Using ecosystem integrity to maximize climate mitigation and minimize risk in international forest policy
- 1Woodwell Climate Research Center, Falmouth, MA, United States
- 2Climate Action Beacon, Griffith University, Gold Coast, QLD, Australia
- 3Department of Crop and Forest Sciences, University of Lleida, Lleida, Spain
- 4International Forests and Climate Programme, Australian Rainforest Conservation Society, Milton, QLD, Australia
- 5Wild Heritage, Berkeley, CA, United States
- 6Global Development and Environment Institute, Fletcher School of Law and Diplomacy, Tufts University, Medford, MA, United States
Several key international policy frameworks involve forests, including the Paris Agreement on Climate Change and the Convention on Biological Diversity (CBD). However, rules and guidelines that treat forest types equally regardless of their ecosystem integrity and risk profiles in terms of forest and carbon loss limit policy effectiveness and can facilitate forest degradation. Here we assess the potential for using a framework of ecosystem integrity to guide policy goals. We review the theory and present a conceptual framework, compare elements of integrity between primary and human-modified forests, and discuss the policy and management implications. We find that primary forests consistently have higher levels of ecosystem integrity and lower risk profiles than human-modified forests. This underscores the need to protect primary forests, develop consistent large-scale data products to identify high-integrity forests, and operationalize a framework of ecosystem integrity. Doing so will optimize long-term carbon storage and the provision of other ecosystem services, and can help guide evolving forest policy at the nexus of the biodiversity and climate crises.
Introduction
Forest ecosystems are central to international agreements and frameworks that support and set policy agendas, including the United Nations (UN) Framework Convention on Climate Change (UNFCCC), Convention on Biological Diversity (CBD), Sustainable Development Goals (SDGs), and Convention to Combat Desertification (UNCCD). Forests and their ecosystem services provide critical data to inform global environmental assessments such as the Global Forest Resource Assessments (FRAs) of the UN Food and Agriculture Organization (FAO), the Intergovernmental Science-Policy Platform on Biodiversity and Ecosystem Services (IPBES), the System of Environmental Economic Accounting–Ecosystem Accounting (SEEA-EA), and the World Bank’s reports on the Changing Wealth of Nations (Lange et al., 2018). The mitigation significance of forests is recognized in Article 5 of the Paris Agreement. Given their mitigation value, updating forest management practices to reduce emissions and increase withdrawals from the atmosphere should be included in many countries’ Nationally Determined Contributions (NDCs; Forsell et al., 2016; Grassi et al., 2017; Roe et al., 2019). Forestry practices have the potential to provide a majority fraction of the Agriculture, Forestry, and Other Land Use (AFOLU) sector’s contributions to climate mitigation, which may represent up to one-third of net emission reductions needed to limit warming below 1.5–2°C above pre-industrial levels (Federici et al., 2017; Grassi et al., 2017; Griscom et al., 2017; Roe et al., 2019). The current emissions gap between NDCs and what is required to limit warming to 1.5 or 2°C (UNEP, 2019) means that the role of forests may be even greater; for example, forests are referenced heavily in the Intergovernmental Panel on Climate Change (IPCC) special report on 1.5°C in the context of negative emissions (Dooley et al., 2018; IPCC, 2018).
However, given the finite area of available land and the many ecosystem services they provide, there are often conflicting goals for the management of forests in national and international policy contexts, resulting in incoherent policies and policy objectives (Kalaba et al., 2014; Koff et al., 2016; Tegegne et al., 2018; Timko et al., 2018). For example, many of the UN SDGs focused on promoting economic development are at odds with conserving forests and biodiversity (Ibisch et al., 2016). Unclear and inconsistent definitions and accounting rules mean that forest mitigation measures can have a range of results from large-scale protection that preserves carbon storage, sequestration, and ecosystem services, to perverse outcomes with net carbon loss, degraded ecosystems, and negative impacts on other policy goals (Mackey et al., 2013). For example, bioenergy with carbon capture and storage (BECCS) is used in the majority of current global socioeconomic model scenarios to stay below 1.5–2°C of warming (Roe et al., 2019). At these scales, BECCS will require the conversion of vast quantities of native forests into tree plantations or short-rotation forests (Fuss et al., 2014; Creutzig et al., 2015; Smith et al., 2016; IPCC, 2018). Increased bioenergy use is currently resulting in forest degradation and deforestation that will generate net carbon emissions for decades or longer (Birdsey et al., 2018; Booth, 2018; Sterman et al., 2022). Part of the problem is that forest cover and types are largely seen as fungible within the UNFCCC guidelines (UNFCCC, 2002), with no criteria for forest condition or carbon longevity (Ajani et al., 2013; Hansen A. J. et al., 2020; Keith et al., 2021).
From a carbon perspective, “risk of loss” of the stock is of central importance. The risk of loss from disturbances means that some land-based carbon activities will not provide long-term protection of carbon from release into the atmosphere (e.g., Anderegg et al., 2020). This risk is a primary reason that forest-based solutions are often not considered as reliable ways to reduce net emissions and hence are not prioritized as mitigation activities (Grassi et al., 2017). Yet little consideration has been given to differentiating forest types and management schemes based on their “risk of loss” profiles. The Paris Agreement mentions criteria for mitigation that speak to risk, such as equity, sustainability, and integrity, but as of yet there is little guidance on implementation.
The concept of “ecosystem integrity,” or related “ecological integrity,” has a long history in theoretical and applied ecology (e.g., Kay, 1991; Tierney et al., 2009; Wurtzebach and Schultz, 2016) and is explicitly referenced [e.g., Paris Agreement, CBD post-2020 Global Biodiversity Framework (Convention on Biological Diversity [CBD], 2021), IPCC Working Group II (IPCC, 2022)] or implied in international agreements and national-level legislation and agency directives (e.g., Australian Government, 1999). By providing a holistic view of ecosystem structure, function, composition, and adaptive capacity, the objective of maximizing ecosystem integrity may have the potential to minimize risk of carbon loss and maximize the ecosystem services provided by forests, thereby facilitating greater policy coherence across sectors (Koff et al., 2016; Dooley et al., 2018; Barber et al., 2020). However, the concept is not prioritized in international policy nor operationalized in most national forest policies, thus falling well short of its potential. There are no specific actions or supporting mechanisms for ecosystem integrity in the Paris Agreement, and parties have not articulated how they will identify and protect high-integrity ecosystems. Instead of representing a guiding framework, ecosystem integrity is largely viewed as a potential co-benefit (Bryan et al., 2016; Funk et al., 2019). Particularly important is providing a definition and framework for ecosystem integrity that the CBD (though the Global Biodiversity Framework) and the UNFCCC (through the Global Stocktake) can utilize to achieve their biodiversity and climate mitigation objectives.
Here we review the potential for a framework of ecosystem integrity to minimize risk in forest-based mitigation policies and maximize ecosystem service co-benefits. We first discuss the theory of ecosystem integrity and provide a working conceptual framework. We then compare important elements of ecosystem integrity between primary and human-modified forests, with a focus on elements most relevant for carbon mitigation including risk profiles. Finally, we discuss the policy and management implications of this comparative analysis. By drawing on ecological theory and several sub-disciplines within ecology, we integrate knowledge into a coherent framework of ecosystem integrity (Figure 1) that can be used to guide both forest policy at the international level as well as implementation in the form of land use decisions, metrics, and priorities at the national and jurisdictional levels. Our review draws upon decades of evolving forest policy and published literature, including but not limited to peer-reviewed articles, as well as engagement with stakeholders, practitioners, policy makers, and forest ecologists.
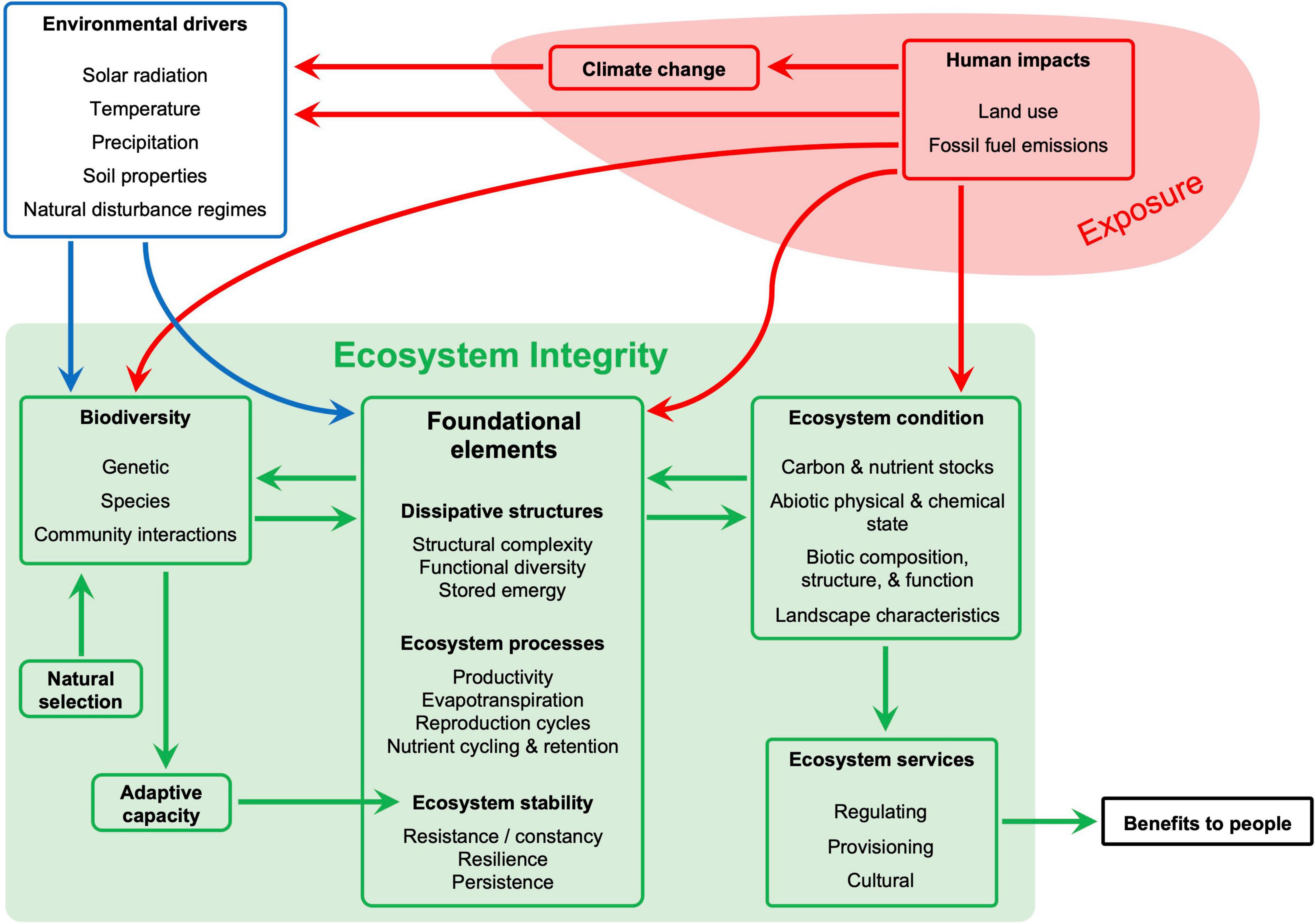
Figure 1. Conceptual framework of ecosystem integrity. Integrity is based on foundational elements including dissipative structures, ecosystem processes, and ecosystem stability. These are underpinned by biodiversity, natural selection, and adaptive capacity, and in turn generate a given ecosystem condition and benefits to people. Ecosystem integrity is impacted by environmental drivers and human impacts, including land use and climate change.
Framework for forest ecosystem integrity
Definition
Many definitions of ecosystem integrity exist because ecosystem integrity is not a simple absolute physical property but rather a multidimensional and scale-dependent emergent phenomenon that encompasses important system components and their interactions. The concept has received considerable attention over the past several decades because of the human benefits derived from natural processes and ecosystem states. As noted by Muller et al. (2000), “ecosystem integrity turns out to be the ecological branch of sustainability.”
Here we adopt and build upon the general framework originally provided by Kay (1991), whereby ecosystem integrity integrates different characteristics of an ecosystem that collectively describe its ability to achieve and maintain its optimum operating state, given the prevailing environmental drivers and perturbations, and continue its processes of self-organization and regeneration (i.e., autopoiesis). One of the main theoretical divides about ecosystem integrity relates to differentiating compositional (e.g., species richness, genetic diversity, or presence of threatened species), structural (e.g., vegetation density, biomass, food chains, and trophic levels) or functional (e.g., productivity, energy flows, and nutrient cycling) aspects of integrity (De Leo and Levin, 1997; Pimentel et al., 2013; Roche and Campagne, 2017). We suggest these are largely inseparable given the fundamental importance of structural and compositional elements in supporting functional forest ecosystem integrity and the many interdependencies among composition, structure, and function. In practice, available data and resources will determine what can be measured at a particular spatial and temporal scale. Because ecosystem integrity includes the provision of ecosystem services for human benefit, its evaluation typically includes a human dimension (Kay, 1991; De Leo and Levin, 1997; Kay and Regier, 2000; Dorren et al., 2004; Roche and Campagne, 2017).
Components of ecosystem integrity
Based on decades of theoretical and applied studies, we provide a framework for understanding the components of forest ecosystem integrity, their drivers, and their inter-linkages (Figure 1). It is important to note that all elements of ecosystem integrity are affected by the prevailing environmental and site characteristics of a given forested location, which must be accounted for when comparing specific locations in space and/or time.
Foundational elements
Forest ecosystem integrity is based on physiological structures that efficiently use and dissipate energy (Figure 1). These dissipative structures, or “ecological orientors” (Muller et al., 2000), generate a gradient of energy degradation via metabolic reactions that create and maintain themselves (i.e., self-organization). Progressively accumulated exergy (i.e., available energy) becomes stored emergy (i.e., all the energy used to generate a product or service) (Campbell, 2000; Kay and Regier, 2000; Muller et al., 2000). Over the course of evolution, community assembly, and forest succession, this process generates optimized (generally high but not too high; Hengeveld, 1989; May, 2001) ecosystem complexity and distance from thermodynamic equilibrium (Odum, 1969; Kay, 1991; Holling, 1992; Campbell, 2000; Muller et al., 2000), with associated levels of structural complexity, functional diversity, and niche complementarity (Tilman, 1996; Tilman and Lehman, 2001; Thompson et al., 2009). Ecosystem processes that sustain and regulate this self-organizing system, such as productivity, evapotranspiration, reproduction cycles, and nutrient cycling and retention, are optimized in the process (Muller et al., 2000; Dorren et al., 2004; Migliavacca et al., 2021). The resulting forest is a non-linear, self-organizing, holarchic and open system, with reciprocal power relationships between levels (Kay and Regier, 2000).
A critical property of ecosystem integrity that is difficult to assess from structural or compositional elements alone is stability. Following Grimm and Wissel (1997), stability is comprised of resistance (or constancy), resilience, and persistence, which collectively represent an ecosystem’s ability to resist or be resilient to change at both short and long time scales (Kay, 1991, 1993; Regier, 1993; Muller, 1998; Kay and Regier, 2000; Andreasen et al., 2001; Parrish et al., 2003). In the case of forest ecosystem integrity, primary drivers of change (exposure) include human land use and other human pressures, and climate change including extreme weather events and increasing disturbances. Resistance indicates a forest’s ability to maintain stability via dynamic equilibrium within defined ecosystem bounds (Hughes et al., 2002; Loreau et al., 2002) in response to these drivers. Forest resistance is conferred by negative feedbacks and buffers, for example stable microhabitats in forest interiors and functional redundancy across species. Resilience indicates the ability to return to optimal operating conditions after a state-altering perturbation (Holling, 1973; Kay, 1991; Kay and Regier, 2000; Muller et al., 2000; Thompson et al., 2009). The resulting ecosystem state can be somewhat altered (i.e., “ecological resilience” as opposed to “engineering resilience”), but when viewed over an appropriate time span, a resilient forest is able to maintain its “identity” in terms of taxonomic composition, structure, ecological functions, and process rates–and hence exhibit persistence (Thompson et al., 2009). Forest resilience is generally conferred by regenerative capacity via biological legacies (Franklin et al., 2000; Lindenmayer et al., 2019). These components of stability are supported by an ecosystem’s adaptive capacity, or the capacity for adaptive change in response to new conditions (Angeler et al., 2019). For example, genetic diversity, species diversity, and phenotypic plasticity allow for varied and time-evolving expression of adaptive traits and species within an ecosystem in response to changing environmental conditions, disturbances, or other pressures (Savolainen et al., 2007; Reed et al., 2011; Rogers et al., 2017). Hence, adaptive capacity is supported by biodiversity (Figure 1).
Biodiversity
These foundational elements of integrity are derivatives of the underlying biodiversity of a forest ecosystem, including diversity at the genetic, species, and community levels (Figure 1). A wealth of literature provides evidence that biodiversity supports net primary productivity (Chapin et al., 1997; Diaz and Cabido, 2001; Hooper et al., 2005; Thompson et al., 2009; Tilman et al., 2014; Liang et al., 2016; Duffy et al., 2017; de Souza et al., 2019; Matos et al., 2020), adaptation (Steffen et al., 2015; King et al., 2019), resistance (Pimm, 1984; Walker, 1995; Ives et al., 1999; Lehman and Tilman, 2000; McCann, 2000; Loreau et al., 2002; Dorren et al., 2004; Hooper et al., 2005; Thompson et al., 2009; Hautier et al., 2015), resilience (Peterson et al., 1998; Loreau et al., 2001; Hooper et al., 2005; Drever et al., 2006; Thompson et al., 2009; Ajani et al., 2013; Oliver et al., 2015; King et al., 2019), functional diversity (Cadotte et al., 2011; Levin, 2013; Karadimou et al., 2016), and overall ecosystem functioning (e.g., Lawton, 1997; Tilman, 1997; Hooper et al., 2005; Cardinale et al., 2012; Watson et al., 2018; King et al., 2019). These relationships exist because natural selection yields the characteristic biodiversity and phenotypic plasticity best suited to prevailing environmental conditions, including fluctuating resource inputs, extreme events, periods of stress, and natural disturbances. Specific mechanisms include biotic control of grazing, population density, and nutrient cycling; niche selection and complementarity; biotic and abiotic facilitation; and functional redundancy (i.e., the “insurance hypothesis”) (e.g., Naeem et al., 1995; Tilman, 1996; Tilman et al., 1997; Yachi and Loreau, 1999; Loreau, 2000; Tilman and Lehman, 2001; Pretzsch, 2005; Scherer-Lorenzen and Schulze, 2005; Jactel and Brockerhoff, 2007; Thompson et al., 2009; Hantsch et al., 2014; Wright et al., 2017; Liu et al., 2018).
Ecosystem condition
The foundational elements of ecosystem integrity form the basis for assessing ecosystem condition (Keith et al., 2020), specifically in the context of the System of Environmental-Economic Accounting (Committee of Experts on Environmental-Economic Accounting, 2021). Ecosystem condition is defined as “the quality of an ecosystem that may reflect multiple values, measured in terms of its abiotic and biotic characteristics across a range of temporal and spatial scales” (Keith et al., 2020). Ecosystem condition is measured in terms of variables that reflect the state, processes, and changes in the ecosystem, including (i) carbon and nutrient stocks, (ii) abiotic physical and chemical states such as water quantity and quality; (iii) biotic composition, structure, and function; and (iv) landscape diversity and connectivity. Indicators of condition are derived when variables are transformed by assessment against a reference condition. For a given biome and prevailing environmental conditions, these state variables are optimized by the foundational elements of ecosystem integrity and biodiversity (Phillips et al., 1994; Thompson et al., 2009; Roche and Campagne, 2017; Di Marco et al., 2018; Liu et al., 2018).
Ecosystem services
Characteristics of ecosystem condition that relate to the supply of ecosystem services represent an instrumental anthropocentric dimension. Specific ecosystem services can be linked to characteristics of ecosystem condition, and condition indicators can be associated with multiple services (Keith et al., 2020). Ecosystem services can be broadly categorized as regulating, provisioning, and cultural services (Millennium Ecosystem Assessment, 2005; Kandziora et al., 2013; IPBES, 2019; Committee of Experts on Environmental-Economic Accounting, 2021). Regulating services include clean and regulated water flow, air quality, pest and pathogen containment, erosion control, nutrient regulation, resistance and resilience to natural hazards, waste regulation, carbon sequestration and storage, and climate regulation from local to global scales. Provisioning services include the animals, plants, and minerals used for food, medicine, energy, and infrastructure. Cultural services include customary values, ecotourism and nature-based recreation, scientific research, and education.
The concept of ecosystem integrity is useful because it integrates across many properties of forest ecosystems, and thereby optimizes values useful to humans and other organisms. In the words of Koff et al. (2016), “ecosystem integrity is a scientific paradigm that fits the political needs of the present global development agenda focused on complex human-environmental interactions.” The concept is holistic and can be adapted to local, national, or international contexts. At jurisdictional levels, the related concepts of “ecological integrity” and “biological integrity” have been used operationally to provide benchmarks for natural resource management (Karr, 1996; Harwell et al., 1999; Campbell, 2000; Muller et al., 2000; Parrish et al., 2003; Tierney et al., 2009; Wurtzebach and Schultz, 2016; Roche and Campagne, 2017). However, as noted above, the international policy community has yet to implement these terms. This is important because ecosystem integrity may be directly linked to forest and carbon risk profiles that, if understood and prioritized, could greatly aid our ability to utilize forests for mitigation and adaptation.
Comparison of ecosystem integrity between forest types
Here, we compare components of ecosystem integrity most relevant for international policy across commonly recognized broad categories of forest types, focusing on primary forests and forests with significant levels of human modification and pressure. We focus on components of ecosystem integrity most pertinent to forest-based climate mitigation, including forest risk profiles as governed by exposure and stability as well as carbon stocks and fluxes. As noted previously, direct comparisons between forest types must account for environmental and site drivers, including the prevailing biome (e.g., tropical, temperate, or boreal) and heterogeneity within as determined by climate, soils, hydrology, and natural disturbance regimes.
Following Kormos et al. (2018), Food and Agriculture Organization of the United Nations [FAO] (2020), and IUCN (2020), primary forests are defined as: (i) largely undisturbed by industrial-scale land uses such as logging, mining, hydroelectric development, and road construction; (ii) established and regenerated by natural biological, ecological, and evolutionary processes; (iii) including the full range of successional stages at a landscape level from pioneer, secondary growth, and old-growth forest stands; and (iv) with the vegetation structure, community networks, and taxonomic composition principally reflecting natural processes including natural disturbance regimes. Primary forests can therefore be distinguished from naturally regenerating forests that are subject to conventional forestry management for commodity production (Puettmann et al., 2015), as well as planted forests, including plantations. For our purposes, primary forest therefore encompasses a range of commonly recognized forest descriptors including intact, virgin, ecologically mature, and old growth forests (Buchwald, 2005; Mackey et al., 2013; DellaSala et al., 2022b).
Foundational elements of ecosystem integrity
Comparison of dissipative structures
In this section we focus on structural complexity because of its importance for carbon stocks. Other components of dissipative structures (Figure 1) will be highlighted for their role in supporting ecosystem integrity in following sections (including functional diversity as it relates to biodiversity in the section “Biodiversity,” and stored emergy as manifested in biomass and carbon stocks in section “Ecosystem condition”). High-integrity forests that have been allowed time to respond to their emergy signature develop a set of relatively complex ecosystem structures (Campbell, 2000). Canopy structure is particularly influential for other elements of ecosystem integrity such as microclimate, runoff, nutrient cycling, and biodiversity (Hobbie, 1992; Parker, 1995; Didham and Lawton, 1999; Siitonen, 2001; Asner et al., 2010; Goetz et al., 2010; Hansen et al., 2014). Primary tropical forests in particular develop tall, multi-story dense canopies with large variations in plant size and emergent canopy dominants (Kricher, 2011; Hansen A. J. et al., 2020). Temperate forests also develop complex forest canopies as they age, which is associated with high levels of biodiversity and carbon storage (DellaSala et al., 2022b).
Canopy height, in turn, is positively related to aboveground biomass and carbon storage. For example, in Brazil, Democratic Republic of the Congo, and Indonesia, primary forests were 38–59% taller and contained 70–148% more aboveground biomass than other dense tree cover types, including degraded forests, secondary regrowth, and tree plantations (Turubanova et al., 2018). When felling the largest trees or clear-cutting entire stands, logging decreases canopy height, homogenizes forest canopies, and reduces structural complexity (Pfeifer et al., 2016; Rappaport et al., 2018; Bourgoin et al., 2020), which can take centuries to recover. Structural complexity also relates to non-living forest structures, such as dead wood, that provide supporting functions including nutrient cycling, soil formation, and habitat for myriad species (Janisch and Harmon, 2002; Millennium Ecosystem Assessment, 2005; Gamfeldt et al., 2013). When directly compared, primary forests consistently contain a greater volume and diversity of dead wood than forests managed for commodity production (e.g., Guby and Dobbertin, 1996; Siitonen et al., 2000; Siitonen, 2001; Debeljak, 2006).
Comparison of ecosystem processes
Here we focus on ecosystem productivity given its importance for climate mitigation, but note that other ecosystem processes will be highlighted in following sections (evapotranspiration as it relates to drought risk in section “Comparison of risks from drought,” reproduction cycles as they relate to regeneration in section “Comparison of regenerative capacity,” and nutrient cycling and retention as it relates to nutrient stocks in section “Comparison of ecosystem condition”). Differences in ecosystem productivity and carbon fluxes among forest seral stages have been the subject of much debate. One viewpoint is that forests containing younger trees are more productive, with both higher net primary productivity (NPP, including photosynthesis and autotrophic respiration) and net ecosystem productivity (NEP, also including heterotrophic respiration) than ecologically mature forests (e.g., Ryan et al., 1997; Simard et al., 2007; Goulden et al., 2010). This view has often justified the conversion of primary forests into regrowth forests. While it is true that secondary forests often have higher rates of photosynthesis, this is not always the case, particularly when accounting for the impacts of higher species richness in older primary forests (Liu et al., 2018) and the entire age profile of timber rotations, including times with bare soil and young trees. A wealth of evidence clearly shows that old-growth forests continue to sequester carbon in significant quantities in aboveground biomass, dead wood, litter, and soil organic matter (Phillips et al., 1998; Zhao and Zhou, 2006; Luyssaert et al., 2008; Lewis et al., 2009; Thompson et al., 2013; Gatti et al., 2014; Grace et al., 2014; McGarvey et al., 2015; Schimel et al., 2015; Lacroix et al., 2016; Baccini et al., 2017; Phillips and Brienen, 2017; Qie et al., 2017; Lafleur et al., 2018; Mitchard, 2018). This is why Pugh et al. (2019) found that old-growth forests (defined in that study as >140 years) cover roughly 39% of global forest area and contribute 40% of the current global forest carbon sink, which in turn represents roughly two-thirds of the terrestrial carbon sink (Friedlingstein et al., 2019).
More importantly, when comparing these CO2 fluxes in the context of mitigation actions, the entire life cycle of management and disturbance must be taken into account. From a carbon balance perspective, converting primary forests into young forests logged for biomass energy, wood supply, or other uses does not offset the original conversion emissions for many decades to centuries (Cherubini et al., 2011; Holtsmark, 2012; Mitchell et al., 2012; Keith et al., 2015; Birdsey et al., 2018; Hudiburg et al., 2019; Malcolm et al., 2020), creating a large carbon debt on policy-relevant timescales (generally years to 1–3 decades). Hence the size, longevity, and stability of accumulated forest carbon stocks, including in the soils, are important mitigation metrics in addition to the rate of annual sequestration (Mackey et al., 2013; Keith et al., 2021).
Stability and risk profiles
Ecosystem stability is comprised of resistance, resilience, and longer-term persistence (Figure 1). Combined with exposure to external perturbations, properties of ecosystem stability provide critical information for risk assessments. Risk assessments are undertaken and utilized in a wide variety of scientific and operational contexts (Fussel and Klein, 2006; Glick et al., 2011; Oppenheimer et al., 2014; Rogers et al., 2017), and are critically important to ensure mitigation actions result in long-term carbon storage. Nevertheless, risk assessments are currently either not undertaken or done so in mostly rudimentary and incomplete ways for forest-based carbon mitigation (Mignone et al., 2009; Ajani et al., 2013; Anderegg et al., 2020). Here we focus on the risk of a forest ecosystem experiencing a state-altering disturbance that results in carbon loss to the atmosphere.
Comparison of risks from wildfire
Wildfires are major natural disturbances in temperate and boreal forest ecosystems, although historically rare in tropical wet forests unless caused by humans (Randerson et al., 2012; Archibald et al., 2013; Giglio et al., 2013; Andela et al., 2017). The area burned by wildfire has been increasing in high-canopy cover forests globally over the past 20 years (Andela et al., 2017), and human-caused fires are a major driver of the loss of intact forest landscapes (Potapov et al., 2017). Extreme fire weather conditions have increased in most forests globally over the last half-century (Jolly et al., 2015; Jain et al., 2017; Dowdy, 2018), and wildfires are projected to become more widespread and intense due to climate change (Ward et al., 2012; Flannigan et al., 2013; Abatzoglou et al., 2019; Dowdy et al., 2019; Rogers et al., 2020). Humans have increased forest fire risk by augmenting forest fuels through active management (DellaSala et al., 2022a) and by increasing the number and sources of ignition (Balch et al., 2017). The majority of documented megafires globally have been started by humans under extreme fire weather conditions (Ferreira-Leite et al., 2015; Bowman et al., 2017).
A large body of literature shows that forests managed for commodity production, degraded, or disturbed forests are generally more susceptible to fires because of drier microclimates and fuels, higher land surface temperatures that promote air movement between forests and neighboring open areas, and human ignitions due to access and proximity, particularly in the tropics (e.g., Uhl and Kauffman, 1990; Holdsworth and Uhl, 1997; Cochrane et al., 1999; Laurance and Williamson, 2001; Siegert et al., 2001; Donato et al., 2006; Lindenmayer et al., 2009, 2011; Brando et al., 2014; DellaSala et al., 2022a). Although fires are a natural disturbance agent throughout most boreal forests (Viereck, 1973; Payette, 1992; Gromtsev, 2002; Soja et al., 2007; Rogers et al., 2015), fire frequency in boreal forests increases in proximity to human land use due to fuel drying, human access, and forestry practices such as leaving slash on site, particularly in Siberia (Kovacs et al., 2004; Achard et al., 2008; Ponomarev, 2008; Laflamme, 2020; Terrail et al., 2020; Shvetsov et al., 2021).
In many forest systems, fires in previously logged or managed landscapes can be more intense/severe, emit more carbon to the atmosphere, and take longer to recover than fires in ecologically mature or primary forests due to increased fuel availability, lower fuel moisture, and dense secondary forests that carry crown fires and are susceptible to extensive tree mortality (Odion et al., 2004; Stone et al., 2004; Thompson et al., 2007; Lindenmayer et al., 2009, 2011; Price and Bradstock, 2012; Kukavskaya et al., 2013; Taylor et al., 2014; Bradley et al., 2016; Dieleman et al., 2020; De Faria et al., 2021; Landi et al., 2021). In general, larger and older trees have a greater chance of surviving fires due to thicker bark and lower relative scorch height (Laurance and Williamson, 2001; Lindenmayer et al., 2019). Increased fuel availability in secondary forests can also facilitate fire spread (Lindenmayer et al., 2011). Positive feedbacks between fires and secondary vegetation can lead to permanent forest loss, i.e. “landscape traps,” at the warm / dry edge of forest ranges (Payette and Delwaide, 2003; Hirota et al., 2011; Lindenmayer et al., 2011; Staver et al., 2011; Brando et al., 2014; Kukavskaya et al., 2016; Lindenmayer and Sato, 2018). Primary forests are generally more resistant to fire because of higher humidity and fuel moisture, the presence of understory species such as ferns and mosses that limit light penetration to the forest floor and increase water retention, and much less human access (Ough, 2001; Lindenmayer et al., 2009; Taylor et al., 2014; Zylstra, 2018; Funk et al., 2019).
Comparison of risks from drought
Severe droughts represent 60–90% of climate extremes impacting gross primary productivity in the past 30 years (Zscheischler et al., 2014), are a major driver of tree mortality and forest die-off (Allen et al., 2010, 2015; Anderegg et al., 2013; McDowell and Allen, 2015; McDowell et al., 2016; Rogers et al., 2018), and are expected to increase with future climate change (Cook et al., 2014; Trenberth et al., 2014; Yi et al., 2014; Xu et al., 2019; Zhou et al., 2019; De Faria et al., 2021). A large body of literature indicates closed canopy forests are more resistant to drought, particularly in the tropics, due to shading, biophysical microclimate buffering, thicker litter layers, deeper roots, and increased water use efficiency as trees develop (e.g., Briant et al., 2010; von Arx et al., 2013; Frey et al., 2016; Brienen et al., 2017; Qie et al., 2017; Giardina et al., 2018; Caioni et al., 2020; Elias et al., 2020). For a given level of realized drought, some evidence points to larger older trees being more susceptible to drought impacts (Phillips et al., 2010; Girardin et al., 2012; Bennett et al., 2015; McDowell and Allen, 2015; McIntyre et al., 2015; Chen et al., 2016; Clark et al., 2016). Yet there is also contrasting evidence. For example, younger boreal forests can be more susceptible to drought compared to mature forests (Luo and Chen, 2013; Hember et al., 2017) due to competition for space and nutrients and less extensive and shallower root systems. Tree diversity, which is generally higher in primary compared to human-modified forests (see section “Biodiversity”), may increase resistance and resilience to drought via adaptive responses and functional redundancy (Jump et al., 2009; Sthultz et al., 2009; Dale et al., 2010; Harter et al., 2015), and intact forest canopies can be relatively resistant and resilient to short-term climate anomalies including drought (Williamson et al., 2000; Saleska et al., 2007). Evidence also suggests that mechanical “thinning,” which is frequently proposed and implemented to combat drought, decreases stand-level water use in the short-term but actually increases individual tree water demand via higher leaf-to-sapwood ratios and hence drought vulnerability in the long-term (McDowell et al., 2006; Kolb et al., 2007; D’Amato et al., 2013; Clark et al., 2016).
Mature forests transpire large quantities of water from relatively deep in the soil profile, increasing regional cloud cover and precipitation. This acts to increase the proportion of “recycled” water within a given region and thereby decreases the prevalence of regional droughts (Foley et al., 2007; Spracklen et al., 2012; Ellison et al., 2017). For example, air passing over intact tropical forest landscapes can contain twice the moisture content as air over degraded forests or non-forest landscapes (Sheil and Murdiyarso, 2009). Degradation and the loss of intact forest landscapes increases dry and hot days, decreases daily rainfall intensity and levels, and exacerbates regional droughts (Deo et al., 2009; Alkama and Cescatti, 2016).
Comparison of risks from pests and pathogens
Pests and pathogens are an increasing threat to many forests globally, particularly as climate change alters life cycles, potential ranges, and host-pest interactions (Carnicer et al., 2011; Kautz et al., 2017; Seidl et al., 2017; Simler-Williamson et al., 2019). Mature boreal and temperate forests can be more susceptible to pests and pathogens compared to younger forests, in part due to decreases in the resin flow of defense compounds (Christiansen and Horntvedt, 1983; Hansen and Goheen, 2000; Baier et al., 2002; Dymond et al., 2010). Prominent examples include bark beetle and defoliator susceptibility (Kurz et al., 2008; Raffa et al., 2008; Taylor and MacLean, 2009; Krivets et al., 2015; Kautz et al., 2017). Nevertheless, ecologically mature forests tend to be resilient to biotic infestations, as these cyclical events initiate succession and lead to stand- and landscape-level heterogeneity (Holsten et al., 2008; Thompson et al., 2009). Moreover, tree diversity (measured in terms of genetic, species, and age) tends to limit pest and pathogen spread and damage because of resource dilution, host concealment, phenological mismatches, increased predators and parasitoids, alternative hosts, and metapopulation dynamics (Root, 1973; Karieva, 1983; Pimm, 1991; Watt, 1992; Zhang et al., 2001; Jactel et al., 2005; Pautasso et al., 2005; Scherer-Lorenzen and Schulze, 2005; Thompson et al., 2009; Guyot et al., 2016).
In terms of human influence, anthropogenic disturbances such as selective logging can introduce forest pests and diseases (Gilbert and Hubbell, 1996), including non-native, and evidence suggests forest edges and logged forests are more susceptible to beetle attacks due to increases in available host niches and altered moisture conditions (Sakai et al., 2001). Many pests, particularly in temperate and boreal forests, take advantage of weakened tree defenses during drought (Raffa et al., 2008; McDowell et al., 2011; Anderegg and Callaway, 2012; Hicke et al., 2012; Keith et al., 2012; Poyatos et al., 2013; Anderegg et al., 2015). Monocultures, or tree plantations, have been shown to be particularly vulnerable due to a lack of tree diversity, high tree density, and the associated host-pest interactions (Jactel et al., 2005; Macpherson et al., 2017; Lee, 2018).
Comparison of risks from windthrow
Windthrow events can lead to forest mortality and are expected to increase in some regions with climate change (Klaus et al., 2011; Saad et al., 2017). Although these events are somewhat stochastic, they are also influenced by soils, orography, regional climate regimes, and forest composition and structure. Similar to the risks of pests and pathogens, within a given stand there is evidence that older and taller trees are more susceptible to windthrow due to the physics of taller trees and root rot (Lohmander and Helles, 1987; Ruel, 1995). Nevertheless, fragmented or thinned forests experience elevated mortality and collapse of trees from windthrow because of increased exposure (Laurance and Curran, 2008; Reinhardt et al., 2008; Schwartz et al., 2017).
Comparison of risks from species range shifts
Climate regimes have strong influences on the potential and realized ranges of forest tree species, evidenced by the paleoecological record (Overpeck et al., 1991; DeHayes et al., 2000; Davis and Shaw, 2001) and current assemblages (e.g., Neilson, 1995; Foley et al., 2000), and considerable scientific effort is focused on projecting future responses to climate change (e.g., Sitch et al., 2003; Elith and Leathwick, 2009; Rogers et al., 2011, 2017; Ehrlen and Morris, 2015; Prasad et al., 2020). How trees and forest ecosystems will respond is uncertain due to complex interactions between the pace of climate change, physiological tolerances, dispersal and migration rates, phenotypic plasticity and adaptation, the presence of climate refugia, migration of associated species / symbionts, and forest fragmentation, among others (Davis and Shaw, 2001; Iverson et al., 2004; Jump and Penuelas, 2005; Mackey et al., 2008; Nicotra et al., 2010; Prasad, 2015; Rogers et al., 2017). In general, current and projected climate change is expected to degrade biodiversity due to species extinctions and the contraction of realized ranges (Miles et al., 2004; Campbell et al., 2009). Forest and landscape fragmentation in particular is known to hinder resilience and species migration because of the loss of suitable areas for dispersal and limitations on gene flow (Collingham and Huntley, 2000; Loreau et al., 2002; Scheller and Mladenoff, 2008; Thompson et al., 2009). Large areas of primary forests are expected to have higher adaptive capacity and stability compared to forests under human pressure because of their connectivity, biodiversity, and microclimate buffering (Mackey et al., 2015; Watson et al., 2018; Thom et al., 2019; see section “Biodiversity”).
Comparison of risks from land use degradation
Human land use pressures on forests generally result in both direct environmental impacts as well as further, often unplanned, degradation or deforestation that accumulates spatially and temporally. This is exemplified by the fact that smaller fragments of primary forest have an elevated likelihood of loss (Hansen M. C. et al., 2020). New roads are the primary driver of further degradation as a result of their construction, use, and continued access (e.g., Trombulak and Frissell, 2000; Wilkie et al., 2000; Laurance et al., 2009; Laurance and Balmford, 2013; Ibisch et al., 2016; Alamgir et al., 2017; Venier et al., 2018; Maxwell et al., 2019). Roads render the surrounding forests much more susceptible to agricultural conversion (Asner et al., 2006; Boakes et al., 2010; Gibbs et al., 2010; Laurance et al., 2014; Kormos et al., 2018), logging (Laurance et al., 2009; Barber et al., 2014), and expanded networks of secondary and tertiary roads (Arima et al., 2008, 2016; Ahmed et al., 2014). Logging and transportation can also lead to severe erosion and nutrient runoff, impacting downstream water quality and quantity (Carignan et al., 2000; Hartanto et al., 2003; Foley et al., 2007), and damage the surrounding forest. For example, in the Amazon, it has been estimated that for every commercial tree removed via selective logging, roughly 40 m of roads are created, nearly 30 other trees greater than 10 cm in diameter are damaged, and between 600 and 8,000 m2 of canopy is opened (Holloway, 1993; Asner et al., 2004). Furthermore, roads reduce animal habitat, are barriers to animal movement and lead to increased animal mortality, including from unregulated hunting, all of which decrease connectivity and genetic exchange (Dyer et al., 2002; Frair et al., 2008; Laurance et al., 2009; Taylor and Goldingay, 2010; Clements et al., 2014). One consequence is a decline in carbon-dense tree species due to overhunting of seed-dispersing animals (Osuri et al., 2016; Maxwell et al., 2019). It is important to note that roughly 95% of deforestation in the Amazon occurs within 5.5 km of a road (Barber et al., 2014), and that illegal logging represents 85–90% of all logging in the tropics (Lawson and MacFaul, 2010; Lawson, 2014; Hoare, 2015) and still roughly one-quarter of logging in Russia (Food and Agriculture Organization of the United Nations [FAO], 2012; Kabanets et al., 2013), which contains the largest areal forest coverage of any country (Food and Agriculture Organization of the United Nations [FAO], 2020). Overall, road building and industrial logging are the largest drivers of initial forest degradation and fragmentation (Hosonuma et al., 2012).
In addition to their direct impacts, roads and land use further degrade forests due to edge effects. Forests at or near an edge can have substantially drier microclimates, increased windshear and movement of dry air into forests, invasive species (dispersed via roads and more favorable microclimate conditions for competition), weeds and vines, sun exposure, soil erosion, and fuel loads due to drying and previous logging and fire (Laurance and Williamson, 2001; Mortensen et al., 2009; Brando et al., 2014). This leads to a variety of unfavorable impacts and further risks. Carbon densities tend to be significantly lower near forest edges. For example, biomass is reduced by roughly 50% within 100 m, 25% within 500 m, and 10% within 1.5 km of a forest edge (Laurance et al., 1997; Chaplin-Kramer et al., 2015; Maxwell et al., 2019). Aggregated across the tropics, edge effects are estimated to account for up to one quarter of all carbon loss from tropical deforestation (Putz et al., 2014). Primary productivity is also generally lower near forest edges, and fire susceptibility is higher due to elevated and drier fuel loads and increased human access (Laurance et al., 1998; Cochrane et al., 1999; Nepstad et al., 1999; Laurance and Williamson, 2001; Foley et al., 2007; Adeney et al., 2009; Brando et al., 2014). For example, roads are strong predictors of ignition and wildfire frequency in temperate forests (Hawbaker et al., 2013; Faivre et al., 2016; Parisien et al., 2016; Balch et al., 2017; Ricotta et al., 2018), and road expansion in Siberia has been shown to promote logging and human-caused forest fires (Kovacs et al., 2004). A variety of ecosystem services are degraded due to edge effects, including hydrologic regulation, water quality, modulation of regional climate, and amelioration of infectious diseases (Laurance and Williamson, 2001; Foley et al., 2007). Although the impacts are strongest at a forest edge, the effects can generally be detected up to 2 km from the edge, with higher tree mortality up to 1 km and wind disturbance up to 500 m (Broadbent et al., 2008). Globally, fragmentation is thought to be at a critical threshold, with roughly 70% of the world’s forest within 1 km of a human-created forest edge (Haddad et al., 2015; Taubert et al., 2018).
Comparison of regenerative capacity
Ecosystem resilience is underpinned by the natural regenerative capacity of a forest ecosystem, and hence represents a major component of ecosystem stability and integrity (Figure 1). Regeneration from major disturbance events requires biological legacies, which are broadly defined as the remaining living and dead structures and organisms that can influence recovery (Franklin et al., 2000; Jogiste et al., 2017). These include living and dead trees, shrubs and other plants, seeds, spores, fungi, eggs, soil communities, and living animals (Franklin et al., 2000; Stahlheber et al., 2015; Lindenmayer et al., 2019). Compared to secondary or human-modified forests, primary forests tend to have the biological legacies (Catterall, 2016; Chazdon and Uriarte, 2016; Lu et al., 2016; Poorter et al., 2016; Lindenmayer et al., 2019) and favorable microclimates (von Arx et al., 2013) required for optimal regeneration. This is evidenced by the fact that secondary forest regeneration is aided by proximity to primary forests (Schwartz et al., 2015; Kukavskaya et al., 2016). Clearcut logging also generates low levels of biological legacies and higher regeneration failures after subsequent fires compared to forests not previously logged (Perrault-Hebert et al., 2017), which is exacerbated by post-fire “salvage” logging (Donato et al., 2006; Lindenmayer et al., 2019). Successive disturbances continue to decrease regenerative capacity, and can lead to permanent forest loss and emergence of non-forest ecosystems (Payette and Delwaide, 2003; Johnstone et al., 2016; Kukavskaya et al., 2016). Compared to degraded or human-modified forests, primary forests with large extents also host a much larger array of seed dispersers and pollinators (Muller-Landau, 2007; Wright et al., 2007; Abernethy et al., 2013; Harrison et al., 2013; Peres et al., 2016).
Comparison of biodiversity
Biodiversity underpins and is affected by the foundational elements of ecosystem integrity (Figure 1), but is also a metric of ecosystem condition and can be considered an ecosystem service in its own right. Globally, trees are among the most genetically diverse of all organisms, and forests collectively support the majority (roughly 80%) of terrestrial biodiversity (Hamrick and Godt, 1990; Barlow et al., 2007; Pimm et al., 2014; Federici et al., 2017). There is a substantial body of literature on the effects of disturbance and stand age on biodiversity, with some disagreement among studies depending on context (e.g., Paillet et al., 2010; Edwards et al., 2011; Moreno-Mateos et al., 2017; Kuuluvainen and Gauthier, 2018; Matos et al., 2020). Nevertheless, there are clear and definitive negative impacts of human disturbance and land use on biodiversity (Cairns and Meganck, 1994; Ellison et al., 2005; Barlow et al., 2007, 2016; Gibson et al., 2011; Alroy, 2017; Giam, 2017). Primary and ecologically mature forests typically harbor higher biodiversity than human-modified forests (Lesica et al., 1991; Herbeck and Larsen, 1999; Rey Benayas et al., 2009; Zlonis and Niemi, 2014; Miller et al., 2018; Watson et al., 2018; Lindenmayer et al., 2019; Thom et al., 2019), especially in the understory (e.g., Lafleur et al., 2018). Disturbance generally results in a change in species composition toward early pioneer species (e.g., Bawa and Seidler, 1998; Liebsch et al., 2008; Venier et al., 2014). The effect of human activities on the provision of ecosystem services is evident even if there is little change in the overall forest cover. Degradation in logged forests can be in the form of structural changes such as reduction in old age classes of trees that can cause loss in breeding habitat, particularly for birds (Rosenberg et al., 2019; Betts et al., 2022), and compositional changes such as shifts in tree species abundance that differ in foliar nutrient concentrations that support arboreal folivores (Au et al., 2019). Under less intensive agriculture management, agroforestry can maintain a significant fraction of biodiversity, but it is still considerably lower than in native forests (De Beenhouwer et al., 2013; Vallejo-Ramos et al., 2016).
Biodiversity analyses are also strongly dependent on spatial scale, whereby higher levels of management and disturbance homogenize forest composition and age structure across the landscape, and consequently the biota it supports (e.g., Devictor et al., 2008; de Castro Solar et al., 2015; Tomas Ibarra and Martin, 2015). What can be concluded is that (i) degraded and intensively managed forests tend to harbor lower biological and functional diversity compared to primary forests, which support many as yet unidentified species and act as repositories for species that cannot survive in secondary or degraded forests (Barlow et al., 2007; Gibson et al., 2011), and (ii) natural disturbances are effective at maintaining landscape heterogeneity and the species that depend on disturbed and young forests (Lindenmayer et al., 2019). Global biodiversity loss is currently orders of magnitude higher than background rates and is driven primarily by deforestation and forest degradation (Newbold et al., 2016; Giam, 2017). It is worth noting that although natural tree diversity in boreal forests is typically much lower than in temperate or tropical forests (Thompson et al., 2009; Hill et al., 2019), the biodiversity of other species groups such as bryophytes and lichens can be very high (DellaSala, 2011; Kuuluvainen and Gauthier, 2018), functional diversity in boreal forests is generally high (Esseen et al., 1997; Wirth, 2005), and the broad genetic variability and phenotypic plasticity of boreal trees allows them to tolerate a wide range of environmental conditions (Gordon, 1996; Howe et al., 2003).
Comparison of ecosystem condition
Given our focus on climate mitigation, the primary metric of concern for ecosystem condition is carbon stocks. Primary and ecologically older forests have been consistently found to have the highest carbon stocks compared to secondary, degraded, intensively managed, or plantation forests (e.g., Harmon et al., 1990; Cairns and Meganck, 1994; Nunery and Keeton, 2010; Burrascano et al., 2013; Mackey et al., 2013; Keith et al., 2015, 2017; Federici et al., 2017; Lafleur et al., 2018; Watson et al., 2018). For example, a recent meta-analysis shows that primary tropical forests store on average 35% more carbon than forests affected by conventional management for commodity production (Mackey et al., 2020). Across the tropics, intact forest landscapes cover approximately 20% of total area but store 40% of total aboveground biomass (Potapov et al., 2017; Maxwell et al., 2019). This is fundamentally a function of where carbon is stored in these forests. In wet tropical and some temperate primary forests, roughly half the biomass carbon is stored in the largest 1–3% diameter trees (Stephenson et al., 2014; Lutz et al., 2018; Mildrexler et al., 2020), which have long residence times (Koerner, 2017; van der Sande et al., 2017), and are typically the first to be felled (Cannon et al., 1998; Sist et al., 2014; Gatti et al., 2015; Rutishauser et al., 2016). Agricultural landscapes store comparatively less carbon, but the addition of trees via agroforestry has the potential to add up to 9 Pg C globally (Chapman et al., 2020). In boreal forests, especially those that are poorly drained, the majority of forest ecosystem carbon is stored in dead biomass, peat, and soil organic layers that accumulate over the course of forest succession, often protected by permafrost (Deluca and Boisvenue, 2012; Bradshaw and Warkentin, 2015; Lafleur et al., 2018; Walker X J et al., 2020). Boreal forests managed for timber are kept at younger ages, with soils that store significantly less carbon due to mechanical disturbance, tree species conversion, and impacts on litter composition, nutrient cycling, and bryophyte communities (Liski et al., 1998; Jiang et al., 2002; Seedre et al., 2014; Lafleur et al., 2018). Even outside the boreal zone, soil carbon can be a significant fraction of total ecosystem carbon (e.g., Keith et al., 2009), and logging activities generally deplete forest soil carbon due to soil compaction and disturbance, erosion, changes in microclimate that increase respiration rates, reduced leaf litter and root exudates, loss of micorrhizal network carbon, and post-logging “slash” burning (Rab, 2004; Zummo and Friedland, 2011; Buchholz et al., 2014; James and Harrison, 2016; Hume et al., 2018; Mayer et al., 2020). Globally, forests are thought to store only half of their potential carbon stock, with 42–47% of the reduction due to forest management and modification (the remainder being deforestation and land cover changes; Erb et al., 2018). Natural regeneration of forests could in turn restore 123 Pg C, or 27% of the total biomass carbon that has been lost (Erb et al., 2018).
Forest management, degradation, and conversion can also result in the loss of key nutrients such as nitrogen and phosphorous, among others, which are otherwise retained efficiently in undisturbed forests (Likens et al., 1970; Markewitz et al., 2004; Olander et al., 2005; Liu et al., 2019). Nutrients can be artificially added, but heavily managed systems require large inputs to maintain their state and productivity capacity (Noss, 1995; Merino et al., 2005; Pandey et al., 2007). Other elements of ecosystem condition are affected similarly and highlighted elsewhere (landscape connectivity / fragmentation in section “Comparison of risks from land use degradation,” biodiversity in section “Comparison of biodiversity,” and water quality and quantity in section “Comparison of ecosystem services”).
Comparison of ecosystem services
A large body of literature indicates the higher number, quality, and value of ecosystem services provided by primary forests compared to human-modified forests and landscapes. These include regulating services such as water quality and quantity (DellaSala, 2011; Brandt et al., 2014; Keith et al., 2017; Kormos et al., 2018; Taylor et al., 2019; Vardon et al., 2019); carbon storage and sequestration as an ecosystem service of global climate regulation (United Nations [UN], 2021) [discussed above, but see Keith et al. (2019) and Uganda Bureau of Statistics [UBOS] (2020) for examples using Ecosystem Accounts]; local to regional biophysical cooling (Spracklen et al., 2012; Lawrence and Vandecar, 2015); regulation of runoff, sediment retention, erosion control, and flood mitigation (Hornbeck and Federer, 1975; Jayasuriya et al., 1993; Dudley and Stolton, 2003; Furniss et al., 2010; van Haaren et al., 2021); provisioning services such as abundance of game and fish (Gamfeldt et al., 2013; Brandt et al., 2014); cultural services such as landscape aesthetics, recreation, and tourism (Brandt et al., 2014; Brockerhoff et al., 2017); cultural practices and knowledge (Normyle et al., 2022); contributions to physical and psychological health (Stier-Jarmer et al., 2021); and general assessments across a suite of services (e.g., Myers, 1997; Harrison et al., 2014; Shimamoto et al., 2018; Maes et al., 2020).
For example, a detailed assessment of the differences between primary forests and post-logging regrowth forests in terms of their ecosystem condition, the physical supply of a suite of ecosystem services, and their monetary valuation showed the superior aggregated value of the primary forest (Keith et al., 2017). The impacts of mechanical disturbance due to logging, roading, and mining on soil properties reduce the ecosystem services of soil nutrient availability, water holding capacity and erosion prevention (Hamburg et al., 2019). A general assessment of the total economic value of ecosystem services provided by forest ecosystem types showed that primary forests had a higher median value (USD 139 ha–1 year–1) compared with secondary forests (USD 128 ha–1 year–1) (Taye et al., 2021). These aggregated values include only the market values for services when known and could not account for non-market values, for example that would be needed to assess biodiversity habitat or many cultural services. The highest reported values for specific ecosystem services were for airflow regulation, water cycle regulation and food for freshwater plants and animals. These services would all have their highest provision from natural ecosystems. In contrast, the value of timber and fiber products is significantly lower.
Lessons from comparative analysis
Taken as a whole and for a given set of environmental conditions, our comparative analysis shows that primary forests have the highest levels of ecosystem integrity compared to human-modified forests, including naturally regenerating forests managed for commodity production, plantations, and previously forested landscapes. One primary set of mechanisms are positive feedbacks whereby forest disturbance tends to beget more disturbance (e.g., Seidl et al., 2017), and degradation begets more degradation (e.g., Venier et al., 2018; Watson et al., 2018). In terms of variables most relevant for mitigation, adaptation, and other international forest policy goals, primary forests store the highest carbon stocks, present the lowest risks of forest and carbon loss reversal, have the highest biodiversity, and provide the largest stocks of ecosystem assets and highest quality flows of ecosystem services, including benefits to the global community, local communities (Vickerman and Kagan, 2014), and Indigenous peoples.
Based on our review, and because human-modified forests can encompass a wide range of management strategies and intensities, we provide further summaries of ecosystem integrity for five main categories of forest types: (A) primary forests; (B) secondary forests; (C) production forests; (D) agro-forests; and (E) plantations (Figure 2 and Table 1). Primary forests have the most developed dissipative structures, the highest levels of ecosystem processes, greater stability and recovery, and thus greater resilience and the lowest risk of loss and damage. As defined here, secondary forests are in recovery from past human impacts especially logging. Although they can transition to primary forests over time, these forests lack some old growth characteristics, are more vulnerable to wildfire and other natural disturbances, and have missing elements of biodiversity. Production forests are a result of conventional forest management for commodity production, and tend to be kept at relatively young ages with associated reductions in dissipative structures, carbon stocks, and resilience. An example of commercial agro-forests is shade coffee where retaining some natural canopy tree cover provides some additional ecosystem service benefits. Subsistence agro-forests are common in many tropical development countries such as Vanuatu where these household and community gardens were, and in many cases still are, the main source of food. Commercial plantations include monocultures of trees species that are essentially tree farms for commodity production (wood, palm oil). Note that there are gradients of human modification, stand age, and ecosystem integrity within these broad categories. For example, mature forests recovering from past human disturbances may not have the full suite of structural, functional, and compositional benefits as primary forests, but they can gain these over time, and generally have higher ecosystem integrity than forests recovering from more recent human disturbance (DellaSala et al., 2022b).
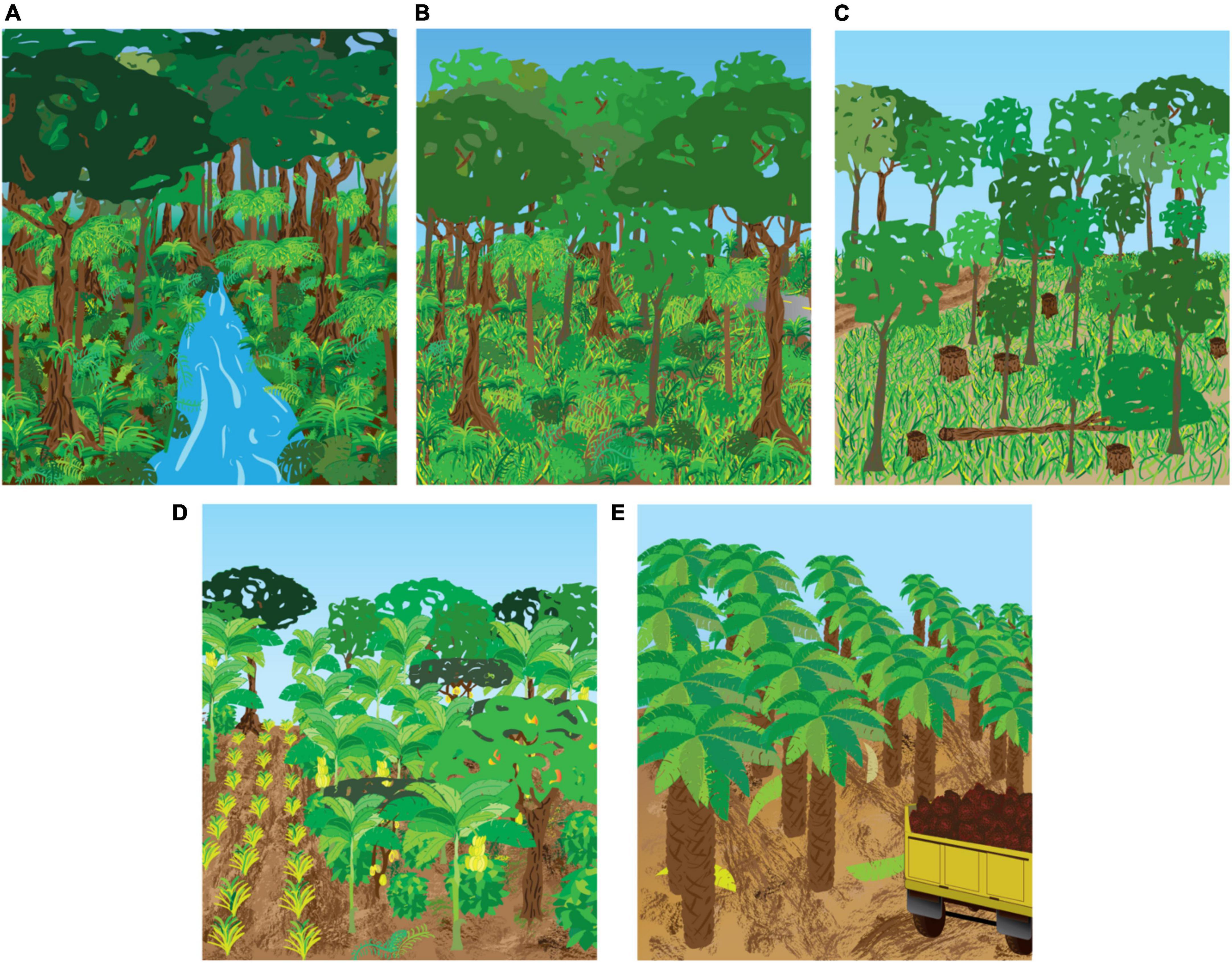
Figure 2. Graphical illustrations of five main forest types considered for ecosystem integrity comparisons, including (A) primary forests, (B) secondary forests, (C) production forests, (D) agro-forests, and (E) plantations. Note this illustration focuses on tropical forests, but the same general differences apply across forest biomes.
Implications for policy, management, and future research
Evaluating ecosystem integrity
We have shown that the risk of forest carbon loss can be minimized by prioritizing actions that maintain and enhance forest ecosystem integrity. Ecosystem integrity therefore has the potential to be used as an integrating framework for evaluating forest-based mitigation and adaptation actions. Because ecosystem integrity is an inherently complex concept, the scientific, management, and policy communities need approaches and tools to measure and interpret gradients of integrity consistently across forest types and jurisdictional boundaries (Karr, 1996; Grantham et al., 2020). The metrics and their interpretation should ideally account for the range of spatial and temporal scales involved: small patches of high-integrity forests are valuable, but landscape context is required; snapshots in time are useful, but longer-term dynamics are needed to fully understand integrity.
A complete and exhaustive global representation of forest ecosystem integrity may currently be beyond our reach. Nevertheless, several existing data products represent important elements of ecosystem integrity, each with their own advantages and limitations, and can be used to guide decision making. In the humid tropics, natural and hinterland forests (primary forests and mature secondary growth) have been mapped using multispectral satellite imagery (Turubanova et al., 2018) and spatial statistics (Tyukavina et al., 2016). Canopy structural integrity has recently been mapped using space-based lidar, multispectral imagery, and human pressure indices (Hansen et al., 2019; Hansen A. J. et al., 2020), representing an important step in delineating gradients of integrity. These mapping approaches are inherently more challenging outside the humid tropics where environmental gradients generate a range of potential forest cover and types. Global products therefore tend to rely more on metrics based on the relationships between forest loss/degradation and proximity to human activities, including roadless areas, forest fragmentation, loss of tree cover, and measures of the “human ecological footprint” (Hansen et al., 2013; Haddad et al., 2015; Ibisch et al., 2016; Venter et al., 2016b,a; Beyer et al., 2020; Grantham et al., 2020; Williams et al., 2020). Global Intact Forest Landscapes (Potapov et al., 2008, 2017) have been widely used, but these include patches of non-forest ecosystems and exclude areas of high-integrity forests in patches <50,000 ha. The Food and Agriculture Organization of the United Nations (FAO) has reported on primary forests since 2005 in their global forest assessment reports (Food and Agriculture Organization of the United Nations [FAO], 2020), but a lack of consistency in national-level reporting makes comparisons and trend detection difficult.
Similar to Grantham et al. (2020), we stress the importance of using local data and field observations to further identify and refine estimates of forest ecosystem integrity derived from coarser-scale global mapping products. These may include landscape-level metrics such as frequency distributions of stand age, biomass, coarse woody debris, biodiversity, forest patch sizes and shapes, and forest types and species composition. Individual countries have data archives, collection programs, and often agency directives that either include ecosystem integrity metrics or those with high relevance for integrity assessments (e.g., Muller et al., 2000; Tierney et al., 2009; Wurtzebach and Schultz, 2016). Applying the internationally endorsed SEEA-EA system should also enable a consistent framework for comparisons across spatial and temporal scales. The SEEA-EA standard provides guidance for classifications, definitions, spatially explicit analysis, and temporal consistency. Technical guidance on ecosystem integrity indicators was recently provided by Hansen et al. (2021). Although criteria were provided in the context of CBD’s post-2020 Global Biodiversity Framework, many would apply outside this context, including a need for biome to global scale products with spatial resolution sufficient for management (≤ 1 km), temporal re-assessment at intervals of 1–5 years, ability for indicators to be spatially aggregated without bias, credibility through validation and peer review, and accounting for reference states within a given climate, geomorphology, and ecology. Finally, we note the importance of understanding how any given metric of ecosystem integrity connects to the conceptual framework of ecosystem integrity (Figure 1).
Implementing ecosystem integrity
Protecting primary forests
Given the superior benefits of primary forests, follows that protecting them would significantly contribute to meeting international climate, biodiversity, and SDGs. Primary forests are disappearing at a rapid rate (e.g., Potapov et al., 2017; Food and Agriculture Organization of the United Nations [FAO], 2020; Hansen M. C. et al., 2020; Silva Junior et al., 2021) and urgently need higher levels of protection to ensure their conservation; only roughly one-fifth of remaining primary forests are found in the International Union for Conservation of Nature (IUCN) Protected Areas Categories I-VI (Mackey et al., 2015). Proven effective mechanisms to protect primary forests include enforcing existing and establishing new reserves and protected area networks, limiting new road construction, payments for ecosystem services, effective governance, and protecting the rights and livelihoods of indigenous peoples and local communities (Mackey et al., 2015; Kormos et al., 2018; Walker W. S et al., 2020). Complementary measures and enabling conditions include supporting legislation and enforcement of protection status, industry re-adjustment to source alternative fuel, food and wood products, and management of weeds, pests, feral animals, and livestock grazing (Mackey et al., 2020).
Protecting primary forests will also be facilitated by changes to current international forest and carbon accounting rules. Existing “net” forest cover accounting rules, such as the IPCC good practice guidelines for national greenhouse gas inventories and the land sector, are problematic because they report net changes and treat all forests equally, regardless of their integrity, thereby incentivizing the conversion of primary forests into commodity production (Mackey et al., 2013, 2015; Peterson and Varela, 2016; Moreno-Mateos et al., 2017; Funk et al., 2019; Skene, 2020). Such changes in forest management can have the perverse effect of accelerating emissions and degrading ecosystems. Similarly, flux-based carbon accounting effectively hides the emissions or lost sequestration potential from logging primary forests (e.g., Skene, 2020) and does not account for the risk profiles of different forest types. Reporting “gross” forest cover changes as well as adopting stock-based accounting (Ajani et al., 2013; Keith et al., 2019, 2021) could more fully leverage an ecosystem integrity framework, and ultimately ensure the maximum mitigation benefits and ecosystem services are secured from Earth’s remaining forests.
Management of other forest types
Management of secondary forests for commodity production, along with tree plantations and agroforestry, can contribute to climate mitigation and other SDGs and reduce pressure on primary forests and other natural forests with high levels of ecosystem integrity (Watson et al., 2018; Roe et al., 2019; Chapman et al., 2020). However, the key is to direct these management activities to previously deforested or degraded lands and accompany them with systematic landscape planning and effective governance (Dooley et al., 2018; Kormos et al., 2018; Martin et al., 2020; Morgan et al., 2020). For example, much of the overall timber demand could be harvested from secondary forests, but these are often overlooked as resources by land owners, the timber industry, and governments (Bawa and Seidler, 1998). Globally, intensively managed tree plantations or planted forests supply over 50% of global wood supply (Warman, 2014) yet occupy only 7% of global forest cover (Food and Agriculture Organization of the United Nations [FAO], 2020). It is therefore feasible to meet global wood supply with existing plantations and additional ones established on previously cleared or degraded land. These land uses, however, are decidedly not beneficial for carbon budgets or ecosystem services when undertaken at the cost of clearing or degrading primary forests.
Governments and forest managers can aim to optimize the ecosystem integrity of secondary forests (for example in terms of yield, regenerative capacity, and biodiversity) within the confines of their intended uses (Thompson et al., 2009; Grantham et al., 2020). In tandem with alternative fibers, this will help alleviate pressures on primary forests. A similar argument exists for agricultural productivity (Laurance et al., 2001; Hawbaker et al., 2006; Sabatini et al., 2018). All of these activities can be done with appropriate landscape planning in ways that collectively increase economic yield and ecosystem services, and serve local communities (Bawa and Seidler, 1998; Burton et al., 2006; Mathey et al., 2008; Food and Agriculture Organization of the United Nations [FAO], 2012; Naumov et al., 2016).
Afforestation, forest restoration, and proforestation (i.e., allowing secondary forests to naturally regrow and restore their ecosystem carbon stocks) are also important components of forest-based mitigation and conservation activities (Giam et al., 2011; Griscom et al., 2017; Verdone and Seidl, 2017; Moomaw et al., 2019; Roe et al., 2019; Cook-Patton et al., 2020). Proforestation holds promise for near-term mitigation because the established trees are already on the steepest part of their growth curve (Moomaw et al., 2019; Mackey et al., 2020). However, none of these forest management activities can replace the carbon stocks and ecosystem services of high-integrity primary forests on decadal to century timeframes. It is also generally less expensive to protect primary forests than to reforest or restore forests (Possingham et al., 2015; Griscom et al., 2017). Furthermore, potential “overcrediting” for offset and restoration schemes can result in net harm and carbon emissions, whereas “overcrediting” for primary forest protection only reduces the benefits, but does not lead to net societal and climate damages (Anderegg et al., 2020). We therefore urge that forest restoration should be conducted in concert with protection of primary forests, and not instead.
Finally, we note that selective logging, or so called “reduced impact logging” in tropical forests has been shown many times to be unsustainable (Zimmerman and Kormos, 2012; Kormos et al., 2018), as it results in significant damage to the target forests as well as collateral damages to surrounding forests due to road building, transportation, and further clearing for land uses such as agriculture (Kormos and Zimmerman, 2014; Mackey et al., 2020). Generally, as timber extraction becomes less intensive, the per-tree collateral damages increase exponentially (Gullison and Hardner, 1993; Boot and Gullison, 1995; Bawa and Seidler, 1998; Umunay et al., 2019; Zalman et al., 2019). After the first cut, selective logging is much less economically viable compared to plantations and intensive forestry (Bawa and Seidler, 1998; Naumov et al., 2016). Even measures aimed at reducing emissions via collateral damages from selective logging may not generate benefits and merely serve to justify and subsidize the degradation of high-integrity primary forests (Macintosh, 2013; Watkins, 2014; Gatti et al., 2015). Overall, selective logging and its associated degradation may be as much or more harmful than outright deforestation for pan-tropical forests and their carbon stocks (Nepstad et al., 1999; Foley et al., 2007; Baccini et al., 2017; Erb et al., 2018; Bullock et al., 2020; Matricardi et al., 2020).
Relevance for international policy
There has been a recent uptick in the recognition of the importance of ecosystem integrity and primary forests for multiple climate, biodiversity, and SDGs. For example, the preamble to the Paris Agreement notes the importance of ensuring the integrity of all ecosystems, and recent international policy developments point to the importance of maintaining and restoring ecosystem integrity for achieving the goals of the Rio Conventions and all of the SDGs, but in particular SDG 15 (Life on Land). The importance of primary forests for achieving synergistic climate and biodiversity outcomes was also reflected in Working Group II (IPCC, 2022) and III (Nabuurs et al., 2022) of the IPCC’s Sixth Assessment Report, as well as key decisions from the CBD 14th Conference of the Parties (14/5 and 14/30) (Convention on Biological Diversity [CBD], 2018).
We strongly recommend an increased focus on integrating climate and biodiversity action, which provides an opportunity to deliver multiple societal goals through ensuring the integrity of ecosystems (Barber et al., 2020). The importance of the nexus between effective action on climate change and biodiversity is reflected in the findings of the first ever joint workshop of the IPCCC and IPBES held in 2021 (Pörtner et al., 2021), which encouraged synergistic climate and biodiversity action and identified priorities for action, in particular the protection and restoration of carbon and species rich natural ecosystems such as forests.
The integrity of ecosystems is also being promoted by civil society as an important factor to consider in the UNFCCC Global Stocktake, a central pillar of the Paris Agreement against which its success or failure will be judged (Climate Action Network, 2022). We suggest that utilizing the UN SEEA-EA to benchmark protection and restoration actions would provide critical information on ecosystem integrity elements for the Global Stocktake to inform high-benefit / low-risk nature-based solutions in evolving NDCs. Successful implementation of the ecosystem provisions of the UNFCCC and the Paris Agreement, including decisions made at COP 25 (1.CP 25 para. 15) calling for integrated action to prevent biodiversity loss and climate change; and COP 26 (CMA/3 para. 21 and 1.CP/26 para. 38) emphasizing “…the importance of protecting, conserving and restoring nature and ecosystems, including forests …,” depends upon understanding the significance of ecosystem integrity for stable long term carbon storage and the overall health of the biosphere.
Other recent policies and guiding documents include the Glasgow Leaders’ Declaration on Forests and Land Use (United Nations Climate Change, 2021), CBD post-2020 Global Biodiversity Framework (Convention on Biological Diversity [CBD], 2021), IUCN Policy Statement on Primary Forests Including Intact Forest Landscapes (IUCN, 2020), IPBES Global Assessment Report (IPBES, 2019), the New York Declaration on Forests 5-Year Assessment Report (NYDF Assessment Partners, 2019), the European Parliament resolution to protect and restore forests (European Parliament, 2020), and Indonesia’s moratorium on converting primary forests and peatlands (Austin et al., 2019).
Nevertheless, there is still much work to be done at national and international levels, with the evolving Paris Rulebook and country NDC’s arguably representing the largest opportunity. Translating all these international declarations into coherent national and jurisdictional policies will require an agreed-upon framework of ecosystem integrity, such as provided here, and applicable data products tools for implementation.
Future research directions
Because ecosystem integrity is such an integrative and multidisciplinary concept, research gaps are relatively extensive. We therefore do not offer an exhaustive list, but rather a prioritized assessment of future research directions to improve the understanding, valuation, and operationalization of ecosystem integrity. First and foremost, operationalizing forest ecosystem integrity at scales relevant to policy and planning that span from landscape planning (Morgan et al., 2022) to national strategies (Center for Biological Diversity [CBD], 2022) and international agreements (United Nations [UN], 2021) requires accurate and updated maps of ecosystem integrity and its components. Existing products (described in section “Evaluating ecosystem integrity”) touch on aspects of canopy structural integrity, can be used to identify areas of remaining natural forests, and, using time series data, can locate where they have been lost (Figure 3). However, their ability to differentiate levels of integrity between forests is limited, and they do not account for the longer-term ecosystem dynamics that comprise functional integrity. It will therefore be helpful to leverage the time series of now decades-long satellite records such as Landsat and the Moderate Resolution Imaging Spectroradiometer (MODIS) to incorporate metrics of stability / resistance, and to capture smaller patches of high-integrity forests, such as in Shestakova et al. (2022). In boreal and temperate forests with naturally occurring stand-replacing disturbances, for example wildfire, it will be critical to accurately separate these from human disturbances, for example by using spatial pattern recognition techniques (e.g., Curtis et al., 2018).
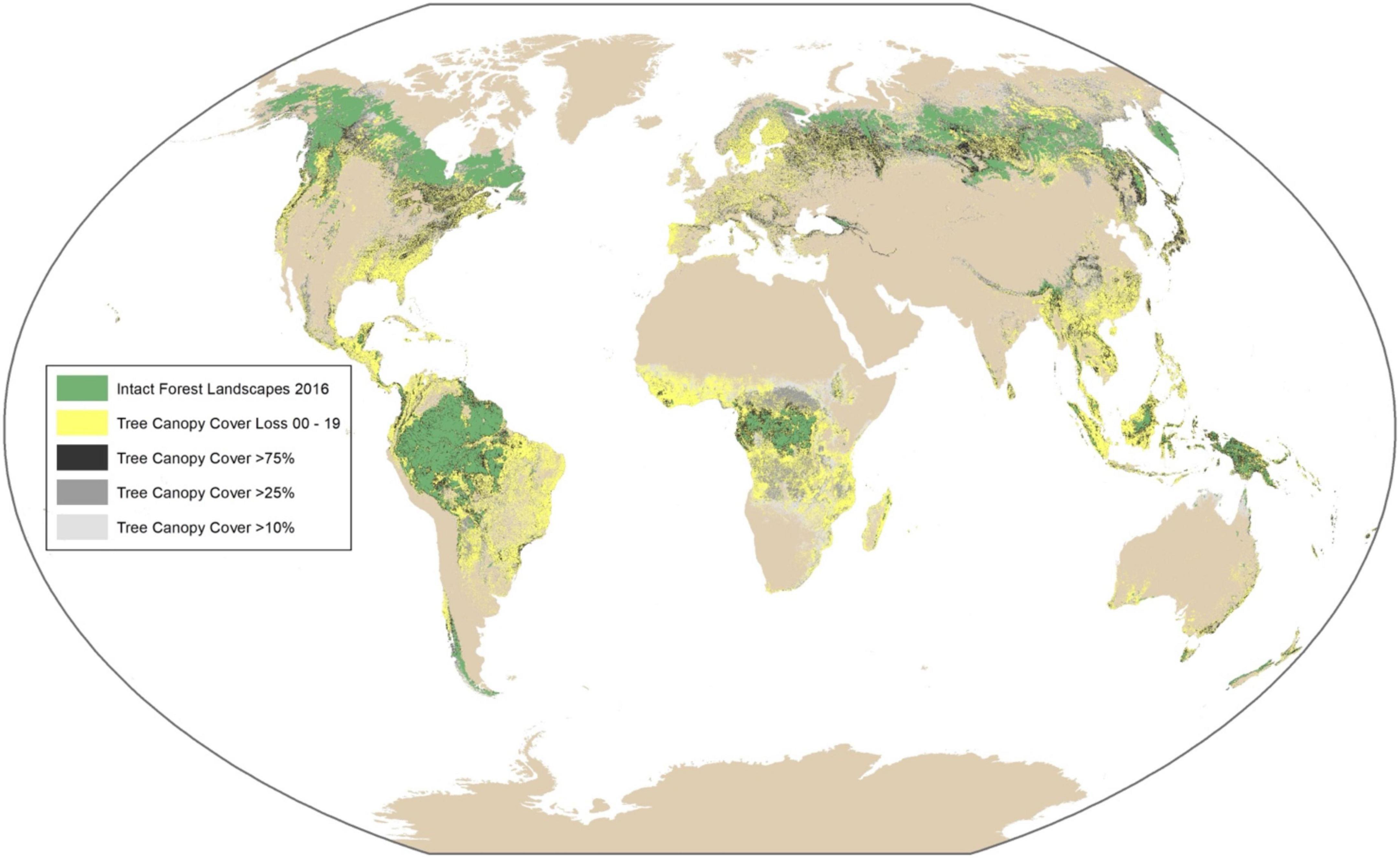
Figure 3. Global forest condition as indicated by metrics of Intact Forest Landscapes (IFLs), tree canopy cover, and tree canopy cover loss (from 2000 to 2019). IFLs for the year 2016 are taken from Potapov et al. (2017), and tree cover and tree cover loss outside of IFLs are from Hansen et al. (2013).
For the purpose of primary forest protection, accurate maps of regularly updated primary forests are needed at sufficient spatial scales and accuracy to support both country-level assessments as well as local decision making. Spatial assessments of forest ecosystem integrity and components, as opposed to categorical maps of forest/no-forest or broad forest types, are particularly needed. In addition to developing countries, this information is needed in the United States, Europe, and other developed countries with little remaining primary forests. In these cases, the most ecologically mature forests for a given ecosystem type (e.g., DellaSala et al., 2022b) likely represent the highest integrity levels rather than primary forests per se (Table 1 and Figure 2) and similarly require both field and remote sensing analysis to be defined and identified (e.g., Federal Register, 2022). Aside from mapping methodologies and data products, we stress the need for continued and new field monitoring programs that evaluate and track ecosystem integrity components as they are impacted by climate and human land use at various scales.
More focused scientific studies on the components of ecosystem integrity as described here (Figure 1) are needed to better define, quantify, and monitor integrity in different ecoregions. For example, we know relatively little about how biodiversity and ecosystem composition in many forested regions globally is responding to the combined impacts of climate change, landscape fragmentation, and land use, nor how these will continue to evolve in the future. Such understanding would facilitate management decisions to increase ecosystem integrity or limit its decline, which is particularly important for managing future risks and vulnerability of carbon stocks in the context of carbon markets and offsets (Anderegg et al., 2020). Developing methods for comprehensive yet transferable ecosystem service valuations are particularly important for both scientific understanding as well as conservation mechanisms such as Payments for Ecosystem Services and the UN System of Environmental Ecosystem Accounting.
Finally, we suggest prioritizing research that optimizes the distribution of secondary forest management, including intensive plantations, to alleviate the pressure on primary and high integrity natural forests worldwide, as well as policy mechanisms needed for incentivization. Such research needs to account for regionally varying economic and equity issues in order to be effective.
Conclusion
In this paper we reviewed the components, importance, and potential for ecosystem integrity to help guide international forest policy and foster greater policy coherence across the climate, biodiversity, and sustainable development sectors. Our operating framework for forest ecosystem integrity encompasses biodiversity, dissipative structures, ecosystem processes, ecosystem stability, and the resulting ecosystem condition and services. A comparative analysis showed that, compared to forests with significant human modification, primary forests generally have higher ecosystem integrity and thus lower risk profiles for climate mitigation.
The scientific and management communities need better tools to accurately forecast the risks associated with different forest ecosystems, particularly those being managed for natural climate solutions and mitigation (Anderegg et al., 2020). Given these tools may be years or more away, we suggest focusing on ecosystem integrity is an optimal solution for categorizing forest-based risks and protecting ecosystem services. Doing so would (i) optimize investment in land carbon stocks and mitigation potential, (ii) identify stocks that provide the best insurance against risk of loss, and (iii) ensure the highest levels of benefits from ecosystem services, thereby optimizing compatibility and synergy between mitigation, adaptation, and SDGs. A number of large-scale data products exist to guide this focus. Nevertheless, there are substantial remaining gaps in terms of understanding, mapping, monitoring, and forecasting forest ecosystem integrity and its components in the midst of increasing human pressure and climate changes. Because primary forests have a higher level of ecosystem integrity than forests managed for commodity production, plantations, or degraded forests, we stress the continuing and increased need for their protection. An effective strategy is to create high carbon density strategic carbon and biodiversity reserves that include primary forests and recovering secondary forests that are quickly accumulating carbon (Law et al., 2022).
Author contributions
BR, BM, VY, and HK conceived the study. BR, BM, and HK led the writing, with contributions from CK, DD, GB, JD, RH, RB, TS, VY, and WM. All authors contributed to the article and approved the submitted version.
Funding
This research reported here was supported in part by a grant from a charitable organization which neither seeks nor permits publicity for its efforts. The grantor has had no influence on the design, analysis, interpretation, and documentation of this research.
Conflict of interest
The authors declare that the research was conducted in the absence of any commercial or financial relationships that could be construed as a potential conflict of interest.
Publisher’s note
All claims expressed in this article are solely those of the authors and do not necessarily represent those of their affiliated organizations, or those of the publisher, the editors and the reviewers. Any product that may be evaluated in this article, or claim that may be made by its manufacturer, is not guaranteed or endorsed by the publisher.
References
Abatzoglou, J. T., Williams, A. P., and Barbero, R. (2019). Global emergence of anthropogenic climate change in fire weather indices. Geophys. Res. Lett. 46, 326–336. doi: 10.1029/2018GL080959
Abernethy, K. A., Coad, L., Taylor, G., Lee, M. E., and Maisels, F. (2013). Extent and ecological consequences of hunting in Central African rainforests in the twenty-first century. Philos. Trans. R. Soc. B Biol. Sci. 368:20120303. doi: 10.1098/rstb.2012.0303
Achard, F., Eva, H. D., Mollicone, D., and Beuchle, R. (2008). The effect of climate anomalies and human ignition factor on wildfires in Russian boreal forests. Philos. Trans. R. Soc. B Biol. Sci. 363, 2331–2339. doi: 10.1098/rstb.2007.2203
Adeney, J. M., Christensen, N. L., and Pimm, S. L. (2009). Reserves protect against deforestation fires in the Amazon. PLoS One 4:e5014. doi: 10.1371/journal.pone.0005014
Ahmed, S. E., Ewers, R. M., and Smith, M. J. (2014). Large scale spatio-temporal patterns of road development in the Amazon rainforest. Environ. Conserv. 41, 253–264. doi: 10.1017/S0376892913000520
Ajani, J. I., Keith, H., Blakers, M., Mackey, B. G., and King, H. P. (2013). Comprehensive carbon stock and flow accounting: A national framework to support climate change mitigation policy. Ecol. Econ. 89, 61–72. doi: 10.1016/j.ecolecon.2013.01.010
Alamgir, M., Campbell, M. J., Sloan, S., Goosem, M., Clements, G. R., Mahmoud, M. I., et al. (2017). Economic, socio-political and environmental risks of road development in the tropics. Curr. Biol. 27, R1130–R1140. doi: 10.1016/j.cub.2017.08.067
Alkama, R., and Cescatti, A. (2016). Biophysical climate impacts of recent changes in global forest cover. Science 351, 600–604. doi: 10.1126/science.aac8083
Allen, C. D., Breshears, D. D., and McDowell, N. G. (2015). On underestimation of global vulnerability to tree mortality and forest die-off from hotter drought in the Anthropocene. Ecosphere 6:129. doi: 10.1890/ES15-00203.1
Allen, C. D., Macalady, A. K., Chenchouni, H., Bachelet, D., McDowell, N., Vennetier, M., et al. (2010). A global overview of drought and heat-induced tree mortality reveals emerging climate change risks for forests. For. Ecol. Manag. 259, 660–684. doi: 10.1016/j.foreco.2009.09.001
Alroy, J. (2017). Effects of habitat disturbance on tropical forest biodiversity. Proc. Natl. Acad. Sci. U.S.A. 114, 6056–6061. doi: 10.1073/pnas.1611855114
Andela, N., Morton, D. C., Giglio, L., Chen, Y., van der Werf, G. R., Kasibhatla, P. S., et al. (2017). A human-driven decline in global burned area. Science 356, 1356–1361. doi: 10.1126/science.aal4108
Anderegg, W. R. L., and Callaway, E. S. (2012). Infestation and hydraulic consequences of induced carbon starvation. Plant Physiol. 159, 1866–1874. doi: 10.1104/pp.112.198424
Anderegg, W. R. L., Hicke, J. A., Fisher, R. A., Allen, C. D., Aukema, J., Bentz, B., et al. (2015). Tree mortality from drought, insects, and their interactions in a changing climate. New Phytol. 208, 674–683. doi: 10.1111/nph.13477
Anderegg, W. R. L., Kane, J. M., and Anderegg, L. D. L. (2013). Consequences of widespread tree Mortality triggered by drought and temperature stress. Nat. Clim. Change 3, 30–36.
Anderegg, W. R. L., Trugman, A. T., Badgley, G., Anderson, C. M., Bartuska, A., Ciais, P., et al. (2020). Climate-driven risks to the climate mitigation potential of forests. Science 368:eaaz7005. doi: 10.1126/science.aaz7005
Andreasen, J. K., O’Neill, R. V., Noss, R., and Slosser, N. C. (2001). Considerations for the development of a terrestrial index of ecological integrity. Ecol. Indic. 1, 21–35. doi: 10.1016/S1470-160X(01)00007-3
Angeler, D. G., Fried-Petersen, H. B., Allen, C. R., Garmestani, A., Twidwell, D., Chuang, W.-C., et al. (2019). “Chapter one - adaptive capacity in ecosystems,” in Advances in ecological research resilience in complex socio-ecological systems, eds D. A. Bohan and A. J. Dumbrell (Cambridge, MA: Academic Press), 1–24. doi: 10.1016/bs.aecr.2019.02.001
Archibald, S., Lehmann, C. E. R., Gomez-Dans, J. L., and Bradstock, R. A. (2013). Defining pyromes and global syndromes of fire regimes. Proc. Natl. Acad. Sci. U.S.A. 110, 6442–6447. doi: 10.1073/pnas.1211466110
Arima, E. Y., Walker, R. T., Perz, S., and Souza, C. (2016). Explaining the fragmentation in the Brazilian Amazonian forest. J. Land Use Sci. 11, 257–277. doi: 10.1080/1747423X.2015.1027797
Arima, E. Y., Walker, R. T., Sales, M., Souza, C., and Perz, S. G. (2008). The fragmentation of space in the Amazon basin: Emergent road networks. Photogramm. Eng. Remote Sens. 74, 699–709. doi: 10.14358/PERS.74.6.699
Asner, G. P., Broadbent, E. N., Oliveira, P. J. C., Keller, M., Knapp, D. E., and Silva, J. N. M. (2006). Condition and fate of logged forests in the Brazilian Amazon. Proc. Natl. Acad. Sci. U.S.A. 103, 12947–12950. doi: 10.1073/pnas.0604093103
Asner, G. P., Keller, M., and Silva, J. N. M. (2004). Spatial and temporal dynamics of forest canopy gaps following selective logging in the eastern Amazon. Glob. Change Biol. 10, 765–783. doi: 10.1111/j.1529-8817.2003.00756.x
Asner, G. P., Powell, G. V. N., Mascaro, J., Knapp, D. E., Clark, J. K., Jacobson, J., et al. (2010). High-resolution forest carbon stocks and emissions in the Amazon. Proc. Natl. Acad. Sci. U.S.A. 107, 16738–16742. doi: 10.1073/pnas.1004875107
Au, J., Clark, R. G., Allen, C., Marsh, K. J., Foley, W. J., and Youngentob, K. N. (2019). A nutritional mechanism underpinning folivore occurrence in disturbed forests. For. Ecol. Manag. 453:117585. doi: 10.1016/j.foreco.2019.117585
Austin, K. G., Schwantes, A., Gu, Y., and Kasibhatla, P. S. (2019). What causes deforestation in Indonesia? Environ. Res. Lett. 14:024007. doi: 10.1088/1748-9326/aaf6db
Australian Government (1999). Environment protection and biodiversity conservation act 1999. Canberra, ACT: Australian Government Federal Register of Legislation.
Baccini, A., Walker, W., Carvalho, L., Farina, M., Sulla-Menashe, D., and Houghton, R. A. (2017). Tropical forests are a net carbon source based on aboveground measurements of gain and loss. Science 358, 230–233. doi: 10.1126/science.aam5962
Baier, P., Fuhrer, E., Kirisits, T., and Rosner, S. (2002). Defence reactions of Norway spruce against bark beetles and the associated fungus Ceratocystis polonica in secondary pure and mixed species stands. For. Ecol. Manag. 159, 73–86. doi: 10.1016/S0378-1127(01)00711-3
Balch, J. K., Bradley, B. A., Abatzoglou, J. T., Nagy, R. C., Fusco, E. J., and Mahood, A. L. (2017). Human-started wildfires expand the fire niche across the United States. Proc. Natl. Acad. Sci. U.S.A. 114, 2946–2951. doi: 10.1073/pnas.1617394114
Barber, C. P., Cochrane, M. A., Souza, C. M., and Laurance, W. F. (2014). Roads, deforestation, and the mitigating effect of protected areas in the Amazon. Biol. Conserv. 177, 203–209. doi: 10.1016/j.biocon.2014.07.004
Barber, C. V., Petersen, R., Young, V., Mackey, B., and Kormos, C. (2020). Thenexus report: Nature based solutions to the biodiversity and climate crisis. F20 foundations, campaign for nature and SEE foundation. Available online at: https://nature4climate.org/articles/the-nexus-report-nature-based-solutions-to-the-biodiversity-and-climate-crisis/ (accessed January 22, 2021).
Barlow, J., Gardner, T. A., Araujo, I. S., Avila-Pires, T. C., Bonaldo, A. B., Costa, J. E., et al. (2007). Quantifying the biodiversity value of tropical primary, secondary, and plantation forests. Proc. Natl. Acad. Sci. U.S.A. 104, 18555–18560. doi: 10.1073/pnas.0703333104
Barlow, J., Lennox, G. D., Ferreira, J., Berenguer, E., Lees, A. C., Mac Nally, R., et al. (2016). Anthropogenic disturbance in tropical forests can double biodiversity loss from deforestation. Nature 535, 144–147. doi: 10.1038/nature18326
Bawa, K. S., and Seidler, R. (1998). Natural forest management and conservation of biodiversity in tropical forests. Conserv. Biol. 12, 46–55. doi: 10.1046/j.1523-1739.1998.96480.x
Bennett, A. C., McDowell, N. G., Allen, C. D., and Anderson-Teixeira, K. J. (2015). Larger trees suffer most during drought in forests worldwide. Nat. Plants 1:15139. doi: 10.1038/NPLANTS.2015.139
Betts, M. G., Yang, Z., Hadley, A. S., Smith, A. C., Rousseau, J. S., Northrup, J. M., et al. (2022). Forest degradation drives widespread avian habitat and population declines. Nat. Ecol. Evol. 6, 709–719. doi: 10.1038/s41559-022-01737-8
Beyer, H. L., Venter, O., Grantham, H. S., and Watson, J. E. M. (2020). Substantial losses in ecoregion intactness highlight urgency of globally coordinated action. Conserv. Lett. 13:e12592. doi: 10.1111/conl.12692
Birdsey, R., Duffy, P., Smyth, C., Akurz, W., Dugan, A. J., and Houghton, R. (2018). Climate, economic, and environmental impacts of producing wood for bioenergy. Environ. Res. Lett. 13:050201. doi: 10.1088/1748-9326/aab9d5
Boakes, E. H., Mace, G. M., McGowan, P. J. K., and Fuller, R. A. (2010). Extreme contagion in global habitat clearance. Proc. R. Soc. B Biol. Sci. 277, 1081–1085. doi: 10.1098/rspb.2009.1771
Boot, R., and Gullison, R. (1995). Approaches to developing sustainable extraction systems for tropical forest products. Ecol. Appl. 5, 896–903. doi: 10.2307/2269340
Booth, M. S. (2018). Not carbon neutral: Assessing the net emissions impact of residues burned for bioenergy. Environ. Res. Lett. 13:035001. doi: 10.1088/1748-9326/aaac88
Bourgoin, C., Betbeder, J., Couteron, P., Blanc, L., Dessard, H., Oszwald, J., et al. (2020). UAV-based canopy textures assess changes in forest structure from long-term degradation. Ecol. Indic. 115:106386. doi: 10.1016/j.ecolind.2020.106386
Bowman, D. M. J. S., Williamson, G. J., Abatzoglou, J. T., Kolden, C. A., Cochrane, M. A., and Smith, A. M. S. (2017). Human exposure and sensitivity to globally extreme wildfire events. Nat. Ecol. Evol. 1:0058. doi: 10.1038/s41559-016-0058
Bradley, C. M., Hanson, C. T., and DellaSala, D. A. (2016). Does increased forest protection correspond to higher fire severity in frequent-fire forests of the western United States? Ecosphere 7:e01492. doi: 10.1002/ecs2.1492
Bradshaw, C. J. A., and Warkentin, I. G. (2015). Global estimates of boreal forest carbon stocks and flux. Glob. Planet. Change 128, 24–30. doi: 10.1016/j.gloplacha.2015.02.004
Brando, P. M., Balch, J. K., Nepstad, D. C., Morton, D. C., Putz, F. E., Coe, M. T., et al. (2014). Abrupt increases in Amazonian tree mortality due to drought-fire interactions. Proc. Natl. Acad. Sci. U.S.A. 111, 6347–6352. doi: 10.1073/pnas.1305499111
Brandt, P., Abson, D. J., DellaSala, D. A., Feller, R., and von Wehrden, H. (2014). Multifunctionality and biodiversity: Ecosystem services in temperate rainforests of the Pacific Northwest, USA. Biol. Conserv. 169, 362–371. doi: 10.1016/j.biocon.2013.12.003
Briant, G., Gond, V., and Laurance, S. G. W. (2010). Habitat fragmentation and the desiccation of forest canopies: A case study from eastern Amazonia. Biol. Conserv. 143, 2763–2769. doi: 10.1016/j.biocon.2010.07.024
Brienen, R. J. W., Gloor, E., Clerici, S., Newton, R., Arppe, L., Boom, A., et al. (2017). Tree height strongly affects estimates of water-use efficiency responses to climate and CO2 using isotopes. Nat. Commun. 8:288. doi: 10.1038/s41467-017-00225-z
Broadbent, E. N., Asner, G. P., Keller, M., Knapp, D. E., Oliveira, P. J. C., and Silva, J. N. (2008). Forest fragmentation and edge effects from deforestation and selective logging in the Brazilian Amazon. Biol. Conserv. 141, 1745–1757. doi: 10.1016/j.biocon.2008.04.024
Brockerhoff, E. G., Barbaro, L., Castagneyrol, B., Forrester, D. I., Gardiner, B., Ramon Gonzalez-Olabarria, J., et al. (2017). Forest biodiversity, ecosystem functioning and the provision of ecosystem services. Biodivers. Conserv. 26, 3005–3035. doi: 10.1007/s10531-017-1453-2
Bryan, B. A., Runting, R. K., Capon, T., Perring, M. P., Cunningham, S. C., Kragt, M. E., et al. (2016). Designer policy for carbon and biodiversity co-benefits under global change. Nat. Clim. Change 6, 301–305. doi: 10.1038/NCLIMATE2874
Buchholz, T., Friedland, A. J., Hornig, C. E., Keeton, W. S., Zanchi, G., and Nunery, J. (2014). Mineral soil carbon fluxes in forests and implications for carbon balance assessments. Glob. Change Biol. Bioenergy 6, 305–311. doi: 10.1111/gcbb.12044
Buchwald, E. (2005). “A hierarchical terminology for more or less natural forests in relation to sustainable management and biodiversity conservation,” in Proceedings of the third expert meeting on harmonizing forest-related definitions for use by various stakeholders, (Rome: Food and Agriculture Organization of the United Nations), 17–19.
Bullock, E. L., Woodcock, C. E., Souza, C. Jr., and Olofsson, P. (2020). Satellite-based estimates reveal widespread forest degradation in the Amazon. Glob. Change Biol. 26, 2956–2969. doi: 10.1111/gcb.15029
Burrascano, S., Keeton, W. S., Sabatini, F. M., and Blasi, C. (2013). Commonality and variability in the structural attributes of moist temperate old-growth forests: A global review. For. Ecol. Manag. 291, 458–479. doi: 10.1016/j.foreco.2012.11.020
Burton, P. J., Messier, C., Adamowicz, W. L., and Kuuluvainen, T. (2006). Sustainable management of Canada’s boreal forests: Progress and prospects. Ecoscience 13, 234–248. doi: 10.2980/i1195-6860-13-2-234.1
Cadotte, M. W., Carscadden, K., and Mirotchnick, N. (2011). Beyond species: Functional diversity and the maintenance of ecological processes and services. J. Appl. Ecol. 48, 1079–1087. doi: 10.1111/j.1365-2664.2011.02048.x
Caioni, C., Silverio, D. V., Macedo, M. N., Coe, M. T., and Brando, P. M. (2020). Droughts amplify differences between the energy balance components of amazon forests and croplands. Remote Sens. 12:525. doi: 10.3390/rs12030525
Cairns, M., and Meganck, R. (1994). Carbon sequestration, biological diversity, and sustainable development - integrated forest management. Environ. Manage. 18, 13–22. doi: 10.1007/BF02393746
Campbell, A., Kapos, V., Scharlemann, J. P. W., Bubb, P., Chenery, A., Coad, L., et al. (2009). Review of the literature on the links between biodiversity and climate change: Impacts, adaptation and mitigation. Montreal: Secretariat of the Convention on Biological Diversity.
Campbell, D. E. (2000). Using energy systems theory to define, measure, and interpret ecological integrity and ecosystem health. Ecosyst. Health 6, 181–204. doi: 10.1046/j.1526-0992.2000.006003181.x
Cannon, C. H., Peart, D. R., and Leighton, M. (1998). Tree species diversity in commercially logged bornean rainforest. Science 281, 1366–1368. doi: 10.1126/science.281.5381.1366
Cardinale, B. J., Duffy, J. E., Gonzalez, A., Hooper, D. U., Perrings, C., Venail, P., et al. (2012). Biodiversity loss and its impact on humanity. Nature 486, 59–67. doi: 10.1038/nature11148
Carignan, R., D’Arcy, P., and Lamontagne, S. (2000). Comparative impacts of fire and forest harvesting on water quality in Boreal Shield lakes. Can. J. Fish. Aquat. Sci. 57, 105–117. doi: 10.1139/cjfas-57-S2-105
Carnicer, J., Coll, M., Ninyerola, M., Pons, X., Sanchez, G., and Penuelas, J. (2011). Widespread crown condition decline, food web disruption, and amplified tree mortality with increased climate change-type drought. Proc. Natl. Acad. Sci. U.S.A. 108, 1474–1478. doi: 10.1073/pnas.1010070108
Catterall, C. P. (2016). Roles of non-native species in large-scale regeneration of moist tropical forests on anthropogenic grassland. Biotropica 48, 809–824. doi: 10.1111/btp.12384
Center for Biological Diversity [CBD] (2022). Biden launches process for protecting mature, old-growth forests on federal lands. Tucson, AZ: Center for Biological Diversity.
Chapin, F. S., Walker, B. H., Hobbs, R. J., Hooper, D. U., Lawton, J. H., Sala, O. E., et al. (1997). Biotic control over the functioning of ecosystems. Science 277, 500–504. doi: 10.1126/science.277.5325.500
Chaplin-Kramer, R., Ramler, I., Sharp, R., Haddad, N. M., Gerber, J. S., West, P. C., et al. (2015). Degradation in carbon stocks near tropical forest edges. Nat. Commun. 6:10158. doi: 10.1038/ncomms10158
Chapman, M., Walker, W. S., Cook-Patton, S. C., Ellis, P. W., Farina, M., Griscom, B. W., et al. (2020). Large climate mitigation potential from adding trees to agricultural lands. Glob. Change Biol. 26, 4357–4365. doi: 10.1111/gcb.15121
Chazdon, R. L., and Uriarte, M. (2016). Natural regeneration in the context of large-scale forest and landscape restoration in the tropics. Biotropica 48, 709–715. doi: 10.1111/btp.12409
Chen, H. Y. H., Luo, Y., Reich, P. B., Searle, E. B., and Biswas, S. R. (2016). Climate change-associated trends in net biomass change are age dependent in western boreal forests of Canada. Ecol. Lett. 19, 1150–1158. doi: 10.1111/ele.12653
Cherubini, F., Peters, G. P., Berntsen, T., Stromman, A. H., and Hertwich, E. (2011). CO2 emissions from biomass combustion for bioenergy: Atmospheric decay and contribution to global warming. Glob. Change Biol. Bioenergy 3, 413–426. doi: 10.1111/j.1757-1707.2011.01102.x
Christiansen, E., and Horntvedt, R. (1983). Combined Ips/ceratocystis attack on Norway spruce, and defensive-mechanisms of the trees. Z. Angew. Entomol. J. Appl. Entomol. 96, 110–118.
Clark, J. S., Iverson, L., Woodall, C. W., Allen, C. D., Bell, D. M., Bragg, D. C., et al. (2016). The impacts of increasing drought on forest dynamics, structure, and biodiversity in the United States. Glob. Change Biol. 22, 2329–2352. doi: 10.1111/gcb.13160
Clements, G. R., Lynam, A. J., Gaveau, D., Yap, W. L., Lhota, S., Goosem, M., et al. (2014). Where and how are roads endangering mammals in Southeast Asia’s forests? PLoS One 9:e115376. doi: 10.1371/journal.pone.0115376
Climate Action Network (2022). Submission for the first input phase of the global stocktake. Bonn: CAN International.
Cochrane, M. A., Alencar, A., Schulze, M. D., Souza, C. M., Nepstad, D. C., Lefebvre, P., et al. (1999). Positive feedbacks in the fire dynamic of closed canopy tropical forests. Science 284, 1832–1835. doi: 10.1126/science.284.5421.1832
Collingham, Y. C., and Huntley, B. (2000). Impacts of habitat fragmentation and patch size upon migration rates. Ecol. Appl. 10, 131–144. doi: 10.2307/2640991
Committee of Experts on Environmental-Economic Accounting (2021). System of environmental-economic accounting - ecosystem accounting. New York, NY: United Nations Department of Economic and Social Affairs.
Convention on Biological Diversity [CBD] (2018). COP decisions. Available Online at: https://www.cbd.int/decisions/cop/?m=cop-14 [accessed September 30, 2022].
Convention on Biological Diversity [CBD] (2021). First draft of the post-2020 global biodiversity framework. Available Online at: https://www.cbd.int/article/draft-1-global-biodiversity-framework (accessed November 12, 2021).
Cook, B. I., Smerdon, J. E., Seager, R., and Coats, S. (2014). Global warming and 21st century drying. Clim. Dyn. 43, 2607–2627. doi: 10.1007/s00382-014-2075-y
Cook-Patton, S. C., Leavitt, S. M., Gibbs, D., Harris, N. L., Lister, K., Anderson-Teixeira, K. J., et al. (2020). Mapping carbon accumulation potential from global natural forest regrowth. Nature 585, 545–550. doi: 10.1038/s41586-020-2686-x
Creutzig, F., Ravindranath, N. H., Berndes, G., Bolwig, S., Bright, R., Cherubini, F., et al. (2015). Bioenergy and climate change mitigation: An assessment. Glob. Change Biol. Bioenergy 7, 916–944. doi: 10.1111/gcbb.12205
Curtis, P. G., Slay, C. M., Harris, N. L., Tyukavina, A., and Hansen, M. C. (2018). Classifying drivers of global forest loss. Science 361, 1108–1111. doi: 10.1126/science.aau3445
Dale, V. H., Tharp, M. L., Lannom, K. O., and Hodges, D. G. (2010). Modeling transient response of forests to climate change. Sci. Total Environ. 408, 1888–1901. doi: 10.1016/j.scitotenv.2009.11.050
D’Amato, A. W., Bradford, J. B., Fraver, S., and Palik, B. J. (2013). Effects of thinning on drought vulnerability and climate response in north temperate forest ecosystems. Ecol. Appl. 23, 1735–1742. doi: 10.1890/13-0677.1
Davis, M. B., and Shaw, R. G. (2001). Range shifts and adaptive responses to Quaternary climate change. Science 292, 673–679. doi: 10.1126/science.292.5517.673
De Beenhouwer, M., Aerts, R., and Honnay, O. (2013). A global meta-analysis of the biodiversity and ecosystem service benefits of coffee and cacao agroforestry. Agric. Ecosyst. Environ. 175, 1–7. doi: 10.1016/j.agee.2013.05.003
de Castro Solar, R. R., Barlow, J., Ferreira, J., Berenguer, E., Lees, A. C., Thomson, J. R., et al. (2015). How pervasive is biotic homogenization in human-modified tropical forest landscapes? Ecol. Lett. 18, 1108–1118. doi: 10.1111/ele.12494
De Faria, B. L., Marano, G., Piponiot, C., Silva, C. A., Dantas, V., de, L., et al. (2021). Model-based estimation of Amazonian forests recovery time after drought and fire events. Forests 12:8. doi: 10.3390/f12010008
De Leo, G. A., and Levin, S. (1997). The multifaceted aspects of ecosystem integrity. Conserv. Ecol. 1:3.
de Souza, F. C., Dexter, K. G., Phillips, O. L., Pennington, R. T., Neves, D., Sullivan, M. J. P., et al. (2019). Evolutionary diversity is associated with wood productivity in Amazonian forests. Nat. Ecol. Evol. 3, 1754–1761. doi: 10.1038/s41559-019-1007-y
Debeljak, M. (2006). Coarse woody debris in virgin and managed forest. Ecol. Indic. 6, 733–742. doi: 10.1016/j.ecolind.2005.08.031
DeHayes, D. H., Jacobson, G. L. Jr., Schaberg, P. G., Bongarten, B., Iverson, L., and Dieffenbacher-Krall, A. C. (2000). “Forest responses to changing climate: Lessons from the past and uncertainty for the future,” in Responses of northern US forests to environmental change ecological studies, eds R. A. Mickler, R. A. Birdsey, and J. Hom (New York, NY: Springer), 495–540.
DellaSala, D. A. (2011). Temperate and boreal rainforests of the world: Ecology and conservation. Washington, DC: Island Press.
DellaSala, D. A., Baker, B. C., Hanson, C. T., Ruediger, L., and Baker, W. (2022a). Have western USA fire suppression and megafire active management approaches become a contemporary Sisyphus? Biol. Conserv. 268:109499. doi: 10.1016/j.biocon.2022.109499
DellaSala, D. A., Mackey, B., Norman, P., Campbell, C., Comer, P. J., Kormos, C. F., et al. (2022b). Mature and old-growth forests contribute to large-scale conservation targets in the conterminous United States. Front. For. Glob. Change 5:979528. doi: 10.3389/ffgc.2022.979528
Deluca, T. H., and Boisvenue, C. (2012). Boreal forest soil carbon: Distribution, function and modelling. Forestry 85, 161–184. doi: 10.1093/forestry/cps003
Deo, R. C., Syktus, J. I., McAlpine, C. A., Lawrence, P. J., McGowan, H. A., and Phinn, S. R. (2009). Impact of historical land cover change on daily indices of climate extremes including droughts in eastern Australia. Geophys. Res. Lett. 36:L08705. doi: 10.1029/2009GL037666
Devictor, V., Julliard, R., Clavel, J., Jiguet, F., Lee, A., and Couvet, D. (2008). Functional biotic homogenization of bird communities in disturbed landscapes. Glob. Ecol. Biogeogr. 17, 252–261. doi: 10.1111/j.1466-8238.2007.00364.x
Di Marco, M., Watson, J. E. M., Currie, D. J., Possingham, H. P., and Venter, O. (2018). The extent and predictability of the biodiversity-carbon correlation. Ecol. Lett. 21, 365–375. doi: 10.1111/ele.12903
Diaz, S., and Cabido, M. (2001). Vive la difference: Plant functional diversity matters to ecosystem processes. Trends Ecol. Evol. 16, 646–655. doi: 10.1016/S0169-5347(01)02283-2
Didham, R. K., and Lawton, J. H. (1999). Edge structure determines the magnitude of changes in microclimate and vegetation structure in tropical forest fragments. Biotropica 31, 17–30. doi: 10.1111/j.1744-7429.1999.tb00113.x
Dieleman, C. M., Rogers, B. M., Potter, S., Veraverbeke, S., Johnstone, J. F., Laflamme, J., et al. (2020). Wildfire combustion and carbon stocks in the southern Canadian boreal forest: Implications for a warming world. Glob. Change Biol. 26, 6062–6079. doi: 10.1111/gcb.15158
Donato, D. C., Fontaine, J. B., Campbell, J. L., Robinson, W. D., Kauffman, J. B., and Law, B. E. (2006). Post-wildfire logging hinders regeneration and increases fire risk. Science 311, 352–352. doi: 10.1126/science.1122855
Dooley, K., Stabinsky, D., Stone, K., Sharma, S., Anderson, T., Gurian-Sherman, D., et al. (2018). Missing pathways to 1.5°C: The role of the land sector in ambitious climate action. Climate land ambition and rights alliance. Available Online at: climatelandambitionrightsalliance.org/report (accessed January 22, 2021).
Dorren, L. K. A., Berger, F., Imeson, A. C., Maier, B., and Rey, F. (2004). Integrity, stability and management of protection forests in the European Alps. For. Ecol. Manag. 195, 165–176. doi: 10.1016/j.foreco.2004.02.057
Dowdy, A. J. (2018). Climatological variability of fire weather in Australia. J. Appl. Meteorol. Climatol. 57, 221–234. doi: 10.1175/JAMC-D-17-0167.1
Dowdy, A. J., Ye, H., Pepler, A., Thatcher, M., Osbrough, S. L., Evans, J. P., et al. (2019). Future changes in extreme weather and pyroconvection risk factors for Australian wildfires. Sci. Rep. 9:10073. doi: 10.1038/s41598-019-46362-x
Drever, C. R., Peterson, G., Messier, C., Bergeron, Y., and Flannigan, M. (2006). Can forest management based on natural disturbances maintain ecological resilience? Can. J. For. Res. 36, 2285–2299. doi: 10.1139/X06-132
Dudley, N., and Stolton, S. (2003). Running pure: The importance of forest protected areas to drinking water. Number 15006. Washington, DC: The World Bank.
Duffy, J. E., Godwin, C. M., and Cardinale, B. J. (2017). Biodiversity effects in the wild are common and as strong as key drivers of productivity. Nature 549, 261–264. doi: 10.1038/nature23886
Dyer, S. J., O’Neill, J. P., Wasel, S. M., and Boutin, S. (2002). Quantifying barrier effects of roads and seismic lines on movements of female woodland caribou in Northeastern Alberta. Can. J. Zool. Rev. Can. Zool. 80, 839–845. doi: 10.1139/Z02-060
Dymond, C. C., Neilson, E. T., Stinson, G., Porter, K., MacLean, D. A., Gray, D. R., et al. (2010). Future spruce budworm outbreak may create a carbon source in eastern Canadian forests. Ecosystems 13, 917–931. doi: 10.1007/s10021-010-9364-z
Edwards, D. P., Larsen, T. H., Docherty, T. D. S., Ansell, F. A., Hsu, W. W., Derhe, M. A., et al. (2011). Degraded lands worth protecting: The biological importance of Southeast Asia’s repeatedly logged forests. Proc. R. Soc. B Biol. Sci. 278, 82–90. doi: 10.1098/rspb.2010.1062
Ehrlen, J., and Morris, W. F. (2015). Predicting changes in the distribution and abundance of species under environmental change. Ecol. Lett. 18, 303–314. doi: 10.1111/ele.12410
Elias, F., Ferreira, J., Lennox, G. D., Berenguer, E., Ferreira, S., Schwartz, G., et al. (2020). Assessing the growth and climate sensitivity of secondary forests in highly deforested Amazonian landscapes. Ecology 101:e02954. doi: 10.1002/ecy.2954
Elith, J., and Leathwick, J. R. (2009). “Species distribution models: Ecological explanation and prediction across space and time,” in Proceedings of the annual review of ecology evolution and systematics, (Palo Alto, CA: Annual Reviews), 677–697. doi: 10.1111/gcb.12548
Ellison, A. M., Bank, M. S., Clinton, B. D., Colburn, E. A., Elliott, K., Ford, C. R., et al. (2005). Loss of foundation species: Consequences for the structure and dynamics of forested ecosystems. Front. Ecol. Environ. 3, 479–486.
Ellison, D., Morris, C. E., Locatelli, B., Sheil, D., Cohen, J., Murdiyarso, D., et al. (2017). Trees, forests and water: Cool insights for a hot world. Glob. Environ. Change Hum. Policy Dimens. 43, 51–61. doi: 10.1016/j.gloenvcha.2017.01.002
Erb, K.-H., Kastner, T., Plutzar, C., Bais, A. L. S., Carvalhais, N., Fetzel, T., et al. (2018). Unexpectedly large impact of forest management and grazing on global vegetation biomass. Nature 553, 73–76. doi: 10.1038/nature25138
Esseen, P.-A., Ehnström, B., Ericson, L., and Sjöberg, K. (1997). Boreal forests. Ecol. Bull. 46, 16–47.
European Parliament (2020). Green deal: Measures to step up the fight against global deforestation | news |. Strasbourg: European Parliament.
Faivre, N. R., Jin, Y., Goulden, M. L., and Randerson, J. T. (2016). Spatial patterns and controls on burned area for two contrasting fire regimes in Southern California. Ecosphere 7:e01210. doi: 10.1002/ecs2.1210
Federal Register (2022). Request for Information (RFI) on federal old-growth and mature forests. US department of agriculture, forest service, department of the interior, and bureau of land management. Washington, DC: Bureau of Land Management.
Federici, S., Lee, D., and Herold, M. (2017). Forest mitigation: A permanent contribution to the Paris agreement?. Available online at: http://www.climateandlandusealliance.org/wp-content/uploads/2017/10/Forest_Mitigation_A_Permanent_Contribution_to_Paris_Agreement.pdf (accessed May 18, 2020).
Ferreira-Leite, F., Bento-Goncalves, A. J., Vieira, A. A. B., and da Vinha, L. (2015). “Mega-fires around the world: A literature review,” in Wildland fires - a worldwide reality, eds A. J. Bento-Goncalves and A. A. B. Vieira (Hauppauge, NY: Nova Science Publishers, Inc), 15–33.
Flannigan, M., Cantin, A. S., de Groot, W. J., Wotton, M., Newbery, A., and Gowman, L. M. (2013). Global wildland fire season severity in the 21st century. For. Ecol. Manag. 294, 54–61. doi: 10.1016/j.foreco.2012.10.022
Foley, J. A., Asner, G. P., Costa, M. H., Coe, M. T., DeFries, R., Gibbs, H. K., et al. (2007). Amazonia revealed: Forest degradation and loss of ecosystem goods and services in the Amazon Basin. Front. Ecol. Environ. 5, 25–32.
Foley, J. A., Levis, S., Costa, M. H., Cramer, W., and Pollard, D. (2000). Incorporating dynamic vegetation cover within global climate models. Ecol. Appl. 10, 1620–1632. doi: 10.2307/2641227
Food and Agriculture Organization of the United Nations [FAO] (2012). The Russian federation forest sector: Outlook study to 2030. Rome: FAO.
Food and Agriculture Organization of the United Nations [FAO] (2020). Global forest resources assessment 2020: Main report. Rome: FAO.
Forsell, N., Turkovska, O., Gusti, M., Obersteiner, M., den Elzen, M., and Havlik, P. (2016). Assessing the INDCs’ land use, land use change, and forest emission projections. Carbon Balance Manag. 11:26. doi: 10.1186/s13021-016-0068-3
Frair, J. L., Merrill, E. H., Beyer, H. L., and Morales, J. M. (2008). Thresholds in landscape connectivity and mortality risks in response to growing road networks. J. Appl. Ecol. 45, 1504–1513. doi: 10.1111/j.1365-2664.2008.01526.x
Franklin, J. F., Lindenmayer, D., MacMahon, J. A., McKee, A., Magnuson, J., Perry, D. A., et al. (2000). Threads of continuity. Conserv. Pract. 1, 8–17. doi: 10.1111/j.1526-4629.2000.tb00155.x
Frey, S. J. K., Hadley, A. S., Johnson, S. L., Schulze, M., Jones, J. A., and Betts, M. G. (2016). Spatial models reveal the microclimatic buffering capacity of old-growth forests. Sci. Adv. 2:e1501392. doi: 10.1126/sciadv.1501392
Friedlingstein, P., Jones, M. W., O’Sullivan, M., Andrew, R. M., Hauck, J., Peters, G. P., et al. (2019). Global carbon budget 2019. Earth Syst. Sci. Data 11, 1783–1838. doi: 10.5194/essd-11-1783-2019
Funk, J. M., Aguilar-Amuchastegui, N., Baldwin-Cantello, W., Busch, J., Chuvasov, E., Evans, T., et al. (2019). Securing the climate benefits of stable forests. Clim. Policy 19, 845–860. doi: 10.1080/14693062.2019.1598838
Furniss, M. J., Staab, B. P., Hazelhurst, S., Clifton, C. F., Roby, K. B., Ilhadrt, B. L., et al. (2010). Water, climate change, and forests: Watershed stewardship for a changing climate. Portland, OR: U.S. Department of Agriculture.
Fuss, S., Canadell, J. G., Peters, G. P., Tavoni, M., Andrew, R. M., Ciais, P., et al. (2014). COMMENTARY: Betting on negative emissions. Nat. Clim. Change 4, 850–853. doi: 10.1038/nclimate2392
Fussel, H. M., and Klein, R. J. T. (2006). Climate change vulnerability assessments: An evolution of conceptual thinking. Clim. Change 75, 301–329. doi: 10.1007/s10584-006-0329-3
Gamfeldt, L., Snall, T., Bagchi, R., Jonsson, M., Gustafsson, L., Kjellander, P., et al. (2013). Higher levels of multiple ecosystem services are found in forests with more tree species. Nat. Commun. 4:1340. doi: 10.1038/ncomms2328
Gatti, L. V., Gloor, M., Miller, J. B., Doughty, C. E., Malhi, Y., Domingues, L. G., et al. (2014). Drought sensitivity of Amazonian carbon balance revealed by atmospheric measurements. Nature 506, 76–80. doi: 10.1038/nature12957
Gatti, R. C., Castaldi, S., Lindsell, J. A., Coomes, D. A., Marchetti, M., Maesano, M., et al. (2015). The impact of selective logging and clearcutting on forest structure, tree diversity and above-ground biomass of African tropical forests. Ecol. Res. 30, 119–132. doi: 10.1007/s11284-014-1217-3
Giam, X. (2017). Global biodiversity loss from tropical deforestation. Proc. Natl. Acad. Sci. U.S.A. 114, 5775–5777. doi: 10.1073/pnas.1706264114
Giam, X., Clements, G. R., Aziz, S. A., Chong, K. Y., and Miettinen, J. (2011). Rethinking the “back to wilderness” concept for Sundaland’s forests. Biol. Conserv. 144, 3149–3152. doi: 10.1016/j.biocon.2011.10.001
Giardina, F., Konings, A. G., Kennedy, D., Alemohammad, S. H., Oliveira, R. S., Uriarte, M., et al. (2018). Tall Amazonian forests are less sensitive to precipitation variability. Nat. Geosci. 11, 405–409. doi: 10.1038/s41561-018-0133-5
Gibbs, H. K., Ruesch, A. S., Achard, F., Clayton, M. K., Holmgren, P., Ramankutty, N., et al. (2010). Tropical forests were the primary sources of new agricultural land in the 1980s and 1990s. Proc. Natl. Acad. Sci. U.S.A. 107, 16732–16737. doi: 10.1073/pnas.0910275107
Gibson, L., Lee, T. M., Koh, L. P., Brook, B. W., Gardner, T. A., Barlow, J., et al. (2011). Primary forests are irreplaceable for sustaining tropical biodiversity. Nature 478, 378–381. doi: 10.1038/nature10425
Giglio, L., Randerson, J. T., and van der Werf, G. R. (2013). Analysis of daily, monthly, and annual burned area using the fourth-generation global fire emissions database (GFED4). J. Geophys. Res. Biogeosci. 118, 317–328. doi: 10.1002/jgrg.20042
Gilbert, G. S., and Hubbell, S. P. (1996). Plant diseases and the conservation of tropical forests - conservation planners need to consider the roles diseases play in natural communities. Bioscience 46, 98–106. doi: 10.2307/1312812
Girardin, M. P., Guo, X. J., Bernier, P. Y., Raulier, F., and Gauthier, S. (2012). Changes in growth of pristine boreal North American forests from 1950 to 2005 driven by landscape demographics and species traits. Biogeosciences 9, 2523–2536. doi: 10.5194/bg-9-2523-2012
Glick, P., Stein, B., and Edelson, N. A. (2011). Scanning the conservation horizon: A guide to climate change vulnerability assessment. Washington, DC: National Wildlife Federation.
Goetz, S. J., Steinberg, D., Betts, M. G., Holmes, R. T., Doran, P. J., Dubayah, R., et al. (2010). Lidar remote sensing variables predict breeding habitat of a Neotropical migrant bird. Ecology 91, 1569–1576. doi: 10.1890/09-1670.1
Gordon, A. G. (1996). The sweep of the boreal in time and space, from forest formations to genes, and implications for management. For. Chron. 72, 19–30. doi: 10.5558/tfc72019-1
Goulden, M. L., McMillan, A. M. S., Winston, G. C., Rocha, A. V., Manies, K. L., Harden, J. W., et al. (2010). Patterns of NPP, GPP, respiration, and NEP during boreal forest succession. Glob. Change Biol. 17, 855–871. doi: 10.1111/j.1365-2486.2010.02274.x
Grace, J., Mitchard, E., and Gloor, E. (2014). Perturbations in the carbon budget of the tropics. Glob. Change Biol. 20, 3238–3255. doi: 10.1111/gcb.12600
Grantham, H. S., Duncan, A., Evans, T. D., Jones, K. R., Beyer, H. L., Schuster, R., et al. (2020). Anthropogenic modification of forests means only 40% of remaining forests have high ecosystem integrity. Nat. Commun. 11:5978. doi: 10.1038/s41467-020-19493-3
Grassi, G., House, J., Dentener, F., Federici, S., den Elzen, M., and Penman, J. (2017). The key role of forests in meeting climate targets requires science for credible mitigation. Nat. Clim. Change 7, 220–226. doi: 10.1038/NCLIMATE3227
Grimm, V., and Wissel, C. (1997). Babel, or the ecological stability discussions: An inventory and analysis of terminology and a guide for avoiding confusion. Oecologia 109, 323–334. doi: 10.1007/s004420050090
Griscom, B. W., Adams, J., Ellis, P. W., Houghton, R. A., Lomax, G., Miteva, D. A., et al. (2017). Natural climate solutions. Proc. Natl. Acad. Sci. U.S.A. 114, 11645–11650. doi: 10.1073/pnas.1710465114
Gromtsev, A. (2002). Natural disturbance dynamics in the boreal forests of European Russia: A review. Silva Fenn. 36, 41–55. doi: 10.14214/sf.549
Guby, N. A. B., and Dobbertin, M. (1996). Quantitative estimates of coarse woody debris and standing dead trees in selected Swiss forests. Glob. Ecol. Biogeogr. Lett. 5, 327–341. doi: 10.2307/2997588
Gullison, R., and Hardner, J. (1993). The effects of road design and harvest intensity on forest damage caused by selective logging - empirical results and a simulation-model from the Bosque Chimanes, Bolivia. For. Ecol. Manag. 59, 1–14. doi: 10.1016/0378-1127(93)90067-W
Guyot, V., Castagneyrol, B., Vialatte, A., Deconchat, M., and Jactel, H. (2016). Tree diversity reduces pest damage in mature forests across Europe. Biol. Lett. 12:20151037. doi: 10.1098/rsbl.2015.1037
Haddad, N. M., Brudvig, L. A., Clobert, J., Davies, K. F., Gonzalez, A., Holt, R. D., et al. (2015). Habitat fragmentation and its lasting impact on Earth’s ecosystems. Sci. Adv. 1:e1500052. doi: 10.1126/sciadv.1500052
Hamburg, S. P., Vadeboncoeur, M. A., Johnson, C. E., and Sanderman, J. (2019). Losses of mineral soil carbon largely offset biomass accumulation 15years after whole-tree harvest in a northern hardwood forest. Biogeochemistry 144, 1–14. doi: 10.1007/s10533-019-00568-3
Hamrick, J. L., and Godt, M. J. W. (1990). “Allozyme diversity in plant species,” in Plant population genetics, breeding, and genetic resources, eds A. H. D. Brown, M. T. Clegg, A. L. Kahler, and B. S. Weir (Sunderland, MA: Sinauer Associates Inc), 43–63.
Hansen, A., Barnett, K., Jantz, P., Phillips, L., Goetz, S. J., Hansen, M., et al. (2019). Global humid tropics forest structural condition and forest structural integrity maps. Sci. Data 6:232. doi: 10.1038/s41597-019-0214-3
Hansen, A. J., Burns, P., Ervin, J., Goetz, S. J., Hansen, M., Venter, O., et al. (2020). A policy-driven framework for conserving the best of Earth’s remaining moist tropical forests. Nat. Ecol. Evol. 4, 1377–1384. doi: 10.1038/s41559-020-1274-7
Hansen, A. J., Noble, B. P., Veneros, J., East, A., Goetz, S. J., Supples, C., et al. (2021). Toward monitoring forest ecosystem integrity within the post-2020 global biodiversity framework. Conserv. Lett. 14:e12822. doi: 10.1111/conl.12822
Hansen, A. J., Phillips, L. B., Dubayah, R., Goetz, S., and Hofton, M. (2014). Regional-scale application of lidar: Variation in forest canopy structure across the Southeastern US. For. Ecol. Manag. 329, 214–226. doi: 10.1016/j.foreco.2014.06.009
Hansen, E. M., and Goheen, E. M. (2000). Phellinus weirii and other native root pathogens as determinants of forest structure and process in western North America. Annu. Rev. Phytopathol. 38, 515–539. doi: 10.1146/annurev.phyto.38.1.515
Hansen, M. C., Potapov, P. V., Moore, R., Hancher, M., Turubanova, S. A., Tyukavina, A., et al. (2013). High-resolution global maps of 21st-century forest cover change. Science 342, 850–853. doi: 10.1126/science.1244693
Hansen, M. C., Wang, L., Song, X.-P., Tyukavina, A., Turubanova, S., Potapov, P., et al. (2020). The fate of tropical forest fragments. Sci. Adv. 6:eaax8574. doi: 10.1126/sciadv.aax8574
Hantsch, L., Bien, S., Radatz, S., Braun, U., Auge, H., and Bruelheide, H. (2014). Tree diversity and the role of non-host neighbour tree species in reducing fungal pathogen infestation. J. Ecol. 102, 1673–1687. doi: 10.1111/1365-2745.12317
Harmon, M., Ferrell, W., and Franklin, J. (1990). Effects on carbon storage of conversion of old-growth forests to young forests. Science 247, 699–702. doi: 10.1126/science.247.4943.699
Harrison, P. A., Berry, P. M., Simpson, G., Haslett, J. R., Blicharska, M., Bucur, M., et al. (2014). Linkages between biodiversity attributes and ecosystem services: A systematic review. Ecosyst. Serv. 9, 191–203. doi: 10.1016/j.ecoser.2014.05.006
Harrison, R. D., Tan, S., Plotkin, J. B., Slik, F., Detto, M., Brenes, T., et al. (2013). Consequences of defaunation for a tropical tree community. Ecol. Lett. 16, 687–694. doi: 10.1111/ele.12102
Hartanto, H., Prabhu, R., Widayat, A. S. E., and Asdak, C. (2003). Factors affecting runoff and soil erosion: Plot-level soil loss monitoring for assessing sustainability of forest management. For. Ecol. Manag. 180, 361–374. doi: 10.1016/S0378-1127(02)00656-4
Harter, D. E. V., Nagy, L., Backhaus, S., Beierkuhnlein, C., Fussi, B., Huber, G., et al. (2015). A comparison of genetic diversity and phenotypic plasticity among European beech (Fagus sylvatica L.) populations from Bulgaria and Germany under drought and temperature manipulation. Int. J. Plant Sci. 176, 232–244. doi: 10.1086/679349
Harwell, M. A., Myers, V., Young, T., Bartuska, A., Gassman, N., Gentile, J. H., et al. (1999). A framework for an ecosystem integrity report card. Bioscience 49, 543–556. doi: 10.2307/1313475
Hautier, Y., Tilman, D., Isbell, F., Seabloom, E. W., Borer, E. T., and Reich, P. B. (2015). Anthropogenic environmental changes affect ecosystem stability via biodiversity. Science 348, 336–340. doi: 10.1126/science.aaa1788
Hawbaker, T. J., Radeloff, V. C., Clayton, M. K., Hammer, R. B., and Gonzalez-Abraham, C. E. (2006). Road development, housing growth, and landscape fragmentation in northern Wisconsin: 1937-1999. Ecol. Appl. 16, 1222–1237. doi: 10.1890/1051-0761(2006)016[1222:rdhgal]2.0.co;2
Hawbaker, T. J., Radeloff, V. C., Stewart, S. I., Hammer, R. B., Keuler, N. S., and Clayton, M. K. (2013). Human and biophysical influences on fire occurrence in the United States. Ecol. Appl. 23, 565–582. doi: 10.1890/12-1816.1
Hember, R. A., Kurz, W. A., and Coops, N. C. (2017). Relationships between individual-tree mortality and water-balance variables indicate positive trends in water stress-induced tree mortality across North America. Glob. Change Biol. 23, 1691–1710. doi: 10.1111/gcb.13428
Hengeveld, R. (1989). The dynamics of biological invasions–Chapman & Hall, 1st Edn. Dordrecht: Springer Netherlands.
Herbeck, L. A., and Larsen, D. R. (1999). Plethodontid salamander response to silvicultural practices in Missouri Ozark forests. Conserv. Biol. 13, 623–632. doi: 10.1046/j.1523-1739.1999.98097.x
Hicke, J. A., Allen, C. D., Desai, A. R., Dietze, M. C., Hall, R. J., Hogg, E. H., et al. (2012). Effects of biotic disturbances on forest carbon cycling in the United States and Canada. Glob. Change Biol. 18, 7–34. doi: 10.1111/j.1365-2486.2011.02543.x
Hill, S. L. L., Arnell, A., Maney, C., Butchart, S. H. M., Hilton-Taylor, C., Ciciarelli, C., et al. (2019). Measuring forest biodiversity status and changes globally. Front. For. Glob. Change 2:70. doi: 10.3389/ffgc.2019.00070
Hirota, M., Holmgren, M., Van Nes, E. H., and Scheffer, M. (2011). Global resilience of tropical forest and Savanna to critical transitions. Science 334, 232–235. doi: 10.1126/science.1210657
Hoare, A. (2015). Tackling illegal logging and the related trade: What progress and where next? London: The Royal Institute of International Affairs.
Hobbie, S. (1992). Effects of plant-species on nutrient cycling. Trends Ecol. Evol. 7, 336–339. doi: 10.1016/0169-5347(92)90126-V
Holdsworth, A. R., and Uhl, C. (1997). Fire in Amazonian selectively logged rain forest and the potential for fire reduction. Ecol. Appl. 7, 713–725.
Holling, C. (1992). Cross-scale morphology, geometry, and dynamics of ecosystems. Ecol. Monogr. 62, 447–502. doi: 10.2307/2937313
Holling, C. S. (1973). Resilience and stability of ecological systems. Annu. Rev. Ecol. Syst. 4, 1–23.
Holloway, M. (1993). Sustaining the Amazon. Sci. Am. 269, 90–99. doi: 10.1038/scientificamerican0793-90
Holsten, E., Hennon, P., Trummer, L., Kruse, J., Schultz, M., and Lundquist, J. (2008). Insects and diseases of Alaskan forests. Washington, DC: USDA Forest Service.
Holtsmark, B. (2012). Harvesting in boreal forests and the biofuel carbon debt. Clim. Change 112, 415–428. doi: 10.1007/s10584-011-0222-6
Hooper, D. U., Chapin, F. S., Ewel, J. J., Hector, A., Inchausti, P., Lavorel, S., et al. (2005). Effects of biodiversity on ecosystem functioning: A consensus of current knowledge. Ecol. Monogr. 75, 3–35. doi: 10.1890/04-0922
Hornbeck, J. W., and Federer, C. A. (1975). “Effects of management practices on water quality and quantity: Hubbard brook experimental forest, New Hampshire,” in Proceedings of the municipal watershed management symposium general technical report NE-13, (Upper Darby, PA: USDA Forest Service), 58–65.
Hosonuma, N., Herold, M., De Sy, V., De Fries, R. S., Brockhaus, M., Verchot, L., et al. (2012). An assessment of deforestation and forest degradation drivers in developing countries. Environ. Res. Lett. 7:044009. doi: 10.1088/1748-9326/7/4/044009
Howe, G. T., Aitken, S. N., Neale, D. B., Jermstad, K. D., Wheeler, N. C., and Chen, T. H. H. (2003). From genotype to phenotype: Unraveling the complexities of cold adaptation in forest trees. Can. J. Bot. Rev. Can. Bot. 81, 1247–1266. doi: 10.1139/B03-141
Hudiburg, T. W., Law, B. E., Moomaw, W. R., Harmon, M. E., and Stenzel, J. E. (2019). Meeting GHG reduction targets requires accounting for all forest sector emissions. Environ. Res. Lett. 14:095005. doi: 10.1088/1748-9326/ab28bb
Hughes, J. B., Ives, A. R., and Norberg, J. (2002). “A new look at the relationship between diversity and stability,” in Biodiversity and ecosystem functioning: Synthesis and perspectives, eds M. Loreau, S. Naeem, and P. Inchausti (New York, NY: Oxford University Press), 92–101.
Hume, A. M., Chen, H. Y. H., and Taylor, A. R. (2018). Intensive forest harvesting increases susceptibility of northern forest soils to carbon, nitrogen and phosphorus loss. J. Appl. Ecol. 55, 246–255. doi: 10.1111/1365-2664.12942
Ibisch, P. L., Hoffmann, M. T., Kreft, S., Pe’er, G., Kati, V., Biber-Freudenberger, L., et al. (2016). A global map of roadless areas and their conservation status. Science 354, 1423–1427. doi: 10.1126/science.aaf7166
IPBES (2019). Summary for policymakers of the global assessment report on biodiversity and ecosystem services of the intergovernmental science-policy platform on biodiversity and ecosystem services. Bonn: IPBES.
IPCC (2018). Global warming of 1.5°C. An IPCC special report on the impacts of global warming of 1.5°C above pre-industrial levels and related global greenhouse gas emission pathways, in the context of strengthening the global response to the threat of climate change, sustainable development, and efforts to eradicate poverty. Available online at: https://www.ipcc.ch/sr15/chapter/spm/ (accessed September 23, 2019).
IPCC (2022). “Summary for policymakers,” in Climate change 2022: Impacts, adaptation, and vulnerability. Contribution of working group II to the sixth assessment report of the intergovernmental panel on climate change, eds H.-O. Portner, D. C. Roberts, E. S. Poloczanska, K. Mintenbeck, A. Tignor, A. Alegria, et al. (Cambridge: Cambridge University Press).
IUCN (2020). IUCN policy statement on primary forests including intact forest landscapes. Gland: International Union for Conservation of Nature.
Iverson, L. R., Schwartz, M. W., and Prasad, A. M. (2004). How fast and far might tree species migrate in the eastern United States due to climate change? Glob. Ecol. Biogeogr. 13, 209–219. doi: 10.1111/j.1466-822X.2004.00093.x
Ives, A. R., Gross, K., and Klug, J. L. (1999). Stability and variability in competitive communities. Science 286, 542–544. doi: 10.1126/science.286.5439.542
Jactel, H., Brockerhoff, E., and Duelli, P. (2005). “A test of the biodiversity-stability theory: Meta-analysis of tree species diversity effects on insect pest infestations, and re-examination of responsible factors,” in Forest diversity and function: Temperate and boreal systems ecological studies, eds M. Scherer-Lorenzen, C. Körner, and E.-D. Schulze (Berlin: Springer), 235–262. doi: 10.1007/3-540-26599-6_12
Jactel, H., and Brockerhoff, E. G. (2007). Tree diversity reduces herbivory by forest insects. Ecol. Lett. 10, 835–848. doi: 10.1111/j.1461-0248.2007.01073.x
Jain, P., Wang, X., and Flannigan, M. D. (2017). Trend analysis of fire season length and extreme fire weather in North America between 1979 and 2015. Int. J. Wildland Fire 26, 1009–1020. doi: 10.1071/WF17008
James, J., and Harrison, R. (2016). The effect of harvest on forest soil carbon: A meta-analysis. Forests 7:308. doi: 10.3390/f7120308
Janisch, J. E., and Harmon, M. E. (2002). Successional changes in live and dead wood carbon stores: Implications for net ecosystem productivity. Tree Physiol. 22, 77–89. doi: 10.1093/treephys/22.2-3.77
Jayasuriya, M. D. A., Dunn, G., Benyon, R., and O’shaughnessy, P. J. (1993). Some factors affecting water yield from mountain ash (Eucalyptus regnans) dominated forests in south-east Australia. J. Hydrol. 150, 345–367.
Jiang, H., Apps, M. J., Peng, C. H., Zhang, Y. L., and Liu, J. X. (2002). Modelling the influence of harvesting on Chinese boreal forest carbon dynamics. For. Ecol. Manag. 169, 65–82. doi: 10.1016/S0378-1127(02)00299-2
Jogiste, K., Korjus, H., Stanturf, J. A., Frelich, L. E., Baders, E., Donis, J., et al. (2017). Hemiboreal forest: Natural disturbances and the importance of ecosystem legacies to management. Ecosphere 8:e01706. doi: 10.1002/ecs2.1706
Johnstone, J. F., Allen, C. D., Franklin, J. F., Frelich, L. E., Harvey, B. J., Higuera, P. E., et al. (2016). Changing disturbance regimes, ecological memory, and forest resilience. Front. Ecol. Environ. 14, 369–378. doi: 10.1002/fee.1311
Jolly, W. M., Cochrane, M. A., Freeborn, P. H., Holden, Z. A., Brown, T. J., Williamson, G. J., et al. (2015). Climate-induced variations in global wildfire danger from 1979 to 2013. Nat. Commun. 6:7537. doi: 10.1038/ncomms8537
Jump, A. S., Marchant, R., and Penuelas, J. (2009). Environmental change and the option value of genetic diversity. Trends Plant Sci. 14, 51–58. doi: 10.1016/j.tplants.2008.10.002
Jump, A. S., and Penuelas, J. (2005). Running to stand still: Adaptation and the response of plants to rapid climate change. Ecol. Lett. 8, 1010–1020. doi: 10.1111/j.1461-0248.2005.00796.x
Kabanets, A. G., Milakovsky, B. J., Lepeshkin, E. A., and Sychikov, D. V. (2013). Illegal logging in the Russian Far East: Flobal demand and taiga destruction. Moscow: World Wildlife Fund Russia.
Kalaba, F. K., Quinn, C. H., and Dougill, A. J. (2014). Policy coherence and interplay between Zambia’s forest, energy, agricultural and climate change policies and multilateral environmental agreements. Int. Environ. Agreem. Polit. Law Econ. 14, 181–198. doi: 10.1007/s10784-013-9236-z
Kandziora, M., Burkhard, B., and Mueller, F. (2013). Interactions of ecosystem properties, ecosystem integrity and ecosystem service indicators-A theoretical matrix exercise. Ecol. Indic. 28, 54–78. doi: 10.1016/j.ecolind.2012.09.006
Karadimou, E. K., Kallimanis, A. S., Tsiripidis, I., and Dimopoulos, P. (2016). Functional diversity exhibits a diverse relationship with area, even a decreasing one. Sci. Rep. 6:35420. doi: 10.1038/srep35420
Karieva, P. (1983). “Influence of vegetation texture on herbivore populations: Resource concentration and herbivore movement,” in Variable plants and herbivores in natural and managed systems, eds R. F. Denno and M. S. McClure (New York, NY: Academic Press), 259–289.
Karr, J. R. (1996). “Ecological integrity and ecological health are not the same,” in Engineering within ecological constraints, ed. P. Schulze (Washington, DC: National Academies Press), 97–109.
Kautz, M., Meddens, A. J. H., Hall, R. J., and Arneth, A. (2017). Biotic disturbances in Northern Hemisphere forests - a synthesis of recent data, uncertainties and implications for forest monitoring and modelling. Glob. Ecol. Biogeogr. 26, 533–552. doi: 10.1111/geb.12558
Kay, J. (1991). A nonequilibrium thermodynamic framework for discussing ecosystem integrity. Environ. Manage. 15, 483–495. doi: 10.1007/BF02394739
Kay, J. J. (1993). “On the nature of ecological integrity: Some closing comments,” in Ecological integrity and the management of ecosystems, eds S. Woodley, J. Kay, and G. Francis (Delray Beach, FL: St. Lucie Press), 201–212.
Kay, J. J., and Regier, H. A. (2000). “Uncertainty, complexity, and ecological integrity: Insights from an ecosystem approach,” in Implementing ecological integrity: Restoring regional and global environmental and human health nato science series, eds P. Crabbé, A. Holland, L. Ryszkowski, and L. Westra (Dordrecht: Springer Netherlands), 121–156. doi: 10.1007/978-94-011-5876-3_9
Keith, H., Czúcz, B., Jackson, B., Driver, A., Nicholson, E., and Maes, J. (2020). A conceptual framework and practical structure for implementing ecosystem condition accounts. One Ecosyst. 5:e58216. doi: 10.3897/oneeco.5.e58216
Keith, H., Leuning, R., Jacobsen, K. L., Cleugh, H. A., van Gorsel, E., Raison, R. J., et al. (2009). Multiple measurements constrain estimates of net carbon exchange by a Eucalyptus forest. Agric. For. Meteorol. 149, 535–558. doi: 10.1016/j.agrformet.2008.10.002
Keith, H., Lindenmayer, D., Macintosh, A., and Mackey, B. (2015). Under what circumstances do wood products from native forests benefit climate change mitigation? PLoS One 10:e0139640. doi: 10.1371/journal.pone.0139640
Keith, H., van Gorsel, E., Jacobsen, K. L., and Cleugh, H. A. (2012). Dynamics of carbon exchange in a Eucalyptus forest in response to interacting disturbance factors. Agric. For. Meteorol. 153, 67–81. doi: 10.1016/j.agrformet.2011.07.019
Keith, H., Vardon, M., Obst, C., Young, V., Houghton, R. A., and Mackey, B. (2021). Evaluating nature-based solutions for climate mitigation and conservation requires comprehensive carbon accounting. Sci. Total Environ. 769:144341. doi: 10.1016/j.scitotenv.2020.144341
Keith, H., Vardon, M., Stein, J. A., and Lindenmayer, D. (2019). Contribution of native forests to climate change mitigation - A common approach to carbon accounting that aligns results from environmental-economic accounting with rules for emissions reduction. Environ. Sci. Policy 93, 189–199. doi: 10.1016/j.envsci.2018.11.001
Keith, H., Vardon, M., Stein, J. A., Stein, J. L., and Lindenmayer, D. (2017). Ecosystem accounts define explicit and spatial trade-offs for managing natural resources. Nat. Ecol. Evol. 1, 1683–1692. doi: 10.1038/s41559-017-0309-1
King, S., Ferrier, S., Turner, K., and Badura, T. (2019). Discussion paper 11: Research paper on habitat and biodiversity related ecosystem services. United Nations statistics division, department of economic and social affairs. New York, NY: United Nations Statistics Division.
Klaus, M., Holsten, A., Hostert, P., and Kropp, J. P. (2011). Integrated methodology to assess windthrow impacts on forest stands under climate change. For. Ecol. Manag. 261, 1799–1810. doi: 10.1016/j.foreco.2011.02.002
Koff, H., Zamora, M. E., Maganda, C., and Pérez-Maqueo, O. (2016). Ecosystem integrity and policy coherence for development: Tools aimed at achieving balance as the basis for transformative development. Reg. Cohes. 6, 77–92. doi: 10.3167/reco.2016.060304
Kolb, T. E., Agee, J. K., Fule, P. Z., McDowell, N. G., Pearson, K., Sala, A., et al. (2007). Perpetuating old ponderosa pine. For. Ecol. Manag. 249, 141–157. doi: 10.1016/j.foreco.2007.06.002
Kormos, C. F., Mackey, B., DellaSala, D. A., Kumpe, N., Jaeger, T., Mittermeier, R. A., et al. (2018). “Primary forests: Definition, status and future prospects for global conservation,” in Reference module in earth systems and environmental sciences. Encyclopedia of the Anthropocene, eds D. A. DellaSala and M. I. Goldstein (Amsterdam: Elsevier), 31–41.
Kormos, C. F., and Zimmerman, B. L. (2014). Response to: Putz et al., sustaining conservation values in selectively logged tropical forests: The attained and the attainable. Conserv. Lett. 7, 143–144. doi: 10.1111/conl.12043
Kovacs, K., Ranson, K. J., Sun, G., and Kharuk, V. I. (2004). The relationship of the terra MODIS fire product and anthropogenic features in the central Siberian landscape. Earth Interact. 8:18.
Krivets, S. A., Bisirova, E. M., Kerchev, I. A., Pats, E. N., and Chernova, N. A. (2015). Transformation of taiga ecosystems in the Western Siberian invasion focus of four-eyed fir bark beetle Polygraphus proximus Blandford (Coleoptera: Curculionidae, Scolytinae). Russ. J. Biol. Invasions 6, 94–108. doi: 10.1134/S2075111715020058
Kukavskaya, E. A., Buryak, L. V., Ivanova, G. A., Conard, S. G., Kalenskaya, O. P., Zhila, S. V., et al. (2013). Influence of logging on the effects of wildfire in Siberia. Environ. Res. Lett. 8:045034. doi: 10.1088/1748-9326/8/4/045034
Kukavskaya, E. A., Buryak, L. V., Shvetsov, E. G., Conard, S. G., and Kalenskaya, O. P. (2016). The impact of increasing fire frequency on forest transformations in southern Siberia. For. Ecol. Manag. 382, 225–235. doi: 10.1016/j.foreco.2016.10.015
Kurz, W. A., Stinson, G., Rampley, G. J., Dymond, C. C., and Neilson, E. T. (2008). Risk of natural disturbances makes future contribution of Canada’s forests to the global carbon cycle highly uncertain. Proc. Natl. Acad. Sci. U.S.A. 105, 1551–1555. doi: 10.1073/pnas.0708133105
Kuuluvainen, T., and Gauthier, S. (2018). Young and old forest in the boreal: Critical stages of ecosystem dynamics and management under global change. For. Ecosyst. 5:26. doi: 10.1186/s40663-018-0142-2
Lacroix, E. M., Petrenko, C. L., and Friedland, A. J. (2016). Evidence for losses from strongly bound SOM pools after clear cutting in a Northern hardwood forest. Soil Sci. 181, 202–207. doi: 10.1097/SS.0000000000000147
Laflamme, J. (2020). Cumulative patterns of fire and harvest disturbance: Comparing case studies from Russia and Canada. Available Online at: http://hdl.handle.net/1807/99029 (accessed January 23, 2021).
Lafleur, B., Fenton, N. J., Simard, M., Leduc, A., Pare, D., Valeria, O., et al. (2018). Ecosystem management in paludified boreal forests: Enhancing wood production, biodiversity, and carbon sequestration at the landscape level. For. Ecosyst. 5:27. doi: 10.1186/s40663-018-0145-z
Landi, M. A., Di Bella, C. M., Bravo, S. J., and Bellis, L. M. (2021). Structural resistance and functional resilience of the Chaco forest to wildland fires: An approach with MODIS time series. Austral Ecol. 46, 277–289. doi: 10.1111/aec.12977
Lange, G.-M., Wodon, Q., and Carey, K. (2018). The changing wealth of nations 2018: Building a sustainable future. Washington, DC: World Bank Group.
Laurance, W. F., and Balmford, A. (2013). A global map for road building. Nature 495, 308–309. doi: 10.1038/495308a
Laurance, W. F., Cochrane, M. A., Bergen, S., Fearnside, P. M., Delamonica, P., Barber, C., et al. (2001). Environment - the future of the Brazilian Amazon. Science 291, 438–439. doi: 10.1126/science.291.5503.438
Laurance, W. F., and Curran, T. J. (2008). Impacts of wind disturbance on fragmented tropical forests: A review and synthesis. Austral Ecol. 33, 399–408. doi: 10.1111/j.1442-9993.2008.01895.x
Laurance, W. F., Ferreira, L. V., Rankin-De Merona, J. M., and Laurance, S. G. (1998). Rain forest fragmentation and the dynamics of Amazonian tree communities. Ecology 79, 2032–2040.
Laurance, W. F., Goosem, M., and Laurance, S. G. W. (2009). Impacts of roads and linear clearings on tropical forests. Trends Ecol. Evol. 24, 659–669. doi: 10.1016/j.tree.2009.06.009
Laurance, W. F., Laurance, S. G., Ferreira, L. V., RankindeMerona, J. M., Gascon, C., and Lovejoy, T. E. (1997). Biomass collapse in Amazonian forest fragments. Science 278, 1117–1118. doi: 10.1126/science.278.5340.1117
Laurance, W. F., Sayer, J., and Cassman, K. G. (2014). Agricultural expansion and its impacts on tropical nature. Trends Ecol. Evol. 29, 107–116. doi: 10.1016/j.tree.2013.12.001
Laurance, W. F., and Williamson, G. B. (2001). Positive feedbacks among forest fragmentation, drought, and climate change in the Amazon. Conserv. Biol. 15, 1529–1535. doi: 10.1046/j.1523-1739.2001.01093.x
Law, B. E., Moomaw, W. R., Hudiburg, T. W., Schlesinger, W. H., Sterman, J. D., and Woodwell, G. M. (2022). Creating strategic reserves to protect forest carbon and reduce biodiversity losses in the United States. Land 11:721. doi: 10.3390/land11050721
Lawrence, D., and Vandecar, K. (2015). Effects of tropical deforestation on climate and agriculture. Nat. Clim. Change 5, 27–36. doi: 10.1038/NCLIMATE2430
Lawson, S. (2014). Illegal wood import and re-export: The scale of the problem and the response in Thailand, South Korea and India. London: The Royal Institute of International Affairs.
Lawson, S., and MacFaul, L. (2010). Illegal logging and related trade: Indicators of the global response. London: The Royal Institute of International Affairs.
Lawton, J. H. (1997). “The role of species in ecosystems: Aspects of ecological complexity and biological diversity,” in Biodiversity: An ecological perspective, eds T. Abe, S. A. Levin, and M. Higashi (New York, NY: Springer), 215–228. doi: 10.1007/978-1-4612-1906-4_13
Lee, S. S. (2018). Observations on the successes and failures of acacia plantations in Sabah and Sarawak and the way forward. J. Trop. For. Sci. 30, 468–475. doi: 10.26525/jtfs2018.30.5.468475
Lehman, C. L., and Tilman, D. (2000). Biodiversity, stability, and productivity in competitive communities. Am. Nat. 156, 534–552. doi: 10.1086/303402
Lesica, P., McCune, B., Cooper, S. V., and Hong, W. S. (1991). Differences in lichen and bryophyte communities between old-growth and managed second-growth forests in the Swan Valley, Montana. Can. J. Bot. 69, 1745–1755.
Lewis, S. L., Lopez-Gonzalez, G., Sonke, B., Affum-Baffoe, K., Baker, T. R., Ojo, L. O., et al. (2009). Increasing carbon storage in intact African tropical forests. Nature 457, 1003–1006. doi: 10.1038/nature07771
Liang, J., Crowther, T. W., Picard, N., Wiser, S., Zhou, M., Alberti, G., et al. (2016). Positive biodiversity-productivity relationship predominant in global forests. Science 354:aaf8957. doi: 10.1126/science.aaf8957
Liebsch, D., Marques, M. C. M., and Goldenbeg, R. (2008). How long does the Atlantic Rain Forest take to recover after a disturbance? Changes in species composition and ecological features during secondary succession. Biol. Conserv. 141, 1717–1725. doi: 10.1016/j.biocon.2008.04.013
Likens, G. E., Bormann, F. H., Johnson, N. M., Fisher, D. W., and Pierce, R. S. (1970). Effects of forest cutting and herbicide treatment on nutrient budgets in the Hubbard brook watershed-ecosystem. Ecol. Monogr. 40, 23–47. doi: 10.2307/1942440
Lindenmayer, D. B., Hobbs, R. J., Likens, G. E., Krebs, C. J., and Banks, S. C. (2011). Newly discovered landscape traps produce regime shifts in wet forests. Proc. Natl. Acad. Sci. U.S.A. 108, 15887–15891. doi: 10.1073/pnas.1110245108
Lindenmayer, D. B., Hunter, M. L., Burton, P. J., and Gibbons, P. (2009). Effects of logging on fire regimes in moist forests. Conserv. Lett. 2, 271–277. doi: 10.1111/j.1755-263X.2009.00080.x
Lindenmayer, D. B., and Sato, C. (2018). Hidden collapse is driven by fire and logging in a socioecological forest ecosystem. Proc. Natl. Acad. Sci. U.S.A. 115, 5181–5186. doi: 10.1073/pnas.1721738115
Lindenmayer, D. B., Westgate, M. J., Scheele, B. C., Foster, C. N., and Blair, D. P. (2019). Key perspectives on early successional forests subject to stand-replacing disturbances. For. Ecol. Manag. 454:117656. doi: 10.1016/j.foreco.2019.117656
Liski, J., Ilvesniemi, H., Makela, A., and Starr, M. (1998). Model analysis of the effects of soil age, fires and harvesting on the carbon storage of boreal forest soils. Eur. J. Soil Sci. 49, 407–416. doi: 10.1046/j.1365-2389.1998.4930407.x
Liu, X., Trogisch, S., He, J.-S., Niklaus, P. A., Bruelheide, H., Tang, Z., et al. (2018). Tree species richness increases ecosystem carbon storage in subtropical forests. Proc. R. Soc. B Biol. Sci. 285:20181240. doi: 10.1098/rspb.2018.1240
Liu, Y., Wang, S., Wang, Z., Zhang, Z., Qin, H., Wei, Z., et al. (2019). Soil microbiome mediated nutrients decline during forest degradation process. Soil Ecol. Lett. 1, 59–71. doi: 10.1007/s42832-019-0009-7
Lohmander, P., and Helles, F. (1987). Windthrow probability as a function of stand characteristics and shelter. Scand. J. For. Res. 2, 227–238. doi: 10.1080/02827588709382460
Loreau, M. (2000). Biodiversity and ecosystem functioning: Recent theoretical advances. Oikos 91, 3–17. doi: 10.1034/j.1600-0706.2000.910101.x
Loreau, M., Downing, A., Emmerson, M., Gonzalez, A., Hughes, J., Inchausti, P., et al. (2002). “A new look at the relationship between diversity and stability,” in Biodiversity and ecosystem functioning: Synthesis and perspectives, eds M. Loreau, S. Naeem, and P. Inchausti (New York, NY: Oxford University Press), 79–91.
Loreau, M., Naeem, S., Inchausti, P., Bengtsson, J., Grime, J. P., Hector, A., et al. (2001). Ecology - Biodiversity and ecosystem functioning: Current knowledge and future challenges. Science 294, 804–808. doi: 10.1126/science.1064088
Lu, X., Zang, R., Ding, Y., and Huang, J. (2016). Changes in biotic and abiotic drivers of seedling species composition during forest recovery following shifting cultivation on Hainan Island, China. Biotropica 48, 758–769. doi: 10.1111/btp.12392
Luo, Y., and Chen, H. Y. H. (2013). Observations from old forests underestimate climate change effects on tree mortality. Nat. Commun. 4:1655. doi: 10.1038/ncomms2681
Lutz, J. A., Furniss, T. J., Johnson, D. J., Davies, S. J., Allen, D., Alonso, A., et al. (2018). Global importance of large-diameter trees. Glob. Ecol. Biogeogr. 27, 849–864. doi: 10.1111/geb.12747
Luyssaert, S., Schulze, E.-D., Boerner, A., Knohl, A., Hessenmoeller, D., Law, B. E., et al. (2008). Old-growth forests as global carbon sinks. Nature 455, 213–215. doi: 10.1038/nature07276
Macintosh, A. (2013). Chipping away at Tasmania’s future: Alternatives to subsidising the forestry industry. Canberra: The Australia Institute.
Mackey, B., DellaSala, D. A., Kormos, C., Lindenmayer, D., Kumpel, N., Zimmerman, B., et al. (2015). Policy options for the world’s primary forests in multilateral environmental agreements. Conserv. Lett. 8, 139–147. doi: 10.1111/conl.12120
Mackey, B., Kormos, C. F., Keith, H., Moomaw, W. R., Houghton, R. A., Mittermeier, R. A., et al. (2020). Understanding the importance of primary tropical forest protection as a mitigation strategy. Mitig. Adapt. Strateg. Glob. Change 25, 763–787. doi: 10.1007/s11027-019-09891-4
Mackey, B., Prentice, I. C., Steffen, W., House, J. I., Lindenmayer, D., Keith, H., et al. (2013). Untangling the confusion around land carbon science and climate change mitigation policy. Nat. Clim. Change 3, 552–557. doi: 10.1038/NCLIMATE1804
Mackey, B. G., Watson, J. E. M., Hope, G., and Gilmore, S. (2008). Climate change, biodiversity conservation, and the role of protected areas: An Australian perspective. Biodiversity 9, 11–18. doi: 10.1080/14888386.2008.9712902
Macpherson, M. F., Kleczkowski, A., Healey, J. R., Quine, C. P., and Hanley, N. (2017). The effects of invasive pests and pathogens on strategies for forest diversification. Ecol. Model. 350, 87–99. doi: 10.1016/j.ecolmodel.2017.02.003
Maes, J., Teller, A., Erhard, M., Conde, S., Vallecillo, S., Barredo, J. I., et al. (2020). Mapping and assessment of ecosystems and their services: An EU ecosystem assessment. JRC120383 ed. Ispra: Publications Office of the European Union.
Malcolm, J. R., Holtsmark, B., and Piascik, P. W. (2020). Forest harvesting and the carbon debt in boreal east-central Canada. Clim. Change 161, 433–449. doi: 10.1007/s10584-020-02711-8
Markewitz, D., Davidson, E., Moutinho, P., and Nepstad, D. (2004). Nutrient loss and redistribution after forest clearing on a highly weathered soil in Amazonia. Ecol. Appl. 14, S177–S199.
Martin, D. A., Osen, K., Grass, I., Hoelscher, D., Tscharntke, T., Wurz, A., et al. (2020). Land-use history determines ecosystem services and conservation value in tropical agroforestry. Conserv. Lett. 13:e12740. doi: 10.1111/conl.12740
Mathey, A.-H., Krcmar, E., Innes, J., and Vertinsky, I. (2008). Opportunities and costs of intensification and clustering of forest management activities. Can. J. For. Res. 38, 711–720. doi: 10.1139/X07-197
Matos, F. A. R., Magnago, L. F. S., Aquila Chan Miranda, C., de Menezes, L. F. T., Gastauer, M., Safar, N. V. H., et al. (2020). Secondary forest fragments offer important carbon and biodiversity cobenefits. Glob. Change Biol. 26, 509–522. doi: 10.1111/gcb.14824
Matricardi, E. A. T., Skole, D. L., Costa, O. B., Pedlowski, M. A., Samek, J. H., and Miguel, E. P. (2020). Long-term forest degradation surpasses deforestation in the Brazilian Amazon. Science 369, 1378–1382. doi: 10.1126/science.abb3021
Maxwell, S. L., Evans, T., Watson, J. E. M., Morel, A., Grantham, H., Duncan, A., et al. (2019). Degradation and forgone removals increase the carbon impact of intact forest loss by 626%. Sci. Adv. 5:eaax2546. doi: 10.1126/sciadv.aax2546
May, R. (2001). Stability and complexity in model ecosystems. Available Online at: https://press.princeton.edu/books/paperback/9780691088617/stability-and-complexity-in-model-ecosystems [accessed August 12, 2020].
Mayer, M., Prescott, C. E., Abaker, W. E. A., Augusto, L., Cecillon, L., Ferreira, G. W. D., et al. (2020). Tamm review: Influence of forest management activities on soil organic carbon stocks: A knowledge synthesis. For. Ecol. Manag. 466:118127. doi: 10.1016/j.foreco.2020.118127
McDowell, N. G., Adams, H. D., Bailey, J. D., Hess, M., and Kolb, T. E. (2006). Homeostatic maintenance of ponderosa pine gas exchange in response to stand density changes. Ecol. Appl. 16, 1164–1182. doi: 10.1890/1051-0761(2006)016[1164:hmoppg]2.0.co;2
McDowell, N. G., and Allen, C. D. (2015). Darcy’s law predicts widespread forest mortality under climate warming. Nat. Clim. Change 5, 669–672. doi: 10.1038/NCLIMATE2641
McDowell, N. G., Beerling, D. J., Breshears, D. D., Fisher, R. A., Raffa, K. F., and Stitt, M. (2011). The interdependence of mechanisms underlying climate-driven vegetation mortality. Trends Ecol. Evol. 26, 523–532. doi: 10.1016/j.tree.2011.06.003
McDowell, N. G., Williams, A. P., Xu, C., Pockman, W. T., Dickman, L. T., Sevanto, S., et al. (2016). Multi-scale predictions of massive conifer mortality due to chronic temperature rise. Nat. Clim. Change 6, 295–300. doi: 10.1038/NCLIMATE2873
McGarvey, J. C., Thompson, J. R., Epstein, H. E., and Shugart, H. H. (2015). Carbon storage in old-growth forests of the Mid-Atlantic: Toward better understanding the eastern forest carbon sink. Ecology 96, 311–317. doi: 10.1890/14-1154.1
McIntyre, P. J., Thorne, J. H., Dolanc, C. R., Flint, A. L., Flint, L. E., Kelly, M., et al. (2015). Twentieth-century shifts in forest structure in California: Denser forests, smaller trees, and increased dominance of oaks. Proc. Natl. Acad. Sci. U.S.A. 112, 1458–1463. doi: 10.1073/pnas.1410186112
Merino, A., Balboa, M. A., Soalleiro, R. R., and Gonzalez, J. G. A. (2005). Nutrient exports under different harvesting regimes in fast-growing forest plantations in southern Europe. For. Ecol. Manag. 207, 325–339. doi: 10.1016/j.foreco.2004.10.074
Migliavacca, M., Musavi, T., Mahecha, M. D., Nelson, J. A., Knauer, J., Baldocchi, D. D., et al. (2021). The three major axes of terrestrial ecosystem function. Nature 598, 468–472. doi: 10.1038/s41586-021-03939-9
Mignone, B. K., Hurteau, M. D., Chen, Y., and Sohngen, B. (2009). Carbon offsets, reversal risk and US climate policy. Carbon Balance Manag. 4:3. doi: 10.1186/1750-0680-4-3
Mildrexler, D. J., Berner, L. T., Law, B. E., Birdsey, R. A., and Moomaw, W. R. (2020). Large trees dominate carbon storage in forests east of the cascade crest in the United States Pacific Northwest. Front. For. Glob. Change 3:594274. doi: 10.3389/ffgc.2020.594274
Miles, L., Grainger, A., and Phillips, O. (2004). The impact of global climate change on tropical forest biodiversity in Amazonia. Glob. Ecol. Biogeogr. 13, 553–565. doi: 10.1111/j.1466-822X.2004.00105.x
Millennium Ecosystem Assessment (2005). Ecosystems and human well-being. Washington, DC: Island Press.
Miller, K. M., McGill, B. J., Mitchell, B. R., Comiskey, J., Dieffenbach, F. W., Matthews, E. R., et al. (2018). Eastern national parks protect greater tree species diversity than unprotected matrix forests. For. Ecol. Manag. 414, 74–84. doi: 10.1016/j.foreco.2018.02.018
Mitchard, E. T. A. (2018). The tropical forest carbon cycle and climate change. Nature 559, 527–534. doi: 10.1038/s41586-018-0300-2
Mitchell, S. R., Harmon, M. E., and O’Connell, K. E. B. (2012). Carbon debt and carbon sequestration parity in forest bioenergy production. Glob. Change Biol. Bioenergy 4, 818–827. doi: 10.1111/j.1757-1707.2012.01173.x
Moomaw, W. R., Masino, S. A., and Faison, E. K. (2019). Intact forests in the United States: Proforestation mitigates climate change and serves the greatest good. Front. For. Glob. Change 2:27. doi: 10.3389/ffgc.2019.00027
Moreno-Mateos, D., Barbier, E. B., Jones, P. C., Jones, H. P., Aronson, J., Lopez-Lopez, J. A., et al. (2017). Anthropogenic ecosystem disturbance and the recovery debt. Nat. Commun. 8:14163. doi: 10.1038/ncomms14163
Morgan, E. A., Cadman, T., and Mackey, B. (2020). Integrating forest management across the landscape: A three pillar framework. J. Environ. Plan. Manag. 64, 1735–1769. doi: 10.1080/09640568.2020.1837747
Morgan, E. A., Osborne, N., and Mackey, B. (2022). Evaluating planning without plans: Principles, criteria and indicators for effective forest landscape approaches. Land Use Policy 115:106031. doi: 10.1016/j.landusepol.2022.106031
Mortensen, D. A., Rauschert, E. S. J., Nord, A. N., and Jones, B. P. (2009). Forest roads facilitate the spread of invasive plants. Invasive Plant Sci. Manag. 2, 191–199. doi: 10.1614/IPSM-08-125.1
Muller, F. (1998). Gradients in ecological systems. Ecol. Model. 108, 3–21. doi: 10.1016/S0304-3800(98)00015-5
Muller, F., Hoffmann-Kroll, R., and Wiggering, H. (2000). Indicating ecosystem integrity - theoretical concepts and environmental requirements. Ecol. Model. 130, 13–23. doi: 10.1016/S0304-3800(00)00210-6
Muller-Landau, H. C. (2007). Predicting the long-term effects of hunting on plant species composition and diversity in tropical forests. Biotropica 39, 372–384. doi: 10.1111/j.1744-7429.2007.00290.x
Myers, N. (1997). “The world’s forests and their ecosystem services,” in Nature’s services: Societal dependence on natural ecosystems, ed. G. C. Daily (Washington, DC: Island Press).
Nabuurs, G.-J., Mrabet, R., Abu Hatab, A., Bustamante, M., Clark, H., Havlik, P., et al. (2022). “Chapter 7: Agriculture, forestry and other land uses (AFOLU),” in IPCC, 2022: Climate change 2022: Mitigation of climate change. Contribution of working group III contribution to the sixth assessment report of the intergovernmental panel on climate change, eds P. R. Shukla, J. Skea, R. Slade, A. Al Khourdajie, R. van Diemen, D. McCollum, et al. (Cambridge: Cambridge University Press). doi: 10.1017/9781009157926.009
Naeem, S., Thompson, L., Lawler, S., Lawton, J., and Woodfin, R. (1995). Empirical-evidence that declining species-diversity may alter the performance of terrestrial ecosystems. Philos. Trans. R. Soc. B Biol. Sci. 347, 249–262. doi: 10.1098/rstb.1995.0025
Naumov, V., Angelstam, P., and Elbakidze, M. (2016). Barriers and bridges for intensified wood production in Russia: Insights from the environmental history of a regional logging frontier. For. Policy Econ. 66, 1–10. doi: 10.1016/j.forpol.2016.02.001
Neilson, R. (1995). A model for predicting continental-scale vegetation distribution and water-balance. Ecol. Appl. 5, 362–385. doi: 10.2307/1942028
Nepstad, D. C., Verissimo, A., Alencar, A., Nobre, C., Lima, E., Lefebvre, P., et al. (1999). Large-scale impoverishment of Amazonian forests by logging and fire. Nature 398, 505–508. doi: 10.1038/19066
Newbold, T., Hudson, L. N., Arnell, A. P., Contu, S., De Palma, A., Ferrier, S., et al. (2016). Has land use pushed terrestrial biodiversity beyond the planetary boundary? A global assessment. Science 353, 288–291. doi: 10.1126/science.aaf2201
Nicotra, A. B., Atkin, O. K., Bonser, S. P., Davidson, A. M., Finnegan, E. J., Mathesius, U., et al. (2010). Plant phenotypic plasticity in a changing climate. Trends Plant Sci. 15, 684–692. doi: 10.1016/j.tplants.2010.09.008
Normyle, A., Doran, B., Vardon, M., Mathews, D., and Melbourne, J. (2022). Land cover and fire accounts to support Indigenous land management: A pilot study of Yawuru Country. J. Environ. Manage. 313:115003. doi: 10.1016/j.jenvman.2022.115003
Noss, R. F. (1995). Maintaining ecological integrity in representative reserve networks. Toronto: World Wildlife Fund.
Nunery, J. S., and Keeton, W. S. (2010). Forest carbon storage in the Northeastern United States: Net effects of harvesting frequency, post-harvest retention, and wood products. For. Ecol. Manag. 259, 1363–1375. doi: 10.1016/j.foreco.2009.12.029
NYDF Assessment Partners (2019). Protecting and restoring forests: A story of large commitments yet limited progress. New York declaration on forests five-year assessment report. Amsterdam: Climate Focus.
Odion, D. C., Frost, E. J., Strittholt, J. R., Jiang, H., Dellasala, D. A., and Moritz, M. A. (2004). Patterns of fire severity and forest conditions in the western Klamath Mountains, California. Conserv. Biol. 18, 927–936. doi: 10.1111/j.1523-1739.2004.00493.x
Odum, E. P. (1969). The strategy of ecosystem development. Science 164, 262–270. doi: 10.1126/science.164.3877.262
Olander, L. P., Bustamante, M. M., Asner, G. P., Telles, E., Prado, Z., and Camargo, P. B. (2005). Surface soil changes following selective logging in an eastern amazon forest. Earth Interact. 9:4.
Oliver, T. H., Heard, M. S., Isaac, N. J. B., Roy, D. B., Procter, D., Eigenbrod, F., et al. (2015). Biodiversity and resilience of ecosystem functions. Trends Ecol. Evol. 30, 673–684. doi: 10.1016/j.tree.2015.08.009
Oppenheimer, M. M., Campos, R., Warren, R., Birkmann, J., Luber, G., O’Neill, B., et al. (2014). “Emergent risks and key vulnerabilities,” in Climate change 3014: Impacts, adaptation, and vulnerability. Part A: Global and sectoral aspects. Contribution of working group II to the fifth assessment report of the intergovernmental panel on climate change, eds C. B. Field, V. R. Barros, D. J. Dokken, K. J. Mach, M. D. Mastrandrea, T. E. Bilir, et al. (Cambridge: Cambridge University Press), 1039–1099.
Osuri, A. M., Ratnam, J., Varma, V., Alvarez-Loayza, P., Hurtado Astaiza, J., Bradford, M., et al. (2016). Contrasting effects of defaunation on aboveground carbon storage across the global tropics. Nat. Commun. 7:11351. doi: 10.1038/ncomms11351
Ough, K. (2001). Regeneration of Wet Forest flora a decade after clear-felling or wildfire - is there a difference? Aust. J. Bot. 49, 645–664. doi: 10.1071/BT99053
Overpeck, J., Bartlein, P., and Webb, T. (1991). Potential magnitude of future vegetation change in Eastern North-America - comparisons with the past. Science 254, 692–695. doi: 10.1126/science.254.5032.692
Paillet, Y., Berges, L., Hjalten, J., Odor, P., Avon, C., Bernhardt-Roemermann, M., et al. (2010). Biodiversity differences between managed and unmanaged forests: Meta-analysis of species richness in Europe. Conserv. Biol. 24, 101–112. doi: 10.1111/j.1523-1739.2009.01399.x
Pandey, R. R., Sharma, G., Tripathi, S. K., and Singh, A. K. (2007). Litterfall, litter decomposition and nutrient dynamics in a subtropical natural oak forest and managed plantation in Northeastern India. For. Ecol. Manag. 240, 96–104. doi: 10.1016/j.foreco.2006.12.013
Parisien, M.-A., Miller, C., Parks, S. A., DeLancey, E. R., Robinne, F.-N., and Flannigan, M. D. (2016). The spatially varying influence of humans on fire probability in North America. Environ. Res. Lett. 11:075005. doi: 10.1088/1748-9326/11/7/075005
Parker, G. G. (1995). “Structure and microclimate of forest canopies,” in Forest canopies, eds M. D. Lowman and N. M. Nadkarni (San Diego, CA: Academic Press), 73–106.
Parrish, J. D., Braun, D. P., and Unnasch, R. S. (2003). Are we conserving what we say we are? Measuring ecological integrity within protected areas. Bioscience 53, 851–860.
Pautasso, M., Holdenrieder, O., and Stenlid, J. (2005). “Susceptibility to fungal pathogens of forests differing in tree diversity,” in Forest diversity and function: Temperate and boreal systems ecological studies, eds M. Scherer-Lorenzen, C. Körner, and E.-D. Schulze (Berlin: Springer), 263–289. doi: 10.1007/3-540-26599-6_13
Payette, S. (1992). “Fire as a controlling process in the North American boreal forest,” in A systems analysis of the global boreal forest, eds H. H. Shugart, R. Leemans, and G. B. Bonan (New York, NY: Cambridge University Press), 144–169. doi: 10.1046/j.1365-2486.2000.06022.x
Payette, S., and Delwaide, A. (2003). Shift of conifer boreal forest to lichen-heath parkland caused by successive stand disturbances. Ecosystems 6, 540–550. doi: 10.1007/s10021-002-0182-9
Peres, C. A., Emilio, T., Schietti, J., Desmouliere, S. J. M., and Levi, T. (2016). Dispersal limitation induces long-term biomass collapse in overhunted Amazonian forests. Proc. Natl. Acad. Sci. U.S.A. 113, 892–897. doi: 10.1073/pnas.1516525113
Perrault-Hebert, M., Boucher, Y., Fournier, R., Girard, F., Auger, I., Thiffault, N., et al. (2017). Ecological drivers of post-fire regeneration in a recently managed boreal forest landscape of eastern Canada. For. Ecol. Manag. 399, 74–81. doi: 10.1016/j.foreco.2017.05.026
Peterson, G., Allen, C. R., and Holling, C. S. (1998). Ecological resilience, biodiversity, and scale. Ecosystems 1, 6–18. doi: 10.1007/s100219900002
Peterson, K., and Varela, J. B. (2016). INDC analysis: An overview of the forest sector. Toronto: World Wildlife Fund.
Pfeifer, M., Kor, L., Nilus, R., Turner, E., Cusack, J., Lysenko, I., et al. (2016). Mapping the structure of Borneo’s tropical forests across a degradation gradient. Remote Sens. Environ. 176, 84–97. doi: 10.1016/j.rse.2016.01.014
Phillips, O., Hall, P., Gentry, A., Sawyer, S., and Vasquez, R. (1994). Dynamics and species richness of tropical rain-forests. Proc. Natl. Acad. Sci. U.S.A. 91, 2805–2809. doi: 10.1073/pnas.91.7.2805
Phillips, O. L., and Brienen, R. J. W. (2017). Carbon uptake by mature Amazon forests has mitigated Amazon nations’ carbon emissions. Carbon Balance Manag. 12:1. doi: 10.1186/s13021-016-0069-2
Phillips, O. L., Malhi, Y., Higuchi, N., Laurance, W. F., Nunez, P. V., Vasquez, R. M., et al. (1998). Changes in the carbon balance of tropical forests: Evidence from long-term plots. Science 282, 439–442. doi: 10.1126/science.282.5388.439
Phillips, O. L., van der Heijden, G., Lewis, S. L., Lopez-Gonzalez, G., Aragao, L. E. O. C., Lloyd, J., et al. (2010). Drought-mortality relationships for tropical forests. New Phytol. 187, 631–646. doi: 10.1111/j.1469-8137.2010.03359.x
Pimentel, D., Westra, L., and Noss, R. F. (2013). Ecological integrity: Integrating environment, conservation, and health. Washington, DC: Island Press.
Pimm, S. (1984). The complexity and stability of ecosystems. Nature 307, 321–326. doi: 10.1038/307321a0
Pimm, S. L. (1991). The balance of nature?: Ecological issues in the conservation of species and communities. Chicago, IL: University of Chicago Press.
Pimm, S. L., Jenkins, C. N., Abell, R., Brooks, T. M., Gittleman, J. L., Joppa, L. N., et al. (2014). The biodiversity of species and their rates of extinction, distribution, and protection. Science 344:1246752. doi: 10.1126/science.1246752
Ponomarev, E. I. (2008). Geographic conditionality of wildfires and estimation of damages of forests of Central Siberia. For. Res. Pap. 69, 109–115.
Poorter, L., Bongers, F., Aide, T. M., Almeyda Zambrano, A. M., Balvanera, P., Becknell, J. M., et al. (2016). Biomass resilience of Neotropical secondary forests. Nature 530, 211–214. doi: 10.1038/nature16512
Pörtner, H.-O., Scholes, R. J., Agard, J., Archer, E., Bai, X., Barnes, D., et al. (2021). IPBES-IPCC co-sponsored workshop report on biodiversity and climate change. Genéve: Zenodo, 28. doi: 10.5281/ZENODO.4782538
Possingham, H. P., Bode, M., and Klein, C. J. (2015). Optimal conservation outcomes require both restoration and protection. PLoS Biol. 13:e1002052. doi: 10.1371/journal.pbio.1002052
Potapov, P., Hansen, M. C., Laestadius, L., Turubanova, S., Yaroshenko, A., Thies, C., et al. (2017). The last frontiers of wilderness: Tracking loss of intact forest landscapes from 2000 to 2013. Sci. Adv. 3:e1600821. doi: 10.1126/sciadv.1600821
Potapov, P., Yaroshenko, A., Turubanova, S., Dubinin, M., Laestadius, L., Thies, C., et al. (2008). Mapping the world’s intact forest landscapes by remote sensing. Ecol. Soc. 13:51. doi: 10.5751/es-02670-130251
Poyatos, R., Aguade, D., Galiano, L., Mencuccini, M., and Martinez-Vilalta, J. (2013). Drought-induced defoliation and long periods of near-zero gas exchange play a key role in accentuating metabolic decline of Scots pine. New Phytol. 200, 388–401. doi: 10.1111/nph.12278
Prasad, A., Pedlar, J., Peters, M., McKenney, D., Iverson, L., Matthews, S., et al. (2020). Combining US and Canadian forest inventories to assess habitat suitability and migration potential of 25 tree species under climate change. Divers. Distrib. 26, 1142–1159. doi: 10.1111/ddi.13078
Prasad, A. M. (2015). Macroscale intraspecific variation and environmental heterogeneity: Analysis of cold and warm zone abundance, mortality, and regeneration distributions of four eastern US tree species. Ecol. Evol. 5, 5033–5048. doi: 10.1002/ece3.1752
Pretzsch, H. (2005). “Diversity and productivity in forests: Evidence from long-term experimental plots,” in Forest diversity and function: Temperate and boreal systems ecological studies, eds M. Scherer-Lorenzen, C. Körner, and E.-D. Schulze (Berlin: Springer), 41–64. doi: 10.1007/3-540-26599-6_3
Price, O. F., and Bradstock, R. A. (2012). The efficacy of fuel treatment in mitigating property loss during wildfires: Insights from analysis of the severity of the catastrophic fires in 2009 in Victoria, Australia. J. Environ. Manage. 113, 146–157. doi: 10.1016/j.jenvman.2012.08.041
Puettmann, K. J., Wilson, S. M., Baker, S. C., Donoso, P. J., Droessler, L., Amente, G., et al. (2015). Silvicultural alternatives to conventional even-aged forest management - what limits global adoption? For. Ecosyst. 2:8. doi: 10.1186/s40663-015-0031-x
Pugh, T. A. M., Lindeskog, M., Smith, B., Poulter, B., Arneth, A., Haverd, V., et al. (2019). Role of forest regrowth in global carbon sink dynamics. Proc. Natl. Acad. Sci. U.S.A. 116, 4382–4387. doi: 10.1073/pnas.1810512116
Putz, S., Groeneveld, J., Henle, K., Knogge, C., Martensen, A. C., Metz, M., et al. (2014). Long-term carbon loss in fragmented Neotropical forests. Nat. Commun. 5:5037. doi: 10.1038/ncomms6037
Qie, L., Lewis, S. L., Sullivan, M. J. P., Lopez-Gonzalez, G., Pickavance, G. C., Sunderland, T., et al. (2017). Long-term carbon sink in Borneo’s forests halted by drought and vulnerable to edge effects. Nat. Commun. 8:1966. doi: 10.1038/s41467-017-01997-0
Rab, M. A. (2004). Recovery of soil physical properties from compaction and soil profile disturbance caused by logging of native forest in Victorian Central Highlands, Australia. For. Ecol. Manag. 191, 329–340. doi: 10.1016/j.foreco.2003.12.010
Raffa, K. F., Aukema, B. H., Bentz, B. J., Carroll, A. L., Hicke, J. A., Turner, M. G., et al. (2008). Cross-scale drivers of natural disturbances prone to anthropogenic amplification: The dynamics of bark beetle eruptions. Bioscience 58, 501–517. doi: 10.1641/B580607
Randerson, J. T., Chen, Y., Werf, G. R., van der, Rogers, B. M., and Morton, D. C. (2012). Global burned area and biomass burning emissions from small fires. J. Geophys. Res. 117:G04012. doi: 10.1029/2012JG002128
Rappaport, D. I., Morton, D. C., Longo, M., Keller, M., Dubayah, R., and dos-Santos, M. N. (2018). Quantifying long-term changes in carbon stocks and forest structure from Amazon forest degradation. Environ. Res. Lett. 13:065013. doi: 10.1088/1748-9326/aac331
Reed, T. E., Schindler, D. E., and Waples, R. S. (2011). Interacting effects of phenotypic plasticity and evolution on population persistence in a changing climate. Conserv. Biol. 25, 56–63. doi: 10.1111/j.1523-1739.2010.01552.x
Regier, H. A. (1993). “The notion of natural and cultural integrity,” in Ecological integrity and the management of ecosystems, eds S. Woodley, J. Kay, and G. Francis (Delray Beach, FL: St. Lucie Press), 3–18.
Reinhardt, E. D., Keane, R. E., Calkin, D. E., and Cohen, J. D. (2008). Objectives and considerations for wildland fuel treatment in forested ecosystems of the interior western United States. For. Ecol. Manag. 256, 1997–2006. doi: 10.1016/j.foreco.2008.09.016
Rey Benayas, J. M., Newton, A. C., Diaz, A., and Bullock, J. M. (2009). Enhancement of biodiversity and ecosystem services by ecological restoration: A meta-analysis. Science 325, 1121–1124. doi: 10.1126/science.1172460
Ricotta, C., Bajocco, S., Guglietta, D., and Conedera, M. (2018). Assessing the influence of roads on fire ignition: Does land cover matter? Fire 1:24. doi: 10.3390/fire1020024
Roche, P. K., and Campagne, C. S. (2017). From ecosystem integrity to ecosystem condition: A continuity of concepts supporting different aspects of ecosystem sustainability. Curr. Opin. Environ. Sustain. 29, 63–68. doi: 10.1016/j.cosust.2017.12.009
Roe, S., Streck, C., Obersteiner, M., Frank, S., Griscom, B., Drouet, L., et al. (2019). Contribution of the land sector to a 1.5 degrees C world. Nat. Clim. Change 9, 817–828. doi: 10.1038/s41558-019-0591-9
Rogers, B. M., Balch, J. K., Goetz, S. J., Lehmann, C. E. R., and Turetsky, M. (2020). Focus on changing fire regimes: Interactions with climate, ecosystems, and society. Environ. Res. Lett. 15:030201. doi: 10.1088/1748-9326/ab6d3a
Rogers, B. M., Jantz, P., and Goetz, S. J. (2017). Vulnerability of eastern US tree species to climate change. Glob. Change Biol. 23, 3302–3320. doi: 10.1111/gcb.13585
Rogers, B. M., Neilson, R. P., Drapek, R., Lenihan, J. M., Wells, J. R., Bachelet, D., et al. (2011). Impacts of climate change on fire regimes and carbon stocks of the U.S. Pacific Northwest. J. Geophys. Res. Biogeosci. 116:G03037.
Rogers, B. M., Soja, A. J., Goulden, M. L., and Randerson, J. T. (2015). Influence of tree species on continental differences in boreal fires and climate feedbacks. Nat. Geosci. 8, 228–234. doi: 10.1038/ngeo2352
Rogers, B. M., Solvik, K., Hogg, E. H., Ju, J., Masek, J. G., Michaelian, M., et al. (2018). Detecting early warning signals of tree mortality in boreal North America using multiscale satellite data. Glob. Change Biol. 24, 2284–2304. doi: 10.1111/gcb.14107
Root, R. B. (1973). Organization of a plant-arthropod association in simple and diverse habitats: The fauna of collards (Brassica oleracea). Ecol. Monogr. 43, 95–124. doi: 10.2307/1942161
Rosenberg, K. V., Dokter, A. M., Blancher, P. J., Sauer, J. R., Smith, A. C., Smith, P. A., et al. (2019). Decline of the North American avifauna. Science 366, 120–124. doi: 10.1126/science.aaw1313
Ruel, J. (1995). Understanding windthrow - silvicultural implications. For. Chron. 71, 434–445. doi: 10.5558/tfc71434-4
Rutishauser, E., Herault, B., Petronelli, P., and Sist, P. (2016). Tree height reduction after selective logging in a tropical forest. Biotropica 48, 285–289. doi: 10.1111/btp.12326
Ryan, M. G., Binkley, D., and Fownes, J. H. (1997). “Age-related decline in forest productivity: Pattern and process,” in Advances in ecological research, Vol. 27, eds M. Begon and A. H. Fitter (London: Academic Press Ltd), 213–262. doi: 10.1016/S0065-2504(08)60009-4
Saad, C., Boulanger, Y., Beaudet, M., Gachon, P., Ruel, J.-C., and Gauthier, S. (2017). Potential impact of climate change on the risk of windthrow in eastern Canada’s forests. Clim. Change 143, 487–501. doi: 10.1007/s10584-017-1995-z
Sabatini, F. M., Burrascano, S., Keeton, W. S., Levers, C., Lindner, M., Poetzschner, F., et al. (2018). Where are Europe’s last primary forests? Divers. Distrib. 24, 1426–1439. doi: 10.1111/ddi.12778
Sakai, A. K., Allendorf, F. W., Holt, J. S., Lodge, D. M., Molofsky, J., With, K. A., et al. (2001). The population biology of invasive species. Annu. Rev. Ecol. Syst. 32, 305–332. doi: 10.1146/annurev.ecolsys.32.081501.114037
Saleska, S. R., Didan, K., Huete, A. R., and da Rocha, H. R. (2007). Amazon forests green-up during 2005 drought. Science 318, 612–612. doi: 10.1126/science.1146663
Savolainen, O., Pyhajarvi, T., and Knurr, T. (2007). Gene flow and local adaptation in trees. Annu. Rev. Ecol. Evol. Syst. 38, 595–619. doi: 10.1146/annurev.ecolsys.38.091206.095646
Scheller, R. M., and Mladenoff, D. J. (2008). Simulated effects of climate change, fragmentation, and inter-specific competition on tree species migration in northern Wisconsin, USA. Clim. Res. 36, 191–202. doi: 10.3354/cr00745
Scherer-Lorenzen, M., and Schulze, E.-D. (2005). Forest diversity and function: Temperate and boreal systems. Berlin: Springer Science & Business Media.
Schimel, D., Stephens, B. B., and Fisher, J. B. (2015). Effect of increasing CO2 on the terrestrial carbon cycle. Proc. Natl. Acad. Sci. U.S.A. 112, 436–441. doi: 10.1073/pnas.1407302112
Schwartz, G., Ferreira, M., do, S., Lopes, J., and do, C. (2015). Silvicultural intensification and agroforestry systems in secondary tropical forests: A review. Amaz. J. Agric. Environ. Sci. 58, 319–326. doi: 10.4322/rca.1830
Schwartz, N. B., Uriarte, M., DeFries, R., Bedka, K. M., Fernandes, K., Gutierrez-Velez, V., et al. (2017). Fragmentation increases wind disturbance impacts on forest structure and carbon stocks in a western Amazonian landscape. Ecol. Appl. 27, 1901–1915. doi: 10.1002/eap.1576
Seedre, M., Taylor, A. R., Brassard, B. W., Chen, H. Y. H., and Jogiste, K. (2014). Recovery of ecosystem carbon stocks in young boreal forests: A comparison of harvesting and wildfire disturbance. Ecosystems 17, 851–863. doi: 10.1007/s10021-014-9763-7
Seidl, R., Thom, D., Kautz, M., Martin-Benito, D., Peltoniemi, M., Vacchiano, G., et al. (2017). Forest disturbances under climate change. Nat. Clim. Change 7, 395–402. doi: 10.1038/NCLIMATE3303
Sheil, D., and Murdiyarso, D. (2009). How forests attract rain: An examination of a new hypothesis. Bioscience 59, 341–347. doi: 10.1525/bio.2009.59.4.12
Shestakova, T. A., Mackey, B., Hugh, S., Dean, J., Kukavskaya, E. A., Laflamme, J., et al. (2022). Mapping forest stability within major biomes using canopy indices derived from MODIS time series. Remote Sens. 14:3813. doi: 10.3390/rs14153813
Shimamoto, C. Y., Padial, A. A., da Rosa, C. M., and Marques, M. C. M. (2018). Restoration of ecosystem services in tropical forests: A global meta-analysis. PLoS One 13:e0208523. doi: 10.1371/journal.pone.0208523
Shvetsov, E. G., Kukavskaya, E. A., Shestakova, T. A., Laflamme, J., and Rogers, B. M. (2021). Increasing fire and logging disturbances in Siberian boreal forests: A case study of the Angara region. Environ. Res. Lett. 16:115007. doi: 10.1088/1748-9326/ac2e37
Siegert, F., Ruecker, G., Hinrichs, A., and Hoffmann, A. A. (2001). Increased damage from fires in logged forests during droughts caused by El Nino. Nature 414, 437–440. doi: 10.1038/35106547
Siitonen, J. (2001). Forest management, coarse woody debris and saproxylic organisms: Fennoscandian boreal forests as an example. Ecol. Bull. 49, 11–41.
Siitonen, J., Martikainen, P., Punttila, P., and Rauh, J. (2000). Coarse woody debris and stand characteristics in mature managed and old-growth boreal mesic forests in southern Finland. For. Ecol. Manag. 128, 211–225. doi: 10.1016/S0378-1127(99)00148-6
Silva Junior, C. H. L., Pessoa, A. C. M., Carvalho, N. S., Reis, J. B. C., Anderson, L. O., and Aragao, L. E. O. C. (2021). The Brazilian Amazon deforestation rate in 2020 is the greatest of the decade. Nat. Ecol. Evol. 5, 144–145. doi: 10.1038/s41559-020-01368-x
Simard, M., Lecomte, N., Bergeron, Y., Bernier, P. Y., and Pare, D. (2007). Forest productivity decline caused by successional paludification of boreal soils. Ecol. Appl. 17, 1619–1637. doi: 10.1890/06-1795.1
Simler-Williamson, A. B., Rizzo, D. M., and Cobb, R. C. (2019). “Interacting effects of global change on forest pest and pathogen dynamics,” in Annual review of ecology, evolution, and systematics, Vol. 50, ed. D. J. Futuyma (Palo Alto, CA: Annual Reviews), 381–403. doi: 10.1146/annurev-ecolsys-110218-024934
Sist, P., Mazzei, L., Blanc, L., and Rutishauser, E. (2014). Large trees as key elements of carbon storage and dynamics after selective logging in the Eastern Amazon. For. Ecol. Manag. 318, 103–109. doi: 10.1016/j.foreco.2014.01.005
Sitch, S., Smith, B., Prentice, I. C., Arneth, A., Bondeau, A., Cramer, W., et al. (2003). Evaluation of ecosystem dynamics, plant geography and terrestrial carbon cycling in the LPJ dynamic global vegetation model. Glob. Change Biol. 9, 161–185. doi: 10.1046/j.1365-2486.2003.00569.x
Skene, J. (2020). The logging loophole: How the logging industry’s unregulated carbon emissions undermine Canada’s climate goals. New York, NY: Natural Resources Defense Council.
Smith, P., Davis, S. J., Creutzig, F., Fuss, S., Minx, J., Gabrielle, B., et al. (2016). Biophysical and economic limits to negative CO2 emissions. Nat. Clim. Change 6, 42–50. doi: 10.1038/NCLIMATE2870
Soja, A. J., Tchebakova, N. M., French, N. H. F., Flannigan, M. D., Shugart, H. H., Stocks, B. J., et al. (2007). Climate-induced boreal forest change: Predictions versus current observations. Glob. Planet. Change 56, 274–296. doi: 10.1016/j.gloplacha.2006.07.028
Spracklen, D. V., Arnold, S. R., and Taylor, C. M. (2012). Observations of increased tropical rainfall preceded by air passage over forests. Nature 489, 282–285. doi: 10.1038/nature11390
Stahlheber, K. A., Crispin, K. L., Anton, C., and D’Antonio, C. M. (2015). The ghosts of trees past: Savanna trees create enduring legacies in plant species composition. Ecology 96, 2510–2522. doi: 10.1890/14-2035.1
Staver, A. C., Archibald, S., and Levin, S. A. (2011). The global extent and determinants of Savanna and forest as alternative biome states. Science 334, 230–232. doi: 10.1126/science.1210465
Steffen, W., Richardson, K., Rockstrom, J., Cornell, S. E., Fetzer, I., Bennett, E. M., et al. (2015). Planetary boundaries: Guiding human development on a changing planet. Science 347:1259855. doi: 10.1126/science.1259855
Stephenson, N. L., Das, A. J., Condit, R., Russo, S. E., Baker, P. J., Beckman, N. G., et al. (2014). Rate of tree carbon accumulation increases continuously with tree size. Nature 507, 90–93. doi: 10.1038/nature12914
Sterman, J., Moomaw, W., Rooney-Varga, J. N., and Siegel, L. (2022). Does wood bioenergy help or harm the climate? Bull. At. Sci. 78, 128–138. doi: 10.1080/00963402.2022.2062933
Sthultz, C. M., Gehring, C. A., and Whitham, T. G. (2009). Deadly combination of genes and drought: Increased mortality of herbivore-resistant trees in a foundation species. Glob. Change Biol. 15, 1949–1961. doi: 10.1111/j.1365-2486.2009.01901.x
Stier-Jarmer, M., Throner, V., Kirschneck, M., Immich, G., Frisch, D., and Schuh, A. (2021). The psychological and physical effects of forests on human health: A systematic review of systematic reviews and meta-analyses. Int. J. Environ. Res. Public Health 18:1770. doi: 10.3390/ijerph18041770
Stone, C., Hudak, A., and Morgan, P. (2004). “Forest harvest can increase subsequent forest fire severity,” in Proceedings of the second international symposium on fire economics, planning, and policy: A global view, (Washington, DC: USDA Forest Service).
Taubert, F., Fischer, R., Groeneveld, J., Lehmann, S., Mueller, M. S., Roedig, E., et al. (2018). Global patterns of tropical forest fragmentation. Nature 554, 519–522. doi: 10.1038/nature25508
Taye, F. A., Folkersen, M. V., Fleming, C. M., Buckwell, A., Mackey, B., Diwakar, K. C., et al. (2021). The economic values of global forest ecosystem services: A meta-analysis. Ecol. Econ. 189:107145. doi: 10.1016/j.ecolecon.2021.107145
Taylor, B. D., and Goldingay, R. L. (2010). Roads and wildlife: Impacts, mitigation and implications for wildlife management in Australia. Wildl. Res. 37, 320–331. doi: 10.1071/WR09171
Taylor, C., Blair, D., Keith, H., and Lindenmayer, D. (2019). Modelling water yields in response to logging and Representative Climate Futures. Sci. Total Environ. 688, 890–902. doi: 10.1016/j.scitotenv.2019.06.298
Taylor, C., McCarthy, M. A., and Lindenmayer, D. B. (2014). Nonlinear effects of stand age on fire severity. Conserv. Lett. 7, 355–370. doi: 10.1111/conl.12122
Taylor, S. L., and MacLean, D. A. (2009). Legacy of insect defoliators: Increased wind-related mortality two decades after a spruce budworm outbreak. For. Sci. 55, 256–267.
Tegegne, Y. T., Cramm, M., and van Brusselen, J. (2018). Sustainable forest management, FLEGT, and REDD plus: Exploring interlinkages to strengthen forest policy coherence. Sustainability 10:4841. doi: 10.3390/su10124841
Terrail, R., Morin-Rivat, J., de Lafontaine, G., Fortin, M.-J., and Arseneault, D. (2020). Effects of 20th-century settlement fires on landscape structure and forest composition in eastern Quebec, Canada. J. Veg. Sci. 31, 40–52. doi: 10.1111/jvs.12832
Thom, D., Golivets, M., Edling, L., Meigs, G. W., Gourevitch, J. D., Sonter, L. J., et al. (2019). The climate sensitivity of carbon, timber, and species richness covaries with forest age in boreal-temperate North America. Glob. Change Biol. 25, 2446–2458. doi: 10.1111/gcb.14656
Thompson, I., Mackey, B., McNulty, S., and Mosseler, A. (2009). Forest resilience, biodiversity, and climate change. A synthesis of the biodiversity/resilience/stability relationship in forest ecosystems. Montreal: Secretariat of the Convention on Biological Diversity.
Thompson, I. D., Guariguata, M. R., Okabe, K., Bahamondez, C., Nasi, R., Heymell, V., et al. (2013). An operational framework for defining and monitoring forest degradation. Ecol. Soc. 18:20. doi: 10.1016/j.cub.2020.06.032
Thompson, J. R., Spies, T. A., and Ganio, L. M. (2007). Reburn severity in managed and unmanaged vegetation in a large wildfire. Proc. Natl. Acad. Sci. U.S.A. 104, 10743–10748. doi: 10.1073/pnas.0700229104
Tierney, G. L., Faber-Langendoen, D., Mitchell, B. R., Shriver, W. G., and Gibbs, J. P. (2009). Monitoring and evaluating the ecological integrity of forest ecosystems. Front. Ecol. Environ. 7, 308–316. doi: 10.1890/070176
Tilman, D. (1996). Biodiversity: Population versus ecosystem stability. Ecology 77, 350–363. doi: 10.2307/2265614
Tilman, D. (1997). “Biodiversity and ecosystem functioning,” in Nature’s services: Societal dependence on natural ecosystems, ed. G. C. Daily (Washington, DC: Island Press), 93–112.
Tilman, D., Isbell, F., and Cowles, J. M. (2014). “Biodiversity and ecosystem functioning,” in Annual review of ecology, evolution, and systematics, Vol. 45, ed. D. J. Futuyma (Palo Alto, CA: Annual Reviews), 471–493. doi: 10.1146/annurev-ecolsys-120213-091917
Tilman, D., and Lehman, C. (2001). “Biodiversity, composition, and ecosystem processes: Theory and concepts,” in The functional consequences of biodiversity: Empirical progress and theoretical extensions, eds A. P. Kinzig, S. W. Pacala, and D. Tilman (Princeton, NJ: Princeton University Press), 9–41.
Tilman, D., Lehman, C. L., and Thomson, K. T. (1997). Plant diversity and ecosystem productivity: Theoretical considerations. Proc. Natl. Acad. Sci. U.S.A. 94, 1857–1861. doi: 10.1073/pnas.94.5.1857
Timko, J., Le Billon, P., Zerriffi, H., Honey-Roses, J., de la Roche, I., Gaston, C., et al. (2018). A policy nexus approach to forests and the SDGs: Tradeoffs and synergies. Curr. Opin. Environ. Sustain. 34, 7–12. doi: 10.1016/j.cosust.2018.06.004
Tomas Ibarra, J., and Martin, K. (2015). Biotic homogenization: Loss of avian functional richness and habitat specialists in disturbed Andean temperate forests. Biol. Conserv. 192, 418–427. doi: 10.1016/j.biocon.2015.11.008
Trenberth, K. E., Dai, A., van der Schrier, G., Jones, P. D., Barichivich, J., Briffa, K. R., et al. (2014). Global warming and changes in drought. Nat. Clim. Change 4, 17–22. doi: 10.1038/NCLIMATE2067
Trombulak, S. C., and Frissell, C. A. (2000). Review of ecological effects of roads on terrestrial and aquatic communities. Conserv. Biol. 14, 18–30. doi: 10.1046/j.1523-1739.2000.99084.x
Turubanova, S., Potapov, P. V., Tyukavina, A., and Hansen, M. C. (2018). Ongoing primary forest loss in Brazil, democratic republic of the Congo, and Indonesia. Environ. Res. Lett. 13:074028. doi: 10.1088/1748-9326/aacd1c
Tyukavina, A., Hansen, M. C., Potapov, P. V., Krylov, A. M., and Goetz, S. J. (2016). Pan-tropical hinterland forests: Mapping minimally disturbed forests. Glob. Ecol. Biogeogr. 25, 151–163. doi: 10.1111/geb.12394
Uganda Bureau of Statistics [UBOS] (2020). Towards ecosystem accounts for Uganda. Uganda natural capital accounting program, WAVES partnership program. Washington, DC: World Bank Group.
Uhl, C., and Kauffman, J. (1990). Deforestation, fire susceptibility, and potential tree responses to fire in the Eastern Amazon. Ecology 71, 437–449. doi: 10.2307/1940299
Umunay, P. M., Gregoire, T. G., Gopalakrishna, T., Ellis, P. W., and Putz, F. E. (2019). Selective logging emissions and potential emission reductions from reduced-impact logging in the Congo Basin. For. Ecol. Manag. 437, 360–371. doi: 10.1016/j.foreco.2019.01.049
UNFCCC (2002). Report of the conference of the parties on its seventh session, held at Marrakesh from 29 October to 10 November 2001. Marrakesh: United Nations.
United Nations [UN] (2021). Glasgow leaders’ declaration on forests and land use. UN Climate Change Conference COP26 SEC – Glasg. 2021. Available Online at: https://ukcop26.org/glasgow-leaders-declaration-on-forests-and-land-use/ [accessed August 31, 2022].
United Nations Climate Change (2021). Glasgow leaders’ declaration on forests and land use. UN Climate Change Conference COP26 SEC – Glasg. 2021. Available Online at: https://ukcop26.org/glasgow-leaders-declaration-on-forests-and-land-use/ [accessed April 19, 2022].
Vallejo-Ramos, M., Moreno-Calles, A. I., and Casas, A. (2016). TEK and biodiversity management in agroforestry systems of different socio-ecological contexts of the Tehuacan Valley. J. Ethnobiol. Ethnomed. 12:31. doi: 10.1186/s13002-016-0102-2
van der Sande, M. T., Poorter, L., Kooistra, L., Balvanera, P., Thonicke, K., Thompson, J., et al. (2017). Biodiversity in species, traits, and structure determines carbon stocks and uptake in tropical forests. Biotropica 49, 593–603. doi: 10.1111/btp.12453
van Haaren, B., Andreoli, R., Dumas, P., Lille, D., and Géraux, H. (2021). Characterizing forest ecosystem services degradation within water catchments. An application to a South West Pacific tropical and semi-arid island (New Caledonia). Environ. Chall. 4:100151. doi: 10.1016/j.envc.2021.100151
Vardon, M., Keith, H., and Lindenmayer, D. (2019). Accounting and valuing the ecosystem services related to water supply in the Central Highlands of Victoria, Australia. Ecosyst. Serv. 39:101004. doi: 10.1016/j.ecoser.2019.101004
Venier, L. A., Thompson, I. D., Fleming, R., Malcolm, J., Aubin, I., Trofymow, J. A., et al. (2014). Effects of natural resource development on the terrestrial biodiversity of Canadian boreal forests. Environ. Rev. 22, 457–490. doi: 10.1139/er-2013-0075
Venier, L. A., Walton, R., Thompson, I. D., Arsenault, A., and Titus, B. D. (2018). A review of the intact forest landscape concept in the Canadian boreal forest: Its history, value, and measurement. Environ. Rev. 26, 369–377. doi: 10.1139/er-2018-0041
Venter, O., Sanderson, E. W., Magrach, A., Allan, J. R., Beher, J., Jones, K. R., et al. (2016a). Global terrestrial human footprint maps for 1993 and 2009. Sci. Data 3:160067. doi: 10.1038/sdata.2016.67
Venter, O., Sanderson, E. W., Magrach, A., Allan, J. R., Beher, J., Jones, K. R., et al. (2016b). Sixteen years of change in the global terrestrial human footprint and implications for biodiversity conservation. Nat. Commun. 7:12558. doi: 10.1038/ncomms12558
Verdone, M., and Seidl, A. (2017). Time, space, place, and the Bonn Challenge global forest restoration target. Restor. Ecol. 25, 903–911. doi: 10.1111/rec.12512
Vickerman, S., and Kagan, J. S. (2014). Assessing ecological integrity across jurisdictions and scales. Portland: Institute of Natural Resources.
von Arx, G., Pannatier, E. G., Thimonier, A., and Rebetez, M. (2013). Microclimate in forests with varying leaf area index and soil moisture: Potential implications for seedling establishment in a changing climate. J. Ecol. 101, 1201–1213. doi: 10.1111/1365-2745.12121
Walker, B. (1995). Conserving biological diversity through ecosystem resilience. Conserv. Biol. 9, 747–752. doi: 10.1046/j.1523-1739.1995.09040747.x
Walker, W. S., Gorelik, S. R., Baccini, A., Luis Aragon-Osejo, J., Josse, C., Meyer, C., et al. (2020). The role of forest conversion, degradation, and disturbance in the carbon dynamics of Amazon indigenous territories and protected areas. Proc. Natl. Acad. Sci. U.S.A. 117, 3015–3025. doi: 10.1073/pnas.1913321117
Walker, X. J., Baltzer, J. L., Bourgeau-Chavez, L., Day, N. J., Dieleman, C. M., Johnstone, J. F., et al. (2020). Patterns of ecosystem structure and wildfire carbon combustion across six ecoregions of the North American boreal forest. Front. For. Glob. Change 3:87. doi: 10.3389/ffgc.2020.00087
Ward, D. S., Kloster, S., Mahowald, N. M., Rogers, B. M., Randerson, J. T., and Hess, P. G. (2012). The changing radiative forcing of fires: Global model estimates for past, present and future. Atmos. Chem. Phys. 12, 10857–10886. doi: 10.5194/acp-12-10857-2012
Warman, R. D. (2014). Global wood production from natural forests has peaked. Biodivers. Conserv. 23, 1063–1078. doi: 10.1007/s10531-014-0633-6
Watkins, K. (2014). Grain fish money: Financing Africa’s green and blue revolutions - world. Geneva: Africa Progress Panel.
Watson, J. E. M., Evans, T., Venter, O., Williams, B., Tulloch, A., Stewart, C., et al. (2018). The exceptional value of intact forest ecosystems. Nat. Ecol. Evol. 2, 599–610. doi: 10.1038/s41559-018-0490-x
Watt, A. D. (1992). “Insect pest population dynamics: Effects of tree species diversity,” in The ecology of mixed species stands of trees, eds M. G. R. Cannell, D. C. Malcolm, and P. A. Robertson (Oxford: Blackwell), 267–275.
Wilkie, D., Shaw, E., Rotberg, F., Morelli, G., and Auzel, P. (2000). Roads, development, and conservation in the Congo basin. Conserv. Biol. 14, 1614–1622. doi: 10.1046/j.1523-1739.2000.99102.x
Williams, B. A., Venter, O., Allan, J. R., Atkinson, S. C., Rehbein, J. A., Ward, M., et al. (2020). Change in terrestrial human footprint drives continued loss of intact ecosystems. One Earth 3, 371–382. doi: 10.1016/j.oneear.2020.08.009
Williamson, G. B., Laurance, W. F., Oliveira, A. A., Delamonica, P., Gascon, C., Lovejoy, T. E., et al. (2000). Amazonian tree mortality during the 1997 El Nino drought. Conserv. Biol. 14, 1538–1542. doi: 10.1046/j.1523-1739.2000.99298.x
Wirth, C. (2005). “Fire regime and tree diversity in boreal forests: Implications for the carbon cycle,” in Forest diversity and function ecological studies, eds D. M. Scherer-Lorenzen, P. D. C. Körner, and P. D. E.-D. Schulze (Berlin: Springer), 309–344.
Wright, A. J., Wardle, D. A., Callaway, R., and Gaxiola, A. (2017). The overlooked role of facilitation in biodiversity experiments. Trends Ecol. Evol. 32, 383–390. doi: 10.1016/j.tree.2017.02.011
Wright, S. J., Stoner, K. E., Beckman, N., Corlett, R. T., Dirzo, R., Muller-Landau, H. C., et al. (2007). The plight of large animals in tropical forests and the consequences for plant regeneration. Biotropica 39, 289–291. doi: 10.1111/j.1744-7429.2007.00293.x
Wurtzebach, Z., and Schultz, C. (2016). Measuring ecological integrity: History, practical applications, and research opportunities. Bioscience 66, 446–457. doi: 10.1093/biosci/biw037
Xu, L., Chen, N., and Zhang, X. (2019). Global drought trends under 1.5 and 2 degrees C warming. Int. J. Climatol. 39, 2375–2385. doi: 10.1002/joc.5958
Yachi, S., and Loreau, M. (1999). Biodiversity and ecosystem productivity in a fluctuating environment: The insurance hypothesis. Proc. Natl. Acad. Sci. U.S.A. 96, 1463–1468. doi: 10.1073/pnas.96.4.1463
Yi, C., Wei, S., and Hendrey, G. (2014). Warming climate extends dryness-controlled areas of terrestrial carbon sequestration. Sci. Rep. 4:5472. doi: 10.1038/srep05472
Zalman, J., Ellis, P. W., Crabbe, S., and Roopsind, A. (2019). Opportunities for carbon emissions reduction from selective logging in Suriname. For. Ecol. Manag. 439, 9–17. doi: 10.1016/j.foreco.2019.02.026
Zhang, Q.-H., Liu, G.-T., Schlyter, F., Birgersson, G., Anderson, P., and Valeur, P. (2001). Olfactory responses of Ips duplicatus from Inner Mongolia, China to nonhost leaf and bark volatiles. J. Chem. Ecol. 27, 995–1009. doi: 10.1023/A:1010395221953
Zhao, M., and Zhou, G.-S. (2006). Carbon storage of forest vegetation in China and its relationship with climatic factors. Clim. Change 74, 175–189. doi: 10.1007/s10584-006-6775-0
Zhou, S., Zhang, Y., Williams, A. P., and Gentine, P. (2019). Projected increases in intensity, frequency, and terrestrial carbon costs of compound drought and aridity events. Sci. Adv. 5:eaau5740. doi: 10.1126/sciadv.aau5740
Zimmerman, B. L., and Kormos, C. F. (2012). Prospects for sustainable logging in tropical forests. Bioscience 62, 479–487. doi: 10.1525/bio.2012.62.5.9
Zlonis, E. J., and Niemi, G. J. (2014). Avian communities of managed and wilderness hemiboreal forests. For. Ecol. Manag. 328, 26–34. doi: 10.1016/j.foreco.2014.05.017
Zscheischler, J., Mahecha, M. D., von Buttlar, J., Harmeling, S., Jung, M., Rammig, A., et al. (2014). A few extreme events dominate global interannual variability in gross primary production. Environ. Res. Lett. 9:035001. doi: 10.1088/1748-9326/9/3/035001
Zummo, L. M., and Friedland, A. J. (2011). Soil carbon release along a gradient of physical disturbance in a harvested northern hardwood forest. For. Ecol. Manag. 261, 1016–1026. doi: 10.1016/j.foreco.2010.12.022
Keywords: Paris Agreement, primary forest, carbon, forest degradation, deforestation
Citation: Rogers BM, Mackey B, Shestakova TA, Keith H, Young V, Kormos CF, DellaSala DA, Dean J, Birdsey R, Bush G, Houghton RA and Moomaw WR (2022) Using ecosystem integrity to maximize climate mitigation and minimize risk in international forest policy. Front. For. Glob. Change 5:929281. doi: 10.3389/ffgc.2022.929281
Received: 26 April 2022; Accepted: 06 October 2022;
Published: 25 October 2022.
Edited by:
Arshad Ali, Hebei University, ChinaReviewed by:
Osbert Jianxin Sun, Beijing Forestry University, ChinaTanzeel J. A. Farooqi, Yibin University, China
Copyright © 2022 Rogers, Mackey, Shestakova, Keith, Young, Kormos, DellaSala, Dean, Birdsey, Bush, Houghton and Moomaw. This is an open-access article distributed under the terms of the Creative Commons Attribution License (CC BY). The use, distribution or reproduction in other forums is permitted, provided the original author(s) and the copyright owner(s) are credited and that the original publication in this journal is cited, in accordance with accepted academic practice. No use, distribution or reproduction is permitted which does not comply with these terms.
*Correspondence: Brendan M. Rogers, brogers@woodwellclimate.org