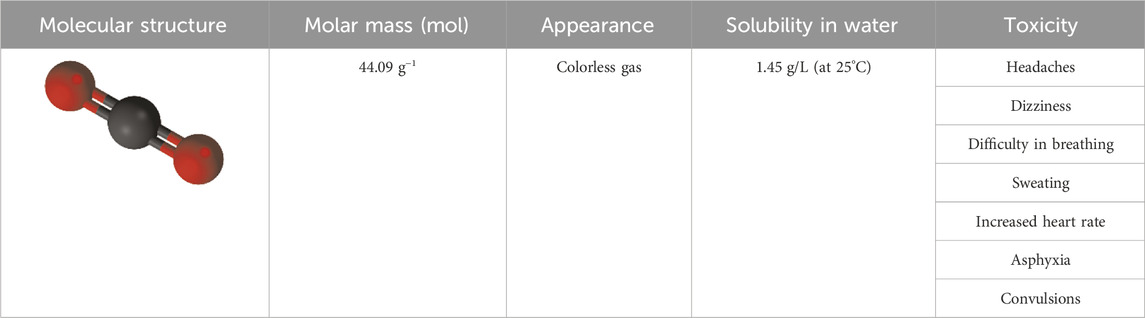
Table 1. Detailed information about CO2 (Stein, 2022; Guais et al., 2011; Vesovic et al., 1990; Cao et al., 2021/09).
In recent years, membrane-based separation technology has been of prime attention in various situations such as CO2 removal, air dehumidification, solvent extraction and precious metals recovery due to its noteworthy advantages such as energy efficiency and environmental benefits (Cannone et al., 2021; Cao et al., 2021; Cao et al., 2021/04; Taghvaie Nakhjiri et al., 2022; Ghadiri et al., 2020; Pishnamazi et al., 2020/09). Appropriate microporous membranes should possess great permeability and selectivity toward separation of specific gases. Despite the significant modification of membranes using physical techniques (i.e., fabrication route and membrane configuration), the advancement of more efficient materials with brilliant separation capabilities has been of great attention among academic researchers (Zou and Zhu, 2018; Babanezhad et al., 2020). Polymers (i.e., cellulose acetate (CA), polyamides (PAs), and polyimides (PIs)) are known as the most prevalent employed membrane materials in industrial gas separation applications, which have been prosperously commercialized since the 1980s (Zhang et al., 2013; Baker and Low, 2014). Generally, polymeric membranes with high selectivity have low permeability, and vice versa. Dense or low-porous phase of polymeric membranes is the reason of justifying this trade-off relationship, which is able to be empirically and theoretically confirmed by Robeson and Freeman, respectively (Freeman, 1999; Robeson, 2008). Porous organic materials (POMs) are developing as an emerging solution for the issue. POMs are known as the hydrocarbons including pores/voids in the microporous region. Their structures are created by organic moieties adjoined via vigorous covalent bonds, often eventuating in ordered and rigid structures. Porous Aromatic Frameworks (PAFs), Conjugated Microporous Polymers (CMPs), Hyper-Cross-Linked Polymers (HCPs), Polymers of Intrinsic Microporosity (PIMs), Covalent Organic Frameworks (COFs) and Covalent Triazine Frameworks (CTFs) are regarded as the certain classifications of the POMs (Nakhjiri and Roudsari, 2016; Das et al., 2020; Krishnaraj et al., 2020; Lee and Cooper, 2020; Lee et al., 2020; Pishnamazi et al., 2020; Ramezanipour Penchah et al., 2020). Based on the suggestion of International Union of Pure and Applied Chemistry (IUPAC), those pores with persistent connection routes with the external surfaces of the porous structure are called open pores, while those pores that are segregated from others are considered as the closed pores (Rouquerol et al., 1994). The open pores possess brilliant capabilities to be applied in fluid dynamics and gas separation, and therefore, have been of great interest among numerous chemists and chemical engineers all over the world (Rouquerol et al., 1994). The classification of the POMs based on their pore size (according to the IUPAC recommendation) is as follows (Das et al., 2017):
A) Microporous POMs with pore size lower than 2 nm;
B) Mesoporous POMs with pore size 2–50 nm;
C) Macroporous POMs with pore size higher than 50 nm.
Figure 1 presents the development of the membrane separation industry.
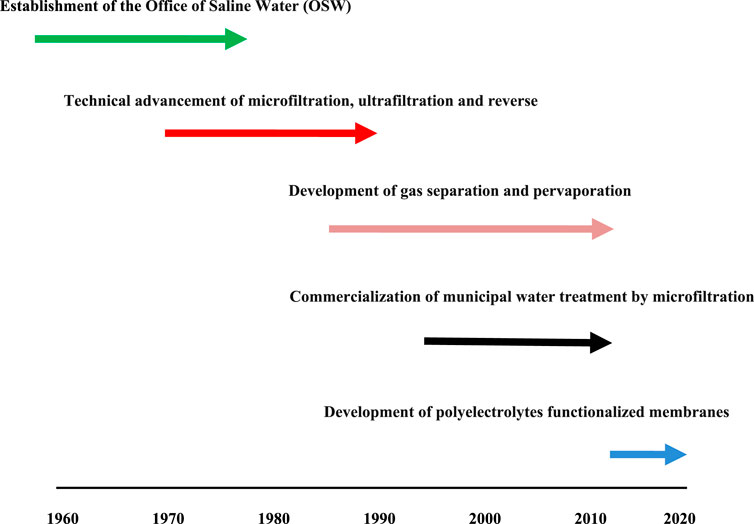
Figure 1. The development of the membrane separation industry, 1960–2020. Adopted from (Baker, 2004; Li et al., 2020).
Most of POMs have noteworthy characteristics such as great surface areas, appropriate thermal stability and negligible framework density. The aforementioned features have made them promising for application in gas separation, catalysis, and biomedical systems (Ding and Wang, 2013; Zou et al., 2013; Little and Cooper, 2020). Therefore, significant endeavors have been made to synthesize and consequently characterize the POMs with disparate chemical structures. POMs-based membranes may act as a novel classification of molecular sieves thanks to their high porosity and small pores at molecular levels. Therefore, great separation efficiency of gas molecules can be expected if POMs are correctly processed for the membranes (Budd and McKeown, 2010; Marjani et al., 2021).
The main objective of this review study is to discuss the current advancements in the application of POMs in CO2 separation. Various classes of POMs such as HCPs, PIMs, COFs, CMPs, CTFs and PAFs following with their advantages and disadvantages in CO2 separation processes are subjected. In addition to reviewing the breakthrough applications of MOFs in CO2 separation, the challenges/restrictions towards the true understanding of POMs with tailored structures for reasonable applications are discussed.
2 Classification of POMs
Here, different types of POMs accompanying with the characteristics of each classification are described.
2.1 HCPs
HCPs are known as one of the most important amorphous polymers possessing great surface areas and low densities, which are synthesized applying disparate chemical procedures (i.e., Friedel − Crafts alkylation chemistry) from other POMs. Moderate reaction conditions, cheap reagents, and ease of scale up are the privileges of HCPs, which make them promising in gas separation, catalysis and removal of aromatic molecules from water (Wood et al., 2008; Xu S. et al., 2013; Masoumi et al., 2020). Wang et al. evaluated the adsorption efficacy of CO2 applying different types of HCPs functionalized by ethylenediamine (EDA). Based on the influence of amine functionalization, they perceived that the selectivity of amine functionalized HCPs (HCPs-A) significantly enhanced and reached to 85.71 and 8.12 for CO2/N2 and CO2/CH4 gaseous mixtures due to increased specific surface area and microporosity (Wang et al., 2020).
The prevalent techniques applied for the synthesis of HCPs are (Xu S. et al., 2013; Tan and Tan, 2017; Yang et al., 2020):
A) Post-cross-linking (PCL) of polymers;
B) Direct one-step polycondensation (DOP);
C) Application of external crosslinkers (ECLs).
The PCL procedure starts by polymeric precursors’ dissolution in solvent. Whenever the swelling process initiates, the polymeric chains are released from a tangled or twisted state and the free area between them is occupied by the solvent. Then, the polymeric chains are exposed to cross-linking process. After solvent removal, the polymeric chains are disassociated using the cross-links, finally eventuating in the preparation of an inter-linked porous polymer. Discovery of Davankov resins has eventuated in an instant advancement in the design, characterization, synthesis and application of HCPs. The most noteworthy privilege of DOP is the direct application of commercial-based accessible polymeric products as precursors for PCL process (Tan and Tan, 2017). Various synthetic procedures consisting of self or co-condensation of chloromethyl/hydroxymethyl-based monomers have been offered for the appropriate synthesis of HCPs. However, all of the abovementioned procedures suffer from certain drawbacks like the need of great amounts of organic reagents and solvents following with energy cost for further purification steps. An outstanding development is ECL strategy in which active formaldehyde dimethyl acetal is used as an external crosslinker to blend simple aromatic components such as benzene with rigid methylene bridges by means of the anhydrous FeCl3 catalyzed Friedel–Crafts reaction (Tan and Tan, 2017). Application of external cross-linkers as the third synthesis procedure, which has resulted in increasing the variety of HCPs (Germain et al., 2007). Schematic demonstration of hyper cross-linked polystyrene is presented in Figure 2.
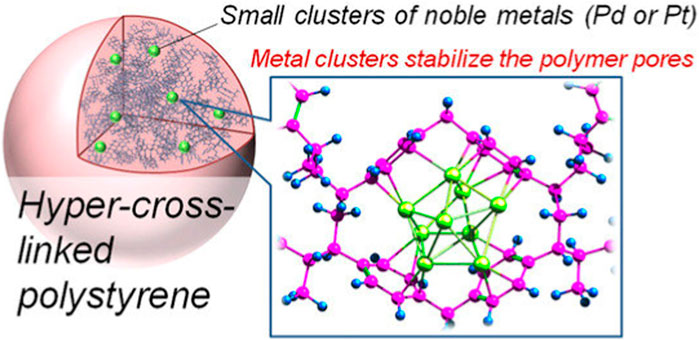
Figure 2. Illustration of hyper cross-linked polystyrene (Bykov et al., 2021).
2.2 PIMs
PIMs are known as the polymers with amorphous microporosity and rigid main chains. Appropriate solubility in organic solvents, great surface area and their film formation characteristics eventuate in the development of PIMs in industry (McKeown, 2012; Agarwal et al., 2020; Perry et al., 2020; Polak-Kraśna et al., 2021). PIMs are an excellent instance of how changing a co-monomer makes a considerable impact on the porosity of polymer. PIMs are characteristically different from other classes of porous polymeric materials. PIMs have microporosity but do not include designated frameworks (Perry et al., 2020). Figure 2 presents a schematic illustration of the phthalocyanine-based PIM. In an investigation, Budd et al. studied the synthesis process of a hexaazatrinapthylene (Hatn)-based PIM. Based on their investigation (Hatn)-based PIM has a great capability to adsorb approximately 3.9 mmol g-1 of the metal complex when exposing to an excess of palladium (II) dichloride in chloroform solution (Budd et al., 2003). Ling et al. fabricated a series of metalized PIMs from a carboxyl-based functionalized PIM (C-PIM). They corroborated that C-PIM-Na demonstrated the greatest CO2 capture capacity of 2.44 mmol g-1 compared to other metalized PIMs (Ling et al., 2020). Stanovsky et al. conducted an experiment with the aim of purifying flue gas applying the ultra-permeable tetramethyl tetrahydronaphthalene - based PIM coupled with bicyclic triptycene. Acceptable CO2/N2 selectivity (in the range of 11–18) encouraged the potential application of this PIM for industrial MCS (Stanovsky et al., 2020). Figure 3 schematically depict the process of PIMs fabrication.
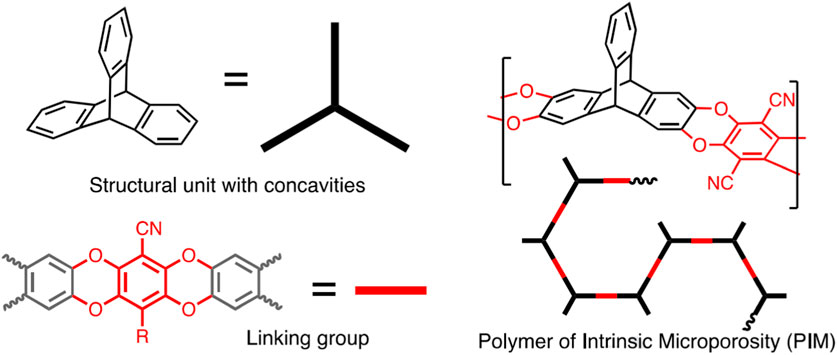
Figure 3. Illustration of PIMs fabrication. Reprinted from (McKeown, 2020) with permission from Elsevier.
The synthesis of PIMs is an important milestone toward the use of this type of POMs in membrane-based CO2 separation. PIMs can be synthesized via a polymerization reaction on the basis of a double-aromatic nucleophilic substitution mechanism (Scherf, 1999). This reaction possesses great potential to form two simultaneous covalent bonds with appropriate performance to provide a linking group composed of fused rings (McKeown, 2012). Overally, aromatic nucleophilic substitutions can be of great interest to be done particularly if the halide-including monomer is activated via an electron-withdrawing substituent (i.e., –CN, F, etc.) (Eastmond et al., 2001).
2.3 COFs
COFs are known as one of the significant members of POMs, which are fabricated via molecular-structured blocks interconnected by covalent bonds. Figure 3 depicts the molecular structure of different COFs. Various features such as custom-made properties (achieved by functionalization), structural versatility and uniform pore size distribution have authorized the COFs to be used in an extensive range of applications like membrane-based gas separation and cancer treatments (Guan et al., 2020; Sharma et al., 2020; Guo et al., 2022). The remarkable development of COFs is prominently justified because of their self-healing capability and thermodynamically manageable covalent chemistry, which eventuate in long-range crystalline structure (Pachfule et al., 2018/01). Compared to MOFs, COFs usually have lower density and therefore show outstanding stability in organic solvents. Moreover, COFs have the ability to tolerate harsh situations and maintain their crystallinity. In comparison with inorganic zeolites and porous silica materials, COFs possess greater efficiency due to higher porosity and tunable pore size, which accelerate the penetrant diffusion (Cooper, 2013). In Figure 4, the molecular structure of different COFs is presented.
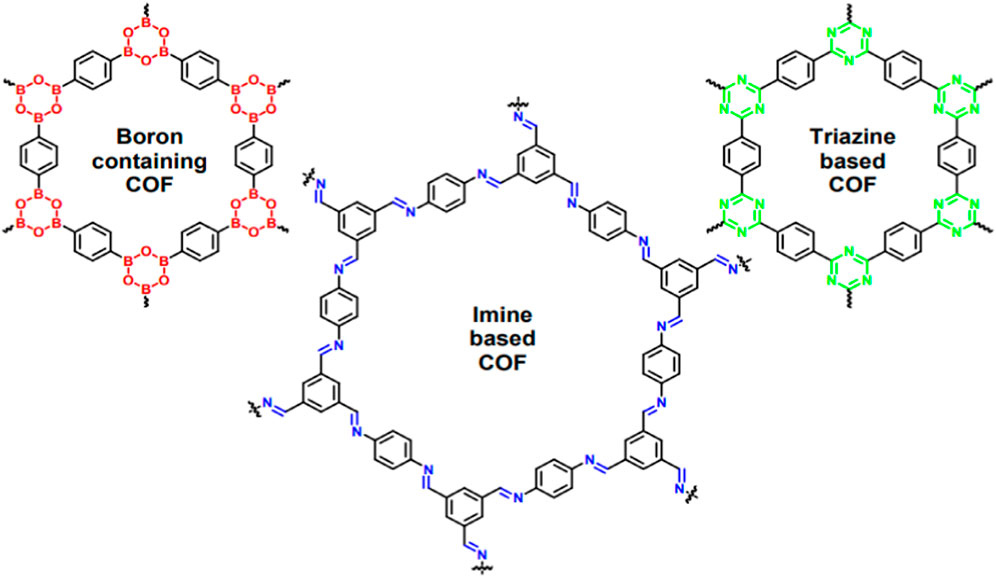
Figure 4. Molecular structure of different COFs (Bagheri and Aramesh, 2021).
Noteworthy advantages of COFs like inherent porosity, adjusted channel structure, low density, great stability and designable functionality have made them suitable for application in different scientific fields like CO2 separation, optoelectronics, drug delivery and adsorption (Wu and Yang, 2017; Altundal et al., 2020; Ghosh and Banerjee, 2023). In the case of membrane-based CO2 separation, porous COFs have been of paramount attentions owing to their brilliant ability for the storage and separation of major greenhouse gases (i.e., CO2), H2 and methane (Xia and Liu, 2016).
2.4 CMPs and CPPs
CMPs/CPPs refer to an important category of polymeric materials that mix extended π-conjugation with a microporous structure. Generally, CMPs/CPPs are known as microporous polymeric materials but can be accompanied by great amount of mesoporosity (Lee and Cooper, 2020). CMPs/CPPs possess great potential to be extensively used in disparate applications (i.e., separation processes, heterogeneous catalysis, energy storage and so on) owing to their brilliant properties like high porosity, tunable chemistry, appropriate chemical resistance, and thermal stability (Talapaneni et al., 2016; Wang et al., 2017; Liao et al., 2018; Cao et al., 2022). Although gas separation and storage is regarded as the most prevalent area of investigation for CMPs/CPPs, some drawbacks such as the application of expensive transition metals in their synthesis deteriorates their popularity in large-scale separation applications (Lee and Cooper, 2020). Figure 5 represents a schematic demonstration of the synthesis process of core−shell structured CMPs/CPPs. CMPs can be well identified as the most efficient POMs for the separation of CO2 greenhouse gas due to their noteworthy advantages including high surface area and tunable properties (Reddy et al., 2021; Zhang et al., 2023). The importance of CMPs is because of the necessary bond conjugation and amorphous morphology. Poly (arylene ethynylene)s were introduced for the first time in 2007 as the synthesized CMPs (Jiang et al., 2007).
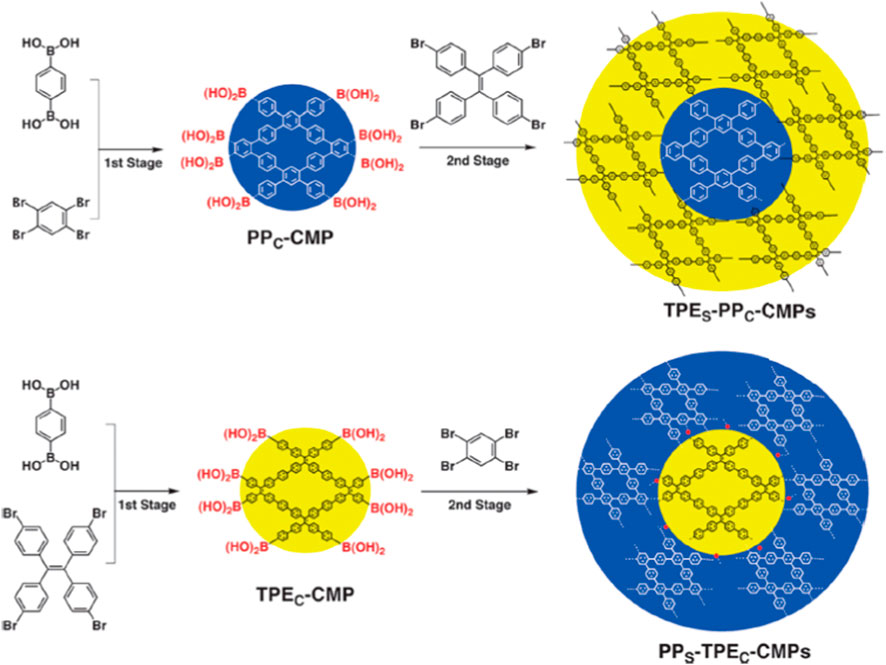
Figure 5. Synthesis process of core−shell structured CMPs. Reprinted from (Xu Y. et al., 2013) with permission from ACS Publications.
2.5 CTFs
CTFs can be defined as an important type of organic polymers fabricated by aromatic 1,3,5-triazine rings with planar π-conjugation properties (Wei et al., 2019; Liao et al., 2023). The occurrence of conjugation between aromatic rings and triazine rings significantly declines overall energy of π-conjugated molecules in the frameworks and therefore, significantly enhances the chemical stability. In the majority of cases, N-containing CTFs frameworks have shown their great potential in adsorption/separation and catalysis (Jadhav et al., 2019; Jiang et al., 2019). Most recently, CTFs have been appeared as a promising type of POMs, which is regarded as an adaptable platform for various applications due to their impressive characteristics such as permanent microporosity and appropriate thermal/mechanical stability and chemical resistance even in the strong acidic/basic environment.
The powerful covalent linkages, intrinsically great content of nitrogen atoms and excellent capability to add hetero-atoms in the structural skeleton have made CTFs versatile for numerous potential applications like gas separation and dye adsorption (Krishnaraj et al., 2020). Additionally, they are reproducible and recyclable, which permits them to be a noteworthy candidate in terms of sustainable materials. Figure 6 represents a schematic demonstration of photocatalytic CO2 separation from formic acid using CTFs.
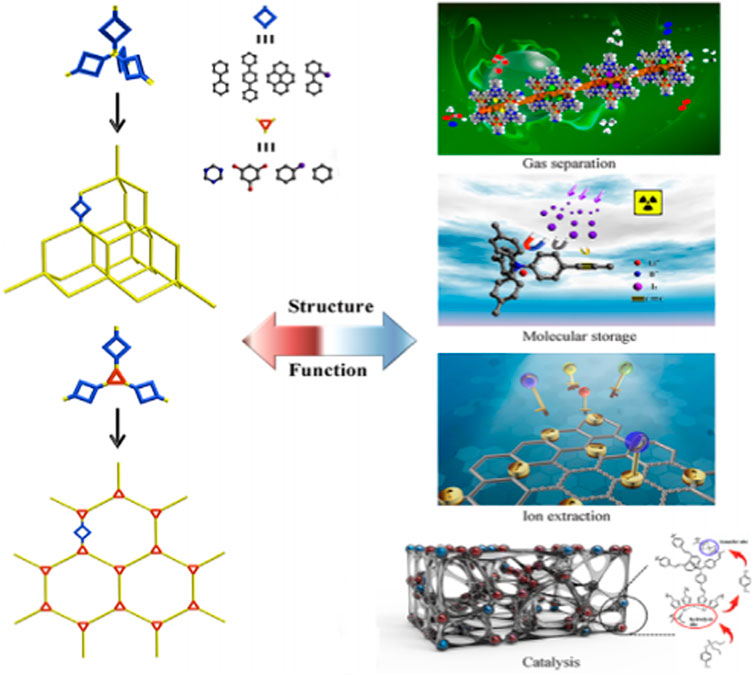
Figure 6. Schematic depiction of PAFs for various industrial applications (Yuan and Zhu, 2019).
2.6 Composite membranes
In the recent years, the industrial applications of membrane processes for the effective separation of CO2 pollutant owing to their great potential to overcome the negative disadvantages of conventional technologies like cryogenic distillation and desorption (Dai et al., 2023; Yang et al., 2022/03; Shirazian et al., 2020; Faraji et al., 2020). One of the most efficient membrane-based approaches for the separation of CO2 is the use of composite membranes. Composite membranes possess brilliant capacity to simultaneously combine the positive points of both inorganic and polymeric membranes, which make them a hotspot for scientific research (Pishnamazi et al., 2020/09). Composite membranes can be considered as mixed matrix membranes (MMMs), which are often used for the efficient separation of CO2. Due to the presence of disparate challenges toward the use of inorganic or polymeric membranes, composite membranes have been employed to solve the existed limitations of both aforementioned membranes. The industrial use of MMMs is being significantly enhanced due to their ability to combine the compactness of polymeric membranes and great permeability of inorganic membranes (Saqib et al., 2019).
2.7 PAFs
Figure 6 presents the schematic depiction of PAFs and their various applications in industry. PAFs are another category of POMs. PAFs, which are manufactured by carbon-carbon-bond-linked aromatic-based building units (CCBLABU), possess remarkable features such as rigid structures and great surface area. The presence of strong carbon−carbon linkage eventuates in enhancing the chemical resistance of this class of POMs in undesirable chemical environments (Tian and Zhu, 2020). Hence, PAFs illustrate excellent characteristics in chemistry and functionalities in comparison with traditional POMs like zeolites and MOFs. PAFs can be freely functionalized by severe chemical treatments (Tian and Zhu, 2020). Table 2 gives a comprehensive summarization about the characteristics of various POMs investigated in this review paper.
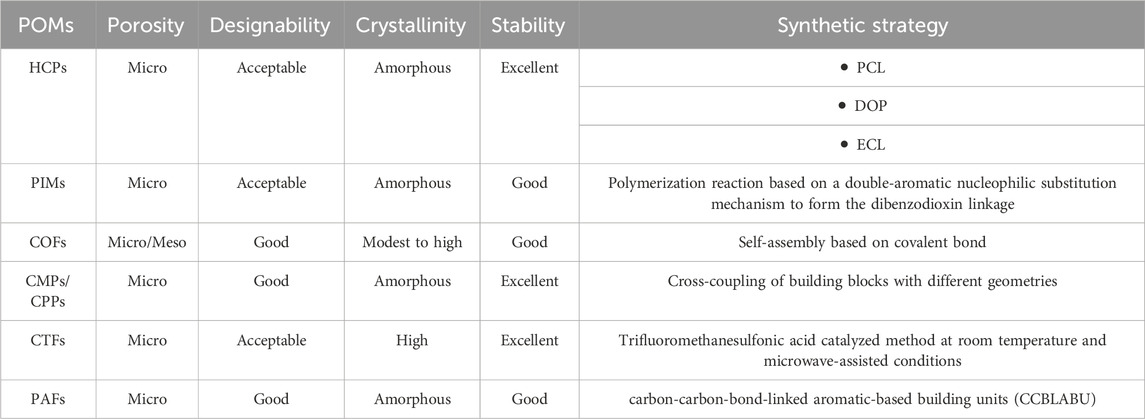
Table 2. Detailed summarization about the characteristics of various POMs (Bildirir et al., 2017; McKeown, 2017; Huang and Turner, 2018; Liu et al., 2019; Guan et al., 2020; Lee and Cooper, 2020; Sharma et al., 2020; Tian and Zhu, 2020).
3 POMs for CO2 separation: challenges and opportunities
Development of economical/novel technologies for CO2 separation from emission sources is considered as the most appropriate strategy to decrease the anthropogenic emissions of this acidic pollutant (Vesovic et al., 1990). POMs have been provided excellent capabilities for CO2 separation processes due to their brilliant privileges compared to porous inorganic materials (i.e., zeolite) or inorganic-organic hybrids (i.e., MOFs), such as appropriate stability and chemical robustness to acid and base (Zhang and Dai, 2017). In the recent 20 years, remarkable progressions have been made by the appearance of various POMs such as PIMs, CTFs, COFs, CMPs, HCPs and PAFs (Zhang et al., 2015). Generally, POM synthesis needs particular rigid monomers, which possesses acceptable resistance against the intermolecular packing, and consequently results in high porosity (Hao et al., 2015). POMs with different functionalities have been developed for better physico-chemical and CO2 separation properties. A summary of the more significant results is presented in Table 3.
Table 4 gives detailed information about the challenges towards the development of functionalized POMs-based membrane for CO2 separation.
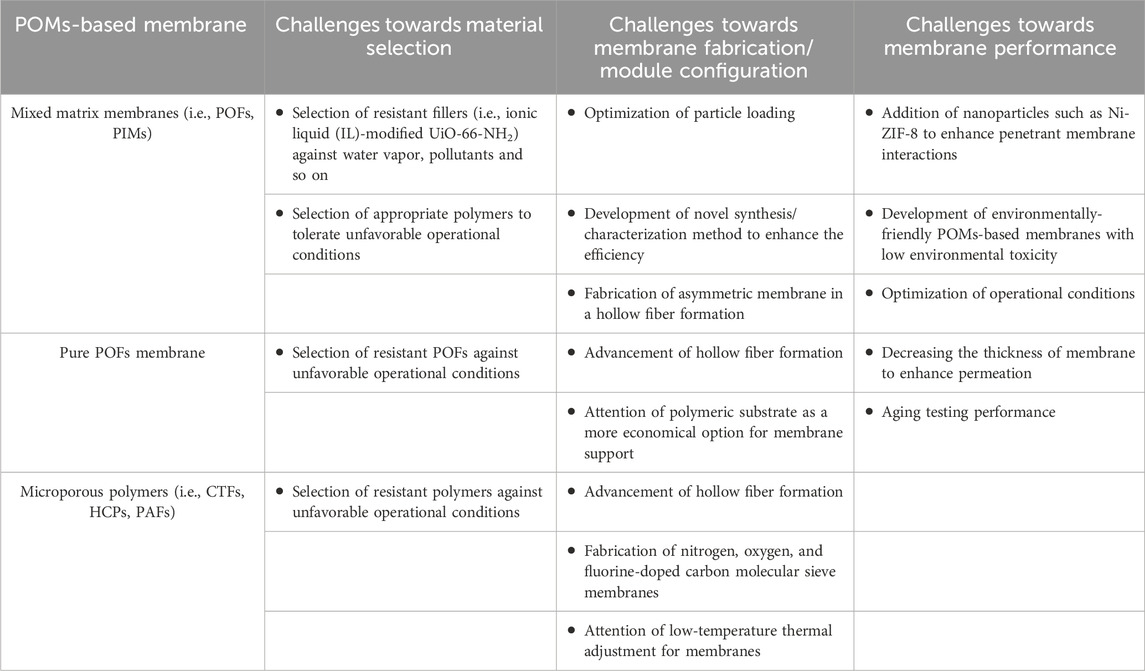
Table 4. Challenges towards the development of POMs-based membrane for CO2 separation (Krishnaraj et al., 2020; Prasetya et al., 2019; Johnson et al., 2008; Haq et al., 2021; Fouladivanda et al., 2021; Yang et al., 2020/03).
4 Conclusion and future directions
This article presents a review about the possibility of POMs application in CO2 separation processes. Critical investigation on the functionalization features of different classes of POMs including HCPs, PIMs, COFs, CMPs, CTFs, and PAFs is implemented and a detailed summarization about the characteristics of various POMs such as porosity, designability, crystallinity, stability, and synthetic strategy is presented to clarify the advantages and disadvantages of these materials in CO2 separation. Specially, reviewing the state-of-the-art applications of functionalized POMs in CO2 separation and the challenges and future directions towards the correct perception of POMs are presented to find the existing research gaps in this field. One of the prominent challenges towards the application of POMs is the perception of their promising efficiency in industrial-based CO2 separation processes. Several challenges are existed that their interpretation seems to be mandatory. For instance, fabrication of POMs-based membrane in hollow-fiber configuration may be a good choice to improve the efficacy and components interaction. Appropriate optimization of POMs-based membranes’ operational condition is regarded as another matter. Optimum operational conditions (i.e., pressure and temperature) must be precisely evaluated, especially for studying CO2-induced plasticization. Environmental consideration and fabrication of environmentally-friendly POMs-based membranes’ with the minimum detriments and toxicities is an important challenge towards the development of these types of polymeric membranes, which must be thoroughly investigated. Eventually, investigation of membrane aging must be in the priority of evaluation due to its significant impact on the long-term performance of POMs. More comprehensive investigation in the development of emerging POMs for MCS process is definitely required. The study not only should not be conducted in discovering new POMs but also must be towards optimizing the currently developed polymers due to their promising efficiency in CO2 separation. Furthermore, an extensive economic feasibility analysis is needed to be implemented to evaluate the possibility of POMs application in different industries. If the economic feasibility analysis justifies the use of POMs-based membranes in industries, this technology possesses the potential to replace the traditional methods contributing in efficacious CO2 separation processes.
Author contributions
YC: Writing–original draft, Data curation, Investigation. AN: Writing–original draft, Conceptualization, Formal Analysis. MG: Project administration, Resources, Supervision, Writing–review and editing.
Funding
The author(s) declare that no financial support was received for the research, authorship, and/or publication of this article.
Conflict of interest
The authors declare that the research was conducted in the absence of any commercial or financial relationships that could be construed as a potential conflict of interest.
Publisher’s note
All claims expressed in this article are solely those of the authors and do not necessarily represent those of their affiliated organizations, or those of the publisher, the editors and the reviewers. Any product that may be evaluated in this article, or claim that may be made by its manufacturer, is not guaranteed or endorsed by the publisher.
References
Agarwal, P., Hefner, R. E., Ge, S., Tomlinson, I., Rao, Y., and Dikic, T. (2020). Nanofiltration membranes from crosslinked troger's base polymers of intrinsic microporosity (PIMs). J. Membr. Sci. 595, 117501. doi:10.1016/j.memsci.2019.117501
Altundal, O. F., Altintas, C., and Keskin, S. (2020). Can COFs replace MOFs in flue gas separation? high-throughput computational screening of COFs for CO 2/N 2 separation. J. Mater. Chem. A 8, 14609–14623. doi:10.1039/d0ta04574h
Babanezhad, M., Behroyan, I., Nakhjiri, A. T., Marjani, A., and Shirazian, S. (2020). Computational modeling of transport in porous media using an adaptive network-based fuzzy inference system. ACS omega 5, 30826–30835. doi:10.1021/acsomega.0c04497
Bagheri, A. R., and Aramesh, N. (2021). Towards the room-temperature synthesis of covalent organic frameworks: a mini-review. J. Mater. Sci. 56, 1116–1132. doi:10.1007/s10853-020-05308-9
Baker, R. W., and Low, B. T. (2014). Gas separation membrane materials: a perspective. Macromolecules 47, 6999–7013. doi:10.1021/ma501488s
Ben, T., and Qiu, S. (2020). Porous aromatic frameworks for carbon dioxide capture. Mater. Carbon Capture, 97–115. doi:10.1002/9781119091219.ch4
Bildirir, H., Gregoriou, V. G., Avgeropoulos, A., Scherf, U., and Chochos, C. L. (2017). Porous organic polymers as emerging new materials for organic photovoltaic applications: current status and future challenges. Mater. Horizons 4, 546–556. doi:10.1039/c6mh00570e
Budd, P. M., Ghanem, B., Msayib, K., McKeown, N. B., and Tattershall, C. (2003). A nanoporous network polymer derived from hexaazatrinaphthylene with potential as an adsorbent and catalyst support. J. Mater. Chem. 13, 2721–2726. doi:10.1039/b303996j
Budd, P. M., and McKeown, N. B. (2010). Highly permeable polymers for gas separation membranes. Polym. Chem. 1, 63–68. doi:10.1039/b9py00319c
Bykov, A. V., Demidenko, G. N., Nikoshvili, L. Z., and Kiwi-Minsker, L. (2021). Hyper-cross-linked polystyrene as a stabilizing medium for small metal clusters. Molecules 26, 5294. doi:10.3390/molecules26175294
Cannone, S. F., Lanzini, A., and Santarelli, M. (2021). A review on CO2 capture technologies with focus on CO2-enhanced methane recovery from hydrates. Energies 14, 387. doi:10.3390/en14020387
Cao, Y., Khan, A., Nakhjiri, A. T., Albadarin, A. B., Kurniawan, T. A., and Rezakazemi, M. (2021/09/01/2021). Recent advancements in molecular separation of gases using microporous membrane systems: a comprehensive review on the applied liquid absorbents. J. Mol. Liq. 337, 116439. doi:10.1016/j.molliq.2021.116439
Cao, Y., Rehman, Z. U., Ghasem, N., Al-Marzouqi, M., Abdullatif, N., Nakhjiri, A. T., et al. (2021). Intensification of CO 2 absorption using MDEA-based nanofluid in a hollow fibre membrane contactor. Sci. Rep. 11, 2649–2712. doi:10.1038/s41598-021-82304-2
Cao, Y., Seyed Alizadeh, S. M., Fouladvand, M. T., Khan, A., Taghvaie Nakhjiri, A., Heidari, Z., et al. (2021/04/01/2021). Mathematical modeling and numerical simulation of CO2 capture using MDEA-based nanofluids in nanostructure membranes. Process Saf. Environ. Prot. 148, 1377–1385. doi:10.1016/j.psep.2021.03.007
Cao, Y., Taghvaie Nakhjiri, A., and Ghadiri, M. (2022). CFD investigation of CO2 separation from anesthesia gaseous stream applying novel cholinium lysinate amino acid-based ionic liquid inside the gas–liquid membrane contactor. Eur. Phys. J. Plus 137, 1–12. doi:10.1140/epjp/s13360-022-03261-x
Carnicer, J., Alegria, A., Giannakopoulos, C., Di Giuseppe, F., Karali, A., Koutsias, N., et al. (2022). Global warming is shifting the relationships between fire weather and realized fire-induced CO2 emissions in Europe. Sci. Rep. 12, 10365–10366. doi:10.1038/s41598-022-14480-8
Dai, Y., Niu, Z., Luo, W., Wang, Y., Mu, P., and Li, J. (2023). A review on the recent advances in composite membranes for CO2 capture processes. Sep. Purif. Technol. 307, 122752./02/15/2023. doi:10.1016/j.seppur.2022.122752
Das, S., Feng, J., and Wang, W. (2020). Covalent organic frameworks in separation. Annu. Rev. Chem. Biomol. Eng. 11, 131–153. doi:10.1146/annurev-chembioeng-112019-084830
Das, S., Heasman, P., Ben, T., and Qiu, S. (2017). Porous organic materials: strategic design and structure–function correlation. Chem. Rev. 117, 1515–1563. doi:10.1021/acs.chemrev.6b00439
Ding, S.-Y., and Wang, W. (2013). Covalent organic frameworks (COFs): from design to applications. Chem. Soc. Rev. 42, 548–568. doi:10.1039/c2cs35072f
Eastmond, G. C., Paprotny, J., Steiner, A., and Swanson, L. (2001). Synthesis of cyanodibenzo [1, 4] dioxines and their derivatives by cyano-activated fluoro displacement reactions. New J. Chem. 25, 379–384. doi:10.1039/b008508l
Faraji, D., Zabihi, S., Ghadiri, M., Sadighi, S., Nakhjiri, A. T., and Shirazian, S. (2020). Computational fluid dynamic modeling and simulation of hydrocracking of vacuum gas oil in a fixed-bed reactor. ACS omega 5, 16595–16601. doi:10.1021/acsomega.0c01394
Fouladivanda, M., Karimi-Sabet, J., Abbasi, F., and Moosavian, M. A. (2021). Step-by-step improvement of mixed-matrix nanofiber membrane with functionalized graphene oxide for desalination via air-gap membrane distillation. Sep. Purif. Technol. 256, 117809. doi:10.1016/j.seppur.2020.117809
Freeman, B. D. (1999). Basis of permeability/selectivity tradeoff relations in polymeric gas separation membranes. Macromolecules 32, 375–380. doi:10.1021/ma9814548
Germain, J., Fréchet, J. M., and Svec, F. (2007). Hypercrosslinked polyanilines with nanoporous structure and high surface area: potential adsorbents for hydrogen storage. J. Mater. Chem. 17, 4989–4997. doi:10.1039/b711509a
Ghadiri, M., Hemmati, A., Nakhjiri, A. T., and Shirazian, S. (2020). Modelling tyramine extraction from wastewater using a non-dispersive solvent extraction process. Environ. Sci. Pollut. Res. 27, 39068–39076. doi:10.1007/s11356-020-09943-2
Ghosh, P., and Banerjee, P. (2023). Drug delivery using biocompatible covalent organic frameworks (COFs) towards a therapeutic approach. Chem. Commun. 59, 12527–12547. doi:10.1039/d3cc01829f
Guais, A., Brand, G., Jacquot, L., Karrer, M., Dukan, S., Grévillot, G., et al. (2011). Toxicity of carbon dioxide: a review. Chem. Res. Toxicol. 24, 2061–2070. doi:10.1021/tx200220r
Guan, Q., Zhou, L. L., Li, W. Y., Li, Y. A., and Dong, Y. B. (2020). Covalent organic frameworks (COFs) for cancer therapeutics. Chemistry–A Eur. J. 26, 5583–5591. doi:10.1002/chem.201905150
Guo, Z., Wu, H., Chen, Y., Zhu, S., Jiang, H., Song, S., et al. (2022). Missing-linker defects in covalent organic framework membranes for efficient CO2 separation. Angew. Chem. 134, e202210466. doi:10.1002/ange.202210466
Hao, L., Ning, J., Luo, B., Wang, B., Zhang, Y., Tang, Z., et al. (2015). Structural evolution of 2D microporous covalent triazine-based framework toward the study of high-performance supercapacitors. J. Am. Chem. Soc. 137, 219–225. doi:10.1021/ja508693y
Haq, I. U., Wang, T., Zhang, A. S., Mao, H., Khan, R., Xu, L. H., et al. (2021). Fabrication of zeolitic imidazolate frameworks based mixed matrix membranes and mass transfer properties of C4H6 and N2 in membrane separation. AIChE J. 67, e17114. doi:10.1002/aic.17114
Huang, J., and Turner, S. R. (2018). Hypercrosslinked polymers: a review. Polym. Rev. 58, 1–41. doi:10.1080/15583724.2017.1344703
Huhe, F., King, J., and Chuang, S. S. (2023). Amine-based sorbents for CO2 capture from air and flue gas—a short review and perspective. Res. Chem. Intermed. 49, 791–817. doi:10.1007/s11164-022-04902-7
Jadhav, T., Fang, Y., Patterson, W., Liu, C. H., Hamzehpoor, E., and Perepichka, D. F. (2019). 2D poly (arylene vinylene) covalent organic frameworks via aldol condensation of trimethyltriazine. Angew. Chem. Int. Ed. 58, 13753–13757. doi:10.1002/anie.201906976
Jiang, J.-X., Su, F., Trewin, A., Wood, C. D., Campbell, N. L., Niu, H., et al. (2007). Conjugated microporous poly (aryleneethynylene) networks. Angew. Chem. 119, 8728–8732. doi:10.1002/ange.200701595
Jiang, S.-Y., Gan, S.-X., Zhang, X., Li, H., Qi, Q.-Y., Cui, F.-Z., et al. (2019). Aminal-linked covalent organic frameworks through condensation of secondary amine with aldehyde. J. Am. Chem. Soc. 141, 14981–14986. doi:10.1021/jacs.9b08017
Johnson, J., Reck, B., Wang, T., and Graedel, T. (2008). The energy benefit of stainless steel recycling. Energy Policy 36, 181–192. doi:10.1016/j.enpol.2007.08.028
Krishnaraj, C., Jena, H. S., Leus, K., and Van Der Voort, P. (2020). Covalent triazine frameworks–a sustainable perspective. Green Chem. 22, 1038–1071. doi:10.1039/c9gc03482j
Lee, J.-S. M., and Cooper, A. I. (2020). Advances in conjugated microporous polymers. Chem. Rev. 120, 2171–2214. doi:10.1021/acs.chemrev.9b00399
Lee, W. H., Seong, J. G., Hu, X., and Lee, Y. M. (2020). Recent progress in microporous polymers from thermally rearranged polymers and polymers of intrinsic microporosity for membrane gas separation: pushing performance limits and revisiting trade-off lines. J. Polym. Sci. 58, 2450–2466. doi:10.1002/pol.20200110
Li, X., Liu, C., and Van der Bruggen, B. (2020). Polyelectrolytes self-assembly: versatile membrane fabrication strategy. J. Mater. Chem. A 8, 20870–20896. doi:10.1039/d0ta07154d
Liao, C., Liang, Z., Liu, B., Chen, H., Wang, X., and Li, H. (2020). Phenylamino-Phenoxy-and benzenesulfenyl-linked covalent triazine frameworks for CO2 capture. ACS Appl. Nano Mater. 3, 2889–2898. doi:10.1021/acsanm.0c00155
Liao, L., Li, M., Yin, Y., Chen, J., Zhong, Q., Du, R., et al. (2023). Advances in the synthesis of covalent triazine frameworks. ACS omega 8, 4527–4542. doi:10.1021/acsomega.2c06961
Liao, Y., Wang, H., Zhu, M., and Thomas, A. (2018). Efficient supercapacitor energy storage using conjugated microporous polymer networks synthesized from Buchwald–Hartwig coupling. Adv. Mater. 30, 1705710. doi:10.1002/adma.201705710
Ling, H., Qazvini, O. T., Telfer, S. G., and Jin, J. (2020). Effective enhancement of selectivities and capacities for CO2 over CH4 and N2 of polymers of intrinsic microporosity via postsynthesis metalation. J. Polym. Sci. 58, 2619–2624. doi:10.1002/pol.20200104
Little, M. A., and Cooper, A. I. (2020). The chemistry of porous organic molecular materials. Adv. Funct. Mater. 30, 1909842. doi:10.1002/adfm.201909842
Liu, M., Guo, L., Jin, S., and Tan, B. (2019). Covalent triazine frameworks: synthesis and applications. J. Mater. Chem. A 7, 5153–5172. doi:10.1039/c8ta12442f
Liu, Y., Wu, H., Wu, S., Song, S., Guo, Z., Ren, Y., et al. (2021). Multifunctional covalent organic framework (COF)-Based mixed matrix membranes for enhanced CO2 separation. J. Membr. Sci. 618, 118693. doi:10.1016/j.memsci.2020.118693
Marjani, A., Nakhjiri, A. T., Pishnamazi, M., and Shirazian, S. (2021). Evaluation of potassium glycinate, potassium lysinate, potassium sarcosinate and potassium threonate solutions in CO2 capture using membranes. Arabian J. Chem. 14, 102979. doi:10.1016/j.arabjc.2020.102979
Masoumi, H., Ghaemi, A., and Gilani, H. G. (2020). Evaluation of hyper-cross-linked polymers performances in the removal of hazardous heavy metal ions: a Review. Sep. Purif. Technol. 260, 118221. doi:10.1016/j.seppur.2020.118221
Mazzetti, M. J., Skagestad, R., Mathisen, A., and Eldrup, N. H. (2014). CO2 from natural gas sweetening to kick-start EOR in the North Sea. Energy procedia, 63, 7280–7289. doi:10.1016/j.egypro.2014.11.764
McKeown, N. B. (2012). Polymers of intrinsic microporosity. Int. Sch. Res. Notices 2012, 1–16. doi:10.5402/2012/513986
McKeown, N. B. (2017). The synthesis of polymers of intrinsic microporosity (PIMs). Sci. China Chem. 60, 1023–1032. doi:10.1007/s11426-017-9058-x
McKeown, N. B. (2020). Polymers of intrinsic microporosity (PIMs). Polymer 202, 122736. doi:10.1016/j.polymer.2020.122736
Nakhjiri, A. T., Heydarinasab, A., Bakhtiari, O., and Mohammadi, T. (2018/11/01/2018). Experimental investigation and mathematical modeling of CO2 sequestration from CO2/CH4 gaseous mixture using MEA and TEA aqueous absorbents through polypropylene hollow fiber membrane contactor. J. Membr. Sci. 565, 1–13. doi:10.1016/j.memsci.2018.07.095
Nakhjiri, A. T., Heydarinasab, A., Bakhtiari, O., and Mohammadi, T. (2020). Numerical simulation of CO2/H2S simultaneous removal from natural gas using potassium carbonate aqueous solution in hollow fiber membrane contactor. J. Environ. Chem. Eng. 8, 104130. doi:10.1016/j.jece.2020.104130
Nakhjiri, A. T., and Roudsari, M. H. (2016). Modeling and simulation of natural convection heat transfer process in porous and non-porous media. Appl. Res. J. 2, 199–204.
Pachfule, P., Acharjya, A., Roeser, J., Langenhahn, T., Schwarze, M., Schomäcker, R., et al. (2018/01/31 2018). Diacetylene functionalized covalent organic framework (COF) for photocatalytic hydrogen generation. J. Am. Chem. Soc. 140, 1423–1427. doi:10.1021/jacs.7b11255
Perry, S. C., Gateman, S. M., Malpass-Evans, R., McKeown, N., Wegener, M., Nazarovs, P., et al. (2020). Polymers with intrinsic microporosity (PIMs) for targeted CO2 reduction to ethylene. Chemosphere 248, 125993. doi:10.1016/j.chemosphere.2020.125993
Pishnamazi, M., Babanezhad, M., Nakhjiri, A. T., Rezakazemi, M., Marjani, A., and Shirazian, S. (2020). ANFIS grid partition framework with difference between two sigmoidal membership functions structure for validation of nanofluid flow. Sci. Rep. 10, 15395. doi:10.1038/s41598-020-72182-5
Pishnamazi, M., Nakhjiri, A. T., Taleghani, A. S., Marjani, A., Heydarinasab, A., and Shirazian, S. (2020/09/15/2020). Computational investigation on the effect of [Bmim] [BF4] ionic liquid addition to MEA alkanolamine absorbent for enhancing CO2 mass transfer inside membranes. J. Mol. Liq. 314, 113635. doi:10.1016/j.molliq.2020.113635
Polak-Kraśna, K., Tian, M., Rochat, S. b., Gathercole, N., Yuan, C., Hao, Z., et al. (2021). Solvent sorption-induced actuation of composites based on a polymer of intrinsic microporosity. ACS Appl. Polym. Mater. 3, 920–928. doi:10.1021/acsapm.0c01215
Prasetya, N., Himma, N. F., Sutrisna, P. D., Wenten, I. G., and Ladewig, B. P. (2019). A review on emerging organic-containing microporous material membranes for carbon capture and separation. Chem. Eng. J. 391, 123575. doi:10.1016/j.cej.2019.123575
Ramezanipour Penchah, H., Ghanadzadeh Gilani, H., and Ghaemi, A. (2020). CO2, N2, and H2 adsorption by hyper-cross-linked polymers and their selectivity evaluation by gas–solid equilibrium. J. Chem. Eng. Data 65, 4905–4913. doi:10.1021/acs.jced.0c00541
Reddy, M. S. B., Ponnamma, D., Sadasivuni, K. K., Kumar, B., and Abdullah, A. M. (2021). Carbon dioxide adsorption based on porous materials. RSC Adv. 11, 12658–12681. doi:10.1039/d0ra10902a
Robeson, L. M. (2008). The upper bound revisited. J. Membr. Sci. 320, 390–400. doi:10.1016/j.memsci.2008.04.030
Rouquerol, J., Avnir, D., Fairbridge, C., Everett, D., Haynes, J., Pernicone, N., et al. (1994). Recommendations for the characterization of porous solids (Technical Report). Pure Appl. Chem. 66, 1739–1758. doi:10.1351/pac199466081739
Saqib, S., Rafiq, S., Chawla, M., Saeed, M., Muhammad, N., Khurram, S., et al. (2019). Facile CO2 separation in composite membranes. Chem. Eng. Technol. 42, 30–44. doi:10.1002/ceat.201700653
Sharma, R. K., Yadav, P., Yadav, M., Gupta, R., Rana, P., Srivastava, A., et al. (2020). Recent development of covalent organic frameworks (COFs): synthesis and catalytic (organic-electro-photo) applications. Mater. Horizons 7, 411–454. doi:10.1039/c9mh00856j
Shirazian, S., Taghvaie Nakhjiri, A., Heydarinasab, A., and Ghadiri, M. (2020). Theoretical investigations on the effect of absorbent type on carbon dioxide capture in hollow-fiber membrane contactors. PLoS One 15, e0236367. doi:10.1371/journal.pone.0236367
Stanovsky, P., Zitkova, A., Karaszova, M., Šyc, M., Jansen, J. C., Gándara, B. C., et al. (2020). Flue gas purification with membranes based on the polymer of intrinsic microporosity PIM-TMN-Trip. Sep. Purif. Technol. 242, 116814. doi:10.1016/j.seppur.2020.116814
Stein, T. (2022). Carbon dioxide now more than 50% higher than pre-industrial levels. can be found under Available At: https://www.noaa.gov/news-release/carbon-dioxide-now-more-than-50-higher-than-pre-industrial-levels.
Taghvaie Nakhjiri, A., Sanaeepur, H., Ebadi Amooghin, A., and Shirazi, M. M. A. (2022). Recovery of precious metals from industrial wastewater towards resource recovery and environmental sustainability: a critical review. Desalination 527, 115510. doi:10.1016/j.desal.2021.115510
Talapaneni, S. N., Kim, D., Barin, G., Buyukcakir, O., Je, S. H., and Coskun, A. (2016). Pillar [5] arene based conjugated microporous polymers for propane/methane separation through host–guest complexation. Chem. Mater. 28, 4460–4466. doi:10.1021/acs.chemmater.6b01667
Tan, L., and Tan, B. (2017). Hypercrosslinked porous polymer materials: design, synthesis, and applications. Chem. Soc. Rev. 46, 3322–3356. doi:10.1039/c6cs00851h
Tian, Y., and Zhu, G. (2020). Porous aromatic frameworks (PAFs). Chem. Rev. 120, 8934–8986. doi:10.1021/acs.chemrev.9b00687
Vesovic, V., Wakeham, W., Olchowy, G., Sengers, J., Watson, J., and Millat, J. (1990). The transport properties of carbon dioxide. J. Phys. Chem. reference data 19, 763–808. doi:10.1063/1.555875
Wang, C.-A., Li, Y.-W., Cheng, X.-L., Zhang, J.-P., and Han, Y.-F. (2017). Eosin Y dye-based porous organic polymers for highly efficient heterogeneous photocatalytic dehydrogenative coupling reaction. RSC Adv. 7, 408–414. doi:10.1039/c6ra25123d
Wang, L., Zhang, Y.-s., Jiang, H.-r., and Wang, H. (2020). Carbonyl-incorporated aromatic hyper-cross-linked polymers with microporous structure and their functional materials for CO2 adsorption. Industrial Eng. Chem. Res. 59, 15955–15966. doi:10.1021/acs.iecr.0c02165
Wang, S., Liu, Y., Yu, Y., Du, J., Cui, Y., Song, X., et al. (2018). Conjugated microporous polymers based on biphenylene for CO 2 adsorption and luminescence detection of nitroaromatic compounds. New J. Chem. 42, 9482–9487. doi:10.1039/c8nj01306c
Wei, S., Zhang, F., Zhang, W., Qiang, P., Yu, K., Fu, X., et al. (2019). Semiconducting 2D triazine-cored covalent organic frameworks with unsubstituted olefin linkages. J. Am. Chem. Soc. 141, 14272–14279. doi:10.1021/jacs.9b06219
Wood, C. D., Tan, B., Trewin, A., Su, F., Rosseinsky, M. J., Bradshaw, D., et al. (2008). Microporous organic polymers for methane storage. Adv. Mater. 20, 1916–1921. doi:10.1002/adma.200702397
Wu, M.-X., and Yang, Y.-W. (2017). Applications of covalent organic frameworks (COFs): from gas storage and separation to drug delivery. Chin. Chem. Lett. 28, 1135–1143. doi:10.1016/j.cclet.2017.03.026
Xia, L., and Liu, Q. (2016). Lithium doping on covalent organic framework-320 for enhancing hydrogen storage at ambient temperature. J. Solid State Chem. 244, 1–5. doi:10.1016/j.jssc.2016.09.007
Xu, S., Luo, Y., and Tan, B. (2013a). Recent development of hypercrosslinked microporous organic polymers. Macromol. rapid Commun. 34, 471–484. doi:10.1002/marc.201200788
Xu, Y., Jin, S., Xu, H., Nagai, A., and Jiang, D. (2013b). Conjugated microporous polymers: design, synthesis and application. Chem. Soc. Rev. 42, 8012–8031. doi:10.1039/c3cs60160a
Yang, S., Abdalkareem Jasim, S., Bokov, D., Chupradit, S., Nakhjiri, A. T., and El-Shafay, A. S. (2022/03/01/2022). Membrane distillation technology for molecular separation: a review on the fouling, wetting and transport phenomena. J. Mol. Liq. 349, 118115. doi:10.1016/j.molliq.2021.118115
Yang, Z., Guo, W., Mahurin, S. M., Wang, S., Chen, H., Cheng, L., et al. (2020/03/12/2020). Surpassing Robeson upper limit for CO2/N2 separation with fluorinated carbon molecular sieve membranes. Chem 6, 631–645. doi:10.1016/j.chempr.2019.12.006
Yang, Z., Li, X., Xu, F., Wang, W., Shi, Y., Zhang, Z., et al. (2020). Synthesis of bio-based polycarbonate via one-step melt polycondensation of isosorbide and dimethyl carbonate by dual site-functionalized ionic liquid catalysts. Green Chem. 23, 447–456. doi:10.1039/d0gc03247f
Yuan, Y., and Zhu, G. (2019). Porous aromatic frameworks as a platform for multifunctional applications. ACS central Sci. 5, 409–418. doi:10.1021/acscentsci.9b00047
Zhang, P., and Dai, S. (2017). Mechanochemical synthesis of porous organic materials. J. Mater. Chem. A 5, 16118–16127. doi:10.1039/c7ta04829g
Zhang, P., Li, M., Yang, B., Fang, Y., Jiang, X., Veith, G. M., et al. (2015). Polymerized ionic networks with high charge density: quasi-solid electrolytes in lithium-metal batteries. Adv. Mater 27, 8088–8094. doi:10.1002/adma.201502855
Zhang, Y., Sunarso, J., Liu, S., and Wang, R. (2013). Current status and development of membranes for CO2/CH4 separation: a review. Int. J. Greenh. Gas Control 12, 84–107. doi:10.1016/j.ijggc.2012.10.009
Zhang, Y., Tian, F., Guo, X., Bai, M., Tang, T., Di, X., et al. (2023). Sustainable porous carbons from tannic acid based KOH activated as high-performance CO 2 capture sorbents. Sustain. Energy and Fuels 7, 2063–2073. doi:10.1039/d3se00220a
Zhou, S., Sun, Y., Xue, B., Li, S., Zheng, J., and Zhang, S. (2020). Controlled superacid-catalyzed self-cross-linked polymer of intrinsic microporosity for high-performance CO2 separation. Macromolecules 53, 7988–7996. doi:10.1021/acs.macromol.0c01590
Zou, X., Ren, H., and Zhu, G. (2013). Topology-directed design of porous organic frameworks and their advanced applications. Chem. Commun. 49, 3925–3936. doi:10.1039/c3cc00039g
Keywords: porous organic materials (POMs), functionalization, CO2 separation, membrane, chemical characterization
Citation: Cao Y, Taghvaie Nakhjiri A and Ghadiri M (2024) Breakthrough applications of porous organic materials for membrane-based CO2 separation: a review. Front. Chem. 12:1381898. doi: 10.3389/fchem.2024.1381898
Received: 04 February 2024; Accepted: 11 March 2024;
Published: 21 March 2024.
Edited by:
Shahid Ul Islam, Jamia Millia Islamia, IndiaReviewed by:
Kun Zhao, University of Washington, United StatesJikuan Qiu, Henan Normal University, China
Copyright © 2024 Cao, Taghvaie Nakhjiri and Ghadiri. This is an open-access article distributed under the terms of the Creative Commons Attribution License (CC BY). The use, distribution or reproduction in other forums is permitted, provided the original author(s) and the copyright owner(s) are credited and that the original publication in this journal is cited, in accordance with accepted academic practice. No use, distribution or reproduction is permitted which does not comply with these terms.
*Correspondence: Ali Taghvaie Nakhjiri, taghvaiali@yahoo.com