The potential therapeutic roles of dental pulp stem cells in spinal cord injury
- 1Department of Stomatology, Hangzhou Xixi Hospital Affiliated to Zhejiang Chinese Medical University, Hangzhou, China
- 2Department of Anesthesiology and Surgery, Qingdao Municipal Hospital Group, Qingdao, China
- 3Yuncheng Central Hospital Affiliated to Shanxi Medical University, Yuncheng, China
- 4College of Pharmacy, Guizhou Medical University, Guiyang, China
- 5Department of Orthopedics, The First Affiliated Hospital, College of Medicine, Zhejiang University, Hangzhou, China
- 6The Eighth Clinical Medical College of Shanxi Medical University, Yuncheng, China
Spinal cord injury (SCI) can lead to serious functional disorders, which have serious impacts on patients and society. The current traditional treatments of SCI are not effective the injured spinal cord is difficult to repair and regenerate. In recent years, stem cell transplantation for the treatment of SCI has been a hot research topic. Dental pulp stem cells have strong abilities of self-renewal and multi-directional differentiation, and have been applied for tissue engineering and regenerative medicine. And dental pulp stem cells have certain advantages in neuro-regenetation, bringing new hope to biotherapy for SCI. This article reviews the characteristics of dental pulp stem cells and their research progress in the treatment of SCI.
1 Introduction
Spinal cord injury (SCI) is one of the most devastating of traumatic events, which can lead to irreversible motor and sensory dysfunction below the lesion site (McDonald and Sadowsky, 2002; Eli et al., 2021). Generally, the pathophysiology of SCI usually consists of the primary injury and secondary injuries (McDonald and Sadowsky, 2002). The primary injury mainly refers to the mechanical injury involving falls from height, sports and traffic accidents. Following the primary injury, several pathological changes occur in the secondary injury, including hemorrhage, edema, demyelination, axonal and neuronal necrosis and inflammatory reaction, which primarily correlate with patient’s morbidity and mortality (Rowland et al., 2008; Ahuja and Fehlings, 2016). Therefore, the current neuroprotective and regenerative strategies mainly targeting the secondary injury are expected to be used as therapy for SCI (McDonald and Sadowsky, 2002; Rowland et al., 2008; Ahuja and Fehlings, 2016).
In recent years, biological regenerative therapy with stem cells has attracted more attention in the treatment of SCI (Bonaventura et al., 2020; Andrzejewska et al., 2021; Zipser et al., 2022). Previous experimental studies demonstrated that in animal models of SCI, stem cell therapy can improve motor and sensory functions through multifarious mechanisms, involving restoration of cell population, paracrine action, and microenvironment modulation (Bonaventura et al., 2020; Andrzejewska et al., 2021). Therefore, stem cell therapy is considered as the most promising regenerative treatment of SCI.
Mesenchymal stem cells (MSCs) have been extensively studied for biological regenerative medicine. MSCs can be sourced from a variety of tissues and organs, including bone marrow, adipose tissue, umbilical cord, blood, skeletal muscle, and oral cavity (Bonaventura et al., 2020; Andrzejewska et al., 2021). Oral-derived mesenchymal stem cells have attracted growing attention in the regenerative field based on their unique features including multipotency, easy accessibility, genomic stability, and faster proliferation rates (Bianco et al., 2016; Xu et al., 2019; Bonaventura et al., 2020; Luzuriaga et al., 2021). Dental pup stem cell (DPSC) has remained as the most extensively studied subtype of oral-derived mesenchymal stem cells (Bianco et al., 2016; Xu et al., 2019; Bonaventura et al., 2020; Bar et al., 2021; Luzuriaga et al., 2021; Mattei and Monache, 2023). Derived from the neural crest, DPSCs exhibit notable neuroregenerative potential, neurotrophic effects, and immunomodulation, which indicate that DPSCs is an ideal cell source for the injured spinal cord regeneration (Bianco et al., 2016; Xu et al., 2019; Bonaventura et al., 2020; Bar et al., 2021; Luzuriaga et al., 2021; Mattei and Monache, 2023). In this review, we will summarize the biological characteristics of DPSCs and the recent progress for the application of DPSCs in SCI treatment, with a focus on their possible regenerative mechanisms for future application in DPSC-based therapy.
2 The pathophysiology of the secondary injury following SCI
The secondary damage of SCI mainly involved cell death, inflammatory response, axonal collapse and demyelination, glial scar formation (Figure 1). In the acute phase of SCI, a primary mechanical damage disrupts spinal cord tissue homeostasis, which activates resident microglia and recruit macrophages from the bloodstream to the lesion site (Schwab et al., 2006; Venkatesh et al., 2019). These inflammatory cells secret multiple neurotoxic factors that induce necrotic and apoptotic death in neurons, astrocytes, and oligodendrocytes (Dumont et al., 2001; Kwon et al., 2004; Fan et al., 2018; Venkatesh et al., 2019). And the initial mechanical injury, ischemia, inflammatory response can cause irreversible axonal damage, and the necrosis and apoptosis of oligodendrocytes, which eventually results in the process of demyelination (Dumont et al., 2001; Kwon et al., 2004; Fan et al., 2018; Venkatesh et al., 2019). In addition, reactive astrocytes and oligodendrocytes near the injured spinal cord center generate chondroitin sulfate proteoglycans and myelin proteins, which can cause growth cone collapse, neurite retraction and inhibition of axon regeneration (Siebert et al., 2014). Furthermore, the chondroitin sulfate proteoglycans generated by activated microglia, macrophages and astrocytes, can form a glial scar that also inhibits spontaneous axonal regeneration (Ohtake and Li, 2015). Finally, multiple pathogenic signals synergistically accelerate progressive neuronal deterioration after SCI. Thus, an ideal biological therapy for SCI not only can markedly reduce secondary damage, but also replace the damaged neuron, axons, and circuits within the spinal cord. Transplantation of stem cells has been applied for biological treatment in animal models of SCI, with favorable functional recovery (Ahuja and Fehlings, 2016; Andrzejewska et al., 2021; Zipser et al., 2022). Despite the biological characteristics of various types of stem cells differ, the therapeutic benefits of stem cells in SCI have been reported including replacing lost cells, modulating inflammatory reaction, improving the microenvironment and promoting regeneration (Zipser et al., 2022).
3 Biological characteristics of DPSCs
DPSCs deriving from child or adult human dental pulp were initially discovered by Gronthos and colleagues in 2000 (Gronthos et al., 2000). The dental pulp tissue generated by the neural crest, is rich in odontoblasts, blood vessels, nerve fibres, immune cells and mesenchymal stem cells. DPSCs isolated from dental pulp, can express neural markers, including glial fibrillary acidic protein (GFAP), β-III tubulin, and microtubule-associated protein-2 (MAP-2) (Martens et al., 2012; Li et al., 2019). In addition, DPSCs have been found to express mesenchymal-like phenotypic markers (CD29, CD90, and CD73) (Li et al., 2019), express stemness-related markers (Oct-4, Nanog, and Sox-2) (Kerkis et al., 2006), cytoskeleton-related markers (Nestin and Vimentin), tumor necrosis factor receptor superfamily proteins (CD40, CD120a, CD261, CD262, CD264 and CD266), some integrins (alpha-4, alpha-6 and alpha-10) and IL receptors (CD121a, CD130, CD213a1, CD217 and CDw210b) (Niehage et al., 2016). Recently, several new special markers are identified, such as Calreticulin, Annexin A5, and Rho GDP dissociation inhibitor alpha (Lei et al., 2021). Isolated DPSCs can not only maintain and repair periodontal tissue, with the feature of high proliferation rate, but also show plasticity in multi-lineage differentiation. Several in vitro studies have confirmed DPSCs have the potential to differentiate into multiple cell types such as osteoblasts-, chondrocytes-, adipocytes-, odontoblasts-, neural- and myocytes-like cells (Fu et al., 2023) (Figure 2).
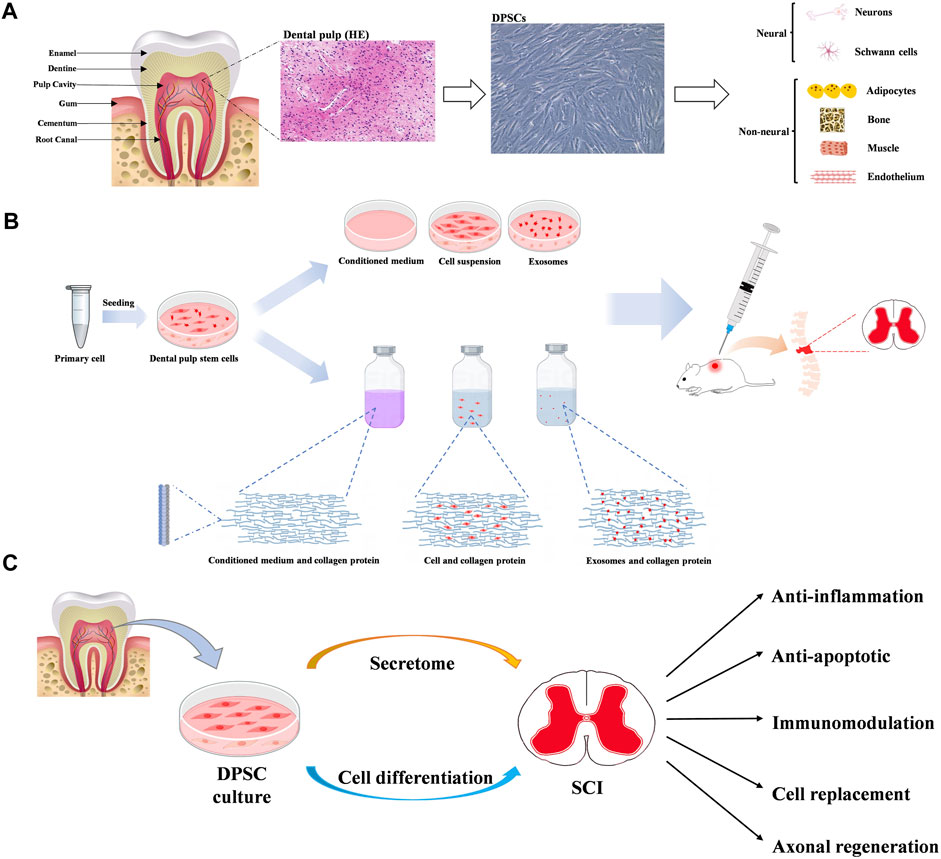
Figure 2. (A) Diagram of tooth, dental pulp and dental pulp stem cells (DPSCs). And multiple differentiation potentials of DPSCs into various cell types. (B) The main mechanisms of dental pulp stem cells in neuroprotection. (C) The potential benefits of dental pulp stem cells in therapy for spinal cord injury.
Furthermore, DPSCs have some biological advantages over other tissue-derived MSCs. Because the dental pulp has similar structure like neurovascular tissue, DPSCs have very higher ability to generate neural and vascular cells than other MSCs (Luzuriaga et al., 2020; Fu et al., 2023). And It has been demonstrated the secretion of nerve growth factor (NGF) and brain derived neurotrophic factor (BDNF) by DPSCs are very higher than bone mesenchymal stem cells. (McDonald and Sadowsky, 2002; Eli et al., 2021). The secrecion of vascular endothelial growth factor-A and vascular endothelial growth factor-D by DPSCs are higher than other MSCs (Rowland et al., 2008; Ahuja and Fehlings, 2016). These results indicate DPSCs have very higher capacity for angiogenesis and neurogenesis.
4 The potential mechanisms of DPSCs in SCI
Previous studies have demonstrated DPSCs show the potential therapies for SCI through multiple mechanisms, mainly involving neuronal differentiation, paracrine effects and exosome secretion (Xu et al., 2019; Bonaventura et al., 2020; Fu et al., 2023) (Figure 2).
4.1 Neuronal-like differentiation from hDPSCs
DPSCs retain the properties of neural crest cells, which possess the differentiation capacity into neural crest-derived tissue. Several neural markers can be expressed in non-differentiated DPSCs, such as musashi12, nestin, MAP2ab, βIII-tubulin, N-tubulin, and neurogenin-2 (Kawashima, 2012; Luzuriaga et al., 2019a). Thus, DPSCs have been promoted as potential candidates for damaged neuron replacements. Previous studies have demonstrated DPSCs not only can express several typical neural stem/progenitor markers under the specific stimulation, but also differentiate toward phenotypes of functionally active neurons, with the higher expression of neurogenin-2, neuron-specific enolase, neurofilament-M and glial fibrillary acidic protein, and activation of voltage-gated sodium and potassium channels (Luzuriaga et al., 2021). Multiple stimulations have been used to differentiate DPSCs into neuron-like cells, involving several differentiating protocols containing complex mixture of supplements (Luzuriaga et al., 2019b), optogenetics (Niyazi et al., 2020), activation of K+ channels (Kogo et al., 2020), and Rho kinase inhibitor (Srikawnawan et al., 2022). Darvishi et al. demonstrated human DPSCs can differentiate to functional motor neuron in organotypic culture system containing growth factors in two stages (Darvishi et al., 2021). Differentiated DPSCs not only display motor neuron-like morphology, with Nissl bodies in the cytoplasm, but also express several motor neuron specific genes in post-induction stage, such as Olig2, Islet-1 and HB9. In animal models of SCI, transplantation of dental-derived mesenchymal stem cell alone or as part of designed engineered tissue also have been shown to differentiate neuron-like phenotype, replacing the defective neuronal tissue and improving functional recovery after SCI (Zhang et al., 2016; Yang et al., 2017; Kabatas et al., 2018; Qian et al., 2023; Zhou et al., 2023). Recently, Qian et al. found the DPSC-loaded microspheres have improved stemness and higher neurogenic differentiation potential of DPSCs, promoting neural tissue regeneration in rat models of SCI (Qian et al., 2023). The combination of biomaterials with DPSCs represents a promising approach to enhance neurogenic differentiation of DPSCs. The combination of chitosan 3D porous scaffolds with bFGF enhances the expression of neural markers in DPSCs through the activation of the extracellular signal-regulated kinase (ERK) pathway (Zhang et al., 2016). Moreover, usage of the DPSC-chitosan grafts improve locomotor function in animal models of SCI, by the secretion of BDNF, GDNF and NT-3, reducing the accumulation of active-caspase 3, and impairing axonal loss and degradation. Zhou et al. reported the roles of bioactive material zeolitic imidazolate framework 8 (ZIF-8) on neural differentiation of DPSCs (Zhou et al., 2023). These authors found after injection of methacryloyl hydrogel containing ZIF-8-introduced DPSCs into the lesion, axon number and axon length of DPSCs-differentiated neuron-like cells were significantly increased. ZIF-8 can promote neural differentiation and angiogenesis of DPSCs by activating the mitogen-activated protein kinase signaling pathway. In addition, DPSCs can induce the formation of extensive axonal regeneration and establish close contacts with cocultured neurons (Pagella et al., 2020), which indicates functional synapses may be formed. All mentioned above indicate DPSCs can display a capacity for neuronal differentiation both in vivo and in vitro.
4.2 Paracrine effects
The paracrine effects of DPSCs have been highlighted in regenerative medicine. It has been reported that DPSCs can secrete many bioactive molecules such as interleukin (IL)-10, IL-13, follistatin, transforming growth factor-β1, hepatocyte growth factor, neural cell adhesion molecule-1, and adiponectin (Ogata et al., 2021; Ogata et al., 2022). The bioactive molecules secreted by DPSCs can directly affect their own intracellular signal transduction, and can also indirectly cause neighboring cells to secrete functional active substances (Mattei et al., 2021). Moreover, several neuroprotective factors can be detected in DPSC-conditioned medium (DPSC-CM), including nerve growth factor (NGF), brain-derived neurotrophic factor (BDNF), glial cell line derived neurotrophic factor (GDNF), neurotrophin 3 (NT-3), vascular endothelial growth factor (VEGF) and platelet-derived growth factor (PDGF) (Matsumura-Kawashima et al., 2021; Bousnaki et al., 2022; Li et al., 2023). Asadi-Golshan et al. found intraspinal administration of DPSC-CMs loaded in collagen hydrogel can dramatically improve functional recovery in rat SCI model (Asadi-Golshan et al., 2018). And the total loss number of neurons and oligodendrocytes, and the spinal cord lesion volume were significantly decreased after DPSC-CM therapy, which indicated DPSC-CM containing growth factors are able to promote axon regeneration and neuron survival in the early stages of SCI. Moreover, de Almeida et al. found DPSCs transplanted in a rat model of SCI could release trophic factors into the damaged spinal cord tissue, inducing the presence of axons, expressing some glial markers and improving locomotor functions (Asadi-Golshan et al., 2021). Furthermore, DPSCs can secrete several immunomodulatory and anti-inflammatory cytokines such as Interleukin-8 (IL-8), Interleukin-6 (IL-6), Transforming Growth Factor Beta (TGF-β), Hepatocyte Growth Factor (HGF) and Indoleamine 2,3-dioxygenase (IDO) (Mattei et al., 2021). Matsubara et al. show that DPSC-CM containing EDSiglec-9 and monocyte chemoattractant protein-1can induce significant functional recovery in a rodent SCI model by promoting polarization of M2 macrophages (Kano et al., 2017). In addition, intraperitoneally injected-DPSC-CM effectively decreased the microglial pyroptosis by inhibiting the NLRP3/caspase-1/interleukin-1β pathway, thereby promoting the functional recovery after SCI (Liu et al., 2024). Furthermore, DPSCs can secrete several immunomodulatory and anti-inflammatory cytokines such as Interleukin-8, Interleukin-6, Transforming Growth Factor Beta, Hepatocyte Growth Factor and Indoleamine 2,3-dioxygenase (Yamamoto et al., 2014). Matsubara et al. show that DPSC-CM containing EDSiglec-9 and monocyte chemoattractant protein-1 can induce significant functional recovery in a rodent SCI model by promoting polarization of M2 macrophages (Asadi-Golshan et al., 2021). In addition, intraperitoneally injected- DPSC-CM effectively decreased the microglial pyroptosis by inhibiting the NLRP3/caspase-1/interleukin-1β pathway, thereby promoting the functional recovery after SCI (Kano et al., 2017). Furthermore, several bioactive factors secreted from DPSCs can also promote the regeneration of transected axons, through directly inhibiting multiple axon growth inhibitors, including chondroitin sulfate proteoglycan and myelin-associated glycoprotein (Sakai et al., 2012). Therefore, the paracrine effects of DPSCs, mainly consisting of trophic support and immunomodulation, can be used specifically in the repair of damaged spinal cord tissue. Therefore, the paracrine effects of DPSCs, mainly consisting of trophic support and immunomodulation, can be used specifically in the repair of damaged spinal cord tissue.
4.3 DPSCs-exosomes in SCI
MSCs can secret multiple molecules as soluble factors and extracellular vesicles, which are involved in the transference of various proteins and genetic materials (Hade et al., 2021). Exosomes (EXs), one small particule of extracellular vesicles (usually 40–120 nm in diameter), containing many bioactive macromolecules, such as proteins, nucleic acids and lipids involved in biological regulation of cells (Liu et al., 2021). Several previous studies demonstrated MSC-derived EXs have the ability to enhance functional recovery in animal model of SCI by reducing cell apoptosis and inflammation response, and promoting angiogenesis (Liu et al., 2021; Mohebichamkhorami et al., 2022). Depending on the cell sources, MSC-derived EXs have specific set of proteins and nucleic acids that can promote tissue regeneration. Currently, Oral-derived EXs have gained more attention due to their potentials in therapy for SCI, which have more biological regulatory property in anti-inflammation, immunomodulatory and neuroprotection (Lambrichts et al., 2017; Li et al., 2021; Li et al., 2022; Liu et al., 2022). Periodontal ligament-derived EXs in SCI models lead to decrease in pro-inflammatory CD4-positive T cells and inflammation cytokine expression such as IL-6, IL-17, IL-1β, IFN-γ, and TNF-α (Li et al., 2021). Jonavice et al. showed that DPSC-EVs can alter the phenotypes of microglia by suppressing NF-κB signalling pathway. The shifting microglia M1/M2 polarization can eventually contribute to improve microenvironment and functional recovery after traumatic brain injury (Li et al., 2022). Recently, Liu et al. demonstrate that administration of DPSCs-EXs can decrease the inflammatory response and minimize neurological impairment by reducing macrophage M1 polarization through suppressing ROS-MAPK-NFkB P65 signaling pathway (Liu et al., 2022). In addition, DPSC-EXs play important roles in neuronal axon regeneration, which indicated their potential therapies for neurite growth, and axon remodeling of spinal cord tissue (Lambrichts et al., 2017). Therefore, DPSC-EXs as biological extracellular vesicles can promote functional recovery in SCI through multiple neuroprotecitve mechanisms.
5 Conclusion
Previous published literature demonstrate DPSCs represent a promising new cell source for cell-based treatment of SCI, with the evidence of three mainly effective patterns, including neuronal differentiation, paracrine effects and exosome secretion (Table 1). Transplant of DPSCs or DPSCs-secretome can provide several neuroprotective benefits for the treatment of SCI, such as suppressing inflammatory response, inhibiting SCI-induced cellular apoptosis, modulating promoting axonal regeneration, and cell replacement especially in providing functional neuronal-like cell (Figure 2). These regenerative mechanisms have shown great potential in reconstructing the injured spinal cord and promoting functional recovery in experimental model of SCI. However, many problems remain to be resolved before the clinical usage of this therapy. Firstly, it is preliminary important to achieve large-scale DPSCs manufacture, storage, and transportation with minimum possibility of contamination. In addition, concerns remain raised about the biological safety of DPSCs therapy such as immunotoxicity, immunogenicity, and tumorigenicity. Moreover, the quality of DPSCs is critical to clinical usage, such as the standard source of dental pulp and culture conditions. Therefore, future researches should be further investigated to guide the clinical application of DPSCs.
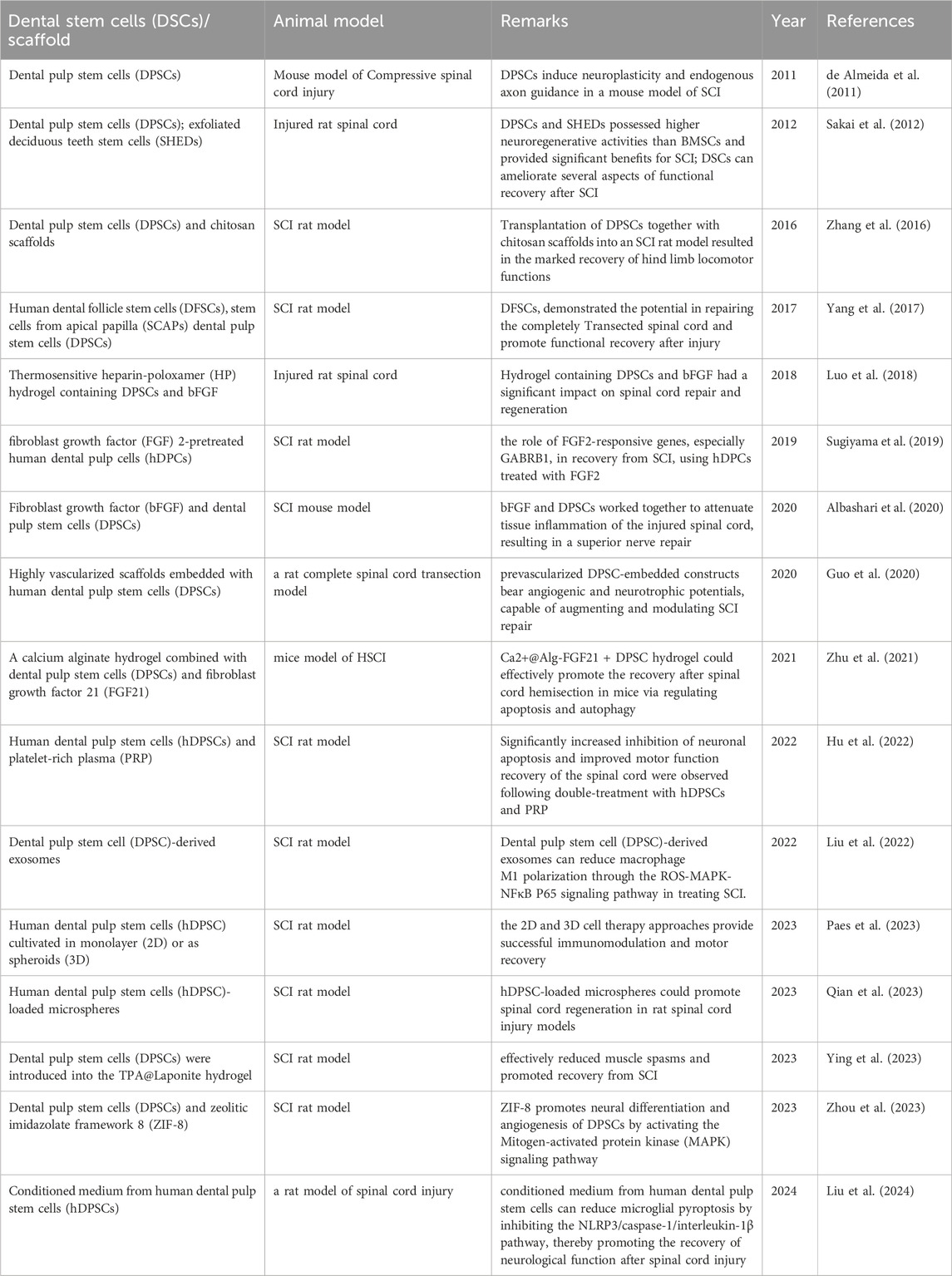
Table 1. Studies that assessed the use of dental pulp stem cells for spinal cord repair and regeneration.
Author contributions
JF: Funding acquisition, Formal Analysis, Conceptualization, Writing–original draft. WL: Writing–original draft, Software, Conceptualization, TM: Writing–original draft, Investigation, Data curation. ZC: Writing–original draft, Supervision, Methodology. LL: Writing–original draft, Project administration, Formal Analysis. JL: Writing–review and editing, Validation, Project administration. ZN: Writing–review and editing, Visualization, Validation, Resources. YS: Writing–review and editing, Visualization, Methodology. YC: Writing–original draft, Supervision, Project administration, Formal Analysis. ZG: Writing–review and editing, Visualization, Resources, Investigation. XL: Writing–review and editing.
Funding
The author(s) declare that financial support was received for the research, authorship, and/or publication of this article. This study was funded by the Medical and Health Science and Technology Project of Zhejiang Province (No. 2024KY211); Medical Health Science and Technology Project of Zhejiang Provincial Health Commission (No. 2024ZL550). The funding bodies had no role in the design of the study; in the collection, analysis, and interpretation of data; in drafting the manuscript.
Conflict of interest
The authors declare that the research was conducted in the absence of any commercial or financial relationships that could be construed as a potential conflict of interest.
Publisher’s note
All claims expressed in this article are solely those of the authors and do not necessarily represent those of their affiliated organizations, or those of the publisher, the editors and the reviewers. Any product that may be evaluated in this article, or claim that may be made by its manufacturer, is not guaranteed or endorsed by the publisher.
References
Ahuja, C. S., and Fehlings, M. (2016). Concise review: bridging the gap: novel neuroregenerative and neuroprotective strategies in spinal cord injury. Stem Cells Transl. Med. 5 (7), 914–924. doi:10.5966/sctm.2015-0381
Albashari, A., He, Y., Zhang, Y., Ali, J., Lin, F., Zheng, Z., et al. (2020). Thermosensitive bFGF-modified hydrogel with dental pulp stem cells on neuroinflammation of spinal cord injury. ACS Omega 5 (26), 16064–16075. doi:10.1021/acsomega.0c01379
Andrzejewska, A., Dabrowska, S., Lukomska, B., and Janowski, M. (2021). Mesenchymal stem cells for neurological disorders. Adv. Sci. (Weinh). 8 (7), 2002944. doi:10.1002/advs.202002944
Asadi-Golshan, R., Razban, V., Mirzaei, E., Rahmanian, A., Khajeh, S., Mostafavi-Pour, Z., et al. (2018). Sensory and motor behavior evidences supporting the usefulness of conditioned medium from dental pulp-derived stem cells in spinal cord injury in rats. Asian Spine J. 12 (5), 785–793. doi:10.31616/asj.2018.12.5.785
Asadi-Golshan, R., Razban, V., Mirzaei, E., Rahmanian, A., Khajeh, S., Mostafavi-Pour, Z., et al. (2021). Efficacy of dental pulp-derived stem cells conditioned medium loaded in collagen hydrogel in spinal cord injury in rats: stereological evidence. J. Chem. Neuroanat. 116, 101978. doi:10.1016/j.jchemneu.2021.101978
Bar, J. K., Lis-Nawara, A., and Grelewski, P. G. (2021). Dental pulp stem cell-derived secretome and its regenerative potential. Int. J. Mol. Sci. 22 (21), 12018. doi:10.3390/ijms222112018
Bianco, J., Berdt, P. D., Deumens, R., and Rieux, A. (2016). Taking a bite out of spinal cord injury: do dental stem cells have the teeth for it? Cell Mol. Life Sci. 73 (7), 1413–1437. doi:10.1007/s00018-015-2126-5
Bonaventura, G., Incontro, S., Iemmolo, R., Cognata, V. L., Barbagallo, I., Costanzo, E., et al. (2020). Dental mesenchymal stem cells and neuro-regeneration: a focus on spinal cord injury. Cell Tissue Res. 379 (3), 421–428. doi:10.1007/s00441-019-03109-4
Bousnaki, M., Bakopoulou, A., Pich, A., Papachristou, E., Kritis, A., and Koidis, P. (2022). Mapping the secretome of dental pulp stem cells under variable microenvironmental conditions. Stem Cell Rev. Rep. 18 (4), 1372–1407. doi:10.1007/s12015-021-10255-2
Darvishi, M., Hamidabadi, H. G., Bojnordi, M. N., Saeednia, S., Zahiri, M., Niapour, A., et al. (2021). Differentiation of human dental pulp stem cells into functional motor neuron: in vitro and ex vivo study. Tissue Cell 72, 101542. doi:10.1016/j.tice.2021.101542
de Almeida, F. M., Marques, S. A., Ramalho, B. d. S., Rodrigues, R. F., Cadilhe, D. V., Furtado, D., et al. (2011). Human dental pulp cells: a new source of cell therapy in a mouse model of compressive spinal cord injury. J. Neurotrauma. 28 (9), 1939–1949. doi:10.1089/neu.2010.1317
Dumont, R. J., Do, O., Verma, S., Hurlbert, R. J., Boulos, P. T., Ellegala, D. B., et al. (2001). Acute spinal cord injury, part I: pathophysiologic mechanisms. Clin. Neuropharmacol. 24 (5), 254–264. doi:10.1097/00002826-200109000-00002
Eli, I., Lerner, D. P., and Ghogawala, Z. (2021). Acute traumatic spinal cord injury. Neurol. Clin. 39 (2), 471–488. doi:10.1016/j.ncl.2021.02.004
Fan, B., Wei, Z., Yao, X., Shi, G., Cheng, X., Zhou, X., et al. (2018). Microenvironment imbalance of spinal cord injury. Cell Transpl. 27 (6), 853–866. doi:10.1177/0963689718755778
Fu, J., Li, X., Jin, F., Dong, Y., Zhou, H., Alhaskawi, A., et al. (2023). The potential roles of dental pulp stem cells in peripheral nerve regeneration. Front. Neurol. 13, 1098857. doi:10.3389/fneur.2022.1098857
Gronthos, S., Mankani, M., Brahim, J., Robey, P. G., and Shi, S. (2000). Postnatal human dental pulp stem cells (DPSCs) in vitro and in vivo. Proc. Natl. Acad. Sci. U. S. A. 97 (25), 13625–13630. doi:10.1073/pnas.240309797
Guo, S., Redenski, I., Landau, S., Szklanny, A., Merdler, U., and Levenberg, S. (2020). Prevascularized scaffolds bearing human dental pulp stem cells for treating complete spinal cord injury. Adv. Healthc. Mater 9 (20), e2000974. doi:10.1002/adhm.202000974
Hade, M. D., Suire, C. N., and Suo, Z. (2021). Mesenchymal stem cell-derived exosomes: applications in regenerative medicine. Cells 10 (8), 1959. doi:10.3390/cells10081959
Hu, Z. B., Chen, H. C., Wei, B., Zhang, Z. M., Wu, S. K., Sun, J. C., et al. (2022). Platelet rich plasma enhanced neuro-regeneration of human dental pulp stem cells in vitro and in rat spinal cord. Ann. Transl. Med. 10 (10), 584. doi:10.21037/atm-22-1745
Kabatas, S., Demir, C. S., Civelek, E., Yilmaz, I., Kircelli, A., Yilmaz, C., et al. (2018). Neuronal regeneration in injured rat spinal cord after human dental pulp derived neural crest stem cell transplantation. Bratisl. Lek. Listy 119 (3), 143–151. doi:10.4149/BLL_2018_028
Kano, F., Matsubara, K., Ueda, M., Hibi, H., and Yamamoto, A. (2017). Secreted ectodomain of sialic acid-binding ig-like lectin-9 and monocyte chemoattractant protein-1 synergistically regenerate transected rat peripheral nerves by altering macrophage polarity. Stem Cells 35 (3), 641–653. doi:10.1002/stem.2534
Kawashima, N. (2012). Characterisation of dental pulp stem cells: a new horizon for tissue regeneration? Arch. Oral Biol. 57 (11), 1439–1458. doi:10.1016/j.archoralbio.2012.08.010
Kerkis, I., Kerkis, A., Dozortsev, D., Stukart-Parsons, G. C., Massironi, S. M. G., Pereira, L. V., et al. (2006). Isolation and characterization of a population of immature dental pulp stem cells expressing OCT-4 and other embryonic stem cell markers. Cells Tissues Organs 184 (3-4), 105–116. doi:10.1159/000099617
Kogo, Y., Seto, C., Totani, Y., Mochizuki, M., Nakahara, T., Oka, K., et al. (2020). Rapid differentiation of human dental pulp stem cells to neuron-like cells by high K+ stimulation. Biophys. Physicobiol 17, 132–139. doi:10.2142/biophysico.BSJ-2020023
Kwon, B. K., Tetzlaff, W., Grauer, J. N., Beiner, J., and Vaccaro, A. R. (2004). Pathophysiology and pharmacologic treatment of acute spinal cord injury. Spine J. 4 (4), 451–464. doi:10.1016/j.spinee.2003.07.007
Lambrichts, I., Driesen, R. B., Dillen, Y., Gervois, P., Ratajczak, J., Vangansewinkel, T., et al. (2017). Dental pulp stem cells: their potential in reinnervation and angiogenesis by using scaffolds. J. Endod. 43 (9S), S12–S16. doi:10.1016/j.joen.2017.06.001
Lei, T., Wang, J., Liu, Y., Chen, P., Zhang, Z., Zhang, X., et al. (2021). Calreticulin as a special marker to distinguish dental pulp stem cells from gingival mesenchymal stem cells. Int. J. Biol. Macromol. 178, 229–239. doi:10.1016/j.ijbiomac.2021.02.126
Li, D., Zou, X. Y., El-Ayachi, I., Romero, L. O., Yu, Z., Iglesias-Linares, A., et al. (2019). Human dental pulp stem cells and gingival mesenchymal stem cells display action potential capacity in vitro after neuronogenic differentiation. Stem Cell Rev. Rep. 15 (1), 67–81. doi:10.1007/s12015-018-9854-5
Li, F., Wang, X., Shi, J., Wu, S., Xing, W., and He, Y. (2023). Anti-inflammatory effect of dental pulp stem cells. Front. Immunol. 14, 1284868. doi:10.3389/fimmu.2023.1284868
Li, S., Luo, L., He, Y., Li, R., Xiang, Y., Xing, Z., et al. (2021). Dental pulp stem cell-derived exosomes alleviate cerebral ischaemia-reperfusion injury through suppressing inflammatory response. Cell Prolif. 54 (8), e13093. doi:10.1111/cpr.13093
Li, Y., Sun, M., Wang, X., Cao, X., Li, N., Pei, D., et al. (2022). Dental stem cell-derived extracellular vesicles transfer miR-330-5p to treat traumatic brain injury by regulating microglia polarization. Int. J. Oral Sci. 14 (1), 44. doi:10.1038/s41368-022-00191-3
Liu, C., Hu, F., Jiao, G., Guo, Y., Zhou, P., Zhang, Y., et al. (2022). Dental pulp stem cell-derived exosomes suppress M1 macrophage polarization through the ROS-MAPK-NFκB P65 signaling pathway after spinal cord injury. J. Nanobiotechnology 20 (1), 65. doi:10.1186/s12951-022-01273-4
Liu, T., Ma, Z., Liu, L., Pei, Y., Wu, Q., Xu, S., et al. (2024). Conditioned medium from human dental pulp stem cells treats spinal cord injury by inhibiting microglial pyroptosis. Neural Regen. Res. 19 (5), 1105–1111. doi:10.4103/1673-5374.385309
Liu, W. Z., Ma, Z. J., Li, J., and Kang, X. W. (2021). Mesenchymal stem cell-derived exosomes: therapeutic opportunities and challenges for spinal cord injury. Stem Cell Res. Ther. 12 (1), 102. doi:10.1186/s13287-021-02153-8
Luo, L., Albashari, A. A., Wang, X., Jin, L., Zhang, Y., Zheng, L., et al. (2018). Effects of transplanted heparin-poloxamer hydrogel combining dental pulp stem cells and bFGF on spinal cord injury repair. Stem Cells Int. doi:10.1155/2018/2398521
Luzuriaga, J., Irurzun, J., Irastorza, I., Unda, F., Ibarretxe, G., and Pineda, J. R. (2020). Vasculogenesis from human dental pulp stem cells grown in matrigel with fully defined serum-free culture media. Biomedicines 8 (11), 483. doi:10.3390/biomedicines8110483
Luzuriaga, J., Pastor-Alonso, O., Encinas, J. M., Unda, F., Ibarretxe, G., and Pineda, J. R. (2019b). Human dental pulp stem cells grown in neurogenic media differentiate into endothelial cells and promote neovasculogenesis in the mouse brain. Front. Physiol. 10, 347. doi:10.3389/fphys.2019.00347
Luzuriaga, J., Pineda, J. R., Irastorza, I., Uribe-Etxebarria, V., García-Gallastegui, P., Encinas, J. M., et al. (2019a). BDNF and NT3 reprogram human ectomesenchymal dental pulp stem cells to neurogenic and gliogenic neural crest progenitors cultured in serum-free medium. Cell Physiol. Biochem. 52 (6), 1361–1380. doi:10.33594/000000096
Luzuriaga, J., Polo, Y., Pastor-Alonso, O., Pardo-Rodríguez, B., Larrañaga, A., Unda, F., et al. (2021). Advances and perspectives in dental pulp stem cell based neuroregeneration therapies. Int. J. Mol. Sci. 22 (7), 3546. doi:10.3390/ijms22073546
Martens, W., Wolfs, E., Struys, T., Politis, C., Bronckaers, A., and Lambrichts, I. (2012). Expression pattern of basal markers in human dental pulp stem cells and tissue. Cells Tissues Organs 196 (6), 490–500. doi:10.1159/000338654
Matsumura-Kawashima, M., Ogata, K., Moriyama, M., Murakami, Y., Kawado, T., and Nakamura, S. (2021). Secreted factors from dental pulp stem cells improve Sjögren’s syndrome via regulatory T cell-mediated immunosuppression. Stem Cell Res. Ther. 12 (1), 182. doi:10.1186/s13287-021-02236-6
Mattei, V., Martellucci, S., Pulcini, F., Santilli, F., Sorice, M., and Monache, S. D. (2021). Regenerative potential of DPSCs and revascularization: direct, paracrine or autocrine effect? Stem Cell Rev. Rep. 17 (5), 1635–1646. doi:10.1007/s12015-021-10162-6
Mattei, V., and Monache, S. D. (2023). Dental pulp stem cells (DPSCs) and tissue regeneration: mechanisms mediated by direct, paracrine, or autocrine effects. Biomedicines 11 (2), 386. doi:10.3390/biomedicines11020386
McDonald, J. W., and Sadowsky, C. (2002). Spinal-cord injury. Lancet 359 (9304), 417–425. doi:10.1016/S0140-6736(02)07603-1
Mohebichamkhorami, F., Fattahi, R., Niknam, Z., Aliashrafi, M., Naeimi, S. K., Gilanchi, S., et al. (2022). Periodontal ligament stem cells as a promising therapeutic target for neural damage. Stem Cell Res. Ther. 13 (1), 273. doi:10.1186/s13287-022-02942-9
Niehage, C., Karbanová, J., Steenblock, C., Corbeil, D., and Hoflack, B. (2016). Cell surface proteome of dental pulp stem cells identified by label-free mass spectrometry. PLoS One 11 (8), e0159824. doi:10.1371/journal.pone.0159824
Niyazi, M., Zibaii, M. I., Chavoshinezhad, S., Hamidabadi, H. G., Dargahi, L., Bojnordi, M. N., et al. (2020). Neurogenic differentiation of human dental pulp stem cells by optogenetics stimulation. J. Chem. Neuroanat. 109, 101821. doi:10.1016/j.jchemneu.2020.101821
Ogata, K., Matsumura-Kawashima, M., Moriyama, M., Kawado, T., and Nakamura, S. (2021). Dental pulp-derived stem cell-conditioned media attenuates secondary Sjögren’s syndrome via suppression of inflammatory cytokines in the submandibular glands. Regen. Ther. 16, 73–80. doi:10.1016/j.reth.2021.01.006
Ogata, K., Moriyama, M., Matsumura-Kawashima, M., Kawado, T., Yano, A., and Nakamura, S. (2022). The therapeutic potential of secreted factors from dental pulp stem cells for various diseases. Biomedicines 10 (5), 1049. doi:10.3390/biomedicines10051049
Ohtake, Y., and Li, S. (2015). Molecular mechanisms of scar-sourced axon growth inhibitors. Brain Res. 1619, 22–35. doi:10.1016/j.brainres.2014.08.064
Paes, S. M., Castro, M. V., Barbosa, R. M., Politti Cartarozzi, L., Coser, L. O., Kempe, P. R. G., et al. (2023). Human dental pulp stem cell monolayer and spheroid therapy after spinal motor root avulsion in adult rats. Brain Res. 1802, 148229. doi:10.1016/j.brainres.2022.148229
Pagella, P., Miran, S., Neto, E., Martin, I., Lamghari, M., and Mitsiadis, T. A. (2020). Human dental pulp stem cells exhibit enhanced properties in comparison to human bone marrow stem cells on neurites outgrowth. FASEB J. 34 (4), 5499–5511. doi:10.1096/fj.201902482R
Qian, Y., Gong, J., Lu, K., Hong, Y., Zhu, Z., Zhang, J., et al. (2023). DLP printed hDPSC-loaded GelMA microsphere regenerates dental pulp and repairs spinal cord. Biomaterials 299, 122137. doi:10.1016/j.biomaterials.2023.122137
Rowland, J. W., Hawryluk, G. W. J., Kwon, B., and Fehlings, M. G. (2008). Current status of acute spinal cord injury pathophysiology and emerging therapies: promise on the horizon. Neurosurg. Focus 25 (5), E2. doi:10.3171/FOC.2008.25.11.E2
Sakai, K., Yamamoto, A., Matsubara, K., Nakamura, S., Naruse, M., Yamagata, M., et al. (2012). Human dental pulp-derived stem cells promote locomotor recovery after complete transection of the rat spinal cord by multiple neuro-regenerative mechanisms. J. Clin. Invest. 122 (1), 80–90. doi:10.1172/JCI59251
Schwab, J. M., Brechtel, K., Mueller, C. A., Failli, V., Kaps, H. P., Tuli, S. K., et al. (2006). Experimental strategies to promote spinal cord regeneration--an integrative perspective. Prog. Neurobiol. 78 (2), 91–116. doi:10.1016/j.pneurobio.2005.12.004
Siebert, J. R., Steencken, A. C., and Osterhout, D. J. (2014). Chondroitin sulfate proteoglycans in the nervous system: inhibitors to repair. Biomed. Res. Int. 2014, 845323. doi:10.1155/2014/845323
Srikawnawan, W., Songsaad, A., Gonmanee, T., Thonabulsombat, C., Phruksaniyom, C., White, K. L., et al. (2022). Rho kinase inhibitor induced human dental pulp stem cells to differentiate into neurons. Life Sci. 300, 120566. doi:10.1016/j.lfs.2022.120566
Sugiyama, K., Nagashima, K., Miwa, T., Shimizu, Y., Kawaguchi, T., Iida, K., et al. (2019). FGF2-responsive genes in human dental pulp cells assessed using a rat spinal cord injury model. J. Bone Miner Metab. 37 (3), 467–474. doi:10.1007/s00774-018-0954-8
Venkatesh, K., Ghosh, S. K., Mullick, M., Manivasagam, G., and Sen, D. (2019). Spinal cord injury: pathophysiology, treatment strategies, associated challenges, and future implications. Cell Tissue Res. 377 (2), 125–151. doi:10.1007/s00441-019-03039-1
Xu, Y., Chen, M., Zhang, T., Ma, Y., Chen, X., Zhou, P., et al. (2019). Spinal cord regeneration using dental stem cell-based therapies. Acta Neurobiol. Exp. (Wars) 79 (4), 319–327.
Yamamoto, A., Sakai, K., Matsubara, K., Kano, F., and Ueda, M. (2014). Multifaceted neuro-regenerative activities of human dental pulp stem cells for functional recovery after spinal cord injury. Neurosci. Res. 78, 16–20. doi:10.1016/j.neures.2013.10.010
Yang, C., Li, X., Sun, L., Guo, W., and Tian, W. (2017). Potential of human dental stem cells in repairing the complete transection of rat spinal cord. J. Neural Eng. 14 (2), 026005. doi:10.1088/1741-2552/aa596b
Ying, Y., Huang, Z., Tu, Y., Wu, Q., Li, Z., Zhang, Y., et al. (2022). A shear-thinning, ROS-scavenging hydrogel combined with dental pulp stem cells promotes spinal cord repair by inhibiting ferroptosis. Bioact. Mater. 22, 274–290. doi:10.1016/j.bioactmat.2022.09.019
Zhang, J., Lu, X., Feng, G., Gu, Z., Sun, Y., Bao, G., et al. (2016). Chitosan scaffolds induce human dental pulp stem cells to neural differentiation: potential roles for spinal cord injury therapy. Cell Tissue Res. 366 (1), 129–142. doi:10.1007/s00441-016-2402-1
Zhou, H., Jing, S., Xiong, W., Zhu, Y., Duan, X., Li, R., et al. (2023). Metal-organic framework materials promote neural differentiation of dental pulp stem cells in spinal cord injury. J. Nanobiotechnology 21 (1), 316. doi:10.1186/s12951-023-02001-2
Zhu, S. P., Ying, Y. B., Wu, Q. J., Ni, Z. C., Huang, Z. Y., and Cai, P. H. (2021). Alginate self-adhesive hydrogel combined with dental pulp stem cells and FGF21 repairs hemisection spinal cord injury via apoptosis and autophagy mechanisms. Chemical Engineering Journal 426, 130827.
Keywords: cell therapy, human dental stem cells, mesenchymal stem cells, spinal cord injury, the potential therapeutic roles
Citation: Fu J, Li W, Mao T, Chen Z, Lai L, Lin J, Nie Z, Sun Y, Chen Y, Zhang Q and Li X (2024) The potential therapeutic roles of dental pulp stem cells in spinal cord injury. Front. Mol. Biosci. 11:1363838. doi: 10.3389/fmolb.2024.1363838
Received: 31 December 2023; Accepted: 04 March 2024;
Published: 29 April 2024.
Edited by:
Bo Li, Sichuan University, ChinaReviewed by:
Sheng Yi, Nantong University, ChinaYuanyuan Liu, National Institute of Dental and Craniofacial Research (NIH), United States
Copyright © 2024 Fu, Li, Mao, Chen, Lai, Lin, Nie, Sun, Chen, Zhang and Li. This is an open-access article distributed under the terms of the Creative Commons Attribution License (CC BY). The use, distribution or reproduction in other forums is permitted, provided the original author(s) and the copyright owner(s) are credited and that the original publication in this journal is cited, in accordance with accepted academic practice. No use, distribution or reproduction is permitted which does not comply with these terms.
*Correspondence: Xigong Li, lixigong@zju.edu.cn