- 1 Institute of Molecular Systems Biology, Swiss Federal Institute of Technology, Zurich, Switzerland
- 2 Competence Center of Systems Physiology and Metabolic Disease, Swiss Federal Institute of Technology, Zurich, Switzerland
In the recent past, deorphanization studies have described intermediates of energy metabolism to activate G protein-coupled receptors and to thereby regulate metabolic functions. GPR81, GPR109A, and GPR109B, formerly known as the nicotinic acid receptor family, are encoded by clustered genes and share a high degree of sequence homology. Recently, hydroxy-carboxylic acids were identified as endogenous ligands of GPR81, GPR109A, and GPR109B, and therefore these receptors have been placed into a novel receptor family of hydroxy-carboxylic acid (HCA) receptors. The HCA1 receptor (GPR81) is activated by the glycolytic metabolite 2-hydroxy-propionic acid (lactate), the HCA2 receptor is activated by the ketone body 3-hydroxy-butyric acid, and the HCA3 receptor (GPR109B) is a receptor for the β-oxidation intermediate 3-hydroxy-octanoic acid. While HCA1 and HCA2 receptors are present in most mammalian species, the HCA3 receptor is exclusively found in humans and higher primates. HCA receptors are expressed in adipose tissue and mediate anti-lipolytic effects in adipocytes through Gi-type G protein-dependent inhibition of adenylyl cyclase. HCA2 and HCA3 inhibit lipolysis during conditions of increased β-oxidation such as prolonged fasting, whereas HCA1 mediates the anti-lipolytic effects of insulin in the fed state. As HCA2 is a receptor for the established anti-dyslipidemic drug nicotinic acid, HCA1 and HCA3 also represent promising drug targets and several synthetic ligands for HCA receptors have been developed. In this article, we will summarize the deorphanization and pharmacological characterization of HCA receptors. Moreover, we will discuss recent progress in elucidating the physiological and pathophysiological role to further evaluate the therapeutic potential of the HCA receptor family for the treatment of metabolic disease.
Introduction
G protein-coupled receptors (GPCRs) form one of the largest protein families and are encoded by about 800 genes in mammalian organisms (Lander et al., 2001; Venter et al., 2001; Pierce et al., 2002). More than half of these genes are thought to encode odorant GPCRs like olfactory receptors. While among the non-odorant receptors endogenous ligands of approximately 210 receptors have been identified, there are still more than 100 orphan GPCRs whose natural ligands and biological functions remain unknown (Wise et al., 2004). The typical endogenous ligands of GPCRs are hormones, mediators, and neurotransmitters with broad chemical diversity including glycoproteins, peptides, nucleotides, prostanoids, biogenic amines, amino acids, ions, and even photons of light (Foord et al., 2005). Activation of GPCRs and downstream signaling pathways not only regulate numerous physiological functions, but also mediate the pharmacological effects of many of the approved drugs (Drews, 2000). Thus, the deorphanization and pharmacological characterization of orphan GPCRs is of continued scientific and pharmaceutical interest. Studies on receptor deorphanization often employ “reverse pharmacology”-approaches in high throughput scales, in which compound libraries or tissue extracts are tested for their ability to activate orphan GPCRs (Wise et al., 2004).
During the last decade, deorphanization studies revealed that nutrients and their metabolites, that were previously regarded as basic substrates for energy metabolism, can activate GPCRs and thereby regulate metabolic functions (He et al., 2004; Covington et al., 2006; Ahmed et al., 2009c; Ichimura et al., 2009). While FFA1 (GPR40), FFA2 (GPR43), FFA3 (GPR41), GPR84, and GPR120 were described as receptors for various free fatty acids (Briscoe et al., 2003; Brown et al., 2003; Itoh et al., 2003; Hirasawa et al., 2005; Wang et al., 2006), SUCNR1 (GPR99) and SUCNR2 (GPR91) were identified as receptors for citric acid cycle intermediates (He et al., 2004). In 2003, GPR109A was identified as the receptor of the anti-dyslipidemic drug nicotinic acid or niacin (Tunaru et al., 2003), and shortly thereafter the ketone body 3-hydroxybutyrate was described as the endogenous agonist of GPR109A (Taggart et al., 2005). Most recently, GPR109B and GPR81, which are structurally and phylogenetically closely related to GPR109A, were described as receptors for hydroxy-carboxylic acids. Specifically, GPR81 and GPR109B have been identified as receptors for the glycolytic product 2-hydroxy-propionate (lactate; Cai et al., 2008) and the β-oxidation intermediate 3-hydroxy-octanoate (Ahmed et al., 2009a), respectively. Because all three receptors are activated by endogenous hydroxy-carboxylic acid ligands, GPR109A, GPR109B, and GPR81 have quite recently been classified as Hydroxy-Carboxylic Acid receptors (HCA) with the individual members being HCA1 (GPR81), HCA2 (GPR109A), and HCA3 (GPR109B; Offermanns et al., 2011) (Table 1). In addition, several studies have shed light on the biological functions of HCA receptors (Table 2). While HCA2 and HCA3 inhibit lipolysis during physiological and pathophysiological conditions of increased β-oxidation and ketogenesis (Taggart et al., 2005; Ahmed et al., 2009a), HCA1 was shown to mediate the anti-lipolytic effects of insulin during the postprandial phase (Ahmed et al., 2010). All three HCA receptors have in common that they regulate lipolysis in a negative feedback manner and thus function as metabolic sensors (Figures 1 and 2).
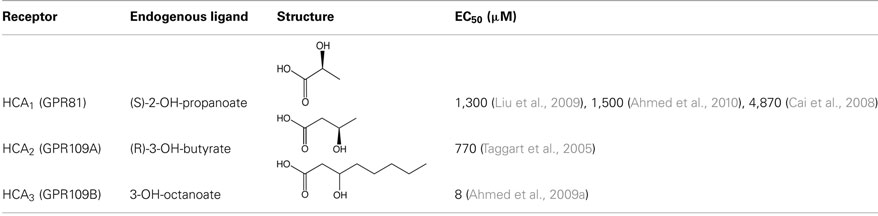
Table 1. Structures and EC50 values of endogenous ligands of HCA receptors. EC50 values were determined by ligand-induced GTPγS-binding assay.
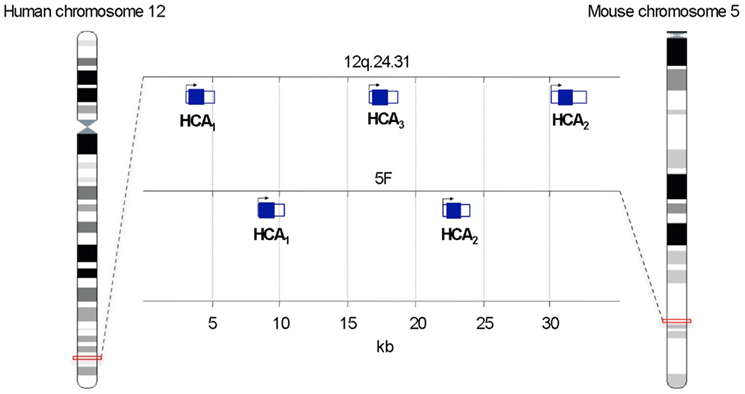
Figure 1. Schematic representation of the chromosomal location of genes encoding hydroxy-carboxylic acid (HCA) receptors. Protein-coding sequences are shown by filled rectangles. Adapted from Ensembl Genome Browser.
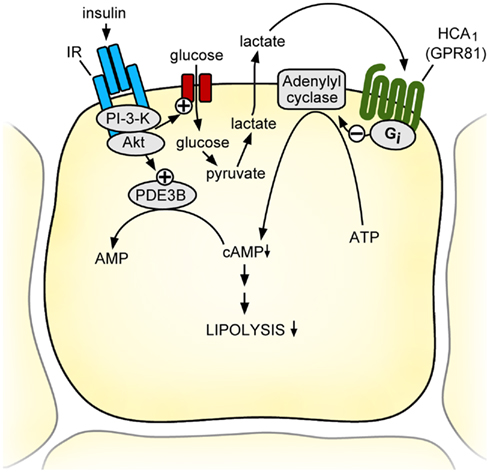
Figure 2. Model of the physiological function of the HCA1 receptor. During feeding, adipocytes can produce and release significant amounts of lactate, a process stimulated by insulin-induced glucose uptake. Lactate activates HCA1 receptors on adipocytes in an autocrine and paracrine fashion and decreases intracellular cAMP levels through Gi/Go-dependent inhibition of adenylyl cyclase. This leads together with the insulin-dependent activation of PDE3B to increased degradation and decreased formation of intracellular cAMP resulting in an inhibition of lipolysis. Thus, the lactate receptor HCA1 mediates the anti-lipolytic of insulin effects during the transition from the fed to the fasted state and thereby helps to preserve endogenous energy stores when food-derived nutrients are abundant. AC, adenylyl cyclase; IR, insulin receptor; PDE3B, phosphodiesterase 3B; PI3K, phosphatidylinositol-3-kinase; PKA, protein kinase A.
This review will summarize the deorphanization and pharmacological characterization of HCA receptors. Moreover, it will discuss the recent progress in elucidating the physiological and pathophysiological functions of HCA receptors and evaluate their therapeutic potential in the treatment of metabolic and immune disease.
Identification and Characterization of HCA Receptors
Cloning of Hydroxy-Carboxylic Acid Receptors
In 1993, the HCA3 receptor (GPR109B) was cloned from a human monocyte cDNA library and originally identified as a new orphan GPCR termed HM74 (Nomura et al., 1993). The HCA1 receptor was first found and identified as orphan receptor GPR81 by BLAST analysis using genomic sequence databases and known GPCR-encoding sequences. It was then cloned from a bacterial artificial chromosome (BAC) clone carrying the human genomic region 12q (Lee et al., 2001). It was noticed that HCA1 shares high sequence homology with the HCA3 receptor (GPR109B/HM74) and both genes were located in proximity on the same BAC clone. In 2001, the HCA2 receptor (GPR109A) was identified as a transcript induced in interferon-γ-treated murine macrophages and subsequently termed PUMA-G (protein up-regulated in macrophages by IFN-γ; Schaub et al., 2001). The sequence of the murine PUMA-G receptor showed high similarity when compared to human HM74/GPR109B.
Sequence Alignment and Phylogenetic Tree
HCA2 and HCA3 receptors are highly homologous with 96% sequence identity on the protein level. The HCA1 receptor shares approximately 55% amino acid sequence identity to both HCA2 and HCA3. The protein sequence of HCA2 and HCA3 differs in only 16 amino acids that are located within the first and second extracellular loops as well as the transmembrane regions 2 and 3. In addition, the C terminal tail of the HCA3 receptor has an extension of 24 amino acids. Importantly, all three receptors share an arginine residue within transmembrane helix 3 that is essential for binding of carboxylic acid ligands (Tunaru et al., 2005). Based on phylogenesis, the orphan receptor GPR31 and OXER1, the receptor for 5-oxo-6,8,11,14-eicosatetraenoic acid (5-oxo-ETE), are the most closely related receptors to the HCA receptor family sharing approximately 30% sequence identity with HCA receptors (Ahmed et al., 2009b; Offermanns et al., 2011). Interestingly, the aforementioned arginine residue of the third transmembrane helix is conserved within GPR31 and OXER1 (Ahmed et al., 2009b). While genes encoding for HCA1 and HCA2 can be found in most mammalian species including humans and rodents, HCA3 is only present in humans and higher primates like chimpanzee. It is likely that the HCA3 gene has evolved by a rather recent gene duplication of HCA2.
Several single nucleotide polymorphisms have been identified for HCA2 and HCA3 in humans (Zellner et al., 2005). To date, it is unclear whether these genetic variations affect the physiological or pharmacological properties of HCA2 and HCA3 receptors.
Deorphanization of Hydroxy-Carboxylic Acid Receptors
HCA1
In 2008, lactate was described as an agonist of the HCA1 receptor (Cai et al., 2008). Lactate stimulated binding of GTPγS to membranes of HCA1-expressing CHO-K1 cells with a half-maximal effective concentration (EC50) of 1.3 mM. In addition, lactate inhibited forskolin-induced stimulation of cyclic AMP (cAMP) production in HCA1-transfected cells with an EC50 of 2.2 mM. Moreover, lactate-induced an increase in intracellular calcium concentrations in cells coexpressing promiscious G protein Gqi5 and HCA1 with an EC50 of 4.7 mM. Lactate had no effect on HCA2- or HCA3-expressing cells. Potency and efficacy of the physiologically relevant stereoisomer (S)-lactate were more than twofold higher when compared to its enantiomer (R)-lactate. Among other carboxylic acids tested for activity toward HCA1, only propionate was able to activate the HCA1 receptor with an EC50 of 2.9 mM. However, given the low systemic plasma levels of propionate, it is quite unlikely that propionate is a physiologically relevant ligand of HCA1. Shortly thereafter, another study also described lactate as a specific agonist of the HCA1 receptor with EC50 values for lactate-induced binding of GTPγS and inhibition of cAMP formation of 4.87 and 4.16 mM, respectively. (Liu et al., 2009). While the ketone body and HCA2 agonist 3-hydroxybutyrate did not activate HCA1, other hydroxy-carboxylic acids like 2-hydroxy and 4-hydroxybutyrate showed some activity toward HCA1 but with reduced potencies (EC50 values of 8 and 15 mM, respectively). Interestingly, dichloroacetate and trifluoroacetate were identified as partial agonists of HCA1. These basic pharmacological properties of HCA1 were later confirmed in an independent study (Ahmed et al., 2010). The reported potencies of lactate to activate HCA1 are rather low when compared to classic GPCR ligands like hormones or neurotransmitters. However, while basal plasma concentrations of lactate are between 0.5 and 2 mM (and would be therefore too low to fully activate the HCA1 receptor), systemic lactate levels can increase up to 20 mM during intensive exercise. In addition, lactate concentrations within the adipose tissue are elevated upon glucose infusion (Ahmed et al., 2010). Thus, it is conceivable that HCA1 would function as a sensor for lactate during certain metabolic conditions.
HCA2
In 2003, HCA2 was identified as the receptor for the anti-dyslipidemic drug nicotinic acid (Tunaru et al., 2003). Nicotinic acid was shown to bind with high affinity to human HCA2 with dissociation constants (Kd) between 55 and 96 nM (Soga et al., 2003; Tunaru et al., 2003; Wise et al., 2003), and it activated mouse and human HCA2 in a Ca2+ reporter assay with EC50 values of about 3 and 1 μM, respectively (Tunaru et al., 2003). Because endogenous plasma levels of nicotinic acid are too low to activate HCA2, it is unlikely that nicotinic functions as a physiological ligand of the HCA2 receptor (Gille et al., 2008). In 2005, the ketone body 3-hydroxybutyrate was described as the first endogenous agonist of HCA2 (Taggart et al., 2005). While other ketone bodies, such as acetoacetate and acetone, were inactive at HCA2, racemic 3-hydroxybutyrate induced GTPγS-binding to human and mouse HCA2 with an EC50 value of approximately 0.7 mM. Notably, the physiologically relevant stereoisomer (R)-3-hydroxybutyrate was twice as potent as its respective (S)-enantiomer at both mouse and human HCA2 receptors. By contrast, HCA1 and HCA3 receptors were not activated by 3-hydroxybutyrate. In addition, several other carboxylic acids with carbon chain lengths ranging from C4 to C8 activated human and mouse HCA2 with EC50 values ranging from 0.13 to 1.6 mM. However, systemic plasma levels of these short chain fatty acids would be too low to activate HCA2. In contrast, plasma concentrations of the ketone body 3-hydroxybutyrate are strongly elevated during fasting and can reach up to 6–8 mM during prolonged starvation (Owen et al., 1969; Cahill, 2006). These plasma levels are sufficient to fully activate the HCA2 receptor.
HCA3
Despite its pronounced homology to HCA2, the HCA3 receptor is not activated by the HCA2-agonists nicotinic acid and 3-hydroxybutyrate and has remained as an orphan receptor until recently, when 2- and 3-hydroxy medium chain fatty acids were described as the first endogenously occurring HCA3-specific agonists (Ahmed et al., 2009a). 2-hydroxy-octanoic acid and 3-hydroxy-octanoic acid-induced GTPγS-binding in membranes of HCA3-transfected HEK-293T cells with an EC50 of 4 and 8 μM, respectively. Notably, both 2- and 3-hydroxy-octanoic acid did not activate HCA1, HCA2, or the free fatty acid receptors FFA1, FFA2, and FFA3. While the physiological relevance of 2-hydroxyoctanoate is unknown, 3-hydroxy medium chain carboxylic acids are intermediates of mitochondrial β-oxidation of fatty acids. Under conditions of increased β-oxidation, such as starvation, ketogenic diet, or diabetic ketoacidosis, plasma levels of 3-hydroxy-octanoate can reach up to 5–20 μM, levels that are sufficient to activate HCA3 receptors (Costa et al., 1998; Ahmed et al., 2009a).
In another study aromatic D-amino acids were reported as specific agonists of the HCA3 receptor (Irukayama-Tomobe et al., 2009). D-phenylalanine and D-tryptophan inhibited forskolin-induced activation of cAMP responsive element (CRE)-driven luciferase activity with EC50 values of 9 and 3.7 μM, respectively (Irukayama-Tomobe et al., 2009). Aromatic D-amino acids have been found in some peptides from amphibian skin (Jilek et al., 2005). Whether aromatic D-amino acids are produced in mammalian species and can reach plasma levels sufficient to activate HCA3 is currently unknown. Thus, the physiological relevance of aromatic D-amino acids as potential endogenous HCA3 agonists remains elusive.
Genomic Location and Expression of HCA Receptors
Genomic Location
The genes encoding for HCA1, HCA2, and HCA3 are tandemly located on human chromosome 12q24.31. In Mus musculus the genes of HCA1 and HCA3 are found next to each other on chromosome 5F. It is noteworthy that in all species the genes encoding for HCA receptors consist of a single exon (Figure 1).
Expression of HCA Receptors
HCA1
Lee et al. (2001) detected mRNA of HCA1 in human pituitary by Northern blot analysis. While others have never confirmed this, several studies have independently shown by quantitative PCR that the HCA1 receptor is predominantly expressed in adipose tissue (Wise et al., 2003; Ge et al., 2008; Jeninga et al., 2009; Liu et al., 2009; Ahmed et al., 2010). By using transgenic reporter mice that express monomeric red fluorescent protein under the transcriptional control of endogenous HCA1 regulatory elements, it was demonstrated on a cellular level that HCA1 expression is indeed localized to adipocytes (Ahmed et al., 2010). Interestingly, HCA1 expression was induced during differentiation of 3T3-L1 adipocyte precursors and highest in terminally differentiated adipocytes (Ge et al., 2008; Jeninga et al., 2009). In addition, it was shown that the peroxisome proliferator-activated receptor-γ (PPARγ) agonist rosiglitazone induced transcription of the HCA1 gene by binding of PPARγ/retinoid X receptor to PPAR-response elements in the HCA1 promoter (Jeninga et al., 2009). Recently, it was shown that mRNA levels of HCA1 were reduced in mouse adipose tissue in response to lipopolysaccharide administration (Feingold et al., 2011).
HCA2
Similar to HCA1, HCA2 expression was detected at high levels in white and brown adipose tissue (Soga et al., 2003; Tunaru et al., 2003; Wise et al., 2003; Benyo et al., 2005), as well as in differentiated 3T3-L1 adipocytes upon treatment with rosiglitazone (Jeninga et al., 2009). In contrast to the HCA1 receptor, HCA2 is expressed in various immune cells including macrophages, neutrophils, dendritic cells, and epidermal Langerhans cells (Schaub et al., 2001; Benyo et al., 2005, 2006; Maciejewski-Lenoir et al., 2006; Kostylina et al., 2008; Tang et al., 2008; Ahmed et al., 2009a). HCA2 expression in macrophages was induced by treatment with IFN-γ or TNF-α (Schaub et al., 2001). Recent studies reported expression of HCA2 in epithelial cells. For instance, expression of HCA2 has been described in mouse retinal pigment epithelium (Martin et al., 2009), as well as in luminal colonic epithelium (Thangaraju et al., 2009). While some reports detected mRNA levels of HCA2 in primary human keratinocytes and immortalized keratinocytes (Maciejewski-Lenoir et al., 2006; Tang et al., 2008; Bermudez et al., 2011), Hanson et al. (2010) recently demonstrated by immunohistochemical analysis of transgenic HCA2-reporter mice that HCA2 expression was localized to keratinocytes. Recently, another study suggested expression of HCA2 and HCA3 in the epidermis of human skin sections as well as in a skin cancer cell line by using an antibody against HCA2/HCA3 (Bermudez et al., 2011).
HCA3
The expression pattern of the HCA3 receptor is comparable to that of HCA2. HCA3 is highly expressed in human white adipose tissue (Soga et al., 2003; Tunaru et al., 2003; Wise et al., 2003), and HCA3 expression was induced at least in vitro by treatment with PPARγ agonists in human multipotent adipose-derived stem cells (Jeninga et al., 2009). Similar to HCA2, HCA3 is also found in various immune cells, such as macrophages, monocytes, and neutrophils (Nomura et al., 1993; Yousefi et al., 2000; Ahmed et al., 2009a; Irukayama-Tomobe et al., 2009). Moreover, HCA3 expression was induced upon stimulation of neutrophils with cytokines (Yousefi et al., 2000). Some data suggest expression of HCA3 receptor at the apical membrane of human colonic epithelium by using antibodies directed at HCA3 (Thangaraju et al., 2009).
G Protein Coupling and Signaling
G Protein Coupling
Aktories et al. (1983) showed that the nicotinic acid-induced effects on adenylyl cyclase activity in rat adipocyte membranes were sensitive to treatment with pertussis toxin, a protein that specifically inactivates α-subunits of Gi/Go-type G proteins. With the deorphanization of HCA receptors several studies have independently demonstrated that the effects mediated by HCA1, HCA2, and HCA3 were sensitive to pertussis toxin treatment (Soga et al., 2003; Tunaru et al., 2003; Wise et al., 2003; Cai et al., 2008; Ahmed et al., 2009a, 2010; Irukayama-Tomobe et al., 2009; Liu et al., 2009) and, thus, HCA1, HCA2, and HCA3 receptors couple to Gi/Go-type G proteins.
Downstream Signaling
Activation of Gi/Go coupled GPCRs results in decreased adenylyl cyclase activity and decreased production of cAMP. For all three HCA receptors it was shown that receptor activation leads decreased cAMP levels in heterologous expression systems as well as in primary human and mouse adipocytes (Soga et al., 2003; Tunaru et al., 2003; Wise et al., 2003; Cai et al., 2008; Ge et al., 2008; Ahmed et al., 2009a, 2010; Irukayama-Tomobe et al., 2009). In adipocytes, cAMP is an important second messenger to induce lipolysis by activation protein kinase A, which in turn phosphorylates and activates lipolytic enzymes including hormone-sensitive lipase (Duncan et al., 2007). Thus, activation of HCA receptors has been demonstrated to result in an anti-lipolytic effect in primary adipocytes and in mice (Tunaru et al., 2003; Taggart et al., 2005; Ahmed et al., 2009a, 2010). In immune cells, activation of Gi/Go coupled receptors leads to increased intracellular calcium release through activation of phospholipase C β-isoforms which is most likely dependent on βγ-subunits (Exton, 1996). Accordingly, HCA2 and HCA3 activation in immune cells has been shown to evoke an increase in intracellular calcium concentrations (Benyo et al., 2006; Ahmed et al., 2009a; Irukayama-Tomobe et al., 2009). It has been hypothesized that the niacin-induced HCA2-dependent increase in intracellular calcium levels may activate Ca2+-sensitive phospholipase A2 (PLA2) which would result in the release of arachidonic acid and the subsequent formation of prostanoids. Indeed, this mechanism could explain the rapid formation of prostaglandin E2 and prostaglandin D2 observed in response to nicotinic acid. An alternative mechanism of nicotinic acid-induced PLA2-activation and formation of prostanoids could involve HCA2-dependent activation and phosphorylation of extracellular signal regulated kinase (ERK), which has been described for all HCA receptors (Tunaru et al., 2003; Richman et al., 2007; Ahmed et al., 2009a; Liu et al., 2009; Walters et al., 2009). Walters et al. (2009) provide evidence that this effect requires the recruitment of β-arrestin to the plasma membrane upon receptor activation. Moreover, it was shown that β-arrestin could bind and activate cytosolic PLA2, which resulted in increased liberation of arachidonic acid (Walters et al., 2009).
Receptor Desensitization
Observations in patients described the development of tolerance to flushing in response to treatment with niacin which was accompanied by reduced plasma levels of prostanoids (Stern et al., 1991). A study by Benyo et al. (2005) showed that nicotinic acid-induced HCA2 receptor-dependent flushing in mice desensitizes within minutes as well as nicotinic acid-induced increases in intracellular calcium concentrations in neutrophils (Kostylina et al., 2008). To date, the precise molecular mechanisms underlying these desensitization phenomena are unknown. However, when HCA1 and HCA2 were heterologously expressed, receptor internalization upon agonist exposure has been observed (Richman et al., 2007; Liu et al., 2009; Li et al., 2010). Interestingly, partial agonists of HCA2, which did not activate ERK and showed reduced flushing, were unable to induce receptor internalization (Richman et al., 2007). Moreover, internalization of the HCA2 receptor in response to nicotinic acid seems to involve G protein-coupled receptor kinase 2 (GRK2) and arrestin 3 (Li et al., 2010).
Receptor Dimerization
Some GPCRs can assemble into homo-/heterodimeric or oligomeric complexes. By using bioluminescence resonance energy transfer it was recently shown that HCA2 and HCA3 receptor constructs can interact when heterologously expressed in human embryonic kidney cells, suggesting the formation of homodimers and heterodimers, respectively (Mandrika et al., 2010). HCA2 and HCA3 are highly homologous and have a largely overlapping expression pattern in humans. However, whether dimeric complexes of HCA2 and HCA3 receptors occur in native tissues and whether they affect HCA receptor function or signaling remains unknown.
Biological Roles of HCA Receptors
HCA1
Given the fact that the HCA1 receptor is expressed in adipocytes and that it mediates lactate-induced Gi-dependent inhibition of adenylyl cyclase, it is plausible that HCA1 would mediate an inhibition of lipolysis in adipocytes. Indeed, several studies have shown that HCA1 mediates lactate-induced inhibition of lipolysis in primary adipocytes as well as in mice (Cai et al., 2008; Liu et al., 2009; Ahmed et al., 2010).
During intensive exercise, the skeletal muscle is the major source of lactate production in the body (Brooks and Mercier, 1994). With increasing exercise intensity, carbohydrate utilization becomes the major energy source and lactate accumulates in the plasma since lactate removal by oxidative metabolism is reduced (Brooks and Mercier, 1994). Since the accumulation of lactate in the blood during intensive exercise coincides with a decrease in fatty acid oxidation, a direct effect of lactate on fatty acid release from adipocytes has been suggested (Issekutz and Miller, 1962; Fredholm, 1971; Boyd et al., 1974). Although a causal link between elevated lactate levels and decreased fatty acid release and oxidation is plausible, no evidence for this concept has been provided; it has rather remained controversial (Trudeau et al., 1999). Exercise studies on wild type and HCA1-deficient mice that were trained to exercise at an intensity to reach plasma levels of lactate sufficient to activate HCA1, showed no differences in free fatty acid or glycerol plasma concentrations between wild type and HCA1-deficient mice (Ahmed et al., 2010). These findings suggest that it is quite unlikely that HCA1 is critically involved in the regulation of lipolysis during intensive exercise.
Another source of lactate is the adipose tissue. In the presence of insulin, it can convert more that 50% of the metabolized glucose into lactate (DiGirolamo et al., 1992). Lactate is released by adipocytes and serves mainly as a substrate for hepatic gluconeogenesis. Microdialysis studies in adipose tissue of humans and mice have demonstrated that insulin-induced glucose uptake results in a several-fold increase in interstitial lactate concentration within the adipose tissue (Jansson et al., 1990, 1994; Qvisth et al., 2007; Ahmed et al., 2010) raising the intriguing possibility that lactate produced by adipocytes in the postabsorptive state would inhibit lipolysis through HCA1 and thereby contribute to the anti-lipolytic effects of insulin. By using mice lacking the HCA1 receptor, Ahmed et al. (2010) demonstrated that insulin-induced decrease in cAMP and insulin-induced inhibition of lipolysis were strongly reduced in HCA1-deficient mice. Similarly, the anti-lipolytic effects of insulin on isolated adipocytes incubated in the presence of high glucose, were greatly reduced in HCA1-deficient adipocytes (Ahmed et al., 2010). Thus, lactate and HCA1 function in an autocrine and paracrine loop to mediate insulin-induced inhibition of lipolysis in the fed state (Figure 2).
When placed on a hypercaloric diet, mice lacking HCA1 show a reduced weight gain by about 10% as compared to wild type littermates (Ahmed et al., 2010). The reduced body weight was not accompanied by an increase in glucose tolerance, most likely due to transient elevations of free fatty acids, which may impair insulin sensitivity.
HCA2
The ketone body 3-hydroxybutyrate has been identified as the endogenous agonist of the HCA2 receptor and activates the receptor with an EC50 of 0.7 mM (Taggart et al., 2005). In the fed state, plasma concentrations of 3-hydroxybutyrate are below 0.5 mM but they increase up to 2 mM after an overnight fast and can reach even up to 6–8 mM during prolonged starvation (Owen et al., 1969; Cahill, 2006). Ketone bodies are produced by the liver by enzymatic condensation of acetyl-CoA molecules that arise from β-oxidation of fatty acids. Because the lipolytic rate determines the amount of fatty acids available for β-oxidation and subsequent ketone body formation, activation of HCA2 by 3-hydroxybutyrate and the subsequent anti-lipolytic effect represent a negative feedback regulation of lipolysis during starvation. Taggart et al. (2005) have shown that the anti-lipolytic effect of 3-hydroxybutyrate observed in adipocytes from wild type mice is abolished in adipocytes isolated from HCA2-deficient mice. It is therefore suggested that HCA2, being activated by 3-hydroxybutyrate, senses increased β-oxidation and ketone body formation, and, through inhibition of lipolysis, restricts further supply of fatty acids for ketogenesis in order to economize triglyceride utilization and to maintain energy homeostasis (Figure 3).
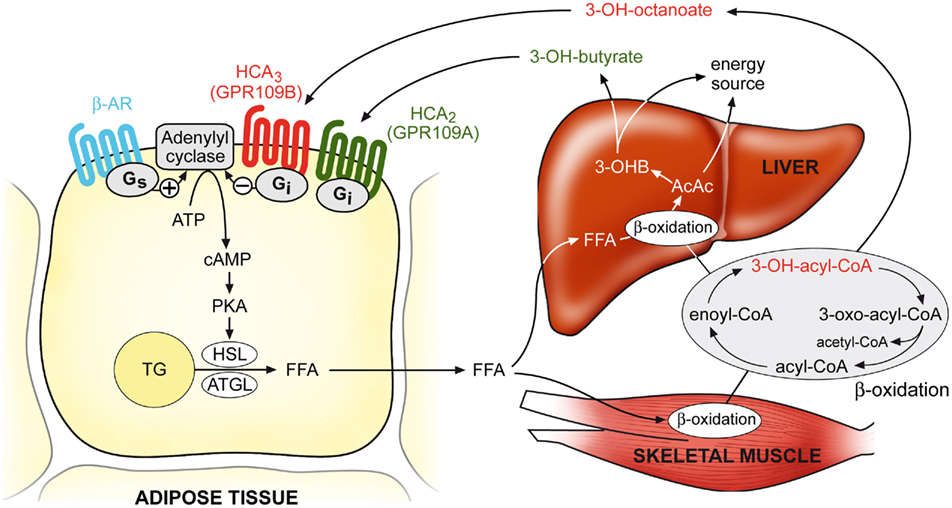
Figure 3. Model of the physiological functions of HCA2 and HCA3 receptors. During conditions of increased β-oxidation rates (e.g., during fasting), plasma concentrations of the ketone body 3-hydroxybutyrate and β-oxidation intermediates, in particular 3-hydroxy-octanoate, reach levels sufficient to activate their respective receptors HCA2 and HCA3, which then mediate an inhibition of adipocyte lipolysis through Gi/Go-dependent inhibition of adenylyl cyclase. Thereby, HCA2 and HCA3 regulate lipolysis in a negative feedback manner, and counter-regulate pro-lipolytic stimuli in order to economize triglyceride utilization and avoid a waste of energy substrates. AC, adenylyl cyclase; β-AR, β-adrenergic receptor; PKA, protein kinase A; TG, triglyceride; HSL, hormone-sensitive lipase; ATGL, adipocyte triglyceride lipase; FFA, free fatty acids; 3-OHB, 3-hydroxybutyrate; AcAc, acetoacetate.
The HCA2 receptor not only mediates the anti-lipolytic effects of the drug nicotinic acid, it is also involved in its lipid-modifying and anti-atherosclerotic effects (Gille et al., 2008; Lukasova et al., 2011). At pharmacological doses, nicotinic acid reduces plasma concentrations of VLDL and LDL cholesterol, triglycerides, and lipoprotein(a) while it increases levels of HDL cholesterol (Gille et al., 2008). Specifically, activation of HCA2 by nicotinic acid rapidly decreases plasma levels of free fatty acids which lead to lower fatty acid supply to the liver and in turn to reduced synthesis of VLDL cholesterol and triglycerides, and subsequently to lower plasma levels of LDL cholesterol. To date, the underlying mechanism of nicotinic acid-induced increases in HDL cholesterol and a role of HCA2 in this respect are unclear. One hypothesis is that a reduced triglyceride content in apolipoprotein B containing particles, such as VLDL and LDL, lead to decreased exchange of triglycerides for cholesteryl esters from HDL particles, a process mediated by cholesterol ester transfer protein (CETP) (Gille et al., 2008). In mice that were engineered to express human CETP it was shown that HDL elevation depends on the presence of CETP (Hernandez et al., 2007). Because mice naturally lack CETP and have a quite different lipoprotein metabolism as compared to humans, the evaluation of genetic mouse models with regard to HDL metabolism becomes challenging. The development of HCA2 receptor-selective antagonists would provide essential tools to dissect the role of HCA2 in vivo.
Apart from adipocytes, the HCA2 receptor is expressed in various immune cells including macrophages. A recent study has shown that the anti-atherosclerotic effects of nicotinic acid are not only based on changes in lipid profile but also involve direct effects of nicotinic acid on immune cells expressing HCA2 (Lukasova et al., 2011). Nicotinic acid-induced reduction in atherosclerotic lesion size was observed in wild type mice, whereas this effect was reduced in HCA2-deficient mice or mice that were transplanted with HCA2-deficient bone marrow. Moreover, it was shown that HCA2 was expressed in macrophages localized to atherosclerotic plaques, and HCA2 mediated the nicotinic acid-induced inhibition of macrophage recruitment to atherosclerotic lesions (Lukasova et al., 2011). In addition, nicotinic acid stimulated cholesterol efflux from macrophages through HCA2. However, the physiological role of HCA2 receptors in immune cells remains unclear. Whether the natural agonist of HCA2, 3-hydroxybutyrate, which is elevated during prolonged starvation, would exert anti-inflammatory effects during starvation and whether these effects are of physiological relevance is currently unknown.
HCA2 is also expressed in epidermal Langerhans cells, a population of dendritic cells residing in the skin, as well as in keratinocytes and both cell types have been shown to mediate flushing, a cutaneous vasodilation, and the major side effect of nicotinic acid (Benyo et al., 2005, 2006; Hanson et al., 2010). In fact, flushing albeit harmless is the main reason for patients to abrogate nicotinic acid therapy. Nicotinic acid and the anti-psoriatic drug monomethylfumarate, which also activates HCA2, induce flushing through HCA2, a reaction that is characterized by a biphasic increase in dermal blood flow (Hanson et al., 2010). The first peak is mediated by HCA2 on Langerhans cells and cyclooxygenase-1-dependent formation of PDE2 and PGD2, whereas the second peak involves HCA2 on keratinocytes and cyclooxygenase-2-dependent production of PGE2 (Hanson et al., 2010).
Besides its pharmacological role, the physiological function of HCA2 in the skin is rather unknown. One may speculate that HCA2 would be involved in modulation of various inflammatory conditions in the skin, including tissue damage through UV radiation or even cancer, for instance. A recent study reported expression of HCA2 in the squamous cell carcinoma cell line SCC-25, however a functional role of HCA2 has not been established (Bermudez et al., 2011). Another type of cells expressing HCA2 are cells of the intestinal and colonic epithelium where HCA2 may respond to butyrate, a short chain fatty acid present at millimolar concentrations in the gut lumen, and to thereby act as an anti-inflammatory receptor or tumor suppressor (Thangaraju et al., 2009).
HCA3
Similar to HCA2, HCA3 mediates an inhibition of lipolysis in adipocytes (Ahmed et al., 2009a). During various conditions of increased β-oxidation rates, including fasting, ketogenic diet, diabetic ketoacidosis and inherited mitochondrial β-oxidation disorders, plasma concentrations of β-oxidation intermediates, like 3-hydroxy-octanoate, reach levels sufficient to activate HCA3 (Costa et al., 1998; Jones et al., 2000; Ahmed et al., 2009a). Under these conditions, HCA3 mediates – similarly to HCA2 – a negative feedback mechanism by inducing an inhibition of lipolysis (Figure 3). Adipose tissue stores an enormous amount of energy in the form of fat and its mobilization must be precisely controlled in order to optimize triglyceride utilization and to avoid waste of energy. Because HCA3 is exclusively expressed in humans and higher primates, it is conceivable that during evolution HCA3 may have been advantageous in these species acting as an additional mechanism to control lipolysis during periods of starvation.
Similar to HCA2, HCA3 was reported to be expressed in various human immune cells and shown to be activated by endogenous agonists resulting in intracellular calcium release (Ahmed et al., 2009a; Irukayama-Tomobe et al., 2009). In addition, HCA3 is suggested to be expressed in gut epithelial cells (Thangaraju et al., 2009). Therefore one may expect similar functions of HCA2 and HCA3 in immune cells, however, the physiological role of HCA3 in human immune functions has yet to be determined.
Therapeutic Potential of HCA Receptors
To date, the HCA2 receptor is the only member of the HCA family that is used as a target in clinical therapy. Nicotinic acid has been used for more than 50 years in anti-dyslipidemic therapy (Carlson, 2005), and it was rather recently shown that nicotinic mediates its anti-lipolytic, anti-dyslipidemic, and anti-atherosclerotic effects through HCA2 (Tunaru et al., 2003; Lukasova et al., 2011). Several clinical trials indicated that a combination of nicotinic acid and HMG-CoA reductase inhibitors (statins) is effective in lowering LDL cholesterol and raising HDL cholesterol plasma levels and reducing cardiovascular events as well as size of atherosclerotic lesions (Brown et al., 2001; Taylor et al., 2004, 2009). Currently, large clinical studies evaluate the efficacy of a nicotinic acid in combination with statins in reducing relevant clinical endpoints including progression of cardiovascular-disease, incidence of major cardiovascular events, and cardiovascular-disease associated mortality.
Flushing is the major side effect of nicotinic acid treatment and is mediated by HCA2-induced cyclooxygenase-dependent formation of prostanoids. Because flushing severely affects patients’ compliance, several anti-flushing strategies have been developed. While extended-release formulations of nicotinic acid did not sufficiently reduce flushing (Capuzzi et al., 1998), blockade of prostaglandin synthesis, or signaling, would be a causal approach to decrease flushing in patients. Inhibition of cyclooxygenase-1 using acetylsalicylic acid can reduce flushing (Oberwittler and Baccara-Dinet, 2006), however, long-term treatment with effective doses of acetylsalicylic acid may in turn cause severe side effects. A more recent approach is to selectively inhibit prostaglandin D2 (PGD2)-mediated effects in the development of flushing (Cheng et al., 2006). In clinical studies, the combination of nicotinic acid with the PGD2 receptor antagonist laropiprant was able to effectively reduce flushing symptoms (McKenney et al., 2010). As a consequence, a fixed dosage formulation of nicotinic acid and laropiprant has been approved for therapy by the European Medical Agency.
Several groups have developed synthetic HCA2-specific ligands including full receptor agonists such as pyrazole-3-carboxylic acids and anthranilic acid derivatives, allosteric agonists like pyrazolopyrimidines as well as partial agonists such like bicyclic pyrazoles (van Herk et al., 2003; Shen et al., 2007, 2008; Boatman et al., 2008; Semple et al., 2008). A detailed discussion of synthetic HCA ligands is not a main theme of this article, however, a recent review on the nomenclature and classification of HCA receptors by Offermanns et al. (2011), comprehensively covers this topic, to which the interested reader is kindly referred to. Interestingly, a partial agonist of HCA2, MK-0354, shows full anti-lipolytic activity but reduced vasodilation in mice when compared to nicotinic acid (Richman et al., 2007). While the anti-lipolytic effect is mediated by Gi proteins, molecular evidence has been provided that β-arrestin-dependent recruitment of ERK is involved in the mechanism of flushing and thus would be independent of G protein signaling (Walters et al., 2009). Thus, the efficacy of MK-0354 has been evaluated in clinical trials on dyslipidemic patients, however, while showing reduced flushing, no beneficial effects on plasma lipids have been observed (Lai et al., 2008).
Because the expression pattern of HCA3 is quite similar to HCA2, it is rather unlikely that HCA3-specific agonists would cause reduced flushing as compared to HCA2-agonists. Several HCA3-specific agonists have been synthesized that are expected to inhibit lipolysis in human adipocytes (Semple et al., 2006; Skinner et al., 2007, 2009), however, because rodents and most other mammals lack HCA3 studies in humans or primates would be required to evaluate the pharmacological effects of HCA3 agonists. Alternatively, a transgenic mouse model expressing HCA3 may serve as a system to examine HCA3-specific ligands in vivo. In contrast to HCA2 and HCA3, HCA1 is not expressed in immune cells but is also present in adipocytes where it couples to Gi proteins (Table 2). Therefore, agonists of HCA1 may represent a rational approach to preserve the anti-lipolytic effects while eliminating the unwanted effects of flushing. However, a recent study using HCA1-deficient mice showed that HCA1 mediates the anti-lipolytic effects of insulin and that lack of HCA1-mediated anti-lipolysis causes reduced weight gain on a hypercaloric diet (Ahmed et al., 2010). Therefore, also antagonizing HCA1 is of pharmacotherapeutic potential yet to be explored. To date, synthetic ligands of HCA1 have only been reported in the patent literature that is not being discussed here.
Apart from anti-dyslipidemic treatment strategies, HCA2 and HCA3 receptors being expressed in immune represent potential drug targets for anti-inflammatory therapy. Recently, monomethyl ester of fumaric acid, an established drug in anti-psoriatic therapy, has been shown to specifically activate HCA2 but not HCA3 or HCA1 (Tang et al., 2008). Fumaric esters have long been used as oral formulations and their efficacy and safety has been evaluated in clinical studies. Interestingly, some side effects of monomethylfumarate including diarrhea and especially flushing are similar to the unwanted effects of nicotinic acid (Nugteren-Huying et al., 1990; Hoefnagel et al., 2003; Reich et al., 2009). In mice it has been shown that both nicotinic acid and monomethylfumarate-induced flushing is mediated by HCA2 (Hanson et al., 2010). Finally, studies in mice lacking HCA2 in a disease model of psoriasis are needed to determine whether the anti-psoriatic effects of monomethylfumarate are essentially mediated by HCA2. In addition, antagonists of HCA2 would help to further dissect the pharmacological effects of fumaric acid esters in vivo. Consistent with the anti-inflammatory effects of monomethylfumarate observed in psoriasis therapy, the dimethyl ester of fumaric acid exerted anti-inflammatory and neuroprotective effects in a phase II clinical trail with patients suffering from multiple sclerosis (Kappos et al., 2008). Currently, the same formulation named BG-12 consisting of dimethyl fumarate is being evaluated in a larger phase III clinical study (Gold, 2011). HCA2 and HCA3 receptors are also expressed in the apical membrane of human intestinal epithelial cells. It is intriguing to speculate about the pathophysiological function and therapeutic potential of HCA2 and HCA3 for the treatment of immune diseases like psoriasis, multiple sclerosis, or inflammatory bowel disease.
Conclusion and Future Perspectives
In 2003, the HCA2 receptor was identified as the target of the anti-dyslipidemic drug nicotinic acid (niacin) and various synthetic agonists of HCA2 have been developed since then. However, the physiological ligands of HCA receptors remained elusive until recently, when hydroxy-carboxylic acids have been identified as endogenous agonists of HCA1, HCA2, and HCA3 (Table 1). Traditionally, hormones, mediators, and neurotransmitters were regarded as the classic endogenous ligands of GPCRs, and therefore metabolites themselves, including hydroxy-carboxylic acids or fatty acids may have been overlooked in the search for potential ligands of orphan GPCRs. In addition, the relatively low potencies of HCA receptor ligands have probably hampered their identification, especially in the case of HCA1 and HCA2, with EC50 values being in the millimolar range (Table 1). However, under various physiological and pathophysiological conditions plasma concentrations of these hydroxy-carboxylic acids reach levels sufficient to activate their respective receptors and to inhibit lipolysis. Similar to the receptor families of free fatty acids and citric acid cycle metabolites, HCA receptors represent another subfamily of GPCRs that is activated by intermediates of energy metabolism and functions as a metabolic sensor.
Besides the established drug target HCA2, HCA1, and HCA3 are also being evaluated as potential targets for anti-dyslipidemic therapies. Activation of HCA1, which is not expressed in immune cells or keratinocytes, would be expected to exert anti-lipolytic effects that are possibly not accompanied by flushing. In contrast, studies on HCA1-deficient mice suggest that antagonism at HCA1 could be used for treatment of obesity by reducing the postprandial anti-lipolytic effects of insulin mediated by HCA1. However, to date, no antagonists of HCA receptors have been reported. The development of HCA1-specific antagonists will help to fully explore the therapeutic potential of HCA1 in the treatment of obesity. Most importantly, it needs to be further investigated under which conditions patients would benefit from one or the other treatment strategy, i.e., the anti-lipolytic effects through activation of HCA1 or the weight-lowering effects of blocking HCA1 receptors.
Moreover, there is growing evidence that agonism at HCA2 and HCA3, which are both present in various immune cells, could be exploited for the treatment of various inflammatory diseases. For instance, HCA2 was recently shown to reduce atherosclerotic lesions by inhibiting macrophage recruitment and increasing cholesterol efflux from macrophages in atherosclerotic plaques, providing a causal mechanism of HCA2 to reduce atherogenesis. In this respect, it is intriguing to speculate about the therapeutic potential of other (orphan) Gi-coupled GPCRs to reduce inflammation and atherosclerosis. In addition, HCA2, which is expressed in keratinocytes and dermal immune cells, is activated by the anti-psoriatic drug monomethyl fumarate. Studies using animal disease models of psoriasis and the development of HCA2-specific antagonists are needed to explore a possible role of HCA2 in the etiology and progression of psoriasis and to evaluate the therapeutic potential of HCA2 in anti-psoriatic therapy. Much more future work is needed to investigate the pathophysiological function of HCA2 and HCA3 in intestinal epithelial cells with respect to the development of intestinal autoimmune diseases or cancer.
In conclusion, the generation and analysis of genetic animal models in combination with the development of potent and subtype-specific agonists and antagonists of HCA receptors will be a powerful strategy to further advance the evaluation of HCA receptors as potential drug targets for new therapeutic strategies against metabolic and immune disease.
Conflict of Interest Statement
The author declares that the research was conducted in the absence of any commercial or financial relationships that could be construed as a potential conflict of interest.
Acknowledgments
The author is funded by an EMBO Long-Term Fellowship of the European Molecular Biology Organization (EMBO).
References
Ahmed, K., Tunaru, S., Langhans, C. D., Hanson, J., Michalski, C. W., Kolker, S., Jones, P. M., Okun, J. G., and Offermanns, S. (2009a). Deorphanization of GPR109B as a receptor for the beta-oxidation intermediate 3-OH-octanoic acid and its role in the regulation of lipolysis. J. Biol. Chem. 284, 21928–21933.
Ahmed, K., Tunaru, S., and Offermanns, S. (2009b). GPR109A, GPR109B and GPR81, a family of hydroxy-carboxylic acid receptors. Trends Pharmacol. Sci. 30, 557–562.
Ahmed, K., Tunaru, S., and Offermanns, S. (2009c). GPR109A, GPR109B and GPR81, a family of hydroxy-carboxylic acid receptors. Trends Pharmacol. Sci. 30, 557–562.
Ahmed, K., Tunaru, S., Tang, C., Muller, M., Gille, A., Sassmann, A., Hanson, J., and Offermanns, S. (2010). An autocrine lactate loop mediates insulin-dependent inhibition of lipolysis through GPR81. Cell Metab. 11, 311–319.
Aktories, K., Schultz, G., and Jakobs, K. H. (1983). Islet-activating protein prevents nicotinic acid-induced GTPase stimulation and GTP but not GTP gamma S-induced adenylate cyclase inhibition in rat adipocytes. FEBS Lett. 156, 88–92.
Benyo, Z., Gille, A., Bennett, C. L., Clausen, B. E., and Offermanns, S. (2006). Nicotinic acid-induced flushing is mediated by activation of epidermal langerhans cells. Mol. Pharmacol. 70, 1844–1849.
Benyo, Z., Gille, A., Kero, J., Csiky, M., Suchankova, M. C., Nusing, R. M., Moers, A., Pfeffer, K., and Offermanns, S. (2005). GPR109A (PUMA-G/HM74A) mediates nicotinic acid-induced flushing. J. Clin. Invest. 115, 3634–3640.
Bermudez, Y., Benavente, C. A., Meyer, R. G., Coyle, W. R., Jacobson, M. K., and Jacobson, E. L. (2011). Nicotinic Acid receptor abnormalities in human skin cancer: implications for a role in epidermal differentiation. PLoS ONE 6, e20487.
Boatman, P. D., Richman, J. G., and Semple, G. (2008). Nicotinic acid receptor agonists. J. Med. Chem. 51, 7653–7662.
Boyd, A. E. 3rd, Giamber, S. R., Mager, M., and Lebovitz, H. E. (1974). Lactate inhibition of lipolysis in exercising man. Metab. Clin. Exp. 23, 531–542.
Briscoe, C. P., Tadayyon, M., Andrews, J. L., Benson, W. G., Chambers, J. K., Eilert, M. M., Ellis, C., Elshourbagy, N. A., Goetz, A. S., Minnick, D. T., Murdock, P. R., Sauls, H. R. Jr., Shabon, U., Spinage, L. D., Strum, J. C., Szekeres, P. G., Tan, K. B., Way, J. M., Ignar, D. M., Wilson, S., and Muir, A. I. (2003). The orphan G protein-coupled receptor GPR40 is activated by medium and long chain fatty acids. J. Biol. Chem. 278, 11303–11311.
Brooks, G. A., and Mercier, J. (1994). Balance of carbohydrate and lipid utilization during exercise: the “crossover” concept. J. Appl. Physiol. 76, 2253–2261.
Brown, A. J., Goldsworthy, S. M., Barnes, A. A., Eilert, M. M., Tcheang, L., Daniels, D., Muir, A. I., Wigglesworth, M. J., Kinghorn, I., Fraser, N. J., Pike, N. B., Strum, J. C., Steplewski, K. M., Murdock, P. R., Holder, J. C., Marshall, F. H., Szekeres, P. G., Wilson, S., Ignar, D. M., Foord, S. M., Wise, A., and Dowell, S. J. (2003). The Orphan G protein-coupled receptors GPR41 and GPR43 are activated by propionate and other short chain carboxylic acids. J. Biol. Chem. 278, 11312–11319.
Brown, B. G., Zhao, X. Q., Chait, A., Fisher, L. D., Cheung, M. C., Morse, J. S., Dowdy, A. A., Marino, E. K., Bolson, E. L., Alaupovic, P., Frohlich, J., and Albers, J. J. (2001). Simvastatin and niacin, antioxidant vitamins, or the combination for the prevention of coronary disease. N. Engl. J. Med. 345, 1583–1592.
Cai, T. Q., Ren, N., Jin, L., Cheng, K., Kash, S., Chen, R., Wright, S. D., Taggart, A. K., and Waters, M. G. (2008). Role of GPR81 in lactate-mediated reduction of adipose lipolysis. Biochem. Biophys. Res. Commun. 377, 987–991.
Capuzzi, D. M., Guyton, J. R., Morgan, J. M., Goldberg, A. C., Kreisberg, R. A., Brusco, O. A., and Brody, J. (1998). Efficacy and safety of an extended-release niacin (Niaspan): a long-term study. Am. J. Cardiol. 82, 74U–81U; discussion 85U–86U.
Carlson, L. A. (2005). Nicotinic acid: the broad-spectrum lipid drug. A 50th anniversary review. J. Intern. Med. 258, 94–114.
Cheng, K., Wu, T. J., Wu, K. K., Sturino, C., Metters, K., Gottesdiener, K., Wright, S. D., Wang, Z., O’Neill, G., Lai, E., and Waters, M. G. (2006). Antagonism of the prostaglandin D2 receptor 1 suppresses nicotinic acid-induced vasodilation in mice and humans. Proc. Natl. Acad. Sci. U.S.A. 103, 6682–6687.
Costa, C. G., Dorland, L., Holwerda, U., de Almeida, I. T., Poll-The, B. T., Jakobs, C., and Duran, M. (1998). Simultaneous analysis of plasma free fatty acids and their 3-hydroxy analogs in fatty acid beta-oxidation disorders. Clin. Chem. 44, 463–471.
Covington, D. K., Briscoe, C. A., Brown, A. J., and Jayawickreme, C. K. (2006). The G-protein-coupled receptor 40 family (GPR40-GPR43) and its role in nutrient sensing. Biochem. Soc. Trans. 34, 770–773.
DiGirolamo, M., Newby, F. D., and Lovejoy, J. (1992). Lactate production in adipose tissue: a regulated function with extra-adipose implications. FASEB J. 6, 2405–2412.
Duncan, R. E., Ahmadian, M., Jaworski, K., Sarkadi-Nagy, E., and Sul, H. S. (2007). Regulation of lipolysis in adipocytes. Annu. Rev. Nutr. 27, 79–101.
Exton, J. H. (1996). Regulation of phosphoinositide phospholipases by hormones, neurotransmitters, and other agonists linked to G proteins. Annu. Rev. Pharmacol. Toxicol. 36, 481–509.
Feingold, K. R., Moser, A., Shigenaga, J. K., and Grunfeld, C. (2011). Inflammation inhibits GPR81 expression in adipose tissue. Inflamm. Res. 60, 991–995.
Foord, S. M., Bonner, T. I., Neubig, R. R., Rosser, E. M., Pin, J. P., Davenport, A. P., Spedding, M., and Harmar, A. J. (2005). International union of pharmacology. XLVI. G protein-coupled receptor list. Pharmacol. Rev. 57, 279–288.
Fredholm, B. B. (1971). The effect of lactate in canine subcutaneous adipose tissue in situ. Acta Physiol. Scand. 81, 110–123.
Ge, H., Weiszmann, J., Reagan, J. D., Gupte, J., Baribault, H., Gyuris, T., Chen, J. L., Tian, H., and Li, Y. (2008). Elucidation of signaling and functional activities of an orphan GPCR, GPR81. J. Lipid Res. 49, 797–803.
Gille, A., Bodor, E. T., Ahmed, K., and Offermanns, S. (2008). Nicotinic acid: pharmacological effects and mechanisms of action. Annu. Rev. Pharmacol. Toxicol. 48, 79–106.
Gold, R. (2011). Oral therapies for multiple sclerosis: a review of agents in phase III development or recently approved. CNS Drugs 25, 37–52.
Hanson, J., Gille, A., Zwykiel, S., Lukasova, M., Clausen, B. E., Ahmed, K., Tunaru, S., Wirth, A., and Offermanns, S. (2010). Nicotinic acid- and monomethyl fumarate-induced flushing involves GPR109A expressed by keratinocytes and COX-2-dependent prostanoid formation in mice. J. Clin. Invest. 120, 2910–2919.
He, W., Miao, F. J., Lin, D. C., Schwandner, R. T., Wang, Z., Gao, J., Chen, J. L., Tian, H., and Ling, L. (2004). Citric acid cycle intermediates as ligands for orphan G-protein-coupled receptors. Nature 429, 188–193.
Hernandez, M., Wright, S. D., and Cai, T. Q. (2007). Critical role of cholesterol ester transfer protein in nicotinic acid-mediated HDL elevation in mice. Biochem. Biophys. Res. Commun. 355, 1075–1080.
Hirasawa, A., Tsumaya, K., Awaji, T., Katsuma, S., Adachi, T., Yamada, M., Sugimoto, Y., Miyazaki, S., and Tsujimoto, G. (2005). Free fatty acids regulate gut incretin glucagon-like peptide-1 secretion through GPR120. Nat. Med. 11, 90–94.
Hoefnagel, J. J., Thio, H. B., Willemze, R., and Bouwes Bavinck, J. N. (2003). Long-term safety aspects of systemic therapy with fumaric acid esters in severe psoriasis. Br. J. Dermatol. 149, 363–369.
Ichimura, A., Hirasawa, A., Hara, T., and Tsujimoto, G. (2009). Free fatty acid receptors act as nutrient sensors to regulate energy homeostasis. Prostaglandins Other Lipid Mediat. 89, 82–88.
Irukayama-Tomobe, Y., Tanaka, H., Yokomizo, T., Hashidate-Yoshida, T., Yanagisawa, M., and Sakurai, T. (2009). Aromatic D-amino acids act as chemoattractant factors for human leukocytes through a G protein-coupled receptor, GPR109B. Proc. Natl. Acad. Sci. U.S.A. 106, 3930–3934.
Issekutz, B. Jr., and Miller, H. I. (1962). Plasma free fatty acids during exercise and the effect of lactic acid. Proc. Soc. Exp. Biol. Med. 237–239.
Itoh, Y., Kawamata, Y., Harada, M., Kobayashi, M., Fujii, R., Fukusumi, S., Ogi, K., Hosoya, M., Tanaka, Y., Uejima, H., Tanaka, H., Maruyama, M., Satoh, R., Okubo, S., Kizawa, H., Komatsu, H., Matsumura, F., Noguchi, Y., Shinohara, T., Hinuma, S., Fujisawa, Y., and Fujino, M. (2003). Free fatty acids regulate insulin secretion from pancreatic beta cells through GPR40. Nature 422, 173–176.
Jansson, P. A., Larsson, A., Smith, U., and Lonnroth, P. (1994). Lactate release from the subcutaneous tissue in lean and obese men. J. Clin. Invest. 93, 240–246.
Jansson, P. A., Smith, U., and Lonnroth, P. (1990). Evidence for lactate production by human adipose tissue in vivo. Diabetologia 33, 253–256.
Jeninga, E. H., Bugge, A., Nielsen, R., Kersten, S., Hamers, N., Dani, C., Wabitsch, M., Berger, R., Stunnenberg, H. G., Mandrup, S., and Kalkhoven, E. (2009). Peroxisome proliferator-activated receptor gamma regulates expression of the anti-lipolytic G-protein-coupled receptor 81 (GPR81/Gpr81). J. Biol. Chem. 284, 26385–26393.
Jilek, A., Mollay, C., Tippelt, C., Grassi, J., Mignogna, G., Mullegger, J., Sander, V., Fehrer, C., Barra, D., and Kreil, G. (2005). Biosynthesis of a D-amino acid in peptide linkage by an enzyme from frog skin secretions. Proc. Natl. Acad. Sci. U.S.A. 102, 4235–4239.
Jones, P. M., Quinn, R., Fennessey, P. V., Tjoa, S., Goodman, S. I., Fiore, S., Burlina, A. B., Rinaldo, P., Boriack, R. L., and Bennett, M. J. (2000). Improved stable isotope dilution-gas chromatography-mass spectrometry method for serum or plasma free 3-hydroxy-fatty acids and its utility for the study of disorders of mitochondrial fatty acid beta-oxidation. Clin. Chem. 46, 149–155.
Kappos, L., Gold, R., Miller, D. H., Macmanus, D. G., Havrdova, E., Limmroth, V., Polman, C. H., Schmierer, K., Yousry, T. A., Yang, M., Eraksoy, M., Meluzinova, E., Rektor, I., Dawson, K. T., Sandrock, A. W., O’Neill, G. N., and BG-12 Phase IIb Study Investigators. (2008). Efficacy and safety of oral fumarate in patients with relapsing-remitting multiple sclerosis: a multicentre, randomised, double-blind, placebo-controlled phase IIb study. Lancet 372, 1463–1472.
Kostylina, G., Simon, D., Fey, M. F., Yousefi, S., and Simon, H. U. (2008). Neutrophil apoptosis mediated by nicotinic acid receptors (GPR109A). Cell Death Differ. 15, 134–142.
Lai, E., Waters, M. G., Tata, J. R., Radziszewski, W., Perevozskaya, I., Zheng, W., Wenning, L., Connolly, D. T., Semple, G., Johnson-Levonas, A. O., Wagner, J. A., Mitchel, Y., and Paolini, J. F. (2008). Effects of a niacin receptor partial agonist, MK-0354, on plasma free fatty acids, lipids, and cutaneous flushing in humans. J. Clin. Lipidol. 2, 375–383.
Lander, E. S., Linton, L. M., Birren, B., Nusbaum, C., Zody, M. C., Baldwin, J., Devon, K., Dewar, K., Doyle, M., FitzHugh, W., Funke, R., Gage, D., Harris, K., Heaford, A., Howland, J., Kann, L., Lehoczky, J., LeVine, R., McEwan, P., McKernan, K., Meldrim, J., Mesirov, J. P., Miranda, C., Morris, W., Naylor, J., Raymond, C., Rosetti, M., Santos, R., Sheridan, A., Sougnez, C., Stange-Thomann, N., Stojanovic, N., Subramanian, A., Wyman, D., Rogers, J., Sulston, J., Ainscough, R., Beck, S., Bentley, D., Burton, J., Clee, C., Carter, N., Coulson, A., Deadman, R., Deloukas, P., Dunham, A., Dunham, I., Durbin, R., French, L., Grafham, D., Gregory, S., Hubbard, T., Humphray, S., Hunt, A., Jones, M., Lloyd, C., McMurray, A., Matthews, L., Mercer, S., Milne, S., Mullikin, J. C., Mungall, A., Plumb, R., Ross, M., Shownkeen, R., Sims, S., Waterston, R. H., Wilson, R. K., Hillier, L. W., McPherson, J. D., Marra, M. A., Mardis, E. R., Fulton, L. A., Chinwalla, A. T., Pepin, K. H., Gish, W. R., Chissoe, S. L., Wendl, M. C., Delehaunty, K. D., Miner, T. L., Delehaunty, A., Kramer, J. B., Cook, L. L., Fulton, R. S., Johnson, D. L., Minx, P. J., Clifton, S. W., Hawkins, T., Branscomb, E., Predki, P., Richardson, P., Wenning, S., Slezak, T., Doggett, N., Cheng, J. F., Olsen, A., Lucas, S., Elkin, C., Uberbacher, E., Frazier, M., Gibbs, R. A., Muzny, D. M., Scherer, S. E., Bouck, J. B., Sodergren, E. J., Worley, K. C., Rives, C. M., Gorrell, J. H., Metzker, M. L., Naylor, S. L., Kucherlapati, R. S., Nelson, D. L., Weinstock, G. M., Sakaki, Y., Fujiyama, A., Hattori, M., Yada, T., Toyoda, A., Itoh, T., Kawagoe, C., Watanabe, H., Totoki, Y., Taylor, T., Weissenbach, J., Heilig, R., Saurin, W., Artiguenave, F., Brottier, P., Bruls, T., Pelletier, E., Robert, C., Wincker, P., Smith, D. R., Doucette-Stamm, L., Rubenfield, M., Weinstock, K., Lee, H. M., Dubois, J., Rosenthal, A., Platzer, M., Nyakatura, G., Taudien, S., Rump, A., Yang, H., Yu, J., Wang, J., Huang, G., Gu, J., Hood, L., Rowen, L., Madan, A., Qin, S., Davis, R. W., Federspiel, N. A., Abola, A. P., Proctor, M. J., Myers, R. M., Schmutz, J., Dickson, M., Grimwood, J., Cox, D. R., Olson, M. V., Kaul, R., Raymond, C., Shimizu, N., Kawasaki, K., Minoshima, S., Evans, G. A., Athanasiou, M., Schultz, R., Roe, B. A., Chen, F., Pan, H., Ramser, J., Lehrach, H., Reinhardt, R., McCombie, W. R., de la Bastide, M., Dedhia, N., Blöcker, H., Hornischer, K., Nordsiek, G., Agarwala, R., Aravind, L., Bailey, J. A., Bateman, A., Batzoglou, S., Birney, E., Bork, P., Brown, D. G., Burge, C. B., Cerutti, L., Chen, H. C., Church, D., Clamp, M., Copley, R. R., Doerks, T., Eddy, S. R., Eichler, E. E., Furey, T. S., Galagan, J., Gilbert, J. G., Harmon, C., Hayashizaki, Y., Haussler, D., Hermjakob, H., Hokamp, K., Jang, W., Johnson, L. S., Jones, T. A., Kasif, S., Kaspryzk, A., Kennedy, S., Kent, W. J., Kitts, P., Koonin, E. V., Korf, I., Kulp, D., Lancet, D., Lowe, T. M., McLysaght, A., Mikkelsen, T., Moran, J. V., Mulder, N., Pollara, V. J., Ponting, C. P., Schuler, G., Schultz, J., Slater, G., Smit, A. F., Stupka, E., Szustakowski, J., Thierry-Mieg, D., Thierry-Mieg, J., Wagner, L., Wallis, J., Wheeler, R., Williams, A., Wolf, Y. I., Wolfe, K. H., Yang, S. P., Yeh, R. F., Collins, F., Guyer, M. S., Peterson, J., Felsenfeld, A., Wetterstrand, K. A., Patrinos, A., Morgan, M. J., de Jong, P., Catanese, J. J., Osoegawa, K., Shizuya, H., Choi, S., Chen, Y. J., and International Human Genome Sequencing Consortium (2001). Initial sequencing and analysis of the human genome. Nature 409, 860–921.
Lee, D. K., Nguyen, T., Lynch, K. R., Cheng, R., Vanti, W. B., Arkhitko, O., Lewis, T., Evans, J. F., George, S. R., and O’Dowd, B. F. (2001). Discovery and mapping of ten novel G protein-coupled receptor genes. Gene 275, 83–91.
Li, G., Shi, Y., Huang, H., Zhang, Y., Wu, K., Luo, J., Sun, Y., Lu, J., Benovic, J. L., and Zhou, N. (2010). Internalization of the human nicotinic acid receptor GPR109A is regulated by G(i), GRK2, and arrestin3. J. Biol. Chem. 285, 22605–22618.
Liu, C., Wu, J., Zhu, J., Kuei, C., Yu, J., Shelton, J., Sutton, S. W., Li, X., Yun, S. J., Mirzadegan, T., Mazur, C., Kamme, F., and Lovenberg, T. W. (2009). Lactate inhibits lipolysis in fat cells through activation of an orphan G-protein-coupled receptor, GPR81. J. Biol. Chem. 284, 2811–2822.
Lukasova, M., Malaval, C., Gille, A., Kero, J., and Offermanns, S. (2011). Nicotinic acid inhibits progression of atherosclerosis in mice through its receptor GPR109A expressed by immune cells. J. Clin. Invest. 121, 1163–1173.
Maciejewski-Lenoir, D., Richman, J. G., Hakak, Y., Gaidarov, I., Behan, D. P., and Connolly, D. T. (2006). Langerhans cells release prostaglandin D2 in response to nicotinic acid. J. Invest. Dermatol. 126, 2637–2646.
Mandrika, I., Petrovska, R., and Klovins, J. (2010). Evidence for constitutive dimerization of niacin receptor subtypes. Biochem. Biophys. Res. Commun. 395, 281–287.
Martin, P. M., Ananth, S., Cresci, G., Roon, P., Smith, S., and Ganapathy, V. (2009). Expression and localization of GPR109A (PUMA-G/HM74A) mRNA and protein in mammalian retinal pigment epithelium. Mol. Vis. 15, 362–372.
McKenney, J., Bays, H., Koren, M., Ballantyne, C. M., Paolini, J. F., Mitchel, Y., Betteridge, A., Kuznetsova, O., Sapre, A., Sisk, C. M., and Maccubbin, D. (2010). Safety of extended-release niacin/laropiprant in patients with dyslipidemia. J. Clin. Lipidol. 4, 105–112 e101.
Nomura, H., Nielsen, B. W., and Matsushima, K. (1993). Molecular cloning of cDNAs encoding a LD78 receptor and putative leukocyte chemotactic peptide receptors. Int. Immunol. 5, 1239–1249.
Nugteren-Huying, W. M., van der Schroeff, J. G., Hermans, J., and Suurmond, D. (1990). Fumaric acid therapy for psoriasis: a randomized, double-blind, placebo-controlled study. J. Am. Acad. Dermatol. 22, 311–312.
Oberwittler, H., and Baccara-Dinet, M. (2006). Clinical evidence for use of acetyl salicylic acid in control of flushing related to nicotinic acid treatment. Int. J. Clin. Pract. 60, 707–715.
Offermanns, S., Colletti, S. L., Lovenberg, T. W., Semple, G., Wise, A., and AP, I. J. (2011). International union of basic and clinical pharmacology. LXXXII: nomenclature and classification of hydroxy-carboxylic acid receptors (GPR81, GPR109A, and GPR109B). Pharmacol. Rev. 63, 269–290.
Owen, O. E., Felig, P., Morgan, A. P., Wahren, J., and Cahill, G. F. Jr. (1969). Liver and kidney metabolism during prolonged starvation. J. Clin. Invest. 48, 574–583.
Pierce, K. L., Premont, R. T., and Lefkowitz, R. J. (2002). Seven-transmembrane receptors. Nat. Rev. Mol. Cell Biol. 3, 639–650.
Qvisth, V., Hagstrom-Toft, E., Moberg, E., Sjoberg, S., and Bolinder, J. (2007). Lactate release from adipose tissue and skeletal muscle in vivo: defective insulin regulation in insulin-resistant obese women. Am. J. Physiol. Endocrinol. Metab. 292, E709–714.
Reich, K., Thaci, D., Mrowietz, U., Kamps, A., Neureither, M., and Luger, T. (2009). Efficacy and safety of fumaric acid esters in the long-term treatment of psoriasis–a retrospective study (FUTURE). J. Dtsch. Dermatol. Ges. 7, 603–611.
Richman, J. G., Kanemitsu-Parks, M., Gaidarov, I., Cameron, J. S., Griffin, P., Zheng, H., Guerra, N. C., Cham, L., Maciejewski-Lenoir, D., Behan, D. P., Boatman, D., Chen, R., Skinner, P., Ornelas, P., Waters, M. G., Wright, S. D., Semple, G., and Connolly, D. T. (2007). Nicotinic acid receptor agonists differentially activate downstream effectors. J. Biol. Chem. 282, 18028–18036.
Schaub, A., Futterer, A., and Pfeffer, K. (2001). PUMA-G, an IFN-gamma-inducible gene in macrophages is a novel member of the seven transmembrane spanning receptor superfamily. Eur. J. Immunol. 31, 3714–3725.
Semple, G., Skinner, P. J., Cherrier, M. C., Webb, P. J., Sage, C. R., Tamura, S. Y., Chen, R., Richman, J. G., and Connolly, D. T. (2006). 1-Alkyl-benzotriazole-5-carboxylic acids are highly selective agonists of the human orphan G-protein-coupled receptor GPR109b. J. Med. Chem. 49, 1227–1230.
Semple, G., Skinner, P. J., Gharbaoui, T., Shin, Y. J., Jung, J. K., Cherrier, M. C., Webb, P. J., Tamura, S. Y., Boatman, P. D., Sage, C. R., Schrader, T. O., Chen, R., Colletti, S. L., Tata, J. R., Waters, M. G., Cheng, K., Taggart, A. K., Cai, T. Q., Carballo-Jane, E., Behan, D. P., Connolly, D. T., and Richman, J. G. (2008). 3-(1H-tetrazol-5-yl)-1,4,5,6-tetrahydro-cyclopentapyrazole (MK-0354): a partial agonist of the nicotinic acid receptor, G-protein coupled receptor 109a, with antilipolytic but no vasodilatory activity in mice. J. Med. Chem. 51, 5101–5108.
Shen, H. C., Ding, F. X., Luell, S., Forrest, M. J., Carballo-Jane, E., Wu, K. K., Wu, T. J., Cheng, K., Wilsie, L. C., Krsmanovic, M. L., Taggart, A. K., Ren, N., Cai, T. Q., Deng, Q., Chen, Q., Wang, J., Wolff, M. S., Tong, X., Holt, T. G., Waters, M. G., Hammond, M. L., Tata, J. R., and Colletti, S. L. (2007). Discovery of biaryl anthranilides as full agonists for the high affinity niacin receptor. J. Med. Chem. 50, 6303–6306.
Shen, H. C., Taggart, A. K., Wilsie, L. C., Waters, M. G., Hammond, M. L., Tata, J. R., and Colletti, S. L. (2008). Discovery of pyrazolopyrimidines as the first class of allosteric agonists for the high affinity nicotinic acid receptor GPR109A. Bioorg. Med. Chem. Lett. 18, 4948–4951.
Skinner, P. J., Cherrier, M. C., Webb, P. J., Sage, C. R., Dang, H. T., Pride, C. C., Chen, R., Tamura, S. Y., Richman, J. G., Connolly, D. T., and Semple, G. (2007). 3-Nitro-4-amino benzoic acids and 6-amino nicotinic acids are highly selective agonists of GPR109b. Bioorg. Med. Chem. Lett. 17, 6619–6622.
Skinner, P. J., Webb, P. J., Sage, C. R., Dang, T. H., Pride, C. C., Chen, R., Tamura, S. Y., Richman, J. G., Connolly, D. T., and Semple, G. (2009). 5-N,N-Disubstituted 5-aminopyrazole-3-carboxylic acids are highly potent agonists of GPR109b. Bioorg. Med. Chem. Lett. 19, 4207–4209.
Soga, T., Kamohara, M., Takasaki, J., Matsumoto, S., Saito, T., Ohishi, T., Hiyama, H., Matsuo, A., Matsushime, H., and Furuichi, K. (2003). Molecular identification of nicotinic acid receptor. Biochem. Biophys. Res. Commun. 303, 364–369.
Stern, R. H., Spence, J. D., Freeman, D. J., and Parbtani, A. (1991). Tolerance to nicotinic acid flushing. Clin. Pharmacol. Ther. 50, 66–70.
Taggart, A. K., Kero, J., Gan, X., Cai, T. Q., Cheng, K., Ippolito, M., Ren, N., Kaplan, R., Wu, K., Wu, T. J., Jin, L., Liaw, C., Chen, R., Richman, J., Connolly, D., Offermanns, S., Wright, S. D., and Waters, M. G. (2005). (D)-beta-Hydroxybutyrate inhibits adipocyte lipolysis via the nicotinic acid receptor PUMA-G. J. Biol. Chem. 280, 26649–26652.
Tang, H., Lu, J. Y., Zheng, X., Yang, Y., and Reagan, J. D. (2008). The psoriasis drug monomethylfumarate is a potent nicotinic acid receptor agonist. Biochem. Biophys. Res. Commun. 375, 562–565.
Taylor, A. J., Sullenberger, L. E., Lee, H. J., Lee, J. K., and Grace, K. A. (2004). Arterial biology for the investigation of the treatment effects of reducing cholesterol (ARBITER) 2: a double-blind, placebo-controlled study of extended-release niacin on atherosclerosis progression in secondary prevention patients treated with statins. Circulation 110, 3512–3517.
Taylor, A. J., Villines, T. C., Stanek, E. J., Devine, P. J., Griffen, L., Miller, M., Weissman, N. J., and Turco, M. (2009). Extended-release niacin or ezetimibe and carotid intima-media thickness. N. Engl. J. Med. 361, 2113–2122.
Thangaraju, M., Cresci, G. A., Liu, K., Ananth, S., Gnanaprakasam, J. P., Browning, D. D., Mellinger, J. D., Smith, S. B., Digby, G. J., Lambert, N. A., Prasad, P. D., and Ganapathy, V. (2009). GPR109A is a G-protein-coupled receptor for the bacterial fermentation product butyrate and functions as a tumor suppressor in colon. Cancer Res. 69, 2826–2832.
Trudeau, F., Bernier, S., de Glisezinski, I., Crampes, F., Dulac, F., and Riviere, D. (1999). Lack of antilipolytic effect of lactate in subcutaneous abdominal adipose tissue during exercise. J. Appl. Physiol. 86, 1800–1804.
Tunaru, S., Kero, J., Schaub, A., Wufka, C., Blaukat, A., Pfeffer, K., and Offermanns, S. (2003). PUMA-G and HM74 are receptors for nicotinic acid and mediate its anti-lipolytic effect. Nat. Med. 9, 352–355.
Tunaru, S., Lattig, J., Kero, J., Krause, G., and Offermanns, S. (2005). Characterization of determinants of ligand binding to the nicotinic acid receptor GPR109A (HM74A/PUMA-G). Mol. Pharmacol. 68, 1271–1280.
van Herk, T., Brussee, J., van den Nieuwendijk, A. M., van der Klein, P. A., AP, I. J., Stannek, C., Burmeister, A., and Lorenzen, A. (2003). Pyrazole derivatives as partial agonists for the nicotinic acid receptor. J. Med. Chem. 46, 3945–3951.
Venter, J. C., Adams, M. D., Myers, E. W., Li, P. W., Mural, R. J., Sutton, G. G., Smith, H. O., Yandell, M., Evans, C. A., Holt, R. A., Gocayne, J. D., Amanatides, P., Ballew, R. M., Huson, D. H., Wortman, J. R., Zhang, Q., Kodira, C. D., Zheng, X. H., Chen, L., Skupski, M., Subramanian, G., Thomas, P. D., Zhang, J., Gabor Miklos, G. L., Nelson, C., Broder, S., Clark, A. G., Nadeau, J., McKusick, V. A., Zinder, N., Levine, A. J., Roberts, R. J., Simon, M., Slayman, C., Hunkapiller, M., Bolanos, R., Delcher, A., Dew, I., Fasulo, D., Flanigan, M., Florea, L., Halpern, A., Hannenhalli, S., Kravitz, S., Levy, S., Mobarry, C., Reinert, K., Remington, K., Abu-Threideh, J., Beasley, E., Biddick, K., Bonazzi, V., Brandon, R., Cargill, M., Chandramouliswaran, I., Charlab, R., Chaturvedi, K., Deng, Z., Di Francesco, V., Dunn, P., Eilbeck, K., Evangelista, C., Gabrielian, A. E., Gan, W., Ge, W., Gong, F., Gu, Z., Guan, P., Heiman, T. J., Higgins, M. E., Ji, R. R., Ke, Z., Ketchum, K. A., Lai, Z., Lei, Y., Li, Z., Li, J., Liang, Y., Lin, X., Lu, F., Merkulov, G. V., Milshina, N., Moore, H. M., Naik, A. K., Narayan, V. A., Neelam, B., Nusskern, D., Rusch, D. B., Salzberg, S., Shao, W., Shue, B., Sun, J., Wang, Z., Wang, A., Wang, X., Wang, J., Wei, M., Wides, R., Xiao, C., Yan, C., Yao, A., Ye, J., Zhan, M., Zhang, W., Zhang, H., Zhao, Q., Zheng, L., Zhong, F., Zhong, W., Zhu, S., Zhao, S., Gilbert, D., Baumhueter, S., Spier, G., Carter, C., Cravchik, A., Woodage, T., Ali, F., An, H., Awe, A., Baldwin, D., Baden, H., Barnstead, M., Barrow, I., Beeson, K., Busam, D., Carver, A., Center, A., Cheng, M. L., Curry, L., Danaher, S., Davenport, L., Desilets, R., Dietz, S., Dodson, K., Doup, L., Ferriera, S., Garg, N., Gluecksmann, A., Hart, B., Haynes, J., Haynes, C., Heiner, C., Hladun, S., Hostin, D., Houck, J., Howland, T., Ibegwam, C., Johnson, J., Kalush, F., Kline, L., Koduru, S., Love, A., Mann, F., May, D., McCawley, S., McIntosh, T., McMullen, I., Moy, M., Moy, L., Murphy, B., Nelson, K., Pfannkoch, C., Pratts, E., Puri, V., Qureshi, H., Reardon, M., Rodriguez, R., Rogers, Y. H., Romblad, D., Ruhfel, B., Scott, R., Sitter, C., Smallwood, M., Stewart, E., Strong, R., Suh, E., Thomas, R., Tint, N. N., Tse, S., Vech, C., Wang, G., Wetter, J., Williams, S., Williams, M., Windsor, S., Winn-Deen, E., Wolfe, K., Zaveri, J., Zaveri, K., Abril, J. F., Guigó, R., Campbell, M. J., Sjolander, K. V., Karlak, B., Kejariwal, A., Mi, H., Lazareva, B., Hatton, T., Narechania, A., Diemer, K., Muruganujan, A., Guo, N., Sato, S., Bafna, V., Istrail, S., Lippert, R., Schwartz, R., Walenz, B., Yooseph, S., Allen, D., Basu, A., Baxendale, J., Blick, L., Caminha, M., Carnes-Stine, J., Caulk, P., Chiang, Y. H., Coyne, M., Dahlke, C., Mays, A., Dombroski, M., Donnelly, M., Ely, D., Esparham, S., Fosler, C., Gire, H., Glanowski, S., Glasser, K., Glodek, A., Gorokhov, M., Graham, K., Gropman, B., Harris, M., Heil, J., Henderson, S., Hoover, J., Jennings, D., Jordan, C., Jordan, J., Kasha, J., Kagan, L., Kraft, C., Levitsky, A., Lewis, M., Liu, X., Lopez, J., Ma, D., Majoros, W., McDaniel, J., Murphy, S., Newman, M., Nguyen, T., Nguyen, N., Nodell, M., Pan, S., Peck, J., Peterson, M., Rowe, W., Sanders, R., Scott, J., Simpson, M., Smith, T., Sprague, A., Stockwell, T., Turner, R., Venter, E., Wang, M., Wen, M., Wu, D., Wu, M., Xia, A., Zandieh, A., and Zhu, X. (2001). The sequence of the human genome. Science 291, 1304–1351.
Walters, R. W., Shukla, A. K., Kovacs, J. J., Violin, J. D., DeWire, S. M., Lam, C. M., Chen, J. R., Muehlbauer, M. J., Whalen, E. J., and Lefkowitz, R. J. (2009). beta-Arrestin1 mediates nicotinic acid-induced flushing, but not its antilipolytic effect, in mice. J. Clin. Invest. 119, 1312–1321.
Wang, J., Wu, X., Simonavicius, N., Tian, H., and Ling, L. (2006). Medium-chain fatty acids as ligands for orphan G protein-coupled receptor GPR84. J. Biol. Chem. 281, 34457–34464.
Wise, A., Foord, S. M., Fraser, N. J., Barnes, A. A., Elshourbagy, N., Eilert, M., Ignar, D. M., Murdock, P. R., Steplewski, K., Green, A., Brown, A. J., Dowell, S. J., Szekeres, P. G., Hassall, D. G., Marshall, F. H., Wilson, S., and Pike, N. B. (2003). Molecular identification of high and low affinity receptors for nicotinic acid. J. Biol. Chem. 278, 9869–9874.
Wise, A., Jupe, S. C., and Rees, S. (2004). The identification of ligands at orphan G-protein coupled receptors. Annu. Rev. Pharmacol. Toxicol. 44, 43–66.
Yousefi, S., Cooper, P. R., Mueck, B., Potter, S. L., and Jarai, G. (2000). cDNA representational difference analysis of human neutrophils stimulated by GM-CSF. Biochem. Biophys. Res. Commun. 277, 401–409.
Keywords: hydroxy-carboxylic acid receptors, GPR81, GPR109A, GPR109B, deorphanization, G protein-coupled receptors, nicotinic acid, lactate, 3-hydroxy-octanoic acid
Citation: Ahmed K (2011) Biological roles and therapeutic potential of hydroxy-carboxylic acid receptors. Front. Endocrin. 2:51. doi: 10.3389/fendo.2011.00051
Received: 29 July 2011;
Paper pending published: 19 August 2011;
Accepted: 23 September 2011;
Published online: 25 October 2011.
Edited by:
Nicola J. Smith, Victor Chang Cardiac Research Institute, AustraliaReviewed by:
Daniel James Scott, University of Zurich, SwitzerlandRamasamy Paulmurugan, Stanford University, USA
Sanam Mustafa, University of Western Australia, Australia
Copyright: © 2011 Ahmed. This is an open-access article subject to a non-exclusive license between the authors and Frontiers Media SA, which permits use, distribution and reproduction in other forums, provided the original authors and source are credited and other Frontiers conditions are complied with.
*Correspondence: Kashan Ahmed, Institute of Molecular Systems Biology, Swiss Federal Institute of Technology Zurich, Wolfgang-Pauli-Strasse 16, CH-8093 Zurich, Switzerland. e-mail: ahmed@imsb.biol.ethz.ch