Current perspective on retinal remodeling: Implications for therapeutics
- Moran Eye Center, Department of Ophthalmology, University of Utah, Salt Lake City, UT, United States
The retinal degenerative diseases retinitis pigmentosa and age-related macular degeneration are a leading cause of irreversible vision loss. Both present with progressive photoreceptor degeneration that is further complicated by processes of retinal remodeling. In this perspective, we discuss the current state of the field of retinal remodeling and its implications for vision-restoring therapeutics currently in development. Here, we discuss the challenges and pitfalls retinal remodeling poses for each therapeutic strategy under the premise that understanding the features of retinal remodeling in totality will provide a basic framework with which therapeutics can interface. Additionally, we discuss the potential for approaching therapeutics using a combined strategy of using diffusible molecules in tandem with other vision-restoring therapeutics. We end by discussing the potential of the retina and retinal remodeling as a model system for more broadly understanding the progression of neurodegeneration across the central nervous system.
Introduction
Retinal degenerative diseases (RDDs) impact millions of people. Age-related macular degeneration (AMD) currently impacts 196 million people worldwide and is projected to nearly double by 2050 (Wong et al., 2014). This expected increase in AMD is due to a greater portion of the global population exceeding 65 years old when the probability of AMD becomes much higher (Lim et al., 2012). AMD is by far the most prevalent RDD; however, its root cause, particularly the dry form of AMD, is not entirely clear. We know that genetics combined with environmental factors are strongly associated with the development of AMD, presenting difficulty in its study (Al-Zamil and Yassin, 2017). Although other RDDs like retinitis pigmentosa (RP) are less prevalent, RP affects 1 in 4,000 people and typically onsets early in life (Hartong et al., 2006). RP is associated with many specific genetic defects, enabling the identification and creation of numerous naturally occurring or transgenic animal models (Keeler, 1924; Aguirre, 1978; Narfstrom, 1983; Barnett and Curtis, 1985; Pittler et al., 1993; Suber et al., 1993; Brockerhoff et al., 1995; Petters et al., 1997; Semple-Rowland and Lee, 2000; Chang et al., 2002; Kondo et al., 2009; Han et al., 2013; Chow et al., 2015). These RP models are used in conjunction with donor tissue from patients with RP and AMD to understand the progression and downstream effects of photoreceptor degeneration, revealing fundamental changes to neuronal and glial populations (Strettoi and Pignatelli, 2000; Strettoi et al., 2002, 2003; Jones et al., 2003, 2011, 2016a,b; Marc and Jones, 2003; Pfeiffer et al., 2020b). Due to this pressing public health concern, an increasing number of research groups are searching for therapies to stop or reverse the photoreceptor loss and retinal damage associated with AMD and RP. Additionally, many groups are working to restore light perception following the loss of photoreceptors in more advanced cases of retinal degeneration (Yue et al., 2016; Menon and Vijayavenkataraman, 2022).
Here we provide a perspective of the fundamental progression of retinal remodeling and its impacts on some of the more common approaches being investigated for recovery of vision loss. We will conclude with a discussion of some promising molecular strategies for neuroprotection that, when used in conjunction with vision rescue techniques, may prove to be instrumental in overcoming the obstacles generated by retinal remodeling.
Retinal remodeling
Photoreceptor loss and subsequent retinal remodeling are caused by numerous primary mechanisms, including RDDs (described above), or caused by injuries such as light-induced damage, retinal detachment, or ischemia (Erickson et al., 1983; Lewis et al., 1989, 1991; Jones et al., 2006; Krigel et al., 2016). It has been noted for nearly a century that photoreceptor degeneration leads to several negative plasticity events. In 2003, these observations were consolidated into the term: retinal remodeling and broadly divided into 3 phases and ending with widespread neurodegeneration (Jones et al., 2003; Marc and Jones, 2003).
Phase 1
Phase 1 remodeling is characterized by the initiation of photoreceptor stress and degeneration. The impact of photoreceptor degeneration upon the retina, even in the earliest stages, is not limited to photoreceptors. Glial cells rapidly respond to photoreceptor stress through the activation and interaction of microglia and Müller cells (Jones et al., 2003, 2011, 2014; Pfeiffer et al., 2016; Di Pierdomenico et al., 2020). In phase 1 remodeling, microglia invade the retina, contributing to photoreceptor degeneration (Peng et al., 2014; Di Pierdomenico et al., 2019), while Müller cells begin to hypertrophy and alter their metabolic signature, showing variability in concentrations of taurine and glutamine (Figure 1A). Changes in taurine levels are a proposed mechanism contributing to retinal degeneration (Garcia-Ayuso et al., 2019; Di Pierdomenico et al., 2022; Martinez-Vacas et al., 2022). Simultaneously, there is a pharmacologic and/or protein expression shift in bipolar cells demonstrating increased responses consistent with ionotropic glutamate receptors (Marc et al., 2007; Chua et al., 2009; Jones et al., 2011), generally associated with the OFF bipolar cell class (Figure 1B). Based on more recent work in pathoconnectomics we see substantial inner retinal rewiring (Pfeiffer et al., 2020). Rod bipolar cells (RodBCs), demonstrate synaptic connectivity with cone photoreceptor pedicles and neurite outgrowths off of cone photoreceptors (Figures 2A–D). These novel connections are made while many of the RodBCs still maintain some degree of connectivity with surviving rod photoreceptors, creating complex bipolar cell input paradigms. In the inner plexiform layer, RodBCs make gap junctions with their primary postsynaptic partner, the Aii amacrine cell. This phenomenon is never observed in the normal, healthy retina, potentially explaining the reports of poor adaptation by RP patients early in the course of their disease. Beyond immediate partner rewiring, GABAergic amacrine cells project from the inner nuclear layer up into the outer plexiform layer, making morphologically identifiable synapses with horizontal cells, and bipolar cell dendrites. This topology is also entirely novel and pathological as it introduces network corruption in the visual pathway.
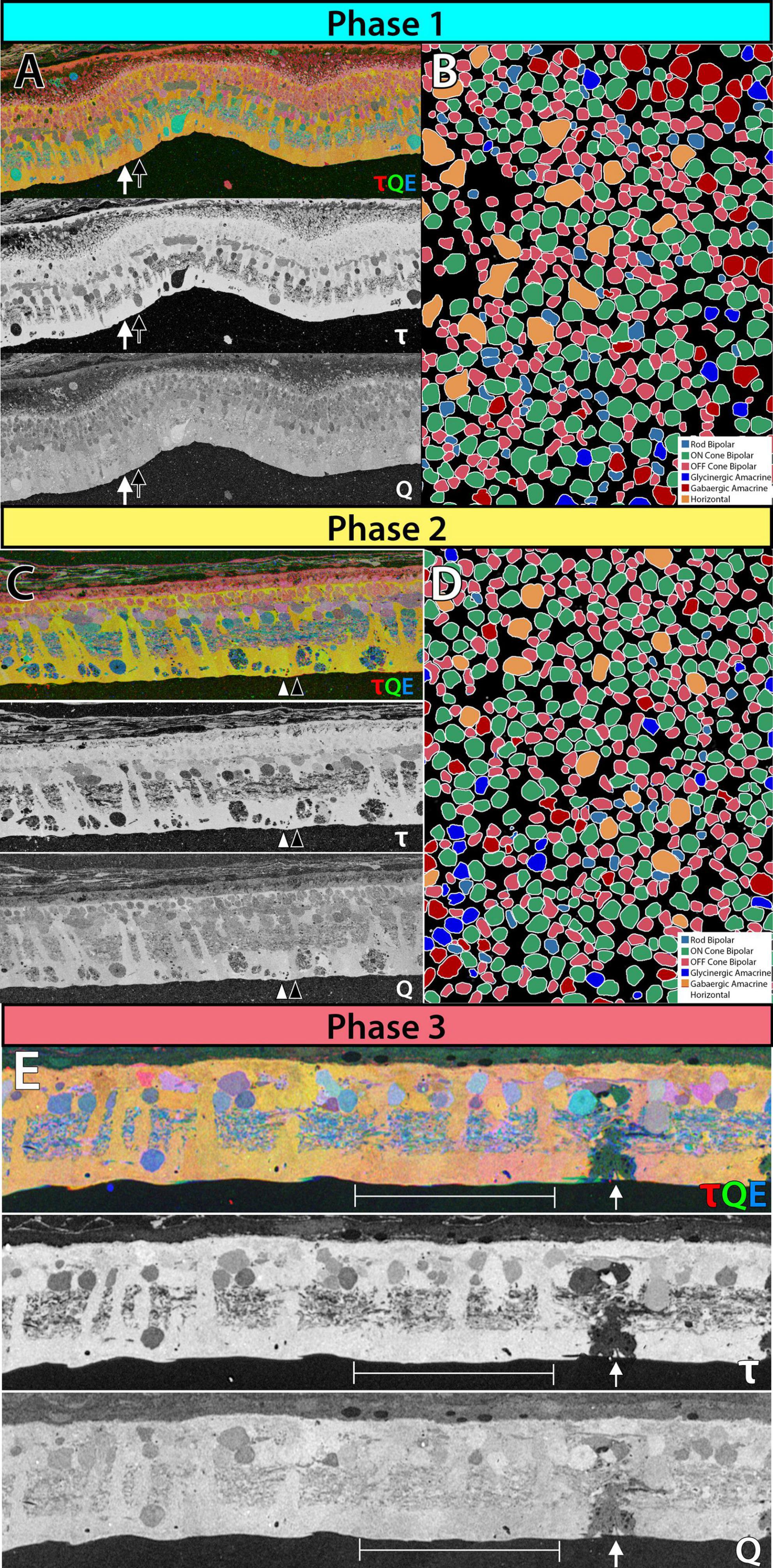
Figure 1. Metabolic and receptor changes phase 1 remodeling in 3mo P347L rabbit: (A) top panel: 3-channel composite of metabolites taurine, glutamine and glutamate. Lower panels: individual metabolites taurine and glutamine. Arrows indicate neighboring Müller cells. (B) Theme map of cell types in a horizontal section of retina identified based on ionotropic glutamate receptor function. Phase 2 remodeling in 2yo P347L rabbit: (C) top panel: 3-channel composite of metabolites taurine, glutamine and glutamate. Lower panels: individual metabolites taurine and glutamine. Arrows indicate neighboring Müller cells. (D) Theme map of cell types in a horizontal section of retina identified based on ionotropic glutamate receptor function. Phase 3 remodeling in 4yo P347L rabbit: (E) top panel: 3-channel composite of metabolites taurine, glutamine, and glutamate. Lower panels: individual metabolites taurine and glutamine. Arrows indicate Müller cell devoid of taurine and containing reduced glutamine. Bracket indicates region of Müller cells with varying levels of small molecules.
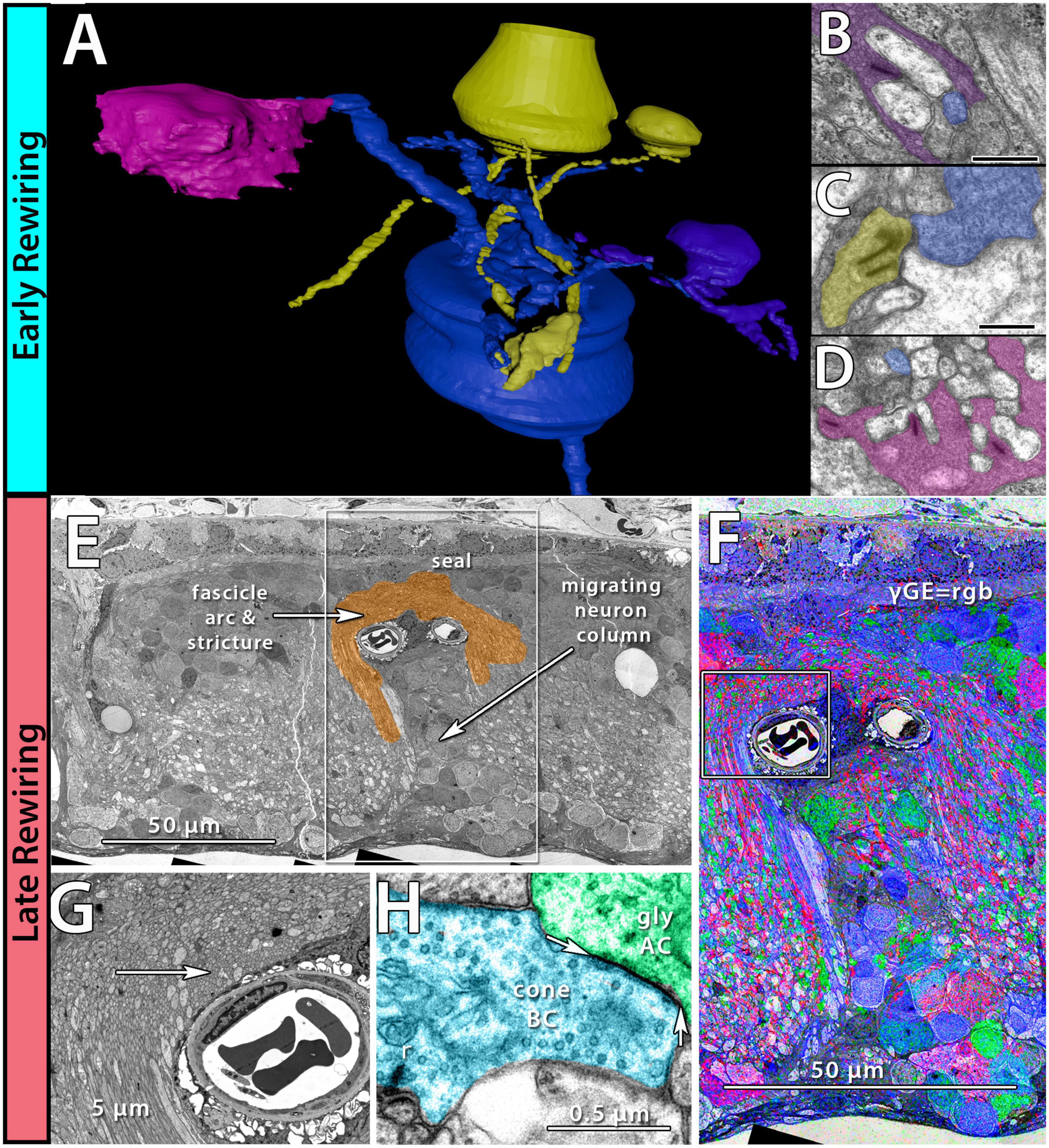
Figure 2. Early and late-stage rewiring. Early rewiring in 10mo P347L rabbit: (A) 3D rendering of rod bipolar cell (RodBC) dendrites from RPC1. RodBC in blue, cone photoreceptor in pink, rod photoreceptor in purple, and indeterminate in yellow. (B) Pseudocolored TEM image of rod photoreceptor ribbon synapse onto RodBC rendered in panel (A). (C) Pseudocolored TEM image of indeterminate photoreceptor ribbon synapse onto RodBC rendered in panel (A). (D) Pseudocolored TEM image of Cone photoreceptor ribbon synapse onto RodBC rendered in panel (A). Scale bars: 500 nm late rewiring in the pnd 900 RCS rat: (E) TEM image of microneuroma extended over a blood vessel invading inner nuclear layer. (F) GABA, Glycine, and Glutamate channels overlayed on top of the region outlined with a box in panel (E). (G) Higher resolution image of region highlighted with box in panel (F). (H) Pseudocolored high-resolution image of area indicated with arrow in panel (G). Arrows indicate edges of gap junction found intact within microneuroma.
In summary, phase 1 remodeling demonstrates glial activation and widespread rewiring or retinal network revision occurs early in degeneration, potentially complicating many therapeutic interventions.
Phase 2
Prolonged photoreceptor degeneration characterizes phase 2 remodeling. Here, glial activation becomes increasingly pronounced through microglial migration (Di Pierdomenico et al., 2017), greater metabolic variability (Figure 1C), morphological entanglement of Müller cell endfeet (Pfeiffer et al., 2019), beginning of the formation of the Müller seal (Jones and Marc, 2005; Pfeiffer et al., 2016; Pfeiffer et al., 2019), and upregulation of proteins such as GFAP (Erickson et al., 1987). Reprogramming continues with the mislocation of mGLuR6 receptors away from ON-BC dendrites (Nomura et al., 1994; Chua et al., 2009), and ionotropic receptor consistent responses in bipolar cell population increase (Figure 1D). Rewiring also becomes more pronounced through the extension of large neurites from horizontal cells into the inner plexiform layer (Strettoi and Pignatelli, 2000; Strettoi et al., 2002, 2003). Remaining photoreceptors may also extend neurites beyond the outer plexiform layer into the inner plexiform layer or into the ganglion cell layer (Li et al., 1995; Fariss et al., 2000; Sethi et al., 2005). Additionally, some bipolar cells retract their dendrites completely (Strettoi and Pignatelli, 2000; Strettoi et al., 2003). The total effects of rewiring on the inner plexiform networks and the implications for current flow through the altered network are currently unknown. However, the investigation into pathoconnectome volumes will provide a framework to begin hanging gene and protein expression data, as well as providing complete networks for modeling. At the end of Phase 2, the last of the rod photoreceptor somas disappear, and most of the cone somas are also gone. Some rare cone photoreceptor somas may persist, underneath which there may be localized areas of some retinal preservation, but remodeling is progressing regardless.
Phase 3
The complete loss of photoreceptor somas characterizes phase 3 remodeling. The retina engages in a prolonged phase of revision, potentially driven by Müller cell gliosis. Phase 3 remodeling ultimately renders the retina unrecognizable as it transitions into the neurodegeneration phase. Glial metabolic variability persists (Figure 1E) while structural alterations seal off the neural retina through a Müller cell seal and increasing entanglement of Müller cell processes (Lewis et al., 1989; Pfeiffer et al., 2020a,b). Unlike the glutamate receptor reprogramming seen in phases 1 and 2, by phase 3, AGB loading shows glutamate receptors to be functionally or pharmacologically absent in remaining bipolar cells (Marc et al., 2007). Gross rewiring is prevalent throughout the retina, with neurite sprouts from all remaining neuronal cell classes coalescing into tangles called microneuromas (Figures 2E–H). Within microneuromas, neurites form synapses with ultrastructure consistent with numerous synaptic types. However, the pairing of these and whether they follow network rules similar to the healthy retina is unknown (Jones et al., 2003; Jones and Marc, 2005). Our pathoconnectomics initiatives are actively exploring this question. Ultimately, loss of inner retinal neurons devolves into a complete neurodegenerative phenotype, though there is not a clear aspect that delineates the neurodegenerative phase from phase 3 remodeling.
Widespread neurodegeneration
If retinal remodeling persists long enough, the retina will eventually lose upward of 90% of its neurons (Pfeiffer et al., 2020b). At this point, even ganglion cells, previously reported to be spared from neurodegeneration in RDDs (Medeiros and Curcio, 2001; Stefanov et al., 2019), also degenerate (Garcia-Ayuso et al., 2015, 2018). This is the most severe outcome, occurring only after prolonged remodeling. However, as people live longer, we may see more instances of widespread retinal degeneration in patients, particularly given the increase in diabetic retinopathy and AMD projected to increase substantially over the next two decades.
Retinal degeneration therapies
Genetic therapies
Genetic intervention is undoubtedly one of the therapeutic interventions most heavily invested in Botto et al. (2022). Intervening genetically prior to the degeneration of neurons would be ideal to avoid many complications of remodeling (Jones et al., 2016a,b; Pfeiffer et al., 2020b), but it faces many logistical challenges. That said, the genetic approach has seen clinical success, particularly in Luxterna, the RPE65 genetic therapy for treating Leber’s Congenital Amaurosis (Acland et al., 2001; Le Meur et al., 2007; Maguire et al., 2021). Numerous other genetic therapies are currently being developed based on this success (Prado et al., 2020). However, initial successes in treatment were followed by the progression of visual deficits afterward, suggesting that the retinal remodeling processes, initiated prior to gene therapy, is an ongoing program not arrested by this particular gene therapy intervention. This would certainly track with our failed optogenetic study (unreported) in rabbits that did not stop the process of retinal remodeling.
In principle, treating neurodegenerative diseases prior to the damage of the initially affected neurons is ideal. In practice, this would be effective on single genetic cause diseases, not complex diseases, including AMD. When not suspected due to familial history, even single genetic cause disorders may have substantial neurodegeneration before diagnosis, and the initiation of retinal remodeling is likely underway. Realistically, photoreceptor degeneration diseases are associated with roughly 300 known primary mutations (Daiger et al., 2014), making it challenging to produce a novel therapeutic for each genetic cause, as each gene defect is effectively an orphan disease. There may also be more gene-gene or gene-environment disorders within the constellation of RDD than we can realistically generate genetic therapies for. Combined, this demonstrates a need for further understanding of remodeling processes.
Optogenetics
The field of optogenetics allows control or activation of cells using light (Nagel et al., 2003; Boyden et al., 2005). Optogenetic therapies have been proposed for classic neurodegenerative diseases such as Alzheimer’s and Parkinson’s disease, and perhaps more intuitively for retinitis pigmentosa to restore light-sensing cells to the retina following photoreceptor degeneration (Marc et al., 2014). Early clinical trials for optogenetics usage in retinitis pigmentosa are coming out with encouraging results (Sengupta et al., 2016; Sahel et al., 2021). However, as highlighted by Harris and Gilbert (2022), even promising studies are not without potential concern. The usage of AAVs to deliver photosensitive proteins may cause future complications in treatment, particularly in the potential for an immune response to AAVs as they become more therapeutically widespread. Additionally, the permanent nature of optogenetic interventions essentially removes the ability to stop or reverse treatment should the treatment prove long-term to have adverse effects. Therefore, although optogenetic treatments demonstrate great promise for the treatment of RDDs, clinical trials should proceed with caution. Within the context of retinal remodeling, optogenetic interventions are complicated by the early rewiring and glutamate receptor reprogramming of bipolar cells, causing conflicting input to ganglion cells. Appropriate targeting to the precise class of cells is also potentially problematic. With >40 classes of ganglion cells, which ganglion cell classes will we target for gene therapy? Also, what are the psychophysics of introducing additional sensory percepts within a normal retinal network? What happens when that network is altered as it is in retinal degeneration and remodeling?
Photoswitches
Another mechanism to induce photo-sensitivity in neurons that are not innately photosensitive is through the use of photoswitches (Kramer et al., 2009; Polosukhina et al., 2012; Marc et al., 2014; Tochitsky et al., 2014). Photoswitches are photosensitive small molecules which reversibly alter their conformation in response to light, conferring a light response to the cells they are interacting with (Szymanski et al., 2013). Photoswitches are easily applied through simple intravitreal injection and are reported to be non-toxic. Many of the early generations of photoswitches interact with ion channels allowing direct control of the channel state by light, blocked or unblocked (Kramer et al., 2009). Newer photoswitches have been engineered to create light-inducible forms of glutamate receptors, ionotropic, or metabotropic, allowing a more direct recapitulation of intact retinal function. One primary concern is that these compounds appear to be most effective in non-light sensing retinas (Tochitsky et al., 2014), although preservation of vision requires intervention earlier in degeneration. Despite the promising results of early studies of these compounds in animals, more research is needed prior to use in the clinic. The use of photoswitches in RDD is also complicated by rewiring and reprogramming, but the temporary nature of this intervention allows for more trial and error in their implementation.
Cell replacement
Over the last decade, our capabilities differentiating stem cells into specific neural lineages have increased dramatically (Yamanaka, 2020). This advancement has led to renewed interest in replacing damaged neurons in neurodegenerative diseases (Gagliardi et al., 2019). Photoreceptor degenerations are especially attractive for this therapy because of the nature of disease progression, namely the initial damage of the photoreceptors, their subsequent loss, and the ease of accessing the retina compared with other CNS regions. Additionally, the success of culturing retinal organoids has led to a diverse array of available retinal neural types, including photoreceptors (Cowan et al., 2020; Li et al., 2021). Cell replacement therapy as a discipline is still in its infancy, leaving many technical challenges and questions involving correct circuit integration within an altered network, long-term survival of the transplanted cells (Wu et al., 2018), and impacts on native cells (Zhou et al., 2021) to be explored. Another related approach is the dedifferentiation of Müller glia into stem cells for an endogenous approach to cell replacement (Lamba et al., 2009; Hoang et al., 2020). However, its implementation is hindered by cell specificity in Müller cell targeting (Le et al., 2022) and the same circuit integration complications as neuronal transplants. That said, Müller cells are ideally positioned within the retina, and would also have intimate contact with other Müller cell populations as they de-differentiate to maintain access to Müller cells’ retinal metabolic support.
Bionics
The use of electrode implants to restore vision has advanced considerably (Weiland et al., 2011; Chuang et al., 2014; Stingl et al., 2017; Farnum and Pelled, 2020). A primary advantage of implants is the ability to select their placement depending on the state of retinal degeneration. If the inner retinal circuitry is still largely intact, a subretinal implant may be most effective at restoring more acute vision by utilizing the inner retinal processing (Zrenner, 2002). Alternatively, should the inner retinal wiring be corrupted through remodeling processes, ganglion cells serve as a potential target through epi-retinal implants. Lastly, implants can also be engineered to bypass the degenerating retina and directly interface with the cortex. Clinical trials of all implants demonstrate early successes in restoring some vision to patients who were previously non-light perceiving (Ahuja et al., 2011; Dorn et al., 2013; Beauchamp et al., 2020); however, movement of the implant, long-term efficacy, and sustained support for permanent implants are ongoing concerns (Kuehlewein et al., 2019). Despite the early successes of bionics, all implants are longitudinally impacted by issues of device movement and gliosis. More broadly, retinal bionic implants have not been shown to slow down or reverse the retinal plasticity associated with retinal degeneration. To address some of these concerns, modeling groups have begun exploring how remodeled retinal networks respond to electrical stimulation in contrast to the responses predicted from a healthy retina (Kosta et al., 2021).
Diffusible molecules and neuroprotection
The extensive inner retinal damage and complications for therapeutic interventions associated with retinal remodeling can seem overwhelming. However, when combined with current neuroprotective strategies, many avenues of treatment may prove effective in the retina. This is not an exhaustive list of neuroprotective strategies, and we refer readers to some excellent reviews (Froger et al., 2014; Kolomeyer and Zarbin, 2014; Vecino et al., 2016; Pardue and Allen, 2018).
One active area of exploration for neuroprotection is in releasable signaling molecules like dopamine. Dopamine released from dopaminergic amacrine cells plays important roles in light adaptation and signal adaptation (Witkovsky, 2004). It is also implicated in the progression of numerous neurodegenerative diseases, including Parkinson’s disease, diabetic retinopathy, and may play a role in AMD (Mor et al., 2019; Figueroa et al., 2021). Therapeutically, dopamine analogs like L-DOPA or dopamine agonists have been used for a long time to treat Parkinson’s disease to counteract the loss of dopaminergic neurons in the brain. In the retina, the loss of dopaminergic neurons coincides with disease progression and experimental treatment paradigms in animal models are promising (Ivanova et al., 2016). In humans, L-DOPA administration in Parkinson’s patients potentially delays the onset of AMD, though this could be acting through a downstream target (Brilliant et al., 2016). These results indicate that dopamine may be critical for the function and survival of many neuronal classes across the CNS and warrants further study within the realm of neuroprotection.
Another potential molecule class to combat neurodegeneration are the steroid hormones progesterone and estrogen. Progesterone and estrogen have been implicated as neuroprotectants in various CNS insults, including traumatic brain injury, and experimentally in light-induced retinal degeneration (Attella et al., 1987; Zhu et al., 2015). However, despite promising animal studies, progesterone treatments have not yet demonstrated efficacy in clinical trials (Sitruk-Ware et al., 2021). The mechanism of action leading to neuroprotection by progesterone and estrogen is not entirely clear. They both act on pathways inducing decreasing microglial activation, decreasing cytokine activation, increasing levels of brain-derived neurotrophic factor (BDNF), acting as an agonist of GABA receptors, and reducing edema (Singh and Su, 2013; Roche et al., 2016; Guennoun, 2020). These roles could be useful for managing numerous retinal and brain neurodegenerative diseases, and warrant further exploration.
Finally, one of the more commonly explored neuroprotective strategies is the direct modulation of neurotrophic factors (Garcia et al., 2003). There are two primary families of neurotropic factors: glial-derived neurotrophic factors (GDNF) and neurotrophins which include BDNF, nerve growth factor (NGF), and neurotrophin 3 and 4 (NT3 and NT4) (Bringmann et al., 2009). Neurotrophic factors have important roles in neuronal development and survival (Hodgetts and Harvey, 2017; Skaper, 2018). Many neurotrophic factors are predominately released by glial cells and work through multiple pathways to modulate synaptic strength, promote neuronal survival, and are involved in neurite outgrowth during development. The potential of neurotropic factors for treating neurodegenerative diseases has not gone unnoticed (Rangasamy et al., 2010; Johnson et al., 2011). However, their hydrophilic properties has complicated their delivery (Thorne and Frey, 2001). Despite this hurdle, experimental usage in vitro and in animal models is encouraging and neurotrophic factors may prove to be an important component of neurodegenerative disease treatment in the brain and retina (Allen et al., 2013).
Discussion
Retinal degenerative diseases such as RP and AMD are debilitating diseases leading to progressive vision loss and eventual neurodegeneration of the inner retina. Although the progressive nature of these diseases and the accompanying retinal remodeling complicate most approaches to therapeutic interventions, there is substantial hope for treatment including through the evaluation of remodeling processes themselves (Peng et al., 2014; Di Pierdomenico et al., 2018, 2019). Additionally, many aspects of retinal remodeling and degeneration recapitulate components observed in other CNS neurodegenerations (Pfeiffer et al., 2020b). These observations open a new avenue of exploration into fundamental mechanisms of neurodegeneration potentially conserved across the nervous system. The retina is ideal for evaluating these mechanisms because of its compact size, immune privilege, entire circuit topologies, and the accessibility for non-invasive monitoring. Combined, it is our hypothesis that key treatments for neurodegenerative diseases will be combinations of engineering approaches like cell replacement combined with molecular interventions of diffusible molecules to delay or prevent neuronal loss and deleterious remodeling.
Data availability statement
The original contributions presented in this study are included in this article/supplementary material, further inquiries can be directed to the corresponding authors.
Ethics statement
The animal study was reviewed and approved by the University of Utah IACUC.
Author contributions
Both authors wrote the perspective and contributed panels to the figures.
Funding
This work was supported by the National Institutes of Health [RO1 EY015128(BWJ), RO1 EY028927(BWJ), and P30 EY014800(Core)]; the National Science Foundation (2014862), and an Unrestricted Research Grant from Research to Prevent Blindness, New York, NY to the Department of Ophthalmology & Visual Sciences, University of Utah.
Conflict of interest
The authors declare that the research was conducted in the absence of any commercial or financial relationships that could be construed as a potential conflict of interest.
Publisher’s note
All claims expressed in this article are solely those of the authors and do not necessarily represent those of their affiliated organizations, or those of the publisher, the editors and the reviewers. Any product that may be evaluated in this article, or claim that may be made by its manufacturer, is not guaranteed or endorsed by the publisher.
References
Acland, G. M., Aguirre, G. D., Ray, J., Zhang, Q., Aleman, T. S., Cideciyan, A. V., et al. (2001). Gene therapy restores vision in a canine model of childhood blindness. Nat. Genet. 28, 92–95. doi: 10.1038/ng0501-92
Aguirre, G. (1978). Retinal degenerations in the dog. I. Rod dysplasia. Exp. Eye Res. 26, 233–253. doi: 10.1016/0014-4835(78)90072-6
Ahuja, A. K., Dorn, J. D., Caspi, A., McMahon, M. J., Dagnelie, G., Dacruz, L., et al. (2011). Blind subjects implanted with the Argus II retinal prosthesis are able to improve performance in a spatial-motor task. Br. J. Ophthalmol. 95, 539–543. doi: 10.1136/bjo.2010.179622
Allen, S. J., Watson, J. J., Shoemark, D. K., Barua, N. U., and Patel, N. K. (2013). GDNF, NGF and BDNF as therapeutic options for neurodegeneration. Pharmacol. Ther. 138, 155–175. doi: 10.1016/j.pharmthera.2013.01.004
Al-Zamil, W. M., and Yassin, S. A. (2017). Recent developments in age-related macular degeneration: A review. Clin. Interv. Aging 12, 1313–1330. doi: 10.2147/CIA.S143508
Attella, M. J., Nattinville, A., and Stein, D. G. (1987). Hormonal state affects recovery from frontal cortex lesions in adult female rats. Behav. Neural Biol. 48, 352–367. doi: 10.1016/S0163-1047(87)90918-6
Barnett, K. C., and Curtis, R. (1985). Autosomal dominant progressive retinal atrophy in Abyssinian cats. J. Hered. 76, 168–170. doi: 10.1093/oxfordjournals.jhered.a110058
Beauchamp, M. S., Oswalt, D., Sun, P., Foster, B. L., Magnotti, J. F., Niketeghad, S., et al. (2020). Dynamic stimulation of visual cortex produces form vision in sighted and blind humans. Cell 181, 774–783.e5. doi: 10.1016/j.cell.2020.04.033
Botto, C., Rucli, M., Tekinsoy, M. D., Pulman, J., Sahel, J. A., and Dalkara, D. (2022). Early and late stage gene therapy interventions for inherited retinal degenerations. Prog. Retin. Eye Res. 86:100975. doi: 10.1016/j.preteyeres.2021.100975
Boyden, E. S., Zhang, F., Bamberg, E., Nagel, G., and Deisseroth, K. (2005). Millisecond-timescale, genetically targeted optical control of neural activity. Nat. Neurosci. 8, 1263–1268. doi: 10.1038/nn1525
Brilliant, M. H., Vaziri, K., Connor, T. B. Jr., Schwartz, S. G., Carroll, J. J., McCarty, C. A., et al. (2016). Mining retrospective data for virtual prospective drug repurposing: L-DOPA and age-related macular degeneration. Am. J. Med. 129, 292–298. doi: 10.1016/j.amjmed.2015.10.015
Bringmann, A., Iandiev, I., Pannicke, T., Wurm, A., Hollborn, M., Wiedemann, P., et al. (2009). Cellular signaling and factors involved in Muller cell gliosis: Neuroprotective and detrimental effects. Prog. Retin. Eye Res. 28, 423–451. doi: 10.1016/j.preteyeres.2009.07.001
Brockerhoff, S. E., Hurley, J. B., Janssen-Bienhold, U., Neuhauss, S. C., Driever, W., and Dowling, J. E. (1995). A behavioral screen for isolating zebrafish mutants with visual system defects. Proc. Natl. Acad. Sci. U.S.A. 92, 10545–10549. doi: 10.1073/pnas.92.23.10545
Chang, B., Hawes, N. L., Hurd, R. E., Davisson, M. T., Nusinowitz, S., and Heckenlively, J. R. (2002). Retinal degeneration mutants in the mouse. Vision Res. 42, 517–525. doi: 10.1016/S0042-6989(01)00146-8
Chow, C. Y., Kelsey, K. J., Wolfner, M. F., and Clark, A. G. (2015). Candidate genetic modifiers of retinitis pigmentosa identified by exploiting natural variation in Drosophila. Hum. Mol. Genet. 25, 651–659. doi: 10.1093/hmg/ddv502
Chua, J., Fletcher, E. L., and Kalloniatis, M. (2009). Functional remodeling of glutamate receptors by inner retinal neurons occurs from an early stage of retinal degeneration. J. Comp. Neurol. 514, 473–491. doi: 10.1002/cne.22029
Chuang, A. T., Margo, C. E., and Greenberg, P. B. (2014). Retinal implants: A systematic review. Br. J. Ophthalmol. 98, 852–856. doi: 10.1136/bjophthalmol-2013-303708
Cowan, C. S., Renner, M., De Gennaro, M., Gross-Scherf, B., Goldblum, D., Hou, Y., et al. (2020). Cell types of the human retina and its organoids at single-cell resolution. Cell 182, 1623–1640.e34. doi: 10.1016/j.cell.2020.08.013
Daiger, S. P., Sullivan, L. S., and Bowne, S. (2014). Genes and mapped loci causing retinal diseases. RetNet. Available online at: https://sph.uth.edu/Retnet/ (accessed March 10, 2022).
Di Pierdomenico, J., Garcia-Ayuso, D., Agudo-Barriuso, M., Vidal-Sanz, M., and Villegas-Perez, M. P. (2019). Role of microglial cells in photoreceptor degeneration. Neural Regen. Res. 14, 1186–1190. doi: 10.4103/1673-5374.251204
Di Pierdomenico, J., Garcia-Ayuso, D., Pinilla, I., Cuenca, N., Vidal-Sanz, M., Agudo-Barriuso, M., et al. (2017). Early events in retinal degeneration caused by rhodopsin mutation or pigment epithelium malfunction: Differences and similarities. Front. Neuroanat. 11:14. doi: 10.3389/fnana.2017.00014
Di Pierdomenico, J., Martinez-Vacas, A., Hernandez-Munoz, D., Gomez-Ramirez, A. M., Valiente-Soriano, F. J., Agudo-Barriuso, M., et al. (2020). Coordinated intervention of microglial and muller cells in light-induced retinal degeneration. Invest. Ophthalmol. Vis. Sci. 61:47. doi: 10.1167/iovs.61.3.47
Di Pierdomenico, J., Martinez-Vacas, A., Picaud, S., Villegas-Perez, M. P., and Garcia-Ayuso, D. (2022). Taurine: An essential amino sulfonic acid for retinal health. Neural Regen. Res. 18, 807–808. doi: 10.4103/1673-5374.353491
Di Pierdomenico, J., Scholz, R., Valiente-Soriano, F. J., Sanchez-Migallon, M. C., Vidal-Sanz, M., Langmann, T., et al. (2018). Neuroprotective effects of FGF2 and minocycline in two animal models of inherited retinal degeneration. Invest. Ophthalmol. Vis. Sci. 59, 4392–4403. doi: 10.1167/iovs.18-24621
Dorn, J. D., Ahuja, A. K., Caspi, A., da Cruz, L., Dagnelie, G., Sahel, J. A., et al. (2013). The detection of motion by blind subjects with the epiretinal 60-electrode (Argus II) retinal prosthesis. JAMA Ophthalmol. 131, 183–189. doi: 10.1001/2013.jamaophthalmol.221
Erickson, P. A., Fisher, S. K., Anderson, D. H., Stern, W. H., and Borgula, G. A. (1983). Retinal detachment in the cat: The outer nuclear and outer plexiform layers. Invest. Ophthalmol. Vis. Sci. 24, 927–942.
Erickson, P. A., Fisher, S. K., Guerin, C. J., Anderson, D. H., and Kaska, D. D. (1987). Glial fibrillary acidic protein increases in Muller cells after retinal detachment. Exp. Eye Res. 44, 37–48. doi: 10.1016/S0014-4835(87)80023-4
Fariss, R. N., Li, Z. Y., and Milam, A. H. (2000). Abnormalities in rod photoreceptors, amacrine cells, and horizontal cells in human retinas with retinitis pigmentosa. Am. J. Ophthalmol. 129, 215–223. doi: 10.1016/S0002-9394(99)00401-8
Farnum, A., and Pelled, G. (2020). New vision for visual prostheses. Front. Neurosci. 14:36. doi: 10.3389/fnins.2020.00036
Figueroa, A. G., Boyd, B. M., Christensen, C. A., Javid, C. G., McKay, B. S., Fagan, T. C., et al. (2021). Levodopa positively affects neovascular age-related macular degeneration. Am. J. Med. 134, 122–128.e3. doi: 10.1016/j.amjmed.2020.05.038
Froger, N., Moutsimilli, L., Cadetti, L., Jammoul, F., Wang, Q. P., Fan, Y., et al. (2014). Taurine: The comeback of a neutraceutical in the prevention of retinal degenerations. Prog. Retin. Eye Res. 41, 44–63. doi: 10.1016/j.preteyeres.2014.03.001
Gagliardi, G., Ben M’Barek, K., and Goureau, O. (2019). Photoreceptor cell replacement in macular degeneration and retinitis pigmentosa: A pluripotent stem cell-based approach. Prog. Retin. Eye Res. 71, 1–25. doi: 10.1016/j.preteyeres.2019.03.001
Garcia, M., Forster, V., Hicks, D., and Vecino, E. (2003). In vivo expression of neurotrophins and neurotrophin receptors is conserved in adult porcine retina in vitro. Invest. Ophthalmol. Vis. Sci. 44, 4532–4541. doi: 10.1167/iovs.03-0419
Garcia-Ayuso, D., Di Pierdomenico, J., Agudo-Barriuso, M., Vidal-Sanz, M., and Villegas-Perez, M. P. (2018). Retinal remodeling following photoreceptor degeneration causes retinal ganglion cell death. Neural Regen. Res. 13, 1885–1886. doi: 10.4103/1673-5374.239436
Garcia-Ayuso, D., Di Pierdomenico, J., Esquiva, G., Nadal-Nicolas, F. M., Pinilla, I., Cuenca, N., et al. (2015). Inherited photoreceptor degeneration causes the death of melanopsin-positive retinal ganglion cells and increases their coexpression of Brn3a. Invest. Ophthalmol. Vis. Sci. 56, 4592–4604. doi: 10.1167/iovs.15-16808
Garcia-Ayuso, D., Di Pierdomenico, J., Valiente-Soriano, F. J., Martinez-Vacas, A., Agudo-Barriuso, M., Vidal-Sanz, M., et al. (2019). Beta-alanine supplementation induces taurine depletion and causes alterations of the retinal nerve fiber layer and axonal transport by retinal ganglion cells. Exp. Eye Res. 188:107781. doi: 10.1016/j.exer.2019.107781
Guennoun, R. (2020). Progesterone in the brain: Hormone, neurosteroid and neuroprotectant. Int. J. Mol. Sci. 21:5271. doi: 10.3390/ijms21155271
Han, J., Dinculescu, A., Dai, X., Du, W., Smith, W. C., and Pang, J. (2013). Review: The history and role of naturally occurring mouse models with Pde6b mutations. Mol. Vis. 19, 2579–2589.
Harris, A. R., and Gilbert, F. (2022). Restoring vision using optogenetics without being blind to the risks. Graefes Arch. Clin. Exp. Ophthalmol. 260, 41–45. doi: 10.1007/s00417-021-05477-6
Hartong, D. T., Berson, E. L., and Dryja, T. P. (2006). Retinitis pigmentosa. Lancet 368, 1795–1809. doi: 10.1016/S0140-6736(06)69740-7
Hoang, T., Wang, J., Boyd, P., Wang, F., Santiago, C., Jiang, L., et al. (2020). Gene regulatory networks controlling vertebrate retinal regeneration. Science 370, eabb8598. doi: 10.1126/science.abb8598
Hodgetts, S. I., and Harvey, A. R. (2017). Neurotrophic factors used to treat spinal cord injury. Vitam. Horm. 104, 405–457. doi: 10.1016/bs.vh.2016.11.007
Ivanova, E., Yee, C. W., and Sagdullaev, B. T. (2016). Disruption in dopaminergic innervation during photoreceptor degeneration. J. Comp. Neurol. 524, 1208–1221. doi: 10.1002/cne.23899
Johnson, T. V., Bull, N. D., and Martin, K. R. (2011). Neurotrophic factor delivery as a protective treatment for glaucoma. Exp. Eye Res. 93, 196–203. doi: 10.1016/j.exer.2010.05.016
Jones, B. W., and Marc, R. E. (2005). Retinal remodeling during retinal degeneration. Exp. Eye Res. 81, 123–137. doi: 10.1016/j.exer.2005.03.006
Jones, B. W., Kondo, M., Terasaki, H., Watt, C. B., Rapp, K., Anderson, J., et al. (2011). Retinal remodeling in the Tg P347L rabbit, a large-eye model of retinal degeneration. J. Comp. Neurol. 519, 2713–2733. doi: 10.1002/cne.22703
Jones, B. W., Marc, R. E., and Pfeiffer, R. L. (2014). “Retinal remodeling and plasticity,” in Webvision: The organization of the retina and visual system, eds H. Kolb, R. Nelson, E. Fernandez, and B. W. Jones (Salt Lake City, UT: University of Utah Health Sciences Center).
Jones, B. W., Marc, R. E., Watt, C. B., Vaughan, D. K., and Organisciak, D. T. (2006). Neural plasticity revealed by light-induced photoreceptor lesions. Adv. Exp. Med. Biol. 572, 405–410. doi: 10.1007/0-387-32442-9_57
Jones, B. W., Pfeiffer, R. L., Ferrell, W. D., Watt, C. B., Marmor, M., and Marc, R. E. (2016a). Retinal remodeling in human retinitis pigmentosa. Exp. Eye Res. 150, 149–165. doi: 10.1016/j.exer.2016.03.018
Jones, B. W., Pfeiffer, R. L., Ferrell, W. D., Watt, C. B., Tucker, J., and Marc, R. E. (2016b). Retinal remodeling and metabolic alterations in human AMD. Front. Cell. Neurosci. 10:103. doi: 10.3389/fncel.2016.00103
Jones, B. W., Watt, C. B., Frederick, J. M., Baehr, W., Chen, C. K., Levine, E. M., et al. (2003). Retinal remodeling triggered by photoreceptor degenerations. J. Comp. Neurol. 464, 1–16. doi: 10.1002/cne.10703
Keeler, C. E. (1924). The inheritance of a retinal abnormality in white mice. Proc. Natl. Acad. Sci. U.S.A. 10, 329–333. doi: 10.1073/pnas.10.7.329
Kolomeyer, A. M., and Zarbin, M. A. (2014). Trophic factors in the pathogenesis and therapy for retinal degenerative diseases. Surv. Ophthalmol. 59, 134–165. doi: 10.1016/j.survophthal.2013.09.004
Kondo, M., Sakai, T., Komeima, K., Kurimoto, Y., Ueno, S., Nishizawa, Y., et al. (2009). Generation of a transgenic rabbit model of retinal degeneration. Invest. Ophthalmol. Vis. Sci. 50, 1371–1377. doi: 10.1167/iovs.08-2863
Kosta, P., Iseri, E., Loizos, K., Paknahad, J., Pfeiffer, R. L., Sigulinsky, C. L., et al. (2021). Model-based comparison of current flow in rod bipolar cells of healthy and early-stage degenerated retina. Exp. Eye Res. 207:108554. doi: 10.1016/j.exer.2021.108554
Kramer, R. H., Fortin, D. L., and Trauner, D. (2009). New photochemical tools for controlling neuronal activity. Curr. Opin. Neurobiol. 19, 544–552. doi: 10.1016/j.conb.2009.09.004
Krigel, A., Berdugo, M., Picard, E., Levy-Boukris, R., Jaadane, I., Jonet, L., et al. (2016). Light-induced retinal damage using different light sources, protocols and rat strains reveals LED phototoxicity. Neuroscience 339, 296–307. doi: 10.1016/j.neuroscience.2016.10.015
Kuehlewein, L., Troelenberg, N., Stingl, K., Schleehauf, S., Kusnyerik, A., Jackson, T. L., et al. (2019). Changes in microchip position after implantation of a subretinal vision prosthesis in humans. Acta Ophthalmol. 97, e871–e876. doi: 10.1111/aos.14077
Lamba, D. A., Karl, M. O., and Reh, T. A. (2009). Strategies for retinal repair: Cell replacement and regeneration. Prog. Brain Res. 175, 23–31. doi: 10.1016/S0079-6123(09)17502-7
Le Meur, G., Stieger, K., Smith, A. J., Weber, M., Deschamps, J. Y., Nivard, D., et al. (2007). Restoration of vision in RPE65-deficient Briard dogs using an AAV serotype 4 vector that specifically targets the retinal pigmented epithelium. Gene Ther. 14, 292–303. doi: 10.1038/sj.gt.3302861
Le, N., Appel, H., Pannullo, N., Hoang, T., and Blackshaw, S. (2022). Ectopic insert-dependent neuronal expression of GFAP promoter-driven AAV constructs in adult mouse retina. Front. Cell. Dev. Biol. 10:914386. doi: 10.3389/fcell.2022.914386
Lewis, G. P., Erickson, P. A., Anderson, D. H., and Fisher, S. K. (1991). Opsin distribution and protein incorporation in photoreceptors after experimental retinal detachment. Exp. Eye Res. 53, 629–640. doi: 10.1016/0014-4835(91)90223-2
Lewis, G. P., Erickson, P. A., Guerin, C. J., Anderson, D. H., and Fisher, S. K. (1989). Changes in the expression of specific muller cell proteins during long-term retinal detachment. Exp. Eye Res. 49, 93–111. doi: 10.1016/0014-4835(89)90079-1
Li, X., Zhang, L., Tang, F., and Wei, X. (2021). Retinal organoids: Cultivation, differentiation, and transplantation. Front. Cell. Neurosci. 15:638439. doi: 10.3389/fncel.2021.638439
Li, Z. Y., Kljavin, I. J., and Milam, A. H. (1995). Rod photoreceptor neurite sprouting in retinitis pigmentosa. J. Neurosci. 15, 5429–5438. doi: 10.1523/JNEUROSCI.15-08-05429.1995
Lim, L. S., Mitchell, P., Seddon, J. M., Holz, F. G., and Wong, T. Y. (2012). Age-related macular degeneration. Lancet 379, 1728–1738. doi: 10.1016/S0140-6736(12)60282-7
Maguire, A. M., Bennett, J., Aleman, E. M., Leroy, B. P., and Aleman, T. S. (2021). Clinical perspective: Treating RPE65-associated retinal dystrophy. Mol. Ther. 29, 442–463. doi: 10.1016/j.ymthe.2020.11.029
Marc, R. E., and Jones, B. W. (2003). Retinal remodeling in inherited photoreceptor degenerations. Mol. Neurobiol. 28, 139–147. doi: 10.1385/MN:28:2:139
Marc, R. E., Jones, B. W., Anderson, J. R., Kinard, K., Marshak, D. W., Wilson, J. H., et al. (2007). Neural reprogramming in retinal degeneration. Invest. Ophthalmol. Vis. Sci. 48, 3364–3371. doi: 10.1167/iovs.07-0032
Marc, R., Pfeiffer, R., and Jones, B. (2014). Retinal prosthetics, optogenetics, and chemical photoswitches. ACS Chem. Neurosci. 5, 895–901. doi: 10.1021/cn5001233
Martinez-Vacas, A., Di Pierdomenico, J., Gallego-Ortega, A., Valiente-Soriano, F. J., Vidal-Sanz, M., Picaud, S., et al. (2022). Systemic taurine treatment affords functional and morphological neuroprotection of photoreceptors and restores retinal pigment epithelium function in RCS rats. Redox Biol. 57:102506. doi: 10.1016/j.redox.2022.102506
Medeiros, N. E., and Curcio, C. A. (2001). Preservation of ganglion cell layer neurons in age-related macular degeneration. Invest. Ophthalmol. Vis. Sci. 42, 795–803.
Menon, A., and Vijayavenkataraman, S. (2022). Novel vision restoration techniques: 3D bioprinting, gene and stem cell therapy, optogenetics, and the bionic eye. Artif. Organs 46, 1463–1474. doi: 10.1111/aor.14241
Mor, D. E., Daniels, M. J., and Ischiropoulos, H. (2019). The usual suspects, dopamine and alpha-synuclein, conspire to cause neurodegeneration. Mov. Disord. 34, 167–179. doi: 10.1002/mds.27607
Nagel, G., Szellas, T., Huhn, W., Kateriya, S., Adeishvili, N., Berthold, P., et al. (2003). Channelrhodopsin-2, a directly light-gated cation-selective membrane channel. Proc. Natl. Acad. Sci. U.S.A. 100, 13940–13945. doi: 10.1073/pnas.1936192100
Narfstrom, K. (1983). Hereditary progressive retinal atrophy in the Abyssinian cat. J. Hered. 74, 273–276. doi: 10.1093/oxfordjournals.jhered.a109782
Nomura, A., Shigemoto, R., Nakamura, Y., Okamoto, N., Mizuno, N., and Nakanishi, S. (1994). Developmentally regulated postsynaptic localization of a metabotropic glutamate receptor in rat rod bipolar cells. Cell 77, 361–369. doi: 10.1016/0092-8674(94)90151-1
Pardue, M. T., and Allen, R. S. (2018). Neuroprotective strategies for retinal disease. Prog. Retin. Eye Res. 65, 50–76. doi: 10.1016/j.preteyeres.2018.02.002
Peng, B., Xiao, J., Wang, K., So, K. F., Tipoe, G. L., and Lin, B. (2014). Suppression of microglial activation is neuroprotective in a mouse model of human retinitis pigmentosa. J. Neurosci. 34, 8139–8150. doi: 10.1523/JNEUROSCI.5200-13.2014
Petters, R. M., Alexander, C. A., Wells, K. D., Collins, E. B., Sommer, J. R., Blanton, M. R., et al. (1997). Genetically engineered large animal model for studying cone photoreceptor survival and degeneration in retinitis pigmentosa. Nat. Biotechnol. 15, 965–970. doi: 10.1038/nbt1097-965
Pfeiffer, R. L., Anderson, J. R., Dahal, J., Garcia, J. C., Yang, J. H., Sigulinsky, C. L., et al. (2020). A pathoconnectome of early neurodegeneration: Network changes in retinal degeneration. Exp. Eye Res. 199:108196. doi: 10.1016/j.exer.2020.108196
Pfeiffer, R. L., Anderson, J. R., Emrich, D. P., Dahal, J., Sigulinsky, C. L., Morrison, H. A. B., et al. (2019). Pathoconnectome analysis of muller cells in early retinal remodeling. Adv. Exp. Med. Biol. 1185, 365–370. doi: 10.1007/978-3-030-27378-1_60
Pfeiffer, R. L., Marc, R. E., and Jones, B. W. (2020a). Muller cell metabolic signatures: Evolutionary conservation and disruption in disease. Trends Endocrinol. Metab. 31, 320–329. doi: 10.1016/j.tem.2020.01.005
Pfeiffer, R. L., Marc, R. E., and Jones, B. W. (2020b). Persistent remodeling and neurodegeneration in late-stage retinal degeneration. Prog. Retin. Eye Res. 74:100771. doi: 10.1016/j.preteyeres.2019.07.004
Pfeiffer, R. L., Marc, R. E., Kondo, M., Terasaki, H., and Jones, B. W. (2016). Muller cell metabolic chaos during retinal degeneration. Exp. Eye Res. 150, 62–70. doi: 10.1016/j.exer.2016.04.022
Pittler, S. J., Keeler, C. E., Sidman, R. L., and Baehr, W. (1993). PCR analysis of DNA from 70-year-old sections of rodless retina demonstrates identity with the mouse rd defect. Proc. Natl. Acad. Sci. U.S.A. 90, 9616–9619. doi: 10.1073/pnas.90.20.9616
Polosukhina, A., Litt, J., Tochitsky, I., Nemargut, J., Sychev, Y., Kouchkovsky, I. De, et al. (2012). Photochemical restoration of visual responses in blind mice. Neuron 75, 271–282. doi: 10.1016/j.neuron.2012.05.022
Prado, D. A., Acosta-Acero, M., and Maldonado, R. S. (2020). Gene therapy beyond luxturna: A new horizon of the treatment for inherited retinal disease. Curr. Opin. Ophthalmol. 31, 147–154. doi: 10.1097/ICU.0000000000000660
Rangasamy, S. B., Soderstrom, K., Bakay, R. A., and Kordower, J. H. (2010). Neurotrophic factor therapy for Parkinson’s disease. Prog. Brain Res. 184, 237–264. doi: 10.1016/S0079-6123(10)84013-0
Roche, S. L., Wyse-Jackson, A. C., Gomez-Vicente, V., Lax, P., Ruiz-Lopez, A. M., Byrne, A. M., et al. (2016). Progesterone attenuates microglial-driven retinal degeneration and stimulates protective fractalkine-CX3CR1 signaling. PLoS One 11:e0165197. doi: 10.1371/journal.pone.0165197
Sahel, J. A., Boulanger-Scemama, E., Pagot, C., Arleo, A., Galluppi, F., Martel, J. N., et al. (2021). Partial recovery of visual function in a blind patient after optogenetic therapy. Nat. Med. 27, 1223–1229. doi: 10.1038/s41591-021-01351-4
Semple-Rowland, S. L., and Lee, N. R. (2000). Avian models of inherited retinal disease. Methods Enzymol. 316, 526–536. doi: 10.1016/S0076-6879(00)16747-3
Sengupta, A., Chaffiol, A., Mace, E., Caplette, R., Desrosiers, M., Lampic, M., et al. (2016). Red-shifted channelrhodopsin stimulation restores light responses in blind mice, macaque retina, and human retina. EMBO Mol. Med. 8, 1248–1264. doi: 10.15252/emmm.201505699
Sethi, C. S., Lewis, G. P., Fisher, S. K., Leitner, W. P., Mann, D. L., Luthert, P. J., et al. (2005). Glial remodeling and neural plasticity in human retinal detachment with proliferative vitreoretinopathy. Invest. Ophthalmol. Vis. Sci. 46, 329–342. doi: 10.1167/iovs.03-0518
Singh, M., and Su, C. (2013). Progesterone, brain-derived neurotrophic factor and neuroprotection. Neuroscience 239, 84–91. doi: 10.1016/j.neuroscience.2012.09.056
Sitruk-Ware, R., Bonsack, B., Brinton, R., Schumacher, M., Kumar, N., Lee, J. Y., et al. (2021). Progress in progestin-based therapies for neurological disorders. Neurosci. Biobehav. Rev. 122, 38–65. doi: 10.1016/j.neubiorev.2020.12.007
Skaper, S. D. (2018). Neurotrophic factors: An overview. Methods Mol. Biol. 1727, 1–17. doi: 10.1007/978-1-4939-7571-6_1
Stefanov, A., Novelli, E., and Strettoi, E. (2019). Inner retinal preservation in the photoinducible I307N rhodopsin mutant mouse, a model of autosomal dominant retinitis pigmentosa. J. Comp. Neurol. 528, 1502–1522. doi: 10.1002/cne.24838
Stingl, K., Schippert, R., Bartz-Schmidt, K. U., Besch, D., Cottriall, C. L., Edwards, T. L., et al. (2017). Interim results of a multicenter trial with the new electronic subretinal implant alpha AMS in 15 patients blind from inherited retinal degenerations. Front. Neurosci. 11:445. doi: 10.3389/fnins.2017.00445
Strettoi, E., and Pignatelli, V. (2000). Modifications of retinal neurons in a mouse model of retinitis pigmentosa. Proc. Natl. Acad. Sci. U.S.A. 97, 11020–11025. doi: 10.1073/pnas.190291097
Strettoi, E., Pignatelli, V., Rossi, C., Porciatti, V., and Falsini, B. (2003). Remodeling of second-order neurons in the retina of rd/rd mutant mice. Vision Res. 43, 867–877. doi: 10.1016/S0042-6989(02)00594-1
Strettoi, E., Porciatti, V., Falsini, B., Pignatelli, V., and Rossi, C. (2002). Morphological and functional abnormalities in the inner retina of the rd/rd mouse. J. Neurosci. 22, 5492–5504. doi: 10.1523/JNEUROSCI.22-13-05492.2002
Suber, M. L., Pittler, S. J., Qin, N., Wright, G. C., Holcombe, V., Lee, R. H., et al. (1993). Irish setter dogs affected with rod/cone dysplasia contain a nonsense mutation in the rod cGMP phosphodiesterase beta-subunit gene. Proc. Natl. Acad. Sci. U.S.A. 90, 3968–3972. doi: 10.1073/pnas.90.9.3968
Szymanski, W., Beierle, J. M., Kistemaker, H. A., Velema, W. A., and Feringa, B. L. (2013). Reversible photocontrol of biological systems by the incorporation of molecular photoswitches. Chem. Rev. 113, 6114–6178. doi: 10.1021/cr300179f
Thorne, R. G., and Frey, W. H. II (2001). Delivery of neurotrophic factors to the central nervous system: Pharmacokinetic considerations. Clin. Pharmacokinet. 40, 907–946. doi: 10.2165/00003088-200140120-00003
Tochitsky, I., Polosukhina, A., Degtyar, V. E., Gallerani, N., Smith, C. M., Friedman, A., et al. (2014). Restoring visual function to blind mice with a photoswitch that exploits electrophysiological remodeling of retinal ganglion cells. Neuron 81, 800–813. doi: 10.1016/j.neuron.2014.01.003
Vecino, E., Rodriguez, F. D., Ruzafa, N., Pereiro, X., and Sharma, S. C. (2016). Glia-neuron interactions in the mammalian retina. Prog. Retin. Eye Res. 51, 1–40. doi: 10.1016/j.preteyeres.2015.06.003
Weiland, J. D., Cho, A. K., and Humayun, M. S. (2011). Retinal prostheses: Current clinical results and future needs. Ophthalmology 118, 2227–2237. doi: 10.1016/j.ophtha.2011.08.042
Witkovsky, P. (2004). Dopamine and retinal function. Doc. Ophthalmol. 108, 17–40. doi: 10.1023/B:DOOP.0000019487.88486.0a
Wong, W. L., Su, X., Li, X., Cheung, C. M., Klein, R., Cheng, C. Y., et al. (2014). Global prevalence of age-related macular degeneration and disease burden projection for 2020 and 2040: A systematic review and meta-analysis. Lancet Glob. Health 2, e106–e116. doi: 10.1016/S2214-109X(13)70145-1
Wu, S., Chang, K. C., Nahmou, M., and Goldberg, J. L. (2018). Induced pluripotent stem cells promote retinal ganglion cell survival after transplant. Invest. Ophthalmol. Vis. Sci. 59, 1571–1576. doi: 10.1167/iovs.17-23648
Yamanaka, S. (2020). Pluripotent stem cell-based cell therapy-promise and challenges. Cell Stem Cell 27, 523–531. doi: 10.1016/j.stem.2020.09.014
Yue, L., Weiland, J. D., Roska, B., and Humayun, M. S. (2016). Retinal stimulation strategies to restore vision: Fundamentals and systems. Prog. Retin. Eye Res. 53, 21–47. doi: 10.1016/j.preteyeres.2016.05.002
Zhou, J., Flores-Bellver, M., Pan, J., Benito-Martin, A., Shi, C., Onwumere, O., et al. (2021). Human retinal organoids release extracellular vesicles that regulate gene expression in target human retinal progenitor cells. Sci. Rep. 11:21128. doi: 10.1038/s41598-021-00542-w
Zhu, C., Wang, S., Wang, B., Du, F., Hu, C., Li, H., et al. (2015). 17beta-Estradiol up-regulates Nrf2 via PI3K/AKT and estrogen receptor signaling pathways to suppress light-induced degeneration in rat retina. Neuroscience 304, 328–339. doi: 10.1016/j.neuroscience.2015.07.057
Keywords: retinal remodeling, neurodegeneration, therapeutics, age-related macular degeneration, retinitis pigmentosa
Citation: Pfeiffer RL and Jones BW (2022) Current perspective on retinal remodeling: Implications for therapeutics. Front. Neuroanat. 16:1099348. doi: 10.3389/fnana.2022.1099348
Received: 15 November 2022; Accepted: 09 December 2022;
Published: 22 December 2022.
Edited by:
Diego García-Ayuso, University of Murcia, SpainReviewed by:
Johnny Di Pierdomenico, University of Murcia, SpainNicolás Cuenca, University of Alicante, Spain
Natalia Ziółkowska, University of Warmia and Mazury in Olsztyn, Poland
Copyright © 2022 Pfeiffer and Jones. This is an open-access article distributed under the terms of the Creative Commons Attribution License (CC BY). The use, distribution or reproduction in other forums is permitted, provided the original author(s) and the copyright owner(s) are credited and that the original publication in this journal is cited, in accordance with accepted academic practice. No use, distribution or reproduction is permitted which does not comply with these terms.
*Correspondence: Rebecca L. Pfeiffer, ✉ R.Pfeiffer@utah.edu; Bryan W. Jones, ✉ bryan.jones@m.cc.utah.edu