A safe agricultural space for biodiversity
- 1Institut du Développement Durable et des Relations Internationales (IDDRI), Paris, France
- 2Centre de Coopération Internationale en Recherche Agronomique Pour le Développement (CIRAD), UMR CIRED, Nogent-sur-Marne, France
- 3Institute for Resources, Environment and Sustainability, University of British Columbia, Vancouver, BC, Canada
- 4Department of Zoology, University of British Columbia, Vancouver, BC, Canada
- 5Biodiversity Research Center, University of British Columbia, Vancouver, BC, Canada
- 6Sciences Po, Paris, France
Agriculture is the main driver of the rapid collapse of biodiversity, upon which all life on Earth, including agricultural production, depends. As we face the challenge of feeding a growing human population under a changing climate regime, the pressure on biodiversity is expected to further intensify. While the potential to expand and improve natural habitats for biodiversity conservation has been widely explored in large-scale scenarios of agricultural systems, the critical role of agricultural landscapes’ management on halting the loss of biodiversity remains unexplored at this scale. We argue that, to achieve an effective conservation of biodiversity (both natural and agricultural), the combined multivariate effects of agriculture on biodiversity must be accounted for, including its surface area as well as its management. Based on a literature review, we identified the main biodiversity pressures stemming from agriculture: land-use change, contribution to climate change, water withdrawal, pesticide pollution, nutrient (nitrogen and phosphorus) pollution, and landscape and farm-scale simplification (of croplands and pastures). For each one, we proposed a critical boundary, based on reviews of studies covering a range of taxa, biodiversity metrics, and biomes, below or above which negative impacts on biodiversity are minimized or positive effects arise. Implemented simultaneously, the identified boundaries would integrate biodiversity conservation within and across farmlands and minimize agriculture’s far-reaching impacts on biodiversity. We present a framework called “agricultural boundaries for biodiversity” that will allow to explore the potential of developing agricultural systems that effectively reconcile food production and biodiversity conservation at large scales.
1 Introduction
Agriculture has dramatically altered the Earth’s face, as it now claims half of the ice-free terrestrial surface (IPBES, 2019). Through its expansion, intensification, and major contribution to climate change, it is primarily responsible for today’s large-scale collapse of biodiversity (Ceballos et al., 2015; Newbold et al., 2016; Beckmann et al., 2019; IPBES, 2019; Sánchez-Bayo and Wyckhuys, 2019; Rigal et al., 2023). As the functioning of ecosystems and their contributions to basic human needs are contingent upon biodiversity (Cardinale et al., 2012), agriculture is not only compromising the integrity of the biosphere, but also our capacity to feed ourselves in the near future (Dainese et al., 2019; FAO, 2019a; Seppelt et al., 2020).
Far from receding, pressure from agriculture is expected to further increase as the global demand for food (and especially resource-intensive products like meat and dairy), fiber and biofuel are projected to double by 2050 compared to 2005 (FAO 2009; Tilman et al., 2011; Miyake et al., 2012; Searchinger et al., 2019). Considering that the pressure of climate change on agricultural production is also intensifying (Ray et al., 2019), that yields of the major staple crops have already peaked across much of the globe (Ray et al., 2012), and that land degradation processes are affecting 80% of the world’s arable lands (Prăvălie et al., 2021), sustainably feeding the growing human population is a critical task requiring urgent attention and action.
As we hit biophysical limits, the need to adopt more plant-based diets, significantly cut food waste and loss, and halt deforestation, are widely agreed upon (Springmann et al., 2018; Willett et al., 2019). However, the definition of what a sustainable agricultural system would look like has remained highly controversial for decades (Green et al., 2005; Immovili and Kok, 2020). In some circles, the debate has polarized into “land sparing vs. land sharing”: respectively, increasing yields within farmlands to free up space for wildlife conservation (Phalan, 2018), or integrating biodiversity conservation within farmlands, even if it means lower yields and a greater farmland area (Perfecto and Vandermeer, 2010). Although it launched an important conversation, the limits of this framework have become evident, because neither extreme is ecologically desirable (Kremen, 2015), and because of its limited ability to take into account market dynamics (Desquilbet et al., 2017). Instead, a combination of both large, protected areas and hospitable farmlands are needed to achieve effective biodiversity conservation (Kremen, 2015; DeClerck et al., 2023).
In exploring how to reconcile the food and biodiversity imperatives, we argue that three key elements need to be better accounted for: (1) the importance of conserving agricultural biodiversity to maintaining the long-term functioning of agroecosystems, and the critical effects of agricultural management on natural biodiversity through (2) the structuring of the landscape matrix and (3) the spillover of biodiversity stressors beyond the limits of the farm.
Firstly, productive landscapes and their species communities represent a significant part of the biosphere, including many endangered species (Wintle et al., 2018). Millennia of low-intensity farming and extensive grassland management have formed unique ecological communities of high conservation value throughout Europe (Pärtel et al., 2005; Halada et al., 2011; Lomba et al., 2015). Further, agricultural biodiversity contributes, among others, to nurturing soils (Wagg et al., 2014), pollinating crops (Klein et al., 2006; Dainese et al., 2019), reducing pests’ pressures (Pretty and Bharucha, 2015; Dainese et al., 2019), and increasing the overall performance of crops (Wan et al., 2022). Maintaining the productive capacity of agroecosystems in the long run largely depends upon the conservation of agrobiodiversity (Altieri, 1999; Kremen and Miles, 2012; Seppelt et al., 2020). Despite its critical ecological and productive value, agricultural biodiversity is systematically unaccounted for in large-scale biodiversity assessments and prospective models exploring scenarios of sustainable agriculture. Some of the most commonly used biodiversity indices do not capture farmland communities: the Biodiversity Intactness Index (BII) and Mean Species Abundance (MSA) measure the richness and abundance of remaining “original” species communities, based on primary vegetation or pre-industrial era records (e.g., Alkemade et al., 2009; Newbold et al., 2016; Gerten et al., 2020; Leclère et al., 2020). Thus, “novel” species found in farmlands cannot score high, even if they form ecologically diverse and functional communities (Vačkář et al., 2012). Other indices like the Living Planet Index (LPI) only consider wild vertebrate species, which are also overrepresented in measures of Red List Index (RLI) and extinction risk and rate due to sampling bias (e.g., Tilman et al., 2017; Willett et al., 2019; Leclère et al., 2020; WWF, 2022), and thus omit most agrobiodiversity. Finally, agricultural area has been widely used as a proxy of biodiversity impacts (e.g., Foley et al., 2011; Balmford et al., 2018; Springmann et al., 2018, 2020), which implicitly disregards the value of agricultural biodiversity. Specific indices have been developed for farmland communities, like the Agrobiodiversity Index (ABDI) (Jones et al., 2021), but are yet to be widely integrated into prospective studies of food systems, and require large amounts of consistent and fine-scale global data.
Moreover, the management of agricultural landscapes has critical effects on natural biodiversity, as farmlands provide connectivity between fragmented patches of natural habitat, where wild species would otherwise remain isolated and progressively decline (i.e., extinction debt; Hylander and Ehrlén, 2013). Wildlife-friendly farmlands could facilitate their movement, provisioning, dispersal, gene flow, and capacity to adapt to climate change, thus promoting species persistence (Kremen and Merenlender, 2018). When placed along the edges of fragmented forests, closed-canopy, low-input agricultural systems like agroforestry can protect forest biodiversity by buffering microclimatic changes (Meza-Elizalde and Armenteras-Pascual, 2021). It is argued that our success to conserve the world’s wildlife will largely depend upon the hospitality of agricultural lands (Mendenhall et al., 2014; Brennan et al., 2022). This ecological function of farmland is never accounted for in large-scale assessments of sustainable agriculture scenarios, except perhaps for the minor integration of crop diversity in Folberth et al.’s (2020) simulations.
Finally, certain agricultural practices within fields can have long-range negative impacts on biodiversity. Nutrients and pesticides applied to farms can travel far and wide across landscapes (Simonich and Hites, 1995; Peñuelas et al., 2013) and alter species communities around the globe (Alkemade et al., 2009; Tang et al., 2021). Natural protected areas may not effectively stop the loss of wild species unless the pressures spilling over from intensive farmlands are halted (Phoenix et al., 2006; Hallmann et al., 2017). Farmlands may further affect distant biodiversity through the withdrawal of water for irrigation, which is a major driver of the rapid collapse of freshwater biodiversity (Vörösmarty et al., 2010), as well as through climate change, as agricultural production contributes a significant part of global greenhouse gas (GHG) emissions (Pörtner et al., 2021), although at highly variable rates across products and production systems (Poore and Nemecek, 2018). However, these stressors are not weighed in the calculations of the biodiversity impacts of agricultural scenarios in large-scale assessments, which solely account for the direct effects of land use change. Only attributing biodiversity loss to the area occupied by agriculture, and not taking into account the important off-farm effects, can lead to proposals to further intensify agriculture that exacerbates stressors like global nutrient pollution (e.g., Foley et al., 2011; Tilman et al., 2011).
To refine those assessments, and better integrate all forms of biodiversity in food systems scenarios, we need a framework that accounts for the combined multivariate effects of agriculture, instead of individual stressors (Benton et al., 2003). Treating drivers of biodiversity loss like N (e.g., de Vries et al., 2021) and P pollution (e.g., Carpenter and Bennett, 2011), GHG emissions (e.g., Popp et al., 2017), or water withdrawal (e.g., Jägermeyr et al., 2017) individually, rather than as interacting components behind biodiversity loss, may lead to partial solutions for biodiversity conservation.
Hereafter, we present such a framework that accounts for the multivariate stressors stemming from agriculture, to define a safe space for biodiversity across the world’s farmlands, which can be used to refine our understanding of the “biophysical option space” to feed the world while minimizing impacts on biodiversity. This framework relies on the identification of a set of boundaries for agricultural systems that, implemented simultaneously, would integrate biodiversity conservation within and across farmlands, and minimize agriculture’s far-reaching impacts on biodiversity. While it would need to be adapted to specific local contexts for management purposes, this framework can be generalized to the global scale.
2 Agricultural boundaries for biodiversity
2.1 Our approach: overview
Based on an extensive but not systematic literature review, we identified the main biodiversity pressures stemming from agriculture and proposed a critical boundary, for each one that would ensure biodiversity conservation. Boundaries include ceilings below which negative impacts on biodiversity are limited, as well as thresholds at or above which positive effects on biodiversity arise. Depending on the nature of the pressure and its relationship to biodiversity, as well as the evidence available, boundaries may apply to pressures and then translate into agricultural practices, or apply directly to practices. Figure 1 illustrates the resulting agricultural system, constrained by the multiple boundaries that would ensure effective biodiversity conservation, which we expand upon in Table 1. Table 2 summarizes the proposed boundaries identified in the literature.
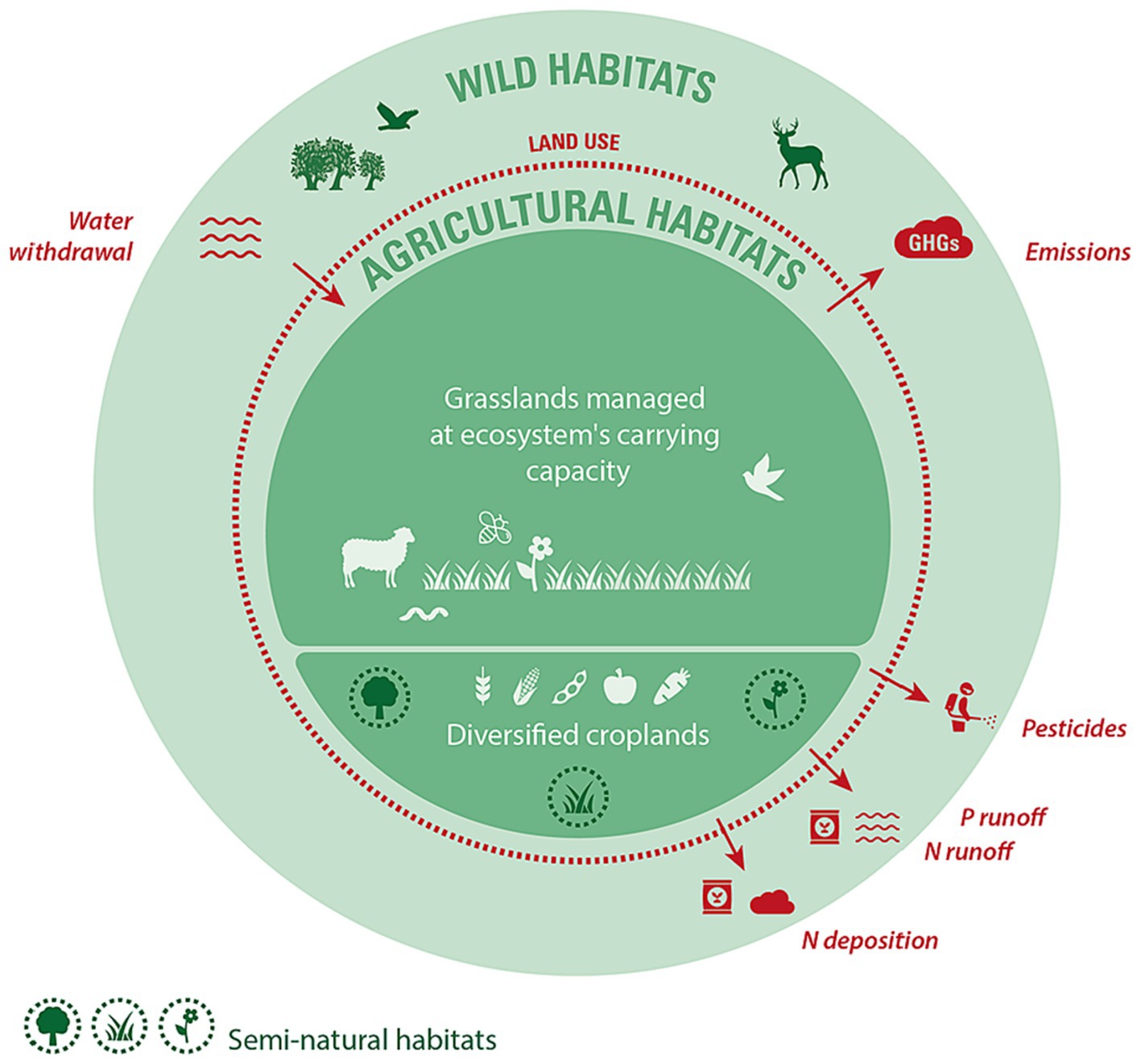
Figure 1. Diagram illustrating the critical boundaries delimiting an agricultural system that is compatible with effective biodiversity conservation. Red boundaries are pressures that need to be contained under a critical limit, while green boundaries are key determinants of biodiversity that need to be maintained at or above a minimal threshold or management regime. Dashed lines indicate that there is continuity and exchange between agricultural, semi-natural, and wild habitats and biodiversity. The relative areas of grasslands (i.e., pastures and rangelands) and croplands shown in the diagram represent current land uses by the IPCC (2019), but do not prescribe these relative proportions.
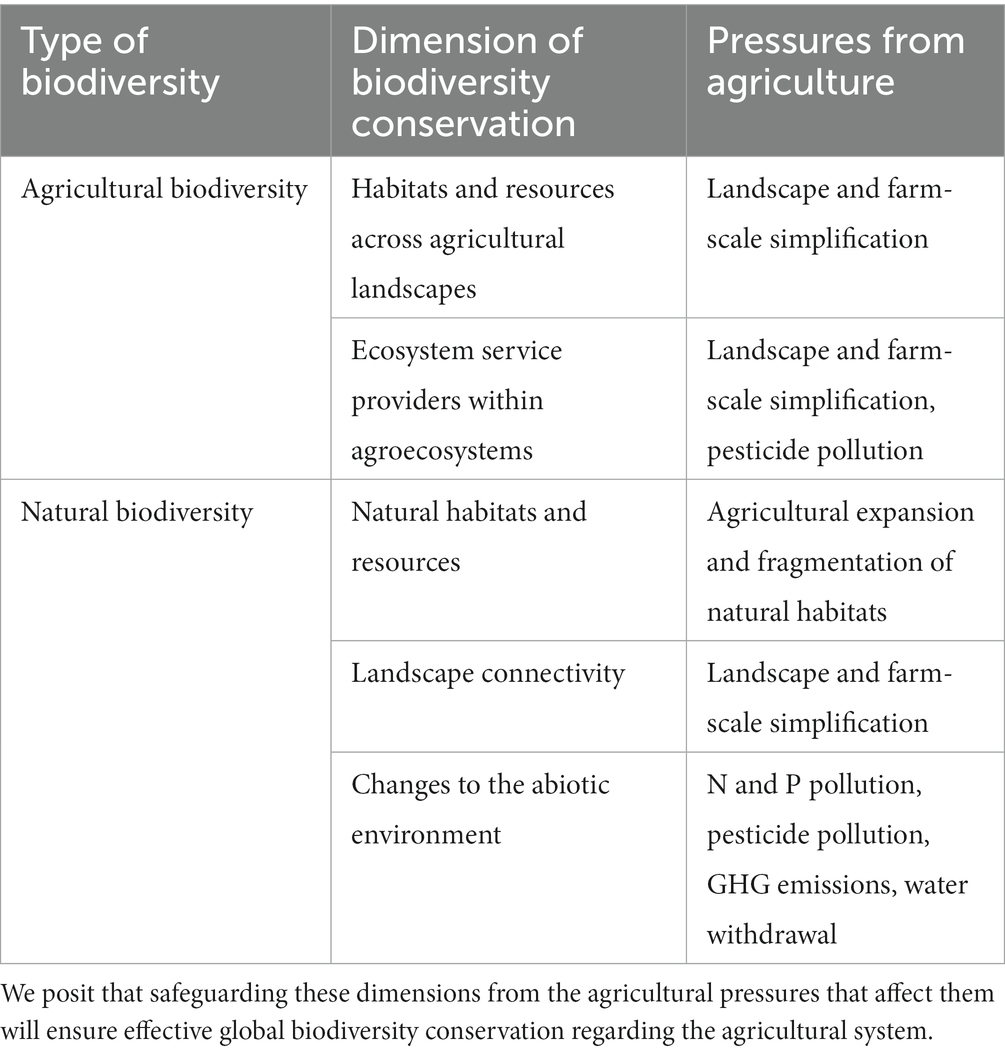
Table 1. The key dimensions of agricultural and natural biodiversity conservation, and their associated pressures stemming from agriculture.
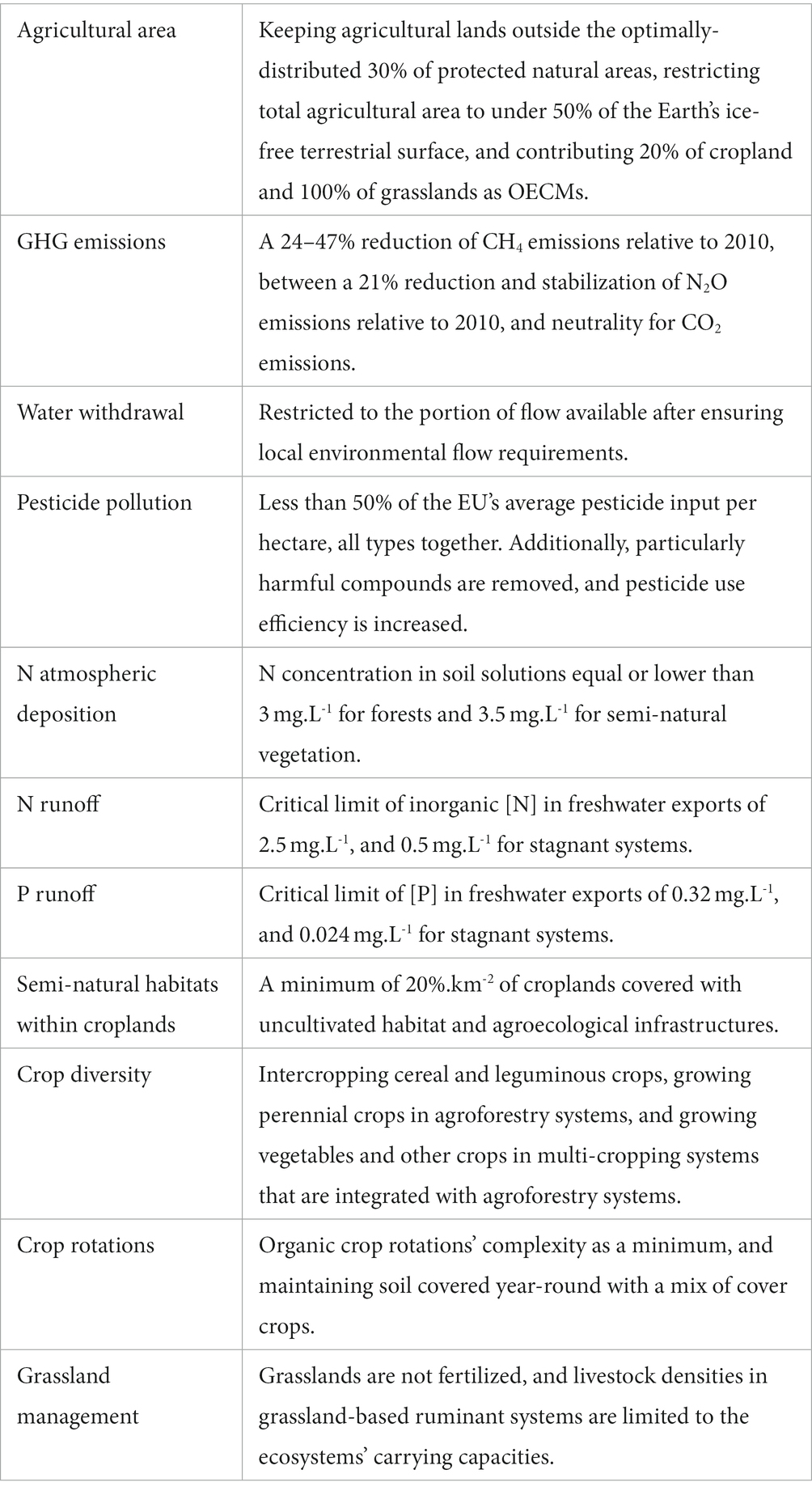
Table 2. The proposed boundaries for each of the 11 key biodiversity pressures and determinants identified in the literature.
We considered five key dimensions of biodiversity conservation, which we understand as requirements for effective conservation of global biodiversity, including both natural and agricultural biodiversity (see Table 1). Two dimensions are important for the conservation of agrobiodiversity: providing habitat and resources for species inhabiting agricultural landscapes (Benton et al., 2003; Estrada-Carmona et al., 2022), as well as conserving functional groups that provide critical ecosystem services at the field level (i.e., pollinators, natural enemies, and soil biota) (Potts et al., 2010; Kremen and Miles, 2012). Key dimensions for natural biodiversity are the conservation of natural habitats for wildlife (Watson et al., 2018; Wintle et al., 2018), the connectivity across landscapes (Perfecto and Vandermeer, 2010; Brennan et al., 2022), and limiting the external impacts of farmland management across the globe (i.e., pesticide and nutrient pollution, GHG emissions, and water withdrawal) (Vörösmarty et al., 2010; Peñuelas et al., 2020; Sánchez-Bayo, 2021). These dimensions affect biodiversity at its three levels: genetic (e.g., gene flow is facilitated by landscape connectivity; Gómez-Fernández et al., 2016, plant genetic diversity is enhanced by pollination; Feigs et al., 2022), interspecific (e.g., species co-existence is allowed by resource partitioning and complementation of habitats; Sirami et al., 2019), and ecosystemic (e.g., ecosystems’ composition, structure, and functions are affected by atmospheric fertilization; Bobbink et al., 2010).
To identify the main pressures through which agriculture affects these key dimensions of biodiversity, a broad initial search was conducted using key words relating to biodiversity and agriculture, and the arguments and evidence that have accumulated around the land sparing-land sharing debate were analyzed. We selected mostly empirical studies assessing the effects of specific agricultural variables on a wide range of taxa, with biodiversity metrics ranging across the Essential Biodiversity Variables, with evidence from across biomes, and preferring global meta-analyses finding consistent patterns and relationships across taxa and biomes. Some modeling and theoretical papers were also included.
We identified, as the main drivers of biodiversity change resulting from agricultural activities, land-use change, climate impacts, water withdrawal, pesticide pollution, nutrient (nitrogen and phosphorus) pollution, and landscape and farm-scale simplification (of croplands and pastures). Table 1 lists the pressures that are associated with each dimension of biodiversity conservation.
We did not consider other biodiversity pressures that are associated with agriculture, such as the degradation of the ozone layer or particulate matter pollution, present in the LCA-Impact framework (Hardaker et al., 2022), as well as the extraction of minerals and fossil fuels, land artificialization, construction of infrastructures, or the proliferation of invasive species, present in the STAR metric (Mair et al., 2021), because their link with agriculture is weak. Further, while the mechanical disturbance of soils through tilling, plowing and heavy machinery may be an additional key pressure of agriculture on soil biodiversity, the evidence was inconclusive and did not allow us to identify a respective boundary (see section “Discussion”).
Assessing the effects of agricultural pressures on biodiversity poses several challenges. Agricultural practices may affect different elements of biodiversity (e.g., varying effects across taxa and across measures of ecological dynamics, like richness, abundance, diversity, dominance, community composition, etc.), and they interact with each other (e.g., the presence of semi-natural elements within fields reduces the impacts of pesticides on insects; Garibaldi et al., 2021, or buffer strips along river banks reduce nutrient losses to freshwater ecosystems; Borin et al., 2010). Thus, studies seeking to measure the effects of agricultural pressures on biodiversity have to make assumptions and simplifications like focusing on a specific pressure (e.g., agricultural area), or a specific element of biodiversity (e.g., a reference taxon, a partial metric of diversity). We do not define our biodiversity-safe agricultural system based on any specific ecological measure, instead, we identify conservative, critical boundaries to the key agricultural pressures that would prevent impacts on a wide array of biodiversity elements and would preserve functioning ecosystems, based on broad literature reviews ranging, to some extent, across taxa, metrics, and biomes. While this methodology is not exempt from assumptions, it allows to better account for the multivariate and interacting effects of agricultural practices on biodiversity (Benton et al., 2003).
Putting all the critical boundaries of agricultural pressures together results in a framework describing an agricultural system that is compatible with effective biodiversity conservation (see Figure 1). The framework is flexible and can be updated as new information becomes available. Further, while it is designed to be generalized at large scales, such as for studies of global agricultural systems, the boundaries may be adapted to specific local or regional conditions for the landscape scale.
The need to move beyond the assessment of broad strategies like land sparing and sharing, and advance toward the definition of the specific management strategies that could reconcile biodiversity conservation with food production, has been called for years (Kremen, 2015). Following is our proposed framework for such an agricultural system.
2.2 Agricultural area
Over the last three centuries, the Earth’s lands have shifted from mostly wild to mostly anthropogenic. In 1700, half of the ice-free land was wild, and another 45% was semi-natural (Ellis et al., 2010). By 2015, wild ecosystems with minimal human use were reduced to 28% of the land surface (12% of which being barren lands), and the rest was directly occupied or exploited by humans: croplands taking up 12% of the land, pastures, and rangelands (i.e., native ecosystems that are grazed) occupying 37%, and managed forests another 22% (IPCC, 2019).
The conversion of natural habitats into human land uses, predominantly driven by agricultural expansion (Pendrill et al., 2022), is considered the strongest driver of biodiversity loss globally (Sala et al., 2000; Newbold et al., 2015). Primary vegetation and mature secondary vegetation ecosystems generally host the highest levels of biodiversity of all land uses across the globe (Newbold et al., 2015), and some biomes’ species communities are particularly sensitive to human disturbance and will only thrive in primary vegetation (García-Vega and Newbold, 2020). Indeed, many species rely on their native, natural habitat and cannot survive even in the most complex human land uses (Phalan, 2018). As such, the world’s remaining intact forests host the majority of terrestrial biodiversity, including many rare and endangered species that are dependent upon the conservation of large contiguous tracts of primary forest (Watson et al., 2018). The loss of habitat also leads to its fragmentation which, although may not be necessarily negative for biodiversity per se (Fahrig, 2017; Fahrig et al., 2019), it can pose additional threats. 70% of remaining forests are within 1 km of the forest edge (Haddad et al., 2015), from whence physical and biotic pressures may intrude, such as hunting, logging (Watson et al., 2018), the proliferation of invasive species (Gibson et al., 2013), microclimatic changes along the edges (Meza-Elizalde and Armenteras-Pascual, 2021), and exposure to weather variability and extreme events (Laurance et al., 2011). Further, isolation of populations in small habitat patches decreases their gene flow and increases inbreeding (Honnay et al., 2005), and prevents species occurring at low densities from maintaining viable populations, leading to their extinction (Watson et al., 2018). Evidence from around the world shows populations of mammals, birds, reptiles, invertebrates, and plants going extinct following the loss of habitat, after a time delay that depends strongly on the area of the remaining habitat patch (Halley and Iwasa, 2011; Halley et al., 2016).
Well-resourced and managed protected areas have proven effective at conserving biodiversity (Gray et al., 2016). Proposed area-based protection targets for biodiversity conservation range between protecting 30 and 70% of ecologically-representative lands (Noss et al., 2012; Woodley et al., 2019). Notably, Jung et al. (2021) find that protecting or implementing effective conservation across the most optimal 30% of terrestrial lands would be enough to conserve 81% of known, extant vertebrate and plant species represented in global databases. Further, Dinerstein et al. (2019) find that a total of 32.6% of the terrestrial area would cover the most important habitats for the rarest species, those with the narrowest ranges, and those most endangered, as well as the most biodiverse ecoregions across all biomes in terms of endemism, highest alpha- and beta-diversities, and wide-ranging species requiring large areas to maintain viable populations. However, including other aspects such as migration routes of megafauna, connectivity, old-growth forests, freshwater ecosystems, and uncertainty, would require a larger area, thought to be around 50% (Dinerstein et al., 2019), which meets other proposals (e.g., Wilson, 2016). The additional 20% of area needed may be managed with other effective area-based conservation measures (OECMs), rather than protected areas (Dudley et al., 2018), where sustained, long-term conservation of biodiversity and ecosystem functions and services should result from their management, which potentially includes natural or semi-natural corridors and grasslands (IUCN, 2019).
Therefore, based on the target of protecting 30% of ecologically-representative and optimally-located natural protected areas, and implementing OECMs across a further 20% of land, we propose keeping agricultural lands outside the optimally-distributed 30% of protected natural areas, restricting total agricultural area to under 50% of the Earth’s ice-free terrestrial surface, and contributing 20% of cropland and 100% of grasslands as OECMs (see sections 7.a. on within-farms semi-natural habitats, and 8. on grassland management).
2.3 Greenhouse gas emissions
The agricultural production of food is responsible for 12.0 ± 2.9 GtCO2e.year-1, or 18–29% of global GHG emissions, including within-farm-gate crop and livestock production as well as land-cover change for food production (Vermeulen et al., 2012; IPCC, 2019; Rosenzweig et al., 2020). Major GHG sources related to agriculture include the clearing of land for agriculture, which emits carbon dioxide (CO2) and nitrous oxide (N2O), the methane (CH4) emissions from enteric fermentation of ruminant livestock, the CH4 emissions from rice paddies, and the CH4 and N2O emissions from livestock manure. Further, the manufacture and application of fertilizers, pesticides and other agrochemical substances emit CO2, N2O, and CH4, and the use of fossil fuel energy for activities related to food production emits CO2 (Clark et al., 2020).
Changes in temperatures, precipitation, and the frequency and intensity of extreme weather events directly affect organisms and biodiversity by affecting their survival (IPBES, 2019). However, it is the indirect effects of climate change that are expected to be most important. They include, notably, the spatial shift of habitats that are suitable for species, which disproportionately affects species with narrow mobility ranges, specialized niches, and those isolated by topography (e.g., mountains), geography (e.g., islands) or the hostility of surrounding landscapes (e.g., intensive farmland) (Foden et al., 2013). The resulting shift in the geographic distribution of species across landscapes alters species communities and biotic interactions (Bellard et al., 2012; Staudinger et al., 2013), like food webs (e.g., Lucas et al., 2018). The effects of shifting climatic suitability across landscapes on the distribution of species are predicted to become the main driver of vertebrate diversity loss by the end of the century (Newbold, 2018), and a major driver of species extinction across taxa (Thomas et al., 2004). Some important agricultural biodiversity such as bumblebees (Kerr et al., 2015; Soroye et al., 2020) and farmland birds (Birdlife International, 2018) are particularly at risk. Other indirect climate impacts include phenological mismatches between interacting species (Scranton and Amarasekare, 2017), the proliferation of invasive alien species and pathogens, and altered river flow regimes (IPBES, 2019), to name a few.
The effects of climate change on biodiversity being nonlinear, multivariate, and interacting (IPBES, 2019), there is no simple limit that would prevent biodiversity impacts. Thus, we decided to take the climate target defined in article 2 of the Paris Agreement, for which there is scientific evidence that impacts on biodiversity, ecosystems, and nature’s contributions to people would be limited, compared to scenarios with higher warming (IPCC, 2018): limiting the increase in global average temperatures to well below 2°C above pre-industrial levels, and pursue efforts to limit it to 1.5°C (UNFCCC, 2015).
Defining a climate target for the Agriculture, Forestry and Other Land Uses (AFOLU) sector depends on the share of the burden assumed by other sectors, as well as on the choice of metric used to express the various GHGs emitted by agriculture, which differ widely in their atmospheric lifespans and radiative efficiencies (Cain et al., 2019). Given that no common emission metric is widely accepted, we propose to define a climate target per GHG type. To integrate different decarburization pathways across sectors, we propose using the interquartile ranges available to the AFOLU sector resulting from the IPCC’s ensemble of integrated 1.5°C scenarios of the Special Report on Global Warming of 1.5°C (IPCC, 2018): a 24–47% reduction of CH4 emissions, and between a 21% reduction and stabilization of N2O emissions, relative to 2010, and neutrality for CO2 emissions (Huppmann et al., 2019).
2.4 Water withdrawal
In under 1% of the Earth’s surface, freshwater habitats host around 10% of all known species of animals and vascular plants, including one third of all vertebrates, and many endemic species (Balian et al., 2008). Primarily driven by pollution (see parts 5. and 6. below on pesticide and nutrient pollution), habitat degradation, and altered flow regimes, freshwater habitats are witnessing the most rapid decline in biodiversity across all ecosystem types (WWF, 2022).
Consuming over 70% of all human withdrawal of freshwater, irrigation represents the largest user of freshwater globally (Siebert and Döll, 2010). Water withdrawal reduces rivers’ flows, and the construction of dams to regulate stream flows, for irrigation and other uses, has fragmented 75% of the world’s main rivers (Pastor et al., 2014). The construction of dikes, levees, and channels threatens floodplain ecosystems (Serra-Llobet et al., 2022). The impoundment and depletion of river flows have contributed to the widespread degradation of aquatic ecosystems around the globe and are significant drivers of the collapse of freshwater biodiversity (Vörösmarty et al., 2010).
River flow regimes are widely considered the “master variable” affecting riverine ecological dynamics. Alterations to streamflow include changes in the magnitude, duration, timing, and frequency of high and low flows, and of extreme events like flooding and drought (Poff and Zimmerman, 2010). They have direct effects on biodiversity, as hydrodynamic forces act on organisms and affect their relative ecological success (e.g., changes to the energy cost of underwater drag, affected dispersal), as well as indirect effects, by modifying biotic and abiotic habitats (e.g., distribution of sediment particles, distribution of organisms entailing altered biotic interactions) (Hart and Finelli, 1999). Altered flow regimes tend to shift community compositions toward more generalist species, and favor colonization by non-native species. Further, the survival of aquatic species usually requires a minimum flow to be maintained throughout time, and wetlands and floodplains depend upon a stable flooding regime (Pastor et al., 2014).
92% of studies assessed in Poff and Zimmerman’s (2010) meta-analysis found negative effects of flow alteration on riverine or riparian ecosystems. Observed changes in stream ecosystems include, among others, the loss of endemic, native and sensitive species, reduced reproduction and recruitment, disruption of spawning cues, altered species assemblages and dominant taxa, and increases in non-native species. In riparian ecosystems, shifts in community composition, increases in exotic species, and reduced germination of seeds and plant growth are reported. Changes in the average and short-term variation of river discharge were found to consistently reduce fish abundance and diversity. Moreover, changes in the total river discharge or minimum flows were generally associated with a decline in macroinvertebrate abundance and diversity. No consistent responses of species diversity and abundance to reduced flood peaks was found in riparian ecosystems, which may be partially explained by changes in species assemblages: in some cases, species numbers increased with the terrestrialisation of the riparian community (Poff and Zimmerman, 2010).
Given the taxonomic and regional variability of ecological responses to flow alterations, as well as the paucity of available data, and the nonlinearity of ecohydrological relationships, defining simple threshold relationships between freshwater biodiversity and flow metrics that can be used at large scales has not been possible (Poff and Zimmerman, 2010; Pastor et al., 2014). Local environmental flow requirements (EFRs), i.e., the amount of water needed by freshwater and estuarine ecosystems to maintain their ecological functions and services (Bribane Declaration, 2007), are considered the best proxy to freshwater biodiversity conservation (Pastor et al., 2014). The variable monthly flow method (VMF) has been shown to be appropriate for large-scale assessments, as it correlates strongly with locally-calculated EFRs (Pastor et al., 2014), and has already been used to assess maximum water withdrawals allowed for irrigation to stay within EFRs (see Jägermeyr et al., 2017). We propose to critically limit water withdrawal to the portion of flow available after ensuring local environmental flow requirements. Other sectors contributing to water withdrawal (i.e., household, industry, and power plants) should be accounted for when back-calculating water available for irrigation (Jägermeyr et al., 2017).
2.5 Pesticide pollution
Pesticides are toxic bioactive substances including herbicides, insecticides, nematicides, fungicides, and soil fumigants. Their effects on non-target organisms, populations, and whole communities, both within and outside of fields, have become a clear major driver of biodiversity loss globally.
A pan-European study assessing the multiple components of agricultural intensification in wheat fields found that the use of insecticides and fungicides had the most consistent negative effects on the diversity of wild plants, ground beetles, and birds (Geiger et al., 2010). Detrimental effects of insecticides, fungicides, and herbicides have also been reported on soil fauna and bacterial communities (Bünemann et al., 2006; Baxter and Cummings, 2008). Systemic insecticides, in particular, which have become predominant in farmlands around the world in the past 2 decades (DiBartolomeis et al., 2019), have proven highly toxic to a broad range of taxa, from microbes to insect pollinators, terrestrial and aquatic invertebrates, fish, birds, mammals, and amphibians, starting at low and acute doses (Pisa et al., 2021). Indirect effects of pesticides cascading across trophic chains and affecting entire populations, communities, and ecosystem services have also been reported (Hawes et al., 2003; Boatman et al., 2004; Köhler and Triebskorn, 2013; Gibbons et al., 2015; Sánchez-Bayo, 2021).
Services and functions of agroecosystems are compromised by the application of these compounds, which are consistently found to hamper biological pest control (Geiger et al., 2010), pollination (Potts et al., 2010), and nutrient recycling and plant nutrition (Giovannetti et al. 2021). Before lethal effects happen, lower doses of pesticides can produce sub-lethal effects that also affect biodiversity and ecosystem services, such as compromising the immune system of honeybees and rendering them vulnerable to disease (Potts et al., 2010). Further, interaction of different pesticides can cause additive and synergistic negative effects on species, lowering critical doses manyfold (e.g., Biddinger et al., 2013).
Finally, pesticides’ impacts on biodiversity extend far beyond agricultural fields. The rapid collapse of entomofauna that has been recently reported around the globe, even within natural protected areas, has been largely attributed to the application of pesticides in agricultural landscapes (Hallmann et al., 2017; Sánchez-Bayo and Wyckhuys, 2019), which travel far and wide across the globe (Lode et al., 1995; Simonich and Hites, 1995).
Although clearly proven, the effects of pesticides on biodiversity and ecosystem services are non-linear, strongly dependent upon the type of compounds, their application regimes, their interactions, biogeographical features (Sabatier et al., 2013), and lethal doses vary widely even within taxonomic groups (e.g., Pisa et al., 2015). Defining a safe critical biodiversity load for pesticides is not possible. While current inputs of pesticides (i.e., 100% in high use regions such as the EU) are not compatible with biodiversity conservation, their total removal (i.e., 0%) is not justified as a necessary condition for effective biodiversity conservation.
Given this uncertainty, we therefore propose applying a midpoint: a global limit corresponding to less than 50% of the EU’s average pesticide input per hectare, all types together. A subsequent, more precautionary limit could range lower than the midpoint. Additionally, particularly harmful compounds like systemic insecticides would be removed, and pesticide use efficiency would be increased through the use of more selective and efficient pesticides and application equipment, and training farmers on best practices.
2.6 Nutrient pollution
Synthetic and mineral fertilizers have dramatically increased the load and changed the stoichiometry of the Earth’s N and P flows (Peñuelas et al., 2020). These nutrients are key limiting factors to plant growth, so their input can alter ecosystem dynamics at multiple levels.
In aquatic ecosystems, nutrient inputs can cause direct toxicity on fauna, water acidification, and can lead to eutrophication and consequent algal blooms, proliferation of toxic algae, water turbidity, and depletion of deep-water oxygen; which are detrimental to a broad range of species across trophic levels and threaten to drive entire ecosystems to their collapse (Smith, 2003; Camargo and Alonso, 2006).
In terrestrial ecosystems, ecological niche theory states that addition of limiting factors to an ecosystem can decrease the number of coexisting species, as it reduces the niche dimensionality (i.e., its number of constraints; Tilman and Lehman, 2001). Indeed, experimental studies find that nutrient inputs alter plant species communities over time: they shift toward ecological dominance by a few competitive species and exclude species adapted to low fertility, consequently decreasing overall species richness (e.g., Harpole and Tilman, 2007; Billeter et al., 2008; Clark and Tilman, 2008). As oligotrophs are rarer, a widespread shift favoring eutrophic species may cause a homogenization of communities across landscapes (Roth et al., 2013). High concentrations of N gases and of reduced-N forms (ammonia and ammonium) in the soil can also cause direct toxicity to sensitive plant species and increase their vulnerability to secondary stressors, and long-term accumulation of N to soils can cause acidification and consequently hamper plant growth (Bobbink et al., 2010).
Finally, anthropogenic shifts in the N:P ratios across terrestrial and aquatic ecosystems alter species competition across taxa and have cascading effects on communities (Peñuelas et al., 2020): while the effects on terrestrial communities remain unclear, N:P ratios are negatively associated with the diversity of zoo- and phytoplankton in marine and freshwater ecosystems, particularly in lakes.
The relative importance of N and P limitation for eutrophication is an ongoing controversy. N has long been considered most important as a limiting factor in terrestrial and marine ecosystems (Vitousek and Howarth, 1991; Soons et al., 2017), while P limitation is considered more important in lakes (Schindler et al., 2016). However, although some ecosystems respond more strongly to the input of one or the other nutrient, co-limitation by both nutrients is found to some extent across all ecosystems (Elser et al., 2007; Bratt et al., 2020). As the debate is not yet settled, we propose limiting both N and P for all ecosystem types.
Agricultural N and P pollution arises when a fraction of the nutrients’ surplus (i.e., the nutrients that are not taken up by plants) are lost through water or air transport. Sources of reactive N and P include synthetic and organic (e.g., compost, green manure) fertilizers, livestock manure applied to cropland or deposited in grasslands, and livestock manure management in barns and feedlots, and different sources vary in their susceptibility to give away nutrients to the air and water. The limits identified below do not apply to a specific source, but to the cumulative inputs from all sources, minus naturally-occurring denitrification in the case of N, and P accumulation in soils. Further, they apply and limit all farming systems, including crops and livestock. Finally, non-agricultural sources of N and P deposition and runoff also exist, including urban losses, industry, food waste, biomass burning, or natural vegetation (Morée et al., 2013; Sapek, 2013), and should be accounted for in the back-calculation of critical inputs.
2.6.1 Nitrogen
2.6.1.1 Atmospheric deposition of NOx and NH3
N is lost to the atmosphere in the form of nitrous oxide (N2O), nitrogen oxides (NOx), and ammonia (NH3). The two latter can then be deposited up to thousands of kilometers away from their source and affect ecosystems through their fertilization. Research on the effects of atmospheric N deposition on terrestrial ecosystems over the past four decades has given way to the concept of critical N loads: N inputs below which plant species diversity is not significantly affected (Bobbink et al., 2010). Critical loads vary across ecosystem types, but they remain uncertain for many regions beyond Europe and North America. They can be determined empirically, through N-addition field experiments or mesocosms, or through modeling, with integrated dynamic soil and multi-plant species models (de Vries et al., 2007).
Ideally, we would back-calculate maximum agricultural N volatilization per ecoregion, based on the corresponding critical N loads (de Vries et al., 2007). However, due to methodology being variable across sites (de Vries et al., 2007), lack of data for some biomes (Bobbink et al., 2010), variability in the distance NOx and NH3 gases travel, and potential underestimations of the effects of long-term accumulation of chronic low N inputs (Clark and Tilman, 2008), as well as synergic effects with P (Schleuss et al., 2020), we propose a simplified approach. de Vries et al. (2021) apply a constant precautionary critical load across all ecosystem types in Europe, obtained through modeling (de Vries et al., 2007): a N concentration in soil solutions equal or lower than 3 mg.L−1 for forests and 3.5 mg.L−1 for semi-natural vegetation may prevent changes in plant species communities. Until finer data are available, we propose to use these critical N loads globally, to back-calculate local maximum N volatilizations, and deduct critical N inputs depending on the mix of nutrient sources used.
2.6.1.2 Runoff of N
N is also lost from the farm through the water in the inorganic forms ammonium (NH4+), nitrite (NO2−), and nitrate (NO3−). Through surface runoff and underground leaching, a fraction of the excess reactive N that is not taken up by plants ends up in surface waters of freshwater ecosystems and coastal zones (Bodirsky et al., 2014). The resulting eutrophication and acidification pose a threat to biodiversity.
As for the maximum N volatilization, we propose to back-calculate maximum agricultural N inputs based on critical inorganic N concentrations [N] (dissolved plus particulate N) (de Vries et al., 2007). In the largest global assessment of the toxicological and ecological effects of inorganic nitrogen pollution in aquatic ecosystems, Camargo and Alonso (2006) proposed a critical limit of total [N] in surface waters at 0.5–1.0 mg.L−1, below which eutrophication, acidification, and direct toxicity of nitrogenous compounds may be prevented. In another study reviewing national water quality objectives of rivers around the world (Liu et al., 2012), 2.5 mg.L−1 was identified as the average maximum allowable inorganic [N]: about twice the natural concentrations.
We propose applying a global critical limit of inorganic [N] in freshwater exports of 2.5 mg.L−1, based on Liu et al. (2012). The limit could be 0.5 mg.L−1 for stagnant systems (i.e., lakes, reservoirs), based on Camargo and Alonso (2006). These critical limits can be used to back-calculate local critical N runoffs and N inputs, depending on geographical specificities and the mix of nutrient sources used.
2.6.2 Phosphorus runoff
P is lost to runoff through the erosion of enriched soil and circulates mostly in particulate forms (Bennett et al., 2001; Liu et al., 2012). The availability of P in surface runoff depends on many factors, including the exposure of soils to erosion, topography, the state of P in different soil compartments, and the lability of different types of P. The complexity of the P cycle consequently results in models with different levels of complexity (e.g., Carpenter and Bennett, 2011; Ringeval et al., 2017; Lun et al., 2018), and it poses additional difficulties to back-calculate critical P inputs from identified critical loads compared to N.
Based on Carlson’s index of eutrophication (Carlson, 1977), Carpenter and Bennett (2011) propose a critical [P] of 0.024 mg.L−1 for lakes and reservoirs, as a boundary between mesotrophy and eutrophy. Further, Carpenter and Bennett (2011) estimate a pre-industrial [P] for rivers of 0.16 mg.L−1, calculated using pre-industrial weathering and discharge rates from Bennett et al. (2001). Liu et al. (2012) propose doubling the natural P concentrations in rivers as maximum concentrations, like for [N]. However, they find higher natural [P] (0.51 mg.L−1) than Carpenter and Bennett’s (2011) pre-industrial estimate.
We propose a global critical [P] in freshwater exports corresponding to the double of pre-industrial [P]: 0.32 mg.L−1, based on Carpenter and Bennett’s (2011) estimate. For lakes, reservoirs, and stationary systems, a lower estimate may be used, such as Carpenter and Bennett’s (2011) eutrophication limit at 0.024 mg.L−1. Local critical P runoffs and inputs can be back-calculated for any given source mix and geographic conditions.
2.7 Landscape complexity
The intensification of agricultural practices has led to a simplification of farmed landscapes. Chemical inputs and mechanization have allowed for the increasing size of fields, their specialization, the shortening of crop rotations, and the loss of unfarmed patches within fields and across landscapes (Aguilar et al., 2015; White and Roy, 2015). This homogenization has negatively impacted farmland biodiversity (Benton et al., 2003; Fahrig et al., 2010; Kremen and Miles, 2012), indirectly and negatively affecting the productivity of agroecosystems through the loss of ecosystem services (Dainese et al., 2019), as well as wild species using the agricultural matrix for refuge, feeding, nesting, dispersal, or any other need (Perfecto and Vandermeer, 2010).
Restoring the complexity of farmlands can significantly increase the biodiversity of both ecosystem service providers as well as species of conservation concern, within fields and across the landscape (Kremen and Merenlender, 2018; Estrada-Carmona et al., 2022). Increasing the variety of resources and habitats available across time and space may benefit species requiring multiple resources throughout their life cycles (i.e., landscape complementation), allow species with similar requirements to co-exist (i.e., resource partitioning), and host more specialist species (Fahrig et al., 2010; Sirami et al., 2019). The configuration of fields into a diverse crop mosaic connected through corridors of semi-natural habitats is further expected to facilitate the colonization, movement, dispersal, and persistence of species across the landscape (Perfecto and Vandermeer, 2010), as well as their contribution to farming through services like pollination and pest control (Alignier et al., 2020).
We consider three variables that encompass agricultural landscapes’ spatial and temporal complexity (Estrada-Carmona et al., 2022): landscape composition, configuration, and heterogeneity. Composition and configuration include the extent and spatial arrangement of uncultivated vegetation, agroecological infrastructures (e.g., hedgerows, ditches, ponds, flower strips, trees, and stone walls), and grazed grasslands kept at an animal density equivalent to the ecosystem’s carrying capacity (Bengtsson et al., 2005). Further, landscape heterogeneity includes the diversity of cultivated crops across space, and their rotations over time.
2.7.1 Semi-natural habitats within croplands
More than half of all species found in agricultural landscapes are dependent upon natural or semi-natural habitats, including some important groups like bees and wasps that are almost fully dependent (Duelli and Obrist, 2003; Lüscher et al., 2016). Increasing the area of semi-natural habitat in agricultural landscapes is seen as one of the most effective ways of enhancing their biodiversity and is found to be a good proxy for overall biodiversity in models (e.g., Hietala-Koivu et al., 2004; Montoya et al., 2020).
Semi-natural cover is found to correlate to a multitrophic diversity index accounting for vascular plants, bees, butterflies, hoverflies, carabids, spiders, and birds (Sirami et al., 2019), to wild plants’ alpha, beta, and gamma diversity within fields (Alignier et al., 2020), and to species richness and Simpson’s diversity index of vascular plants, birds, and arthropods (Billeter et al., 2008). The largest meta-analysis conducted to date on the effects of landscape complexity on biodiversity found significantly higher diversity of vertebrates, invertebrates, and plants in farmland with higher compositional and configurational heterogeneity (Estrada-Carmona et al., 2022). However, it is important to note that most of the evidence found comes from field studies located in Europe and North America.
Although large tracts of natural or semi-natural vegetation are usually considered more important for the conservation of sensitive and rare species (Ekroos et al., 2014), smaller patches within fields also host rare and threatened species (Wintle et al., 2018) and are most important at ensuring biodiversity and provisioning of ecosystem services within fields, as most pollinators forage within a range of 1 km from their nests (Rands and Whitney, 2011).
A semi-natural vegetation (SNV) area ≥ 20%.km−2 has been found as the minimum extent needed for the provisioning of nature’s contributions to people, both for pollination and seed dispersal (Garibaldi et al., 2021), and studies on the impacts on other contributions are under way. Other studies find that the positive effects of crop diversification on biodiversity only arise after SNV > 10% and are strongest at SNV up to 20%, as posited by the intermediate landscape complexity hypothesis (Hass et al., 2018; Sirami et al., 2019; Montoya et al., 2020).
We propose to keep at least 20%.km−2 of croplands as semi-natural vegetation, in the form of both uncultivated patches and agroecological infrastructures. For biodiversity to benefit from SNV, it should include different habitat types, at least woody (woodland and hedgerows) and grassy (permanent and temporary grasslands; Duflot et al., 2014), and habitat compositions and structures suitable for native species communities (Thies et al., 2011; Garibaldi et al., 2021).
2.7.2 Crop rotations and cover crops
The seasonal variation in the habitats and resources available is another important dimension for species, which may have changing requirements over time and throughout their life cycles (Benton et al., 2003). Monocropping systems have shortened or suppressed crop rotations, and become spatially uniform at any one time, while complex crop rotations (i.e., longer cycles and more different crop types) create heterogeneity over time.
Crop rotations have been proven to increase soil microbial diversity (Venter et al., 2016) and ground beetle diversity (Gailis et al., 2017), and cover crops significantly improve biological pest and disease control (Beillouin et al., 2021). A meta-synthesis looking at the effects of crop diversification on the wildlife associated to farmland found that crop rotations and cover crops are, on average, associated with 37% [16–62%] and 21% [17–25%] higher biodiversity levels, respectively (Beillouin et al., 2021).
As no level of complexity for crop rotations is identified as optimal for biodiversity, we propose to use organic crop rotations as a minimal target for biodiversity. The average crop rotation for organic systems around the globe is described by Barbieri et al. (2017): rotations last 4.5 ± 1.7 years, include four different crop categories, and are composed of more temporary fodder, pulses, and secondary cereals, and fewer primary cereals, than conventional rotations. Further, we propose planting a mix of cover crops, including legumes, grasses, and forbs, everywhere possible between main crops and in field margins, maintaining soil covered year-round.
It is important to note, however, that the majority of data informing these averages comes from Europe and North America (Barbieri et al., 2017), and this target should be revisited as soon as data from other regions becomes available.
2.7.3 Crop diversity
Many species living in agricultural landscapes depend on farmed fields for foraging and nesting (e.g., Holzschuh et al., 2013; Raymond et al., 2014). Heterogeneity within fields created by crop diversity, including different species, cultivars, and functional traits (Jones et al., 2021), can thus provide resources and facilitate resource partitioning and a greater co-existence of species (Benton et al., 2003).
The number of crop types grown simultaneously within a field has been found to be associated with significantly higher species richness of arthropods, most strongly bees, carabids and bugs (Billeter et al., 2008), and with a higher multitrophic diversity index (Sirami et al., 2019). Further, the density of crop-crop borders may be associated with higher abundance and movement of pollinators within fields (Hass et al., 2018). In their meta-analysis of meta-analyses, Beillouin et al. (2021) found that agroforestry systems host 61% [25–105%] higher biodiversity levels than monocultures. Further, 7% [3–12%] higher biodiversity is found when multiple crops are grown simultaneously in intercropping systems (Beillouin et al., 2021).
It may not be possible to implement intercropping and agroforestry everywhere, because it requires having enough of each crop to interplant in regional crop mixes. Further, agronomic constraints also exist, for instance inundated rice cannot easily be mixed with legumes, as none tolerates inundation. We propose intercropping as many cereal and leguminous crops as possible consistently with regional crop mixes. Perennial crops should be grown in agroforestry systems, and vegetables and other crops should be grown in multi-cropping systems that are integrated with agroforestry systems whenever possible.
2.8 Grassland management
Intensive management of pastures, characterized by the use of fertilizers and high livestock densities, have caused important declines in plant communities, with cascading effects on arthropods and farmland birds (Vickery et al., 2001). Conversely, non-fertilized grasslands and silvopastoral livestock systems, where extensive grazing or mowing are needed to avoid the encroachment of trees, can host high levels of biodiversity [e.g., in boreal (Herzon et al., 2021), temperate (Pärtel et al., 2005), and tropical (Murgueitio et al., 2011) climates].
Studies around the world have found contradictory effects of grazing activity on the abundance and diversity of species, as they depend upon the studied scale, taxonomic group, climate, and grazing intensity (Liang et al., 2021). A global meta-analysis aggregating all grazing regimes together found negative effects of grazing on the abundance of plants and vertebrates, and the diversity of invertebrates (Filazzola et al., 2020). However, when distinguishing between grazing intensities, another meta-analysis found significantly positive effects on plant and soil microbial diversity at low and moderate grazing intensities, followed by a drop at heavy grazing (Wang and Tang, 2019). This bell-shaped curve of diversity along the gradient of grazing intensity is described by the intermediate disturbance hypothesis of classical ecological theory, which posits that diversity should peak at moderate grazing intensities. Not all taxa followed this trend in Wang and Tang’s (2019) results: arthropods consistently declined with grazing, which was explained by the reduced shelter resulting from the decline in plant biomass and ground cover. Finally, another study found positive effects of grazing on arthropods across five European countries, when control of wild or domestic herbivore populations in rewilding systems led to densities equivalent to less than 1.5 times the ecosystems’ carrying capacities (Van Klink and WallisDeVries, 2018).
This evidence suggests that pastures can host high biodiversity levels when management mimics natural grassland conditions. Therefore, we propose that grasslands are not fertilized, and that livestock densities in grassland-based ruminant systems are limited to the ecosystems’ carrying capacities (set to the vegetation’s net primary productivity (NPP), multiplied by a utilization rate of aboveground biomass through grazing, and possibly modified for slope like done by de Leeuw et al. (2019)).
3 Discussion
3.1 The agricultural boundaries framework compared to existing agronomic systems
3.1.1 Beyond organic agriculture
The defined system shares some similarities with organic farming and draws from its experience. For instance, our boundary for crop rotations is based on the functioning of organic systems around the world. However, our framework goes beyond organic agriculture in terms of biodiversity conservation as well as productive capacity.
While our system is based on the multivariate effects of agriculture on biodiversity, organic agriculture is based on the independence from synthetic inputs (which our system tolerates under critical limits for biodiversity). Thus, the framework of organic farming addresses certain biodiversity stressors stemming from agriculture (i.e., nutrient and pesticide pollution, landscape homogenization), but others may persist in these systems (e.g., agricultural expansion, GHG emissions, water withdrawal, livestock overstocking, and lack of fine-scale semi-natural habitats). Therefore, while organic farms tend to host higher biodiversity levels than conventional ones (e.g., Mäder et al., 2002; Benton et al., 2003; Bengtsson et al., 2005; Tuck et al., 2014), using the organic agronomic system to simulate biodiversity-friendly agricultural landscapes (e.g., Hodgson et al., 2010) overlooks important factors and does not explore the full biodiversity potential of agroecosystems. Further, higher crop yields can be obtained through ecological intensification (Gurr et al., 2016; Tamburini et al., 2020), i.e., optimizing yields by maximizing supporting (e.g., soil fertility) and regulating (e.g., pollination, pest control) ecosystem services within fields while minimizing external inputs (Bommarco et al., 2013), as our framework does, with quantified limits and thresholds, and integrating all biodiversity beyond ecosystem service providers.
3.1.2 Operationalizing agroecology for biodiversity conservation
Our framework fits well within the agronomic principles that define agroecology (FAO, 2018; HLPE, 2019). Given our focus on biodiversity conservation across farmlands, our conceptualization mostly draws from the study of agroecology as a science and practice, and leaves out considerations of agroecology as a social movement (Wezel et al., 2020).
There have been efforts to characterize agroecological systems, operationalize these principles and identify levers that can be used to facilitate agroecological transitions. The Characterization of Agroecological Transitions (CAET) (FAO, 2019b) presents a set of criteria that allow us to assess an abstract agricultural system that would be based on our framework on 13 criteria (among the 37 CAET criteria) that we consider to be relevant for agricultural practices. Based on our own analysis, resilience score is unknown, use of pesticides score is average, five criteria get high scores (crop diversity, diversity of perennials, soil–plant management, integration with trees, and connectivity). Five criteria cannot be directly rated but are indirectly taken into account: the three criteria crop-livestock integration, management of fertility, and nutrient recycling are indirectly considered through limits on fertilizer pollution; the criteria on water use efficiency is indirectly in limits on water withdrawal; and the criteria on energy use is, to some extent, related to limits on GHG emissions. Livestock diversity is not considered in our framework and crop-livestock integration is only considered indirectly through its effect on fertilizer pollution, but considerations on grassland management and area used or yield appear only in our framework. Since we are focused on biodiversity, we do not cover all the criteria of the CAET framework, but when rating is possible, we consider our framework to be consistent with the characterization of Agroecology embedded in the CAET criteria.
Further, Côte et al. (2022) presented a list of biotechnical levers, referring to the design of agroecosystems, including “mixing of plants at the plot scale,” “diverse rotations,” “complementarity between trees and annual crops,” “ecological corridors,” and “mosaic organization at the landscape level,” which are all included in our framework. Our study further operationalizes these levers by providing evidence and measurable thresholds for the capacity of these practices to guarantee the successful integration of biodiversity conservation within agricultural systems. While our framework defines clear boundaries for each practice or stressor, it can encompass a wide variety of different, contextualized agroecological systems, as we recognize that these must be adapted to the local conditions and resources, and hybridized with the local knowledge systems (Côte et al., 2022).
3.2 Comparison with other modeling frameworks
3.2.1 A similar approach to other frameworks
A similar framework is proposed for “soil health” by Yang et al. (2020), who review the effects of cropping systems on soil. This framework is also confronted with the difficulty of finding indicators that encompass the multiple dimensions of “soil health” and, instead, focuses on the link with practices, as we do. We go one step beyond, however, by proposing a set of boundaries that describe a farming system that is compatible with the multiple dimensions of biodiversity conservation.
Further, the approach used in this study is also in line with “strong sustainability” approaches of ecological economics. These consist of, in the context of an environmental assessment, expressing different environmental dimensions with different measures (rather than a common metric), in order to avoid conflicts of values and problems of comparison (Martinez-Alier et al., 1998). In particular, the notion of critical natural capital involves defining thresholds to ensure the sustainable maintenance of environmental functions (Ekins et al., 2003).
3.2.2 Limitations of the biosphere integrity planetary boundary for assessing the biodiversity impacts of agriculture
The planetary boundaries (PBs) framework is useful for assessing the broad, multivariate impacts of human activities on Earth systems stability. However, many PBs interact with one another, as is the case for biodiversity (Mace et al., 2014; Lade et al., 2020), and these interactions and cumulative effects on biodiversity are not captured by the PBs framework. A scenario may stay within the biosphere integrity boundary, but at the same time transgress other boundaries that also impact biodiversity (e.g., Foley et al., 2011; Tilman et al., 2011), therefore only partially addressing the collapse of biodiversity. Further, the use of indicators of biosphere integrity like the BII and extinction rate makes this planetary boundary blind to the biodiversity of farmlands (Steffen et al., 2015). We propose that our framework of agricultural boundaries for biodiversity can better account for the multiple stressors and dimensions of biodiversity in the context of agricultural systems (see Figure 2) than the PBs framework.
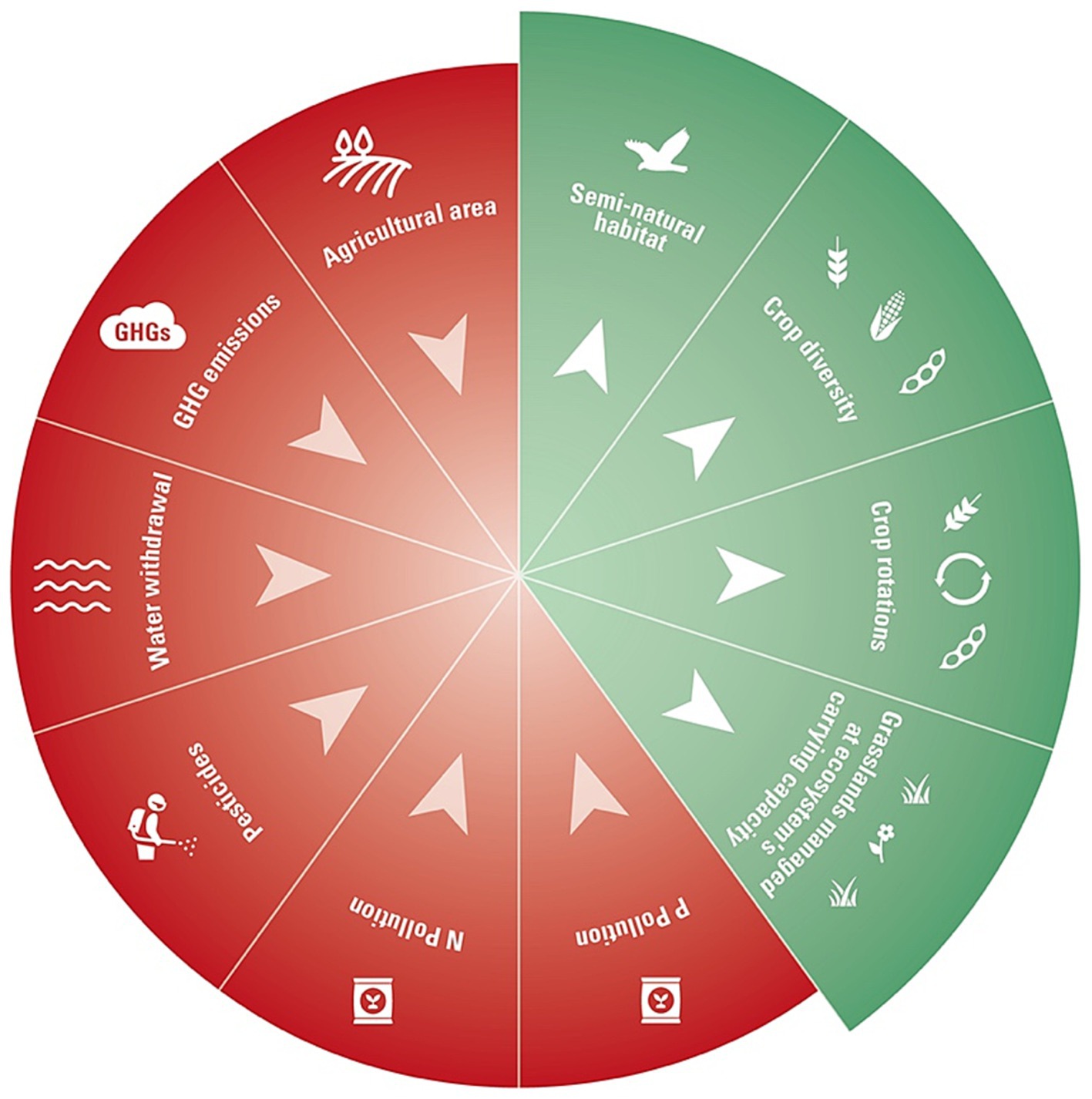
Figure 2. The agricultural boundaries for biodiversity. Red boundaries are pressures that need to be contained under a critical limit, while green boundaries are key determinants of biodiversity across farmlands that need to be maintained at or over a minimal threshold or management regime.
3.3 Limits and gaps of our approach
3.3.1 Elements not accounted for in our framework
3.3.1.1 Soil disturbance
The pressure of soil disturbance on biodiversity was not accounted for in the framework because evidence remains ambiguous in the literature and does not allow to identify a boundary. Tillage can have direct effects on soil biodiversity through mechanical harm to organisms, as well as indirect effects, through the alteration of the soil habitat (e.g., reduced structure and soil organic matter in surface soil) (de Graaff et al., 2019). A meta-analysis found that tillage significantly reduced soil faunal diversity by 22% (de Graaff et al., 2019). Large arthropods like earthworms are known to be most affected by tillage, however, the effects may still vary widely between species, including some that may even benefit (Chan, 2001). De Graaff et al. (2019) also found a moderate reduction in bacterial diversity as a response to tillage, but no effects on soil fungi, which showed variable responses to soil disturbance, ranging from negative to positive effects. Other studies have found no effects of tillage intensity on soil microbial richness, but a significant shift in their community composition, although less important across tillage intensities than across land use types (Frøslev et al., 2022). While we can confidently say that tillage affects overall soil biodiversity, generally negatively, the literature remains ambiguous and does not allow us to identify a clear threshold based on the data of biodiversity responses. Moreover, the use of heavy machinery in farmlands can cause soil compaction, affecting the structure of soil habitats and their biodiversity. However, like for tillage, the literature does not find clear effects of compaction on soil biodiversity and functions, with both positive and negative effects being found across a range of compaction levels, and data from field experiments does not allow to identify threshold values for the conservation of soil biodiversity (Beylich et al., 2010).
Despite the limitations to finding clear boundaries for soil disturbance, because of the intrinsic heterogeneity of soils and their species communities, our framework does take into account the biological component of soils. Other boundaries proposed, including crop diversification, intercropping, rotations, and uncultivated patches within agroecosystems, are all key determinants of “soil health” and, in particular, create a diversity of microhabitats and enhance soil microbial communities and functional complementarity (Yang et al., 2020).
3.3.1.2 Nutrient pollution within fields and on marine coastal areas
Although nutrient addition affects the composition and diversity of weed species communities in fields (Pyšek and Lepš, 1991) and has widely variable effects on soil biodiversity (de Graaff et al., 2019), we did not account for the impacts of nutrient inputs on within-farm biodiversity. We only considered the impacts of nutrient spillovers beyond the fields because available soil space within fields was allocated to crops and cover crops in our framework, which are all managed (i.e., not subject to ecological competition dynamics).
Further, we only control for the effects of nutrient pollution on freshwater biodiversity. The identified critical [N] and [P] limits apply only to freshwater ecosystems, as marine coastal ecosystems respond differently. Although limiting runoff to freshwater should already have a significant effect on coastal waters, this component could be explicitly included in the framework in the future.
3.3.1.3 P deposition
As P does not have a stable gaseous phase in the atmosphere, its emissions and deposition are mainly limited to aerosols (Mahowald et al., 2008). The contribution of agriculture to atmospheric P is low, mainly limited to the burning of biomass like crop residues (Wang et al., 2015). Further, the impacts of P deposition on biodiversity are not mentioned much in the literature. Intentional biomass burning has already been banned across much of the world (Huang et al., 2021), because of its contributions to climate change, hazardous particulate pollution (Adam et al., 2021) and loss of nutrients (N'Dri et al., 2019). Additional contributions of agriculture to atmospheric P could come from fertilizer factories (Mahowald et al., 2008) and from dust emissions from land-use change (Wang et al., 2015), but information is lacking to quantify those sources. These potential P sources would be highly constrained by the boundaries on P runoff, land-use change and cover crops.
3.3.1.4 Fishing and aquaculture
Aquatic systems like fishing and aquaculture were not considered because our focus was on terrestrial biodiversity. For fishing, an area-based conservation boundary could be proposed, like for the agricultural area, at around 30% of marine habitats (O’Leary et al., 2016). However, as in land, fishing exerts multivariate pressures on aquatic biodiversity that should also be accounted for, including particularly destructive fishing practices like bottom trawling (Kaiser et al., 2002), overfishing of keystone species, endangered stocks, or slow-growing organisms (Daskalov, 2002; Morato et al., 2006), and pollution with fishing gear and ghost nets (Richardson et al., 2019). Aquaculture also exerts a multitude of biodiversity pressures that should be accounted for, including nutrient pollution, proliferation of diseases and parasites, the application of antibiotics and hormones, the escape of farmed fish and the consequent genetic alterations of wild populations, or the destruction of multifunctional coastal ecosystems like mangroves (Diana, 2009). Therefore, as we did for the agricultural system, to define a fishing and fish farming system that can ensure the effective conservation of aquatic biodiversity, all key multivariate pressures should be accounted for.
3.4 Potential uses of AG boundaries framework
3.4.1 To assess the potential of biodiversity-friendly agriculture to meet multiple needs
This framework can be implemented in biomass-balance models to assess the “biophysical option space” to feed the world while effectively conserving biodiversity. This will allow to move beyond agricultural scenarios that assess the impacts on biodiversity only partially (such as the studies mobilized in the land-sparing vs. land-sharing debate), to scenarios that tackle the multivariate pressures of agriculture on biodiversity. By simulating an agricultural system constrained by the identified boundaries, at global or other large scales, the biophysical and economic outcomes of transforming agroecosystems to be compatible with global terrestrial biodiversity conservation can be evaluated, which has not been possible up to this date.
The coherence between the identified boundaries and the capacity of the defined system to meet the external constraints put on the food and land systems thus remain to be tested, but we can expect both trade-offs as well as synergies. Since our approach integrates elements from both land sparing and land sharing strategies, some trade-offs are expected, mostly through yield. Critical limits to agricultural area, pesticide and nutrient inputs, and water withdrawal, as well as the expansion of semi-natural habitats within croplands, and natural grasslands’ management, may constrain maximum potential yields and total food production. However, other boundaries may contribute to alleviating the constrained yields, including the significant positive effects on yields of crop diversification (Isbell et al., 2017), particularly intercropping (Ponisio et al., 2015; Li et al., 2020; Beillouin et al., 2021) and cover cropping (Vendig et al., 2023). Yields may be further enhanced by the increased regulating ecosystem services (i.e., pollination and biological pest control) that result from crop diversification and the integration of semi-natural habitats within croplands (Gurr et al., 2016; Dainese et al., 2019; Tamburini et al., 2020; Garibaldi et al., 2021). The implementation of the boundaries defined in our framework would enhance biological pest control of the conservation type (i.e., managing agroecosystems to naturally increase the overall occurrence and efficacy of natural enemies) (Wyckhuys et al., 2013; Redlich et al., 2018), but additional types of pest control such as augmentative (i.e., increasing the population of a natural enemy through direct manipulation for the control of a targeted pest) and importation (i.e., introducing the desired predator of a pest that did not occur naturally) (Bale et al., 2007), as well as input substitution with biopesticides (Ayilara et al., 2023), could further contribute to a pesticide phase down while protecting yields, if done under strictly best practices to avoid ecological and social side-effects (Michaud, 2018). Finally, nutrient limitations resulting from reducing the use of external fertilization may be partially alleviated by increased biological N fixation, achieved by incorporating leguminous plants through crop rotations, intercropping and cover crops (Isbell et al., 2017; Tamburini et al., 2020).
Moreover, even if food production is affected, achieving the availability pillar of food security will depend on additional factors including diets, food waste and loss, and allocation of crops to animal feed and biofuel products (Bodirsky et al., 2014, Desquilbet et al., 2017, Springmann et al., 2018, Barbieri et al., 2022).
An additional external pressure put on the land system is climate mitigation, as an increasing share of land is being claimed for land-based C capture through, for instance, BECCS, afforestation and reforestation (IPCC, 2018), and there is an increasing demand for biomass to substitute fossil-C based energy or material (Searchinger et al., 2022). Our boundary on natural grasslands management, which proposes to maintain pastures grazed by livestock, is a trade-off with climate mitigation compared to other scenarios such as afforestation, and could result in CH4 emissions exceeding those of decarbonization pathways, as well illustrated by the work of Hof et al. (2018). Further, the framework needs to be tested to confirm whether the proposed C budget can be achieved. While practices are not defined based on their contributions to climate mitigation, they can be expected to contribute both positively and negatively. Limits to the use of fertilizers and pesticides would significantly reduce GHG production emissions, and limits to the agricultural area would significantly reduce land-use change GHG emissions, although these limits may be difficult to attain simultaneously for a given production if limited inputs reduce yields. Further, the expansion of semi-natural vegetation (especially woody), ecologically-managed grasslands, and the use of cover crops (Vendig et al., 2023), would increase C storage in soils and vegetation, compared to croplands and intensive pastures, although expansion of semi-natural vegetation could also add pressure on land conversion for production elsewhere, if not followed by changes in demand.
Finally, this framework could be used to explore two additional questions. Firstly, simplified, high-input agricultural systems are extremely vulnerable to climate variability, while diversified systems like the one we have defined are more resilient to perturbations (Lin, 2011). Comparing the yield variability of cropping systems based on our framework to that of high-input agricultural systems would be an interesting perspective. Secondly, at least 80% of the global arable lands are affected by one or several of the five major land degradation processes (increasing aridity, soil erosion, salinization, vegetation decline and the loss of soil organic C), further threatening global food security and biodiversity conservation (Prăvălie et al., 2021). The practices and critical limits proposed to enhance agricultural biodiversity may also be key to creating more resilient and regenerative systems, as described for instance for silvopastoral systems (Murgueitio et al., 2011). The quantitative links of the boundaries to resilience and regeneration could be reviewed, and scenarios based on the proposed framework could be assessed for their effects on climate adaptation and large-scale soil regeneration across the agricultural system, both central questions when exploring the possibility of feeding the world in the coming years.
3.4.2 To apply to farmlands as guidelines for biodiversity-based management
While this framework can be generalized to the globe or other large scales, it would need to be adapted to local contexts for management purposes. For instance, nutrient pollution limits to freshwater ecosystems are relevant for large-scale analysis, but local specificities such as other nutrient limitations, other pollutants, acidity, water column depth, sedimentation, turbidity, or light limitation, should be considered. The framework offers, however, a display of the key practices or environmental factors to consider in order to enhance biodiversity levels across farmlands, and minimize the spillover of pressures beyond their limits. This can be useful for regional agricultural landscape planning in the context of biodiversity conservation actions.
Author contributions
DG-V: Conceptualization, Data curation, Formal Analysis, Funding acquisition, Investigation, Methodology, Project administration, Visualization, Writing – original draft, Writing – review & editing. PD: Conceptualization, Data curation, Formal Analysis, Investigation, Methodology, Supervision, Validation, Writing – review & editing. RP: Methodology, Writing – review & editing, Data curation, Formal Analysis, Investigation. CK: Investigation, Methodology, Writing – review & editing, Validation. P-MA: Supervision, Validation, Writing – review & editing, Conceptualization, Funding acquisition, Methodology, Project administration, Resources, Visualization.
Funding
The author(s) declare financial support was received for the research, authorship, and/or publication of this article. This study has received financial support from the French government in the framework of the program “Investissements d’avenir,” managed by ANR (the French National Research Agency) under the reference ANR-10-LABX-01. Further funding was received in the framework of the TCforBE research project, funded by the European Union’s Horizon Europe research and innovation program under grant agreement no. 101082057. Finally, the main author was supported by a scholarship by the ABIES Doctoral school of AgroParisTech (Université Paris-Saclay) (2021) and a Postgraduate fellowship by Fundación “La Caixa” (2020).
Conflict of interest
The authors declare that the research was conducted in the absence of any commercial or financial relationships that could be construed as a potential conflict of interest.
The author(s) declared that they were an editorial board member of Frontiers, at the time of submission. This had no impact on the peer review process and the final decision.
Publisher’s note
All claims expressed in this article are solely those of the authors and do not necessarily represent those of their affiliated organizations, or those of the publisher, the editors and the reviewers. Any product that may be evaluated in this article, or claim that may be made by its manufacturer, is not guaranteed or endorsed by the publisher.
References
Adam, M. G., Tran, P. T. M., Bolan, N., and Balasubramanian, R. (2021). Biomass burning-derived airborne particulate matter in Southeast Asia: a critical review. J. Hazard. Mater. 407:124760. doi: 10.1016/j.jhazmat.2020.124760
Aguilar, J., Gramig, G. G., Hendrickson, J. R., Archer, D. W., Forcella, F., and Liebig, M. A. (2015). Crop species diversity changes in the United States: 1978–2012. PLoS One 10:e0136580. doi: 10.1371/journal.pone.0136580
Alignier, A., Solé-Senan, X. O., Robleño, I., Baraibar, B., Fahrig, L., Giralt, D., et al. (2020). Configurational crop heterogeneity increases within-field plant diversity. J. Appl. Ecol. 57, 654–663. doi: 10.1111/1365-2664.13585
Alkemade, R., van Oorschot, M., Miles, L., Nellemann, C., Bakkenes, M., and ten Brink, B. (2009). GLOBIO3: a framework to investigate options for reducing global terrestrial biodiversity loss. Ecosystems 12, 374–390. doi: 10.1007/s10021-009-9229-5
Altieri, M. A. (1999). The ecological role of biodiversity in agroecosystems. Agric. Ecosyst. Environ. 74, 19–31. doi: 10.1016/S0167-8809(99)00028-6
Ayilara, M. S., Adeleke, B. S., Akinola, S. A., Fayose, C. A., Adeyemi, U. T., Gbadegesin, L. A., et al. (2023). Biopesticides as a promising alternative to synthetic pesticides: a case for microbial pesticides, phytopesticides, and nanobiopesticides. Front. Microbiol. 14:1040901. doi: 10.3389/fmicb.2023.1040901
Bale, J. S., van Lenteren, J. C., and Bigler, F. (2007). Biological control and sustainable food production. Philos. Trans. Roy. Soc. B. Biol. Sci. 363, 761–776. doi: 10.1098/rstb.2007.2182
Balian, E. V., Segers, H., Lévèque, C., and Martens, K. (2008). The freshwater animal diversity assessment: an overview of the results. Hydrobiologia 595, 627–637. doi: 10.1007/s10750-007-9246-3
Balmford, A., Amano, T., Bartlett, H., Chadwick, D., Collins, A., Edwards, D., et al. (2018). The environmental costs and benefits of high-yield farming. Nat. Sustain. 1, 477–485. doi: 10.1038/s41893-018-0138-5
Barbieri, P., Dumont, B., Benoit, M., and Nesme, T. (2022). Opinion paper: livestock is at the heart of interacting levers to reduce feed-food competition in agroecological food systems. Animal 16:100436. doi: 10.1016/j.animal.2021.100436
Barbieri, P., Pellerin, S., and Nesme, T. (2017). Comparing crop rotations between organic and conventional farming. Sci. Rep. 7:13761. doi: 10.1038/s41598-017-14271-6
Baxter, J., and Cummings, S. P. (2008). The degradation of the herbicide bromoxynil and its impact on bacterial diversity in a top soil. J. Appl. Microbiol. 104, 1605–1616. doi: 10.1111/j.1365-2672.2007.03709.x
Beckmann, M., Gerstner, K., Akin-Fajiye, M., Ceaușu, S., Kambach, S., Kinlock, N. L., et al. (2019). Conventional land-use intensification reduces species richness and increases production: a global meta-analysis. Glob. Chang. Biol. 25, 1941–1956. doi: 10.1111/gcb.14606
Beillouin, D., Ben-Ari, T., Malézieux, E., Seufert, V., and Makowski, D. (2021). Positive but variable effects of crop diversification on biodiversity and ecosystem services. Glob. Chang. Biol. 27, 4697–4710. doi: 10.1111/gcb.15747
Bellard, C., Bertelsmeier, C., Leadley, P., Thuiller, W., and Courchamp, F. (2012). Impacts of climate change on the future of biodiversity. Ecol. Lett. 15, 365–377. doi: 10.1111/j.1461-0248.2011.01736.x
Bengtsson, J., Ahnström, J., and Weibull, A.-C. (2005). The effects of organic agriculture on biodiversity and abundance: a meta-analysis. J. Appl. Ecol. 42, 261–269. doi: 10.1111/j.1365-2664.2005.01005.x
Bennett, E. M., Carpenter, S. R., and Caraco, N. F. (2001). Human impact on Erodable phosphorus and eutrophication: a global perspective: increasing accumulation of phosphorus in soil threatens rivers, lakes, and coastal oceans with eutrophication. Bioscience 51, 227–234. doi: 10.1641/0006-3568(2001)051[0227:HIOEPA]2.0.CO;2
Benton, T. G., Vickery, J. A., and Wilson, J. D. (2003). Farmland biodiversity: is habitat heterogeneity the key? Trends Ecol. Evol. 18, 182–188. doi: 10.1016/S0169-5347(03)00011-9
Beylich, A., Oberholzer, H.-R., Schrader, S., Höper, H., and Wilke, B.-M. (2010). Evaluation of soil compaction effects on soil biota and soil biological processes in soils. Soil Tillage Res. 109, 133–143. doi: 10.1016/j.still.2010.05.010
Biddinger, D. J., Robertson, J. L., Mullin, C., Frazier, J., Ashcraft, S. A., Rajotte, E. G., et al. (2013). Comparative toxicities and synergism of apple orchard pesticides to Apis mellifera (L.) and Osmia cornifrons (Radoszkowski). PLoS One 8:e72587. doi: 10.1371/journal.pone.0072587
Billeter, R., Liira, J., Bailey, D., Bugter, R., Arens, P., Augenstein, I., et al. (2008). Indicators for biodiversity in agricultural landscapes: a pan-European study. J. Appl. Ecol. 45, 141–150. doi: 10.1111/j.1365-2664.2007.01393.x
Birdlife International (2018). State of the world’s birds: Taking the pulse of the planet. BirdLife International, Cambridge, UK.
Boatman, N. D., Brickle, N. W., Hart, J. D., Milsom, T. P., Morris, A. J., Murray, A. W. A., et al. (2004). Evidence for the indirect effects of pesticides on farmland birds. Ibis 146, 131–143. doi: 10.1111/j.1474-919X.2004.00347.x
Bobbink, R., Hicks, K., Galloway, J., Spranger, T., Alkemade, R., Ashmore, M., et al. (2010). Global assessment of nitrogen deposition effects on terrestrial plant diversity: a synthesis. Ecol. Appl. 20, 30–59. doi: 10.1890/08-1140.1
Bodirsky, B. L., Popp, A., Lotze-Campen, H., Dietrich, J. P., Rolinski, S., Weindl, I., et al. (2014). Reactive nitrogen requirements to feed the world in 2050 and potential to mitigate nitrogen pollution. Nat. Commun. 5:3858. doi: 10.1038/ncomms4858
Bommarco, R., Kleijn, D., and Potts, S. G. (2013). Ecological intensification: harnessing ecosystem services for food security. Trends Ecol. Evol. 28, 230–238. doi: 10.1016/j.tree.2012.10.012
Borin, M., Passoni, M., Thiene, M., and Tempesta, T. (2010). Multiple functions of buffer strips in farming areas. Eur. J. Agron. Crop. Syst. Design 32, 103–111. doi: 10.1016/j.eja.2009.05.003
Bratt, A. R., Finlay, J. C., Welter, J. R., Vculek, B. A., and Van Allen, R. E. (2020). Co-limitation by N and P characterizes phytoplankton communities across nutrient availability and land use. Ecosystems 23, 1121–1137. doi: 10.1007/s10021-019-00459-6
Brennan, A., Naidoo, R., Greenstreet, L., Mehrabi, Z., Ramankutty, N., and Kremen, C. (2022). Functional connectivity of the world’s protected areas. Science 376, 1101–1104. doi: 10.1126/science.abl8974
Bribane Declaration (2007). The Brisbane Declaration: Environmental flows are essential for freshwater ecosystem health and human well-being, Declaration of the 10th International River Symposium. Brisbane, Australia.
Bünemann, E. K., Schwenke, G. D., Zwieten, L. V., Bünemann, E. K., Schwenke, G. D., and Zwieten, L. V. (2006). Impact of agricultural inputs on soil organisms—a review. Soil Res. 44, 379–406. doi: 10.1071/SR05125
Cain, M., Lynch, J., Allen, M. R., Fuglestvedt, J. S., Frame, D. J., and Macey, A. H. (2019). Improved calculation of warming-equivalent emissions for short-lived climate pollutants. NPJ Clim. Atmos. Sci. 2, 29–27. doi: 10.1038/s41612-019-0086-4
Camargo, J. A., and Alonso, A. (2006). Ecological and toxicological effects of inorganic nitrogen pollution in aquatic ecosystems: a global assessment. Environ. Int. 32, 831–849. doi: 10.1016/j.envint.2006.05.002
Cardinale, B. J., Duffy, J. E., Gonzalez, A., Hooper, D. U., Perrings, C., Venail, P., et al. (2012). Biodiversity loss and its impact on humanity. Nature 486, 59–67. doi: 10.1038/nature11148
Carlson, R. E. (1977). A trophic state index for lakes1. Limnol. Oceanogr. 22, 361–369. doi: 10.4319/lo.1977.22.2.0361
Carpenter, S. R., and Bennett, E. M. (2011). Reconsideration of the planetary boundary for phosphorus. Environ. Res. Lett. 6:014009. doi: 10.1088/1748-9326/6/1/014009
Ceballos, G., Ehrlich, P. R., Barnosky, A. D., García, A., Pringle, R. M., and Palmer, T. M. (2015). Accelerated modern human–induced species losses: entering the sixth mass extinction. Sci. Adv. 1:e1400253. doi: 10.1126/sciadv.1400253
Chan, K. Y. (2001). An overview of some tillage impacts on earthworm population abundance and diversity — implications for functioning in soils. Soil Tillage Res. 57, 179–191. doi: 10.1016/S0167-1987(00)00173-2
Clark, M. A., Domingo, N. G. G., Colgan, K., Thakrar, S. K., Tilman, D., Lynch, J., et al. (2020). Global food system emissions could preclude achieving the 1.5° and 2°C climate change targets. Science 370, 705–708. doi: 10.1126/science.aba7357
Clark, C. M., and Tilman, D. (2008). Loss of plant species after chronic low-level nitrogen deposition to prairie grasslands. Nature 451, 712–715. doi: 10.1038/nature06503
Côte, F. X., Rapidel, B., Sourisseau, J. M., Affholder, F., Andrieu, N., Bessou, C., et al. (2022). Levers for the agroecological transition of tropical agriculture. Agron. Sustain. Dev. 42:67. doi: 10.1007/s13593-022-00799-z
Dainese, M., Martin, E. A., Aizen, M. A., Albrecht, M., Bartomeus, I., Bommarco, R., et al. (2019). A global synthesis reveals biodiversity-mediated benefits for crop production. Science. Advances 5:eaax0121. doi: 10.1126/sciadv.aax0121
Daskalov, G. M. (2002). Overfishing drives a trophic cascade in the Black Sea. Mar. Ecol. Prog. Ser. 225, 53–63. doi: 10.3354/meps225053
de Graaff, M.-A., Hornslein, N., Throop, H. L., Kardol, P., and van Diepen, L. T. A. (2019). “Chapter one—effects of agricultural intensification on soil biodiversity and implications for ecosystem functioning: a meta-analysis” in Advances in Agronomy. ed. D. L. Sparks (Amsterdam, Netherlands: Academic Press), 1–44.
de Leeuw, J., Rizayeva, A., Namazov, E., Bayramov, E., Marshall, M. T., Etzold, J., et al. (2019). Application of the MODIS MOD 17 net primary production product in grassland carrying capacity assessment. Int. J. Appl. Earth Obs. Geoinf. 78, 66–76. doi: 10.1016/j.jag.2018.09.014
de Vries, W., Kros, H., Reinds, G.J., Wamelink, W., Mol, J., van Dobben, H., et al. (2007). Developments in deriving critical limits and modeling critical loads of nitrogen for terrestrial ecosystems in Europe (No. 1382), Alterra-rapport. Alterra, Wageningen.
de Vries, W., Schulte-Uebbing, L., Kros, H., Voogd, J. C., and Louwagie, G. (2021). Spatially explicit boundaries for agricultural nitrogen inputs in the European Union to meet air and water quality targets. Sci. Total Environ. 786:147283. doi: 10.1016/j.scitotenv.2021.147283
DeClerck, F. A. J., Koziell, I., Benton, T., Garibaldi, L. A., Kremen, C., Maron, M., et al. (2023). “A whole earth approach to nature-positive food: biodiversity and agriculture” in Science and Innovations for Food Systems Transformation. eds. J. von Braun, K. Afsana, L. O. Fresco, and M. H. A. Hassan (Cham: Springer International Publishing), 469–496.
Desquilbet, M., Dorin, B., and Denis, C. (2017). Land sharing vs land sparing to conserve biodiversity: how agricultural markets make the difference. Environ. Model. Assess. 22, 185–200. doi: 10.1007/s10666-016-9531-5
Diana, J. S. (2009). Aquaculture production and biodiversity conservation. Bioscience 59, 27–38. doi: 10.1525/bio.2009.59.1.7
DiBartolomeis, M., Kegley, S., Mineau, P., Radford, R., and Klein, K. (2019). An assessment of acute insecticide toxicity loading (AITL) of chemical pesticides used on agricultural land in the United States. PLoS One 14:e0220029. doi: 10.1371/journal.pone.0220029
Dinerstein, E., Vynne, C., Sala, E., Joshi, A. R., Fernando, S., Lovejoy, T. E., et al. (2019). A global Deal for nature: guiding principles, milestones, and targets. Sci. Adv. 5:eaaw2869. doi: 10.1126/sciadv.aaw2869
Dudley, N., Jonas, H., Nelson, F., Parrish, J., Pyhälä, A., Stolton, S., et al. (2018). The essential role of other effective area-based conservation measures in achieving big bold conservation targets. Glob. Ecol. Conserv. 15:e00424. doi: 10.1016/j.gecco.2018.e00424
Duelli, P., and Obrist, M. K. (2003). Regional biodiversity in an agricultural landscape: the contribution of seminatural habitat islands. Basic Appl. Ecol. 4, 129–138. doi: 10.1078/1439-1791-00140
Duflot, R., Georges, R., Ernoult, A., Aviron, S., and Burel, F. (2014). Landscape heterogeneity as an ecological filter of species traits. Acta Oecol. 56, 19–26. doi: 10.1016/j.actao.2014.01.004
Ekins, P., Simon, S., Deutsch, L., Folke, C., and De Groot, R. (2003). A framework for the practical application of the concepts of critical natural capital and strong sustainability. Ecol. Econ. Identify. Crit. Nat. Capital 44, 165–185. doi: 10.1016/S0921-8009(02)00272-0
Ekroos, J., Olsson, O., Rundlöf, M., Watzold, F., and Smith, H. (2014). Optimizing Agri-environment schemes for biodiversity, ecosystem services or both? Biol. Conserv. 172, 65–71. doi: 10.1016/j.biocon.2014.02.013
Ellis, E. C., Klein Goldewijk, K., Siebert, S., Lightman, D., and Ramankutty, N. (2010). Anthropogenic transformation of the biomes, 1700 to 2000. Glob. Ecol. Biogeogr. 19, 589–606. doi: 10.1111/j.1466-8238.2010.00540.x
Elser, J. J., Bracken, M. E. S., Cleland, E. E., Gruner, D. S., Harpole, W. S., Hillebrand, H., et al. (2007). Global analysis of nitrogen and phosphorus limitation of primary producers in freshwater, marine and terrestrial ecosystems. Ecol. Lett. 10, 1135–1142. doi: 10.1111/j.1461-0248.2007.01113.x
Estrada-Carmona, N., Sánchez, A. C., Remans, R., and Jones, S. K. (2022). Complex agricultural landscapes host more biodiversity than simple ones: a global meta-analysis. Proc. Natl. Acad. Sci. 119:e2203385119. doi: 10.1073/pnas.2203385119
Fahrig, L. (2017). Ecological responses to habitat fragmentation per se. Annu. Rev. Ecol. Evol. Syst. 48, 1–23. doi: 10.1146/annurev-ecolsys-110316-022612
Fahrig, L., Arroyo-Rodríguez, V., Bennett, J. R., Boucher-Lalonde, V., Cazetta, E., Currie, D. J., et al. (2019). Is habitat fragmentation bad for biodiversity? Biol. Conserv. 230, 179–186. doi: 10.1016/j.biocon.2018.12.026
Fahrig, L., Baudry, J., Brotons, L., Burel, F. G., Crist, T. O., Fuller, R. J., et al. (2010). Functional landscape heterogeneity and animal biodiversity in agricultural landscapes. Ecol. Lett. 14, 101–112. doi: 10.1111/j.1461-0248.2010.01559.x
FAO (2018). The 10 elements of agroecology: guiding the transition to sustainable food and agricultural systems. Food and Agriculture Organization of the United Nations.
FAO (2019a). The state of the World’s biodiversity for food and agriculture. FAO commission on genetic resources for food and agriculture assessments. eds. J. Bélanger & D. Pilling Rome: FAO.
FAO (2019b). Tool for agroecology performance evaluation (TAPE)—test version: Process of development and guidelines for application. Rome, Italy, FAO.
Feigs, J. T., Holzhauer, S. I., Huang, S., Brunet, J., Diekmann, M., Hedwall, P.-O., et al. (2022). Pollinator movement activity influences genetic diversity and differentiation of spatially isolated populations of clonal forest herbs. Front. Ecol. Evol. 10:908258. doi: 10.3389/fevo.2022.908258
Filazzola, A., Brown, C., Dettlaff, M. A., Batbaatar, A., Grenke, J., Bao, T., et al. (2020). The effects of livestock grazing on biodiversity are multi-trophic: a meta-analysis. Ecol. Lett. 23, 1298–1309. doi: 10.1111/ele.13527
Foden, W. B., Butchart, S. H. M., Stuart, S. N., Vié, J.-C., Akçakaya, H. R., Angulo, A., et al. (2013). Identifying the World’s Most climate change vulnerable species: a systematic trait-based assessment of all birds, amphibians and corals. PLoS One 8:e65427. doi: 10.1371/journal.pone.0065427
Folberth, C., Khabarov, N., Balkovič, J., Skalský, R., Visconti, P., Ciais, P., et al. (2020). The global cropland-sparing potential of high-yield farming. Nat. Sustain. 3, 281–289. doi: 10.1038/s41893-020-0505-x
Foley, J. A., Ramankutty, N., Brauman, K. A., Cassidy, E. S., Gerber, J. S., Johnston, M., et al. (2011). Solutions for a cultivated planet. Nature 478, 337–342. doi: 10.1038/nature10452
Frøslev, T. G., Nielsen, I. B., Santos, S. S., Barnes, C. J., Bruun, H. H., and Ejrnæs, R. (2022). The biodiversity effect of reduced tillage on soil microbiota. Ambio 51, 1022–1033. doi: 10.1007/s13280-021-01611-0
Gailis, J., Turka, I., and Ausmane, M. (2017). Soil tillage and crop rotation differently affect biodiversity and species assemblage of ground beetles inhabiting winter wheat fields. Agron. Res. 15, 94–111.
García-Vega, D., and Newbold, T. (2020). Assessing the effects of land use on biodiversity in the world’s drylands and Mediterranean environments. Biodivers. Conserv. 29, 393–408. doi: 10.1007/s10531-019-01888-4
Garibaldi, L. A., Oddi, F. J., Miguez, F. E., Bartomeus, I., Orr, M. C., Jobbágy, E. G., et al. (2021). Working landscapes need at least 20% native habitat. Conserv. Lett. 14:e12773. doi: 10.1111/conl.12773
Geiger, F., Bengtsson, J., Berendse, F., Weisser, W. W., Emmerson, M., Morales, M. B., et al. (2010). Persistent negative effects of pesticides on biodiversity and biological control potential on European farmland. Basic Appl. Ecol. 11, 97–105. doi: 10.1016/j.baae.2009.12.001
Gerten, D., Heck, V., Jägermeyr, J., Bodirsky, B. L., Fetzer, I., Jalava, M., et al. (2020). Feeding ten billion people is possible within four terrestrial planetary boundaries. Nat. Sustain. 3, 200–208. doi: 10.1038/s41893-019-0465-1
Gibbons, D., Morrissey, C., and Mineau, P. (2015). A review of the direct and indirect effects of neonicotinoids and fipronil on vertebrate wildlife. Environ. Sci. Pollut. Res. 22, 103–118. doi: 10.1007/s11356-014-3180-5
Gibson, L., Lynam, A. J., Bradshaw, C. J. A., He, F., Bickford, D. P., Woodruff, D. S., et al. (2013). Near-complete extinction of native small mammal fauna 25 years after forest fragmentation. Science 341, 1508–1510. doi: 10.1126/science.1240495
Giovannetti, M., Turrini, A., Strani, P., Sbrana, C., Avio, L., and Pietrangeli, P. (2021). Mycorrhizal fungi in ecotoxicological studies: soil impact of fungicides, insecticides and herbicides. Prevention Today 2, 1–2.
Gómez-Fernández, A., Alcocer, I., and Matesanz, S. (2016). Does higher connectivity lead to higher genetic diversity? Effects of habitat fragmentation on genetic variation and population structure in a gypsophile. Conserv. Genet. 17, 631–641. doi: 10.1007/s10592-016-0811-z
Gray, C. L., Hill, S. L. L., Newbold, T., Hudson, L. N., Börger, L., Contu, S., et al. (2016). Local biodiversity is higher inside than outside terrestrial protected areas worldwide. Nat. Commun. 7:12306. doi: 10.1038/ncomms12306
Green, R. E., Cornell, S. J., Scharlemann, J. P. W., and Balmford, A. (2005). Farming and the fate of wild nature. Science 307, 550–555. doi: 10.1126/science.1106049
Gurr, G. M., Lu, Z., Zheng, X., Xu, H., Zhu, P., Chen, G., et al. (2016). Multi-country evidence that crop diversification promotes ecological intensification of agriculture. Nat. Plants 2:16014. doi: 10.1038/nplants.2016.14
Haddad, N. M., Brudvig, L. A., Clobert, J., Davies, K. F., Gonzalez, A., Holt, R. D., et al. (2015). Habitat fragmentation and its lasting impact on Earth’s ecosystems. Sci. Adv. 1:e1500052. doi: 10.1126/sciadv.1500052
Halada, L., Evans, D., Romão, C., and Petersen, J.-E. (2011). Which habitats of European importance depend on agricultural practices? Biodivers. Conserv. 20, 2365–2378. doi: 10.1007/s10531-011-9989-z
Halley, J. M., and Iwasa, Y. (2011). Neutral theory as a predictor of avifaunal extinctions after habitat loss. Proc. Natl. Acad. Sci. 108, 2316–2321. doi: 10.1073/pnas.1011217108
Halley, J. M., Monokrousos, N., Mazaris, A. D., Newmark, W. D., and Vokou, D. (2016). Dynamics of extinction debt across five taxonomic groups. Nat. Commun. 7:12283. doi: 10.1038/ncomms12283
Hallmann, C. A., Sorg, M., Jongejans, E., Siepel, H., Hofland, N., Schwan, H., et al. (2017). More than 75 percent decline over 27 years in total flying insect biomass in protected areas. PLoS One 12:e0185809. doi: 10.1371/journal.pone.0185809
Hardaker, A., Styles, D., Williams, P., Chadwick, D., and Dandy, N. (2022). A framework for integrating ecosystem services as endpoint impacts in life cycle assessment. J. Clean. Prod. 370:133450. doi: 10.1016/j.jclepro.2022.133450
Harpole, W. S., and Tilman, D. (2007). Grassland species loss resulting from reduced niche dimension. Nature 446, 791–793. doi: 10.1038/nature05684
Hart, D. D., and Finelli, C. M. (1999). Physical-biological coupling in streams: the pervasive effects of flow on benthic organisms. Annu. Rev. Ecol. Syst. 30, 363–395. doi: 10.1146/annurev.ecolsys.30.1.363
Hass, A. L., Kormann, U. G., Tscharntke, T., Clough, Y., Baillod, A. B., Sirami, C., et al. (2018). Landscape configurational heterogeneity by small-scale agriculture, not crop diversity, maintains pollinators and plant reproduction in western Europe. Proc. R. Soc. B Biol. Sci. 285:20172242. doi: 10.1098/rspb.2017.2242
Hawes, C., Haughton, A. J., Osborne, J. L., Roy, D. B., Clark, S. J., Perry, J. N., et al. (2003). Responses of plants and invertebrate trophic groups to contrasting herbicide regimes in the farm scale evaluations of genetically modified herbicide-tolerant crops. Philos. Trans. R. Soc. Lond. Ser. B Biol. Sci. 358, 1899–1913. doi: 10.1098/rstb.2003.1406
Herzon, I., Raatikainen, K., Wehn, S., Rūsiņa, S., Helm, A., Cousins, S., et al. (2021). Semi-natural habitats in boreal Europe: a rise of a social-ecological research agenda. Ecol. Soc. 26:13. doi: 10.5751/ES-12313-260213
Hietala-Koivu, R., Järvenpää, T., and Helenius, J. (2004). Value of semi-natural areas as biodiversity indicators in agricultural landscapes. Agric. Ecosyst. Environ. 101, 9–19. doi: 10.1016/S0167-8809(03)00273-1
HLPE (2019). Agroecological and other innovative approaches for sustainable agriculture and food systems that enhance food security and nutrition (No. 14), HLPE Report. High Level Panel of Experts of the Committee on World Food Security.
Hodgson, J. A., Kunin, W. E., Thomas, C. D., Benton, T. G., and Gabriel, D. (2010). Comparing organic farming and land sparing: optimizing yield and butterfly populations at a landscape scale: organic farming and land sparing. Ecol. Lett. 13, 1358–1367. doi: 10.1111/j.1461-0248.2010.01528.x
Hof, C., Voskamp, A., Biber, M. F., Böhning-Gaese, K., Engelhardt, E. K., Niamir, A., et al. (2018). Bioenergy cropland expansion may offset positive effects of climate change mitigation for global vertebrate diversity. Proc. Natl. Acad. Sci. USA 115, 13294–13299. doi: 10.1073/pnas.1807745115
Holzschuh, A., Dormann, C. F., Tscharntke, T., and Steffan-Dewenter, I. (2013). Mass-flowering crops enhance wild bee abundance. Oecologia 172, 477–484. doi: 10.1007/s00442-012-2515-5
Honnay, O., Jacquemyn, H., Bossuyt, B., and Hermy, M. (2005). Forest fragmentation effects on patch occupancy and population viability of herbaceous plant species. New Phytol. 166, 723–736. doi: 10.1111/j.1469-8137.2005.01352.x
Huang, L., Zhu, Y., Wang, Q., Zhu, A., Liu, Z., Wang, Y., et al. (2021). Assessment of the effects of straw burning bans in China: emissions, air quality, and health impacts. Sci. Total Environ. 789:147935. doi: 10.1016/j.scitotenv.2021.147935
Huppmann, D., Kriegler, E., Krey, V., Riahi, K., Rogelj, J., Calvin, K., et al. (2019). IAMC 1.5°C scenario explorer and data hosted by IIASA. Integrated Assessment Modeling Consortium & International Institute for Applied Systems Analysis.
Hylander, K., and Ehrlén, J. (2013). The mechanisms causing extinction debts. Trends Ecol. Evol. 28, 341–346. doi: 10.1016/j.tree.2013.01.010
Immovili, M, and Kok, M. (2020). Narratives for the ‘half earth’ and ‘sharing the planet’ scenarios: a literature review. URL The Hague, the Netherlands: PBL Netherlands Environmental Assessment Agency. (Accessed July 6, 2023).
IPBES (2019). Global assessment report on biodiversity and ecosystem services of the intergovernmental science-policy platform on biodiversity and ecosystem services. IPBES.
IPCC (2018). Global warming of 1.5°C. An IPCC special report on the impacts of global warming of 1.5°C above pre-industrial levels and related global greenhouse gas emission pathways, in the context of strengthening the global response to the threat of climate change, sustainable development, and efforts to eradicate poverty.
IPCC (2019). Climate change and land: An IPCC special report on climate change, desertification, land degradation, sustainable land management, food security, and greenhouse gas fluxes in terrestrial ecosystems.
Isbell, F., Adler, P. R., Eisenhauer, N., Fornara, D., Kimmel, K., Kremen, C., et al. (2017). Benefits of increasing plant diversity in sustainable agroecosystems. J. Ecol. 105, 871–879. doi: 10.1111/1365-2745.12789
IUCN (2019). Recognising and reporting other effective area-based conservation measures. Gland, Switzerland: IUCN, International Union for Conservation of Nature.
Jägermeyr, J., Pastor, A., Biemans, H., and Gerten, D. (2017). Reconciling irrigated food production with environmental flows for sustainable development goals implementation. Nat. Commun. 8:15900. doi: 10.1038/ncomms15900
Jones, S. K., Estrada-Carmona, N., Juventia, S. D., Dulloo, M. E., Laporte, M.-A., Villani, C., et al. (2021). Agrobiodiversity index scores show agrobiodiversity is underutilized in National Food Systems. Nat. Food 2, 712–723. doi: 10.1038/s43016-021-00344-3
Jung, M., Arnell, A., de Lamo, X., García-Rangel, S., Lewis, M., Mark, J., et al. (2021). Areas of global importance for conserving terrestrial biodiversity, carbon and water. Nat. Ecol. Evol. 5, 1499–1509. doi: 10.1038/s41559-021-01528-7
Kaiser, M. J., Collie, J. S., Hall, S. J., Jennings, S., and Poiner, I. R. (2002). Modification of marine habitats by trawling activities: prognosis and solutions. Fish Fish. 3, 114–136. doi: 10.1046/j.1467-2979.2002.00079.x
Kerr, J. T., Pindar, A., Galpern, P., Packer, L., Potts, S. G., Roberts, S. M., et al. (2015). Climate change impacts on bumblebees converge across continents. Science 349, 177–180. doi: 10.1126/science.aaa7031
Klein, A.-M., Vaissière, B. E., Cane, J. H., Steffan-Dewenter, I., Cunningham, S. A., Kremen, C., et al. (2006). Importance of pollinators in changing landscapes for world crops. Proc. R. Soc. B Biol. Sci. 274, 303–313. doi: 10.1098/rspb.2006.3721
Köhler, H.-R., and Triebskorn, R. (2013). Wildlife ecotoxicology of pesticides: can we track effects to the population level and beyond? Science 341, 759–765. doi: 10.1126/science.1237591
Kremen, C. (2015). Reframing the land-sparing/land-sharing debate for biodiversity conservation. Ann. N. Y. Acad. Sci. 1355, 52–76. doi: 10.1111/nyas.12845
Kremen, C., and Merenlender, A. M. (2018). Landscapes that work for biodiversity and people. Science 362:eaau6020. doi: 10.1126/science.aau6020
Kremen, C., and Miles, A. (2012). Ecosystem Services in Biologically Diversified versus conventional farming systems: benefits, externalities, and trade-offs. Ecol. Soc. 17:40. doi: 10.5751/ES-05035-170440
Lade, S. J., Steffen, W., de Vries, W., Carpenter, S. R., Donges, J. F., Gerten, D., et al. (2020). Human impacts on planetary boundaries amplified by earth system interactions. Nat. Sustain. 3, 119–128. doi: 10.1038/s41893-019-0454-4
Laurance, W. F., Camargo, J. L. C., Luizão, R. C. C., Laurance, S. G., Pimm, S. L., Bruna, E. M., et al. (2011). The fate of Amazonian forest fragments: a 32-year investigation. Biol. Conserv. 144, 56–67. doi: 10.1016/j.biocon.2010.09.021
Leclère, D., Obersteiner, M., Barrett, M., Butchart, S. H. M., Chaudhary, A., De Palma, A., et al. (2020). Bending the curve of terrestrial biodiversity needs an integrated strategy. Nature 585, 551–556. doi: 10.1038/s41586-020-2705-y
Li, C., Hoffland, E., Kuyper, T. W., Yu, Y., Zhang, C., Li, H., et al. (2020). Syndromes of production in intercropping impact yield gains. Nat. Plants 6, 653–660. doi: 10.1038/s41477-020-0680-9
Liang, M., Liang, C., Hautier, Y., Wilcox, K. R., and Wang, S. (2021). Grazing-induced biodiversity loss impairs grassland ecosystem stability at multiple scales. Ecol. Lett. 24, 2054–2064. doi: 10.1111/ele.13826
Lin, B. B. (2011). Resilience in agriculture through crop diversification: adaptive Management for Environmental Change. Bioscience 61, 183–193. doi: 10.1525/bio.2011.61.3.4
Liu, C., Kroeze, C., Hoekstra, A. Y., and Gerbens-Leenes, W. (2012). Past and future trends in grey water footprints of anthropogenic nitrogen and phosphorus inputs to major world rivers. Ecol. Indic. 18, 42–49. doi: 10.1016/j.ecolind.2011.10.005
Lode, O., Eklo, O. M., Holen, B., Svensen, A., and Johnsen, A. M. (1995). Pesticides in precipitation in Norway. Sci. Total Environ. Ecol. Effects Arct. Airborne Contam. 160-161, 421–431. doi: 10.1016/0048-9697(95)04375-B
Lomba, A., Alves, P., Jongman, R. H. G., and McCracken, D. I. (2015). Reconciling nature conservation and traditional farming practices: a spatially explicit framework to assess the extent of high nature value farmlands in the European countryside. Ecol. Evol. 5, 1031–1044. doi: 10.1002/ece3.1415
Lucas, P., Karamanlidis, J., De Barba, M., Selva, N., and Thuiller, W. (2018). Direct and indirect impacts of climate change and land use change over biodiversity: A case of study with the brown bear in Europe.
Lun, F., Liu, J., Ciais, P., Nesme, T., Chang, J., Wang, R., et al. (2018). Global and regional phosphorus budgets in agricultural systems and their implications for phosphorus-use efficiency. Earth Syst. Sci. Data 10, 1–18. doi: 10.5194/essd-10-1-2018
Lüscher, G., Ammari, Y., Andriets, A., Angelova, S., Arndorfer, M., Bailey, D., et al. (2016). Farmland biodiversity and agricultural management on 237 farms in 13 European and two African regions. Ecology 97:1625. doi: 10.1890/15-1985.1
Mace, G. M., Reyers, B., Alkemade, R., Biggs, R., Chapin, F. S., Cornell, S. E., et al. (2014). Approaches to defining a planetary boundary for biodiversity. Glob. Environ. Chang. 28, 289–297. doi: 10.1016/j.gloenvcha.2014.07.009
Mäder, P., Fliessbach, A., Dubois, D., Gunst, L., Fried, P., and Niggli, U. (2002). Soil fertility and biodiversity in organic farming. Science 296, 1694–1697. doi: 10.1126/science.1071148
Mahowald, N., Jickells, T. D., Baker, A. R., Artaxo, P., Benitez-Nelson, C. R., Bergametti, G., et al. (2008). Global distribution of atmospheric phosphorus sources, concentrations and deposition rates, and anthropogenic impacts: global atmospheric phosphorus. Glob. Biogeochem. Cycles 22, 1–19. doi: 10.1029/2008GB003240
Mair, L., Bennun, L. A., Brooks, T. M., Butchart, S. H. M., Bolam, F. C., Burgess, N. D., et al. (2021). A metric for spatially explicit contributions to science-based species targets. Nat. Ecol. Evol. 5, 836–844. doi: 10.1038/s41559-021-01432-0
Martinez-Alier, J., Munda, G., and O’Neill, J. (1998). Weak comparability of values as a foundation for ecological economics. Ecol. Econ. 26, 277–286. doi: 10.1016/S0921-8009(97)00120-1
Mendenhall, C. D., Karp, D. S., Meyer, C. F. J., Hadly, E. A., and Daily, G. C. (2014). Predicting biodiversity change and averting collapse in agricultural landscapes. Nature 509, 213–217. doi: 10.1038/nature13139
Meza-Elizalde, M. C., and Armenteras-Pascual, D. (2021). Edge influence on the microclimate and vegetation of fragments of a north Amazonian forest. For. Ecol. Manag. 498:119546. doi: 10.1016/j.foreco.2021.119546
Michaud, J. P. (2018). Problems inherent to augmentation of natural enemies in open agriculture. Neotrop. Entomol. 47, 161–170. doi: 10.1007/s13744-018-0589-4
Miyake, S., Renouf, M., Peterson, A., McAlpine, C., and Smith, C. (2012). Land-use and environmental pressures resulting from current and future bioenergy crop expansion: a review. J. Rural Stud. Grow. Old Rural Places 28, 650–658. doi: 10.1016/j.jrurstud.2012.09.002
Montoya, D., Gaba, S., de Mazancourt, C., Bretagnolle, V., and Loreau, M. (2020). Reconciling biodiversity conservation, food production and farmers’ demand in agricultural landscapes. Ecol. Model. 416:108889. doi: 10.1016/j.ecolmodel.2019.108889
Morato, T., Watson, R., Pitcher, T. J., and Pauly, D. (2006). Fishing down the deep. Fish Fish. 7, 24–34. doi: 10.1111/j.1467-2979.2006.00205.x
Morée, A. L., Beusen, A. H. W., Bouwman, A. F., and Willems, W. J. (2013). Exploring global nitrogen and phosphorus flows in urban wastes during the twentieth century. Glob. Biogeochem. Cycles 27, 836–846. doi: 10.1002/gbc.20072
Murgueitio, E., Calle, Z., Uribe, F., Calle, A., and Solorio, B. (2011). Native trees and shrubs for the productive rehabilitation of tropical cattle ranching lands. For. Ecol. Manag. Ecol. Ecosyst. Serv. Nat. Trees 261, 1654–1663. doi: 10.1016/j.foreco.2010.09.027
N'Dri, A. B., Kone, A. W., Loukou, S. K. K., Barot, S., and Gignoux, J. (2019). Carbon and nutrient losses through biomass burning, and links with soil fertility and yam (dioscorea Alata) production. Exp. Agric. 55, 738–751. doi: 10.1017/S0014479718000327
Newbold, T. (2018). Future effects of climate and land-use change on terrestrial vertebrate community diversity under different scenarios. Proc. R. Soc. B 285:20180792. doi: 10.1098/rspb.2018.0792
Newbold, T., Hudson, L. N., Arnell, A. P., Contu, S., De Palma, A., Ferrier, S., et al. (2016). Has land use pushed terrestrial biodiversity beyond the planetary boundary? A global assessment. Science 353, 288–291. doi: 10.1126/science.aaf2201
Newbold, T., Hudson, L. N., Hill, S. L. L., Contu, S., Lysenko, I., Senior, R. A., et al. (2015). Global effects of land use on local terrestrial biodiversity. Nature 520, 45–50. doi: 10.1038/nature14324
Noss, R. F., Dobson, A. P., Baldwin, R., Beier, P., Davis, C. R., Dellasala, D. A., et al. (2012). Bolder thinking for conservation. Conserv. Biol. 26, 1–4. doi: 10.1111/j.1523-1739.2011.01738.x
O’Leary, B. C., Winther-Janson, M., Bainbridge, J. M., Aitken, J., Hawkins, J. P., and Roberts, C. M. (2016). Effective coverage targets for ocean protection. Conserv. Lett. 9, 398–404. doi: 10.1111/conl.12247
Pärtel, M., Bruun, H., and Sammul, M. (2005). Biodiversity in temperate European grasslands: origin and conservation. in Grassland Science in Europe 10.
Pastor, A. V., Ludwig, F., Biemans, H., Hoff, H., and Kabat, P. (2014). Accounting for environmental flow requirements in global water assessments. Hydrol. Earth Syst. Sci. 18, 5041–5059. doi: 10.5194/hess-18-5041-2014
Pendrill, F., Gardner, T. A., Meyfroidt, P., Persson, U. M., Adams, J., Azevedo, T., et al. (2022). Disentangling the numbers behind agriculture-driven tropical deforestation. Science 377:eabm9267. doi: 10.1126/science.abm9267
Peñuelas, J., Janssens, I. A., Ciais, P., Obersteiner, M., and Sardans, J. (2020). Anthropogenic global shifts in biospheric N and P concentrations and ratios and their impacts on biodiversity, ecosystem productivity, food security, and human health. Glob. Chang. Biol. 26, 1962–1985. doi: 10.1111/gcb.14981
Peñuelas, J., Poulter, B., Sardans, J., Ciais, P., van der Velde, M., Bopp, L., et al. (2013). Human-induced nitrogen–phosphorus imbalances alter natural and managed ecosystems across the globe. Nat. Commun. 4:2934. doi: 10.1038/ncomms3934
Perfecto, I., and Vandermeer, J. (2010). The agroecological matrix as alternative to the land-sparing/agriculture intensification model. Proc. Natl. Acad. Sci. 107, 5786–5791. doi: 10.1073/pnas.0905455107
Phalan, B. T. (2018). What have we learned from the land sparing-sharing model? Sustain. For. 10:1760. doi: 10.3390/su10061760
Phoenix, G. K., Hicks, W. K., Cinderby, S., Kuylenstierna, J. C. I., Stock, W. D., Dentener, F. J., et al. (2006). Atmospheric nitrogen deposition in world biodiversity hotspots: the need for a greater global perspective in assessing N deposition impacts. Glob. Chang. Biol. 12, 470–476. doi: 10.1111/j.1365-2486.2006.01104.x
Pisa, L. W., Amaral-Rogers, V., Belzunces, L. P., Bonmatin, J. M., Downs, C. A., Goulson, D., et al. (2015). Effects of neonicotinoids and fipronil on non-target invertebrates. Environ. Sci. Pollut. Res. Int. 22, 68–102. doi: 10.1007/s11356-014-3471-x
Pisa, L., Goulson, D., Yang, E.-C., Gibbons, D., Sánchez-Bayo, F., Mitchell, E., et al. (2021). An update of the worldwide integrated assessment (WIA) on systemic insecticides. Part 2: impacts on organisms and ecosystems. Environ. Sci. Pollut. Res. 28, 11749–11797. doi: 10.1007/s11356-017-0341-3
Poff, N. L., and Zimmerman, J. K. H. (2010). Ecological responses to altered flow regimes: a literature review to inform the science and management of environmental flows. Freshw. Biol. 55, 194–205. doi: 10.1111/j.1365-2427.2009.02272.x
Ponisio, L. C., M’Gonigle, L. K., Mace, K. C., Palomino, J., de Valpine, P., and Kremen, C. (2015). Diversification practices reduce organic to conventional yield gap. Proc. R. Soc. B Biol. Sci. 282:20141396. doi: 10.1098/rspb.2014.1396
Poore, J., and Nemecek, T. (2018). Reducing food’s environmental impacts through producers and consumers. Science 360, 987–992. doi: 10.1126/science.aaq0216
Popp, A., Calvin, K., Fujimori, S., Havlik, P., Humpenöder, F., Stehfest, E., et al. (2017). Land-use futures in the shared socio-economic pathways. Glob. Environ. Chang. 42, 331–345. doi: 10.1016/j.gloenvcha.2016.10.002
Pörtner, H.-O., Scholes, R.J., Agard, J., Archer, E., Bai, X., Barnes, D., et al. (2021). IPBES-IPCC co-sponsored workshop report on biodiversity and climate change. Zenodo.
Potts, S. G., Biesmeijer, J. C., Kremen, C., Neumann, P., Schweiger, O., and Kunin, W. E. (2010). Global pollinator declines: trends, impacts and drivers. Trends Ecol. Evol. 25, 345–353. doi: 10.1016/j.tree.2010.01.007
Prăvălie, R., Patriche, C., Borrelli, P., Panagos, P., Roșca, B., Dumitraşcu, M., et al. (2021). Arable lands under the pressure of multiple land degradation processes. A global perspective. Environ. Res. 194:110697. doi: 10.1016/j.envres.2020.110697
Pretty, J., and Bharucha, Z. P. (2015). Integrated Pest Management for Sustainable Intensification of agriculture in Asia and Africa. Insects 6, 152–182. doi: 10.3390/insects6010152
Pyšek, P., and Lepš, J. (1991). Response of a weed community to nitrogen fertilization: a multivariate analysis. J. Veg. Sci. 2, 237–244. doi: 10.2307/3235956
Rands, S. A., and Whitney, H. M. (2011). Field margins, foraging distances and their impacts on nesting pollinator success. PLoS One 6:e25971. doi: 10.1371/journal.pone.0025971
Ray, D. K., Ramankutty, N., Mueller, N. D., West, P. C., and Foley, J. A. (2012). Recent patterns of crop yield growth and stagnation. Nat. Commun. 3:1293. doi: 10.1038/ncomms2296
Ray, D. K., West, P. C., Clark, M., Gerber, J. S., Prishchepov, A. V., and Chatterjee, S. (2019). Climate change has likely already affected global food production. PLoS One 14:e0217148. doi: 10.1371/journal.pone.0217148
Raymond, L., Sarthou, J.-P., Plantegenest, M., Gauffre, B., Ladet, S., and Vialatte, A. (2014). Immature hoverflies overwinter in cultivated fields and may significantly control aphid populations in autumn. Agric. Ecosyst. Environ. 185, 99–105. doi: 10.1016/j.agee.2013.12.019
Redlich, S., Martin, E. A., and Steffan-Dewenter, I. (2018). Landscape-level crop diversity benefits biological pest control. J. Appl. Ecol. 55, 2419–2428. doi: 10.1111/1365-2664.13126
Richardson, K., Hardesty, B. D., and Wilcox, C. (2019). Estimates of fishing gear loss rates at a global scale: a literature review and meta-analysis. Fish Fish. 20, 1218–1231. doi: 10.1111/faf.12407
Rigal, S., Dakos, V., Alonso, H., Auniņš, A., Benkő, Z., Brotons, L., et al. (2023). Farmland practices are driving bird population decline across Europe. Proc. Natl. Acad. Sci. 120:e2216573120. doi: 10.1073/pnas.2216573120
Ringeval, B., Augusto, L., Monod, H., van Apeldoorn, D., Bouwman, L., Yang, X., et al. (2017). Phosphorus in agricultural soils: drivers of its distribution at the global scale. Glob. Chang. Biol. 23, 3418–3432. doi: 10.1111/gcb.13618
Rosenzweig, C., Mbow, C., Barioni, L. G., Benton, T. G., Herrero, M., Krishnapillai, M., et al. (2020). Climate change responses benefit from a global food system approach. Nat. Food 1, 94–97. doi: 10.1038/s43016-020-0031-z
Roth, T., Kohli, L., Rihm, B., and Achermann, B. (2013). Nitrogen deposition is negatively related to species richness and species composition of vascular plants and bryophytes in Swiss mountain grassland. Agric. Ecosyst. Environ. 178, 121–126. doi: 10.1016/j.agee.2013.07.002
Sabatier, R., Meyer, K., Wiegand, K., and Clough, Y. (2013). Non-linear effects of pesticide application on biodiversity-driven ecosystem services and disservices in a cacao agroecosystem: a modeling study. Basic Appl. Ecol. 14, 115–125. doi: 10.1016/j.baae.2012.12.006
Sala, O. E., Stuart Chapin, F., Armesto, J. J., Berlow, E., Bloomfield, J., Dirzo, R., et al. (2000). Global biodiversity scenarios for the year 2100. Science 287, 1770–1774. doi: 10.1126/science.287.5459.1770
Sánchez-Bayo, F. (2021). Indirect effect of pesticides on insects and other arthropods. Toxics 9:177. doi: 10.3390/toxics9080177
Sánchez-Bayo, F., and Wyckhuys, K. A. G. (2019). Worldwide decline of the entomofauna: a review of its drivers. Biol. Conserv. 232, 8–27. doi: 10.1016/j.biocon.2019.01.020
Sapek, A. (2013). Ammonia emissions from non-agricultural sources. Pol. J. Environ. Stud. 22, 63–70.
Schindler, D. W., Carpenter, S. R., Chapra, S. C., Hecky, R. E., and Orihel, D. M. (2016). Reducing phosphorus to curb Lake eutrophication is a success. Environ. Sci. Technol. 50, 8923–8929. doi: 10.1021/acs.est.6b02204
Schleuss, P. M., Widdig, M., Heintz-Buschart, A., Kirkman, K., and Spohn, M. (2020). Interactions of nitrogen and phosphorus cycling promote P acquisition and explain synergistic plant-growth responses. Ecology 101:e03003. doi: 10.1002/ecy.3003
Scranton, K., and Amarasekare, P. (2017). Predicting phenological shifts in a changing climate. Proc. Natl. Acad. Sci. 114, 13212–13217. doi: 10.1073/pnas.1711221114
Searchinger, T., James, O., Dumas, P., Kastner, T., and Wirsenius, S. (2022). EU climate plan sacrifices carbon storage and biodiversity for bioenergy. Nature 612, 27–30. doi: 10.1038/d41586-022-04133-1
Searchinger, T., Waite, R., Hanson, C., Ranganathan, J., Dumas, P., and Matthews, E. (2019). World resources report: creating a sustainable food future [WWW document]. URL world resources institute (Accessed July 6, 2023).
Seppelt, R., Arndt, C., Beckmann, M., Martin, E. A., and Hertel, T. W. (2020). Deciphering the biodiversity–production mutualism in the global food security debate. Trends Ecol. Evol. 35, 1011–1020. doi: 10.1016/j.tree.2020.06.012
Serra-Llobet, A., Jähnig, S. C., Geist, J., Kondolf, G. M., Damm, C., Scholz, M., et al. (2022). Restoring Rivers and floodplains for habitat and flood risk reduction: experiences in multi-benefit floodplain management from California and Germany. Front. Environ. Sci. 9:568. doi: 10.3389/fenvs.2021.778568
Siebert, S., and Döll, P. (2010). Quantifying blue and green virtual water contents in global crop production as well as potential production losses without irrigation. Journal of hydrology. Green-Blue Water Initiat. 384, 198–217. doi: 10.1016/j.jhydrol.2009.07.031
Simonich, S. L., and Hites, R. A. (1995). Global distribution of persistent organochlorine compounds. Science 269, 1851–1854. doi: 10.1126/science.7569923
Sirami, C., Gross, N., Baillod, A. B., Bertrand, C., Carrié, R., Hass, A., et al. (2019). Increasing crop heterogeneity enhances multitrophic diversity across agricultural regions. Proc. Natl. Acad. Sci. 116, 16442–16447. doi: 10.1073/pnas.1906419116
Smith, V. H. (2003). Eutrophication of freshwater and coastal marine ecosystems a global problem. Environ. Sci. Pollut. Res. 10, 126–139. doi: 10.1065/espr2002.12.142
Soons, M. B., Hefting, M. M., Dorland, E., Lamers, L. P. M., Versteeg, C., and Bobbink, R. (2017). Nitrogen effects on plant species richness in herbaceous communities are more widespread and stronger than those of phosphorus. Biol. Conserv. Nitrog. Deposit. Impacts Biodivers. Terrest. Ecosyst. Mech. Perspect. 212, 390–397. doi: 10.1016/j.biocon.2016.12.006
Soroye, P., Newbold, T., and Kerr, J. (2020). Climate change contributes to widespread declines among bumble bees across continents. Science 367, 685–688. doi: 10.1126/science.aax8591
Springmann, M., Clark, M., Mason-D’Croz, D., Wiebe, K., Bodirsky, B. L., Lassaletta, L., et al. (2018). Options for keeping the food system within environmental limits. Nature 562, 519–525. doi: 10.1038/s41586-018-0594-0
Springmann, M., Spajic, L., Clark, M. A., Poore, J., Herforth, A., Webb, P., et al. (2020). The healthiness and sustainability of national and global food based dietary guidelines: modelling study. BMJ 370:m2322. doi: 10.1136/bmj.m2322
Staudinger, M. D., Carter, S. L., Cross, M. S., Dubois, N. S., Duffy, J. E., Enquist, C., et al. (2013). Biodiversity in a changing climate: a synthesis of current and projected trends in the US. Front. Ecol. Environ. 11, 465–473. doi: 10.1890/120272
Steffen, W., Richardson, K., Rockström, J., Cornell, S. E., Fetzer, I., Bennett, E. M., et al. (2015). Planetary boundaries: guiding human development on a changing planet. Science 347:1259855. doi: 10.1126/science.1259855
Tamburini, G., Bommarco, R., Wanger, T. C., Kremen, C., van der Heijden, M. G. A., Liebman, M., et al. (2020). Agricultural diversification promotes multiple ecosystem services without compromising yield. Sci. Adv. 6:eaba1715. doi: 10.1126/sciadv.aba1715
Tang, F. H. M., Lenzen, M., McBratney, A., and Maggi, F. (2021). Risk of pesticide pollution at the global scale. Nat. Geosci. 14, 206–210. doi: 10.1038/s41561-021-00712-5
Thies, C., Haenke, S., Scherber, C., Bengtsson, J., Bommarco, R., Clement, L. W., et al. (2011). The relationship between agricultural intensification and biological control: experimental tests across Europe. Ecol. Appl. 21, 2187–2196. doi: 10.1890/10-0929.1
Thomas, C. D., Cameron, A., Green, R. E., Bakkenes, M., Beaumont, L. J., Collingham, Y. C., et al. (2004). Extinction risk from climate change. Nature 427, 145–148. doi: 10.1038/nature02121
Tilman, D., Balzer, C., Hill, J., and Befort, B. L. (2011). Global food demand and the sustainable intensification of agriculture. Proc. Natl. Acad. Sci. 108, 20260–20264. doi: 10.1073/pnas.1116437108
Tilman, D., Clark, M., Williams, D. R., Kimmel, K., Polasky, S., and Packer, C. (2017). Future threats to biodiversity and pathways to their prevention. Nature 546, 73–81. doi: 10.1038/nature22900
Tilman, D., and Lehman, C. (2001). Human-caused environmental change: impacts on plant diversity and evolution. Proc. Natl. Acad. Sci. USA 98, 5433–5440. doi: 10.1073/pnas.091093198
Tuck, S. L., Winqvist, C., Mota, F., Ahnström, J., Turnbull, L. A., and Bengtsson, J. (2014). Land-use intensity and the effects of organic farming on biodiversity: a hierarchical meta-analysis. J. Appl. Ecol. 51, 746–755. doi: 10.1111/1365-2664.12219
Vačkář, D., ten Brink, B., Loh, J., Baillie, J. E. M., and Reyers, B. (2012). Review of multispecies indices for monitoring human impacts on biodiversity. Ecol. Indic. Environ. Sustain. 17, 58–67. doi: 10.1016/j.ecolind.2011.04.024
van Klink, R., and WallisDeVries, M. F. (2018). Risks and opportunities of trophic rewilding for arthropod communities. Philos. Trans. Roy. Soc. B. Biol. Sci. 373:20170441. doi: 10.1098/rstb.2017.0441
Vendig, I., Guzman, A., De La Cerda, G., Esquivel, K., Mayer, A. C., Ponisio, L., et al. (2023). Quantifying direct yield benefits of soil carbon increases from cover cropping. Nat. Sustain. 6, 1125–1134. doi: 10.1038/s41893-023-01131-7
Venter, Z. S., Jacobs, K., and Hawkins, H.-J. (2016). The impact of crop rotation on soil microbial diversity: a meta-analysis. Pedobiologia 59, 215–223. doi: 10.1016/j.pedobi.2016.04.001
Vermeulen, S., Campbell, B. M., and Ingram, J. (2012). Climate change and food systems. Annu. Rev. Environ. Resour. 37, 195–222. doi: 10.1146/annurev-environ-020411-130608
Vickery, J. A., Tallowin, J. R., Feber, R. E., Asteraki, E. J., Atkinson, P. W., Fuller, R. J., et al. (2001). The management of lowland neutral grasslands in Britain: effects of agricultural practices on birds and their food resources. J. Appl. Ecol. 38, 647–664. doi: 10.1046/j.1365-2664.2001.00626.x
Vitousek, P. M., and Howarth, R. W. (1991). Nitrogen limitation on land and in the sea: how can it occur? Biogeochemistry 13, 87–115. doi: 10.1007/BF00002772
Vörösmarty, C. J., McIntyre, P. B., Gessner, M. O., Dudgeon, D., Prusevich, A., Green, P., et al. (2010). Global threats to human water security and river biodiversity. Nature 467, 555–561. doi: 10.1038/nature09440
Wagg, C., Bender, S. F., Widmer, F., and van der Heijden, M. G. A. (2014). Soil biodiversity and soil community composition determine ecosystem multifunctionality. Proc. Natl. Acad. Sci. 111, 5266–5270. doi: 10.1073/pnas.1320054111
Wan, N.-F., Fu, L., Dainese, M., Hu, Y.-Q., Pødenphant Kiær, L., Isbell, F., et al. (2022). Plant genetic diversity affects multiple trophic levels and trophic interactions. Nat. Commun. 13:7312. doi: 10.1038/s41467-022-35087-7
Wang, R., Balkanski, Y., Boucher, O., Ciais, P., Peñuelas, J., and Tao, S. (2015). Significant contribution of combustion-related emissions to the atmospheric phosphorus budget. Nat. Geosci. 8, 48–54. doi: 10.1038/ngeo2324
Wang, C., and Tang, Y. (2019). A global meta-analyses of the response of multi-taxa diversity to grazing intensity in grasslands. Environ. Res. Lett. 14:114003. doi: 10.1088/1748-9326/ab4932
Watson, J. E. M., Evans, T., Venter, O., Williams, B., Tulloch, A., Stewart, C., et al. (2018). The exceptional value of intact forest ecosystems. Nat. Ecol. Evol. 2, 599–610. doi: 10.1038/s41559-018-0490-x
Wezel, A., Herren, B. G., Kerr, R. B., Barrios, E., Gonçalves, A. L. R., and Sinclair, F. (2020). Agroecological principles and elements and their implications for transitioning to sustainable food systems. A review. Agron. Sustain. Dev. 40:40. doi: 10.1007/s13593-020-00646-z
White, E. V., and Roy, D. P. (2015). A contemporary decennial examination of changing agricultural field sizes using Landsat time series data. Geology 2, 33–54. doi: 10.1002/geo2.4
Willett, W., Rockström, J., Loken, B., Springmann, M., Lang, T., Vermeulen, S., et al. (2019). Food in the Anthropocene: the EAT–lancet commission on healthy diets from sustainable food systems. Lancet 393, 447–492. doi: 10.1016/S0140-6736(18)31788-4
Wilson, E.O. (2016). Half-Earth: Our Planet’s Fight for Life. New York: Liveright Publishing Corporation
Wintle, B. A., Kujala, H., Whitehead, A., Cameron, A., Veloz, S., Kukkala, A., et al. (2018). Global synthesis of conservation studies reveals the importance of small habitat patches for biodiversity. Proc. Natl. Acad. Sci. 116, 909–914. doi: 10.1073/pnas.1813051115
Woodley, S., Locke, H., Laffoley, D., MacKinnon, K., Sandwith, T., and Smart, J. (2019). A review of evidence for area-based conservation targets for the post-2020 global biodiversity framework. PARKS 25, 31–46. doi: 10.2305/IUCN.CH.2019.PARKS-25-2SW2.en
WWF (2022). Living planet report 2022—Building a nature-positive society. (eds.) Almond, R.E.A., Grooten, M., Juffe Bignoli, D. and Petersen, T. WWF: Gland, Switzerland.
Wyckhuys, K. A. G., Lu, Y., Morales, H., Vazquez, L. L., Legaspi, J. C., Eliopoulos, P. A., et al. (2013). Current status and potential of conservation biological control for agriculture in the developing world. Biol. Control 65, 152–167. doi: 10.1016/j.biocontrol.2012.11.010
Keywords: land use, intensification, land sharing and land sparing, ecosystem services, planetary boundaries, agrobiodiversity, agroecology, regenerative agriculture
Citation: García-Vega D, Dumas P, Prudhomme R, Kremen C and Aubert P-M (2024) A safe agricultural space for biodiversity. Front. Sustain. Food Syst. 8:1328800. doi: 10.3389/fsufs.2024.1328800
Edited by:
Kris A. G. Wyckhuys, Chrysalis Consulting, VietnamReviewed by:
Mohammad Ehsan Dulloo, Alliance Bioversity International and CIAT, FranceMatteo Dainese, University of Verona, Italy
Nian-Feng Wan, East China University of Science and Technology, China
Copyright © 2024 García-Vega, Dumas, Prudhomme, Kremen and Aubert. This is an open-access article distributed under the terms of the Creative Commons Attribution License (CC BY). The use, distribution or reproduction in other forums is permitted, provided the original author(s) and the copyright owner(s) are credited and that the original publication in this journal is cited, in accordance with accepted academic practice. No use, distribution or reproduction is permitted which does not comply with these terms.
*Correspondence: Diego García-Vega, diegogavega@gmail.com; Patrice Dumas, patrice.dumas@cirad.fr