- Department Biology I, Ludwig Maximilians University Munich, Planegg-Martinsried, Germany
Beside their central function in respiration plant mitochondria play important roles in diverse processes such as redox homeostasis, provision of precursor molecules for essential biosynthetic pathways, and programmed cell death. These different functions require the organelle to communicate with the rest of the cell by perceiving, transducing, and emitting signals. As the vast majority of mitochondrial proteins are encoded in the nuclear genome, changes in mitochondrial status must be fed back to the nucleus to coordinate gene expression accordingly, a process termed retrograde signaling. However, the nature of these signaling pathways in plants and their underlying signaling molecules – or indirect metabolite or redox signals – are not completely resolved. We explore the potential of different post-translational modifications (PTMs) to contribute to mitochondrial retrograde signaling. Remarkably, the substrates used for modifying proteins in many major PTMs are either central metabolites or redox-active compounds, as for example ATP, acetyl-CoA, NAD+, and glutathione. This suggests that the metabolic status of organelles and of the cell in general could be indirectly gaged by the enzymes catalyzing the various PTMs. We examine the evidence supporting this hypothesis with regard to three major PTMs, namely phosphorylation, lysine acetylation, and glutathionylation and assess their potential to regulate not only organellar processes by modifying metabolic enzymes but also to influence nuclear gene expression.
Introduction
Plant mitochondria are central hubs in the conversion of energy and redox homeostasis and are connected to metabolic pathways from different subcellular compartments. Hence, mitochondria are ideally placed to act as sensors of the energetic and metabolic status of the plant cell (Sweetlove et al., 2007; Millar et al., 2011). Perturbations of the cellular energy status can lead to a reconfiguration of mitochondrial activities which in turn have profound effects on other cellular compartments, including major changes in nuclear gene expression (NGE) and photosynthetic activity (Rhoads, 2011; Schwarzländer et al., 2012). Changes in NGE which are triggered by signals derived from metabolic perturbations in organelles are termed retrograde responses (RRs) and are considered important means of tuning anterograde regulation to retain metabolic plasticity (Rhoads, 2011). However, so far the well understood RRs were uncovered mainly in yeast and animals, whereas in plants conclusive evidence for the exact nature of particular retrograde signals and their transduction is largely lacking. This holds especially true for mitochondrial RR (MRR) for which so far only putative signaling candidates and one downstream transcription factor (ABI4) could be identified (Gray et al., 2004; Giraud et al., 2009). The difficulties in elucidating the nature of RR might originate in their possible complexity. Recently, Leister (2012) defined characteristics of retrograde signals and theoretically explored different scenarios of how single and multiple retrograde signals could transduce information to the nucleus in an isolated or coordinated fashion. In general two types of retrograde signals can be distinguished (Figure 1):
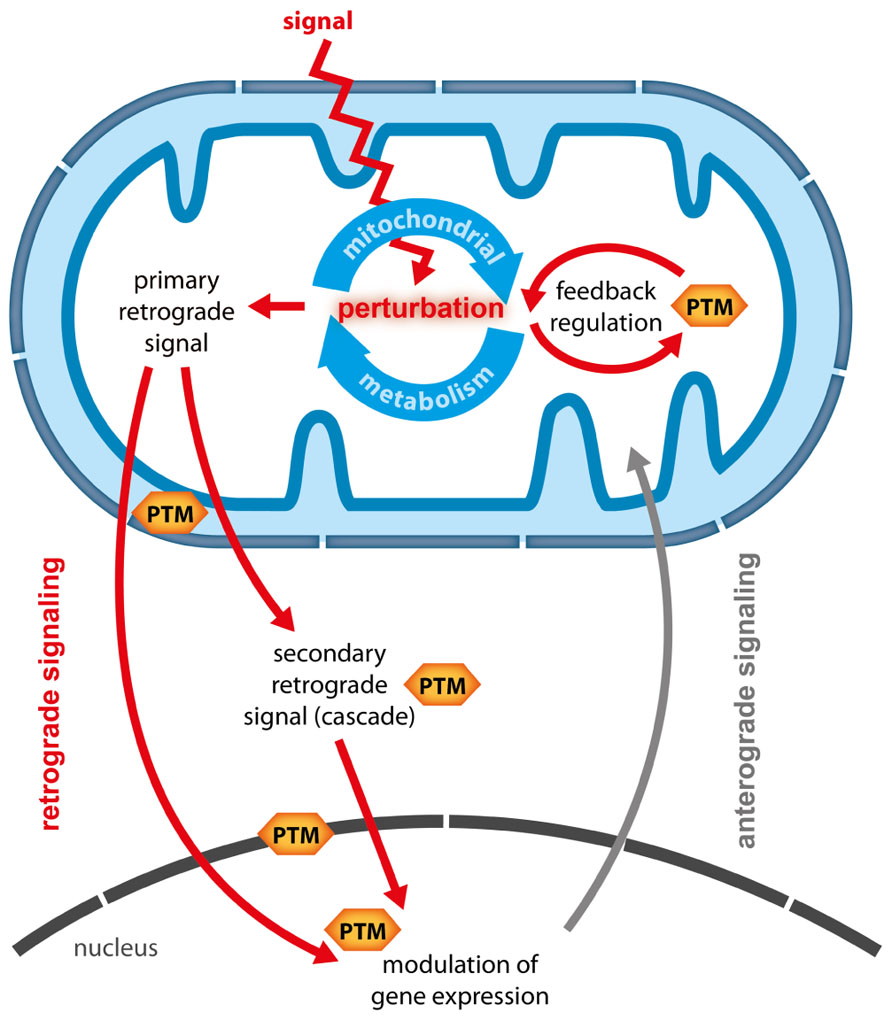
FIGURE 1. Potential functions of PTMs in mitochondrial retrograde signaling. When an external signal or stress causes perturbations in mitochondrial metabolism several responses are possible and PTMs could contribute on multiple levels. (1) Mitochondrial metabolism is adapted via feedback regulation, during which PTM catalyzing enzymes could alter enzymatic activity in response to changes in metabolic status. (2) Metabolic intermediates are generated as primary retrograde signals, which either travel directly to the nucleus, or which are perceived at the mitochondrial boundary or in the cytosol and then translated into secondary signals or signaling cascades. PTMs are likely to be involved in the generation and transduction of such secondary signals. Moreover, PTMs could transduce signals across the inner mitochondrial or the nuclear membrane. In the nucleus PTMs of histones, transcription factors or transcriptional complexes could translate mitochondria-derived signals into specific changes in gene expression.
1. Primary or direct retrograde signals are generated in the organelle and either passively diffuse or are actively transported to the nucleus, where they modulate NGE. Primary retrograde signals are therefore most likely metabolic intermediates generated from metabolic pathways in the respective organelles.
2. Secondary or indirect signals are activated by primary signals inside or outside the organelle, and travel to the nucleus or activate a cascade of signaling events that eventually alter NGE.
Post-translational modifications (PTMs) are ideally suited for signal integration and thus present a likely mechanism to transduce indirect retrograde signals. PTMs such as phosphorylation have key roles in signal transduction and amplification of cytosolic signaling cascades. It is therefore conceivable that primary mitochondrial signals could generate secondary signals (Møller and Sweetlove, 2010) or activate signal transduction cascades that traverse the cytosol. Here, we discuss evidence that PTMs connected to mitochondrial energy and redox metabolism occurring both outside and inside mitochondria are potential key players in transducing indirect MRR signals. We propose that recent technological advances in high resolution mass-spectrometry and sample preparation will allow wide and largely unbiased screens for mitochondrial PTMs and their dynamics (Witze et al., 2007; Choudhary and Mann, 2010). Such PTM-based analyses could help to elucidate the black box of MRR and to reveal some of its signaling components.
Post-Translational Modifications – Light in the Black Box of Mitochondrial Signaling?
Post-translational modifications provide a powerful mechanism to rapidly and temporarily alter protein functions and locations in the cell, and they are also capable of providing information to regulatory proteins by creating docking sites for PTM-recognition domains (Deribe et al., 2010). PTMs which are dynamic, rapid, and reversible are suitable to direct transient changes in cellular metabolism and signaling. Intriguingly, the substrates of several major PTM-modifying enzymes are either central metabolites or redox-active compounds, as for example ATP, acetyl-CoA, NAD+, and glutathione, which suggests that the activity of these enzymes could be directly coupled to the energy status or redox status of the organelle.
Phosphorylation
Although a few phosphorylation-regulated mitochondrial enzymes are well established, such as the pyruvate dehydrogenase (Gemel and Randall, 1992), only recently the regulatory role of phosphorylation in mitochondrial signal processing and metabolic regulation has begun to emerge (Pagliarini and Dixon, 2006; Ito et al., 2007; Juszczuk et al., 2007; Foster et al., 2009). There are indications that all major kinase signaling pathways in mammals can target the mitochondrion and influence mitochondrial function (Horbinski and Chu, 2005; Pagliarini and Dixon, 2006). Recent publications describe 77 phosphoproteins in mitochondria from human muscle cells and 181 phosphoproteins from murine cardiac mitochondria (Deng et al., 2011; Zhao et al., 2011). However, despite remarkable efforts merely 22 phosphoproteins are reported for Arabidopsis (Ito et al., 2009) and 14, mostly different, phosphoproteins for potato (Bykova et al., 2003). It is very likely that mitochondrial status and signaling varies with tissue and environmental conditions, which could explain why putative phosphoproteins remained undetected in studies concentrating on cell cultures or particular tissues. Similar to the fragmentary mitochondrial phosphoproteome, the available data describing mitochondrial protein kinases and phosphatases in plants is scarce (Heazlewood et al., 2004; Juszczuk et al., 2007). Several kinases and phosphatases attached to the outer mitochondrial membrane have recently been identified, but their possible role in mitochondrial signaling remains to be explored (Duncan et al., 2011; Sun et al., 2012). A large-scale yeast two-hybrid screen for interactors of mitogen-activated protein kinases (MAPKs) in rice indicates that these kinases could phosphorylate mitochondrial proteins (Singh et al., 2012). Lundquist et al. (2012) suggested that the so far largely neglected ABC1K protein kinase family could further fill the gap of mitochondrial kinases but clearly more experimental work will be necessary.
The points mentioned above indicate that phosphorylation might influence mitochondrial function but whether it could also influence MRR remains an open question. First evidence that plant MRR is indeed partially regulated via phosphorylation comes from the observation that the citrate-dependent induction of the mitochondrial alternative oxidase can be inhibited by the protein kinase inhibitor staurosporine (Djajanegara et al., 2002). Furthermore, Takahashi et al. (2003) demonstrated that spermine-induced functional changes in tobacco mitochondria trigger a MAPK-cascade and activate specific hypersensitive response genes. However, both pathways were not further explored yet.
Mitochondrial signals could activate phosphorylation-dependent signaling cascades inside or outside the organelle, which transduce MRR. Strong support for the existence of such a mechanism was found in yeast, where the “RTG-dependent pathway” represents one of the best-studied MRR. It has been shown that perturbations of mitochondrial functions in yeast result in lowered mitochondrial membrane potentials, eliciting a kinase-based response that regulates the phosphorylation status and translocation of specific TFs to the nucleus (Liu and Butow, 2006; Jazwinski, 2012). Candidates for phosphorylation-dependent signaling involved in MRR in plants are SNF1-related kinases (SnRKs), with the cytosolic SnRK1 in particular, and the target of rapamycin (TOR) protein kinases. SnRK1 and TOR belong to different evolutionary conserved families of protein kinases, which likely function as master regulators and sensors of energy and nutrient metabolism during growth and development as well as under energy-depleting stress conditions (Polge and Thomas, 2007; Baena-González and Sheen, 2008; Dobrenel et al., 2011; Ghillebert et al., 2011; Ren et al., 2011; Xiong and Sheen, 2012). Although some important modulators of SnRK1- and TOR-activity have been described the main metabolic signals that activate these kinases remain unknown (Baena-González and Sheen, 2008; Ghillebert et al., 2011; Xiong and Sheen, 2012). Interestingly, there is an overlap in regulated transcripts between SnRK1-mediated responses and specific conditions of mitochondrial dysfunction (Schwarzländer et al., 2012) and it will be interesting to ascertain whether SnRK1 integrates metabolic signals from mitochondria, thus contributing to MRR. In yeast and mammals TOR signaling is vital for the maintenance of mitochondrial respiration and it is involved in the MRR (Schieke and Finkel, 2006). For example mammalian TOR (mTOR) interacts with PGC1α (Cunningham et al., 2007), a transcriptional activator regulating mitochondrial biogenesis and activity. Recent results in Arabidopsis suggest that TOR could have a similar function in plants but the exact mechanisms remain to be discovered (Leiber et al., 2010; Xiong and Sheen, 2012).
Lysine Acetylation
Acetylation of the ε-amino group of lysine residues on proteins has recently emerged as a major PTM of proteins that has the potential to rival the regulatory role of phosphorylation (Choudhary et al., 2009; Norvell and McMahon, 2010). For a long time the research on lysine acetylation of proteins has concentrated almost exclusively on histones and transcriptional regulation. However, lysine acetylation of non-histone proteins was confirmed to be a widespread PTM in prokaryotes and eukaryotes that has profound regulatory effects on various metabolic and developmental processes (Close et al., 2010; Xing and Poirier, 2012). From a number of studies it became evident that lysine acetylation is involved in energy-homeostasis and regulation of carbon-flux, by directly altering NGE or the activities of key metabolic enzymes, such as glyceraldehyde 3-phosphate dehydrogenase and isocitrate dehydrogenase in Salmonella enterica (Wang et al., 2010), or mammalian mitochondrial acetyl-CoA-synthetase 2 (Hallows et al., 2006; Schwer et al., 2006). Furthermore, Kim et al. (2006) demonstrated that lysine acetylation patterns varied depending on nutritional status. Anderson and Hirschey (2012) estimated 35% of all mammalian mitochondrial proteins to have at least one acetylation site, and found pathways involved in the generation of energy, fatty acid metabolism, sugar metabolism, and amino acid metabolism to be significantly enriched in acetylated proteins. Important progress has also been made in identifying lysine-acetylated non-histone proteins in Arabidopsis (Finkemeier et al., 2011; Wu et al., 2011). These two studies describe a total number of 125 proteins to be lysine-acetylated in Arabidopsis, with cytochrome c representing the only mitochondrial protein. Only six common proteins were identified in both studies (Xing and Poirier, 2012), which suggests that the overall coverage was fairly low and that more extensive screens are likely to identify a much larger number of lysine-acetylated proteins in plants.
The huge potential of lysine acetylation acting as a metabolic sensor and regulator lies in the chemistry of the enzymes that catalyze this PTM. Lysine acetyltransferases (KATs) transfer the acetyl-moiety from acetyl-CoA to the target proteins. This suggests that the availability of the central metabolic intermediate acetyl-CoA or the acetyl-CoA/CoA ratio could be directly gaged by KATs (Xing and Poirier, 2012). As acetyl-CoA occurs in the cytosol and in organelles but cannot freely diffuse through membranes it has to be synthesized and metabolized independently in each compartment, which makes it a good indicator of local carbon status (Oliver et al., 2009). In Arabidopsis at least 12 KATs were identified by sequence homology and a number of them has been described to influence various developmental processes via acetylation of histones (Pandey et al., 2002; Earley et al., 2007; Servet et al., 2010). Information on KATs that acetylate non-histone proteins outside the nucleus is largely lacking. Only one mitochondrially localized KAT has been identified that acetylates lysine-residues of proteins belonging to the respiratory electron transport chain (ETC; Scott et al., 2012). It is well conceivable that there are other classes of KATs that remain to be discovered (Anderson and Hirschey, 2012).
In contrast, much more evidence is available for the reverse reaction catalyzed by lysine deacetylases (KDACs), in particular the KDAC class III of the so-called sirtuins (for “silent information regulation 2 homolog”). Sirtuins differ from the other three classes of deacetylases in their dependence on NAD+ as substrate and the release of O-acetyl-ribose and nicotinamide (NAM) as by-products of the deacetylation. The NAD+/NADH ratio reflects the energy status of a cell and several studies found that starvation conditions activate sirtuins in yeast and mammals, leading to transcriptional and metabolic adaptation via the deacetylation of histones, TFs, and metabolic enzymes (Zhong and Mostoslavsky, 2011; Houtkooper et al., 2012). These observations led to the hypothesis that protein acetylation could serve as a central switch between oxidative and fermentative metabolism, with acetyl-CoA, NAD+, KATs, and NAD+-dependent KDACs as key players (Guarente, 2011; Xing and Poirier, 2012). Although direct evidence is missing, such a model would allow the integration of MRR (Verdin et al., 2010). For example the activity of PGC1α (see above) is modulated by reversible acetylation through the KAT GCN5 and sirtuin 1 (SIRT1), depending on cellular acetyl-CoA and NAD+ status (Canto and Auwerx, 2009). Furthermore, SIRT3 is regarded to be the major mitochondrial deacetylase in mammals with many targets involved in mitochondrial metabolism. This includes central enzymes as for example complex I, II, and III of the ETC, isocitrate dehydrogenases and glutamate dehydrogenase in the TCA-cycle, mitochondrial acetyl-CoA-synthetase, and long-chain acyl-CoA dehydrogenase, which is involved in fatty acid oxidation (Anderson and Hirschey, 2012; Houtkooper et al., 2012). From these observations a common model has emerged suggesting that under starvation conditions SIRT3 is activated by increased NAD+ levels, stimulating oxidative metabolism and ATP-production (Zhong and Mostoslavsky, 2011). Changes in the cytosolic AMP/ATP ratio could be perceived by the AMP-activated protein kinase (AMPK, a homolog of SnRK1 mentioned above), which promotes catabolism and inactivates energy-consuming pathways (Verdin et al., 2010). Although the AMPK plant homolog SnRK1 is not allosterically regulated by the AMP/ATP ratio (Ghillebert et al., 2011) it is intimately linked with cellular carbon status. Consequently, a sirtuin-dependent metabolic regulatory pathway seems possible in plants. The Arabidopsis genome encodes for two sirtuin genes, of which one (AtSRT2) comes in seven different splice-forms. However, the subcellular localization and the possible functions in metabolic regulation remain to be determined. Apart from sirtuins Arabidopsis expresses at least 16 KDACs of other classes, the functions of which have just started to be unraveled (Pandey et al., 2002; Hollender and Liu, 2008). While some of them were confirmed to deacetylate histones in the nucleus to regulate developmental processes (e.g., HDA6; Wu et al., 2008; Yu et al., 2011), others, like HDA5, HDA8, and HDA14, were found to be expressed in the cytosol, chloroplasts, or mitochondria and seem to be involved in the acetylation of non-histone proteins, as for example α-tubulin in the case of HDA14 (Alinsug et al., 2012; Tran et al., 2012). The dependence on central metabolites as co-substrates and the potential to directly and reversibly influence NGE and enzymatic activities makes lysine acetylation a promising candidate for regulating plant metabolism on multiple levels including MRR.
PTMs Connected to Mitochondrial Redox State
Reactive oxygen species (ROS) are continuously produced as by-products of respiratory metabolism. Their production is increased under conditions of high matrix NADH/NAD+ ratios or when the ubiquinone pool is highly reduced in conjunction with a high membrane potential (Møller, 2001; Murphy, 2009). Hence, the mitochondrial redox state is intimately linked to mitochondrial energy metabolism, and is defined by the balance of ROS produced by metabolic processes and the availability of NADPH as reducing equivalent to sustain the antioxidant system. The mitochondrial antioxidant defense system has a key role in the detoxification of peroxides and thus is a prime target to affect redox signaling. PTMs on plant mitochondrial antioxidant enzymes have not yet been studied in detail, but phosphorylation sites of antioxidant enzymes have been identified in several studies and are awaiting further functional characterization (Nakagami et al., 2010).
Oxidative PTMs of proteins that arise from increased ROS production in mitochondria can either be irreversible or reversible. The chemistry of oxidative modifications on proteins is quite complex and their full potential is not explored yet. The best-characterized irreversible protein oxidation in mitochondria is carbonylation, which is regarded as an indicator of oxidative damage to the cell (Møller et al., 2011). The iron-sulfur cluster of the TCA-cycle enzyme aconitase for example is particularly prone to oxidative inactivation, which might activate MRR in plants by increasing mitochondrial citrate levels (Gray et al., 2004; Rhoads and Subbaiah, 2007). Reversible oxidative modifications only occur at cysteine and methionine residues of proteins, which can be reversed by sulfiredoxins (in case of cysteine sulfinic acids), thioredoxins (TRXs; in case of disulfides), glutaredoxins (GRXs; in case of disulfides and glutathione-mixed disulfides) and methionine sulfoxide reductases (in case of methionine sulfoxides). Disulfides formed during cysteine oxidation play important roles in regulating enzyme activities and protein functions in diverse metabolic processes as well as in transcriptional regulation and signaling in plants and animals (Foyer and Noctor, 2009; Konig et al., 2012; Murphy, 2012). In the matrix of plant mitochondria 50 TRX-linked proteins and 18 GRX-linked proteins have been identified from various metabolic processes (Balmer et al., 2004; Rouhier et al., 2005). However, the in vivo confirmation of the functional regulation of plant mitochondrial metabolism by TRXs and GRXs is still lacking. The plant mitochondrial disulphide proteome was further assessed in a study using diagonal gel electrophoresis (Winger et al., 2007), identifying 21 proteins from major metabolic pathways which form either inter- or intramolecular disulfides under oxidizing conditions. Catalytic cysteines are important for the activities of many enzymes involved in metabolism (e.g., cysteine proteases, ubiquitin ligases, and peroxidases) and signaling (e.g., tyrosine phosphatases). S-Glutathionylation is a reversible PTM of cysteine, which is regarded as mechanism to protect redox-active cysteines from irreversible inactivation beside its role in redox signaling (Gallogly and Mieyal, 2007). Both glycine decarboxylase, a key enzyme in the photorespiratory pathway, as well as galactonolactone dehydrogenase, a major enzyme in ascorbate synthesis, were inactivated by glutathionylation in plant mitochondria (Leferink et al., 2009; Palmieri et al., 2010). Hence, glutathionylation could play a role in the temporary protection of these metabolic enzymes under conditions of oxidative stress. Metabolic changes resulting from inhibitions of these enzymes could then play a role in MRR. Irreversibly inactivated proteins can be degraded in all mitochondrial subcompartments (Fischer et al., 2012). Recent work in C. elegans demonstrated that the mitochondrial peptide exporter HAF-1 is required for MRR (Haynes et al., 2010). Møller and Sweetlove (2010) suggested that oxidatively modified peptides in particular could convey specific information to regulate oxidative stress-induced MRR in plants. Novel proteomic approaches using bifunctional thiol-specific alkylation reagents coupled to an epitope tag, or to a fluorescent or isotope-label will provide useful tools to further explore the functional significance of the mitochondrial redox proteome and its putative role in regulating MRR in plants (Held and Gibson, 2012).
Future Directions
A recent in-depth bioinformatics analysis estimated the plant mitochondrial proteome to contain about 2500 proteins (Cui et al., 2011). Many of these proteins will contain multiple PTMs of different types, which will consequently alter protein functions or even localization of proteins within the mitochondria. When thinking of the role of PTMs in signaling, primarily the classical phosphorylation-based MAPK-cascades come to mind, which are often initiated by receptor kinases. However, it is also conceivable that different types of PTMs could be series-connected or could interact in a codified crosstalk. A recent in silico study demonstrated that lysine acetylation sites have a great potential to affect nearby phosphorylation, methylation, and ubiquitination sites (Lu et al., 2011). Such a crosstalk was indeed observed in an in vivo study using the genome-reduced bacterium Mycoplasma pneumoniae (van Noort et al., 2012). Deletion of the only two protein kinases and a unique protein phosphatase modulated lysine acetylation patterns, while in return deletion of the only two KATs had an impact on protein phosphorylation. Hence, many scenarios are conceivable that include phosphorylation-based signaling cascades regulated by various types of PTMs. Just as conceivable is the inverse scenario, having, phosphorylation or lysine acetylation regulating the activity of metabolic enzymes which would affect metabolic signaling. Such a complex PTM-based crosstalk is for example demonstrated in the regulation of the activity of the mitochondrial manganese superoxide dismutase (MnSOD). MnSOD is responsible for the decomposition of superoxide to hydrogen peroxide in the mitochondrial matrix. The activity of MnSOD can be modified by several PTMs including phosphorylation, lysine acetylation, and tyrosine nitration in mammals (Yamakura et al., 1998; Hopper et al., 2006; Ozden et al., 2011), while phosphorylation, carbonylation, and oxidative degradation of MnSOD have been observed in plants (Sweetlove et al., 2002; Kristensen et al., 2004; Juszczuk et al., 2007). Removal of phosphorylation and lysine acetylation from MnSOD were both shown to increase its activity, however, these occur under different physiological conditions (Finley and Haigis, 2009; Foster et al., 2009; Fukai and Ushio-Fukai, 2011; Ozden et al., 2011). Hence both modifications are probably connected in a codified crosstalk and will impact on mitochondrial redox signaling.
Several key questions will need to be answered in future research to fully understand the complexity and regulation of the mitochondrial proteome in dependence on environmental or metabolic cues: (1) Do different PTMs identified on the same proteins occur at the same time and if not what is their sequential arrangement?, (2) What is the physiological role of the different PTMs with regard to protein function?, and (3) Do different PTMs influence each other? To investigate these questions in detail and to further explore the role of PTMs in MRR, sophisticated proteomic analyses will be necessary that allow a time-resolved and in-depth inventory of the mitochondrial proteome and its modifications under conditions that trigger MRR. Furthermore, shot-gun proteomics approaches that analyze fractionated whole cell proteomes rather than sub-proteomes from isolated organelles might be more suitable for such type of analysis. Mitochondrial isolation procedures often take several hours and can have broad impacts on mitochondrial PTMs such as oxidative modifications. Several examples of PTM-based regulation of central mitochondrial proteins in animals demonstrate the need for further dissection of the functions of PTMs in plant mitochondrial signaling. Furthermore, autotrophic plant tissues differ fundamentally from animal heterotrophic tissues in terms of energy production, consumption, and homeostasis, and it is most likely that novel plant-specific regulatory mechanisms will be identified.
Conflict of Interest Statement
The authors declare that the research was conducted in the absence of any commercial or financial relationships that could be construed as a potential conflict of interest.
Acknowledgments
We apologize to all colleagues whose work could not be cited due to space restrictions. This work was supported by the Deutsche Forschungsgemeinschaft, Germany (Emmy Noether Programme FI-1655/1-1 and Research Unit 804).
References
Alinsug, M. V., Chen, F. F., Luo, M., Tai, R., Jiang, L., and Wu, K. (2012). Subcellular localization of class II HDAs in Arabidopsis thaliana: nucleocytoplasmic shuttling of HDA15 is driven by light. PLoS ONE 7, e30846. doi: 10.1371/journal.pone.0030846
Anderson, K. A., and Hirschey, M. D. (2012). Mitochondrial protein acetylation regulates metabolism. Essays Biochem. 52, 23–35.
Baena-González, E., and Sheen, J. (2008). Convergent energy and stress signaling. Trends Plant Sci. 13, 474–482.
Balmer, Y., Vensel, W. H., Tanaka, C. K., Hurkman, W. J., Gelhaye, E., Rouhier, N., et al. (2004). Thioredoxin links redox to the regulation of fundamental processes of plant mitochondria. Proc. Natl. Acad. Sci. U.S.A. 101, 2642–2647.
Bykova, N. V., Egsgaard, H., and Møller, I. M. (2003). Identification of 14 new phosphoproteins involved in important plant mitochondrial processes. FEBS Lett. 540, 141–146.
Canto, C., and Auwerx, J. (2009). PGC-1alpha, SIRT1 and AMPK, an energy sensing network that controls energy expenditure. Curr. Opin. Lipidol. 20, 98–105.
Choudhary, C., Kumar, C., Gnad, F., Nielsen, M. L., Rehman, M., Walther, T. C., et al. (2009). Lysine acetylation targets protein complexes and co-regulates major cellular functions. Science 325, 834–840.
Choudhary, C., and Mann, M. (2010). Decoding signalling networks by mass spectrometry-based proteomics. Nat. Rev. Mol Cell Biol. 11, 427–439.
Close, P., Creppe, C., Gillard, M., Ladang, A., Chapelle, J.-P., Nguyen, L., et al. (2010). The emerging role of lysine acetylation of non-nuclear proteins. Cell. Mol. Life Sci. 67, 1255–1264.
Cui, J., Liu, J., Li, Y., and Shi, T. (2011). Integrative Identification of Arabidopsis mitochondrial proteome and its function exploitation through protein interaction network. PLoS ONE 6, e16022. doi: 10.1371/journal.pone.0016022
Cunningham, J. T., Rodgers, J. T., Arlow, D. H., Vazquez, F., Mootha, V. K., and Puigserver, P. (2007). mTOR controls mitochondrial oxidative function through a YY1–PGC-1[agr] transcriptional complex. Nature 450, 736–740.
Deng, N., Zhang, J., Zong, C., Wang, Y., Lu, H., Yang, P., et al. (2011). Phosphoproteome analysis reveals regulatory sites in major pathways of cardiac mitochondria. Mol. Cell. Proteomics 10, M110.000117.
Deribe, Y. L., Pawson, T., and Dikic, I. (2010). Post-translational modifications in signal integration. Nat. Struct. Mol. Biol. 17, 666–672.
Djajanegara, I., Finnegan, P. M., Mathieu, C., Mccabe, T., Whelan, J., and Day, D. A. (2002). Regulation of alternative oxidase gene expression in soybean. Plant Mol. Biol. 50, 735–742.
Dobrenel, T., Marchive, C., Sormani, R., Moreau, M., Mozzo, M., Montane, M. H., et al. (2011). Regulation of plant growth and metabolism by the TOR kinase. Biochem. Soc. Trans. 39, 477–481.
Duncan, O., Taylor, N. L., Carrie, C., Eubel, H., Kubiszewski-Jakubiak, S., Zhang, B., et al. (2011). Multiple lines of evidence localize signaling, morphology, and lipid biosynthesis machinery to the mitochondrial outer membrane of Arabidopsis. Plant Physiol. 157, 1093–1113.
Earley, K. W., Shook, M. S., Brower-Toland, B., Hicks, L., and Pikaard, C. S. (2007). In vitro specificities of Arabidopsis co-activator histone acetyltransferases: implications for histone hyperacetylation in gene activation. Plant J. 52, 615–626.
Finkemeier, I., Laxa, M., Miguet, L., Howden, A., and Sweetlove, L. (2011). Proteins of diverse function and subcellular location are lysine-acetylated in Arabidopsis. Plant Physiol. 155, 1779–1790.
Finley, L. W., and Haigis, M. C. (2009). The coordination of nuclear and mitochondrial communication during aging and calorie restriction. Ageing Res. Rev. 8, 173–188.
Fischer, F., Hamann, A., and Osiewacz, H. D. (2012). Mitochondrial quality control: an integrated network of pathways. Trends Biochem. Sci. 37, 284–292.
Foster, D. B., Van Eyk, J. E., Marbán, E., and O’Rourke, B. (2009). Redox signaling and protein phosphorylation in mitochondria: progress and prospects. J. Bioenerg. Biomembr. 41, 159–168.
Foyer, C. H., and Noctor, G. (2009). Redox regulation in photosynthetic organisms: signaling, acclimation, and practical implications. Antioxid. Redox. Signal. 11, 861–905.
Fukai, T., and Ushio-Fukai, M. (2011). Superoxide dismutases: role in redox signaling, vascular function, and diseases. Antioxid. Redox. Signal. 15, 1583–1606.
Gallogly, M. M., and Mieyal, J. J. (2007). Mechanisms of reversible protein glutathionylation in redox signaling and oxidative stress. Curr. Opin. Pharmacol. 7, 381–391.
Gemel, J., and Randall, D. D. (1992). Light regulation of leaf mitochondrial pyruvate dehydrogenase complex: role of photorespiratory carbon metabolism. Plant Physiol. 100, 908–914.
Ghillebert, R., Swinnen, E., Wen, J., Vandesteene, L., Ramon, M., Norga, K., et al. (2011). The AMPK/SNF1/SnRK1 fuel gauge and energy regulator: structure, function and regulation. FEBS J. 278, 3978–3990.
Giraud, E., Van Aken, O., Ho, L. H. M., and Whelan, J. (2009). The transcription factor ABI4 is a regulator of mitochondrial retrograde expression of ALTERNATIVE OXIDASE1a. Plant Physiol. 150, 1286–1296.
Gray, G. R., Maxwell, D. P., Villarimo, A. R., and Mcintosh, L. (2004). Mitochondria/nuclear signaling of alternative oxidase gene expression occurs through distinct pathways involving organic acids and reactive oxygen species. Plant Cell Rep. 23, 497–503.
Hallows, W. C., Lee, S., and Denu, J. M. (2006). Sirtuins deacetylate and activate mammalian acetyl-CoA synthetases. Proc. Natl. Acad. Sci. U.S.A. 103, 10230–10235.
Haynes, C. M., Yang, Y., Blais, S. P., Neubert, T. A., and Ron, D. (2010). The matrix peptide exporter HAF-1 signals a mitochondrial UPR by activating the transcription factor ZC376.7 in C. elegans. Mol. Cell 37, 529–540.
Heazlewood, J. L., Tonti-Filippini, J. S., Gout, A. M., Day, D. A., Whelan, J., and Millar, A. H. (2004). Experimental analysis of the Arabidopsis mitochondrial proteome highlights signaling and regulatory components, provides assessment of targeting prediction programs, and indicates plant-specific mitochondrial proteins. Plant Cell 16, 241.
Held, J. M., and Gibson, B. W. (2012). Regulatory control or oxidative damage? Proteomic approaches to interrogate the role of cysteine oxidation status in biological processes. Mol. Cell. Proteomics 11, R111.013037.
Hollender, C., and Liu, Z. (2008). Histone deacetylase genes in Arabidopsis development. J. Integr. Plant Biol. 50, 875–885.
Hopper, R. K., Carroll, S., Aponte, A. M., Johnson, D. T., French, S., Shen, R. F., et al. (2006). Mitochondrial matrix phosphoproteome: effect of extra mitochondrial calcium. Biochemistry 45, 2524–2536.
Horbinski, C., and Chu, C. T. (2005). Kinase signaling cascades in the mitochondrion: a matter of life or death. Free Radic. Biol. Med. 38, 2–11.
Houtkooper, R. H., Pirinen, E., and Auwerx, J. (2012). Sirtuins as regulators of metabolism and healthspan. Nat. Rev. Mol. Cell Biol. 13, 225–238.
Ito, J., Heazlewood, J. L., and Millar, A. H. (2007). The plant mitochondrial proteome and the challenge of defining the posttranslational modifications responsible for signalling and stress effects on respiratory functions. Physiol. Plant. 129, 207–224.
Ito, J., Taylor, N. L., Castleden, I., Weckwerth, W., Millar, A. H., and Heazlewood, J. L. (2009). A survey of the Arabidopsis thaliana mitochondrial phosphoproteome. Proteomics 9, 4229–4240.
Jazwinski, S. M. (2012). The retrograde response: when mitochondrial quality control is not enough. Biochim. Biophys. Acta doi: 10.1016/j.bbamcr.2012.02.010 [Epub ahead of print]
Juszczuk, I. M., Bykova, N. V., and Møller, I. M. (2007). Protein phosphorylation in plant mitochondria. Physiol. Plant. 129, 90–103.
Kim, S. C., Sprung, R., Chen, Y., Xu, Y., Ball, H., Pei, J., et al. (2006). Substrate and functional diversity of lysine acetylation revealed by a proteomics survey. Mol. Cell 23, 607–618.
Konig, J., Muthuramalingam, M., and Dietz, K. J. (2012). Mechanisms and dynamics in the thiol/disulfide redox regulatory network: transmitters, sensors and targets. Curr. Opin. Plant Biol 15, 261–268.
Kristensen, B. K., Askerlund, P., Bykova, N. V., Egsgaard, H., and Moller, I. M. (2004). Identification of oxidised proteins in the matrix of rice leaf mitochondria by immunoprecipitation and two-dimensional liquid chromatography-tandem mass spectrometry. Phytochemistry 65, 1839–1851.
Leferink, N. G., Van Duijn, E., Barendregt, A., Heck, A. J., and Van Berkel, W. J. (2009). Galactonolactone dehydrogenase requires a redox-sensitive thiol for optimal production of vitamin C. Plant Physiol. 150, 596–605.
Leiber, R.-M., John, F., Verhertbruggen, Y., Diet, A., Knox, J. P., and Ringli, C. (2010). The TOR pathway modulates the structure of cell walls in Arabidopsis. Plant Cell 22, 1898–1908.
Leister, D. (2012). Retrograde signaling in plants: from simple to complex scenarios. Front. Plant Sci. 3:135. doi: 10.3389/fpls.2012.00135
Liu, Z., and Butow, R. A. (2006). Mitochondrial retrograde signaling. Annu. Rev. Genet. 40, 159–185.
Lu, Z., Cheng, Z., Zhao, Y., and Volchenboum, S. L. (2011). Bioinformatic analysis and post-translational modification crosstalk prediction of lysine acetylation. PLoS ONE 6, e28228. doi: 10.1371/journal.pone.0028228
Lundquist, P. K., Davis, J. I., and Van Wijk, K. J. (2012). ABC1K atypical kinases in plants: filling the organellar kinase void. Trends Plant Sci. 17, 546–555.
Millar, A. H., Whelan, J., Soole, K. L., and Day, D. A. (2011). Organization and regulation of mitochondrial respiration in plants. Annu. Rev. Plant Biol. 62, 79–104.
Møller, I. M. (2001). Plant mitochondria and oxidative stress: electron transport, NADPH turnover, and metabolism of reactive oxygen species. Annu. Rev. Plant Physiol. Plant Mol. Biol. 52, 561–591.
Møller, I. M., Rogowska-Wrzesinska, A., and Rao, R. S. (2011). Protein carbonylation and metal-catalyzed protein oxidation in a cellular perspective. J. Proteomics 74, 2228–2242.
Møller, I. M., and Sweetlove, L. J. (2010). ROS signalling – specificity is required. Trends Plant Sci. 15, 370–374.
Murphy, M. P. (2012). Mitochondrial thiols in antioxidant protection and redox signaling: distinct roles for glutathionylation and other thiol modifications. Antioxid. Redox. Signal. 16, 476–495.
Nakagami, H., Sugiyama, N., Mochida, K., Daudi, A., Yoshida, Y., Toyoda, T., et al. (2010). Large-scale comparative phosphoproteomics identifies conserved phosphorylation sites in plants. Plant Physiol. 153, 1161–1174.
Oliver, D. J., Nikolau, B. J., and Wurtele, E. S. (2009). Acetyl-CoA–-life at the metabolic nexus. Plant Sci. 176, 597–601.
Ozden, O., Park, S. H., Kim, H. S., Jiang, H., Coleman, M. C., Spitz, D. R., et al. (2011). Acetylation of MnSOD directs enzymatic activity responding to cellular nutrient status or oxidative stress. Aging (Albany NY) 3, 102–107.
Pagliarini, D. J., and Dixon, J. E. (2006). Mitochondrial modulation: reversible phosphorylation takes center stage? Trends Biochem. Sci. 31, 26–34.
Palmieri, M. C., Lindermayr, C., Bauwe, H., Steinhauser, C., and Durner, J. (2010). Regulation of plant glycine decarboxylase by s-nitrosylation and glutathionylation. Plant Physiol. 152, 1514–1528.
Pandey, R., Müller, A., Napoli, C., Selinger, D., Pikaard, C. S., Richards, E., et al. (2002). Analysis of histone acetyltransferase and histone deacetylase families of Arabidopsis thaliana suggests functional diversification of chromatin modification among multicellular eukaryotes. Nucleic Acids Res. 30, 5036–5055.
Polge, C., and Thomas, M. (2007). SNF1/AMPK/SnRK1 kinases, global regulators at the heart of energy control? Trends Plant Sci. 12, 20–28.
Ren, M., Qiu, S., Venglat, P., Xiang, D., Feng, L., Selvaraj, G., et al. (2011). Target of rapamycin regulates development and ribosomal RNA expression through kinase domain in Arabidopsis. Plant Physiol. 155, 1367–1382.
Rhoads, D. M. (2011). “Plant mitochondrial retrograde regulation,” in Plant Mitochondria, ed. F. Kempken (New York: Springer), 411–437.
Rhoads, D. M., and Subbaiah, C. C. (2007). Mitochondrial retrograde regulation in plants. Mitochondrion 7, 177–194.
Rouhier, N., Villarejo, A., Srivastava, M., Gelhaye, E., Keech, O., Droux, M., et al. (2005). Identification of plant glutaredoxin targets. Antioxid. Redox. Signal. 7, 919–929.
Schieke, S. M., and Finkel, T. (2006). Mitochondrial signaling, TOR, and life span. Biol. Chem. 387, 1357.
Schwarzländer, M., König, A. C., Sweetlove, L. J., and Finkemeier, I. (2012). The impact of impaired mitochondrial function on retrograde signalling: a meta-analysis of transcriptomic responses. J. Exp. Bot. 63, 1735–1750.
Schwer, B., Bunkenborg, J., Verdin, R. O., Andersen, J. S., and Verdin, E. (2006). Reversible lysine acetylation controls the activity of the mitochondrial enzyme acetyl-CoA synthetase 2. Proc. Natl. Acad. Sci. U.S.A. 103, 10224–10229.
Scott, I., Webster, B. R., Li, J. H., and Sack, M. N. (2012). Identification of a molecular component of the mitochondrial acetyltransferase programme: a novel role for GCN5L1. Biochem. J. 443, 655–661.
Servet, C., Conde E Silva, N., Zhou, D.-X., and Conde, N. (2010). Histone acetyltransferase AtGCN5/HAG1 is a versatile regulator of developmental and inducible gene expression in Arabidopsis. Mol. Plant 3, 670–677.
Singh, R., Lee, M.-O., Lee, J.-E., Choi, J., Park, J. H., Kim, E. H., et al. (2012). Rice mitogen-activated protein kinase interactome analysis using the yeast two-hybrid system. Plant Physiol. 160, 477–487.
Sun, F., Carrie, C., Law, S., Murcha, M. W., Zhang, R., Law, Y. S., et al. (2012). AtPAP2 is a tail-anchored protein in the outer membrane of chloroplasts and mitochondria. Plant Signal. Behav. 7, 927–932.
Sweetlove, L. J., Fait, A., Nunes-Nesi, A., Williams, T., and Fernie, A. R. (2007). The mitochondrion: an integration point of cellular metabolism and signalling. Crit. Rev. Plant Sci. 26, 17–43.
Sweetlove, L. J., Heazlewood, J. L., Herald, V., Holtzapffel, R., Day, D. A., Leaver, C. J., et al. (2002). The impact of oxidative stress on Arabidopsis mitochondria. Plant J. 32, 891–904.
Takahashi, Y., Berberich, T., Miyazaki, A., Seo, S., Ohashi, Y., and Kusano, T. (2003). Spermine signalling in tobacco: activation of mitogen-activated protein kinases by spermine is mediated through mitochondrial dysfunction. Plant J. 36, 820–829.
Tran, H. T., Nimick, M., Uhrig, G., Templeton, G., Morrice, N., Gourlay, R., et al. (2012). Arabidopsis thaliana histone deacetylase 14 (HDA14) is an α-tubulin deacetylase that associates with PP2a and enriches in the microtubule fraction with the putative histone acetyltransferase ELP3. Plant J. 71, 263-272.
van Noort, V., Seebacher, J., Bader, S., Mohammed, S., Vonkova, I., Betts, M. J., et al. (2012). Cross-talk between phosphorylation and lysine acetylation in a genome-reduced bacterium. Mol. Syst. Biol. 8, 571.
Verdin, E., Hirschey, M. D., Finley, L. W. S., and Haigis, M. C. (2010). Sirtuin regulation of mitochondria: energy production, apoptosis, and signaling. Trends Biochem. Sci. 35, 669–675.
Wang, Q., Zhang, Y., Yang, C., Xiong, H., Lin, Y., Yao, J., et al. (2010). Acetylation of metabolic enzymes coordinates carbon source utilization and metabolic flux. Science 327, 1004–1007.
Winger, A. M., Taylor, N. L., Heazlewood, J. L., Day, D. A., and Millar, A. H. (2007). Identification of intra- and intermolecular disulphide bonding in the plant mitochondrial proteome by diagonal gel electrophoresis. Proteomics 7, 4158–4170.
Witze, E. S., Old, W. M., Resing, K. A., and Ahn, N. G. (2007). Mapping protein post-translational modifications with mass spectrometry. Nat. Methods 4, 798–806.
Wu, K., Zhang, L., Zhou, C., Yu, C.-W., and Chaikam, V. (2008). HDA6 is required for jasmonate response, senescence and flowering in Arabidopsis. J. Exp. Bot. 59, 225–234.
Wu, X., Oh, M.-H., Schwarz, E., Larue, C. T., Sivaguru, M., Imai, B. S., et al. (2011). Lysine acetylation is a widespread protein modification for diverse proteins in Arabidopsis. Plant Physiol. 155, 1769–1778.
Xing, S., and Poirier, Y. (2012). The protein acetylome and the regulation of metabolism. Trends Plant Sci. 1–8.
Xiong, Y., and Sheen, J. (2012). Rapamycin and glucose-target of rapamycin (TOR) protein signaling in plants. J. Biol. Chem. 287, 2836–2842.
Yamakura, F., Taka, H., Fujimura, T., and Murayama, K. (1998). Inactivation of human manganese-superoxide dismutase by peroxynitrite is caused by exclusive nitration of tyrosine 34 to 3-nitrotyrosine. J. Biol. Chem. 273, 14085–14089.
Yu, C.-W., Liu, X., Luo, M., Chen, C., Lin, X., Tian, G., et al. (2011). HISTONE DEACETYLASE6 interacts with FLOWERING LOCUS D and regulates flowering in Arabidopsis. Plant Physiol. 156, 173–184.
Zhao, X., León, I. R., Bak, S., Mogensen, M., Wrzesinski, K., Højlund, K., et al. (2011). Phosphoproteome analysis of functional mitochondria isolated from resting human muscle reveals extensive phosphorylation of inner membrane protein complexes and enzymes. Mol. Cell. Proteomics 10, M110.000299.
Keywords: plants, mitochondria, retrograde signaling, post-translational modifications, metabolites
Citation: Hartl M and Finkemeier I (2012) Plant mitochondrial retrograde signaling: post-translational modifications enter the stage. Front. Plant Sci. 3:253. doi: 10.3389/fpls.2012.00253
Received: 23 September 2012; Accepted: 22 October 2012;
Published online: 12 November 2012.
Edited by:
Dario Leister, Ludwig Maximilians University Munich, GermanyReviewed by:
Steven Huber, United States Department of Agriculture - Agricultural Research Service, USAIan Max Møller, Aarhus University, Denmark
Copyright: © 2012 Hartl and Finkemeier. This is an open-access article distributed under the terms of the Creative Commons Attribution License, which permits use, distribution and reproduction in other forums, provided the original authors and source are credited and subject to any copyright notices concerning any third-party graphics etc.
*Correspondence: Iris Finkemeier, Department Biology I, Ludwig Maximilians University Munich, Grosshaderner Strasse 2-4, 82152 Planegg-Martinsried, Germany. e-mail: i.finkemeier@lmu.de