- 1Department of Biotechnology, College of Science and Technology, Andhra University, Visakhapatnam, India
- 2Andhra University College of Pharmaceutical Sciences, Andhra University, Visakhapatnam, India
- 3Department of Human Genetics, College of Science and Technology, Andhra University, Visakhapatnam, India
The COVID-19 pandemic has caused a global health crisis, with no specific antiviral to treat the infection and the absence of a suitable vaccine to prevent it. While some individuals contracting the SARS-CoV-2 infection exhibit a well coordinated immune response and recover, others display a dysfunctional immune response leading to serious complications including ARDS, sepsis, MOF; associated with morbidity and mortality. Studies revealed that in patients with a dysfunctional immune response, there is a massive cytokine and chemokine release, referred to as the ‘cytokine storm’. As a result, such patients exhibit higher levels of pro-inflammatory/modulatory cytokines and chemokines like TNFα, INFγ, IL-1β, IL-2, IL-4, IL-6, IL-7, IL-9, IL-10, IL-12, IL-13, IL-17, G-CSF, GM-CSF, MCSF, HGF and chemokines CXCL8, MCP1, IP10, MIP1α and MIP1β. Targeting this cytokine storm is a novel, promising treatment strategy to alleviate this excess influx of cytokines observed at the site of infection and their subsequent disastrous consequences. Natural immunosuppressant compounds, derived from plant sources like curcumin, luteolin, piperine, resveratrol are known to inhibit the production and release of pro-inflammatory cytokines and chemokines. This inhibitory effect is mediated by altering signal pathways like NF-κB, JAK/STAT, MAPK/ERK that are involved in the production and release of cytokines and chemokines. The use of these natural immunosuppressants as adjuvants to ameliorate the cytokine storm; in combination with antiviral agents and other treatment drugs currently in use presents a novel, synergistic approach for the treatment and effective cure of COVID-19. This review briefly describes the immunopathogenesis of the cytokine storm observed in SARS-CoV-2 infection and details some natural immunosuppressants that can be used as adjuvants in treating COVID-19 disease.
Introduction
The coronavirus disease 2019 (COVID-19) caused by a novel ß-coronavirus, SARS-CoV-2 was first reported in Wuhan, China in December 2019 (Wang et al., 2020a). Spreading rapidly across the globe, the outbreak was declared as a Public Health Emergency of International Concern on 30 January 2020 by the World Health Organization (World Health Organization, 2020a). The reality and challenge of the present situation is that there is no specific drug to treat and cure the disease; neither is there a vaccine for prevention from it (at the time of submission of this review) (World Health Organization, 2020b).
One characteristic feature of the COVID-19 disease is the complex immune dysregulation observed in patients. This immune dysfunction causes deleterious clinical manifestations that lead up to organ injury, consequent organ failure and ultimately mortality (Giamarellos-Bourboulis et al., 2020; Li et al., 2020a). The hallmark of the immune dysregulation observed is an exaggerated immune response; manifested as hyperinflammation and hypercytokinemia (cytokine storm syndrome/ cytokine storm) in the COVID-19 patients. The factors that contribute to the state of hyperinflammation are persistent lymphopenia, neutrophilia, over-activation of complement components C3, C3a, C5, C5a and mannose binding lectin-associated serine protease (MASP2); in addition to the cytokine storm (Girija et al., 2020). The cytokine storm observed is the most dangerous and potentially life-threatening event in the COVID-19 disease. This is because it plays a crucial role in disease aggravation by promoting acute respiratory distress syndrome (ARDS) and multiple organ failure (MOF) (Coperchini et al., 2020; Ye et al., 2020). Several studies suggest that in addition to the use of antiviral drugs for the treatment of COVID-19, downregulation of the cytokine storm would prove to be an efficient treatment strategy to successfully combat the disease (Rahmati and Moosavi, 2020; Soy et al., 2020; Zhao, 2020). Plants, plant extracts and their derivatives have been known to possess immunomodulatory properties. Additionally, some plant-derived bioactive compounds have been evaluated for their ability to suppress the cytokine storm associated with inflammation and disease. This paper discusses the cytokine storm syndrome observed in COVID-19 patients in brief and emphasizes on some natural immunosuppressant agents derived from plants sources that can play an important role in targeting and mitigation of the cytokine storm observed in COVID-19.
COVID-19 and the Cytokine Storm
Introduction to COVID-19
COVID-19 is a viral disease caused by a beta coronavirus, SARS-CoV-2; an enveloped, non-segmented RNA virus (Astuti and Ysrafil, 2020; Wang et al., 2020b). The disease outbreak, declared as an ongoing pandemic by the WHO is widespread; affecting 220 countries, areas/ territories across the world with a total of 55,928,327 confirmed cases and with 1,344,003 confirmed deaths reported (World Health Organization, 2020c). Transmission of SARS-CoV-2 occurs primarily through respiratory droplets and contact routes (World Health Organization, 2020d). The spike protein plays an important role for SARS-CoV-2 to gain access to the intracellular compartment of the host. The receptor-binding domain (RBD) of the S1 subunit of the spike protein binds to the hACE2 receptor with high affinity, facilitating viral entry and infection in the lower respiratory tract cells (Hemmat et al., 2020; Shang et al., 2020). Once SARS-CoV-2 enters the host cell, its RNA is translated and viral replication for the production of mature newly synthesized virons occurs, which are released out of the infected cells (Shereen et al., 2020). The mean incubation period for SARS-CoV-2 is 3–7 days (Li et al., 2020b). Symptoms develop post the incubation period (Wujtewicz et al., 2020). Some infected individuals are asymptomatic, others are symptomatic with mild disease and, a third group is symptomatic with severe disease (Tabata et al., 2020). The symptoms of the disease are fever, cough, fatigue, headache, myalgia, sore throat, shortness of breath, sputum production and diarrhea. Other symptoms include chest pain, chills, nasal congestion, rhinorrhea and nausea (Fu et al., 2020a). Clinical presentations include elevated levels of lactate dehydrogenase, creatine kinase, alanine transaminase and aspartate aminotransferase (Wang et al., 2020a). Lymphocytopenia, high exhaustion levels and reduced functional diversity of T-cells (CD4+ and CD8+ T-cells) in peripheral blood is also another clinical feature of COVID-19 patients (Zheng et al., 2020a; Zheng et al., 2020b; Zhao et al., 2020). Higher levels of serum IgM, IgG and IgA can be detected in patients; severely ill SARS-CoV-2 patients have significantly higher antibody levels than mildly ill patients (Ma et al., 2020). The platelet and neutrophil levels are also elevated in patients (Zhou et al., 2020). Elevated D-dimer levels, prolonged prothrombin time (PT) and altered levels of fibrinogen observed point to the state of pulmonary intravascular coagulopathy that develops in patients (Zhang et al., 2020a; Lancet Haematol, 2020; Connors and Lavy, 2020; Long et al., 2020; McGonagle et al., 2020). Severe COVID-19 patients exhibit widespread complement activation which is characterized by C3 activation, C3a generation, C3 fragment deposition and increased serum C5a levels (Mastaglio et al., 2020; Noris et al., 2020; Risitano et al., 2020). Inflammatory markers like erythrocyte sedimentation rate (ESR), IL-6, C-reactive protein (CRP) and procalcitonin (PCT) are also elevated; especially in the patients with severe disease (Zeng et al., 2020). Elevated levels of all these inflammatory markers have been associated with disease severity; indicating the hyper-immune inflammatory state existing in the body resulting in higher morbidity and mortality in this patient group (Chen et al., 2020a; Chen et al., 2020b; Lippi and Plabani, 2020; Sun et al., 2020). Patients also exhibited higher levels of pro-inflammatory cytokines TNFα, IFNγ, IL-1β, IL-2, IL-4, IL-6, IL-7, IL-9, IL-12, IL-13, IL-17, G-CSF, GM-CSF, MCSF and chemokines CXCL8, MCP1, IP10, MIP1α, MIP1β. As in the case of inflammatory markers, the levels of serum cytokines and chemokines are higher in patients with severe disease. A diverse cytokine profile is observed in the two groups of patients; with critically ill patients showing higher levels of IL-1, IL-6, IL-2, IL-7, IL-10, IL-17, G-CSF, IP10, MCP1, MIP1α and TNFα. Among the cytokines, IL-6, IL-10 and CXCL10 are predictive of high risk patients of disease deterioration. As a consequence of the surging cytokine levels, the epithelial lining of the lungs gets injured that leads to deterioration of the alveolar cellular barriers. Changes to the microvasculature are also observed owing to damage in the endothelial cells. These changes results in the development of ARDS (Schett et al., 2020a; Han et al., 2020; Laing et al., 2020; Lingeswaran et al., 2020; Lingeswaran et al., 2020). The immunopathology gradually progresses to respiratory failure, establishment of secondary infections, sepsis and septic shock, multiple organ failure (kidney, liver); an overall poor prognosis that can end up in mortality (Gabriella et al., 2020; Guo et al., 2020; Hou et al., 2020).
Cytokine Storm/ Hypercytokinemia/ Cytokine Storm Syndrome
Cytokine storm and hypercytokinemia are the two terminologies used to describe a severe, life-threatening condition that can occur as a result of an infection, autoimmune condition, or other disease. The National Cancer Institute defines cytokine storm as a severe immune reaction in which the body releases too many cytokines into the blood too quickly (National Cancer Institute, 2020). This condition is also referred to as cytokine storm syndrome sometimes (Shimabukuro-Vornhagen et al., 2018). These cytokines are a diverse group of small, non-structural, low molecular weight protein signaling molecules that are secreted and released by cells that have a complex regulatory influence on immunity and inflammation (Zhang and An, 2007; Ray et al., 2016). They play a significant role in regulating the immune response in health and disease (Duque and Descoteaux, 2014). Escalated cytokine production is associated with disease conditions (Orzechowski et al., 2014). Although the clinical presentations of cytokines vary for different diseases, they are characterized by broadly similar cytokine profiles. Cytokines generally associated with cytokine storm are interferons, interleukins, chemokines, colony stimulating factors (CSFs) and tumor necrosis factors (TNFs) (Tisoncik et al., 2012).
Immunopathogenesis of Cytokine Storm in COVID-19
The immune system plays a crucial role in the control and resolution of SARS-CoV-2 infection. An out-of-control immune response to the pathogen can lead to immunopathogenesis that can prove to be fatal; causing excessive inflammation and even death (Guo et al., 2020; Qin et al., 2020). In most of the individuals infected with SARS-CoV-2, the immune system is primed and cells are recruited which clears the infection in the lung. The immune response then gradually recedes and the patients recover successfully. However, there are some patients wherein a dysfunctional immune response is observed, that triggers a massive cytokine and chemokine release; ‘cytokine storm’, mediating widespread inflammation in the lung (Catanzaro et al., 2020; Tay et al., 2020). One of the major causes of the ARDS and MOF observed in severe SARS-CoV-2 infection is the cytokine storm (Lingeswaran et al., 2020; Ye et al., 2020). Studies revealed that the progression of SARS-CoV-2 infection is associated with cytokine storm; higher level of cytokine storm is associated with more severe disease development (Tang et al., 2020a; Han et al., 2020). Plasma levels of cytokines IL-1β, IL-1RA, IL-7, IL-9, IL-10, FGF, G-CSF, GM-CSF, PDGF, VEGF, IFNγ, TNF and chemokines CXCL8, IP10, MCP1, MIP1α, MIP1β are significantly increased in patients with COVID-19 compared to healthy individuals. Furthermore, pro-inflammatory cytokines IL-2, IL-7, IL-10, G-CSF, TNF and chemokines IP10, MCP1, MIP1α are increased in severe patients compared with mildly-infected patients (Tang et al., 2020b).
Cytokines and chemokines are essential immune system mediators that have a significant role to play in maintenance of anti-viral immunity (Melchjorsen et al., 2003; Sládková and Kostolansky 2006). SARS-CoV-2 activates both the innate and adaptive immune response (Catanzaro et al., 2020). Initially after the entry of the virus into host cells, pathogen recognition receptors (PRRs) like TLR7, TLR8, RLRs and NLRs expressed by epithelial cells and antigen presenting cells like alveolar macrophages facilitate the recognition of virus by recognizing PAMP compromised nucleic acids, carbohydrate moieties, glycoproteins, lipoproteins, intermediate products such as dsRNA and small molecules found in the structural components of virus. TLR3, TLR7 and TLR8 are the first to identify the virus and this is responsible for an enhanced interferon production. Viral recognition triggers the stimulation of the innate immune response that leads to the activation of several signal transduction cascades and consequent downstream transcription factors resulting in the expression of genes encoding pro-inflammatory cytokines and chemokines; the SARS-CoV-2 signature characterized by induction of IL-1Rα, IL-1β, IL-6 and TNF. The chemokines produced serves to attract more innate immune response cells; NK cells, dendritic cells, polymorphonuclear leukocytes and monocytes; which in turn produce chemokines; MIG, IP10 and MCP-1 that are capable of recruiting lymphocytes. These recruited T-lymphocytes serve to recognize the antigen that is presented by the dendritic cells. The primed CD4 T-cells then execute the highly specific adaptive immune response by giving the signal to the B-cells for the production of antibodies and to the cytotoxic CD8 T-cells that are capable of targeting and eliminating the SARS-CoV-2. The antibodies that are produced; mainly IgG, IgM and IgA are highly specific and are directed toward the SARS-CoV-2 surface glycoproteins; primarily the spike glycoprotein and the nucleocapsid protein. This constitutes the humoral immune response to SARS-CoV-2 and these antibodies are vital to neutralize the viral infection of the human cells and tissues expressing ACE2. The cellular immune response to SARS-CoV-2 is mediated by the T-helper cells; the CD4 T-cells that help B-cells produce neutralizing antibodies, the cytotoxic CD8 T-cells; aided by helper T-cells that directly kill SARS-CoV-2-infected cells, the other T-cells; including the Th17-cells that drive the subsequent inflammatory response observed and the regulatory T-cells that are responsible for containing the immune response to preventing an exaggerated response (García, 2020; Herb et al., 2020; Hotez et al., 2020; Poland et al., 2020; Ragab et al., 2020; Shah et al., 2020; Song et al., 2020; Soy et al., 2020; Tay et al., 2020; Vabret et al., 2020).
Studies have revealed that the transition from the innate to adaptive immune response is critical for the clinical progress of the SARS-CoV-2 infection. Though the immune regulatory events in play during this transition are poorly understood at this point, it is clear that it either leads to a protective immune response in some patients or a dysfunctional immune response in others. Another contributing factor is the dysregulated interferon responses. SARS-CoV-2 replication during the incubation period in the host cells is stealthy; without detectably triggering interferon production resulting in high viral load. As a result, there is an initial, suppressed interferon response in early stage of infection. In severe COVID-19 patients, however, robust IFN-1 responses have been reported. Such a dysfunctional immune response results in failure to effectively clear the pathogen and persistent viral replication ensuing an exacerbated inflammatory response and elevated cytokine production. Together, impaired viral clearance, low levels of type I interferons in the initial stage of infection, increased neutrophil extracellular traps and triggered NK cell activation constitute the plausible predisposing factors that drive the cytokine storm or cytokine storm syndrome in COVID-19 contributing to the aggressive inflammatory response. Other contributing factors to the cytokine storm syndrome include pyroptosis; an inflammatory form of programmed cell death and ACE2 receptor mediated-increase in cytokines IL-6 and MCP1 triggered by an increased angiotensin II following the disruption of the rennin-angiotensin system; caused when S1 subunit of spike protein binds to hACE2 in the alveolar macrophages (Acharya et al., 2020; Fu et al., 2020b; Golonka et al., 2020; Iwasaki et al., 2020; Lee and Shin, 2020; Lee et al., 2020; Mahmudpour et al., 2020).
Initially, there is a lag in the production of cytokines and chemokines by the innate immune cells in the COVID-19 disease. This is followed by a sudden acute increase in the pro-inflammatory cytokine and chemokine production by the activated macrophages, monocytes and other recruited lymphocytes. The surge in levels of pro-inflammatory cytokines and chemokines augments infiltration of neutrophils, macrophages, monocytes and T-cells to the site of infection, bringing about an intense and violent inflammatory response in the bronchi and alveoli. This leads to disruption of air-blood barrier, endothelial and epithelial cell damage, breakdown of the alveolar-epithelial barrier, diffuse alveolar damage leading to ARDS and pulmonary fibrosis (PF). In addition to the local damage at the site of infection, the cytokine storm also has ripple effects across the body; contributing to viral sepsis, MOF and finally death in critically-ill patients (Schett et al., 2020b; Li et al., 2020c; Lingeswaran et al., 2020; Matthay et al., 2020; Nile et al., 2020; Prompetchara et al., 2020; Ragab et al., 2020; Risitano et al., 2020; Spagnolo et al., 2020; Tay et al., 2020; Vabret et al., 2020). A diagrammatic representation of the cytokine storm observed in COVID-19 is found in Figure 1 (CAS, 2020).
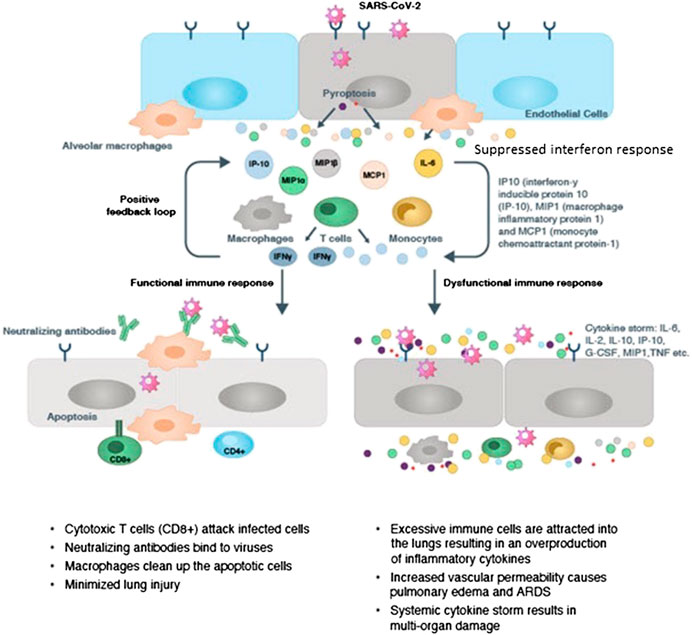
Figure 1. Immunopathology of Cytokine Storm in COVID-19 (CAS, 2020).
Immunomodulatory Agents- Targeting the Cytokine Storm in COVID-19
Despite intense scientific study and research, there are no specific drugs or vaccine available for the treatment or prevention of COVID-19. More than 600 clinical trials have been undertaken, with no promising results to date (at the time of submission of this review). The current standard care for COVID-19 being practiced is just symptomatic, palliative and supportive treatment (Catanzaro et al., 2020; Risitano et al., 2020). A diverse spectrum of pharmaceutical agents is being employed in an attempt to treat and efficiently manage COVID-19 infection. Antiviral agents, other chemical agents, monoclonal antibodies and other drugs are part of the current treatment regime. The antiviral drugs in use are lopinavir, ritonavir, remdesivir, oseltamivir, favipiravir, arbidol, ribavirin, ganciclovir and amantadine. Other chemical agents used for treatment are hydroxychloroquine, chloroquine, azithromycin, ivermectin, colchicine, thalidomide and glucocorticoids like methylprednisolone and dexamethasone. Monoclonal antibody tocilizumab, convalescent plasma interferons and intravenous immunoglobulin therapy are some of the other treatment methods in vogue. Additionally, antibiotics like cephalo-sporins, quinolones, carbapenems, linezolid or antifungal agents may be given (Chakraborty et al., 2020a; National Institutes of Health, 2020a; Saha et al., 2020a; Sethi et al., 2020; Tobaiqy et al., 2020).
While the role played by specific antiviral drugs and other pharmaceutical agents that can effectively target SARS-CoV-2 and reduce the infection is significant and cannot be downplayed, the use of immunomodulatory therapy is potentially effective, considering that SARS-CoV-2 drives immune dysfunction and induces hyperinflammation and a cytokine storm in patients (Iannaccone et al., 2020; Shi et al., 2020; Tufan et al., 2020; Zhong et al., 2020). A treatment strategy goes that beyond antiviral therapy alone, using immunomodulatory agents as adjuvants is a holistic therapeutic approach that takes into account the immune response of the host, hence checking the acute immune/ inflammatory response and preventing ARDS and PF (Alijotas-Reig et al., 2020; Liang et al., 2020). The cytokine storm is one prominent feature of the severe immune aberrations observed in COVID-19 patients (Yazdanpanah et al., 2020). Targeting the cytokine storm in order to ameliorate the state of hyperinflammation is proposed to be a novel therapeutic approach in the treatment of COVID-19 patients (Felsenstein et al., 2020; Prompetchara et al., 2020; Roshanravan et al., 2020a; Vabret et al., 2020). Several synthetic drugs, monoclonal antibodies and stem cell therapies have been proposed and are being explored to treat the cytokine storm in COVID-19 and thus reduce the state of hyperinflammation (Alijotas-Reig et al., 2020). Some of the therapeutic approaches being used currently and are also under clinical trials are discussed as follows.
Anti-malarial drugs hydroxychloroquine and chloroquine that are know to decrease Th17-related cytokines; IL-6, IL-17, IL-22, TNFα and IL-1β have been evaluated for efficacy in COVID-19 treatment in several clinical trials (Felsenstein et al., 2020; da Silva et al., 2020; Karres et al., 2020; Meo et al., 2020). Selective cytokine blockade with monoclonal antibodies tocilizumab and sarilumab that are antagonists of IL-6 receptors are being studied in clinical trials in certain countries (Atal and Fatima, 2020; Chakraborty et al., 2020b; Saha et al., 2020b). Calcineurin inhibitors reduce both the calcium-production of IL-2 and the expression of IL-2 receptor thus reducing T-cell activation. Two such inhibitors; cyclosporine and tacrolimus are being considered as potential drug candidates to reduce the cytokine storm syndrome observed in COVID-19 (Mejia et al., 2014; Cure et al., 2020; Rudnicka et al., 2020; National Institutes of Health, 2020b). The IL-1 receptor antagonist anakinra and the anti-IL-1β monoclonal antibody canakinumab are two drug candidates that efficiently treat cytokine storm syndromes observed in other hyper-inflammatory conditions like Still’s disease that are being evaluated for efficacy against the SARS-CoV-2-induced cytokine storm (Huet et al., 2020; Filocamo et al., 2020; National Institutes of Health, 2020c). Infliximab and adalimumab that are anti-TNF antibodies are being considered for modulating the hyperinflammation observed in COVID-19 by specifically targeting TNF (Roshanravan et al., 2020b). Individual studies and clinical trials to evaluate efficacy of low-molecular weight unfractionated heparin in COVID-19 patients with coagulopathy are underway (Tang et al., 2020b; National Institutes of Health, 2020d). Another immunomodulatory treatment molecule being evaluated is the use of intravenous immunoglobulin (IVIG). A clinical trial studying the benefit of administering intravenous immunoglobulin when compared to standard care alone is currently underway (National Institutes of Health, 2020e). Individual studies evaluating the efficacy of high dose of IVIGs as a therapeutic option in deteriorating patients have been undertaken, yielding mixed results (Xie et al., 2020; Cao et al., 2020). Hyperimmune immunoglobulin treatment for COVID-19 is also being explored (Alwis et al., 2020). A clinical trial that compares the efficacy of convalescent plasma to anti-COVID-19 human immunoglobulin in hospitalized patients is currently in progress (National Institutes of Health, 2020f). The JAK/STAT pathway is the main signaling pathway involved in the control of cytokine production (Bagca and Avci, 2020; Seif et al., 2020). Ruxolitinib selectively inhibits Janus kinase (JAK) 1 and 2 and has a modest to marked selectivity against tyrosine kinase (TYK) 2 and JAK 3. Studies and clinical trials are being conducted to evaluate the efficacy of ruxolitinib to target the excess production of cytokines in COVID-19 patients with hyperinflammation and ARDS (La Rosée et al., 2020; National Institutes of Health, 2020g; National Institutes of Health, 2020h). Baricitinib is another inhibitor that binds to JAK 1/2 and inhibits its activation and consequent cytokine release (National Institutes of Health, 2020i). The use of baricitinib for targeting the COVID-19 cytokine storm is being explored in pilot studies and phase 2 and 3 clinical trials (Cantini et al., 2020; National Institutes of Health, 2020j; National Institutes of Health, 2020k). Corticosteroids which are known for their ability to modulate the inflammatory response and have been used in other viral outbreaks are another option for COVID-19 treatment (Russell et al., 2020). Clinical trials testing the prophylactic action, safety and efficacy of dexamethasone and methylprednisolone in reducing the cytokine storm in COVID-19 patients are ongoing (Horby et al., 2020; National Institutes of Health, 2020l; National Institutes of Health, 2020m; Nature, 2020). Statins are a class of drugs that have immunomodulatory and anti-inflammatory properties and are being considered for treating the cytokine storm in COVID-19 (Castiglione et al., 2020; Lee et al., 2020; National Institutes of Health, 2020n). The entry of SARS-CoV-2 into host cells is mediated by hACE2, which is also involved in the virus-induced acute lung injury (Liu et al., 2020). Clinical trials are ongoing, to check the safety and efficacy of recombinant hACE2 (rhACE2) to mediate direct (via restoration of rennin-angiotensin system) and indirect (the chimeric receptor effect) therapeutic effects in SARS-CoV-2 induced inflammation and ARDS (Barone et al., 2020; National Institutes of Health, 2020o; National Institutes of Health, 2020p). The use of multipotent mesenchymal stem cell (MSCs) to inhibit the exaggerated immune response caused by the cytokine storm in COVID-19 patients is also being studied (Rajarshi et al., 2020).
Though the benefits of these synthetic therapeutic immunomodulatory agents cannot be disputed, their use is frequently associated with adverse side effects. Cardiomyopathy, neurological and gastrointestinal side effects are reported with chloroquine and hydroxychloroquine treatment and as COVID-19 patients are more vulnerable because of co-morbidities, there are concerns about safety (Colafrancesco et al., 2020; U.S. Food and Drug Administration, 2020; Gevers et al., 2020). Treatment of COVID-19 patients with IL-6 antagonists with tocilizumab and sarilumab also raises safety concerns as these are known to cause endocrinological, hematological, gastrointestinal and cardiovascular adverse effects (Atal and Fatima, 2020). IL-2 blockers cyclosporine and tacrolimus are known to cause side effects and can be extremely harmful (Cure et al., 2020; Xia et al., 2020). Anakinra and canakinumab, IL-1 blockers have a reasonably good safety profile, but can cause elevation of liver enzyme levels, myopathy and mild leukopenia (Colafrancesco et al., 2020). Anti-TNF treatment adverse effects are reported to be infrequent (Favalli et al., 2020). Severe drug reactions were reported for JAK/STAT inhibitor, ruxolitinib administered to COVID-19 patients; thus prompting the early stoppage of this drug in the study undertaken (Gaspari et al., 2020). There is also a risk of developing serious infections with the use of another oral JAK/STAT inhibitor, baricitinib (Lilly, 2020). Previously conducted clinical trials on statin treatment in ARDS and sepsis patients was met with negative outcomes, thus causing reluctance in considering them as adjuncts for COVID-19 therapy (Lee et al., 2020). In a study of rhACE2 treatment for ARDS patients, hypernatremia and dysphagia were the noted side effects (Barone et al., 2020). In case of improper administration of MSCs, there is a probability of lethal adverse thrombotic complications and reactions necessitating a thorough understanding of this treatment option and application routes (Moll et al., 2020).
Natural Immunosuppressant Agents- Adjuvants to Target the Cytokine Storm in COVID-19
The discovery of immunomodulatory agents from plants sources with enhanced bioavailability and essentially devoid of toxic side effects is a ray of hope and opens up a novel approach to mitigate the cytokine storm syndrome observed in COVID-19 (Grigore, 2017; Cena and Chieppa, 2020). Several plants and plant-derived bioactive compounds have been studied for their immunomodulatory activity and many have been identified for their immunosuppressive activity in particular (Sagrawat and Khan, 2007; Sahoo and Banik, 2018). These natural immunosuppressive agents provide an alternative therapeutic strategy for the efficient management SARS-CoV-2-associated illness; the cytokine storm and the resulting hyperinflammation. Targeting the cytokine storm with plant-derived immunosuppressants is a very promising strategic treatment method. This is because the individual mediators of the inflammatory cascade; cytokines including IL-1β, IL-6, TNFα and chemokines IP10, MCP1 are neutralized or inhibited, rather than a broad immune suppression that can negatively effect the viral clearance. This specific targeting of cytokines is achieved by the inhibitory action on specific signaling cascades. With their good safety profiles in addition to their capacity to keep a check on the pro-inflammatory cytokine levels all the while without compromising on the ability of the immune system to respond to the SARS-CoV-2; plant-derived immunosuppressant are the need of the hour. Some immunosuppressant agents derived from plants proven to reduce inflammation by downregulating the levels of pro-inflammatory cytokines that can be used as adjuvants in COVID-19 treatment are discussed as follows.
Andrographolide
Andrographolide is a labdane diterpenoid; 4-hydroxy-3-[2-[6-hydroxy-5-(hydroxymethyl)-5,8a-dimethyl-2-methylidene-3,4,4a,6,7,8-hexahydro-1H-naphthalen-1-yl]ethylidene]oxolan-2-one. It is isolated from the medicinal plant Andrographis paniculata (Brum.f.) Nees and has a wide range of therapeutic properties; anti-inflammatory, anti-allergic, anti-platelet aggregation, antineoplastic, anti-HIV and hepatoprotective activity (Jayakumar et al., 2013; National Center for Biotechnology Information, 2020a). Andrographolie is also a potent immunomodulator; known to significantly stimulate the immune response, regulate the production of NK cells and cytokines and stimulate the production of cytotoxic T-lymphocytes (Varma et al., 2011). Andrographolide efficiently brought about a dose-dependent reduction in the levels of inflammatory cytokines TNFα, IL-12, IL-1β, IL-6, IL-18 in LPS/ IL-4-activated murine macrophages (Wang et al., 2010). Andrographolide treatment suppresses inflammatory mediators IL-1β, TNFα, prostaglandin E2 (PGE2), NADPH oxidase 2 (NOX2) and inducible nitric oxide synthase (iNOS) in ischemic brain tissues after pMCAO stimulation (Lu et al., 2019). In LPS-stimulated RAW264.7 cells, andrographolide treatment resulted in a similar dose-dependent reduction in levels of pro-inflammatory cytokines TNFα, IL-1β and IL-6 and their corresponding mRNA expression levels. This reduction in pro-inflammatory cytokine secretion is because andrographolide suppresses LPS-induced NF-κB and MAPK pathways; andrographolide reduced the levels of p65 and IkBα phosphorylation in the NF-κB pathway and the levels of p-JNK, p-ERK1/2 and p-p38 in MAPK pathway (Li et al., 2017). Andrographolide derivatives were found to inhibit TNFα/ NF-κB and TLR4/ NF-κ signaling pathways by inhibiting the nuclear translocation of the NF-κB p65 subunit and attenuating the phosphorylation of p65 and IκBα, thus decreasing the levels of serum pro-inflammatory cytokines and chemokines (Nie et al., 2017). In a study, oral administration of andrographolide significantly attenuated mouse cortical chemokine levels from the C-C (CCL2, CCL5) and C-X-C (CXCL1, CXCL2, CXCL10) subfamilies. Andrographolide also abrogated LPS-induced chemokines (CCL2, CCL5, CXCL1, CXCL5, CX3CL1) and TNFα in astrocytes (Wong et al., 2016). In a study carried out using Jurkat cells stimulated with phorbol myristate acetate and ionomycin (PMA/ionomycin), andrographolide was found to reduce the production of IL-2 and reduce the activity of NF-κB. It also brought about a decrease in the ERK1 and ERK5 phosphorylation induced by anti-CD3 or PMA/ionomycin (Carretta et al., 2009). Andrographolide significantly reduced the production of INFγ and partially inhibited IL-2 production in murine T-cells stimulated with concanavalin A. This reduced INFγ production was associated with a significant decrease in the ERK1/2 phosphorylation on treating the cells with andrographolide. In the same study, andrographolide was also found to reduce hydrocortisone/PMA-induced apoptosis in thymocytes (Burgos et al., 2005). Docking studies carried out revealed that andrographolide is also a potent inhibitor of the main protease of SARS-CoV-2 (Mpro). Moreover, andrographolide is safe and does not interfere with the metabolism of other therapeutic drugs (Enmozhi et al., 2020). This dual beneficial role of andrographolide; as a potent immunosuppressive agent to alleviate the abnormal cytokine and chemokine production and as a potential inhibitor of SARS-CoV-2 by targeting the main protease make andrographolide a promising natural agent to be considered for COVID-19 targeted therapy (Banerjee et al., 2020).
Allicin
Allicin is a thiosulphate; 3-prop-2-enylsulfinylsulfanylprop-1-ene which is a constituent of garlic oil responsible for the typical smell and taste associated with freshly cut garlic (Allium sativum L.). Allicin is also found in garden onion (Allium cepa L.) and in other species in the family Alliaceae (Borlinghaus et al., 2014; National Center for Biotechnology Information, 2020b). Allicin is known to possess antioxidant, antimicrobial, antiviral, anti-inflammatory, anti-tumor and anti-diabetic properties (Batiha et al., 2020). In a study undertaken, allicin brought about a marked inhibition in the spontaneous and TNF-induced secretion of IL-1β, CXCL8 and IP10 in a dose-dependent manner in two different cell lines of intestinal epithelial cells. The mRNA levels of the IL-1β cytokine and CXCL8 chemokine are also reduced. This reduction in cytokine production is because of the effect of allicin on the NF-κB pathway; it suppresses the degradation of IκB (Lang et al., 2004). Allicin treatment to BALB/c mice post Plasmodium yoelii infection resulted in improved defense and survival due to an increase in the production of IFNγ and expansion of CD4+ T-cells. Allicin supplementation along with tamoxifen treatment to Ehrlich ascites carcinoma (EAC) cells resulted in marked decrease in TNFα levels, showing its beneficial role as an adjuvant (Arreola et al., 2015). In clinical trials evaluating the safety of allicin treatment, no adverse effects were reported (Sharifi-Rad et al., 2019). In HT-29 and Caco-2 cells stimulated with TNFα, allicin brought about a marked inhibition in the secretion of IL-1β, IP10, CXCL8 and MIG in a dose-dependent manner. RNA protection assay revealed that allicin reduced the expression of IL-1β and CXCL8 mRNA levels (Lang et al., 2004). Allicin was also responsible for suppressing the degradation of IkappaB. In LPS-treated blood samples, allicin drastically reduced the release of IL-10 (Keiss et al., 2003). Allicin successfully attenuated the LPS-induced inflammatory responses in a study with cultured human umbilical vein endothelial cells (HUVECs). Significant decrease in the levels of TNFα, CXCL8 and NFκB activity were observed on treatment with allicin. Additionally, treatment with allicin also reduced LPS-induced apoptosis in the cultured HUVECs (Zhang et al., 2017). With its ability to alter NF-κB signaling for reduction in cytokine secretion potentially targeting the cytokine storm, improve defense by increasing the IFNγ production for increased antiviral defense and improve expansion of CD4+ T-cells thus targeting the lymphocytopenia, allicin is a promising natural immunomodulatory adjuvant that can be used in conjunction with antiviral therapy in COVID-19 patients. However, its safe use in COVID-19 patients necessitates clinical trials to evaluate safety profile and pharmacokinetics (with antiviral agents in use) in these patients.
Colchicine
Colchicine is a secondary metabolite (alkaloid) extracted from Colchicum autumnale L. and Gloriosa superb L. (Varsha et al., 2017). Chemically, colchicine is N-[(7S)-1,2,3,10-tetramethoxy-9-oxo-6,7-dihydro-5H-benzo[a]heptalen-7-yl]acetamide (National Center for Biotechnology Information, 2020c). It is known to possess anti-inflammatory and immunomodulatory properties and is used in the treatment of gout, FMF and Beçhet’s syndrome. Colchicine is known to inhibit the production of IL-1 (Soy et al., 2020). Microtubules form an important part of the cytoskeleton, which is involved in cellular processes like secretion of cytokines and chemokines and cell migration. Colchicine blocks the polymerization of microtubule by binding to the ß-tubulin subunit with high affinity, thus preventing its assembly and affecting the process of cytokine and chemokine secretion and the migration of inflammatory cells like monocytes and neutrophils. Colchicine also disrupts the activation of inflammasome, suppressing activation of caspase-1 and subsequent release of IL-1β and IL-18 (Montealegre-Gómez et al., 2020). In an experimental study on patients with acute coronary syndromes, colchicine was found to bring a significant reduction in the levels of inflammatory cytokines IL-1β, IL-6 and IL-18 (Martínez et al., 2015). Colchicine was also found to bring about the inhibition of the assembly of the NLRP3 inflammasome thus resulting in the inhibition of the expression of pro-inflammatory cytokines including IL-1β. Given the increased NLRP3 inflammasome activation that contributes to hypercytokinemia observed in SARS-CoV-2 patients, colchicine is definitely an agent of interest to suppressing the NLRP3 inflammasome and thus ameliorate the state of hypercytokinemia observed (Papadopoulos et al., 2020; Ribeiro et al., 2020; Shah, 2020; Vitiello et al., 2020). Individual studies have been undertaken to evaluate the efficacy of cholchicine treatment for COVID-19. Colchicine was observed to bring about a reduction in the cytokine levels, activation of neutrophils and macrophages and inflammasome in SARS-CoV-2 patients. Adverse effects in patients were minimal, mild diarrhea was only effect reported in few patients (Della-Torre et al., 2020; Montealegre-Gómez et al., 2020). Clinical trials are underway to consider the clinical utility, safety and efficacy of colchicine-adjuvant therapy for COVID-19 (Soy et al., 2020).
Curcumin
Curcumin (1E,6E)-1,7-bis(4-hydroxy-3-methoxyphenyl)hepta-1,6-diene-3,5-dione) is a polyphenol pigment derived from the perennial plant Curcuma longa L., commonly known as the turmeric spice. It is a natural antioxidant, possesses anti-inflammatory, neuroprotective and hepatoprotective activity, and also inhibits tumor cell proliferation. Curcumin is also an immunomodulator (Hewlings and Douglas, 2017; National Center for Biotechnology Information, 2020d). The ability of curcumin to inhibit the production and release of different pro-inflammatory cytokines and chemokines IL-1β, IL-2, IL-6, IL-12, TNFα, CXCL8, IP10, MCP1, MIP1α and IFNγ has been proved in several studies. This immunosuppressive action of curcumin is mediated by its effect on different signaling pathways. Curcumin regulates the NF-κB pathways at multiple stages; inhibiting activation of IKKβ, blocking cytokine-mediated NF-κB activation by inhibiting IκBα degradation, blocking NF-κB signaling by activating AMPK, disturbing the NF-κB pathway by acting on p65 and as a consequence downregulating transcription of cytokine genes. On the other hand, curcumin positively regulates anti-inflammatory cytokines, particularly IL-10. IL-10 reduces levels of TNFα, and ICAM 1. Curcumin was found to increase the expression, production and activity of IL-10 (Jain et al., 2009; Sordillo and Helson., 2015; Shimizu et al., 2019; Liu et al., 2020). A study on the effect of curcumin on the pro-inflammatory cytokine IL-18 in LPS-stimulated murine macrophage-like cells RAW264.7 revealed that curcumin significantly inhibited the production of IL-18 (Yadav et al., 2015). In another study on monocyte culture exposed to pre-eclamptic plasma, curcumin caused a significant reduction in the levels of pro-inflammatory cytokines IL-1α, IL-6 and TNFα and also brought about a decrease in the level of nuclear NF-κB p50 (Rahardjo et al., 2014). In Iran, a randomized, double-blind, placebo-controlled study was carried out on COVID-19 patients form the Imam Reza Hospital of Tabriz University of Medical Sciences was carried out. This study aimed to evaluate the efficacy of nano-curcumin (dose- 160 mg of in four 40 mg capsules daily for 14 days) in modulating the levels of inflammatory cytokines IL-1β, IL-6, TNFα and IL-18 in 40 patients. Decreased expression and secretion of IL-1β and IL-6 was observed in the COVID-19 patients. However, there was no positive effect on the IL-18 mRNA expression and TNFα concentration with the treatment of nano-curcumin (Babaei et al., 2020; Valizadeh et al., 2020; Zahedipour et al., 2020). Being a natural spice extract, curcumin is safe and has low toxicity. All these properties motivate further clinical investigations for the use of curcumin as an immunomodulatory adjuvant to mitigate the cytokine storm, hyperinflammation and ARDS observed as a result of SARS-CoV-2 infection (Sordillo and Helson., 2015; Liu and Ying, 2020; Liu et al., 2020).
Eugenol
An aromatic oil, eugenol is 2-methoxy-4-prop-2-enylphenol. Although eugenol is also called clove oil as it is the main component of clove buds (Syzygium aromaticum (L.) Merr. and L.M. Perry), it is found many other plants like cinnamon bark and leaves, tulsi leaves, turmeric, pepper, ginger and organic herbs like oregano, thyme, basil, bay, marjoram, mace and nutmeg (Khalil et al., 2017; National Center for Biotechnology Information, 2020e). Eugenol is known to possess multiple beneficial pharmacological properties. It is a potent antioxidant, analgesic, antimicrobial, anticonvulsant and anticancer agent. Additionally it possesses anti-inflammatory and anti-viral properties and is an immunomodulatory agent (Pramod et al., 2010; Dibazar et al., 2015). In a study, eugenol inhibited the production of IL-6 and IL-10 in vitro (Bachiega et al., 2012). Administration of eugenol prevented increase in IL-4 and IL-5, reduced NF-κB signaling pathways and ultimately protected lung tissue from OVA-induced eosinophilia. In another study, eugenol caused the downregulation of pro-inflammatory cytokines IL-6 and TNFα; reduced the expression of NF-κB signaling, thus reducing leukocyte recruitment and inflammation in the lung tissue. Eugenol also reduced the TNFα and IL-1β as well as the NF-κB, ERK1/2, and p38 MAPK signaling pathways in LPS-induced macrophages (Barboza et al., 2018). When used in low doses, eugenol has few adverse effects; local irritation and rare allergic reactions. In case of accidental overdose, tissue injury and damage to liver and kidney can result (National Institute of Diabetes and Digestive and Kidney Diseases, 2020). Treatment with polymeric nanocarriers of eugenol was found to bring about a significant reduction in the TPA-induced IL-6 levels in Swiss mice (de Araújo Lopes et al., 2018). In a LPS-induced inflammatory model in porcine intestinal epithelial cells, eugenol enervated the inflammatory response by reducing both, the CXCL8 and TNFα mRNA levels significantly (Hui et al., 2020). Another study carried out on LPS-challenged macrophages revealed that eugenol suppressed the production of IL-6 and IL-10. However, there was no effect observed on the production of IL-1β (Bachiega et al., 2012). Mouse peritoneal macrophages were subject to activation with a bacterial LPS in order to evaluate the effect of eugenol on pro-inflammatory mediator genes NF-κB1 and TNFα. Since LPS did not induce the expression of NF-κB1, the effect of eugenol could not be ascertained. In case of TNFα, hypoexpression of was observed on treatment with eugenol in LPS-activated cells (Porto Mde et al., 2014). The effect of eugenol treatment on the two important inflammatory markers IL-6 and TNFα was examined in adult male Wistar rats. The levels of both IL-6 and TNFα were elevated post thioacetamide-induced hepatic injury. Pretreatment with eugenol brought about a significant decrease in the levels of these inflammatory markers (Yogalakshmi et al., 2010). With the good safety profile of eugenol and its ability to downregulate key pro-inflammatory cytokines like IL-6 and TNFα consequently reducing leukocyte recruitment in lung tissue, it can be a suitable natural immunosuppressant that can be used as an adjuvant along with antiviral agents to suppress the hypercytokinemia and hyperinflammation observed in COVID-19.
Gallic Acid
Gallic acid is a phenolic secondary metabolite found in abundance in plants; in the bark, wood, leaf, fruit, root and seeds. Chemically, it is 3,4,5-trihydroxybenzonic acid (Fitzpatrick and Woldermariam, 2017). It is a natural antioxidant that also has anticancer, antifungal, antimicrobial and anti-inflammatory properties. Studies revealed that gallic acid suppresses the levels of pro-inflammatory cytokines IL-1, IL-6, IL-12, IL-17, IL-23, TGFβ, TNFα and chemokines CCL2 and CCL7 in TNBS-induced ulcerative colitis and RA FLS. This anti-inflammatory activity of gallic acid is exerted by inhibiting of NF-κB pathway (Huang et al., 2019; Zhu et al., 2019). Another study revealed that gallic acid selectively suppressed Th2 cytokines IL-4 and IL-5 but did not suppress the Th1 cytokine IFNγ in anti CD3-stimulated spleen cells (Kato et al., 2001). Treatment with gallic acid was found to suppress the activity of NF-κB and inhibited pro-inflammatory cytokine release in high glucose-induced human monocytes (THP-1 cells) (Lee et al., 2015). The inhibitory effect of gallic acid on the production of pro-inflammatory cytokines TNFα and IL-6 was evaluated in human mast cells (HMC-1). HMC-1 cells were stimulated with PMA plus A23187 which caused the secretion of both TNFα and IL-6. Gallic acid treatment significantly blocked the secretion of both these cytokines in the stimulated HMC-1 cells (Kim et al., 2006). Gallic acid treatment was also found to successfully inhibit the PMA plus A23187-induced degradation of IκBα and the nuclear translocation of p65 NF-κB. The inflammatory regulatory effect of gallic acid was assessed in LPS-induced endometriosis cells. Gallic acid was responsible for a significant decrease in the expression of NF-κB and in the secretion profile of pro-inflammatory cytokine IL-6 (Bustami et al., 2018). The effect of polygallic acid, enzymatically produced from gallic acid on the secretion of pro-inflammatory cytokines IL-6, TNFα and IL-1β was evaluated in human monocytes exposed to PMA. A substantial decrease in the production of all three cytokines under study was observed on treatment with polygallic acid (Zamudio-Cuevas et al., 2020). This suggests that gallic acid can be used as an immunosuppressive adjuvant, to selectively suppress pro-inflammatory cytokines thus targeting the cytokine release syndrome observed in SARS-CoV-2 patients without negatively affecting the ability of the host to produce interferons.
Gingerol
A constituent of fresh ginger (Zingiber officinale Roscoe), gingerol is a beta-hydroxy ketone that is 5-hydroxydecan-3-one substituted by a 4-hydroxy-3-methoxyphenyl moiety at position 1 (5S)-5-hydroxy-1-(4-hydroxy-3-methoxyphenyl)decan-3-one (National Center for Biotechnology Information, 2020f). Gingerol is also found in the rhizomes of several members of the Zingiber species. Gingerol possesses antioxidant, anti-inflammatory, anti-metastatic, anti-angiogenic, anti-diabetic, analgesic and antipyretic activity. Additionally, gingerol is also known to possess immunomodulatory properties and anti-allergic activity (Sharifi-Rad et al., 2017). 6-gingerol was found to exert an inhibitory effect on the production of pro-inflammatory cytokines IL-1β, IL-12, TNFα in LPS-stimulated macrophages, without interfering with their antigen presentation ability (Tripathi et al., 2007). In another study, treatment with an effective dose of 50 µM of 6-gingerol was found to reduce the V. cholerae-infection triggered levels of cytokines IL-1α, IL-1β, IL-6 and chemokine CXCL8. The respective mRNA levels were also reduced. This reduction in the levels of pro-inflammatory cytokines was attributed to the down-regulation of NF-κB pathway because of increase in phosphorylated IKBα and downregulation of p65 (Saha et al., 2016). Another study reported that 6-gingerol reduced IL-1β-induced inflammation by reducing the mRNA titers of IL-6 and CXCL8, reducing the COX2 over-expression and the NF-κB activity (Li et al., 2013). In a DSS-induced mouse colitis model, 6-gingerol treatment was found to successfully suppress the DSS-elevated production of pro-inflammatory cytokines IL-1β, IL-12 and TNFα. An in vitro study on DSS-treated Caco-2 cells subject to 6-gingerol therapy revealed AMPK activation following treatment; suggesting that the anti-inflammatory effect of 6-gingerol could be exerted through AMPK activation (Chang et al., 2015). The production of pro-inflammatory cytokines TNFα, IL-1β and IL-12 in LPS stimulated murine peritoneal macrophage was inhibited on treating with 6-gingerol (Tripathi et al., 2007). In another study carried out on C6 astroglioma cells treated with LPS, treatment with 6-gingerol was found to attenuate the production of pro-inflammatory cytokines in a dose dependent manner. Additionally, in the in vivo studies, 6-gingerol also inhibited the LPS-induced increase of TNFα levels in the rat brain (Zhang et al., 2018). The effect of 6-gingerol on cytokine production was evaluated in a DSS-induced colitis mouse model. 6-gingerol inhibited the DSS-stimulated rise in the serum levels of cytokines IL-6 and IL-17 and their respective mRNA levels. Moreover, it also inhibited the DSS-induced decrease in the serum levels and mRNA levels of the anti-inflammatory cytokine. IL-10 (Sheng et al., 2020). Gingerol is a potential natural immunomodulatory agent that may prove to be useful in regulation of the cytokine storm observed in COVID-19 when used in conjunction with standard antiviral therapy.
Luteolin
A naturally occurring flavonoid, luteolin is 2-(3,4-dihydroxyphenyl)-5,7-dihydroxychromen-4-one. It is found in many vegetables (celery, parsley, broccoli, carrots, peppers, and cabbages); fruits (apple skins); flowers (chrysanthemum) and in medicinal herbs. It is a potent antioxidant, inhibits tumor cell proliferation and suppresses metastasis. It is also an anti-inflammatory agent and an immune system modulator (Lin et al., 2008; National Center for Biotechnology Information, 2020g). Luteolin exhibits a concentration-dependent inhibition of the production of TNFα and IL-1β in LPS/IFN-induced primary microglia and BV-2 microglial cells. This inhibitory effect results from an inhibition on NF-κB, STAT1 and IRF1; all essentially required for the transcription of pro-inflammatory genes (Kao et al., 2011). In another study, a significant inhibition of TNFα, IL-6, CXCL8, GM-CS and suppression of NF-κB activation was observed with luteolin treatment on PMA plus A23187-induced HMC-1 cells (Kang et al., 2010). In human monocytes under hyperglycemic condition, luteolin brought about a significant reduction in the release of IL-6 and TNFα by suppressing NF-κB activity (Kim et al., 2014). In a study with human whole blood incubated with LPS, luteolin effectively inhibited the production of IL-1β, IL-6, TNFα and IFNγ (Ribeiro et al., 2010). Primary murine microglia and BV-2 microglial cells stimulated with LPS exhibited significant increase in levels of inflammatory cytokine, IL-6. Pretreatment with luteolin was found to attenuate this increase in IL-6; both at mRNA and protein level. While luteolin was found to bring about a marked reduction in the binding activity of AP1 transcription factor and inhibit JNK phosphorylation, it had no significant effect on the LPS-induced increase in NF-κB DNA binding activity nor the LPS-induced IκBα degradation. Furthermore, the in vivo studies on mice treated with luteolin revealed that it successfully reduced the plasma levels of IL-6 and the mRNA levels of IL-6 in the hippocampus, but not in the cortex or cerebellum. This inhibitory activity of luteolin on the LPS-induced production of IL-6 can be attributed to its ability to inhibit the both JNK signaling pathway and the activation of AP1 in the microglia (Jang et al., 2008). Luteolin was also found to significantly reduce the serum levels of pro-inflammatory cytokines IL-1β, IL-6 and TNFα in MSU induced-inflammation in rats (Lodhi et al., 2019). Luteolin effectively inhibited the production of TNFα and IL-6 in LPS/INFγ stimulated RAW264.7 cells. NF-κB expression was also suppressed in a dose-dependent manner, following luteolin treatment (Lee et al., 2016). Luteolin pretreatment was found to bring a marked decrease in the mRNA expression and the release of IL-6, IL-8 and VEGF in a dose dependent manner in human HaCaT and primary keratinocytes on TNF stimulation. The TNF-induced phosphorylation, nuclear translocation and DNA binding of NF-κB was significantly brought down by treatment with luteolin. Furthermore, the TNF-induced mRNA expression of the NFKB1 and RELA genes which encode the two NF-κB subunits (NF-κB p50 and NF-κB p65, respectively) was also reduced by luteolin treatment, suggesting that luteolin reduces pro-inflammatory cytokine production by decreasing NF-κB induction (Weng et al., 2014). Luteolin also has broad anti-viral activity, inhibits mast cells and potentially inhibits SARS-CoV-2 main protease (3CLpro) as revealed in recent in docking studies (Theoharides, 2020). Taken together with its immunomodulatory properties, luteolin is an important natural agent for consideration as a potential therapeutic that can be further evaluated as an adjuvant to ameliorate the cytokine storm observed in COVID-19 and to potentially inhibit SARS-CoV-2 infection. Further clinical trails in this regard to ascertain the safety, efficacy and dosage for COVID-19 treatment needs to be conducted.
Melatonin
Melatonin is a bioactive compound reported to be found in numerous plants species. Chemically, it is a tryptophan-derived substituted indolamine; N-acetyl-5-methoxytryptamine. It is a universal amphiphilic antioxidant and an anti-inflammatory molecule (Salehi et al., 2019). Melatonin is found in the roots of Huang-qin (Scutellaria baicalensis Georgi.), curcuma (Curcuma aeruginosa Roxb.); leaves and flowers of St. John’s wort (Hypericum perforatum L.); leaves of Tanacetum parthenium (L.) Sch. Bip., black pepper (Piper nigrum L.); seeds of corn (Zea mays L.), rice (Oryza sativa L.), black mustard (Brassica nigra (L.) K.Koch), white mustard (Brassica hirta Moench), wolf berry (Lycium barbarum L.), fennel (Foeniculum vulgare Mill.), sunflower (Helianthus annuus L.), fenugreek (Trigonella foenum-graecum L.), barley (Hordeum vulgare L.), almonds (Prunus amygdalus Batsch), coriander (Coriandrum sativum L.), celery (Apium graveolens L.), anise (Pimpinella anisum L.), poppy (Papaver somniferum L.), flax (Linum usitatissimum L.) and in the bean of coffee (Coffea canephora Pierre. Ex A.Froehner, Coffea arabica L.). The presence of melatonin has been reported in many plant species belonging to the families Rosaceae, Vitaceae, Poaceae, Apiaceae and Brassicaceae (Nawaz et al., 2016). Melatonin has been reported to possess indirect anti-viral activity due to its anti-inflammatory activity. It down-regulates the production and release of pro-inflammatory cytokines, inflammation and cell recruitment. This down-regulation and consequent anti-inflammatory activity of melatonin is because it suppresses the activation of NF-κB, a key transcription factor involved in the production of pro-inflammatory cytokines. In addition, studies revealed that melatonin is able to cause significant decrease in serum levels of IL-6, TNFα, IL-1β (Tarocco et al., 2019; Zhang et al., 2020b). Melatonin efficiently suppressed the production of IL-8 in acrolein-induced human pulmonary fibroblasts (Kim et al., 2012). Treatment with melatonin was found to significantly suppress pro-inflammatory cytokine production and inhibit NLRP3 inflammasome activation in a study carried out in cadmium–induced liver injury in male C57BL/6 mice (Cao et al., 2017). In another study carried out on BV2 murine microglial cells, the LPS-induced production of chemokines MCP1, CCL5 and CCL9 mRNA expression was significantly inhibited by melatonin treatment. Melatonin also inhibited the AkT phosphorylation and NF-κB activation induced by LPS exposure. Moreover, LPS-induced STAT1/3 phosphorylation and interferon-gamma activated sequence (GAS)-driven transcriptional activity was also inhibited as a result of melatonin treatment. This suggests that the anti-inflammatory activity of melatonin is mediated by inhibiting the NF-κB and STAT/GAS activation in LPS-stimulated BV2 murine microglial cell line (Min et al., 2012). A recent mechanistic analysis suggested that supplementation with melatonin can efficiently downregulated the cytokine storm in COVID-19 by reversing aerobic glycolysis in immune cells (Reiter et al., 2020). Short-term usage of melatonin is safe; melatonin has a high safety profile. Infrequently occurring side-effects reported are only occasional dizziness, headache, nausea and sleepiness. These findings support the use of melatonin as an immunosuppressive adjuvant to reduce the cytokine storm syndrome observed in COVID-19 (Zhang et al., 2020b).
Morphine and Codeine
An opiate alkaloid obtained from the plant Papaver somniferum L., morphine (4R,4aR,7S,7aR,12bS)-3-methyl-2,4,4a,7,7a,13-hexahydro-1H-4,12-methanobenzofuro[3,2-e]isoquinoline-7,9-diol) binds to and activates the δ, µ, κ opiate receptors involved in controlling different brain functions. Morphine is an analgesic, brings about sedation, respiratory depression and gastrointestinal system smooth muscle contraction (National Center for Biotechnology Information, 2020h). The immunosuppressive activity of morphine has been known in clinical medicine for over hundred years (Dinda et al., 2005). Several studies have established the immunosuppressive activity of morphine with respective to cytokine production. In vivo studies have revealed that morphine inhibits the production of TNFα, IFNγ, MCP 1, IL-12 and IL-6 (Clark et al., 2007; Fukada et al., 2012; Cruz et al., 2017). Morphine has the ability to reduce the production of TNFα, IL-1 and IL-6 (Chang et al., 2011). Another study evaluated the effect of morphine on the intraperitoneal release of TNFα and MCP1 in Swiss–Webster, C57BL/6J, mast cell deficient Kit Wsh/Wsh (W-sh) and mast cell reconstituted (W-sh-rec) mice. While morphine inhibited the LPS-induced TNFα release, it had no significant effect on the MCP1 release in the intraperitoneal cavity (Madera-Salcedo et al., 2011). Morphine treatment resulted in significant reduction in the levels of TNFα, IL-1, IL-2 and MIP2 in the bronchoalveolar lavage fluids and in lung tissue in CB6F1 male mice. Morphine treatment also inhibited transcription factor NF-κB in lung resident cells (Wang et al., 2005). Codeine (4R,4aR,7S,7aR,12bS)-9-methoxy-3-methyl-2,4,4a,7,7a,13-hexahydro-1H-4,12-methanobenzofuro[3,2-e]isoquinolin-7-ol) is a naturally occurring phenanthrene alkaloid and an opioid agonist also obtained from P. somniferum L., also known for its analgesic, anti-diarrheal and antitussive properties (National Center for Biotechnology Information, 2020i). Treatment with codeine was found to significantly suppress the production of IL-2 in ConA-stimulated splenocytes extracted from male Swiss mice (Sacerdote et al., 1997). Morphine and codeine bind directly to ACE2 with high affinity, as revealed by docking studies. This can hypothetically reduced receptor-mediated cytokine release. This makes morphine and codeine candidate immunosuppressant adjuvants for regulating the cytokine storm observed in COVID-19 (Roshanravan et al., 2020b). However, further studies need to be conducted to study the use of morphine or codeine for COVID-19, given that the timing of morphine administration greatly effects its influence on cytokine production; late administration increases cytokine production and that codeine was found to induce the production of cytokines and chemokines by mast cells in vitro (Sheen et al., 2007; Fukada et al., 2016).
Nicotine
Nicotine is a plant alkaloid found in the tobacco plant (Nicotiana tabacum L.). Chemically, it is 3-(1-methylpyrrolidin-2-yl) pyridine. It is an immunomodulator, a peripheral nervous system drug and a psychotropic drug (National Center for Biotechnology Information, 2020j). Nicotine is can also be found in some members of the families Asclepiadaceae, Crassulaceae, Solanaceae (Dawson et al., 1960). It is used clinically in the treatment of ulcerative colitis to counteract inflammation. As it is a cholinergic agonist, nicotine is an inhibitor of pro-inflammatory cytokines acting through the cholinergic anti-inflammatory pathway via a7-nicotinic acetylcholine receptor (a7-nAChRs). Nicotine inhibits TNF, IL- 1 and IL-6 production. The underlying mechanism of action involves the activation of a7-nAChRs on inflammatory cells like macrophages and neutrophils that induces the suppression of the NF-κB activation and consequently suppression of the secretion of pro-inflammatory cytokines and chemokines from inflammatory cells. Nicotine brought about an inhibition in the secretion of IFNγ, TNFα, IL-1β and IL-2 but had no effect on the production of IL-6 in as study carried out on PBMC triggered by HT-29 colon carcinoma cells. However, nicotine treatment in the in PBMC triggered by RKO colon carcinoma cells showed no suppressive effect on the cytokine production (Djaldetti and Bessler, 2017). Nicotine inhibits the LPS-induced production of TNFα and NF-κB in human macrophages and splenocytes. This inhibitory effect is attributed to the ability of nicotine to activate JAK2 and STAT3 and is mediated by tristetrapolin (TTP) expression in macrophages (Lakhan and Kirchgessner, 2011). Studies have showed that nicotine has the ability to inhibit the production of IL-1, IL-6, IL-12, INFγ, MIP1, TNFα. Nicotine inhibits the transcriptional activity of NF-κB by suppressing the phosphorylation of I-κB (Cloëz-Tayarani and Changeux, 2007; Piao et al., 2009). Nicotine is also an accessible, approved treatment. Hence, it is hypothesized that nicotine is a suitable adjuvant that can ameliorate the cytokine storm in COVID-19 and can likely reduce the rising mortality in the short term (Farsalinos et al., 2020; Gonzalez-Rubio et al., 2020).
Piperine
Piperine is an alkaloid obtained from the plant Piper nigrum L. and other plants from the family Piperaceae. Chemically, it is N-acylpiperidine that is piperidine substituted by a (1E,3E)-1-(1,3-benzodioxol-5-yl)-5-oxopenta-1,3-dien-5-yl group at the nitrogen atom (2E,4E)-5-(1,3-benzodioxol-5-yl)-1-piperidin-1-ylpenta-2,4-dien-1-one (National Center for Biotechnology Information, 2020k). Piperine is known to possess numerous therapeutic effects; antioxidant, anti-inflammatory, antimicrobial, anti-metastatic and hepatoprotective activities, to name a few. It is also an immunomodulator and is known for its bioavailability enhancement property of therapeutic drugs (Gorgani et al., 2017). Piperine was reported to inhibit the production of Th2 cytokines IL-4 and IL-5 in an ovalbumin-induced asthma model (Kim and Lee, 2009). Another study reported that piperine inhibits LPS-induced expression and production of pro-inflammatory cytokines IL-1β, IL-6, IL-10 and TNFα by inhibiting NF-κB activation (Dzoyem et al., 2017). Pipeine also protects macrophages from pyroptosis and suppresses the release of IL-1β by suppressing ATP-induced AMPK activation in murine macrophages (Liang et al., 2016). In another study carried out using human PBMC, piperine was found to inhibit the production of IL-2 and IFNγ in a dose-dependent manner by suppressing the IL-2 and IFNγ mRNA expression, as revealed by ELISA assay and RT-PCR data (Chuchawankul et al., 2012). Piperine was found to selectively suppress the expression of S. aureus-induced pro-inflammatory cytokines IL-1β, IL-6 and TNFα and increase the expression of the anti-inflammatory cytokine IL-10 by inhibiting the NF-κB and MAPK pathways in an experimental study in mice (Zhai et al., 2016). The IL-6 expression by IL-1β stimulated fibroblast-like synoviocytes derived form patients with rheumatoid arthritis was successfully inhibited by piperine treatment. Additionally, piperine could inhibit the migration of activator protein 1 (AP-1), but not nuclear factor (NF)κB into the nucleus (Bang et al., 2009). Piperine was found to bring about a reversal in the LPS-induced increase of pro-inflammatory cytokines IL-1β, IL-6 and TNFα production in a study carried out on human colonic epithelial cells. This attenuation of cytokine release was attributed to the ability of piperine to activate IκBα and consequently suppress NF-κB expression (Buagaew et al., 2020). Significant reduction in the levels of IL-1β, IL-6, TNFα and GM-CSF in B16F-10 melanoma cells was observed on treatment with piperine. Additionally, piperine treatment also reduced the nuclear translocation of p65, p50, c-Rel subunits of NF-kappaB and other transcription factors such as ATF-2, c-Fos and CREB. Piperine inhibits the activation of NF-κB as it suppresses the degradation of IκBα and the translocation of p65 from the cytosol to the nucleus (Pradeep and Kuttan, 2004; Chung, 2019; Yang et al., 2019). Additionally, recent docking studies revealed that piperine exhibits significant binding affinity toward the spike glycoprotein of SARS-CoV-2 and the ACE2 receptor. Hence, piperine may prove to be a useful therapeutic agent not only for restricting attachment of virus to the host cells but also for targeting the cytokine storm by suppressing the activity of NF-κB and MAPK pathways and subsequent pro-inflammatory cytokines release (Maurya et al., 2020).
Quercetin
Quercetin (2-(3,4-dihydroxyphenyl)-3,5,7-trihydroxychromen-4-one) is a polyphenolic flavonoid found in abundance in many plants including broccoli, red onions, eggplant, potatoes and green leafy vegetables including celery, lettuce; fruits including apples, citrus fruits, red grapes, tomatoes; berries including cranberries and raspberries. The extract of quercetin has been used for prevention and treatment of rheumatic disease, cardiovascular disease, hypercholesterolemia, infections and cancers (Li et al., 2016; National Center for Biotechnology Information, 2020l). Quercetin was found to effect the production of cytokines; it was found to decrease the production of pro-inflammatory cytokines TNFα, IL-6, G-CSF, GM-CSF and VEGF and chemokines IP10 and MCP1 in mouse macrophages induced with polyinosinic-polycytidylic acid, and bring about an increase in the level of anti-inflammatory cytokine IL 27 in influenza A-treated MDCK cells (Kim and Park, 2016; Mehrbod et al., 2018). It was also found to stimulate T-helper cells to produce IFNγ and downregulate Th2-derived IL-4 in cultured blood peripheral mononuclear cells (Biancatelli et al., 2020). Carrageenin-induced production of IL-1β was significantly reduced with quercetin treatment (Valério et al., 2009). Quercetin was responsible for a marked reduction in the production of TNFα, in a dose-dependent manner in a study in normal PBMC. This inhibitory effect of quercetin on the pro-inflammatory cytokine TNFα is mediated via the modulation of NF-κB1 and IκB (Nair et al., 2006). Quercetin treatment was found to bring about a reduction in the LPS-induced increasing levels of inflammatory factors IL-1β, IL-6 and TNFα in RAW 264.7 cells. The ability of quercetin to suppress the production of pro-inflammatory cytokines is mediated by its suppression of the activation of ERK and p38 MAP kinase and NF-κB/IκB signal transduction pathways (Tang et al., 2019). In a study on LPS-stimulated RAW 264.7 cells, quercetin decreased the activation of phosphorylated ERK kinase and p38 MAP kinase. No significant effect on JNK MAP kinase was observed. Additionally, quercetin also inhibited the activation of NF-κB/IκB complex and the degradation of IκB (Cho et al., 2003). Quercetin brought about a dose-dependent reduction in the levels of IL-6, IL-8, MCP1 and ICAM1 in IL-1β-stimulated human retinal pigment epithelial (ARPE-19) cells. Quercetin was found to inhibit the signaling pathways related to the inflammatory process; including inhibition of the phosphorylation of mitogen-activated protein kinases (MAPKs), inhibiting the nuclear factor κ-B kinase (IKK)α/β, c-Jun, cAMP response element-binding protein (CREB), activating transcription factor 2 (ATF2) and nuclear factor (NF)-κB p65, and blocking the translocation of NF-κB p65 into the nucleus (Cheng et al., 2019). Another study on patients with coronary artery disease showed that treatment with quercetin brought about a reduction in the levels of IL-1β, TNFα and the levels of IL-10 tended to decrease. This was attributed to a decrease in the transcriptional activity of NF-κB (Chekalina et al., 2018). Oral supplementation with quercetin treatment is relatively safe, causing no significant adverse effects; headache and temporary peripheral paresthesia were the only effects observed in two members out of thirty in a study. The use of quercetin as an adjuvant to concurrently fortify the immune response by promoting IFNs production and modulating the levels of pro- and anti-inflammatory cytokines may complement currently used interventions in treating COVID-19 (Biancatelli et al., 2020).
Resveratrol
A polyphenolic phytoalexin, resveratrol (5-[(E)-2-(4-hydroxyphenyl)ethenyl]benzene-1,3-diol) is found in more than 70 species of plants, such as grapes, cranberry, blueberry, mulberry, peanuts, jackfruit, soy and wine. Resveratrol is known to posses a number of beneficial health effects; it is has antioxidant, anticancer, anti-inflammatory, antiviral, anti-aging and life-prolonging effects (Bansal et al., 2018; National Center for Biotechnology Information, 2020m). Resveratrol is an efficient immunomodulator; it interferes with immune cell regulation, pro-inflammatory cytokine synthesis and gene expression, thus regulating immunity (Malaguarnera, 2019). In a study carried out, resveratrol caused an irreversible inhibition of IFNγ and IL-2 by splenic lymphocytes and the production of TNFα and IL-12 by peritoneal macrophages. The activation of NF-κB was blocked without affecting the basal NF-κB activity (Gao et al., 2001). In another study inflammatory cytokine and chemokine release (GM-CSF and CXCL8) by stimulated alveolar macrophages from COPD patients was blocked by resveratrol (Culpitt et al., 2003). Resveratrol blocks the increased secretion of IL-6 and TNFα in EV71-infected RD cells (Zhang et al., 2015). In yet another study, treatment with resveratrol induced a dose-dependent inhibition of IL-1α, IL-6 and TNFα in vitro and downregulated the production of IL-17 in HTLV-1 infected cells (Fuggetta et al., 2016). Resveratrol treatment was found to be associated with downregulation of NF-κB in the inflammatory cells of the lungs (Rieder et al., 2012). LPS-induced phosphorylation and degradation of IκBα is blocked in macrophages subject to resveratrol treatment decreasing the NF-κB DNA binding activity. The inhibitory effect of resveratrol on the NF-κB pathway correlates with its ability to bring about a reduction in IKK activity (Yamamoto and Gaynor, 2001). In a study evaluating the efficacy of resveratrol dry suspension in pigs, it was found that resveratrol upregulated the release of IFNγ and downregulated the release of TNFα; thus regulating the humoral immune responses (Fu et al., 2018). A dose-dependent reduction in the levels of pro-inflammatory cytokines IL-6, IL-8 and MCP1 was observed with resveratrol treatment in adipocytes under inflammatory conditions (Zagotta et al., 2015). The inhibitory effect of resveratrol on TNFα, IL-6 and MIP2 levels was established in a study on rabbit model of acute pharyngitis. Resveratrol reduced the protein expression of IL-1β and IL-18. Furthermore, treatment with resveratrol resulted in suppression of TLR4 and myeloid differentiation primary response protein 88 protein expression, reduced p-NF-κB and increased p-IκB protein expression (Zhou et al., 2018). A decreased production of cytokines IL-1β, IL-6, IL-8, IL-12 and TNFα was observed on treatment with resveratrol in a dose- and time-dependent (Rizzo et al., 2012). Resveratrol is considered safe when taken at supplemental doses. Resveratrol can be an adjunctive agent to consider for SARS-CoV-2 infection, to mitigate the cytokine storm and consequently reduce inflammation (Marinella et al., 2020).
A few more plant-derived immunosuppressants that can be potentially used as adjuvants in COVID-19 therapy are described in Table 1.
Conclusion
The COVID-19 pandemic is affecting millions of people across the globe. In some patients infected with SARS-CoV-2, the immunological reaction involves mobilization and sustained production of several pro-inflammatory cytokines and chemokines, giving rise to a cytokine storm. This cytokine storm appears to be one of the common causes of mortality in this disease. This necessitates a suitable therapeutic strategy that can efficiently attenuate this excessive host immune response. Different synthetic immunomodulatory agents such as corticosteroids, interleukin receptor antagonists, JAK/STAT inhibitors and monoclonal antibodies like tocilizumab, sarilumab, infliximab and adalimumab that target specific cytokines are being evaluated for treatment of COVID-19 patients. Unfortunately, most of these immunomodulators are known to cause adverse effects, thus limiting their use. Plant-derived immunosuppressants present an invaluable alternative to manage the cytokine storm syndrome observed in COVID-19. They have a good safety profile and specifically target the production and release of certain cytokines by suppressing definite reactions in the signaling cascades. Additionally, some of these agents also exhibit antiviral properties against coronaviruses and some others can potentially inhibit the main protease of SARS-CoV-2. Clinical trails to investigate the dosage, administration timing, bioefficacy, bioavailability, mechanism of action and safety with respect to COVID-19 is the need of the hour to capitalize on this promising therapeutic approach. A combination treatment including plant-derived immunosuppressants along with antiviral agents and other drugs will be effective in tackling SARS-CoV-2 infection, rather than the use of a single drug alone. Such a synergistic therapeutic approach with the inclusion of natural immunosuppressants to ameliorate the cytokine storm holds great treatment potential.
Author Contributions
AEP conceived the idea, surveyed the literature and wrote the manuscript; BVS, BGR, and VLK were involved in manuscript revision, read and approved the submitted version.
Conflict of Interest
The authors declare that the research was conducted in the absence of any commercial or financial relationships that could be construed as a potential conflict of interest.
Acknowledgments
The authors wish to acknowledge Andhra University for providing the necessary facilities.
Glossary
ACE Angiotensin-converting enzyme
AMPK 5’AMP-activated protein kinase
ARDS Acute respiratory distress syndrome
ASLN Accelerated and severe lupus nephritis
BMDM Bone-marrow derived macrophage
COPD Chronic obstructive pulmonary disease
COVID-19 Coronavirus disease 2019
COX Cyclooxygenase
CRP C-reactive protein
DSS Dextran sulfate sodium
EAC Ehrlich ascites carcinoma
ELISA Enzyme-linked immunosorbent assay
ESR Erythrocyte sedimentation rate
FMF Familial Mediterranean fever
FLS Fibroblast-like synoviocytes
G-CSF Granulocyte colony-stimulating factor
GM-CSF Granulocyte-macrophage colony-stimulating factor
HGF Hepatocyte growth factor
HIV Human immunodeficiency virus
HMC Human mast cell line
ICAM Intercellular adhesion molecule
IFN Interferon
II/R Intestinal ischemia and reperfusion
IkBα I-kappa B-alpha
IKKβ Inhibitor of nuclear factor kappa-B kinase subunit beta
IL Interleukin
iNOS Inducible nitric oxide synthase
IP Interferon gamma-induced protein
IRF Interferon regulatory factor
IVIG Intravenous immunoglobulin
JAK Janus kinase
LPS Lipopolysaccharide
MAPK Mitogen activated protein kinase
MASP Mannose-binding lectin serine protease
MCP Monocyte chemoattractant protein
MCSF Macrophage colony-stimulating factor
MIG Monokine induced by gamma; CXCL9
MIP Monocyte chemoattractant protein
MOF Multiple organ failure
MSC Mesenchymal stem cell
MSU Monosodium urate
NADPH Nicotinamide adenine dinucleotide phosphate
NF-κB Nuclear factor kappa-light-chain-enhancer of activated B cells
NK Natural killer
NLR NOD-like receptor
NOX2 NADPH oxidase 2
NOD Nuclear-binding and oligomerization domain
OVA Ovalbumin
PAMP Pathogen-associated molecular pattern
PBMC Peripheral blood mononuclear cells
PCT Procalcitonin time
PDGF Platelet-derived growth factor
pERK Phosphorylated extracellular signal-regulated kinase
PF Pulmonary fibrosis
PGE2 Prostaglandin E2
pJNK Phosphorylated c-Jun N-terminal kinase
PKC protein kinase C
PMA Phorbol 12-myristate 13-acetate
pMACO Permanent middle cerebral artery occlusion
PT Prothrombin time
RA Rheumatoid arthritis
RBD Receptor binding domain
RD Rhabdosarcoma
rhACE recombinant hACE
RIG Retinoic acid-inducible gene I
RLR Rig-I-like receptor
RNA Ribonucleic acid
RT-PCR Reverse transcription polymerase chain reaction
SARS-CoV-2 Severe acute respiratory syndrome coronavirus 2
STAT Signal transducer and activator of transcription
TLR Toll-like receptor
TNBS 2,4,6-trinitrobenzene sulfonic acid
TNF Tumor necrosis factor
VEGF Vascular endothelial growth factor
References
Acharya, D., Liu, G., and Gack, M. U. (2020). Dysregulation of type I interferon responses in COVID-19. Nat. Rev. Immunol. 20, 397. doi:10.1038/s41577-020-0346-x
Alijotas-Reig, J., Esteve-Valverde, E., Belizna, C., Selva-O'Callaghan, A., Pardos-Gea, J., Quintana, A., et al. (2020). Immunomodulatory therapy for the management of severe COVID-19. Beyond the anti-viral therapy: a comprehensive review. Autoimmun. Rev. 19, 102569. doi:10.1016/j.autrev.2020.102569
Alwis, R., Chen, S., Gan, E. S., and Ooi, E. E. (2020). Impact of immune enhancement on COVID-19 polyclonal hyperimmune globulin therapy and vaccine development. EBioMed. 55, 102768. doi:10.1016/j.ebiom.2020.102768
Arreola, R., Quintero-Fabián, S., López-Roa, R. I., Flores-Gutiérrez, E. O., Reyes-Grajeda, J. P., Ortino Sahagun, D., et al. (2015). Immunomodulation and anti-inflammatory effects of garlic compounds. J. Immunol. Res. 2015, 401630. doi:10.1155/2015/401630
Astuti, I., and Ysrafil, (2020). Severe Acute Respiratory Syndrome Coronavirus 2 (SARS-CoV-2): an overview of viral structure and host response. Diabetes Metab. Syndr. 14, 407. doi:10.1016/j.dsx.2020.04.020
Atal, S., and Fatima, Z. (2020). IL-6 inhibitors in the treatment of serious COVID-19: a promising therapy? Pharm. Med. 34, 223. doi:10.1007/s40290-020-00342-z
Babaei, F., Nassiri‐Asl, M., and Hosseinzadeh, H. (2020). Curcumin (a constituent of turmeric): new treatment option against COVID‐19. Food Sci. Nutr. 8, 5215. doi:10.1002/fsn3.1858
Bachiega, T. F., de Sousa, J. P., Bastos, J. K., and Sforcin, J. M. (2012). Clove and eugenol in noncytotoxic concentrations exert immunomodulatory/anti-inflammatory action on cytokine production by murine macrophages. J. Pharm. Pharmacol. 64, 610. doi:10.1111/j.2042-7158.2011.01440.x
Bachiega, T. F., and Sforcin, J. M. (2011). Lemongrass and citral effect on cytokines production by murine macrophages. J. Ethnopharmacol. 137, 909. doi:10.1016/j.jep.2011.07.021
Bagca, B. G., and Avci, C. B. (2020). The potential of JAK/STAT pathway inhibition by ruxolitinib in the treatment of COVID-19. Cytokine Growth Factor Rev. 54. 51–61, doi:10.1016/j.cytogfr.2020.06.013
Banerjee, A., Czinn, S. J., Reiter, R. J., and Blanchard, T. G. (2020). Crosstalk between endoplasmic reticulum stress and anti-viral activities: a novel therapeutic target for COVID-19. Life Sci. 255, 117842. doi:10.1016/j.lfs.2020.117842
Bang, J. S., Oh, D. H., Choi, H. M., Sur, B. J., Lim, S. J., et al. (2009). Anti-inflammatory and antiarthritic effects of piperine in human interleukin 1beta-stimulated fibroblast-like synoviocytes and in rat arthritis models. Arthritis Res. Ther. 11, R49. doi:10.1186/ar2662.R49
Bansal, M., Singh, N., Pal, S., Dev, I., and Ansari, K. M. (2018). Chemopreventive role of dietary phytochemicals in colorectal cancer. Adv. Mol. Toxic. 12, 69. doi:10.1016/B978-0-444-64199-1.00004-X
Barboza, J. N., da Silva Maia Bezerra Filho, C., Silva, R. O., Medeiros, J. V. R., and de Sousa, D. P. (2018). An overview on the anti-inflammatory potential and antioxidant profile of eugenol. Oxid. Med. Cell Longev., 2018, 3957262. doi:10.1155/2018/3957262
Barone, M., Ucciferri, C., Cipollone, G., and Mucilli, F. (2020). Recombinant human angiotensin-converting enzyme 2 and COVID-19 acute respiratory distress syndrome: a theoretical or a real resource? Ejmo 4, 2. doi:10.14744/ejmo.2020.47992
Batiha, G. E. S., Beshbishy, A. M., Wasef, L. G., Elewa, Y. H. A., Al-Sagan, A. A., et al. (2020). Chemical constituents and pharmacological activities of garlic (Allium sativum L.): a review. Nutrients 12, 3. doi:10.3390/nu12030872
Bian, Y., Liu, P., Zhong, J., Hu, Y., Fan, Y., Zhuang, S., et al. (2019). Kaempferol inhibits multiple pathways involved in the secretion of inflammatory mediators from LPS‑induced rat intestinal microvascular endothelial cells. Mol. Med. Rep. 19. 1958–1964. doi:10.3892/mmr.2018.9777
Biancatelli, R. M. L. C., Berrill, M., Catravas, J. D., and Marik, P. E. (2020). Quercetin and vitamin C: an experimental, synergistic therapy for the prevention and treatment of SARS-CoV-2 related disease (COVID-19). Front. Immunol. 11, 1451. doi:10.3389/fimmu.2020.01451
Borlinghaus, J., Albrecht, F., Gruhlke, M. C., Nwachukwu, I. D., and Slusarenko, A. J. (2014). Allicin: chemistry and biological properties. Molecules 19, 12591. doi:10.3390/molecules190812591
Buagaew, A., and Poomipark, N. (2020). Protective effect of piperine from Piper chaba fruits on LPS-induced inflammation in human intestinal cell line. J. Med. Plants Res. 14, 9. doi:10.5897/JMPR2020.6996
Burgos, R. A., Seguel, K., Perez, M., Meneses, A., Ortega, M., Loaiza, A., et al. (2005). Andrographolide inhibits IFN-gamma and IL-2 cytokine production and protects against cell apoptosis. Planta Med. 71, 429. doi:10.1055/s-2005-864138
Bustami, A., Sopiah, P., Muharam, R., and Wibowo, H. (2018). Effects of gallic acid and its derivates on inflammatory regulation of endometriotic primary cultures: study on NF-kB mRNA expression and IL-6 secretion. Biomed. Pharmacol. J. 11, 1479. doi:10.13005/bpj/1514
Cantini, F., Niccoli, L., Matarrese, D., Nicastri, E., Stobbione, P., and Goletti, D. (2020). Baricitinib therapy in COVID-19: a pilot study on safety and clinical impact. J. Infect. 81, 318. doi:10.1016/j.jinf.2020.04.017
Cao, W., Liu, X., Bai, T., Fan, H., Hong, K., Song, H., et al. (2020). High-dose intravenous immunoglobulin as a therapeutic option for deteriorating patients with coronavirus disease 2019. Open Forum infect. Dis., 7, ofaa102. doi:10.1093/ofid/ofaa102
Cao, Z., Fang, Y., Lu, Y., Tan, D., Du, C., Li, Y., et al. (2017). Melatonin alleviates cadmium-induced liver injury by inhibiting the TXNIP-NLRP3 inflammasome. J. Pineal Res. 62, e12389. doi:10.1111/jpi.12389
Carretta, M. D., Alarcón, P., Jara, E., Solis, L., Hancke, J. L., Burgos, R. A., et al. (2009). Andrographolide reduces IL-2 production in T-cells by interfering with NFAT and MAPK activation. Eur. J. Pharmacol. 602, 413–421. doi:10.1016/j.ejphar.2008.11.011
CAS (2020). Storm warning: the critical role of cytokines in severe COVID-19. Available at: https://www.cas.org/blog/covid-19-cytokine-storms (Accessed July 14, 2020).
Castiglione, V., Chiriacò, M., Emdin, M., Taddei, S., and Vergaro, G. (2020). Statin therapy in COVID-19 infection. Eur. Heart J. 6, 258–259, doi:10.1093/ehjcvp/pvaa042
Catanzaro, M., Fagiani, F., Racchi, M., Corsini, E., Govoni, S., and Lanni, C. (2020). Immune response in COVID-19: addressing a pharmacological challenge by targeting pathways triggered by SARS-CoV-2. Sig. Transduct. Target Ther. 5, 84. doi:10.1038/s41392-020-0191-1
Cena, H., and Chieppa, M. (2020). Coronavirus disease (COVID-19-SARS-CoV-2) and nutrition: is infection in Italy suggesting a connection? Front. Immunol. 11, 944. doi:10.3389/fimmu.2020.00944
Chakraborty, C., Sharma, A. R., Bhattacharya, M., Sharma, G., and Lee, S. S. (2020a). COVID-19: consider IL-6 receptor antagonist for the therapy of cytokine storm syndrome in SARS-CoV-2 infected patients. J. Med. Virol. 92, 2260. doi:10.1002/jmv.26078
Chakraborty, C., Sharma, A. R., Sharma, G., Bhattacharya, M., and Lee, S. S. (2020b). SARS-CoV-2 causing pneumonia-associated respiratory disorder (COVID-19): diagnostic and proposed therapeutic options. Eur. Rev. Med. Pharmacol. Sci. 247. 4016–4026, doi:10.26355/eurrev_202004_20871
Chang, K. W., and Kuo, C. Y. (2015). 6-Gingerol modulates pro-inflammatory responses in dextran sodium sulfate (DSS)-treated Caco-2 cells and experimental colitis in mice through adenosine monophosphate-activated protein kinase (AMPK) activation. Food Funct. 6, 3334. doi:10.1039/c5fo00513b
Chang, M. C., Fan, S. Z., Hsiao, P. N., Cheng, W. F., and Sun, W. Z. (2011). Influence of morphine on host immunity. Acta Anaesthesiol. Taiwanica 49, 105. doi:10.1016/j.aat.2011.08.003
Chekalina, N., Burmak, Y., Petrov, Y., Borisova, Z., and Manusha, Y.(2018). Quercetin reduces the transcriptional activity of NF-kB in stable coronary artery disease. Indian Heart J. 70, 593. doi:10.1016/j.ihj.2018.04.006
Chen, W., Zheng, K. I., Liu, S., Yan, Z., and Xu, C. (2020a). Plasma CRP level is positively associated with the severity of COVID-19. Ann. Clin. Microbiol. Antimicrob. 19, 18. doi:10.1186/s12941-020-00362-2
Chen, X., Zhao, B., Qu, Y., Chen, Y., Xiong, J., Yong, F., et al. (2020b). Detectable serum severe acute respiratory syndrome coronavirus 2 viral load (RNAemia) is closely correlated with drastically elevated interleukin 6 level in critically ill patients with coronavirus disease 2019. Clin. Infect. Dis., 71, 1937-1942. doi:10.1093/cid/ciaa449
Cheng, S. C., Huang, W. C., Pang, J. H., Wu, Y. H., and Cheng, C. Y. (2019). Quercetin inhibits the production of IL-1β-induced inflammatory cytokines and chemokines in ARPE-19 cells via the MAPK and NF-κB signaling pathways. Ijms 20, 2957. doi:10.3390/ijms20122957
Cho, S. Y., Park, S. J., Kwon, M. J., Jeong, T. S., Bok, S. H., Woo-Young, C., et al. (2003). Quercetin suppresses pro-inflammatory cytokines production through MAP kinases andNF-kappaB pathway in lipopolysaccharide-stimulated macrophage. Mol. Cell. Biochem. 243, 153–160. doi:10.1023/a:1021624520740
Chuchawankul, S., Khorana, N., and Poovorawan, Y. (2012). Piperine inhibits cytokine production by human peripheral blood mononuclear cells. Genet. Mol. Res. 11, 617. doi:10.4238/2012
Chung, H. Y., Jung, H. J., Kim, D. H., Bang, E., and Ha, S. Inflammatory and antioxidant activities of piperine on t-BHP-induced in Ac2F cells. SDRP-JFST 4, 777. doi:10.25177/JFST.4.4.RA.530
Clark, J. D., Shi, X., Li, X., Qiao, Y., Liang, D., Angst, M. S., et al. (2007). Morphine reduces local cytokine expression and neutrophil infiltration after incision. Mol. Pain 3, 28. doi:10.1186/1744-8069-3-28
Cloëz-Tayarani, I., and Changeux, J. P. (2007). Nicotine and serotonin in immune regulation and inflammatory processes: a perspective. J. Leukoc. Biol. 81, 599. doi:10.1189/jlb.0906544
Colafrancesco, S., Scrivo, R., Barbati, C., Conti, F., and Priori, R. (2020). Targeting the immune system for pulmonary inflammation and cardiovascular complications in COVID-19 patients. Front. Immunol. 11, 1439. doi:10.3389/fimmu.2020.01439
Connors, J. M., and Levy, J. H. (2020). COVID-19 and its implications for thrombosis and anticoagulation. Blood 135, 2033. doi:10.1182/blood.2020006000
Coperchini, F., Chiovato, L., Croce, L., Magri, F., and Rotondi, M. (2020). The cytokine storm in COVID-19: an overview of the involvement of the chemokine/chemokine-receptor system. Cytokine Growth Factor Rev. 53, 25. doi:10.1016/j.cytogfr.2020.05.003
Lancet Haematol (2020). COVID-19 coagulopathy: an evolving story. Lancet Haematol. 7, e425. doi:10.1016/S2352-3026(20)30151-4
Cruz, F. F., Rocco, P. R. M., and Pelosi, P. (2017). Anti-inflammatory properties of anesthetic agents. Crit. Care 21, 67. doi:10.1186/s13054-017-1645-x
Culpitt, S. V., Rogers, D. F., Fenwick, P. S., Shah, P., De Matos, C., Russell, R. E. K., et al. (2003). Inhibition by red wine extract, resveratrol, of cytokine release by alveolar macrophages in COPD. Thorax 58, 942. doi:10.1136/thorax.58.11.942
Cure, E., Kucuk, A., and Cure, M. C. (2020). Cyclosporine therapy in cytokine storm due to coronavirus disease 2019 (COVID-19). Rheumatol. Int., 40, 1177. doi:10.1007/s00296-020-04603-7
da Silva, C., Mariz, H. A., da Rocha Júnior, L. F., de Oliveira, P. S. S., Dantas, A. T., Galdino, S. L., et al. (2020). Hydroxychloroquine decreases Th17-related cytokines in systemic lupus erythematosus and rheumatoid arthritis patients. Clinics 68, 766. doi:10.6061/clinics/2013(06)07
Dawson, R. F., Solt, M. L., and Christman, D. R. (1960). Nicotine and its botanical sources. Ann. N. Y. Acad. Sci. 90, 7. doi:10.1111/j.1749-6632.1960.tb32611.x
de Araújo Lopes, A., da Fonseca, F. N., Rocha, T. M., de Freitas, L. B., Araújo, E. V. O., Wong, D. V. T., et al. (2018). Eugenol as a promising molecule for the treatment of dermatitis: antioxidant and anti-inflammatory activities and its nanoformulation. Oxid Med Cell Longev, 2018, 8194849. doi:10.1155/2018/8194849
Della-Torre, E., Della-Torre, F., Kusanovic, M., Scotti, R., Ramirez, G. A., Dagna, L., et al. (2020). Treating COVID-19 with colchicine in community healthcare setting. Clin. Immunol. 217, 108490. doi:10.1016/j.clim.2020.108490
Dibazar, S. P., Fateh, S., and Daneshmandi, S. (2015). Immunomodulatory effects of clove (Syzygium aromaticum) constituents on macrophages: in vitro evaluations of aqueous and ethanolic components. J. Immunot. 12, 124. doi:10.3109/1547691X.2014.912698
Dinda, A., Gitman, M., and Singhal, P. C. (2005). Immunomodulatory effect of morphine: therapeutic implications. Expet Opin. Drug Saf. 4, 669. doi:10.1517/14740338.4.4.669
Djaldetti, M., and Bessler, H. (2017). Nicotine modifies cytokine production by human mononuclears stimulated by colon cancer cells. Colorectal Canc. 3, 2. doi:10.21767/2471-9943.100046
Dubey, S., Yoon, H., Cohen, M. S., Nagarkatti, P., and Nagarkatti, M. (2018). Withaferin A associated differential regulation of inflammatory cytokines. Front. Immunol. 9, 195. doi:10.3389/fimmu.2018.00195
Duque, G. A., and Descoteaux, A. (2014). Macrophage cytokines: involvement in immunity and infectious diseases. Front. Immunol. 5, 491. doi:10.3389/fimmu.2014.00491
Dzoyem, J. P., McGaw, L. J., Kuete, V., and Bakowsky, U. (2017). “Anti-inflammatory and anti-nociceptive activities of african medicinal spices and vegetables,” in Medicinal spices and vegetables from Africa Editor V. Kuete (Cambridge, MA: Academic Press), 239–270.
Enmozhi, S. K., Raja, K., Sebastine, I., and Joseph, J. (2020). Andrographolide as a potential inhibitor of SARS-CoV-2 main protease: an in silico approach. J. Biomol. Struct. Dyn. 2020, 1. doi:10.1080/07391102.2020.1760136
Farsalinos, K., Niaura, R., Le Houezec, J., Barbouni, A., Tsatsakis, A., Kouretas, D., et al. (2020). Editorial: nicotine and SARS-CoV-2: COVID-19 may be a disease of the nicotinic cholinergic system. Toxicol. Rep. 7, 658. doi:10.1016/j.toxrep.2020.04.012
Favalli, E. G., Ingegnoli, F., De Lucia, O., Cincinelli, G., and Cimaz, R. (2020). COVID-19 infection and rheumatoid arthritis: faraway, so close! Autoimmun. Rev. 19, 102523. doi:10.1016/j.autrev.2020.102523
Felsenstein, S., Herbert, J. A., McNamara, P. S., and Hedrich, C. M. (2020). COVID-19: immunology and treatment options. Clin. Immunol. 215, 108448. doi:10.1016/j.clim.2020.108448
Filocamo, G., Mangioni, D., Tagliabue, P., Aliberti, S., Costantino, G., Minoia, F., et al. (2020). Use of anakinra in severe COVID-19: a case report. Int. J. Infect. Dis. 96, 607. doi:10.1016/j.ijid.2020.05.026
Fitzpatrick, L. R., and Woldemariam, T. (2017). Small-molecule drugs for the treatment of inflammatory bowel disease. Comprehen. Med. Chem. 2017, 495. doi:10.1016/B978-0-12-409547-2.12404-7
Fu, L., Wang, B., Yuan, T., Chen, X., Ao, Y., Li, P., et al. (2020a). Clinical characteristics of coronavirus disease 2019 (COVID-19) in China: a systematic review and meta-analysis. J. Infect. 80, 656. doi:10.1016/j.jinf.2020.03.041
Fu, Y., Cheng, Y., and Wu, Y. (2020b). Understanding SARS-CoV-2-mediated inflammatory responses: from mechanisms to potential therapeutic tools. Virol. Sin. 35, 266. doi:10.1007/s12250-020-00207-4
Fu, Q., Cui, Q., Yang, Y., Zhao, X., Song, X., Wang, G., et al. (2018). Effect of resveratrol dry suspension on immune function of piglets. Evid. base Compl. Alternative Med., 2018, 1. doi:10.1155/2018/5952707
Fuggetta, M. P., Bordignon, V., Cottarelli, A., Macchi, B., Frezza, C., Cordiali-Fei, P., et al. (2016). Downregulation of pro-inflammatory cytokines in HTLV-1-infected T cells by Resveratrol. J. Exp. Clin. Canc. Res. 35, 118. doi:10.1186/s13046-016-0398-8
Fukada, T., Kato, H., Ozaki, M., and Yagi, J. (2016). Impact of the timing of morphine administration on lipopolysaccharide-mediated lethal endotoxic shock in mice. Shock 45, 564. doi:10.1097/SHK.0000000000000541
Fukada, T., Nakayama, R., Iwakiri, H., Kato, H., and Yagi, J. (2012). Opioids inhibit lipopolysaccharide-mediated lethal shock in mice. Available at: http://www.asaabstracts.com/strands/asaabstracts/abstract.htm?year=2012&index=6&absnum=2698 (Accessed July 10, 2020).
Gabriella, D. M., Cristina, S., Concetta, R., Francesco, R., and Annalisa, C. (2020). SARS-CoV-2 infection: response of human immune system and possible implications for the rapid test and treatment. Int. Immunopharm. 84, 106519. doi:10.1016/j.intimp.2020.106519
Gao, X., Xu, Y. X., Janakiraman, N., Chapman, R. A., and Gautam, S. C. (2001). Immunomodulatory activity of resveratrol: suppression of lymphocyte proliferation, development of cell-mediated cytotoxicity, and cytokine production. Biochem. Pharmacol. 62, 1299. doi:10.1016/s0006-2952(01)00775-4
García, L. F. (2020). Immune response, inflammation, and the clinical spectrum of COVID-19. Front. Immunol. 11, 1441. doi:10.3389/fimmu.2020.01441
Gaspari, V., Zengarini, C., Greco, S., Vangeli, V., and Mastroianni, A. (2020). Side effects of ruxolitinib in patients with SARS-CoV-2 infection: two case reports. Int. J. Antimicrob. Agents, 56, 106023. doi:10.1016/j.ijantimicag.2020.106023
Gevers, S., Kwa, M. S. G., Wijnans, E., and van Nieuwkoop, C. (2020). Safety considerations for chloroquine and hydroxychloroquine in the treatment of COVID-19. Clin. Microbiol. Infect. 26, 1276. doi:10.1016/j.cmi.2020.05.006
Giamarellos-Bourboulis, E. J., Netea, M. G., Rovina, N., Akinosoglou, K., Antoniadou, A., Antonakos, N., et al. (2020). Complex immune dysregulation in COVID-19 patients with severe respiratory failure. Cell Host Microbe 27, 992. doi:10.1016/j.chom.2020.04.009
Girija, A. S. S., Shankar, E. M., and Larssson, M. (2020). Could SARS-CoV-2-induced hyperinflammation magnify the severity of coronavirus disease (CoViD-19) leading to acute respiratory distress syndrome? Front. Immunol. 11, 1206. doi:10.3389/fimmu.2020.01206
Golonka, R. M., Saha, P., Yeoh, B. S., Chattopadhyay, S., Gewirtz, A. T., Joe, B., et al. (2020). Harnessing innate immunity to eliminate SARS-CoV-2 and ameliorate COVID-19 disease. Physiol. Genom. 52, 217. doi:10.1152/physiolgenomics.00033.2020
Gonzalez-Rubio, J., Navarro-Lopez, C., Lopez-Najera, E., Lopez-Najera, A., Jimenez-Diaz, L., Navarro-Lopez, J. D., et al. (2020). Cytokine Release Syndrome (CRS) and nicotine in COVID-19 patients: trying to calm the storm. Front. Immunol. 11, 1359. doi:10.3389/fimmu.2020.01359
Gorgani, L., Mohammadi, M., Najafpour, G. D., and Nikzad, M. (2017). Piperine-the bioactive compound of black pepper: from isolation to medicinal formulations. Compr. Rev. Food Sci. Food Saf. 16, 124. doi:10.1111/1541-4337.12246
Grigore, A. (2017). Plant phenolic compounds as immunomodulatory agents. Available at: https://cdn.intechopen.com/pdfs/53564.pdf.
Guo, Y., Cao, Q., HongTan, Z. Y. Y., Chen, S. D., Shou-Deng, C., Yan, Y., et al. (2020). The origin, transmission and clinical therapies on coronavirus disease 2019 (COVID-19) outbreak - an update on the status. Military Med. Res. 7, 11. doi:10.1186/s40779-020-00240-0
Han, H., Ma, Q., Li, C., Liu, R., Zhao, L., Wang, W., et al. (2020). Profiling serum cytokines in COVID-19 patients reveals IL-6 and IL-10 are disease severity predictors. Emerg. Microb. Infect. 9, 1123. doi:10.1080/22221751.2020.1770129
Hemmat, N., Derakhshani, A., Baghi, H. B., Silvestris, N., and Baradaran, B. (2020). Neutrophils, crucial, or harmful immune cells involved in coronavirus infection: a bioinformatics study. Front. Genet. 11, 641. doi:10.3389/fgene.2020.00641
Herb, F. S., Raymond, A. M., Marcia, S., and Denise, K. (2020). Cellular immune responses to COVID-19. Br. Med. J. 370, m3018. doi:10.1136/bmj.m3018
Hewlings, S. J., and Kalman, D. S. (2017). Curcumin: a review of its' effects on human health. Foods 6, 92. doi:10.3390/foods6100092
Horby, P., Lim, W. S., Emberson, J., Mafham, M., Bell, J., Linsell, L., et al. (2020). Dexamethasone in hospitalized patients with COVID-19: preliminary report. N. Engl. J. Med. doi:10.1056/NEJMoa2021436
Hotez, P. J., Bottazzi, M. E., and Corry, D. B. (2020). The potential role of Th17 immune responses in coronavirus immunopathology and vaccine-induced immune enhancement. Microb. Infect. 22 (4-5), 165–167. doi:10.1016/j.micinf.2020.04.005
Hou, W., Zhang, W., Jin, R., Liang, L., Xu, B., et al. (2020). Risk factors for disease progression in hospitalized patients with COVID-19: a retrospective cohort study. Inf. Disp., 52, 498. doi:10.1080/23744235.2020.1759817
Huang, Y. F., Bai, C., He, F., Xie, Y., and Zhou, H. (2019). “Anti-inflammatory properties of Schinus terebinthifolius and its use in arthritic conditions,” in Bioactive Food as Dietary Interventions for Arthritis and Related Inflammatory Diseases. Editor R. R. Watson (Cambridge, MA: Academic Press), 489–505.
Huet, T., Beaussier, H., Voisin, O., Jouveshomme, S., Dauriat, G., Lazareth, I., et al. (2020). Anakinra for severe forms of COVID-19: a cohort study. Lancet Rheumatol. 2, e393. doi:10.1016/S2665-9913(20)30164-8
Hui, Q., Ammeter, E., Liu, S., Yang, R., Lu, P., Lahaye, L., et al. (2020). Eugenol attenuates inflammatory response and enhances barrier function during lipopolysaccharide-induced inflammation in the porcine intestinal epithelial cells. J. Anim. Sci. 98, 8. doi:10.1093/jas/skaa245
Iannaccone, G., Scacciavillani, R., Del Buono, M. G., Camilli, M., Ronco, C., Abbate, A., et al. (2020). Weathering the cytokine storm in COVID-19: therapeutic implications. Cardiorenal Med. 10, 277. doi:10.1159/000509483
Iwasaki, M., Saito, J., Zhao, H., Sakamoto, A., Hirota, K., et al. (2020). Inflammation triggered by SARS-CoV-2 and ACE2 augment drives multiple organ failure of severe COVID-19: molecular mechanisms and implications. Inflammation 44, 13–34. doi:10.1007/s10753-020-01337-3
Jain, S. K., Rains, J., Croad, J., Larson, B., and Jones, K. (2009). Curcumin supplementation lowers TNF-alpha, IL-6, IL-8, and MCP-1 secretion in high glucose-treated cultured monocytes and blood levels of TNF-alpha, IL-6, MCP-1, glucose, and glycosylated hemoglobin in diabetic rats. Antioxidants Redox Signal. 11, 241. doi:10.1089/ars.2008.2140
Jang, S., Kelley, K. W., and Johnson, R. W. (2008). Luteolin reduces IL-6 production in microglia by inhibiting JNK phosphorylation and activation of AP-1. Proc. Natl. Acad. Sci. U.S.A. 105, 7534. doi:10.1073/pnas.0802865105
Jayakumar, T., Hsieh, C. Y., Lee, J. J., and Sheu, J. R. (2013). Experimental and clinical pharmacology of Andrographis paniculata and its major bioactive phytoconstituent andrographolide. Evid. Based Complement Alternat. Med., 2013, 846740. doi:10.1155/2013/846740
Jiang, Y., Zhou, Z., Meng, Q.-T., Sun, Q., Su, W., Lei, S., et al. (2015). Ginsenoside Rb1 treatment attenuates pulmonary inflammatory cytokine release and tissue injury following intestinal ischemia reperfusion injury in mice. Ox. Med. Cel. Longev., 2015, 1. doi:10.1155/2015/843721
Ka, S., Lin, J., Lin, T., Liu, F. C., Chao, L. K., Ho, C. L., et al. (2015). Citral alleviates an accelerated and severe lupus nephritis model by inhibiting the activation signal of NLRP3 inflammasome and enhancing Nrf2 activation. Arthritis Res. Ther. 17, 331. doi:10.1186/s13075-015-0844-6
Kang, O. H., Choi, J. G., Lee, J. H., and Kwon, D. Y. (2010). Luteolin isolated from the flowers of Lonicera japonica suppresses inflammatory mediator release by blocking NF-kappaB and MAPKs activation pathways in HMC-1 cells. Molecules 15, 385. doi:10.3390/molecules15010385
Kao, T. K., Ou, Y. C., Lin, S. Y., Pan, H. C., Song, P. J., Lai, C. Y., et al. (2011). Luteolin inhibits cytokine expression in endotoxin/cytokine-stimulated microglia. J. Nutr. Biochem. 22, 612. doi:10.1016/j.jnutbio.2010.01.011
Karres, I., Kremer, J. P., Dietl, I., Steckholzer, U., Jochum, M., and Ertell, W.(2020). Chloroquine inhibits pro-inflammatory cytokine release into human whole blood. Am. J. Physiol. 274, R1058. doi:10.1152/ajpregu.1998.274.4.R1058
Kato, K., Yamashita, S., Kitanaka, S., and Toyoshima, S. (2001). [Effect of gallic acid derivatives on secretion of Th1 cytokines and Th2 cytokines from anti CD3-stimulated spleen cells]. Yakugaku Zasshi 121, 451. doi:10.1248/yakushi.121.451
Keiss, H. P., Dirsch, V. M., Hartung, T., Haffner, T., Trueman, L., Auger, J., et al. (2003). Garlic (Allium sativum L.) modulates cytokine expression in lipopolysaccharide-activated human blood thereby inhibiting NF-kappaB activity. J. Nutr. 133, 2171. doi:10.1093/jn/133.7.2171
Kempuraj, D., Madhappan, B., Christodoulou, S., Boucher, W., Cao, J., Papadopoulou, N., et al. (2005). Flavonols inhibit pro-inflammatory mediator release, intracellular calcium ion levels and protein kinase C theta phosphorylation in human mast cells. Br. J. Pharmacol. 145, 934. doi:10.1038/sj.bjp.0706246
Khalil, A. A., Rahman, U. u., Khan, M. R., Sahar, A., and Mehmood, T. (2017). Essential oil eugenol: sources, extraction techniques and nutraceutical perspectives. RSC Adv. 7, 32669. doi:10.1039/C7RA04803C
Kim, G. D., Lee, S. E., Kim, T. H., Jin, Y. H., Park, Y. S., and Park, C. S. (2012). Melatonin suppresses acrolein-induced IL-8 production in human pulmonary fibroblasts. J. Pineal Res. 52, 356. doi:10.1111/j.1600-079X.2011.00950.x
Kim, H. J., Lee, W., and Yun, J. M. (2014). Luteolin inhibits hyperglycemia-induced pro-inflammatory cytokine production and its epigenetic mechanism in human monocytes. Phytother Res. 28, 1383. doi:10.1002/ptr.5141
Kim, S. H., Jun, C. D., Suk, K., Choi, B. J., Lim, H., Park, S., et al. (2006). Gallic acid inhibits histamine release and pro-inflammatory cytokine production in mast cells. Toxicol. Sci. 91, 123. doi:10.1093/toxsci/kfj063
Kim, S. H., and Lee, Y. C. (2009). Piperine inhibits eosinophil infiltration and airway hyperresponsiveness by suppressing T cell activity and Th2 cytokine production in the ovalbumin-induced asthma model. J. Pharm. Pharmacol. 61, 353. doi:doi:10.1211/jpp/61.03.0010
Kim, Y. J., and Park, W. (2016). Anti-Inflammatory effect of quercetin on RAW 264.7 mouse macrophages induced with polyinosinic-polycytidylic acid. Molecules 21, 450. doi:10.3390/molecules21040450
La Rosée, F., Bremer, H. C., Gehrke, I., Kher, A., Hochhaus, A., Brindt, S., et al. (2020). The Janus kinase 1/2 inhibitor ruxolitinib in COVID-19 with severe systemic hyperinflammation. Leukemia 34, 1805. doi:10.1038/s41375-020-0891-0
Laing, A. G., Lorenc, A., Del Molino Del Barrio, I., Das, A., Fish, M., Hayday, A. C., et al. (2020). A dynamic COVID-19 immune signature includes associations with poor prognosis. Nat. Med. 26, 1623. doi:10.1038/s41591-020-1038-6
Lakhan, S. E., and Kirchgessner, A. (2011). Anti-inflammatory effects of nicotine in obesity and ulcerative colitis. J. Transl. Med. 9, 129. doi:10.1186/1479-5876-9-129
Lang, A., Lahav, M., Sakhnini, E., Barshack, I., Fidder, H. H., Avidan, B., et al. (2004). Allicin inhibits spontaneous and TNF-alpha induced secretion of pro-inflammatory cytokines and chemokines from intestinal epithelial cells. Clin. Nutr. 23, 1199. doi:10.1016/j.clnu.2004.03.011
Lee, A. Y., Lee, S., Kim, H. Y., Lee, S., and Cho, E. J. (2016). Anti-inflammatory effects of luteolin and luteoloside from Taraxacum coreanum in RAW264.7 macrophage cells. Appl. Biol. Chem. 59, 747. doi:10.1007/s13765-016-0220-5
Lee, J. S., and Shin, E. C. (2020). The type I interferon response in COVID-19: implications for treatment. Nat. Rev. Immunol. 20, 585. doi:10.1038/s41577-020-00429-3
Lee, K. C. H., Sewa, D. W., and Phua, G. C. (2020). Potential role of statins in COVID-19. Int. J. Infect. Dis. 96, 615. doi:10.1016/j.ijid.2020.05.115
Lee, W., Lee, S. Y., Son, Y. J., and Yun, J. M. (2015). Gallic acid decreases inflammatory cytokine secretion through histone acetyltransferase/histone deacetylase regulation in high glucose-induced human monocytes. J. Med. Food 18, 793. doi:10.1089/jmf.2014.3342 Epub 2015 Mar 25
Li, D., Chen, Y., Liu, H., Jia, Y., Li, F., Wang, W., et al. (2020a). Immune dysfunction leads to mortality and organ injury in patients with COVID-19 in China: insights from ERS-COVID-19 study. Sig Transduct Target Ther. 5, 62. doi:10.1038/s41392-020-0163-5
Li, H., Liu, L., Zhang, D., Xu, J., Dai, H., Tang, N., et al. (2020b). SARS-CoV-2 and viral sepsis: observations and hypothesis. Lancet 395. doi:10.1016/S0140-6736(20)30920-X
Li, H., Liu, S.-M., Yu, X.-H., Tang, S.-L., and Tang, C.-K. (2020c). Coronavirus disease 2019 (COVID-19): current status and future perspectives. Int. J. Antimicrob. Agents 55, 105951. doi:10.1016/j.ijantimicag.2020.105951
Li, X. H., McGrath, K. C., Tran, V. H., Li, Y. M., Duke, C. C., Heather, A. K., et al. (2013). Attenuation of pro-inflammatory responses by s-[6]-gingerol via inhibition of ROS/NF-kappa B/COX2 activation in HuH7 cells. Evid Based Complement Alternat Med. 2013, 146142. doi:10.1155/2013/146142
Li, Y., Yao, J., Han, C., Yang, J., Chaudhry, M. T., Wang, S., et al. (2016). Quercetin, inflammation and immunity. Nutrients 8 (3), 167. doi:10.3390/nu8030167
Li, Y., He, S., Tang, J., Ding, N., Chu, X., Cheng, L., et al. (2017). Andrographolide inhibits inflammatory cytokines secretion in LPS-stimulated RAW264.7 cells through suppression of NF-κB/MAPK signaling pathway. Evid. base Compl. Alternative Med. 2017, 1. doi:10.1155/2017/8248142
Liang, Y. D., Bai, W. J., Li, C. G., Xu, L. H., Wei, H. X., Pan, H., et al. (2016). Piperine suppresses pyroptosis and interleukin-1β release upon ATP triggering and bacterial infection. Front. Pharmacol. 7, 390. doi:10.3389/fphar.2016.00390
Liang, Y., Wang, M-L., Chien, C-S., Yarmishyn, A. A., Yang, Y-P., Lai, W. Y., et al. (2020). Highlight of immune pathogenic response and hematopathologic effect in SARS-CoV, MERS-CoV, and SARS-Cov-2 infection. Front. Immunol. 11, 1022. doi:10.3389/fimmu.2020.01022
Lilly (2020). Lilly begins clinical trial testing of therapies for COVID-19. https://investor.lilly.com/news-releases/news-release-details/lilly-begins-clinical-testing-therapies-covid-19 (Accessed July 09, 2020).
Lin, Y., Shi, R., Wang, X., and Shen, H. M. (2008). Luteolin, a flavonoid with potential for cancer prevention and therapy. Curr. Cancer Drug Targets 8, 634. doi:10.2174/156800908786241050
Lingeswaran, M., Goyal, T., Ghosh, R., Suri, S., Mitra, P., Sharma, P., et al. (2020). Inflammation, immunity and immunogenetics in COVID-19: a narrative review. Indian J. Clin. Biochem. 35, 260. doi:10.1007/s12291-020-00897-3
Lippi, G., and Plebani, M. (2020). Procalcitonin in patients with severe coronavirus disease 2019 (COVID-19): a meta-analysis. Clin. Chim. Acta 505, 190. doi:10.1016/j.cca.2020.03.004
Liu, M., Wang, T., Zhou, Y., Zhao, Y., and Zhang, Y. (2020). Potential role of ACE2 in coronavirus disease 2019 (COVID-19) prevention and management. J. Transl. Int. Med. 8, 9. doi:10.2478/jtim-2020-0003
Liu, Z., and Ying, Y. (2020). The inhibitory effect of curcumin on virus-induced cytokine storm and its potential use in the associated severe pneumonia. Front. Cell Dev. Biol. 8, 479. doi:10.3389/fcell.2020.00479
Lodhi, S., Vadnere, G. P., Patil, K. D., and Patil, T. P. (2020). Protective effects of luteolin on injury induced inflammation through reduction of tissue uric acid and pro-inflammatory cytokines in rats. J Tradit Complement Med 10, 60. doi:10.1016/j.jtcme.2019.02.004
Long, H., Nie, L., Xiang, X., Li, H., Zhang, X., Fu, X., et al. (2020). D-dimer and prothrombin time are the significant indicators of severe COVID-19 and poor prognosis. BioMed Res. Int., 2020, 1. doi:10.1155/2020/6159720
Lu, J., Ma, Y., Wu, J., Huang, H., Wang, X., Chen, Z., et al. (2019). A review for the neuroprotective effects of andrographolide in the central nervous system. Biomed. Pharmacother. 117, 109078. doi:10.1016/j.biopha.2019.109078
Ma, H., Zeng, W., He, H., Zhao, D., Jiang, D., Zhao, P., et al. (2020). Serum IgA, IgM, and IgG responses in COVID-19. Cell. Mol. Immunol. 17, 773. doi:10.1038/s41423-020-0474-z
Madera-Salcedo, I. K., Cruz, S. L., and Gonzalez-Espinosa, C. (2011). Morphine decreases early peritoneal innate immunity responses in Swiss-Webster and C57BL6/J mice through the inhibition of mast cell TNF-α release. J. Neuroimmunol. 232, 101–107. doi:10.1016/j.jneuroim.2010.10.017
Mahmudpour, M., Roozbeh, J., Keshavarz, M., Farrokhi, S., and Nabipour, I. (2020). COVID-19 cytokine storm: the anger of inflammation. Cytokine 133, 155151. doi:10.1016/j.cyto.2020.155151
Malaguarnera, L. (2019). Influence of resveratrol on the immune response. Nutrients 11, 5. doi:10.3390/nu11050946
Marinella, M. A. (2020). Indomethacin and resveratrol as potential treatment adjuncts for SARS‐CoV‐2/COVID‐19. Int. J. Clin. Pract. 74, e13535. doi:10.1111/ijcp.13535
Martínez, G. J., Robertson, S., Barraclough, J., Xia, Q., Mallat, Z., Patel, S., et al. (2015). Colchicine acutely suppresses local cardiac production of inflammatory cytokines in patients with an acute coronary syndrome. J Am Heart Assoc 4, e002128. doi:10.1161/JAHA.115.002128
Mastaglio, S., Ruggeri, A., Risitano, A. M., Angelillo, P., Yancopoulou, D., Ciceri, F., et al. (2020). The first case of COVID-19 treated with the complement C3 inhibitor AMY-101. Clin. Immunol. 215, 108450. doi:10.1016/j.clim.2020.108450
Matthay, M. A., Zemans, R. L., Zimmerman, G. A., Yaseen, M., Arabi, J. R., Marcet, A., et al. (2020). Acute respiratory distress syndrome. Nat. Rev. Dis. Primers. 5, 1. doi:10.1038/s41572-019-0069-0
Maurya, V. K., Kumar, S., Prasad, A. K., Bhatt, M. L. B., and Saxena, S. K. (2020). Structure-based drug designing for potential antiviral activity of selected natural products from Ayurveda against SARS-CoV-2 spike glycoprotein and its cellular receptor. Virusdisease 31, 179. doi:10.1007/s13337-020-00598-8
McGonagle, D., O’Donnell, J. S., Sharif, K., Emery, P., and Bridgewood, C. (2020). Immune mechanisms of pulmonary intravascular coagulopathy in COVID-19 pneumonia. Lancet Rheumatol 2, e437. doi:10.1016/S2665-9913(20)30121-1
Mehrbod, P., Abdalla, M. A., Fotouhi, F., Heidarzadeh, M., Aro, A. O., Eloff, J. N., et al. (2018). Immunomodulatory properties of quercetin-3-O-α-L-rhamnopyranoside from Rapanea melanophloeos against influenza a virus. BMC Compl. Alternative Med. 18, 184. doi:10.1186/s12906-018-2246-1
Mejia, J. C., Basu, A., and Shapiro, R. (2014). Kidney transplantation - principles and practice. 7. 231–249. doi:10.1016/b978-1-4557-4096-3.00017-9
Melchjorsen, J., Sørensen, L. N., and Paludan, S. R. (2003). Expression and function of chemokines during viral infections: from molecular mechanisms to in vivo function. J. Leukoc. Biol. 74, 331. doi:10.1189/jlb.1102577
Meo, S. A., Klonoff, D. C., and Akram, J. (2020). Efficacy of chloroquine and hydroxychloroquine in the treatment of COVID-19. Eur. Rev. Med. Pharmacol. Sci. 24, 4539. doi:10.26355/eurrev_202004_21038
Min, K. J., Jang, J. H., and Kwon, T. K. (2012). Inhibitory effects of melatonin on the lipopolysaccharide-induced CC chemokine expression in BV2 murine microglial cells are mediated by suppression of Akt-induced NF-κB and STAT/GAS activity. J. Pineal Res. 52, 296, doi:10.1111/j.1600-079X.2011.00943.x
Moll, G., Drzeniek, N., Kamhieh-Milz, J., Geissler, S., and Volk, H. D. (2020). MSC therapies for COVID-19: importance of patient coagulopathy, thromboprophylaxis, cell product quality and mode of delivery for treatment safety and efficacy. Front. Immunol. 11, 1091. doi:10.3389/fimmu.2020.01091
Montealegre-Gómez, G., Garavito, E., Gómez-López, A., Rojas-Villarraga, A., and Parra-Medina, R. (2020). Colchicine: a potential therapeutic tool against COVID-19. Experience of 5 patients Reumatol. Clínica. doi:10.1016/j.reuma.2020.05.001
Nair, M. P., Mahajan, S., Reynolds, J. L., Aalinkeel, R., Nair, H., et al. (2006). The flavonoid quercetin inhibits pro-inflammatory cytokine (tumor necrosis factor alpha) gene expression in normal peripheral blood mononuclear cells via modulation of the NF-kappa beta system. Clin. Vaccine Immunol. 13, 319. doi:10.1128/CVI.13.3.319-328.2006
National Cancer Institute Cytokine storm https://www.cancer.gov/publications/dictionaries/cancer-terms/def/cytokine-storm (Accessed June 29, 2020).
National Center for Biotechnology Information (2020a). PubChem database. https://pubchem.ncbi.nlm.nih.gov/compound/Andrographolide (Accessed July 13, 2020)
National Center for Biotechnology Information (2020b). PubChem database. Allicin https://pubchem.ncbi.nlm.nih.gov/compound/Allicin (Accessed July 13, 2020).
National Center for Biotechnology Information (2020c). PubChem database. Colchicine https://pubchem.ncbi.nlm.nih.gov/compound/Colchicine (Accessed July 11, 2020)
National Center for Biotechnology Information (2020d). PubChem database. Curcumin https://pubchem.ncbi.nlm.nih.gov/compound/Curcumin (Accessed July 11, 2020).
National Center for Biotechnology Information (2020e). PubChem database. Eugenol https://pubchem.ncbi.nlm.nih.gov/compound/Eugenol (Accessed July 14, 2020)
National Center for Biotechnology Information (2020f). PubChem Database. Gingerol https://pubchem.ncbi.nlm.nih.gov/compound/Gingerol (Accessed July 13, 2020)
National Center for Biotechnology Information (2020g). PubChem database. Luteolin https://pubchem.ncbi.nlm.nih.gov/compound/Luteolin (Accessed July 13, 2020).
National Center for Biotechnology Information (2020h). PubChem database. Morphine https://pubchem.ncbi.nlm.nih.gov/compound/Morphine (Accessed July 11, 2020).
National Center for Biotechnology Information (2020i). PubChem database. Codeine https://pubchem.ncbi.nlm.nih.gov/compound/Codeine (Accessed July 11, 2020)
National Center for Biotechnology Information (2020j). PubChem database. Nicotine https://pubchem.ncbi.nlm.nih.gov/compound/Nicotine (Accessed July 11, 2020).
National Center for Biotechnology Information (2020k). PubChem database. Piperine https://pubchem.ncbi.nlm.nih.gov/compound/Piperine (Accessed July 11, 2020)
National Center for Biotechnology Information (2020l). PubChem database. Quercetin, CID=5280343 https://pubchem.ncbi.nlm.nih.gov/compound/Quercetin (Accessed July 11, 2020).
National Center for Biotechnology Information (2020m). PubChem database. Resveratrol https://pubchem.ncbi.nlm.nih.gov/compound/Resveratrol (Accessed July 13, 2020).
National Institute of Diabetes and Digestive and Kidney Diseases(2020). Clinical and research information on drug-induced liver injury. Bethesda, MD: National Institute of Diabetes and digestive and kidney diseases; eugenol (clove oil).
National Institutes of Health (2020a). COVID-19 Treatment Guidelines. Antiviral drugs that are approved or under evaluation for the treatment of COVID-19 https://www.covid19treatmentguidelines.nih.gov/antiviral-therapy/#:∼:text=Antiviral%20Drugs%20That%20Are%20Approved,the%20Treatment%20of%20COVID%2D19&text=Remdesivir%20is%20the%20only%20Food (Accessed November 19, 2020).
National Institutes of Health (2020b). Clinical trial to evaluate methylprednisolone pulses and tacrolimus in patients with COVID-19 lung injuryTACROVID) https://clinicaltrials.gov/ct2/show/NCT04341038. (Accessed July 09, 2020).
National Institutes of Health (2020c). Study of efficacy and safety of canakinumab treatment for CRS in participants with COVID-19-induced pneumonia https://clinicaltrials.gov/ct2/show/NCT04362813. (Accessed July 09, 2020).
National Institutes of Health (2020d). Nebulized heparin for the treatment of COVID-19 induced lung injury https://clinicaltrials.gov/ct2/show/NCT04397510. (Accessed July 09, 2020).
National Institutes of Health (2020e). Study of standard care plus intravenous immunoglobulin (IVIG) compared to standard care alone in the treatment of COVID-19 infection https://clinicaltrials.gov/ct2/show/NCT04411667 (Accessed July 09, 2020).
National Institutes of Health (2020f). Convalescent plasma compared to anti-COVID-19 human immunoglobulin and standard treatment (TE) in hospitalized patients https://clinicaltrials.gov/ct2/show/NCT04395170. (Accessed July 09, 2020).
National Institutes of Health (2020g). Treatment of SARS caused by COVID-19 with ruxolitinib. https://clinicaltrials.gov/ct2/show/NCT04334044 (Accessed July 09, 2020).
National Institutes of Health (2020h). Ruxolitinib for treatment of COVID-19-induced lung injury ARDS (RuXoCoil) https://clinicaltrials.gov/ct2/show/NCT04359290. (Accessed July 09, 2020).
National Institutes of Health (2020i). PubChem. https://pubchem.ncbi.nlm.nih.gov/compound/Baricitinib. (Accessed July 09, 2020).
National Institutes of Health (2020j). Baricitinib therapy in COVID-19. https://clinicaltrials.gov/ct2/show/NCT04358614. (Accessed July 09, 2020)
National Institutes of Health (2020k). Safety and efficacy of baricitinib for COVID-19. https://clinicaltrials.gov/ct2/show/NCT04340232. (Accessed July 09, 2020).
National Institutes of Health (2020l). Prophylactic corticosteroid to prevent COVID-19 cytokine storm. https://clinicaltrials.gov/ct2/show/NCT04355247. (Accessed July 09, 2020).
National Institutes of Health (2020m). Efficacy and safety of corticosteroids in COVID-19. https://clinicaltrials.gov/ct2/show/NCT04273321. (Accessed July 09, 2020).
National Institutes of Health (2020n). Statin therapy and COVID-19 infection. https://www.clinicaltrials.gov/ct2/show/NCT04407273. (Accessed July 09, 2020).
National Institutes of Health(2020o). Recombinant human angiotensin-converting enzyme 2 (rhACE2) as a treatment for patients with COVID-19 (APN01-COVID-19). https://clinicaltrials.gov/ct2/show/NCT04287686. (Accessed July 09, 2020).
National Institutes of Health(2020p). Recombinant human angiotensin-converting enzyme 2 (rhACE2) as a treatment for patients with COVID-19 (APN01-COVID-19). https://clinicaltrials.gov/ct2/show/NCT04335136. (Accessed July 09, 2020).
Nature (2020). Coronavirus outbreak: dexamethasone is first drug shown to save lives. https://www.nature.com/articles/d41586-020-01824-5.
Nawaz, M. A., Huang, Y., Bie, Z., Ahmed, W., Reiter, R. J., Niu, M., et al. (2016). Melatonin: current status and future perspectives in plant science. Front. Plant Sci. 6, 1230. doi:10.3389/fpls.2015.01230
Nie, X., Chen, S., Wang, K., Peng, Y., Wang, Y. T., Wang, Y, et al. (2017). Attenuation of innate immunity by andrographolide derivatives through NF-κB signaling pathway. Sci. Rep. 7, 4738. doi:10.1038/s41598-017-04673-x
Nile, S. H., Nile, A., Qiu, J., Li, L., Jia, X., Kai, G., et al. (2020). COVID-19: pathogenesis, cytokine storm and therapeutic potential of interferons. Cytokine Growth Factor Rev. 53, 66. doi:10.1016/j.cytogfr.2020.05.002
Noris, M., Benigni, A., and Remuzzi, G. (2020). The case of complement activation in COVID-19 multiorgan impact. Kidney Int. 98, 314. doi:10.1016/j.kint.2020.05.013
Orzechowski, A., Rostagno, A. A., Pucci, S., and Chiocchia, G. (2014). Cytokines and disease. Mediat. Inflamm., 2014, 209041. doi:10.1155/2014/209041
Paik, S., Choe, J. H., Choi, G. E., Kim, J. E., Kim, J. M., Song, J. K., et al. (2019). Rg6, a rare ginsenoside, inhibits systemic inflammation through the induction of interleukin-10 and microRNA-146a. Sci. Rep. 9, 4342. doi:10.1038/s41598-019-40690-8
Papadopoulos, C., Patoulias, D., Teperikidis, E., Mouselimis, D., Tsarouchas, A., Doumas, M., et al. (2020). Colchicine as a potential therapeutic agent against cardiovascular complications of COVID-19: an exploratory review. SN Compr. Clin. Med., 2, 1419. doi:10.1007/s42399-020-00421-x
Piao, W. H., Campagnolo, D., Dayao, C., Lukas, R. J., and Wu, J. (2009). Nicotine and inflammatory neurological disorders. Acta Pharmacol. Sin. 30, 715. doi:10.1038/aps.2009.67
Poland, G. A., Ovsyannikova, I. G., and Kennedy, R. B. (2020). SARS-CoV-2 immunity: review and applications to phase 3 vaccine candidates. Lancet 396, 10262. doi:10.1016/S0140-6736(20)32137-1
Porto Mde, P., da Silva, G. N., Luperini, B. C., Bachiega, T. F., de Castro Marcondes, J. P., Sfrocin, J. M., et al. (2014). Citral and eugenol modulate DNA damage and pro-inflammatory mediator genes in murine peritoneal macrophages. Mol. Biol. Rep. 41, 7043. doi:10.1007/s11033-014-3657-9
Pradeep, C. R., and Kuttan, G. (2004). Piperine is a potent inhibitor of nuclear factor-kappaB (NF-kappaB), c-Fos, CREB, ATF-2 and pro-inflammatory cytokine gene expression in B16F-10 melanoma cells. Int. Immunopharm. 4, 1795. doi:10.1016/j.intimp.2004.08.005
Pramod, K., Ansari, S. H., and Ali, J. (2010). Eugenol: a natural compound with versatile pharmacological actions. Nat. Prod. Commun. 5, 1999. doi:10.1177/1934578X1000501236
Prompetchara, E., Ketloy, C., and Palaga, T. (2020). Immune responses in COVID-9 and potential vaccines: lessons learnt from SARS and MERS epidemic. Asian Pac. J. Allerg. 38, 772. doi:10.12932/AP-200220-0772
Qin, C., Zhou, L., Hu, Z., Zhang, S., Yang, S., au, Y., et al. (2020). Dysregulation of immune response in patients with coronavirus 2019 (COVID-19) in Wuhan, China. Clin. Infect. Dis., 71, 762. doi:10.1093/cid/ciaa248
Ragab, D., Salah Eldin, H., Taeimah, M., and Khattab, R. (2020). The COVID-19 cytokine storm; what we know so far. Front. Immunol. 11, 1446. doi:10.3389/fimmu.2020.01446
Rahardjo, B., Widjajanto, E., Sujuti, H., and Keman, K. (2014). Curcumin decreased level of pro-inflammatory cytokines in monocyte cultures exposed to preeclamptic plasma by affecting the transcription factors NF-κB and PPAR-γ. Biomark. Genom. Med. 6, 105. doi:10.1016/j.bgm.2014.06.002
Rahmati, M., and Moosavi, M. A. (2020). Cytokine-targeted therapy in severely ill COVID-19 patients: options and cautions. Ejmo 4, 2. doi:10.14744/ejmo.2020.72142
Rajarshi, K., Chatterjee, A., and Ray, S. (2020). Combating COVID-19 with mesenchymal stem cell therapy. Biotechnol. Rep. 26, e00467. doi:10.1016/j.btre.2020.e00467
Ray, A., Gulati, K., Joshi, J., Guhathakurta, S., and Rai, S. (2016). Cytokines and their role in health and disease: a brief overview. Moji 4, 2. doi:10.15406/moji.2016.04.00121
Reiter, R. J., Sharma, R., Ma, Q., Dominquez-Rodriguez, A., and Marik, P. E. (2020). Melatonin inhibits COVID-19-induced cytokine storm by reversing aerobic glycolysis in immune cells: a mechanistic analysis. Med Drug Discov 6, 100044. doi:10.1016/j.medidd.2020.100044
Ribeiro, D., Freitas, M., Tomé, S. M., Silva, A. M., Laufer, S., Fernades, E., et al. (2010). Flavonoids inhibit COX-1 and COX-2 enzymes and cytokine/chemokine production in human whole blood. Inflammation 38, 858. doi:10.1007/s10753-014-9995-x
Ribeiro, S. A., Lopes, C., Amaral, R., and Amaral, A. (2020). The therapeutic potential of colchicine in the complications of COVID19. Could the immunometabolic properties of an old and cheap drug help? Metabol Open 7, 100045. doi:10.1016/j.metop.2020.100045
Rieder, S. A., Nagarkatti, P., and Nagarkatti, M. (2012). Multiple anti-inflammatory pathways triggered by resveratrol lead to amelioration of staphylococcal enterotoxin B-induced lung injury. Br. J. Pharmacol. 167, 1244. doi:10.1111/j.1476-5381.2012.02063.x
Risitano, A. M., Mastellos, D. C., Huber-Lang, M., Yancopoulou, D., Garlanda, C., Ciceri, F., et al. (2020). Complement as a target in COVID-19?. Nat. Rev. Immunol. 20, 343. doi:10.1038/s41577-020-0320-7
Rizzo, A., Bevilacqua, N., Guida, L., Annunziata, M., Romano Carratelli, C., and Paolillo, R. (2012). Effect of resveratrol and modulation of cytokine production on human periodontal ligament cells. Cytokine 60, 197. doi:10.1016/j.cyto.2012.06.004
Roshanravan, N., Seif, F., Ostadrahimi, A., Pouraghaei, M., and Ghaffari, S. (2020a). Targeting cytokine storm to manage patients with COVID-19: a mini-review. Arch. Med. Res. 51, 608. doi:10.1016/j.arcmed.2020.06.012
Roshanravan, N., Ghaffari, S., and Hedayati, M. (2020b). Angiotensin converting enzyme-2 as therapeutic target in COVID-19. Diabetes Metabolic Syndrome: Clin. Res. Rev. 14, 637. doi:10.1016/j.dsx.2020.05.022
Rudnicka, L., Glowacka, P., Goldust, M., Sikora, M., Sar-Pomian, M., Rokowska, A., et al. (2020). Cyclosporine therapy during the COVID-19 pandemic. J. Am. Acad. Dermatol. 83, e151. doi:10.1016/j.jaad.2020.04.153
Russell, B., Moss, C., Rigg, A., and Hemelrijck, M. V. (2020). COVID-19 and treatment with NSAIDs and corticosteroids: should we be limiting their use in the clinical setting? ECanc. Med. Sci. 14, 1023. doi:10.3332/ecancer.2020.1023
Sacerdote, P., Manfredi, B., Mantegazza, P., and Panerai, A. E. (1997).Antinociceptive and immunosuppressive effects of opiate drugs: a structure-related activity study. Br. J. Pharmacol. 121, 834. doi:10.1038/sj.bjp.0701138
Sagrawat, H., and Khan, M. Y. (2007). Immunomodulatory plants: a phytopharmacological review. Phog. Rev. 1 (2), 248–260.
Saha, A., Sharma, A. R., Bhattacharya, M., Sharma, G., Lee, S. S., et al. (2020a). Probable molecular mechanism of remdesivir for the treatment of COVID-19: need to know more. Arch. Med. Res. 51, 585. doi:10.1016/j.arcmed.2020.05.001
Saha, A., Sharma, A. R., Bhattacharya, M., Sharma, G., Lee, S. S., et al. (2020b). Tocilizumab: a therapeutic option for the treatment of cytokine storm syndrome in COVID-19. Arch. Med. Res. 51, 595. doi:10.1016/j.arcmed.2020.05.009
Saha, P., Katarkar, A., Das, B., Bhattacharyya, A., and Chaudhuri, K. (2016). 6-Gingerol inhibits Vibrio cholerae-induced pro-inflammatory cytokines in intestinal epithelial cells via modulation of NF-κB. Pharm. Biol. 54 (9), 1606–1615. doi:10.3109/13880209.2015.1110598
Sahoo, B. M., and Banik, B. K. (2018). Medicinal plants: source for immunosuppressive agents. Immunol. Curr. Res. 2, 106.
Salehi, B., Sharopov, F., Fokou, P. V. T., Kobylinska, A., Jonge, L., Tadio, K., et al. (2019). Melatonin in medicinal and food plants: occurrence, bioavailability, and health potential for humans. Cells 8, 7. doi:10.3390/cells8070681
Schett, G., Sticherling, M., and Neurath, M. F. (2020a). COVID-19: risk for cytokine targeting in chronic inflammatory diseases? Nat. Rev. Immunol. 20, 271. doi:10.1038/s41577-020-0312-7
Schett, G., Manger, B., and SimonCaporali, D. R. (2020b). COVID-19 revisiting inflammatory pathways of arthritis. Nat. Rev. Rheumatol., 16, 465. doi:10.1038/s41584-020-0451-z
Seif, F., Aazami, H., Khoshmirsafa, M., Kamali, M., Mohsenzadegan, M., Pouner, M., et al. (2020). JAK inhibition as a new treatment strategy for patients with COVID-19. Int. Arch. Allergy Immunol. 181, 467. doi:10.1159/000508247
Sethi, A., and Bach, H. (2020). Evaluation of current therapies for COVID-19 treatment. Microorganisms 8, 1097. doi:10.3390/microorganisms8081097
Shah, A. (2020). Novel coronavirus-induced NLRP3 inflammasome activation: a potential drug target in the treatment of COVID-19. Front. Immunol. 11, 1021. doi:10.3389/fimmu.2020.01021
Shah, V. K., Firmal, P., Alam, A., Ganguly, D., and Chattopadhyay, S. (2020). Overview of immune response during SARS-CoV-2 infection: lessons from the past. Front. Immunol. 11, 1949. doi:10.3389/fimmu.2020.01949
Shang, J., Wan, Y., Luo, C., Ye, G., Geng, Q., Li, F., et al. (2020). Cell entry mechanisms of SARS-CoV-2. Proc. Natl. Acad. Sci. U. S. A. 117, 11727. doi:10.1073/pnas.2003138117
Sharifi-Rad, J., Silva, N. C. C., Jantwal, A., Bhatt, I. D., Sharopov, F., Cho, W. C., et al. (2019). Therapeutic potential of allicin-rich garlic preparations: emphasis on clinical evidence toward upcoming drugs formulation. Appl. Sci. 9, 5555. doi:10.3390/app9245555
Sharifi-Rad, M., Varoni, E. M., Salehi, B., Sharifi-Rad, J., Matthews, K. R., Mnayer, D., et al. (2017). Plants of the genus Zingiber as a source of bioactive phytochemicals: from tradition to pharmacy. Molecules 22 (12), 2145. doi:10.3390/molecules22122145
Sheen, C. H., Schleimer, R. P., and Kulka, M. (2007). Codeine induces human mast cell chemokine and cytokine production: involvement of G-protein activation. Allergy 62, 532. doi:10.1111/j.1398-9995.2007.01345.x
Sheng, Y., Wu, T., Dai, Y., Ji, K., Zhong, Y., Xue, Y., et al. (2020). The effect of 6-gingerol on inflammatory response and Th17/Treg balance in DSS-induced ulcerative colitis mice. Ann. Transl. Med. 8, 442. doi:10.21037/atm.2020.03.141
Shereen, M. A., Khan, S., Kazmi, A., Bashir, N., and Siddique, R. (2020). COVID-19 infection: origin, transmission, and characteristics of human coronaviruses. J. Adv. Res. 24, 91. doi:10.1016/j.jare.2020.03.005
Shi, Y., Wang, Y., Shao, C., Huang, J., Gan, J., Huang, X., et al. (2020). COVID-19 infection: the perspectives on immune responses. Cell Death Differ. 27, 1451. doi:10.1038/s41418-020-0530-3
Shimabukuro-Vornhagen, A., Gödel, P., Subklewe, M., Stemmler, H. J., Schlößer, H. A., Schlaak, M., et al. (2018). Cytokine release syndrome. J. Immunotherapy Cancer 6, 58. doi:10.1186/s40425-018-0343-9
Shimizu, K., Funamoto, M., Sunagawa, Y., Shimizu, S., Katanasaka, Y., Wada, X., et al. (2019). Anti-inflammatory action of curcumin and its use in the treatment of lifestyle-related diseases. Eur. Cardiol. 14, 117. doi:10.15420/ecr.2019.17.2
Sládková, T., and Kostolanský, F. (2006). The role of cytokines in the immune response to influenza A virus infection. Acta Virol. 50, 3.
Song, P., Li, W., Xie, J., Hou, Y., and You, C. (2020). Cytokine storm induced by SARS-CoV-2. Clin. Chim. Acta 509, 280. doi:10.1016/j.cca.2020.06.017
Sordillo, P. P., and Helson, L. (2015). Curcumin suppression of cytokine release and cytokine storm. A potential therapy for patients with Ebola and other severe viral infections. In Vivo 29, 1.
SoRelle, J. A., Otoh, T., Peng, H., Kanak, M. A., Sugimoto, K., Mastumoto, S., et al. (2013). Withaferin A inhibits pro-inflammatory cytokine-induced damage to islets in culture and following transplantation diabetologia COVID-19 (81). Immunopath. Cytokine Storm 56, 814. doi:10.1007/s00125-012-2813-9
Soy, M., Keser, G., Atagündüz, P., Tabak, F., and Atagündüz, I. (2020). Cytokine storm in COVID-19: pathogenesis and overview of anti-inflammatory agents used in treatment. Clin. Rheumatol. 39, 2085. doi:10.1007/s10067-020-051590-5
Spagnolo, P., Balestro, E., Aliberti, S., Cocconcelli, E., Biondini, D., et al. (2020). Pulmonary fibrosis secondary to COVID-19: a call to arms? Lancet Respir. Med. 8, 750–752. doi:10.1016/S2213-2600(20)30222-8
Sun, Y., Dong, Y., Wang, L., Xie, H., Li, B., Chang, C., et al. (2020). Characteristics and prognostic factors of disease severity in patients with COVID-19: the Beijing experience. J. Autoimmun. 112, 102473. doi:10.1016/j.jaut.2020.102473
Tabata, S., Imai, K., Kawano, S., Ikeda, M., Kodama, T., Mayoshi, K., et al. (2020). Clinical characteristics of COVID-19 in 104 people with SARS-CoV-2 infection on the Diamond Princess cruise ship: a retrospective analysis. Lancet Infect. Dis. 3099, 20. doi:10.1016/S1473-3099(20)30482-5
Tang, D., Comish, P., and Kang, R. (2020a). The hallmarks of COVID-19 disease. PLoS Pathog. 16, e1008536. doi:10.1371/journal.ppat.1008536
Tang, N., Bai, H., Chen, X., Gong, J., Li, D., and Sun, Z. (2020b). Anticoagulant treatment is associated with decreased mortality in severe coronavirus disease 2019 patients with coagulopathy. J. Thromb. Haemostasis 18, 1094. doi:10.1111/jth.14817
Tang, J., Diao, P., Shu, X., Li, L., and Xiong, L. (2019). Quercetin and quercitrin attenuates the inflammatory response and oxidative stress in LPS-induced RAW264.7 cells: in vitro assessment and a theoretical model. BioMed Res. Int. 2019, 7039802. doi:10.1155/2019/7039802
Tarocco, A., Caroccia, N., MorcianoWieckowski, G. M. R., Ancora, G., Garoni, G., Pinton, P., et al. (2019). Melatonin as a master regulator of cell death and inflammation: molecular mechanisms and clinical implications for newborn care. Cell Death Dis. 10, 317. doi:10.1038/s41419-019-1556-7
Tay, M. Z., Poh, C. M., Rénia, L., and MacAry, P. A. (2020). The trinity of COVID-19: immunity, inflammation and intervention. Nat. Rev. Immunol. 20, 363. doi:10.1038/s41577-020-0311-8
Theoharides, T. C. (2020). COVID-19, pulmonary mast cells, cytokine storms, and beneficial actions of luteolin. Biofactors 46, 306. doi:10.1002/biof.1633
Tisoncik, J. R., Korth, M. J., Simmons, C. P., Farrar, J., Martin, T. R., Katze, M. J., et al. (2012). Into the eye of the cytokine storm. Microbiol. Mol. Biol. Rev. 76, 16. doi:10.1128/MMBR.05015-11
Tobaiqy, M., Qashqary, M., Al-Dahery, S., Mujallad, A., Hershan, A. A., Helmi, N., et al. (2020). Therapeutic management of patients with COVID-19: a systematic review. Infect. Prev. Prac. 2, 100061. doi:10.1016/j.infpip.2020.100061
Tripathi, S., Maier, K. G., Bruch, D., and Kittur, D. S. (2007). Effect of 6-gingerol on pro-inflammatory cytokine production and costimulatory molecule expression in murine peritoneal macrophages. J. Surg. Res. 138, 209. doi:10.1016/j.jss.2006.07.051
Tufan, A., Güler, A. A., and Matucci-Cerinic, M. (2020). COVID-19, immune system response, hyperinflammation and repurposing antirheumatic drugs. Turk. J. Med. Sci. 50, 620–632. doi:10.3906/sag-2004-168
U.S. Food and Drug Administration (2020). FDA cautions against the use of hydroxychloroquine or chloroquine for COVID-19 outside of the hospital setting or a clinical trial due to risk of heart rhythm problems. https://www.fda.gov/drugs/drug-safety-and-availability/fda-cautions-against-use-hydroxychloroquine-or-chloroquine-covid-19-outside-hospital-setting-or (Accessed July 09, 2020).
Vabret, N., Britton, G. J., Gruber, C., Hegde, S., Kim, J., Pia, L., et al. (2020). Immunology of COVID-19: current state of the science. Cell Press Immunity 52, 2, doi:10.1016/j.immuni.2020.05.002
Valério, D. A., Georgetti, S. R., Magro, D. A., Casagrande, R., Cunha, T. M., Verri, W. A., et al. (2009). Quercetin reduces inflammatory pain: inhibition of oxidative stress and cytokine production. J Nat. Prod. 72, 1975. doi:np900259y/np900259y.PMID:19899776
Valizadeh, H., Abdolmohammadi-Vahid, S., Danshina, S., Ziya Gencer, M., Ammari, A., Sadeghi, A., et al. (2020). Nano-curcumin therapy, a promising method in modulating inflammatory cytokines in COVID-19 patients. Int. Immunopharm. 89, 107088. doi:10.1016/j.intimp.2020.107088
Varma, A., Padh, H., and Shrivastava, N. (2011). Andrographolide: a new plant-derived antineoplastic entity on horizon. Evid. Based Complement Alternat. Med. 2011, 815390. doi:10.1093/ecam/nep135
Varsha, K., Sharma, A., Kaur, A., Madan, J., Pandey, R. S., et al. (2017). Natural plant-derived anticancer drugs nanotherapeutics: a review on preclinical to clinical success. Nanostruct. Canc. Therapy 2017, 775. doi:10.1016/B978-0-323-46144-3.00028-3
Vitiello, A., Ferrara, F., Pelliccia, C., Granata, G., and La Porta, R. (2020). Cytokine storm and colchicine potential role fighting SARS-CoV-2 pneumonia. Ital. J Med. 14, 2. doi:10.4081/itjm.2020.1284
Wang, J., Barke, R. A., Charboneau, R., and Roy, S. (2005). Morphine impairs host innate immune response and increases susceptibility to Streptococcus pneumoniae lung infection. J. Immunol. 174, 426. doi:10.4049/jimmunol.174.1.426.PMID
Wang, L., Wang, Y., Ye, D., and Liu, Q. (2020a). Review of the 2019 novel coronavirus (SARS-CoV-2) based on current evidence. Int. J. Antimicrob. Agents 55, 105948. doi:10.1016/j.ijantimicag.2020.105948
Wang, Y., Liu, S., Liu, H., Li, W., Lin, F., Li, X., et al. (2020b). SARS-CoV-2 infection of the liver directly contributes to hepatic impairment in patients with COVID-19. J. Hepatol. 73, 807. doi:10.1016/j.jhep.2020.05.002
Wang, W., Wang, J., Dong, S. F., Liu, C. H., Italiani, P., Xu, J., et al. (2010). Immunomodulatory activity of andrographolide on macrophage activation and specific antibody response. Acta Pharmacol. Sin. 31, 191. doi:10.1038/aps.2009.205
Weng, Z., Patel, A. B., Vasiadi, M., Therianou, A., and Theoharides, T. C. (2014). Luteolin inhibits human keratinocyte activation and decreases NF-κB induction that is increased in psoriatic skin. PloS One 9, e90739. doi:10.1371/journal.pone.0090739
Wong, S. Y., Tan, M. G., Banks, W. A., Wong, W. S. F., and Lai, M. P. K. (2016). Andrographolide attenuates LPS-stimulated up-regulation of C-C and C-X-C motif chemokines in rodent cortex and primary astrocytes. J. Neuroinflammation 13, 34. doi:10.1186/s12974-016-0498-6
World Health Organization (2020a). Coronavirus disease 2019. https://www.who.int/emergencies/diseases/novel-coronavirus-2019/advice-for-public/myth-busters (Accessed June 27, 2020).
World Health Organization (2020b). Coronavirus disease (COVID-19) pandemic. https://www.who.int/emergencies/diseases/novel-coronavirus-2019 (Accessed November 20, 2020).
World Health Organization (2020c). Modes of transmission of virus causing COVID-19: implications for IPC precaution recommendations. https://www.who.int/news-room/commentaries/detail/modes-of-transmission-of-virus-causing-covid-19-implications-for-ipc-precaution-recommendations (Accessed June 30, 2020).
World Health Organization (2020d). Rolling updates on coronavirus disease (COVID-19). https://www.who.int/emergencies/diseases/novel-coronavirus-2019/events-as-they-happen (Accessed June 27, 2020).
Wujtewicz, M., Dylczyk-Sommer, A., Aszkiełowicz, A., Zdanowski, S., Piwowarczyk, S., Owczuk, R., et al. (2020). COVID-19 - what should anaethesiologists and intensivists know about it? Ait 52, 34. doi:10.5114/ait.2020.93756
Xia, T., and Wang, Y. (2020). Coronavirus disease 2019 and transplantation: the combination of lopinavir/ritonavir and hydroxychloroquine is responsible for excessive tacrolimus trough level and unfavorable outcome. Am. J. Transplant., 20, 2630. doi:10.1111/ajt.15992
Xie, Y., Cao, S., Dong, H., Li, Q., Chen, E., Fu, S., et al. (2020). Effect of regular intravenous immunoglobulin therapy on prognosis of severe pneumonia in patients with COVID-19. J. Infect. 81, 318. doi:10.1016/j.jinf.2020.03.044
Yadav, R., Jee, B., and Awasthi, S. K. (2015). Curcumin suppresses the production of pro-inflammatory cytokine interleukin-18 in lipopolysaccharide stimulated murine macrophage-like cells. Indian J. Clin. Biochem. 30, 109. doi:10.1007/s12291-014-0452-2
Yamamoto, Y., and Gaynor, R. B. (2001). Therapeutic potential of inhibition of the NF-kappaB pathway in the treatment of inflammation and cancer. J. Clin. Invest. 107, 135. doi:10.1172/JCI11914
Yang, J. Y., Zhang, J., and Zhou, G. (2019). Black pepper and its bioactive constituent piperine: promising therapeutic strategies for oral lichen planus. Inflammopharmacol 27, 5. doi:10.1007/s10787-018-0540-7
Yazdanpanah, F., Hamblin, M. R., and Rezaei, N. (2020). The immune system and COVID-19: friend or foe? Life Sci. 256, 117900. doi:10.1016/j.lfs.2020.117900
Ye, Q., Wang, B., and Mao, J. (2020). The pathogenesis and treatment of the `Cytokine Storm' in COVID-19. J. Infect. 80, 607. doi:10.1016/j.jinf.2020.03.037
Yogalakshmi, B., Viswanathan, P., and Anuradha, C. V. (2010). Investigation of antioxidant, anti-inflammatory and DNA-protective properties of eugenol in thioacetamide-induced liver injury in rats. Toxicology 268, 204. doi:10.1016/j.tox.2009.12.018
Zagotta, I., Dimova, E. Y., Debatin, K. M., Wabitsch, M., Kietzmann, T., and Fischer-Posovszky, P. (2015). Obesity and inflammation: reduced cytokine expression due to resveratrol in a human in vitro model of inflamed adipose tissue. Front. Pharmacol. 6, 79. doi:10.3389/fphar.2015.00079
Zahedipour, F., Hosseini, S. A., Sathyapalan, T., Majeed, M., Jamialahmadi, T., Banach, M., et al. (2020). Potential effects of curcumin in the treatment of COVID-19 infection. Phytother Res. 34, 2911. doi:10.1002/ptr.6738
Zamudio-Cuevas, Y., Andonegui-Elguera, M. A., Aparicio-Juárez, A., Aguillón-Solís, E., Martínez-Flores, K., Ibarra, C., et al. (2020). The enzymatic poly(gallic acid) reduces pro-inflammatory cytokines in vitro, a potential application in inflammatory diseases. Inflammation 44, 174–185. doi:10.1007/s10753-020-01319-5
Zeng, F., Huang, Y., Guo, Y., Yin, M., Chen, X., Deng, G., et al. (2020). Association of inflammatory markers with the severity of COVID-19: a meta-analysis. Int. J. Infect. Dis. 96, 467. doi:10.1016/j.ijid.2020.05.055
Zhai, W. J., Zhang, Z. B., Xu, N. N., Guo, Y. F., Qiu, C., Li, C. Y., et al. (2016). Piperine plays an anti-inflammatory role in Staphylococcus aureus endometritis by inhibiting activation of NF-κB and MAPK pathways in mice. Evid Based Complement Alternat Med. 2016, 8597208. doi:10.1155/2016/8597208
Zhang, F., Zhang, J.-G., Yang, W., Xu, P., and Xiao, Y.-L. (2018). 6-Gingerol attenuates LPS-induced neuroinflammation and cognitive impairment partially via suppressing astrocyte overactivation. Biomed. Pharmacother. 107, 1523. doi:10.1016/j.biopha.2018.08.136
Zhang, J. M., and An, J. (2007). Cytokines, inflammation, and pain. Int. Anesthesiol. Clin. 45, 27. doi:10.1097/AIA.0b013e318034194e
Zhang, L., Li, Y., Gu, Z., Wang, Y., Shi, M., Ji, Y., et al. (2015). Resveratrol inhibits enterovirus 71 replication and pro-inflammatory cytokine secretion in rhabdosarcoma cells through blocking IKKs/NF-κB signaling pathway. PloS One 10, e0116879. doi:10.1371/journal.pone.0116879
Zhang, L., Yan, X., Fan, Q., Liu, H., Liu, X., Liu, Z., et al. (2020a). D‐dimer levels on admission to predict in‐hospital mortality in patients with Covid‐19. J. Thromb. Haemostasis 18, 1324. doi:10.1111/jth.14859
Zhang, M., Pan, H., Xu, Y., Wang, X., Qiu, Z., Jiang, L., et al. (2017). Allicin decreases lipopolysaccharide-induced oxidative stress and inflammation in human umbilical vein endothelial cells through suppression of mitochondrial dysfunction and activation of Nrf2. Cell. Physiol. Biochem. 41, 2255. doi:10.1159/000475640
Zhang, R., Wang, X., Ni, L., Di, X., Ma, B., Niu, S., et al. (2020b). COVID-19: melatonin as a potential adjuvant treatment. Life Sci. 250, 117583. doi:10.1016/j.lfs.2020.11758
Zhao, M. (2020). Cytokine storm and immunomodulatory therapy in COVID-19: role of chloroquine and anti-IL-6 monoclonal antibodies. Int. J. Antimicrob. Agents 55, 105982. doi:10.1016/j.ijantimicag.2020.105982
Zhao, Q., Meng, M., Kumar, R., Wu, Y., Huang, J., Yang, L., et al. (2020). Lymphopenia is associated with severe coronavirus disease 2019 (COVID-19) infections: a systemic review and meta-analysis. Int. J. Infect. Dis. 96, 131. doi:10.1016/j.ijid.2020.04.086
Zheng, H., Zhang, M., Yang, C. X., Zhang, N., Wang, X. C., Yang, X. P., et al. (2020a). Elevated exhaustion levels and reduced functional diversity of T cells in peripheral blood may predict severe progression in COVID-19 patients. Cell. Mol. Immunol. 17, 541. doi:10.1038/s41423-020-0401-3
Zheng, M., Gao, Y., Wang, G., Song, G., Liu, S., Sun, D., et al. (2020b). Functional exhaustion of antiviral lymphocytes in COVID-19 patients. Cell. Mol. Immunol. 17, 533. doi:10.1038/s41423-020-0402-2
Zhong, J., Tang, J., Ye, C., and Dong, L. (2020). The immunology of COVID-19: is immune modulation an option for treatment?. Lancet Rheumatol 2, e428. doi:10.1016/S2665-9913(20)30120-X
Zhou, Y., Guo, S., He, Y., Zuo, Q., Liu, D., Xiao, M., et al. (2020). COVID-19 Is distinct from SARS-CoV-2-negative community-acquired pneumonia. Front. Cell. Infect. Microbiol. 10, 322. doi:10.3389/fcimb.2020.00322
Zhou, Z. X., Mou, S. F., Chen, X. Q., Gong, L. L., and Ge, W. S. (2018). Anti-inflammatory activity of resveratrol prevents inflammation by inhibiting NF‑κB in animal models of acute pharyngitis. Mol. Med. Rep. 17, 1269–1274. doi:10.3892/mmr.2017.7933
Keywords: COVID-19, cytokine storm, immunomodulatory agents, plant-derived immunosuppressants, adjuvant
Citation: Peter AE, Sandeep BV, Rao BG and Kalpana VL (2021) Calming the Storm: Natural Immunosuppressants as Adjuvants to Target the Cytokine Storm in COVID-19. Front. Pharmacol. 11:583777. doi: 10.3389/fphar.2020.583777
Received: 15 July 2020; Accepted: 09 December 2020;
Published: 27 January 2021.
Edited by:
Jon Wardle, Southern Cross University, AustraliaReviewed by:
Andréa Teixeira-Carvalho, René Rachou Institute, Oswaldo Cruz Foundation (Fiocruz), BrazilMohamed Ghonim, St. Jude Children’s Research Hospital, United States
Chiranjib Chakraborty, Adamas University, India
Copyright © 2021 Peter, Sandeep, Rao and Kalpana. This is an open-access article distributed under the terms of the Creative Commons Attribution License (CC BY). The use, distribution or reproduction in other forums is permitted, provided the original author(s) and the copyright owner(s) are credited and that the original publication in this journal is cited, in accordance with accepted academic practice. No use, distribution or reproduction is permitted which does not comply with these terms.
*Correspondence: Angela E. Peter, angelapeter.728@gmail.com