- Lady Davis Institute for Medical Research, Jewish General Hospital, and Department of Medicine, McGill University, Montreal, QC, Canada
Iron regulatory proteins 1 and 2 (IRP1 and IRP2) post-transcriptionally control the expression of several mRNAs encoding proteins of iron, oxygen and energy metabolism. The mechanism involves their binding to iron responsive elements (IREs) in the untranslated regions of target mRNAs, thereby controlling mRNA translation or stability. Whereas IRP2 functions solely as an RNA-binding protein, IRP1 operates as either an RNA-binding protein or a cytosolic aconitase. Early experiments in cultured cells established a crucial role of IRPs in regulation of cellular iron metabolism. More recently, studies in mouse models with global or localized Irp1 and/or Irp2 deficiencies uncovered new physiological functions of IRPs in the context of systemic iron homeostasis. Thus, IRP1 emerged as a key regulator of erythropoiesis and iron absorption by controlling hypoxia inducible factor 2α (HIF2α) mRNA translation, while IRP2 appears to dominate the control of iron uptake and heme biosynthesis in erythroid progenitor cells by regulating the expression of transferrin receptor 1 (TfR1) and 5-aminolevulinic acid synthase 2 (ALAS2) mRNAs, respectively. Targeted disruption of either Irp1 or Irp2 in mice is associated with distinct phenotypic abnormalities. Thus, Irp1−/− mice develop polycythemia and pulmonary hypertension, while Irp2−/− mice present with microcytic anemia, iron overload in the intestine and the liver, and neurologic defects. Combined disruption of both Irp1 and Irp2 is incombatible with life and leads to early embryonic lethality. Mice with intestinal- or liver-specific disruption of both Irps are viable at birth but die later on due to malabsorption or liver failure, respectively. Adult mice lacking both Irps in the intestine exhibit a profound defect in dietary iron absorption due to a “mucosal block” that is caused by the de-repression of ferritin mRNA translation. Herein, we discuss the physiological function of the IRE/IRP regulatory system.
Principles of Mammalian Iron Metabolism
Iron is a vital micronutrient and constituent of several metalloproteins (Aisen et al., 2001). Even though it may acquire many oxidation states (from −2 to +6), within cells iron commonly alternates between the ferrous Fe(II) and ferric Fe(III) forms. Because of its abilities to coordinate with proteins and to act as both an electron donor and an electron acceptor, iron has been utilized throughout evolution by almost all living cells and organisms for metabolic purposes. Thus, iron is an integral component in: oxygen transport, through the heme moiety of hemoglobin; cellular respiration, as part of heme-containing cytochromes and Fe-S cluster-containing proteins of the electron transport chain (ETC); DNA synthesis and cellular growth, as part of the M2 subunit of ribonucleotide reductase.
As essential as iron is to survival, it can also be toxic. “Free” iron engages in Fenton chemistry to catalyze the production of hydroxyl radicals from superoxide and hydrogen peroxide (Papanikolaou and Pantopoulos, 2005). These are extremely reactive species that can damage lipid membranes, proteins and nucleic acids within a cell. To avoid the deleterious effects of the Fenton reaction, iron has to be shielded. Circulating iron is oxidized to Fe(III) and tightly binds to transferrin (Tf), which maintains it in a redox-inert state and delivers it to tissues (Gkouvatsos et al., 2012). Cellular iron uptake involves the binding of iron-loaded Tf to transferrin receptor 1 (TfR1) and the internalization of the complex by endocytosis (Aisen, 2004). Within endosomes, Fe(III) is released from Tf following acidification, and undergoes reduction to Fe(II). Subsequently, Fe(II) crosses the endosomal membrane via the divalent metal transporter 1 (DMT1). Internalized iron is mostly utilized in mitochondria for synthesis of heme and Fe-S clusters, and in the cytosol for incorporation into metalloproteins, while excess of iron is stored and detoxified within ferritin (Arosio et al., 2009). Ferritin is ubiquitously expressed in the cytosol of cells. It is comprised of 24 H- and L-polypeptide subunits that form a shell-like nanocage for iron storage, with a diameter of 7–8 nm. This can accommodate up to 4500 iron atoms, which are safely stored in the form of ferric oxy-hydroxide phosphate, following oxidation of Fe(II) to Fe(III) at the ferroxidase center of the H-ferritin subunit. In addition, a distinct ferritin isoform (M-ferritin) is expressed in mitochondria of some cell types, such as testicular Leydig cells, neuronal cells and pancreatic islets of Langherans (Levi and Arosio, 2004).
The iron content of the adult human body ranges between 3 and 5 g (Gkouvatsos et al., 2012). Most of it (~70%) is utilized in hemoglobin of red blood cells (RBCs) in the bloodstream and of erythroid progenitor cells in the bone marrow, and is recycled by tissue macrophages at a rate of 25–30 mg/day (Figure 1). A substantial amount of body iron (up to 1 g) is stored within ferritin in the liver. Muscles contain ~300 mg of iron (mostly in myoglobin), and all other tissues (excluding the duodenum) merely ~8 mg. Circulating Tf-bound iron represents a small (~3 mg) but dynamic fraction that turns over ~10 times per day (Cavill, 2002). The Tf iron pool is primarily replenished by iron from senescent RBCs that is recycled via macrophages, and to a smaller extent by iron absorbed from the diet via duodenal enterocytes. In adults, dietary iron absorption (1–2 mg/day) serves to compensate for non-specific iron losses (by desquamation or bleeding).
Iron metabolism is regulated at both the systemic and cellular levels. Systemic iron homeostasis is controlled through hepcidin, a liver-derived peptide hormone (Ganz, 2013). Iron and other stimuli (inflammation, endoplasmic reticulum stress) positively regulate hepcidin transcription, while erythropoietic drive suppresses it. Hepcidin binds to the cellular iron exporter ferroportin expressed on enterocytes, macrophages, and hepatocytes causing ferroportin's subsequent internalization and degradation (Nemeth et al., 2004). Ergo hepcidin functions to control iron absorption within the enterocytes and the efflux of iron into the bloodstream from macrophages and other iron exporting cells (Figure 2). Misregulation of hepcidin is associated with disease (hemochromatosis, when iron-regulation of hepcidin is blunted, and anemia, when hepcidin is induced by inflammatory pathways or by genetic inactivation of its inhibitor TMPRSS6) (Sebastiani and Pantopoulos, 2011). Cellular iron metabolism is controlled through the IRE/IRP system (Wang and Pantopoulos, 2011; Joshi et al., 2012), which is the focus of this review.
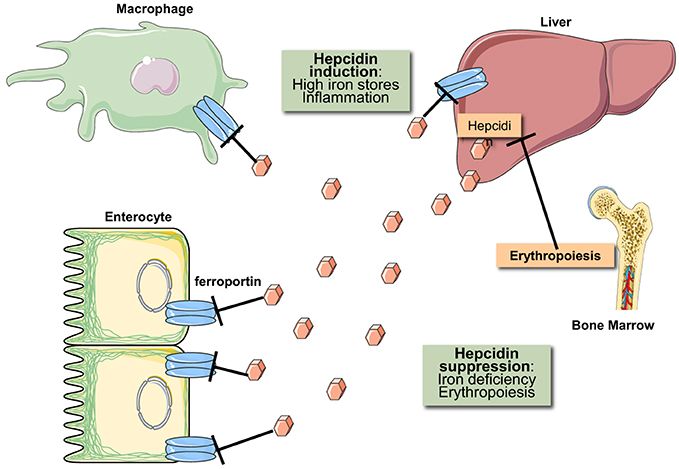
Figure 2. Hormonal regulation of iron efflux from reticuloendothelial macrophages and duodenal enterocytes. The iron regulatory hormone hepcidin is secreted by liver hepatocytes and targets the iron transporter ferroportin on the plasma membrane of iron-exporting cells. The binding of hepcidin promotes ferroportin degradation in lysosomes. Hepcidin expression is induced in response to high iron stores and inflammatory signals. Conversely, hepcidin expression is suppressed in response to iron deficiency and increased erythropoietic drive.
Coordinate Post-Transcriptional Regulation of Cellular Iron Metabolism Via IRE/IRP Interactions
The IRE/IRP regulatory system was first described in the late 1980s with the discovery of iron responsive elements (IREs) in the untranslated regions (UTRs) of the mRNAs encoding ferritin (both H- and L-subunits) and TfR1 (Hentze et al., 1987; Casey et al., 1988; Müllner and Kühn, 1988); see Figure 3. IREs are highly conserved hairpin structures of 25–30 nucleotides (Piccinelli and Samuelsson, 2007). They contain a stem that is stabilized by base pairing, and a loop with the sequence 5′-CAGUGH-3′ (H denotes A, C or U). The stem is interrupted to an upper and lower part by an unpaired C residue or an asymmetric UGC/C bulge/loop. H- and L-ferritin mRNAs contain a single IRE in their 5′ UTR, which is located relatively close to the cap structure at the 5′ end. TfR1 mRNA contains five IREs in its 3′ UTR.
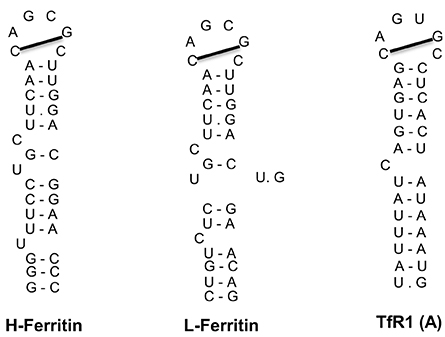
Figure 3. IRE motifs in the untranslated regions of the mRNAs encoding human H-ferritin, L-ferritin and TfR1 (only the A IRE of TfR1 mRNA is shown). Watson-Crick base pairing is depicted by a dash and non-Watson-Crick interactions by a dot. The line connecting the 1st and 5th nucleotide of the IRE loop indicates Watson-Crick base pairing.
The IREs constitute binding sites of two cytoplasmic iron regulatory proteins, IRP1 and IRP2 (Rouault, 2006). Under conditions of iron deficiency, IRP1 and IRP2 bind with high affinity to the IRE in H- and L-ferritin mRNAs, and thereby inhibit their translation by a steric hindrance mechanism. Likewise, they bind to the IREs in TfR1 mRNA and thereby protect it against endonucleolytic degradation. This homeostatic response mediates increased cellular iron uptake from Tf and prevents storage of the metal. By contrast, in iron-replete cells the IRE-binding activities of IRP1 and IRP2 are diminished, allowing TfR1 mRNA degradation and ferritin mRNA translation. This inhibits further iron uptake and stimulates storage of excessive intracellular iron within ferritin. A scheme with the coordinate post-transcriptional regulation of ferritin and TfR1 by IRE/IRP interactions is shown in Figure 4.
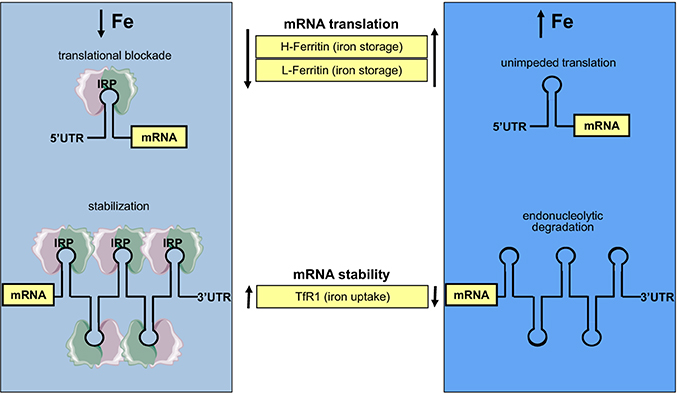
Figure 4. Coordinate iron-dependent regulation of ferritin and TfR1 mRNA expression by IRE/IRP interactions. Iron deficiency promotes the binding of IRPs to cognate IREs in the untranslated regions of ferritin and TfR1 mRNAs. These inhibit ferritin mRNA translation and stabilize TfR1 mRNA against endonucleolytic degradation. In iron-replete cells, IRPs do not bind to IREs, allowing ferritin mRNA translation and TfR1 mRNA degradation.
Additional IRE-containing mRNAs (Figure 5) have been discovered by computational and biochemical approaches, as well as high throughput screens (Dandekar and Hentze, 1995; Gunshin et al., 1997; McKie et al., 2000; Sanchez et al., 2006, 2007, 2011). These include the mRNAs encoding the iron transporters DMT1 and ferroportin, the enzyme 5-aminolevulinic acid synthase 2 (ALAS2) that catalyzes the first reaction for heme biosynthesis in erythroid progenitor cells, the enzyme of the citric acid cycle mitochondrial aconitase, the cell cycle regulator CDC14A, and hypoxia inducible factor 2 alpha (HIF2α), a transcription factor that orchestrates molecular responses to hypoxia. All these transcripts harbor a single IRE. The IRE of ferroportin, ALAS2, mitochondrial aconitase and HIF2α mRNAs is localized in the 5′ UTR and functions as a translational control element, analogous to ferritin IRE. The IRE of DMT1 and CDC14A mRNAs is localized in the 3′ UTR and appears to function as an mRNA stability element. Post-transcriptional regulation of DMT1 expression by the IRE/IRP system is cell-specific (Gunshin et al., 2001) and depends, at least partially, on an alternatively transcribed upstream exon at the 5′ end of DMT1 mRNA (Hubert and Hentze, 2002). Notably, earlier experiments showed that the presence of at least three 3′ UTR IREs is essential for iron-dependent regulation of TfR1 mRNA (Casey et al., 1989), which remains the only transcript containing multiple IREs. It should also be noted that DMT1, ferroportin and CDC14A mRNAs include additional non-IRE-containing isoforms, which are generated by alternative splicing and exhibit differential tissue distribution (Hubert and Hentze, 2002; Sanchez et al., 2006; Zhang et al., 2009).
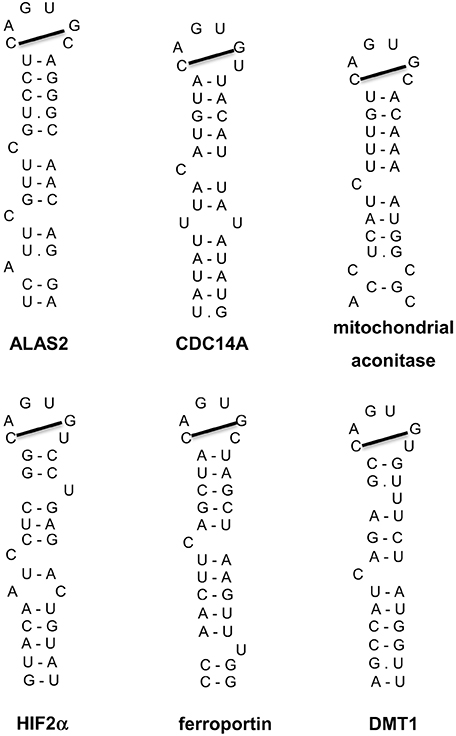
Figure 5. IRE motifs in the untranslated regions of the mRNAs encoding human ALAS2, CDC14A, mitochondrial aconitase, HIF2α, ferroportin, and DMT1. Watson-Crick base pairing is depicted by a dash and non-Watson-Crick interactions by a dot. The line connecting the 1st and 5th nucleotide of the IRE loop indicates Watson-Crick base pairing.
Another level of complexity is added by the fact that iron regulation of HIF2α, ferroportin and DMT1 integrates multiple and often opposing signals (Mastrogiannaki et al., 2013; Shah and Xie, 2014). Thus, while iron stimulates HIF2α and ferroportin mRNA translation, it also promotes the degradation of HIF2α via iron- and oxygen-dependent prolyl hydroxylases (PHDs) and the von Hippel Lindau E3 ubiquitin ligase (pVHL) (Schofield and Ratcliffe, 2004), and the degradation of ferroportin via hepcidin (Ganz, 2013). Conversely, iron deficiency inhibits translation of HIF2α and ferroportin mRNAs but promotes stabilization of the proteins. On the other hand, iron deficiency leads to HIF2α-mediated transcriptional activation of duodenal DMT1 and ferroportin, the apical and basolateral transporters of iron in enterocytes (see below).
Sensing of Intracellular Iron by IRP1 and IRP2
IRP1 and IRP2 are homologous to mitochondrial aconitase (Rouault, 2006; Wang and Pantopoulos, 2011). A characteristic feature of this enzyme is the presence of a 4Fe-4S cluster within its active site, which is indispensable for catalytic isomerization of citrate to iso-citrate. IRP1 assembles an aconitase-type 4Fe-4S cluster in response to increased intracellular iron levels. The complex pathway involves several co-factors, such as Nfs1 (ISCS), frataxin, ISCU, glutaredoxin 5 (GLRX5) and others. Assembly of the 4Fe-4S cluster alters the conformation of IRP1 and precludes IRE-binding (Dupuy et al., 2006; Walden et al., 2006). At the same time holo-IRP1 (containing the 4Fe-4S cluster) acquires enzymatic activity as cytosolic aconitase, which is comparable to that of its mitochondrial counterpart. Iron starvation, as well as other signals, such as nitric oxide (NO) or hydrogen peroxide (H2O2), promotes the loss of the 4Fe-4S cluster and conversion of apo-IRP1 to an IRE-binding protein (Haile et al., 1992; Drapier et al., 1993; Weiss et al., 1993; Pantopoulos and Hentze, 1995). Thus, IRP1 is a bifunctional protein that is regulated by an unusual 4Fe-4S cluster switch. Holo-IRP1 is stabilized by hypoxia and at oxygen concentrations ranging between 3 and 6%, which reflect physiological tissue oxygenation (Meyron-Holtz et al., 2004b). Consistently with this notion, IRP1 predominates in the cytosolic aconitase form within tissues, but a fraction of apo-IRP1 is readily detectable by virtue of its IRE-binding activity (Meyron-Holtz et al., 2004a).
Contrary to IRP1, IRP2 does not bind an iron-sulfur cluster and is regulated at the level of protein stability. IRP2 accumulates in iron-starved and/or hypoxic cells, and undergoes proteasomal degradation in iron-replete oxygenated cells. Mechanistically, this involves ubiquitination of IRP2 by an E3 ubiquitin ligase complex consisting of the F-box protein FBXL5 and the auxiliary proteins SKP1, CUL1, and RBX1 (Salahudeen et al., 2009; Vashisht et al., 2009). FBXL5 exhibits genuine iron-sensing properties, as it is stabilized in the presence of iron and oxygen by forming a Fe-O-Fe center within its N-terminal hemerythrin-like domain. Loss of this center in iron-starved and/or hypoxic cells exposes a degron via a conformational change (Chollangi et al., 2012; Thompson et al., 2012). This allows proteasomal degradation of FBXL5, which leads to concomitant accumulation of IRP2. Interestingly, FBXL5 can also promote the ubiquitination and degradation of IRP1 mutants that cannot form a 4Fe-4S cluster (Salahudeen et al., 2009; Vashisht et al., 2009). Proteasomal degradation of wild type apo-IRP1 under conditions of impairment of the iron-sulfur cluster assembly pathway has been proposed to operate as a reserve mechanism to control the IRE-binding activity of IRP1 (Clarke et al., 2006; Wang et al., 2007).
Human Diseases Linked to the IRE/IRP System
Mutations in the IRE of L-ferritin mRNA that abrogate IRP binding are causatively linked to the hereditary hyperferritinemia-cataract syndrome (HHCS) (Beaumont et al., 1995). The hallmark of this disorder is overexpression of serum ferritin (up to 20-fold) in the absence of systemic iron overload or inflammation (Yin et al., 2014). Patients also have an increased tendency to develop bilateral cataract, which is possibly caused by accumulation of non-functional L-ferritin homopolymers in the lens (Levi et al., 1998). HHCS is transmitted in an autosomal dominant manner. Several HHCS-associated mutations in L-ferritin IRE have been described (Luscieti et al., 2013). Mutations affecting the loop structure or the C bulge result in the highest levels of serum ferritin and increased severity of cataract when compared with mutations in the stem structure of the IRE (Cazzola et al., 1997). Overall, the severity of the clinical phenotype appears to correlate with the degree of inhibition in IRP binding (Allerson et al., 1999). Nevertheless, the involvement of additional factors (genetic, environmental, inflammatory) has not been excluded (Roetto et al., 2002).
A point mutation (A49U) in the loop of H-ferritin IRE has been associated with an autosomal dominant iron overload disorder in a Japanese family (Kato et al., 2001). Iron deposits were documented primarily in hepatocytes, but also in some Kupffer cells. The A49U mutation increased the affinity of IRPs for H-ferritin IRE and suppressed H-ferritin expression in cultured cells (Kato et al., 2001). Nevertheless, it remains unclear how this response promotes iron overload. The lack of follow-up studies to support the validity of these findings should also be noted.
Disruption of the zebrafish glrx5 gene, encoding glutaredoxin 5, leads to constitutive activation of IRP1 for IRE-binding, due to defective assembly of its 4Fe-4S cluster (Wingert et al., 2005). In a case report, a patient with GLRX5 deficiency developed a sideroblastic-like anemia with microcytosis and systemic iron overload (Camaschella et al., 2007). This disease is caused by IRP1-mediated suppression of ALAS2 mRNA translation, which results in impaired heme biosynthesis. A secondary effect is the depletion of cytosolic iron and the development of mitochondrial iron overload (Ye et al., 2010).
The above examples represent the only documented human disorders that are causatively linked to defects in the IRE/IRP system (Table 1). Genome-wide association studies (GWAS) showed that the IRP2 gene (IREB2) confers susceptibility to chronic obstructive pulmonary disease (COPD) (Demeo et al., 2009; Chappell et al., 2011; Zhou et al., 2012) and lies within a lung cancer-associated locus (Hansen et al., 2010; Cho et al., 2012; Fehringer et al., 2012). Apart from the identification of single nucleotide polymorphisms (SNPs) in IREB2, it was shown that COPD patients exhibit increased IRP2 mRNA and protein expression (Demeo et al., 2009) and it was speculated that this may contribute to iron accumulation in the lungs. IREB2 polymorphisms have also been associated with Alzheimer's disease (Coon et al., 2006). Interestingly, biochemical studies showed that IRP1 selectively regulates translation of amyloid precursor protein (APP) mRNA by binding to an atypical putative IRE motif (Cho et al., 2010), which may provide another connection of the IRE/IRP system with Alzheimer's disease. The IRP1 gene (ACO1) has been associated with cutaneous malignant melanoma (Yang et al., 2010) and neuropathic pain in HIV-infected patients (Kallianpur et al., 2014), while the IRE-binding activity of IRP1 was reported to be increased in Friedreichs' ataxia (Lobmayr et al., 2005) and in Parkinson's disease (Faucheux et al., 2002). Finally, polymorphisms in both ACO1 and IREB2 have been linked to age-related macular degeneration (Synowiec et al., 2012). GWAS data with ACO1 and IREB2 are summarized in Table 2. The molecular mechanisms by which IRPs may contribute to pathogenesis of the above disorders remain to be established.
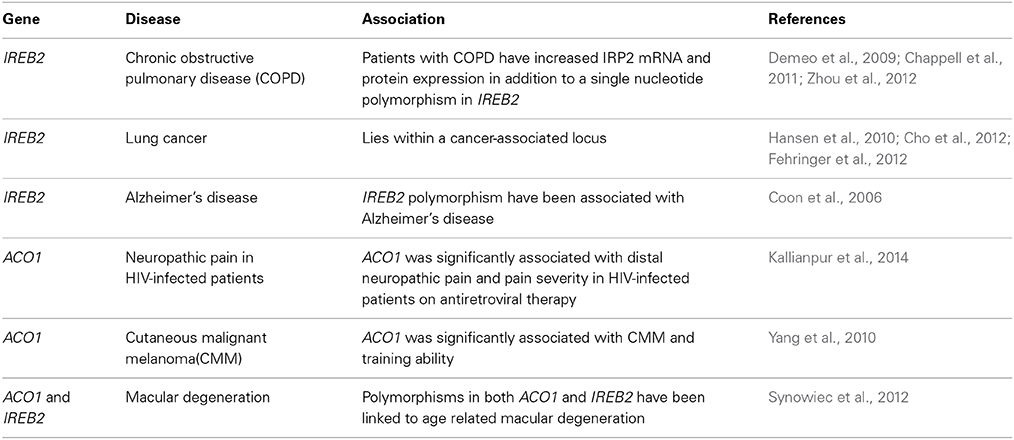
Table 2. Genome-wide association studies (GWAS) with the IRPI and IRP2 genes, ACO1 and IREB2, respectively.
Mouse Models with Ubiquitous Aberration in the IRE/IRP System
Mice with ubiquitous ablation of both Irp1 and Irp2 cannot be generated because the embryos do not survive the blastocyst stage, possibly due to misregulation of iron metabolism (Smith et al., 2006). These data highlight the physiological significance of the IRE/IRP system in early development. On the other hand, single Irp1−/− or Irp2−/− mice are viable. At first glance, this suggests that Irp1 and Irp2 share overlapping functions in vivo. Nonetheless, Irp1−/− and Irp2−/− mice manifest distinct phenotypes, indicating variable target specificity. The phenotypic features of current mouse models with global or tissue-specific “loss” or “gain” of Irp1 and/or Irp2 functions are summarized in Table 3.
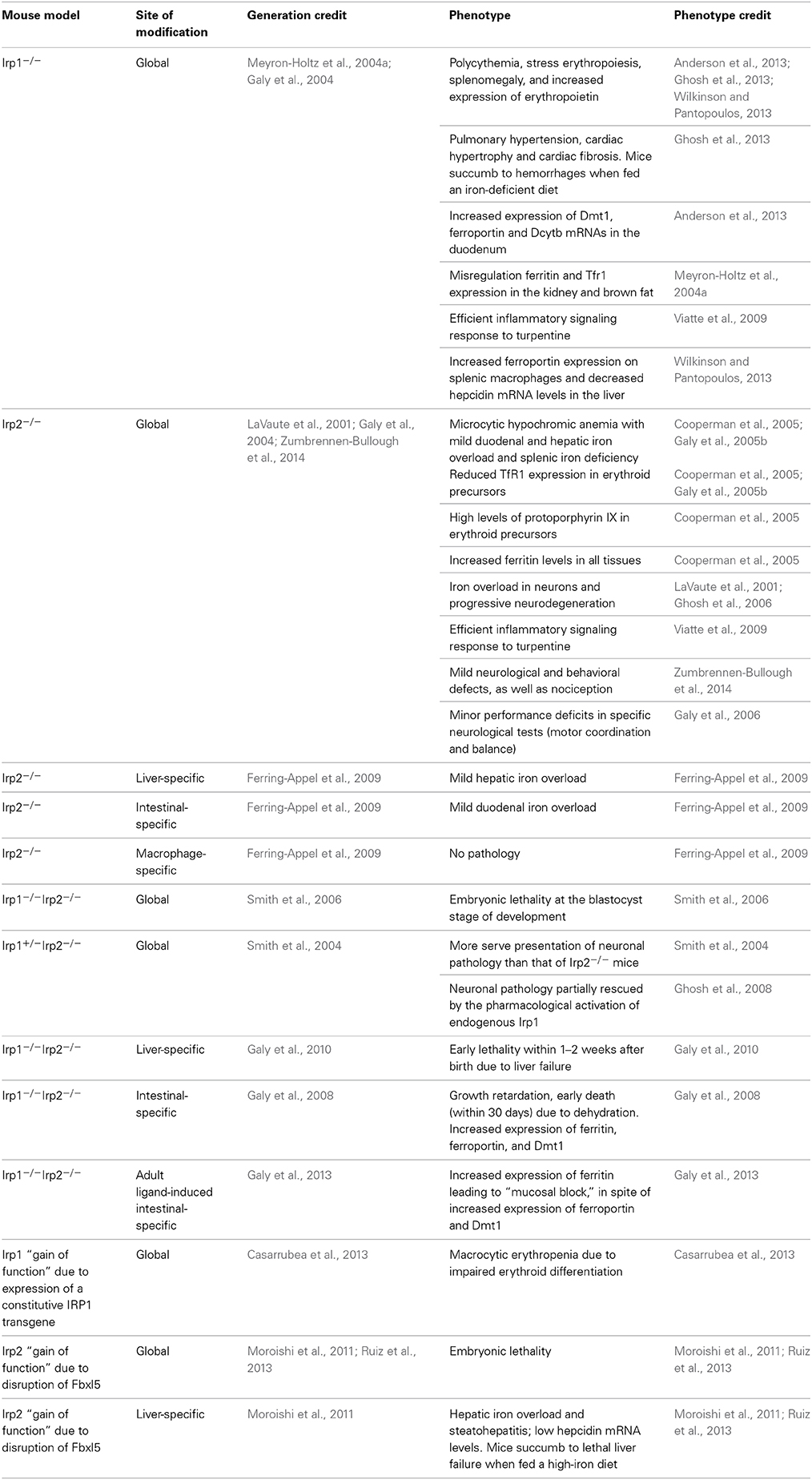
Table 3. Phenotypic features of mouse models with global or tissue-specific “loss” or “gain” of Irp1 and/or Irp2 functions.
Irp1−/− mice were initially reported to lack any overt abnormalities (Meyron-Holtz et al., 2004a; Galy et al., 2005a). Because they merely misregulated ferritin and TfR1 expression in the kidney and the brown fat, tissues with the highest abundance of IRP1, it was proposed that Irp2 dominates cellular iron metabolism in tissues, with Irp1 having an auxiliary physiological function (Meyron-Holtz et al., 2004a). In addition, Irp1−/− (and Irp2−/−) mice did not exhibit any defects when challenged with turpentine to induce an inflammatory response (Viatte et al., 2009), despite the fact that IRPs are modulated in cultured cells by inflammatory reactive species such as H2O2 and NO (Weiss et al., 1993; Pantopoulos and Hentze, 1995; Wang et al., 2005; Hausmann et al., 2011).
Recently, Irp1−/− mice were documented to develop polycythemia and pathological iron metabolism due to stress erythropoiesis, as well as pulmonary hypertension and cardiac hypertrophy and fibrosis (Anderson et al., 2013; Ghosh et al., 2013; Wilkinson and Pantopoulos, 2013). These phenotypes are attributed to relief of translational suppression of Hif2α mRNA, which leads to transcriptional activation of the downstream Hif2α targets erythropoietin and endothelin-1. Thus, murine Irp1 operates as specific regulator of the Hif2α IRE, in agreement with previous in vitro data (Zimmer et al., 2008). Irp1−/− mice exhibit high mortality when placed on an iron-deficient diet, which is known to stabilize Hif2α, due to abdominal hemorrhages (Ghosh et al., 2013). When Irp1−/− mice are fed with a standard diet, polycythemia attenuates after the 10th week of age (Wilkinson and Pantopoulos, 2013), possibly due to enhanced Hif2α degradation by the pVHL pathway. This may explain why their pathology escaped attention in the past.
Irp2−/− mice develop microcytic hypochromic anemia and erythropoietic protoporphyria, associated with relatively mild duodenal and hepatic iron overload and splenic iron deficiency (Cooperman et al., 2005; Galy et al., 2005b). They exhibit a low Tfr1 content and express high levels of protoporphyrin IX in erythroid precursor cells. A closer look on the role of Irp1 and Irp2 in erythropoiesis, duodenal iron absorption and systemic iron metabolism will be provided in the next sections.
Global Irp2−/− deficiency has also been associated with progressive neurodegeneration, loss of Purkinje neurons and iron overload in white matter areas of the brain (LaVaute et al., 2001; Ghosh et al., 2006), with more severe presentation in Irp1 haploinsufficient Irp1+/−Irp2−/− mice (Smith et al., 2004). The neuronal pathology of Irp2−/− mice can be partially rescued by pharmacological activation of endogenous Irp1 for IRE-binding (Ghosh et al., 2008). Mechanistically, the pathology may be caused by functional iron deficiency in neuronal cells due to de-repression of ferritin mRNA translation, which could result in enhanced iron storage and reduced availability for metabolic purposes (Jeong et al., 2011). This scenario is reminiscent of neuroferritinopathy, a neurodegenerative disease caused by expression of mutant L-ferritin (Burn and Chinnery, 2006). Suppression of Tfr1 and enhancement of ferroportin expression in Irp2−/− neurons could also contribute to pathology. Nevertheless, isogenic Irp2−/− mice generated by another strategy did not develop any signs of neurodegeneration but rather manifested minor performance deficits in specific neurological tests (motor coordination and balance) (Galy et al., 2006). A third independent Irp2−/− mouse strain was recently generated and analyzed, corroborating the abnormalities in erythropoiesis and brain iron metabolism of these animals; the latter were linked to mild neurological and behavioral defects, as well as nociception (Zumbrennen-Bullough et al., 2014). The discrepancies in the phenotypes of the three Irp2−/− mouse lines may be related to the targeting strategies and their impact on flanking genomic sequences, genetic factors, and possibly also environmental factors.
Ubiquitous Irp1 “gain of function” mice were generated by the transgenic expression of a constitutively active IRP1 mutant from the Rosa26 locus (Casarrubea et al., 2013). Expression of the transgene was relatively low and the mice developed erythropoietic abnormalities (macrocytic erythropenia due to impaired erythroid differentiation).
The targeted disruption of Fbxl5 could yield ubiquitous Irp2 “gain of function” mice. Nevertheless, Fbxl5−/− mice are not viable and die during embryogenesis (Moroishi et al., 2011; Ruiz et al., 2013). This phenotype is rescued by concomitant disruption of Irp2, but not Irp1, indicating that the major physiological function of Fbxl5 is to regulate Irp2.
The IRE/IRP System in Erythropoiesis
Most iron in the body is utilized in erythropoiesis, a process that occurs mainly in the bone marrow of humans and in the bone marrow and spleen of mice (Cavill, 2002). Erythroid precursor cells express high levels of TfR1 and consume the majority of Tf-bound iron for the production of heme, the oxygen-binding moiety of hemoglobin (Ponka, 1997). An adult human produces about 2 million RBCs per second. Increased erythropoietic drive is known to stimulate iron absorption (Finch, 1994). This is mediated by suppression of hepcidin expression, which allows increased iron efflux to the bloodstream from duodenal enterocytes and tissue macrophages (Ganz, 2013). During iron deficiency, erythroid precursor cells will have priority for iron utilization, for the production of RBCs, over cells in other tissues (Finch, 1994). Maturation of erythroid cell is induced by erythropoeitin (EPO), a circulating glycoprotein hormone that prevents apoptosis of erythroid progenitor cells (Koury and Bondurant, 1990). EPO is primarily synthesized in peritubular interstitial fibroblasts of the kidney, and to a smaller extent in hepatocytes of the liver. Murine Epo is transcriptionally activated during hypoxemia by Hif2α (Rankin et al., 2007; Kapitsinou et al., 2010), while Hif2α indirectly suppresses hepcidin expression by stimulating erythropoiesis via Epo (Liu et al., 2012; Mastrogiannaki et al., 2012). There are several points where the IRE/IRP system regulates erythropoiesis.
First, the phenotype of Irp1−/− mice demonstrates that Irp1 operates as a key upstream regulator of Hif2α expression at the level of mRNA translation (Anderson et al., 2013; Ghosh et al., 2013; Wilkinson and Pantopoulos, 2013). Essentially, these animals exhibit features of Hif2α overexpression, which are also manifested in patients with Chuvash polycythemia (Ang et al., 2002), and other forms of familial polycythemia caused by “gain of function” HIF2α mutations (Percy et al., 2008), or inactivating mutations in pVHL, a negative regulator of HIF2α stability (Percy et al., 2006). One of them, is hyperproduction of Epo, which causes splenomegaly due to extramedullary erythropoiesis, promotes expansion of late stage basophilic erythroblasts, as well as polychromatic and orthochromatic erythroblasts, and finally leads to reticulocytosis and polycythemia. Importantly, overexpression of hepatic Epo is rescued by intercrossing Irp1−/− and liver-specific Hif2α−/−mice (Wilkinson and Pantopoulos, 2013). On the other hand, the defects in erythroid differentiation documented in the constitutive IRP1 transgenic mice can be attributed to reduced Hif2α expression (Casarrubea et al., 2013). Hence, the translational regulation of HIF2α mRNA by IRP1 links iron metabolism with erythropoiesis via EPO. Physiologically, this response probably serves to contain EPO expression and subsequent stimulation of erythropoiesis under conditions of iron deficiency. In addition, it is tempting to speculate that stress activation of IRP1 (by H2O2 or NO) (Weiss et al., 1993; Pantopoulos and Hentze, 1995) may contribute to the impairment of EPO production that is frequently observed under chronic inflammatory conditions or in chronic kidney disease (Weiss and Goodnough, 2005), via suppression of HIF2α mRNA translation.
The above data suggest that IRP1 functions as an iron and oxygen sensor (Figure 6). According to this model, in normoxic renal interstitial fibroblasts the small steady-state fraction of apo-IRP1 inhibits HIF2α mRNA translation and thereby limits EPO production to physiological levels. This fraction is expected to increase in normoxic iron deficiency due to 4Fe-4S cluster disassembly, further suppressing HIF2α and EPO expression as a homeostatic response to reduced iron availability, consistently with iron-restricted erythropoiesis. Conversely, increased iron supply is expected to increase the abundance of holo-IRP1, allowing de-repression of HIF2α mRNA translation. This response is also favored by hypoxia, which is known to stabilize holo-IRP1. Thus, in hypoxic and/or iron-replete renal interstitial fibroblasts, unimpeded HIF2α mRNA translation leads to increased generation of EPO and thereby stimulates erythropoiesis, as a homeostatic adaptation to the scarcity of oxygen and/or the abundance of iron.
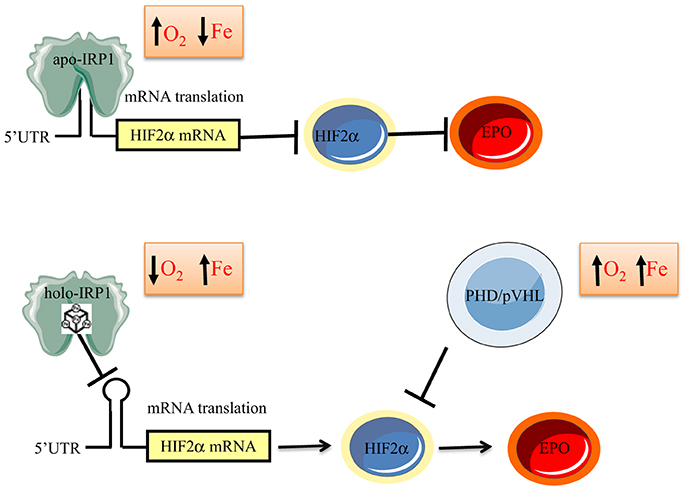
Figure 6. Model for regulation of erythropoiesis by IRP1 via the HIF2α/EPO axis. In iron-deficient or normoxic cells, apo-IRP1 limits HIF2α synthesis by binding to HIF2α mRNA. Iron-replete conditions favor conversion of apo- to holo-IRP1, at the expense of its IRE-binding activity, allowing HIF2α mRNA translation and transcriptional activation of its downstream target EPO. Hypoxia stabilizes holo-IRP1. Accumulation of HIF2α is antagonized by the PHD/pVHL degradation system, which is activated by oxygen and iron.
IRP2 is not involved in the regulation of HIF2α mRNA in vivo. This makes physiological sense, since IRP2 is highly active under hypoxia, when HIF2α synthesis is required. Nevertheless, the phenotype of Irp2−/− mice suggests that IRP2 is a critical regulator of other erythropoietic pathways. These animals present with hypochromic microcytic anemia with reduced iron availability for erythropoiesis (Cooperman et al., 2005; Galy et al., 2005b; Zumbrennen-Bullough et al., 2014). This is not a result of global iron deficiency but rather misregulation of cellular iron traffic. Irp2−/− erythroid cells exhibit a reduced Tfr1 content, apparently due to lack of Tfr1 mRNA stabilization in the absence of Irp2. As a result, these cells fail to acquire sufficient amounts of iron for erythropoiesis, in spite of the physiological iron supply, which is reflected in the normal Tf saturation and total iron-binding capacity (TIBC) in serum. Therefore, Irp2 appears to act as major activator of Tfr1 in erythroid cells. It is conceivable that this function is more pronounced at earlier stages of erythroid development, considering that during terminal erythroid differentiation, Tfr1 mRNA stability remains unresponsive to iron, bypassing the IRE/IRP checkpoint (Schranzhofer et al., 2006).
Irp2−/− mice also manifest very high levels of free and zinc protoporphyrin IX in RBCs (~200-fold increase for free protoporphyrin IX), consistently with erythropoietic protoporphyria (Cooperman et al., 2005). This is very likely caused by enhanced heme biosynthetic activity due to de-repression of Alas2 mRNA translation. In the absence of adequate iron, it results in accumulation of free protoporphyrin IX and in incorporation of zinc in the protoporphyrin IX ring. Therefore, IRP2 also acts as a major regulator of ALAS2 mRNA translation via the IRE in its 5′ UTR. The interpretation that IRP2 regulates erythroid iron uptake and utilization by controlling expression of the IRE-containing TfR1 and ALAS2 mRNAs, respectively, is also supported by data with tissue-specific Irp2−/− mice. Thus, liver- or intestinal-specific ablation of Irp2 recapitulates the iron overload phenotype of these tissues in ubiquitous Irp2−/− mice, but fails to promote microcytic anemia (Ferring-Appel et al., 2009).
The IRE/IRP System in Dietary Iron Absorption
Dietary iron absorption takes place in brush border enterocytes of the duodenum, especially in the upper tract. The pathway is highly regulated at different levels. Hormonal regulation via hepcidin is well established. Hepcidin is produced in the liver in response to high serum or hepatic iron (Corradini et al., 2011; Ramos et al., 2011) and limits further iron absorption by promoting the degradation of duodenal ferroportin (Nemeth et al., 2004; Chung et al., 2009), the basolateral iron transporter. Hepcidin may also promote degradation of DMT1, the apical iron transporter, by the proteasome (Chung et al., 2009; Brasse-Lagnel et al., 2011). Both ferroportin (Taylor et al., 2011) and DMT1 (Mastrogiannaki et al., 2009; Shah et al., 2009) are transcriptionally activated in iron-deficient enterocytes by HIF2α. In addition, ferroportin and DMT1 could be directly regulated by IRPs at the levels of mRNA translation or stability, respectively. Duodenal enterocytes also express ferritin, which limits excessive iron transfer to the circulation (Vanoaica et al., 2010). TfR1 expression is restricted to non-absorptive enterocytes in the intestinal crypts (Waheed et al., 1999).
Iron deficiency elicits multiple and antagonistic signals on duodenal ferroportin: transcriptional activation of its gene via HIF2α (which is negatively regulated by IRP1), translational repression of its mRNA via IRPs, and stabilization of the protein via downregulation of hepcidin. The net result is induction of ferroportin, which is considered a physiologic response to increase iron supply to the bloodstream (McKie et al., 2000). It has been proposed that the IRP blockade can be bypassed via enriched expression of an alternatively spliced non-IRE containing ferroportin transcript (Zhang et al., 2009). Nevertheless, in other reports the canonical IRE-containing ferroportin mRNA was found to be predominant in the duodenum of rats and mice (Darshan et al., 2011; Galy et al., 2013). Another possibility is that protein stabilization due to suppression of hepcidin is dominant.
Likewise, iron deficiency elicits multiple and antagonistic signals on duodenal DMT1: transcriptional activation of its gene via HIF2α (which is negatively regulated by IRP1), possible stabilization of its IRE-containing transcripts by IRPs, and possible stabilization of the protein via downregulation of hepcidin. Again, the net result is induction of DMT1 (Gunshin et al., 1997), which serves to increase dietary iron absorption from the intestinal lumen. Four different DMT1 mRNA isoforms are generated by the combination of alternative promoter usage and alternative splicing, and two of them harbor an IRE (Hubert and Hentze, 2002). Interestingly, the IRE-containing DMT1 mRNAs predominate in the duodenum and their expression is induced in iron deficiency (Canonne-Hergaux et al., 1999; Hubert and Hentze, 2002). Their transcription is selectively activated by HIF2α (Mastrogiannaki et al., 2009; Shah et al., 2009).
Experiments with mice bearing global or enterocyte-specific Irp deficiencies provided insights on the role of the IRE/IRP system in dietary iron absorption. Irp1−/− mice express high levels of duodenal ferroportin and IRE-Dmt1 mRNAs, most likely as a result of de-repression of Hif2α mRNA translation and enhanced Hif2α transcriptional activity in this tissue (Anderson et al., 2013). This interpretation is consistent with the high expression of further Hif2α target genes. Ferroportin and Dmt1 expression was not altered in duodena of Irp2−/− mice, which exhibit iron overload and express high levels of ferritin (Galy et al., 2005b). High levels of duodenal ferritin were also documented in mice with enterocyte-specific disruption of Irp2 (Ferring-Appel et al., 2009). On the other hand, duodenal ferritin was not suppressed in mice expressing the constitutive IRP1 transgene (Casarrubea et al., 2013), in agreement with previous cell culture experiments (Wang and Pantopoulos, 2002), possibly due to alternative ferritin mRNA translation via internal initiation (Daba et al., 2012). Thus, IRP2 alone appears to function as an important regulator of ferritin but not ferroportin and DMT1 in the duodenum.
Mouse pups with enterocyte-specific deletion of both Irp1 and Irp2 are viable at birth but die within 4 weeks due to malabsorption and dehydration, associated with abnormalities in intestinal architecture (Galy et al., 2008). Their enterocytes manifested highly increased expression of ferritin and ferroportin, and reduced levels of Tfr1 and Dmt1. The downregulation of ferritin and ferroportin was not associated with alterations in their mRNA levels, reinforcing the role of IRPs in the regulation of duodenal ferritin synthesis, but also demonstrating a prominent function of the IRE/IRP system in translational control of duodenal ferroportin mRNA. Expression of the major IRE-Dmt1 mRNA isoform was slightly reduced in the mutant mice, providing first in vivo evidence for a role of IRPs in the control of IRE-DMT1 mRNA stability.
Adult mice with enterocyte-specific ablation of both Irp1 and Irp2 were generated by using a tamoxifen-inducible Cre-deleter strain under the control of the villin promoter (Galy et al., 2013). These animals are viable and the ablation of Irps does not significantly alter intestinal architecture. As expected, the expression of ferritin and ferroportin was very high, while Tfr1 levels were low. Dmt1 was also upregulated, in contrast to the data obtained in pups with enterocyte-specific deletion of both Irps (Galy et al., 2008). The induction of Dmt1 was associated with an increase in steady-state levels of the IRE-Dmt1 mRNA isoforms, which was attributed to transcriptional stimulation by Hif2α. Together, the above data suggest that Irps regulate the IRE-Dmt1 mRNA only in newborn mice but not in adult animals. This is consistent with the notion that duodenal iron absorption in suckling pups is enhanced to satisfy metabolic needs of the rapidly growing organism, and is not subjected to negative regulation by hepcidin (Darshan et al., 2011). Stabilization of the IRE-Dmt1 mRNA isoforms by Irps could contribute to the increased iron absorption capacity before weaning. Surprisingly, adult mice with enterocyte-specific ablation of both Irps exhibited impaired iron absorption, in spite of the profound induction of the apical and basolateral iron transporters. This was attributed to a “mucosal block” imposed by the overexpression of ferritin, which stores internalized iron and prevents its delivery to the bloodstream. Therefore, alleviation of the “mucosal block” by limiting the expression of ferritin emerges as a crucial function of IRPs, possibly with a major contribution of IRP2, in enterocytes (Figure 7).
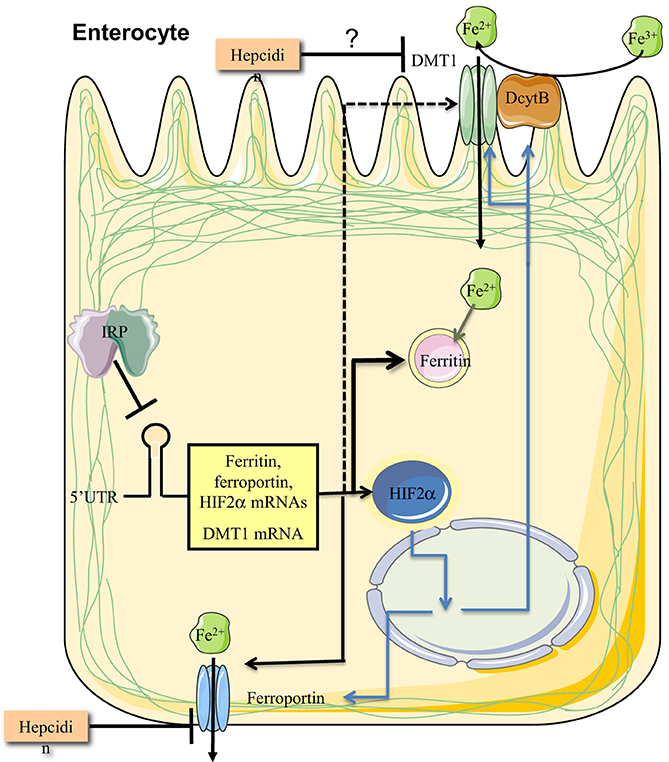
Figure 7. IRPs control dietary iron absorption by limiting ferritin synthesis in duodenal enterocytes. Regulated expression of ferritin is essential to prevent a “mucosal block” following iron intake from the intestinal lumen. In addition, IRPs control the expression of ferroportin and DMT1 mRNAs, the latter only in the period after birth and before weaning. Finally, IRP1 controls the expression of HIF2α mRNA, which in turn transcriptionally induces, among other targets, the iron transporters ferroportin and DMT1, and the ferrireductase Dcytb. Ferroportin and possibly also DMT1 are negatively regulated by hepcidin at the level of protein stability.
The IRE/IRP System in Systemic Iron Metabolism
Systemic iron metabolism is regulated by hepcidin, a peptide hormone synthesized by liver hepatocytes, which targets ferroportin in duodenal enterocytes, tissue macrophages and other iron-exporting cells (Ganz, 2013). The IRE/IRP system appears to intersect with the hepcidin ferroportin axis. Young Irp1−/− mice exhibit a marked suppression of hepcidin mRNA, accompanied by accumulation of ferroportin in splenic macrophages (Wilkinson and Pantopoulos, 2013). This response is caused by the increased erythropoietic activity of these animals due to induction of Hif2α and Epo, which is an established and potent inhibitor of hepcidin expression (Ganz, 2013). Irp2−/− mice were found to have physiological hepcidin mRNA levels, in spite of hepatic iron overload (Cooperman et al., 2005; Galy et al., 2005b). However, at the age of 4–6 weeks, these animals showed a significant induction of hepcidin mRNA expression (Wilkinson and Pantopoulos, 2013). Conceivably, these seemingly discrepant findings are related to the antagonistic signals of erythropoietic drive and iron overload, which act as negative or positive regulators of hepcidin, respectively (Ganz, 2013). These stimuli do not operate in a strictly hierarchical manner, and their dominance depends on signal strength (Huang et al., 2009). Hepatic iron overload may be dominant in young animals, and later neutralized by the erythropoietic drive. Nevertheless, 8–10-week-old liver-specific Irp2−/− mice, which develop hepatic iron overload without microcytic anemia, exhibit physiological hepcidin expression (Ferring-Appel et al., 2009). This may indicate that Irp2 expression is required for iron-dependent hepcidin induction in mice older than 4–6 weeks, but the underlying mechanism is unclear.
The liver is a major site for excessive iron storage within ferritin, but also the central regulator of systemic iron balance via hepcidin (Meynard et al., 2014). The hepatic iron overload phenotype that was observed in mice with global Irp2 disruption persisted in liver-specific Irp2−/− counterparts, which is indicative of a cell autonomous function (Ferring-Appel et al., 2009). Liver-specific disruption of both Irp1 and Irp2 resulted in early lethality within 1–2 weeks after birth due to liver failure (Galy et al., 2010). This was associated with mitochondrial iron deficiency, as well as histological and functional defects in this organelle. Thus, the IRE/IRP system is essential for liver function. Along similar lines, unregulated overexpression of endogenous Irp2 in liver-specific Fbxl5−/− mice impaired hepatic and systemic iron homeostasis (Moroishi et al., 2011). The animals developed hepatic iron overload and steatohepatitis, and exhibited inappropriately low hepcidin mRNA expression. Moreover, they succumbed after feeding with an iron-enriched diet due to lethal liver failure.
The IRE/IRP System in Cancer
Considering that HIF2α may function either as a tumor promoter or suppressor (Keith et al., 2012), the regulation of HIF2α mRNA translation by IRP1 provides a link between the IRE/IRP system and cancer biology. Other links were previously provided by tumor xenograft experiments. Thus, overexpression of IRP1 or a constitutive IRP1 mutant in human H1299 lung cancer cells impaired tumor xenograft growth in nude mice (Chen et al., 2007). Contrary, the overexpression of IRP2 elicited the opposite phenotype, which required the presence of an IRP2-specific 73 amino acids domain (Maffettone et al., 2010). IRE-containing mRNAs were not differentially expressed in IRP1- or IRP2-overexpressing xenografts, which exhibited distinct gene expression profiles (Maffettone et al., 2010). These data raise the possibility for a role of IRPs in modulating cancer growth independently of their IRE-binding activities, which remains to be further explored. Nevertheless, IRP2 was recently shown to be overexpressed in breast cancer cells and to promote tumor growth by modulating iron metabolism (Wang et al., 2014). This finding is consistent with reprogramming of iron metabolism in cancer cells (Torti and Torti, 2013). Notably, in earlier experiments IRP2 was reported to be transcriptionally induced by the proto-oncogene c-myc and to promote cell transformation by suppressing ferritin and by regulating cellular iron availability (Wu et al., 1999). On the other hand, the tumor suppressor p53 upregulated ferritin by reducing IRP activities (Zhang et al., 2008). Development of cancer models with Irp1−/− and Irp2−/− mice is expected to better define the roles of IRPs in cancer and to elucidate the molecular basis underlying the association of IRP2 (IREB2) genomic locus with susceptibility to lung cancer (Hansen et al., 2010; Cho et al., 2012; Fehringer et al., 2012).
Conclusions
The discovery of the IRE/IRP regulatory system provided a framework to understand the coordinate regulation of cellular iron uptake via TfR1, and storage within ferritin. Misregulation of L-ferritin expression due to “loss of function” mutations in the IRE of its mRNA is clinically relevant and underlies the molecular basis of the hyperferritinemia-caratact syndrome. The expansion of IRE-containing mRNAs raised the possibility that IRPs may control further biochemical pathways. This was firmly established by the analysis of mouse models with global or tissue-specific Irp1 and/or Irp2 deficiency. The animal studies highlighted a key role of the IRE/IRP system in regulation of erythropoiesis, dietary iron absorption, hepatic iron metabolism and body iron homeostasis via crosstalk with the hepcidin/ferroportin axis. These findings may be highly relevant to human medical conditions.
Conflict of Interest Statement
The authors declare that the research was conducted in the absence of any commercial or financial relationships that could be construed as a potential conflict of interest.
Acknowledgment
This work was supported by a grant from the Canadian Institutes for Health Research (CIHR; MOP-86514).
References
Aisen, P. (2004). Transferrin receptor 1. Int. J. Biochem. Cell Biol. 36, 2137–2143. doi: 10.1016/j.biocel.2004.02.007
Aisen, P., Enns, C., and Wessling-Resnick, M. (2001). Chemistry and biology of eukaryotic iron metabolism. Int. J. Biochem. Cell Biol. 33, 940–959. doi: 10.1016/S1357-2725(01)00063-2
Allerson, C. R., Cazzola, M., and Rouault, T. A. (1999). Clinical severity and thermodynamic effects of iron-responsive element mutations in hereditary hyperferritinemia-cataract syndrome. J. Biol. Chem. 274, 26439–26447. doi: 10.1074/jbc.274.37.26439
Anderson, S. A., Nizzi, C. P., Chang, Y. I., Deck, K. M., Schmidt, P. J., Galy, B., et al. (2013). The IRP1-HIF-2alpha axis coordinates iron and oxygen sensing with erythropoiesis and iron absorption. Cell Metab. 17, 282–290. doi: 10.1016/j.cmet.2013.01.007
Ang, S. O., Chen, H., Hirota, K., Gordeuk, V. R., Jelinek, J., Guan, Y., et al. (2002). Disruption of oxygen homeostasis underlies congenital Chuvash polycythemia. Nat. Genet. 32, 614–621. doi: 10.1038/ng1019
Arosio, P., Ingrassia, R., and Cavadini, P. (2009). Ferritins: a family of molecules for iron storage, antioxidation and more. Biochim. Biophys. Acta 1790, 589–599. doi: 10.1016/j.bbagen.2008.09.004
Beaumont, C., Leneuve, P., Devaux, I., Scoazec, J.-Y., Berthier, M., Loiseau, M.-N., et al. (1995). Mutation in the iron responsive element of the L ferritin mRNA in a family with dominant hyperferritinaemia and cataract. Nat. Genet. 11, 444–446. doi: 10.1038/ng1295-444
Brasse-Lagnel, C., Karim, Z., Letteron, P., Bekri, S., Bado, A., and Beaumont, C. (2011). Intestinal DMT1 cotransporter is down-regulated by hepcidin via proteasome internalization and degradation. Gastroenterology 140, 1261–1271.e1. doi: 10.1053/j.gastro.2010.12.037
Burn, J., and Chinnery, P. F. (2006). Neuroferritinopathy. Semin. Pediatr. Neurol. 13, 176–181. doi: 10.1016/j.spen.2006.08.006
Camaschella, C., Campanella, A., De Falco, L., Boschetto, L., Merlini, R., Silvestri, L., et al. (2007). The human counterpart of zebrafish shiraz shows sideroblastic-like microcytic anemia and iron overload. Blood 110, 1353–1358. doi: 10.1182/blood-2007-02-072520
Canonne-Hergaux, F., Gruenheid, S., Ponka, P., and Gros, P. (1999). Cellular and subcellular localization of the Nramp2 iron transporter in the intestinal brush border and regulation by dietary iron. Blood 93, 4406–4417.
Casarrubea, D., Viatte, L., Hallas, T., Vasanthakumar, A., Eisenstein, R. S., Schumann, K., et al. (2013). Abnormal body iron distribution and erythropoiesis in a novel mouse model with inducible gain of iron regulatory protein (IRP)-1 function. J. Mol. Med. 91, 871–881. doi: 10.1007/s00109-013-1008-2
Casey, J. L., Hentze, M. W., Koeller, D. M., Caughman, S. W., Rouault, T. A., Klausner, R. D., et al. (1988). Iron-responsive elements: regulatory RNA sequences that control mRNA levels and translation. Science 240, 924–928. doi: 10.1126/science.2452485
Casey, J. L., Koeller, D. M., Ramin, V. C., Klausner, R. D., and Harford, J. B. (1989). Iron regulation of transferrin receptor mRNA levels requires iron-responsive elements and a rapid turnover determinant in the 3′ untranslated region of the mRNA. EMBO J. 8, 3693–3699.
Cavill, I. (2002). Erythropoiesis and iron. Best Pract. Res. Clin. Haematol. 15, 399–409. doi: 10.1016/S1521-6926(02)90004-6
Cazzola, M., Bergamaschi, G., Tonon, L., Arbustini, E., Grasso, M., Vercesi, E., et al. (1997). Hereditary hyperferritinemia-cataract syndrome: relationship between phenotypes and specific mutations in the iron-responsive element of ferritin light-chain mRNA. Blood 90, 814–821.
Chappell, S. L., Daly, L., Lotya, J., Alsaegh, A., Guetta-Baranes, T., Roca, J., et al. (2011). The role of IREB2 and transforming growth factor beta-1 genetic variants in COPD: a replication case-control study. BMC Med. Genet. 12:24. doi: 10.1186/1471-2350-12-24
Chen, G., Fillebeen, C., Wang, J., and Pantopoulos, K. (2007). Overexpression of iron regulatory protein 1 suppresses growth of tumor xenografts. Carcinogenesis 28, 785–791. doi: 10.1093/carcin/bgl210
Cho, H. H., Cahill, C. M., Vanderburg, C. R., Scherzer, C. R., Wang, B., Huang, X., et al. (2010). Selective translational control of the Alzheimer amyloid precursor protein transcript by iron regulatory protein-1. J. Biol. Chem. 285, 31217–31232. doi: 10.1074/jbc.M110.149161
Cho, M. H., Castaldi, P. J., Wan, E. S., Siedlinski, M., Hersh, C. P., Demeo, D. L., et al. (2012). A genome-wide association study of COPD identifies a susceptibility locus on chromosome 19q13. Hum. Mol. Genet. 21, 947–957. doi: 10.1093/hmg/ddr524
Chollangi, S., Thompson, J. W., Ruiz, J. C., Gardner, K. H., and Bruick, R. K. (2012). Hemerythrin-like domain within F-box and leucine-rich repeat protein 5 (FBXL5) communicates cellular iron and oxygen availability by distinct mechanisms. J. Biol. Chem. 287, 23710–23717. doi: 10.1074/jbc.M112.360404
Chung, B., Chaston, T., Marks, J., Srai, S. K., and Sharp, P. A. (2009). Hepcidin decreases iron transporter expression in vivo in mouse duodenum and spleen and in vitro in THP-1 macrophages and intestinal Caco-2 cells. J. Nutr. 139, 1457–1462. doi: 10.3945/jn.108.102905
Clarke, S. L., Vasanthakumar, A., Anderson, S. A., Pondarre, C., Koh, C. M., Deck, K. M., et al. (2006). Iron-responsive degradation of iron-regulatory protein 1 does not require the Fe-S cluster. EMBO J. 25, 544–553. doi: 10.1038/sj.emboj.7600954
Coon, K. D., Siegel, A. M., Yee, S. J., Dunckley, T. L., Mueller, C., Nagra, R. M., et al. (2006). Preliminary demonstration of an allelic association of the IREB2 gene with Alzheimer's disease. J. Alzheimers. Dis. 9, 225–233.
Cooperman, S. S., Meyron-Holtz, E. G., Olivierre-Wilson, H., Ghosh, M. C., Mcconnell, J. P., and Rouault, T. A. (2005). Microcytic anemia, erythropoietic protoporphyria and neurodegeneration in mice with targeted deletion of iron regulatory protein 2. Blood 106, 1084–1091. doi: 10.1182/blood-2004-12-4703
Corradini, E., Meynard, D., Wu, Q., Chen, S., Ventura, P., Pietrangelo, A., et al. (2011). Serum and liver iron differently regulate the bone morphogenetic protein 6 (BMP6)-SMAD signaling pathway in mice. Hepatology 54, 273–284. doi: 10.1002/hep.24359
Daba, A., Koromilas, A. E., and Pantopoulos, K. (2012). Alternative ferritin mRNA translation via internal initiation. RNA 18, 547–556. doi: 10.1261/rna.029322.111
Dandekar, T., and Hentze, M. W. (1995). Finding the hairpin in the haystack: searching for RNA motifs. Trends Genet. 11, 45–50. doi: 10.1016/S0168-9525(00)88996-9
Darshan, D., Wilkins, S. J., Frazer, D. M., and Anderson, G. J. (2011). Reduced expression of ferroportin-1 mediates hyporesponsiveness of suckling rats to stimuli that reduce iron absorption. Gastroenterology 141, 300–309. doi: 10.1053/j.gastro.2011.04.012
Demeo, D. L., Mariani, T., Bhattacharya, S., Srisuma, S., Lange, C., Litonjua, A., et al. (2009). Integration of genomic and genetic approaches implicates IREB2 as a COPD susceptibility gene. Am. J. Hum. Genet. 85, 493–502. doi: 10.1016/j.ajhg.2009.09.004
Drapier, J. C., Hirling, H., Wietzerbin, J., Kaldy, P., and Kühn, L. C. (1993). Biosynthesis of nitric oxide activates iron regulatory factor in macrophages. EMBO J. 12, 3643–3649.
Dupuy, J., Volbeda, A., Carpentier, P., Darnault, C., Moulis, J. M., and Fontecilla-Camps, J. C. (2006). Crystal structure of human iron regulatory protein 1 as cytosolic aconitase. Structure 14, 129–139. doi: 10.1016/j.str.2005.09.009
Faucheux, B. A., Martin, M. E., Beaumont, C., Hunot, S., Hauw, J. J., Agid, Y., et al. (2002). Lack of up-regulation of ferritin is associated with sustained iron regulatory protein-1 binding activity in the substantia nigra of patients with Parkinson's disease. J. Neurochem. 83, 320–330. doi: 10.1046/j.1471-4159.2002.01118.x
Fehringer, G., Liu, G., Pintilie, M., Sykes, J., Cheng, D., Liu, N., et al. (2012). Association of the 15q25 and 5p15 lung cancer susceptibility regions with gene expression in lung tumor tissue. Cancer Epidemiol. Biomarkers Prev. 21, 1097–1104. doi: 10.1158/1055-9965.EPI-11-1123-T
Ferring-Appel, D., Hentze, M. W., and Galy, B. (2009). Cell-autonomous and systemic context-dependent functions of iron regulatory protein 2 in mammalian iron metabolism. Blood 113, 679–687. doi: 10.1182/blood-2008-05-155093
Galy, B., Ferring-Appel, D., Becker, C., Gretz, N., Grone, H. J., Schumann, K., et al. (2013). Iron regulatory proteins control a mucosal block to intestinal iron absorption. Cell Rep. 3, 844–857. doi: 10.1016/j.celrep.2013.02.026
Galy, B., Ferring-Appel, D., Kaden, S., Grone, H. J., and Hentze, M. W. (2008). Iron regulatory proteins are essential for intestinal function and control key iron absorption molecules in the duodenum. Cell Metab. 7, 79–85. doi: 10.1016/j.cmet.2007.10.006
Galy, B., Ferring-Appel, D., Sauer, S. W., Kaden, S., Lyoumi, S., Puy, H., et al. (2010). Iron regulatory proteins secure mitochondrial iron sufficiency and function. Cell Metab. 12, 194–201. doi: 10.1016/j.cmet.2010.06.007
Galy, B., Ferring, D., Benesova, M., Benes, V., and Hentze, M. W. (2004). Targeted mutagenesis of the murine IRP1 and IRP2 genes reveals context-dependent RNA processing differences in vivo. RNA 10, 1019–1025. doi: 10.1261/rna.7220704
Galy, B., Ferring, D., and Hentze, M. W. (2005a). Generation of conditional alleles of the murine iron regulatory protein (IRP)-1 and -2 genes. Genesis 43, 181–188. doi: 10.1002/gene.20169
Galy, B., Ferring, D., Minana, B., Bell, O., Janser, H. G., Muckenthaler, M., et al. (2005b). Altered body iron distribution and microcytosis in mice deficient for iron regulatory protein 2 (IRP2). Blood 106, 2580–2589. doi: 10.1182/blood-2005-04-1365
Galy, B., Hölter, S. M., Klopstock, T., Ferring, D., Becker, L., Kaden, S., et al. (2006). Iron homeostasis in the brain: complete iron regulatory protein 2 deficiency without symptomatic neurodegeneration in the mouse. Nat. Genet. 38, 967–969. doi: 10.1038/ng0906-967
Ganz, T. (2013). Systemic iron homeostasis. Physiol. Rev. 93, 1721–1741. doi: 10.1152/physrev.00008.2013
Ghosh, M. C., Ollivierre-Wilson, H., Cooperman, S., and Rouault, T. A. (2006). Reply to “Iron homeostasis in the brain: complete iron regulatory protein 2 deficiency without symptomatic neurodegeneration in the mouse”. Nat. Genet. 38, 969–970. doi: 10.1038/ng0906-969
Ghosh, M. C., Tong, W. H., Zhang, D., Ollivierre-Wilson, H., Singh, A., Krishna, M. C., et al. (2008). Tempol-mediated activation of latent iron regulatory protein activity prevents symptoms of neurodegenerative disease in IRP2 knockout mice. Proc. Natl. Acad. Sci. U.S.A. 105, 12028–12033. doi: 10.1073/pnas.0805361105
Ghosh, M. C., Zhang, D. L., Jeong, S. Y., Kovtunovych, G., Ollivierre-Wilson, H., Noguchi, A., et al. (2013). Deletion of iron regulatory protein 1 causes polycythemia and pulmonary hypertension in mice through translational derepression of HIF2alpha. Cell Metab. 17, 271–281. doi: 10.1016/j.cmet.2012.12.016
Gkouvatsos, K., Papanikolaou, G., and Pantopoulos, K. (2012). Regulation of iron transport and the role of transferrin. Biochim. Biophys. Acta 1820, 188–202. doi: 10.1016/j.bbagen.2011.10.013
Gunshin, H., Allerson, C. R., Polycarpou-Schwarz, M., Rofts, A., Rogers, J. T., Kishi, F., et al. (2001). Iron-dependent regulation of the divalent metal ion transporter. FEBS Lett. 509, 309–316. doi: 10.1016/S0014-5793(01)03189-1
Gunshin, H., Mackenzie, B., Berger, U. V., Gunshin, Y., Romero, M. F., Boron, W. F., et al. (1997). Cloning and characterization of a mammalian protein-coupled metal-ion transporter. Nature 388, 482–488. doi: 10.1038/41343
Haile, D. J., Rouault, T. A., Harford, J. B., Kennedy, M. C., Blondin, G. A., Beinert, H., et al. (1992). Cellular regulation of the iron-responsive element binding protein: disassembly of the cubane iron-sulfur cluster results in high-affinity RNA binding. Proc. Natl. Acad. Sci. U.S.A. 89, 11735–11739. doi: 10.1073/pnas.89.24.11735
Hansen, H. M., Xiao, Y., Rice, T., Bracci, P. M., Wrensch, M. R., Sison, J. D., et al. (2010). Fine mapping of chromosome 15q25.1 lung cancer susceptibility in African-Americans. Hum. Mol. Genet. 19, 3652–3661. doi: 10.1093/hmg/ddq268
Hausmann, A., Lee, J., and Pantopoulos, K. (2011). Redox control of iron regulatory protein 2 stability. FEBS Lett. 585, 687–692. doi: 10.1016/j.febslet.2011.01.036
Hentze, M. W., Caughman, S. W., Rouault, T. A., Barriocanal, J. G., Dancis, A., Harford, J. B., et al. (1987). Identification of the iron-responsive element for the translational regulation of human ferritin mRNA. Science 238, 1570–1573. doi: 10.1126/science.3685996
Huang, H., Constante, M., Layoun, A., and Santos, M. M. (2009). Contribution of STAT3 and SMAD4 pathways to the regulation of hepcidin by opposing stimuli. Blood 113, 3593–3599. doi: 10.1182/blood-2008-08-173641
Hubert, N., and Hentze, M. W. (2002). Previously uncharacterized isoforms of divalent metal transporter (DMT)-1: implications for regulation and cellular function. Proc. Natl. Acad. Sci. U.S.A. 99, 12345–12350. doi: 10.1073/pnas.192423399
Jeong, S. Y., Crooks, D. R., Wilson-Ollivierre, H., Ghosh, M. C., Sougrat, R., Lee, J., et al. (2011). Iron insufficiency compromises motor neurons and their mitochondrial function in Irp2-null mice. PLoS ONE 6:e25404. doi: 10.1371/journal.pone.0025404
Joshi, R., Morán, E., and Sanchez, M. (2012). “Cellular iron metabolism. The IRP/IRE regulatory network,” in Iron Metabolism, ed S. Arora (Rijeka: InTech), 25–58.
Kallianpur, A. R., Jia, P., Ellis, R. J., Zhao, Z., Bloss, C., Wen, W., et al. (2014). Genetic variation in iron metabolism is associated with neuropathic pain and pain severity in HIV-infected patients on antiretroviral therapy. PLoS ONE. (in press).
Kapitsinou, P. P., Liu, Q., Unger, T. L., Rha, J., Davidoff, O., Keith, B., et al. (2010). Hepatic HIF-2 regulates erythropoietic responses to hypoxia in renal anemia. Blood 116, 3039–3048. doi: 10.1182/blood-2010-02-270322
Kato, J., Fujikawa, K., Kanda, M., Fukuda, N., Sasaki, K., Takayama, T., et al. (2001). A mutation, in the iron-responsive element of H ferritin mRNA, causing autosomal dominant iron overload. Am. J. Hum. Genet. 69, 191–197. doi: 10.1086/321261
Keith, B., Johnson, R. S., and Simon, M. C. (2012). HIF1alpha and HIF2alpha: sibling rivalry in hypoxic tumour growth and progression. Nat. Rev. Cancer 12, 9–22. doi: 10.1038/nrc3183
Koury, M. J., and Bondurant, M. C. (1990). Erythropoietin retards DNA breakdown and prevents programmed death in erythroid progenitor cells. Science 248, 378–381. doi: 10.1126/science.2326648
LaVaute, T., Smith, S., Cooperman, S., Iwai, K., Land, W., Meyron-Holtz, E., et al. (2001). Targeted deletion of the gene encoding iron regulatory protein-2 causes misregulation of iron metabolism and neurodegenerative disease in mice. Nat. Genet. 27, 209–214. doi: 10.1038/84859
Levi, S., and Arosio, P. (2004). Mitochondrial ferritin. Int. J. Biochem. Cell Biol. 36, 1887–1889. doi: 10.1016/j.biocel.2003.10.020
Levi, S., Girelli, D., Perrone, F., Pasti, M., Beaumont, C., Corrocher, R., et al. (1998). Analysis of ferritins in lymphoblastoid cell lines and in the lens of subjects with hereditary hyperferritinemia-cataract syndrome. Blood 91, 4180–4187.
Liu, Q., Davidoff, O., Niss, K., and Haase, V. H. (2012). Hypoxia-inducible factor regulates hepcidin via erythropoietin-induced erythropoiesis. J. Clin. Invest. 122, 4635–4644. doi: 10.1172/JCI63924
Lobmayr, L., Brooks, D. G., and Wilson, R. B. (2005). Increased IRP1 activity in Friedreich ataxia. Gene 354, 157–161. doi: 10.1016/j.gene.2005.04.040
Luscieti, S., Tolle, G., Aranda, J., Campos, C. B., Risse, F., Moran, E., et al. (2013). Novel mutations in the ferritin-L iron-responsive element that only mildly impair IRP binding cause hereditary hyperferritinaemia cataract syndrome. Orphanet J. Rare Dis. 8:30. doi: 10.1186/1750-1172-8-30
Maffettone, C., Chen, G., Drozdov, I., Ouzounis, C., and Pantopoulos, K. (2010). Tumorigenic properties of iron regulatory protein 2 (IRP2) mediated by its specific 73-amino acids insert. PLoS ONE 5:e10163. doi: 10.1371/journal.pone.0010163
Mastrogiannaki, M., Matak, P., Keith, B., Simon, M. C., Vaulont, S., and Peyssonnaux, C. (2009). HIF-2alpha, but not HIF-1alpha, promotes iron absorption in mice. J. Clin. Invest. 119, 1159–1166. doi: 10.1172/JCI38499
Mastrogiannaki, M., Matak, P., Mathieu, J. R., Delga, S., Mayeux, P., Vaulont, S., et al. (2012). Hepatic hypoxia-inducible factor-2 down-regulates hepcidin expression in mice through an erythropoietin-mediated increase in erythropoiesis. Haematologica 97, 827–834. doi: 10.3324/haematol.2011.056119
Mastrogiannaki, M., Matak, P., and Peyssonnaux, C. (2013). The gut in iron homeostasis: role of HIF-2 under normal and pathological conditions. Blood 122, 885–892. doi: 10.1182/blood-2012-11-427765
McKie, A. T., Marciani, P., Rolfs, A., Brennan, K., Wehr, K., Barrow, D., et al. (2000). A novel duodenal iron-regulated transporter IREG1, implicated in the basolateral transfer of iron to the circulation. Mol. Cell 5, 299–309. doi: 10.1016/S1097-2765(00)80425-6
Meynard, D., Babitt, J. L., and Lin, H. Y. (2014). The liver: conductor of systemic iron balance. Blood 123, 168–176. doi: 10.1182/blood-2013-06-427757
Meyron-Holtz, E. G., Ghosh, M. C., Iwai, K., LaVaute, T., Brazzolotto, X., Berger, U. V., et al. (2004a). Genetic ablations of iron regulatory proteins 1 and 2 reveal why iron regulatory protein 2 dominates iron homeostasis. EMBO J. 23, 386–395. doi: 10.1038/sj.emboj.7600041
Meyron-Holtz, E. G., Ghosh, M. C., and Rouault, T. A. (2004b). Mammalian tissue oxygen levels modulate iron-regulatory protein activities in vivo. Science 306, 2087–2090. doi: 10.1126/science.1103786
Moroishi, T., Nishiyama, M., Takeda, Y., Iwai, K., and Nakayama, K. I. (2011). The FBXL5-IRP2 axis is integral to control of iron metabolism in vivo. Cell Metab. 14, 339–351. doi: 10.1016/j.cmet.2011.07.011
Müllner, E. W., and Kühn, L. C. (1988). A stem-loop in the 3′ untranslated region mediates iron-dependent regulation of transferrin receptor mRNA stability in the cytoplasm. Cell 53, 815–825. doi: 10.1016/0092-8674(88)90098-0
Nemeth, E., Tuttle, M. S., Powelson, J., Vaughn, M. B., Donovan, A., Ward, D. M., et al. (2004). Hepcidin regulates cellular iron efflux by binding to ferroportin and inducing its internalization. Science 306, 2090–2093. doi: 10.1126/science.1104742
Pantopoulos, K., and Hentze, M. W. (1995). Rapid responses to oxidative stress mediated by iron regulatory protein. EMBO J. 14, 2917–2924.
Papanikolaou, G., and Pantopoulos, K. (2005). Iron metabolism and toxicity. Toxicol. Appl. Pharmacol. 202, 199–211. doi: 10.1016/j.taap.2004.06.021
Percy, M. J., Furlow, P. W., Lucas, G. S., Li, X., Lappin, T. R., Mcmullin, M. F., et al. (2008). A gain-of-function mutation in the HIF2A gene in familial erythrocytosis. N. Engl. J. Med. 358, 162–168. doi: 10.1056/NEJMoa073123
Percy, M. J., Zhao, Q., Flores, A., Harrison, C., Lappin, T. R., Maxwell, P. H., et al. (2006). A family with erythrocytosis establishes a role for prolyl hydroxylase domain protein 2 in oxygen homeostasis. Proc. Natl. Acad. Sci. U.S.A. 103, 654–659. doi: 10.1073/pnas.0508423103
Piccinelli, P., and Samuelsson, T. (2007). Evolution of the iron-responsive element. RNA 13, 952–966. doi: 10.1261/rna.464807
Ponka, P. (1997). Tissue-specific regulation of iron metabolism and heme synthesis: distinct control mechanisms in erythroid cells. Blood 89, 1–25.
Ramos, E., Kautz, L., Rodriguez, R., Hansen, M., Gabayan, V., Ginzburg, Y., et al. (2011). Evidence for distinct pathways of hepcidin regulation by acute and chronic iron loading in mice. Hepatology 53, 1333–1341. doi: 10.1002/hep.24178
Rankin, E. B., Biju, M. P., Liu, Q., Unger, T. L., Rha, J., Johnson, R. S., et al. (2007). Hypoxia-inducible factor-2 (HIF-2) regulates hepatic erythropoietin in vivo. J. Clin. Invest. 117, 1068–1077. doi: 10.1172/JCI30117
Roetto, A., Bosio, S., Gramaglia, E., Barilaro, M. R., Zecchina, G., and Camaschella, C. (2002). Pathogenesis of hyperferritinemia cataract syndrome. Blood Cells Mol. Dis. 29, 532–535. doi: 10.1006/bcmd.2002.0590
Rouault, T. A. (2006). The role of iron regulatory proteins in mammalian iron homeostasis and disease. Nat. Chem. Biol. 2, 406–414. doi: 10.1038/nchembio807
Ruiz, J. C., Walker, S. D., Anderson, S. A., Eisenstein, R. S., and Bruick, R. K. (2013). F-box and leucine-rich repeat protein 5 (FBXL5) is required for maintenance of cellular and systemic iron homeostasis. J. Biol. Chem. 288, 552–560. doi: 10.1074/jbc.M112.426171
Salahudeen, A. A., Thompson, J. W., Ruiz, J. C., Ma, H. W., Kinch, L. N., Li, Q., et al. (2009). An E3 ligase possessing an iron-responsive hemerythrin domain is a regulator of iron homeostasis. Science 326, 722–726. doi: 10.1126/science.1176326
Sanchez, M., Galy, B., Dandekar, T., Bengert, P., Vainshtein, Y., Stolte, J., et al. (2006). Iron regulation and the cell cycle: identification of an iron-responsive element in the 3′-untranslated region of human cell division cycle 14A mRNA by a refined microarray-based screening strategy. J. Biol. Chem. 281, 22865–22874. doi: 10.1074/jbc.M603876200
Sanchez, M., Galy, B., Muckenthaler, M. U., and Hentze, M. W. (2007). Iron-regulatory proteins limit hypoxia-inducible factor-2alpha expression in iron deficiency. Nat. Struct. Mol. Biol. 14, 420–426. doi: 10.1038/nsmb1222
Sanchez, M., Galy, B., Schwanhaeusser, B., Blake, J., Bahr-Ivacevic, T., Benes, V., et al. (2011). Iron regulatory protein-1 and -2: transcriptome-wide definition of binding mRNAs and shaping of the cellular proteome by iron regulatory proteins. Blood 118, e168–e179. doi: 10.1182/blood-2011-04-343541
Schofield, C. J., and Ratcliffe, P. J. (2004). Oxygen sensing by HIF hydroxylases. Nat. Rev. Mol. Cell Biol. 5, 343–354. doi: 10.1038/nrm1366
Schranzhofer, M., Schifrer, M., Cabrera, J. A., Kopp, S., Chiba, P., Beug, H., et al. (2006). Remodeling the regulation of iron metabolism during erythroid differentiation to ensure efficient heme biosynthesis. Blood 107, 4159–4167. doi: 10.1182/blood-2005-05-1809
Sebastiani, G., and Pantopoulos, K. (2011). Disorders associated with systemic or local iron overload: from pathophysiology to clinical practice. Metallomics 3, 971–986. doi: 10.1039/c1mt00082a
Shah, Y. M., Matsubara, T., Ito, S., Yim, S. H., and Gonzalez, F. J. (2009). Intestinal hypoxia-inducible transcription factors are essential for iron absorption following iron deficiency. Cell Metab. 9, 152–164. doi: 10.1016/j.cmet.2008.12.012
Shah, Y. M., and Xie, L. (2014). Hypoxia-inducible factors link iron homeostasis and erythropoiesis. Gastroenterology 146, 630–642. doi: 10.1053/j.gastro.2013.12.031
Smith, S. R., Cooperman, S., LaVaute, T., Tresser, N., Ghosh, M., Meyron-Holtz, E., et al. (2004). Severity of neurodegeneration correlates with compromise of iron metabolism in mice with iron regulatory protein deficiencies. Ann. N.Y. Acad. Sci. 1012, 65–83. doi: 10.1196/annals.1306.006
Smith, S. R., Ghosh, M. C., Ollivierre-Wilson, H., Hang Tong, W., and Rouault, T. A. (2006). Complete loss of iron regulatory proteins 1 and 2 prevents viability of murine zygotes beyond the blastocyst stage of embryonic development. Blood Cells Mol. Dis. 36, 283–287. doi: 10.1016/j.bcmd.2005.12.006
Synowiec, E., Pogorzelska, M., Blasiak, J., Szaflik, J., and Szaflik, J. P. (2012). Genetic polymorphism of the iron-regulatory protein-1 and -2 genes in age-related macular degeneration. Mol. Biol. Rep. 39, 7077–7087. doi: 10.1007/s11033-012-1539-6
Taylor, M., Qu, A., Anderson, E. R., Matsubara, T., Martin, A., Gonzalez, F. J., et al. (2011). Hypoxia-inducible factor-2alpha mediates the adaptive increase of intestinal ferroportin during iron deficiency in mice. Gastroenterology 140, 2044–2055. doi: 10.1053/j.gastro.2011.03.007
Thompson, J. W., Salahudeen, A. A., Chollangi, S., Ruiz, J. C., Brautigam, C. A., Makris, T. M., et al. (2012). Structural and molecular characterization of iron-sensing hemerythrin-like domain within F-box and leucine-rich repeat protein 5 (FBXL5). J. Biol. Chem. 287, 7357–7365. doi: 10.1074/jbc.M111.308684
Torti, S. V., and Torti, F. M. (2013). Iron and cancer: more ore to be mined. Nat. Rev. Cancer 13, 342–355. doi: 10.1038/nrc3495
Vanoaica, L., Darshan, D., Richman, L., Schumann, K., and Kuhn, L. C. (2010). Intestinal ferritin h is required for an accurate control of iron absorption. Cell Metab. 12, 273–282. doi: 10.1016/j.cmet.2010.08.003
Vashisht, A. A., Zumbrennen, K. B., Huang, X., Powers, D. N., Durazo, A., Sun, D., et al. (2009). Control of iron homeostasis by an iron-regulated ubiquitin ligase. Science 326, 718–721. doi: 10.1126/science.1176333
Viatte, L., Grone, H. J., Hentze, M. W., and Galy, B. (2009). In vivo role(s) of the iron regulatory proteins (IRP) 1 and 2 in aseptic local inflammation. J. Mol. Med. 87, 913–921. doi: 10.1007/s00109-009-0494-8
Waheed, A., Parkkila, S., Saarnio, J., Fleming, R. E., Zhou, X. Y., Tomatsu, S., et al. (1999). Association of HFE protein with transferrin receptor in crypt enterocytes of human duodenum. Proc. Natl. Acad. Sci. U.S.A. 96, 1579–1584. doi: 10.1073/pnas.96.4.1579
Walden, W. E., Selezneva, A. I., Dupuy, J., Volbeda, A., Fontecilla-Camps, J. C., Theil, E. C., et al. (2006). Structure of dual function iron regulatory protein 1 complexed with ferritin IRE-RNA. Science 314, 1903–1908. doi: 10.1126/science.1133116
Wang, J., Chen, G., and Pantopoulos, K. (2005). Nitric oxide inhibits the degradation of IRP2. Mol. Cell. Biol. 25, 1347–1353. doi: 10.1128/MCB.25.4.1347-1353.2005
Wang, J., Fillebeen, C., Chen, G., Biederbick, A., Lill, R., and Pantopoulos, K. (2007). Iron-dependent degradation of apo-IRP1 by the ubiquitin-proteasome pathway. Mol. Cell. Biol. 27, 2423–2430. doi: 10.1128/MCB.01111-06
Wang, J., and Pantopoulos, K. (2002). Conditional de-repression of ferritin synthesis in cells expressing a constitutive IRP1 mutant. Mol. Cell. Biol. 22, 4638–4651. doi: 10.1128/MCB.22.13.4638-4651.2002
Wang, J., and Pantopoulos, K. (2011). Regulation of cellular iron metabolism. Biochem. J. 434, 365–381. doi: 10.1042/BJ20101825
Wang, W., Deng, Z., Hatcher, H., Miller, L. D., Di, X., Tesfay, L., et al. (2014). IRP2 regulates breast tumor growth. Cancer Res. 74, 497–507. doi: 10.1158/0008-5472.CAN-13-1224
Weiss, G., and Goodnough, L. T. (2005). Anemia of chronic disease. N. Engl. J. Med. 352, 1011–1023. doi: 10.1056/NEJMra041809
Weiss, G., Goossen, B., Doppler, W., Fuchs, D., Pantopoulos, K., Werner-Felmayer, G., et al. (1993). Translational regulation via iron-responsive elements by the nitric oxide/NO-synthase pathway. EMBO J. 12, 3651–3657.
Wilkinson, N., and Pantopoulos, K. (2013). IRP1 regulates erythropoiesis and systemic iron homeostasis by controlling HIF2alpha mRNA translation. Blood 122, 1658–1668. doi: 10.1182/blood-2013-03-492454
Wingert, R. A., Galloway, J. L., Barut, B., Foott, H., Fraenkel, P., Axe, J. L., et al. (2005). Deficiency of glutaredoxin 5 reveals Fe-S clusters are required for vertebrate haem synthesis. Nature 436, 1035–1039. doi: 10.1038/nature03887
Wu, K. J., Polack, A., and Dalla-Favera, R. (1999). Coordinated regulation of iron-controlling genes, H-ferritin and IRP2, by c-MYC. Science 283, 676–679. doi: 10.1126/science.283.5402.676
Yang, X. R., Liang, X., Pfeiffer, R. M., Wheeler, W., Maeder, D., Burdette, L., et al. (2010). Associations of 9p21 variants with cutaneous malignant melanoma, nevi, and pigmentation phenotypes in melanoma-prone families with and without CDKN2A mutations. Fam. Cancer 9, 625–633. doi: 10.1007/s10689-010-9356-3
Ye, H., Jeong, S. Y., Ghosh, M. C., Kovtunovych, G., Silvestri, L., Ortillo, D., et al. (2010). Glutaredoxin 5 deficiency causes sideroblastic anemia by specifically impairing heme biosynthesis and depleting cytosolic iron in human erythroblasts. J. Clin. Invest. 120, 1749–1761. doi: 10.1172/JCI40372
Yin, D., Kulhalli, V., and Walker, A. P. (2014). Raised serum ferritin concentration in hereditary hyperferritinemia cataract syndrome is not a marker for iron overload. Hepatology 59, 1204–1206. doi: 10.1002/hep.26681
Zhang, D. L., Hughes, R. M., Ollivierre-Wilson, H., Ghosh, M. C., and Rouault, T. A. (2009). A ferroportin transcript that lacks an iron-responsive element enables duodenal and erythroid precursor cells to evade translational repression. Cell Metab. 9, 461–473. doi: 10.1016/j.cmet.2009.03.006
Zhang, F., Wang, W., Tsuji, Y., Torti, S. V., and Torti, F. M. (2008). Post-transcriptional modulation of iron homeostasis during p53-dependent growth arrest. J. Biol. Chem. 283, 33911–33918. doi: 10.1074/jbc.M806432200
Zhou, H., Yang, J., Li, D., Xiao, J., Wang, B., Wang, L., et al. (2012). Association of IREB2 and CHRNA3/5 polymorphisms with COPD and COPD-related phenotypes in a Chinese Han population. J. Hum. Genet. 57, 738–746. doi: 10.1038/jhg.2012.104
Zimmer, M., Ebert, B. L., Neil, C., Brenner, K., Papaioannou, I., Melas, A., et al. (2008). Small-molecule inhibitors of HIF-2a translation link its 5′UTR iron-responsive element to oxygen sensing. Mol. Cell 32, 838–848. doi: 10.1016/j.molcel.2008.12.004
Keywords: iron metabolism, ferritin, ferroportin, aconitase, transferrin receptor, HIF2α, DMT1, hepcidin
Citation: Wilkinson N and Pantopoulos K (2014) The IRP/IRE system in vivo: insights from mouse models. Front. Pharmacol. 5:176. doi: 10.3389/fphar.2014.00176
Received: 05 May 2014; Accepted: 07 July 2014;
Published online: 28 July 2014.
Edited by:
Paolo Arosio, University of Brescia, ItalyReviewed by:
Mayka Sanchez, Josep Carreras Leukaemia Research Institute (IJC), SpainCarole Beaumont, INSERM, France
Copyright © 2014 Wilkinson and Pantopoulos. This is an open-access article distributed under the terms of the Creative Commons Attribution License (CC BY). The use, distribution or reproduction in other forums is permitted, provided the original author(s) or licensor are credited and that the original publication in this journal is cited, in accordance with accepted academic practice. No use, distribution or reproduction is permitted which does not comply with these terms.
*Correspondence: Kostas Pantopoulos, Lady Davis Institute for Medical Research, Jewish General Hospital, and Department of Medicine, 3755 Cote Ste-Catherine Rd., Montreal, QC H3T 1E2, Canada e-mail: kostas.pantopoulos@mcgill.ca