- 1Muping Coastal Environmental Research Station, Yantai Institute of Coastal Zone Research, Chinese Academy of Sciences, Yantai, China
- 2Research and Development Center for Efficient Utilization of Coastal Bioresources, Yantai Institute of Coastal Zone Research, Chinese Academy of Sciences, Yantai, China
- 3University of Chinese Academy of Sciences, Beijing, China
Negative physiological impacts induced by exposure to acidified seawater might sensitize marine organisms to future environmental stressors, such as disease outbreak. The goal of this study was to evaluate if ocean acidification (OA) could reduce the resistance capability of the Pacific oyster (Crassostrea gigas) to Vibrio splendidus challenge from an energy metabolism perspective. In this study, the Pacific oyster was exposed to OA (pH 7.6) for 28 days and then challenged by V. splendidus for another 72 h. Antioxidative responses, lipid peroxidation, metabolic (energy sensors, aerobic metabolism, and anaerobic metabolism) gene expression, glycolytic enzyme activity, and the content of energy reserves (glycogen and protein) were investigated to evaluate the environmental risk of pathogen infection under the condition of OA. Our results demonstrated that following the exposure to seawater acidification, oysters exhibited an energy modulation with slight inhibition of aerobic energy metabolism, stimulation of anaerobic metabolism, and increased glycolytic enzyme activity. However, the energy modulation ability and antioxidative regulation of oysters exposed to seawater acidification may be overwhelmed by a subsequent pathogen challenge, resulting in increased oxidative damage, decreased aerobic metabolism, stimulated anaerobic metabolism, and decreased energy reserves. Overall, although anaerobic metabolism was initiated to partially compensate for inhibited aerobic energy metabolism, increased oxidative damage combined with depleted energy reserves suggested that oysters were in an unsustainable bioenergetic state and were thereby incapable of supporting long-term population viability under conditions of seawater acidification and a pathogen challenge from V. splendidus.
Introduction
Increased atmospheric CO2 concentrations caused by anthropogenic activities have and will continue to contribute to ocean acidification (OA), as about 30% of CO2 is absorbed by the oceans (Sabine et al., 2004). The pH of surface seawater has already declined approximately 0.1 pH units compared with pre-industrial levels (Intergovernmental Panel on Climate Change, 2014). With further increases of atmospheric CO2 partial pressure (pCO2), surface ocean pH is predicted to decline by another 0.3–0.4 pH units by the end of this century (Caldeira and Wickett, 2005). Due to biogeochemical and biochemical processes, such as eutrophication, anthropogenic sulfur and nitrogen deposition, and the existence of a mid-salinity minimum buffer zone, pH in coastal and estuary areas is more variable than that in the open ocean (Doney et al., 2007; Cai et al., 2011; Hu and Cai, 2013).
Numerous studies have indicated that coastal ecosystems are under more serious threat from OA combined with other environmental stressors, including increased ocean temperature, pollution, and pathogenic challenge (Chen et al., 2015; Queirós et al., 2015; Gunderson et al., 2016; Moreira et al., 2016; Duckworth et al., 2017). Seawater acidification has been found to negatively affect physiological processes in mollusks such as calcification, respiration, filtration, acid–base regulation, and immune responses (Pörtner et al., 2004; Gazeau et al., 2013; Sui et al., 2015; Liu et al., 2016; Castillo et al., 2017; Zhao et al., 2017), which could lead to severe threats for their energy budget (Wang et al., 2015). As a consequence, the negative impact posed by acidified seawater might sensitize marine organisms to future environmental perturbations, such as pathogenic infection (Hooper et al., 2007; Ellis et al., 2015). However, few studies have investigated the integrated environmental impacts of OA and pathogen infection on marine organisms. Vibriosis, one of the major bacterial diseases affecting fish, bivalves, and crustaceans, is caused by pathogenic Vibrio species and has posed a severe challenge to marine organisms inhabiting coastal and estuary areas (Beaz-Hidalgo et al., 2010). It is therefore urgently needed to investigate the environmental and ecological future of coastal and estuarine regions under acidified seawater and pathogenic Vibrio infection.
Marine bivalves are globally recognized as valuable species from ecological and economic perspectives (Dumbauld et al., 2009). The Pacific oyster, Crassostrea gigas, is an important aquaculture bivalve species inhabiting estuaries and intertidal regions, where they are constantly confronted with various environmental perturbations. As a result, oysters are believed to be highly tolerant to multiple environmental stressors (Dineshram et al., 2016). However, some strains of Vibrio splendidus have been associated with summer mortality of juvenile oysters C. gigas (Lacoste et al., 2001; Gay et al., 2004). In addition, recent studies have found that OA could lead to immune suppression of marine invertebrates, especially marine bivalves, which could sensitize these species to pathogen infection (Hernroth et al., 2011; Ivanina et al., 2014; Liu et al., 2016; Wang et al., 2016).
Previous studies have shown that both OA and pathogenic infection could induce disturbances in energy metabolism and oxidative stress in marine organisms (Canesi et al., 2010; Ellis, 2013; Liu et al., 2013; Ellis et al., 2014; Lesser, 2016). The bioenergetics sustainability under environmental perturbations is linked directly to an organism’s fitness and has thus population-level consequences (Sokolova et al., 2012). Our proteomic study has also found that energy metabolism disturbance was correlated with oyster immunosuppression in response to seawater acidification and pathogens challenge (Cao et al., 2018). As a follow-up study, antioxidant enzyme activities (CAT, SOD, and GPx) and lipid peroxidation (LPO) were investigated in C. gigas. Moreover, the expression responses of genes related to energy sensors (Axin1, AMPKβ, and SIRT2), aerobic metabolism [TCA cycle enzymes, electron transport chain (ETC) components, and ATP turnover], and anaerobic metabolism (D-LDH X1 and D-LDH X2) combined with energy reserve content (GLY and PROT) were also investigated to assess the energy expenditure response, and further elucidated whether the altered oyster energy metabolism strategy was long-term sustainable in response to OA and/or V. splendidus.
Materials and Methods
Ocean Acidification Perturbation Experiment
Adult Pacific oysters (8–11 cm in shell length) were collected from a local farm from Muping, Yantai, and Shandong Provinces of China (37°38′N and 121°59′E; pH 8.10 ± 0.03) in December 2016, and acclimated in the laboratory for 2 weeks before commencement of the experiment. During the acclimation period, the organisms were maintained at a temperature of 17.3 ± 0.2°C and pH 8.14 ± 0.02 in aerated seawater (salinity 31.2 ± 0.5). After the preliminary acclimation, oysters were exposed for four weeks to two levels of seawater pH (8.1 or 7.6), followed by a 72 h challenge treatments (non-injection, filter-sterilized seawater-injection, or V. splendidus-injection), for a total of six treatments. The selected pH levels were representative of the present-day condition (pH 8.1) and the predicted condition by the moderate scenarios of Forster et al. (2007) for the year 2250 (pH 7.6). For each treatment, 3 aquaria were used with 20 organisms per aquarium (60 individuals per treatment). The control seawater was bubbled with the atmospheric air. Seawater bubbled with air–CO2 mixtures was adjusted through an air and CO2 gas flow adjustment system and set as the elevated pCO2 treatment (pH 7.6). Oysters were fed twice daily with a commercial algal blend (containing Chlorella vulgaris Beij and Phaeodactylum tricornutum).
During the exposure period, seawater was renewed every other day using pre-equilibrated seawater. No oyster mortality was observed during OA exposure. The pH of each container was monitored daily with a pH electrode (pH meter PB-10, Sartorius Instruments, Germany) calibrated with NBS standard pH solution. Salinity, temperature, and dissolved oxygen (DO) were determined daily by using an YSI meter (YSI® model 85, Yellow Springs, OH, United States). Water samples were collected from each tank every week to determine total alkalinity (TA) by potentiometric titration (Gran, 1952). Other related parameters of the carbonate chemistry were calculated according to known values of pH and TA levels using the software CO2SYS with the constants for seawater pH from Millero et al. (2006) and for KSO4-, from Dickson (1990). Seawater carbonate chemistry parameters of all treatments are presented in Supplementary Table S1.
Bacterial Challenge Experiment
Vibrio splendidus was cultured in 2216E medium at 28°C overnight, and centrifuged at 4000 g for 10 min at 25°C. Then the pellet was re-suspended in filter-sterilized (0.22 μm pore size) seawater (FSSW) and adjusted to a final concentration of 5 × 107CFU mL−1.
After 28 days of OA exposure, either 100 μL of FSSW (fssw-inj group) or 100 μL of V. splendidus suspension (5 × 106 CFU per oyster, Vibrio-inj group) was injected into the adductor muscle of oysters. Oysters without injection (non-inj group) were also sampled after OA exposure. After processing, the animals from each treatment were returned to respective aquariums for another 72 h. No oyster mortality was found during the infection period.
Sampling Procedure and Biochemical Analysis
At the end of the exposure period, six replicates of digestive glands and gills were sampled in each treatment. The tissues were immediately frozen in liquid nitrogen and stored at −80 °C until used for biochemical analyses. Meanwhile, six replicates of digestive glands were carefully excised and stored in RNAlater solution (Ambion, Austin, TX, United States) for subsequent RNA extraction.
Antioxidant Enzyme and Glycolytic Enzyme Activity Assay
Oyster gills were homogenized in phosphate buffer (50 mM potassium dihydrogen phosphate; 50 mM potassium phosphate dibasic; 1 mM EDTA; pH 7.0) and centrifuged at 10,000 g for 20 min at 4°C to obtain the supernatants. The resultant supernatants were subjected to antioxidant enzymes assays and LPO determination. Catalase (CAT) activity was determined according to the modified method of Vega-López et al. (2007), following the protocol proposed by Radi et al. (1991). This method evaluated the alteration in absorbance resulting from hydrogen peroxide (H2O2) degradation at 240 nm, with enzyme activity expressed as U mg−1 of PROT. Superoxide dismutase (SOD) activity was quantified using the method proposed by Beauchamp and Fridovich (1971), with some modifications adapted by Carregosa et al. (2014). One unit of SOD activity was defined as the amount of enzyme that caused 50% inhibition in the rate of nitroblue tetrazolium (NBT) chloride reduction and was expressed as U mg−1 of PROT. Glutathione peroxidase (GPx) activity was measured as described by Lawrence and Burk (1976). The method assessed the decrement in nicotinamide adenine dinucleotide phosphate (NADPH) concentration in an assay coupled to glutathione reductase (GR) that catalyzed NADPH oxidation at 340 nm. The results were expressed as U mg−1 of PROT. LPO levels were quantified by measuring the malondialdehyde (MDA) content, according to the method previously described by Ohkawa et al. (1979). MDA concentrations were measured spectrophotometrically at OD 532 nm and the results were expressed as nmol MDA mg−1 of PROT.
Enzymatic activities of HK and PK were determined in the digestive glands of OA and/or V. splendidus-exposed oysters. The activities of HK and PK were determined as described by Greenway and Storey (1999) and the results were expressed as U g−1 of PROT.
Energy Reserves
Glycogen content was measured by using the phenol-sulfuric acid method described by Yoshikawa (1959). A calibration curve was obtained by using glucose standards. PROT content was quantified following the Biuret method (Robinson and Hogden, 1940). Bovine serum albumin (BSA) was used as the standard material. The GLY and the PROT content was expressed as mg per g FW (fresh weight).
RT-PCR Analysis
The mRNA expression levels of eight genes related to energy metabolism (ACN, IDH, SDH, SCS, COX 1, COX, AK, ATP synthase subunit alpha Axin1, AMPKβ, SIRT2, D-LDH X1, and D-LDH X2) and two immune related genes (Integrin beta-1B and TNF) were determined in OA and/or V. splendidus exposed treatments. Total RNA was extracted with TRIzol reagent (Invitrogen, United States) following the manufacturer’s instructions. DNase I (Promega, United States) treated RNA was used as a template for cDNA synthesis. A standard protocol was undertaken to perform quantitative real-time PCR program by using an Applied Biosystems 7500 fast Real-Time PCR System (Applied Biosystems, United States). The primers designed for quantitative RT-PCR are presented in Supplementary Table S3. A dissociation curve analysis of amplification products was performed at the end of each PCR to confirm that only one PCR product was amplified and detected. Expression of target genes was normalized with the elongation factor 1-alpha reference gene (de Lorgeril et al., 2011), which was stable in the current study (p > 0.05, coefficient of variation < 5%). The comparative 2-ΔΔCT method was used to analyze the expression level of the selected genes (Livak and Schmittgen, 2001).
Integrated Biomarker Response (IBR)
The “Integrated Biomarker Response version 2” (IBRv2) index was utilized to integrate multiple biochemical parameters into one general index representing the environmental stress level of different treatments (Sanchez et al., 2013). To calculate the IBRv2 values, individual biomarker data (Xi) were compared to reference data (X0) and log transformed to reduce variance: Yi = log Xi/X0. The general mean (μ) and SD of each biomarker Yi were computed for all treatments; Yi was standardized as Zi = (Yi-μ)/s. Biomarker deviation index (A) was calculated using the mean of the standardized biomarker response (Zi) and mean of reference biomarker data (Z0): Ai = Zi-Z0. For a single treatment, the biomarker deviation index (A) was presented in star plots, representing the deviation of each investigated biomarker in OA and/or V. splendidus exposed groups, compared to the control group. The area above 0 reflected biomarker induction, and the area below 0 indicated a biomarker inhibition. In addition, the IBRv2 was calculated for each biomarker in each treatment: IBRv2 = Σ|A|.
Statistical Analysis
All measured biochemical parameters were presented as the mean ± SD. The raw data were assessed for normality and homogeneity of variances using the Shapiro–Wilk test and Bartlett’s test, respectively. A two-way ANOVA followed by Fisher’s least significant difference (LSD) post hoc analyses was performed on all data to test for significant differences (p < 0.05) between treatments. The analyses were carried out using SPSS version 23.0 (SPSS Inc., Chicago, IL, United States). ANOVA results for all studied parameters are displayed in Supplementary Tables S4, S5. Principal component analysis (PCA) was performed with CANOCO 5.0 software (Microcomputer Power Inc., United States) using gene expression data from OA and V. splendidus exposed oysters to assess the variability associated with energy metabolism and immune responses.
Results
Antioxidant Enzyme Responses and Oxidative Damage
There was no significant change in any of the physiological parameters measured in fssw-inj-treated oysters compared to non-inj-treated oysters (Supplementary Table S2). As a result, the oysters treated with fssw-injection were set as the control in the present study. The antioxidant enzyme responses and oxidative damage under OA and/or Vibrio exposure are shown in Figure 1. CAT activity increased significantly in oysters treated with Vibrio at pH 7.6 (p < 0.05, Figure 1A). However, no significant changes in CAT activity were observed in oysters exposed to either OA or Vibrio alone. Similarly, there was no significant change in SOD activity among all four treatments (Control, Vibrio, OA, and Vibrio-OA groups; Figure 1B and Supplementary Table S4). Regarding GPx activity, significant inhibition effect was observed in oysters under combined OA and Vibrio exposure (p < 0.05, Figure 1C). Oysters showed significantly higher LPO levels in response to Vibrio challenge, either alone or combined with OA (Figure 1D). However, oysters exposed only to OA showed higher but not significantly different LPO levels compared to the control individuals (Figure 1D and Supplementary Table S4).
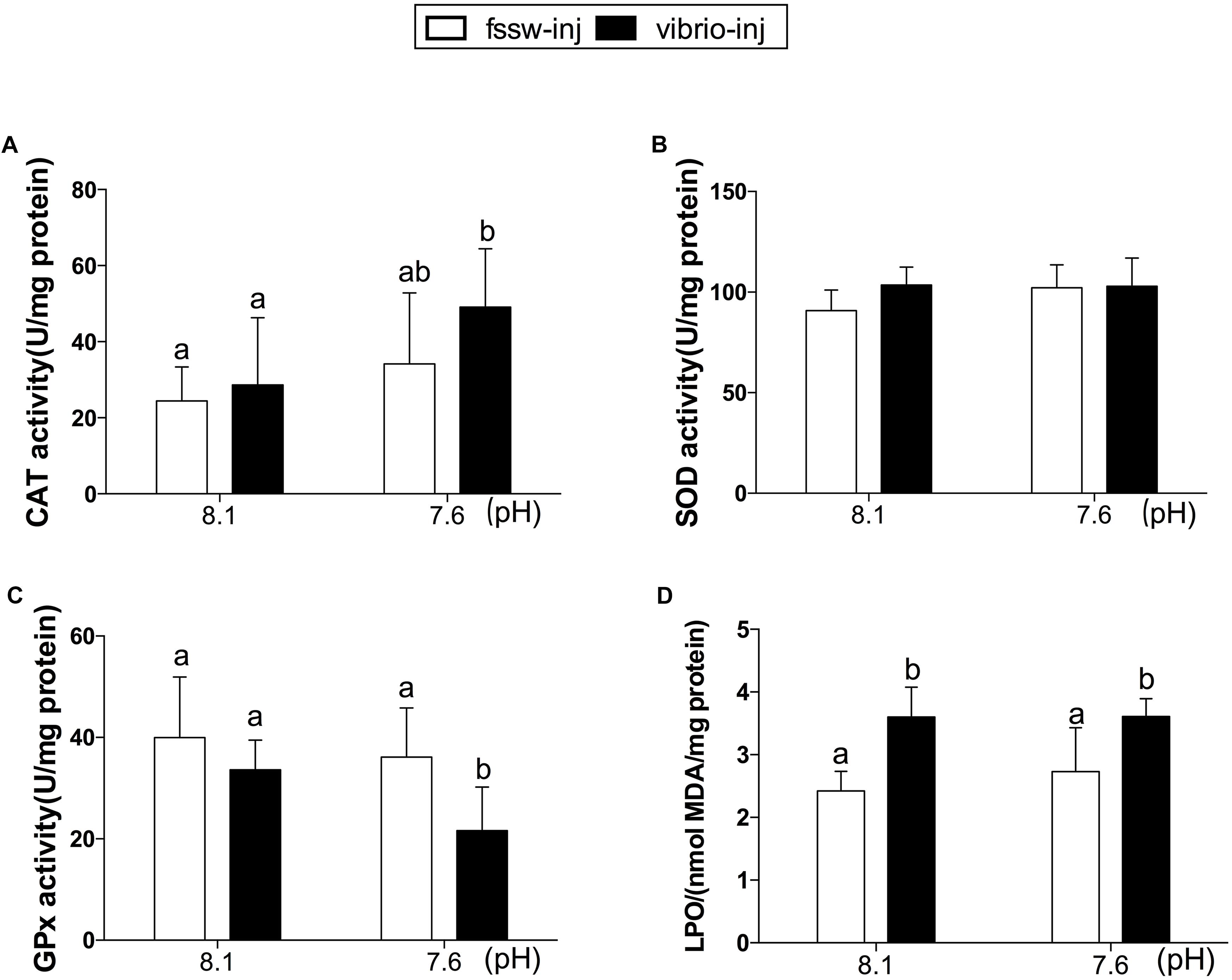
FIGURE 1. Antioxidant enzyme activities and LPO levels in the gills of C. gigas post elevated pCO2 and/or V. splendidus exposure. (A) CAT. (B) SOD. (C) GPx. (D) LPO. Each bar represents mean ± SD (n = 6). Different letters indicate significant differences among different treatments (p < 0.05).
Glycolytic Enzyme Activities and Energy Reserves
No significant difference in HK activity was found among individuals in the four treatments (Figure 2A). However, the enzyme activity of PK was significantly increased in OA-treated oysters, regardless of Vibrio challenge (p < 0.01; Figure 2B and Supplementary Table S4). Further, as a major energy reserve, GLY content was significantly decreased in response to the combined exposure of OA and V. splendidus compared to other treatments (p < 0.05; Figure 2C and Supplementary Table S4). No significant change in GLY content was found in the digestive glands of oysters treated with either OA or Vibrio. However, PROT content was significantly lower in Vibrio and Vibrio-OA treatments compared to the control group (Figure 2D), while no apparent change was found in OA-treated oysters compared with the other three treatments.
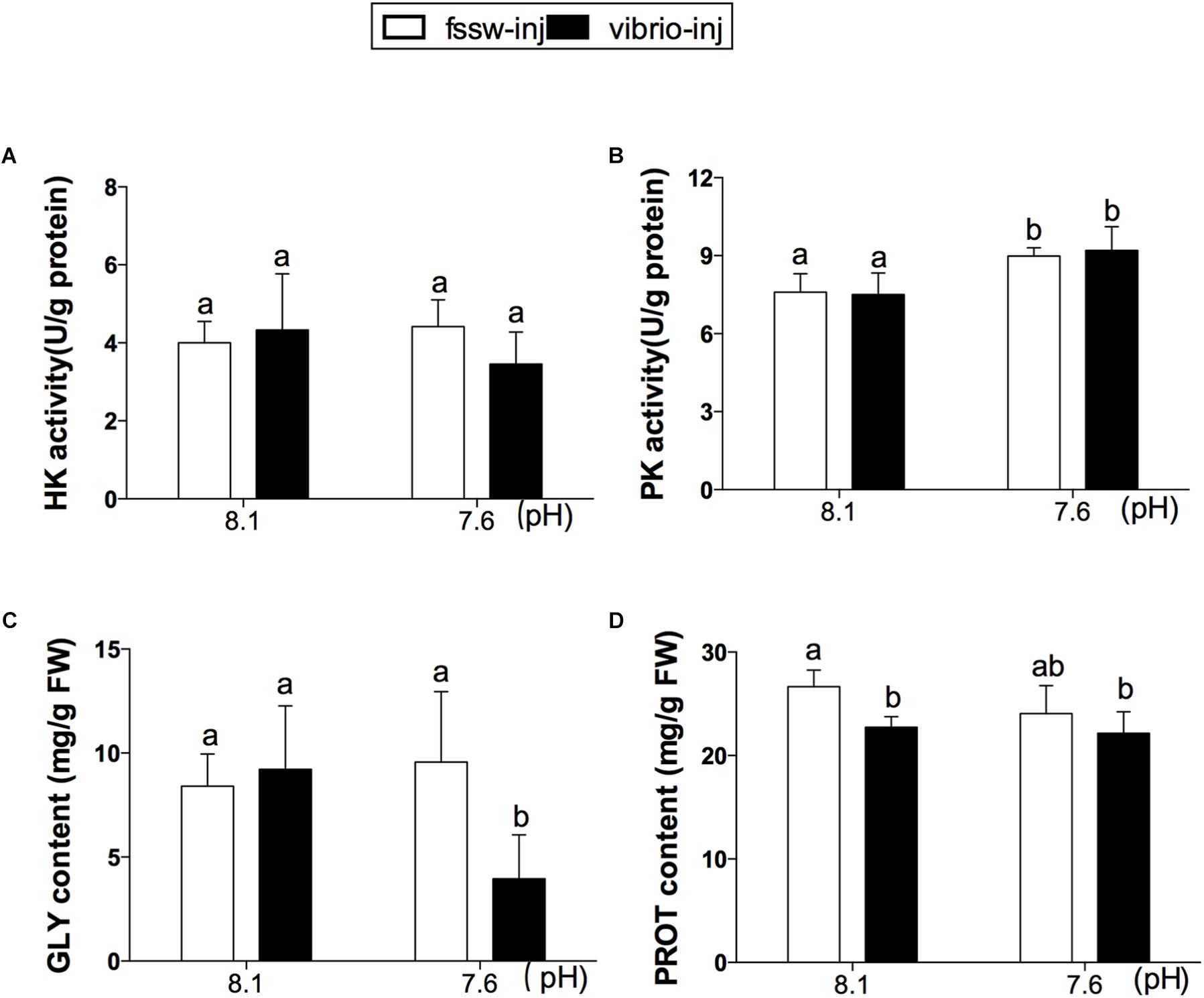
FIGURE 2. Glycolytic enzyme activities and energy reserves in the digestive glands of C. gigas post elevated pCO2 and/or V. splendidus exposure. (A) HK activity. (B) PK activity. (C) GLY content. (D) PROT content. Each bar represents mean ± SD (n = 6). Different letters indicate significant differences among different treatments (p < 0.05).
Expression of Genes Related to Energy Metabolism and Immune Responses
The expression levels of genes related to aerobic metabolism, including the TCA cycle (ACN, IDH, SDH, and SCS), mitochondria electron transfer chain components (COX1 and COX), and ATP metabolism (ATP synthase subunit alpha and AK), and two immune factors (TNF and integrin beta-1B), were investigated in oysters under exposure of OA and/or Vibrio (Figure 3). The mRNA expression of TCA cycle-related genes (ACN, IDH, SDH, and SCS) was significantly inhibited under Vibrio challenge (Figures 3A–D and Supplementary Table S5). The expression level of two TCA cycle enzyme (SDH and SCS) transcripts was significantly inhibited under OA exposure without Vibrio challenge compared to the control individuals (Figures 3C,D). To the contrary, the mRNA expression of ATP metabolism related genes (ATP synthase and AK) were significantly stimulated under OA challenge without Vibrio infection compared to other treatments (Figures 3G,H).
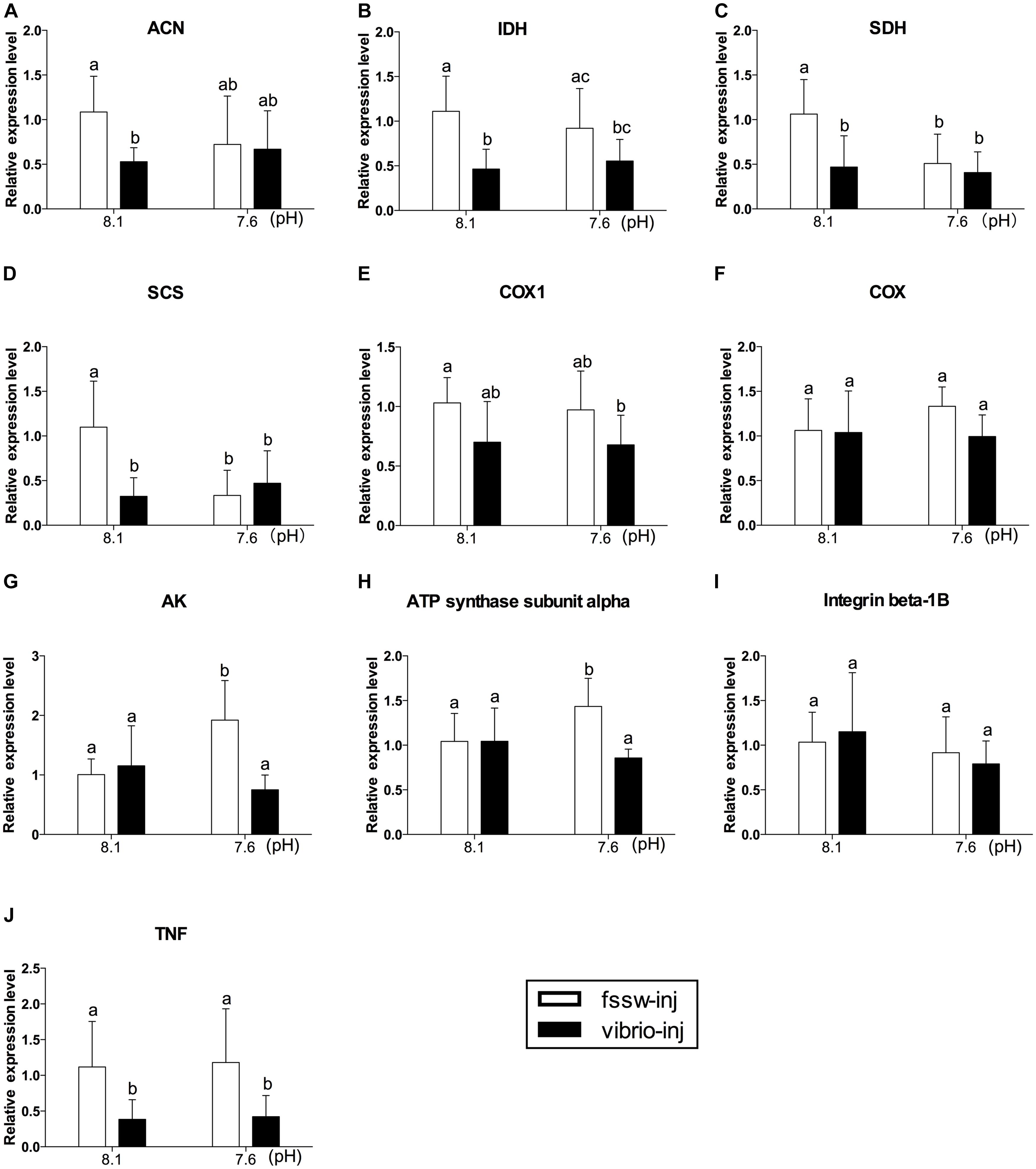
FIGURE 3. The mRNA expression profiles of aerobic energy metabolism- and immune-related genes in the digestive glands of oysters post elevated pCO2 and V. splendidus exposure. (A) ACN. (B) IDH. (C) SDH. (D) SCS. (E) COX 1. (F) COX. (G) AK. (H) ATP synthase subunit alpha. (I) Integrin beta-1B. (J) TNF. Each bar represents mean ± SD (n = 6). Different letters indicate significant differences among treatments (p < 0.05), and identical letters indicate no significant difference.
The expression pattern of all genes tested in Vibrio-OA-treated oysters was similar to that of Vibrio-treated individuals. The expression level of most of the aerobic metabolism-related genes (IDH, SDH, SCS, and COX1) was depressed under combined exposure to OA and Vibrio, but OA individually did not elevate the inhibition effect (Figures 3A–E and Supplementary Table S5). ANOVA results also suggest that there is generally no significant interaction between OA and Vibrio challenges, with the exception of SCS, ATP synthase subunit alpha, and AK (Supplementary Table S5). The mRNA expression of ATP synthase subunit alpha and AK transcripts was significantly decreased in oysters under Vibrio-OA exposure compared to OA treatment, suggesting an antagonistic relationship between Vibrio and OA on the expression of these two genes (Figures 3G,H). The expression level of integrin beta-1B transcripts was not altered in response to OA and Vibrio challenges, individually or in combination (Figure 3I). Vibrio challenge significantly inhibited the expression of TNF transcripts, regardless of OA exposure (Figure 3J), while no significant change was observed in oysters under OA exposure alone.
The expression responses of genes related to metabolic sensors (Axin1, AMPKβ, and SIRT2) and anaerobic glycolysis (D-LDH X1 and D-LDH X2) were also investigated. Our results showed significantly elevated expression of all genes (Axin1, AMPKβ, SIRT2, D-LDH X1, and D-LDH X2) investigated under exposure to Vibrio and OA (Figures 4A–E and Supplementary Table S5). A significant stimulation effect was observed in the expression of AMPKβ transcripts of Vibrio-treated oysters (Figure 4B). In oysters treated with OA alone, the expression of all genes (AMPKβ, SIRT2, D-LDH X1, and D-LDH X2), with the exception of Axin1, was significantly elevated compared to the control group (Figures 4B–E). However, a significant interaction between OA and Vibrio was observed only in the expression of Axin1 transcripts (Supplementary Table S5).
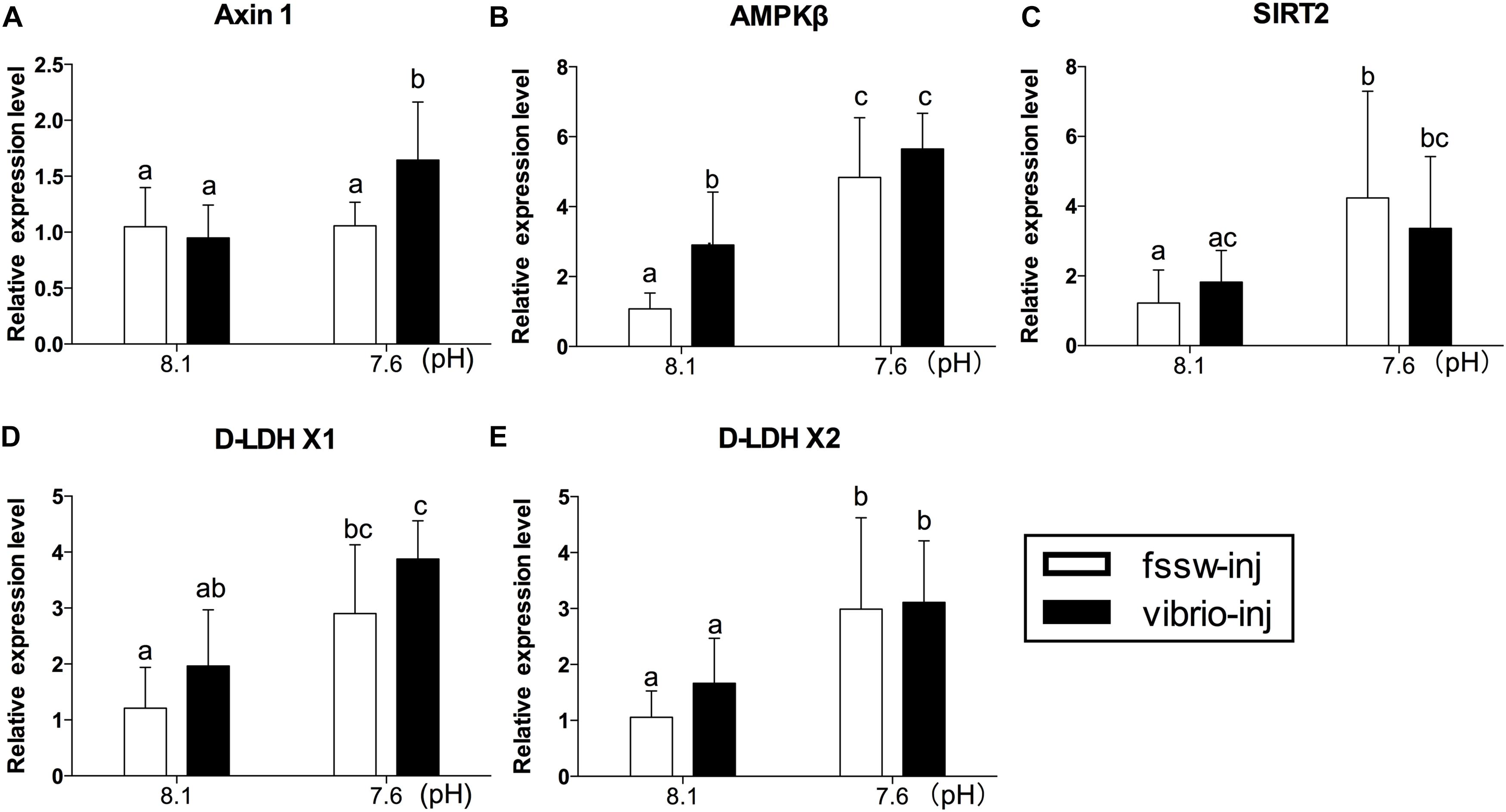
FIGURE 4. The mRNA expression profiles of anaerobic energy metabolism- and energy sensing-related genes in the digestive glands of oysters post elevated pCO2 and V. splendidus exposure. (A) Axin1. (B) AMPKβ. (C) SIRT2. (D) D-LDH X1. (E) D-LDH X2. Each bar represents mean ± SD (n = 6). Different letters indicate significant differences among treatments (p < 0.05), and identical letters indicate no significant difference.
Principal Component Analysis (PCA)
Principal component analysis was applied to all expression abundance data of aerobic energy metabolism-related genes and two immune genes from digestive glands (Figure 5A) and revealed 59.99% of the total variance. PC1 represented 40.53% of variance, highlighting the separation between non- and Vibrio exposed oysters. The second component (explaining only 19.46% of the variance) identified the separation of non-OA and OA exposure treatments without Vibrio challenge. Overall, PCA analysis provided further validation for the clustering of aerobic energy metabolism-related genes on the negative side of PC 1. Furthermore, PC 1 showed the clustering of Vibrio treatments in opposition to the expression elevation of all measured aerobic metabolism-related genes.
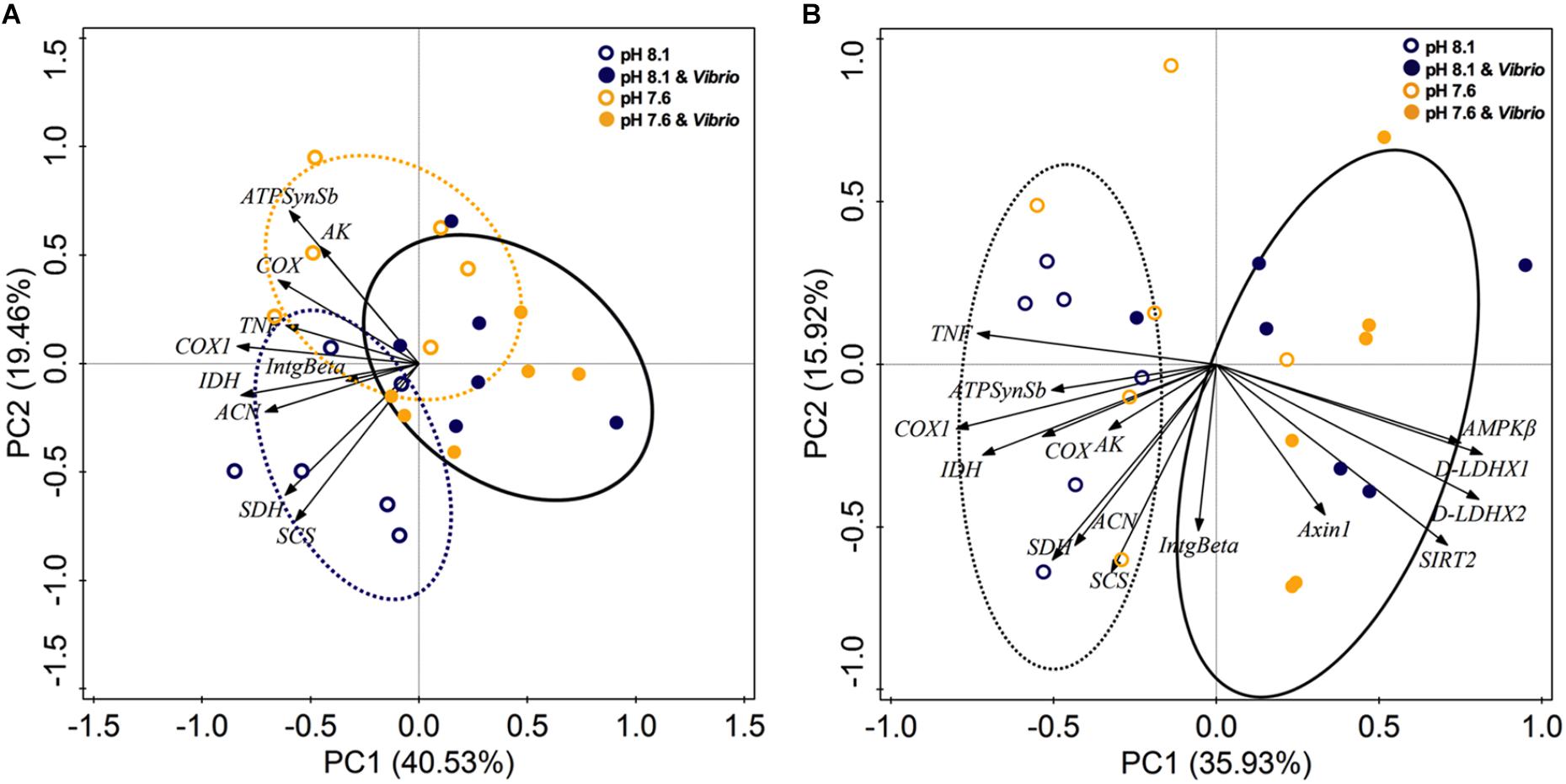
FIGURE 5. (A) Principle component analysis (PCA) ordination biplot of ocean acidification and/or V. splendidus treatments for C. gigas, using expression data of genes related to aerobic metabolism and immune responses. (B) PCA ordination biplot of ocean acidification and/or V. splendidus treatments for C. gigas, using expression data of genes related to energy metabolism and immune responses.
Additionally, PCA clustering of expression abundance data of energy metabolism-related (metabolic sensors, aerobic metabolism, and anaerobic metabolism) genes and two immune genes showed a total 51.85% variance (Figure 5B). PC1 expressed 35.93% variance, revealing separation between non- and Vibrio- exposed treatments. Vibrio and Vibrio-OA treatments correlated positively with metabolic sensor- and anaerobic metabolism-related genes and correlated negatively with aerobic metabolism-related genes. These results clearly demonstrated that in response to Vibrio challenge, either alone or combined with OA, oysters exhibited suppressed aerobic metabolism and stimulated aerobic metabolism.
Integrated Biomarker Response (IBR)
The IBR star plots present transformed data for each measured biochemical parameter (antioxidant enzymes and oxidative damage, glycolytic enzymes, and energy reserves) in oysters exposed to an OA and Vibrio challenge (Figure 6A). Star plots revealed that most of the parameters (CAT, GPx, HK, PROT, and GLY) showed higher response in oysters exposed to both Vibrio and OA than single stressor-treated individuals. The higher IBR index in oysters treated with Vibrio-OA, compared to single stressor-treated individuals, suggested that oysters were under severe stress in response to the combined exposure of Vibrio and OA. The integrated biomarker response (IBR) values in each treatment are shown in Figure 6B. The IBR index was similar between the OA and Vibrio treatments, while the IBR index was exceedingly high in Vibrio-OA treatment compared to either the OA or Vibrio treatments alone. According to the IBR index, the rank order of treatments was: Vibrio-OA > Vibrio = OA.
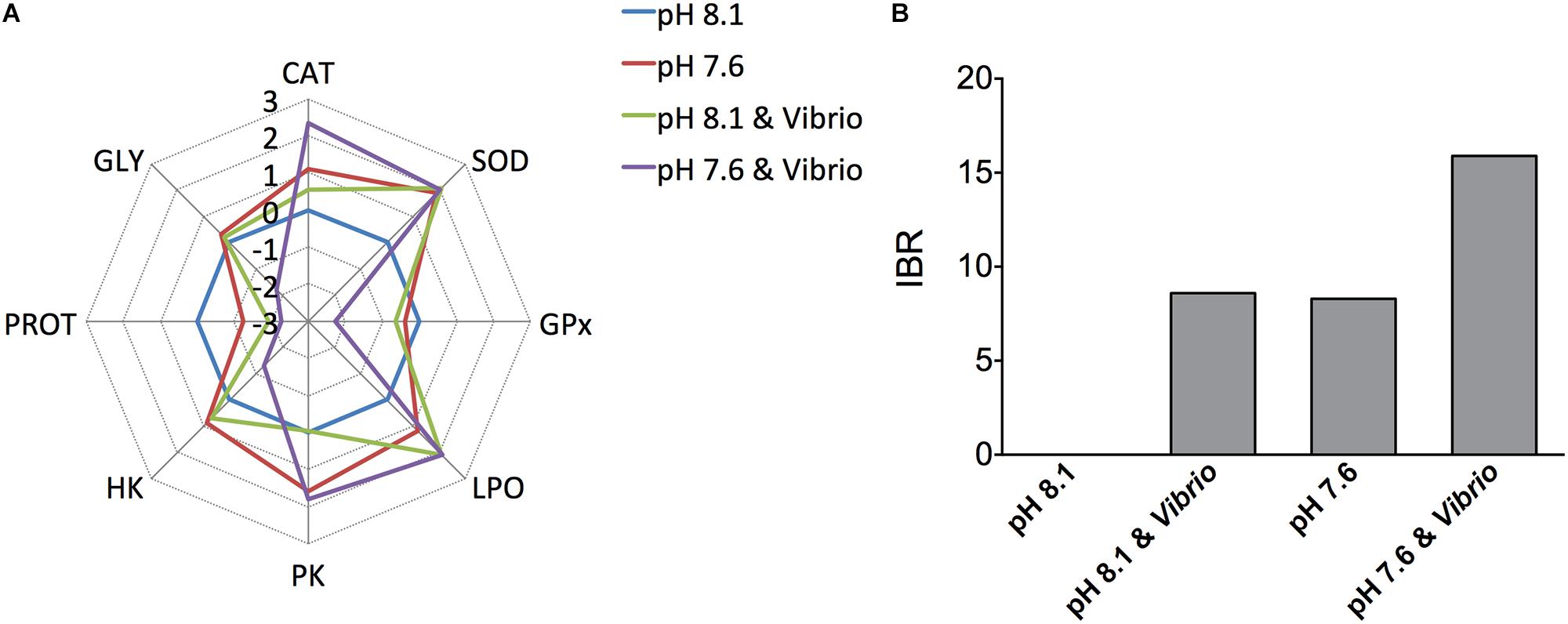
FIGURE 6. Biomarker star plots and integrated biomarker response (IBR). (A) Biomarker star plots for each treatment displaying the general patterns of data variability. SOD, superoxide dismutase; CAT, catalase; GPx, glutathione peroxidase; LPO, lipid peroxidation; PK, pyruvate kinase; HK, hexokinase; PROT, protein; GLY, glycogen. (B) Calculated IBR index using the biochemical parameters measured in C. gigas after exposure to ocean acidification and/or V. splendidus.
Discussion
Antioxidant Responses and Oxidative Damage
ROS are naturally produced and maintained at basal levels during cellular aerobic metabolism (Halliwell and Gutteridge, 1986). Numerous environmental stressors have been found to induce ROS production in marine invertebrates (Canesi et al., 2010; Tomanek et al., 2011; Zhu et al., 2011; Matoo et al., 2013; Ferreira et al., 2015). LPO, caused by excessive ROS production, is a major indicator of oxidative damage in organisms and a major contributor to the loss of cell function under environmental stress, including OA and pollutants (Moreira et al., 2016; Ricevuto et al., 2016; Valerio-García et al., 2017). In the present study, the LPO level increased significantly in Vibrio-treated oysters. Similarly, increased oxidative stress and altered antioxidant enzyme responses were observed in Mytilus galloprovincialis challenged with V. splendidus (Canesi et al., 2010). It has been shown that the adrenergic receptors and PKC-mediated pathways’ activation induced by bacterial components were associated with ROS production (Dailianis et al., 2005; Ciacci et al., 2010). Meanwhile, antioxidant enzymes such as CAT and SOD were shown to remove excessive ROS in organisms under severe stress caused by environmental perturbations (Giarratano et al., 2014). Although the LPO level increased significantly in V. splendidus challenged oysters, no significant change was observed in the antioxidant enzyme activities (CAT, SOD, and GPx) in this study. These results suggest that inefficient activation of antioxidant defenses in Vibrio-treated oysters resulted in higher oxidative damage in these individuals compared to those in the control group.
However, the present findings demonstrated that oysters were able to increase the activities of antioxidant enzymes, such as CAT, to counteract the oxidative stress caused by combined exposure to OA and Vibrio. In contrast, the activity of GPx decreased significantly in the Vibrio-OA treatment. The inhibited responses of the antioxidant enzymes possibly suggested a failure of the antioxidant system in scavenging ROS, and thus resulted in the accumulation of the oxidative substance in the cells (Li et al., 2012). Accordingly, LPO level was increased significantly in response to the combined exposure to OA and V. splendidus.
Oxidative stress has been suggested to be involved in the toxic mechanism of seawater acidification (Benedetti et al., 2016; Moreira et al., 2016; Velez et al., 2016a). For example, a proteomic study on eastern oysters, Crassostrea virginica, suggested an induced oxidative stress after a 2-week exposure to elevated CO2 concentration (∼357 Pa pCO2; Tomanek et al., 2011). However, our results revealed that OA did not stimulate antioxidant responses and oxidative damage in oysters, indicating a low oxidative stress level in OA-exposed oysters. Similar results have also been found in two bivalve species, C. virginica and Mercenaria mercenaria, in which no persistent oxidative stress signal was observed during long-term exposure to OA (∼800 ppm CO2; Matoo et al., 2013).
In general, although OA alone did not induce significant changes in antioxidant enzyme activities, the combined exposure to OA and Vibrio led to higher oxidative stress compared to that of each stressor alone. This outcome was demonstrated by significantly altered antioxidant enzyme activities (CAT and GPx) and was expected as both OA exposure and V. splendidus challenge could lead to increased ROS production in oysters (Canesi et al., 2010; Tomanek et al., 2011). Above all, the results from this study demonstrated that excessive ROS production in oysters exposed to Vibrio alone or combined with an OA challenge induced cellular stress and oxidative damage and contributed therefore to the pathogenesis of Vibriosis.
Aerobic Metabolism and Energy Reserves
Energy metabolism biomarkers were vital for evaluating cellular energy status under environmental perturbations, and could be used to predict ecological consequences of stress exposure (Dong and Zhang, 2015). Under normal conditions, ATP supply via aerobic metabolism was sufficiently high to cover the maintenance costs, as well as energy costs of physiological activity, growth, and reproduction. However, severe environmental stress could lead to depressed aerobic metabolism in invertebrates (Giacomin et al., 2014; Dahlke et al., 2016; Zhao et al., 2017). Hence, the expression levels of aerobic metabolism-related genes were investigated in this study. Specifically, the TCA cycle served as the most important phase in respiratory process, oxidizing acetate into carbon dioxide (Fernie et al., 2004). ACN, IDH, SDH, and SCS are essential enzymes that participate in the mitochondrial TCA cycle. COX1 and COX are components of the mitochondria ETC (Wikstrom, 1977). Two ATP metabolic genes, including ATP synthase subunit alpha and AK, were also investigated in the oyster C. gigas.
A growing body of evidence has suggested that OA could disrupt the energy balance of mollusks by impairing key processes associated with high energy expenditure, such as immunity, development, acid–base homeostasis maintenance, and antioxidant processes (Lannig et al., 2010). A disturbance in energy metabolism by OA could sensitize bivalves to other environmental perturbations such as pathogen infection (Ivanina and Sokolova, 2015; Hernroth et al., 2016). In this study, the suppressed expression of two genes (SDH and SCS) in OA-treated oysters suggested only a mild inhibition effect of OA on aerobic energy metabolism. The increased mRNA expression of ATP synthase subunit alpha and AK might be associated with increased ATP turnover rates in OA treatment, which suggested a partial energy compensation for stress response in OA-treated oysters. In addition, enhanced glycolysis, indicated by increased PK activity, was observed in OA-treated oysters, which may supply the energy needed for acid–base homeostasis maintenance and other stress responses in oysters under OA exposure. The two energy reserves (GLY and PROT) were not altered in oysters under OA exposure, suggesting the maintenance of energy homeostasis in this treatment. Above all, our results indicated that OA-exposed oysters might exploit increased glycolysis and ATP turnover to provide the energy needed for cellular homeostasis, thereby explaining the high resistance of this species to OA.
From our findings, V. splendidus challenge was the major factor affecting oyster energy metabolism. Aerobic energy metabolism was decreased in Vibrio and Vibrio-OA treatments, which was demonstrated by the significantly inhibited expression of most aerobic energy metabolic genes. PCA analysis further confirmed the inhibition effect on the expression of aerobic metabolism-related genes caused by Vibrio exposure, either alone or combined with OA (Figure 5A). Although aerobic metabolism was inhibited, decreased PROT content in oysters treated by Vibrio alone or combined with OA and decreased GLY levels in the Vibrio-OA treatment suggested a disturbed energy balance. In addition, PK catalyzes the final step of glycolysis, which plays an important role in GLY metabolism (Hemre et al., 2002). Increased PK activity found in the present study indicated enhanced levels of glycolysis in Vibrio-OA treatment. Similarly, GLY has been mobilized as a source of energy for cellular protection mechanisms under extreme salinities (Velez et al., 2016b).
Energy Sensors and Anaerobic Metabolism
Energy homeostasis in invertebrates could be maintained through cellular pathways involved in cellular energy status sensing and energy expenditure modulation (Han et al., 2013; Dong et al., 2014; Dong and Zhang, 2015; Jost et al., 2015). When cellular energy status was compromised, activation of AMPK was initiated by assembling the Axin-AMPK-serine-threonine liver kinase B1 (LKB1) complex (Zhang et al., 2013). Histone/PROT deacetylase (SIRT) served as another metabolic sensor in cells under low energy status. The activation of energy metabolism sensors including Axin, AMPK, and SIRT could switch on the catabolic pathways (primarily lipid and glucose catabolism), resulting in ATP generation while simultaneously shutting down ATP consuming anabolic pathways (Cantó et al., 2009; Houtkooper et al., 2012; Hardie et al., 2016). In this study, the observed significantly elevated expression levels of metabolic sensors (Axin1, AMPKβ, and SIRT2) in oysters exposed to OA and/or Vibrio indicated activated catabolism and inhibited anabolic metabolism. This result could further explain the increased glycolytic enzyme activities found in Vibrio- and/or OA-treated oysters, and depleted GLY level in oysters treated by Vibrio alone or combined with OA. Positive correlation between the expression of metabolic sensing genes (Axin, AMPK, and SIRT) and glycolysis-related genes has also been observed in limpets, Cellana toreuma (Han et al., 2013; Dong et al., 2014).
Furthermore, D-LDH has the ability to catalyze the NADH-dependent interconversion of pyruvate and D-lactate in anabolic and catabolic pathways (Simon et al., 1989). In the present study, increased expression of D-LDH X1 and D-LDH X2 observed in OA and/or Vibrio exposed oysters verified our hypothesis that enhanced anaerobic glycolysis was initiated to compensate for depressed energy supplies caused by inhibition of aerobic metabolism. PCA results also indicated that metabolic sensors (Axin1, AMPKβ, and SIRT2) and anaerobic metabolism (D-LDH X1 and D-LDH X2) were negatively correlated with aerobic metabolic genes in Vibrio-treated oysters, regardless of OA.
While the metabolic rate and oxygen consumption were not measured in the present study, previous studies showed that OA could lead to succinate accumulation in both oyster gills and hepatopancreas, as measured by NMR-base metabolomics (Lannig et al., 2010), suggesting partial anaerobiosis of oysters under OA exposure. Furthermore, decreased oxygen consumption and metabolic rate were found in OA-exposed mussel M. galloprovincialis (Michaelidis et al., 2005). Michaelidis et al. (2005) also found that respiratory acidosis in the extracellular fluids of mussels might be associated with the reduction in aerobic scope and aerobic metabolism. In the meantime, reduction in energy reserves within bacterial challenged bivalve species has been observed in numerous studies (Dittman et al., 2001; Paillard et al., 2004; Flye-Sainte-Marie et al., 2007). Correspondingly, OA posed milder suppression on the aerobic metabolism and led to partial anaerobiosis in oysters, while bacterial challenge alone or combined with OA could lead to suppressed aerobic metabolism, partial anaerobiosis, and decreased energy reserves in oyster C. gigas. In addition, severely depleted energy reserves of V. splendidus challenged oysters under decreased pH might be associated with higher energy cost for the acid–base hemostasis maintenance and antioxidant responses.
Correlation of Energy Metabolism With Oxidative and Immune Response
Generally, oysters could suppress aerobic energy metabolism to decrease ROS production. Additionally, the suppressed energy metabolism and milder depleted energy reserves in Vibrio- or OA-treated oysters might be associated with the unaltered antioxidant enzyme response in these two treatments, as energy was mobilized to more urgent cellular stress responses, such as acid–base homeostasis and immune responses. Similarly, previous research has identified increased acid–base homeostasis maintenance under OA (Wang et al., 2017) and stimulated oxidative stress and immune responses under V. splendidus challenge in invertebrates (Canesi et al., 2010; Liu et al., 2013; Li et al., 2017). According to our previous proteomic results, a tradeoff between oxidative stress and energy metabolism was adopted by oysters in Vibrio-OA treatment (Cao et al., 2018). The ETC in mitochondria is a major site of ROS production (Chandel and Schumacker, 2000). In the Vibrio-OA group, both decreased abundance of ETC-related PROT expression found in our previous research and decreased mRNA expression of ETC-related gene COX1 in this study might suggest that a tradeoff exists between oxidative stress and energy metabolism.
In addition, as an immune factor, TNF played important roles in the immune functions of oysters. In this study, immune responses in oysters were perhaps suppressed as indicated by the significantly decreased expression of TNF transcripts. The depressed immune function in Vibrio-treated oysters could be associated with the disturbed energy metabolism caused by this treatment. Previous finding also suggested that V. splendidus challenge could lead to immunosuppression of marine invertebrates (Lacoste et al., 2001; Duperthuy et al., 2010).
Integrated Biomarker Response
The IBR index provides a global view of environmental stress by combining various biomarker signals (Beliaeff and Burgeot, 2002). Numerous laboratory experiments have adopted IBR index to better review and compare results between treatments (Qu et al., 2014; Xu et al., 2016; Valerio-García et al., 2017; Wang et al., 2018). Higher IBR values indicated more stressful conditions as the IBR index was considered to be a general description of “health status” (Leiniö and Lehtonen, 2005). In the present study, oysters exposed to the combination of both stressors displayed a greatly increased IBR value compared to each stressor alone. These results demonstrated that co-exposure is the most stressful condition, which was in coordination with the results that co-exposure induced more severe responses of oxidative stress and depleted energy reserves.
Recently, an energy metabolism model has been proposed by Sokolova et al. (2012), in which transition to partial anaerobiosis and metabolic rate depression are characteristics of organisms on the pessimum range of energy metabolism. As the balance of ATP supply and demand is disrupted in the pessimum range of energy metabolism, populations are unable to survive. Although OA-exposed oysters could maintain energy metabolism homeostasis in this study, the disturbed energy metabolism (decreased aerobic metabolism and increased anaerobic metabolism) led to decreased energy reserves under pathogen infection alone or combined with OA exposure, which was energetically unsustainable in the long run. Furthermore, OA might reduce the ability of oysters to resist infection by the pathogen V. splendidus, as indicated by the higher IBR value of combined exposure compared to a single stressor. As a consequence, marine bivalves are under severe threat from OA caused by global climate change and pathogen infection.
Conclusion
A schematic representation was developed to conclude the oxidative and energy metabolism responses of oysters exposed to OA and Vibrio (Figure 7). In brief, OA and Vibrio challenges could lead to suppressed aerobic metabolism, increased anaerobic metabolism, and oxidative stress. Compromised aerobic metabolism would lead to a low cellular energy condition, which could induce increased expression of metabolic sensors. In turn, anaerobic glycolysis and other catabolic metabolisms were enhanced to provide energy for cellular stress responses and damage repair, resulting in depleted energy reserves (GLY). PROT catabolism also increased to provide additional energy under stressful conditions, thereby causing depleted PROT levels. The increased oyster energy expenditure, in response to pathogen challenge along with exposure to acidified seawater, was expected, as important physiological processes such as immune responses, antioxidant responses, and acid–base homeostasis maintenance were stimulated. Above all, our results emphasize the deleterious effects of OA and pathogen infection on cellular stress and maintenance of metabolic homeostasis in marine invertebrates.
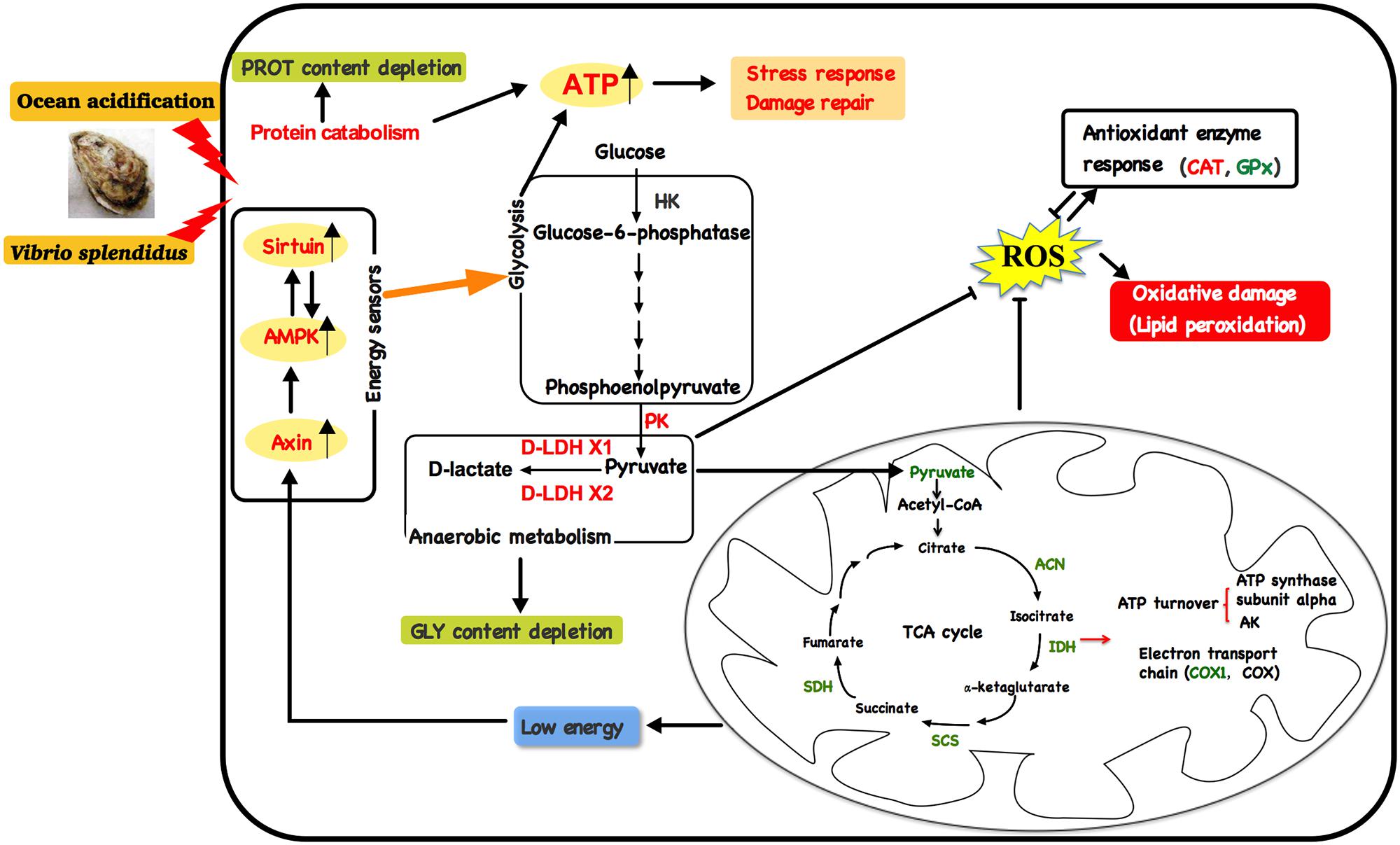
FIGURE 7. A schematic illustration showing the action of energy metabolism and oxidative stress in oysters exposed to Vibrio and ocean acidification.
Author Contributions
RC and JZ conceived and designed the experimental plan, analyzed the data, and drafted the manuscript. RC, YL, QW, DY, HL, WR, and YQ performed the experiments.
Funding
This research was supported by the grants from the Strategic Priority Research Program of the Chinese Academy of Sciences (XDA11020305), National Natural Science Foundation of China (No. 31172388), Key Research Program of the Chinese Academy of Sciences (Grant No. KZZDEW-14), Science and Technology Service Network Initiative (STS) Project (No. KFJ-STS-ZDTP-023), the Instrument Developing Project of the Chinese Academy of Sciences (YJKYYQ20170071), and the Youth Innovation Promotion Association, CAS (2016196).
Conflict of Interest Statement
The authors declare that the research was conducted in the absence of any commercial or financial relationships that could be construed as a potential conflict of interest.
Supplementary Material
The Supplementary Material for this article can be found online at: https://www.frontiersin.org/articles/10.3389/fphys.2018.00880/full#supplementary-material
Abbreviations
ACN, aconitate hydratase; AK, adenylate kinase; AMPK, AMP-activated protein kinase; Axin, Fu gene inhibition axis formation; COX, cytochrome c oxidase; COX 1, subunit 1 of cytochrome c oxidase; D-LDH X1, D-lactate dehydrogenase transcript variant X1 (mitochondrial); D-LDH X2, D-lactate dehydrogenase transcript variant X2 (mitochondrial); GLY, glycogen; HK, hexokinase; IDH, isocitrate dehydrogenase; PK, pyruvate kinase; PROT, protein; SCS, succinyl-CoA synthetase alpha subunit; SDH, succinate dehydrogenase; SIRT2, NAD-dependent deacetylase sirtuin-2.
References
Beauchamp, C., and Fridovich, I. (1971). Superoxide dismutase: improved assays and an assay applicable to acrylamide gels. Anal. Biochem. 44, 276–287. doi: 10.1016/0003-2697(71)90370-8
Beaz-Hidalgo, R., Balboa, S., Romalde, J. L., and Figueras, M. J. (2010). Diversity and pathogenecity of Vibrio species in cultured bivalve molluscs. Environ. Microbiol. Rep. 2, 34–43. doi: 10.1111/j.1758-2229.2010.00135.x
Beliaeff, B., and Burgeot, T. (2002). Integrated biomarker response: a useful tool for ecological risk assessment. Environ. Toxicol. Chem. 21, 1316–1322. doi: 10.1002/etc.5620210629
Benedetti, M., Lanzoni, I., Nardi, A., d’Errico, G., Di Carlo, M., Fattorini, D., et al. (2016). Oxidative responsiveness to multiple stressors in the key Antarctic species, Adamussium colbecki: interactions between temperature, acidification and cadmium exposure. Mar. Environ. Res. 121, 20–30. doi: 10.1016/j.marenvres.2016.03.011
Cai, W.-J., Hu, X., Huang, W.-J., Murrell, M. C., Lehrter, J. C., Lohrenz, S. E., et al. (2011). Acidification of subsurface coastal waters enhanced by eutrophication. Nat. Geosci. 4, 766–770. doi: 10.1038/ngeo1297
Caldeira, K., and Wickett, M. E. (2005). Ocean model predictions of chemistry changes from carbon dioxide emissions to the atmosphere and ocean. J. Geophys. Res. Oceans 110:C09S04. doi: 10.1029/2004JC002671
Canesi, L., Barmo, C., Fabbri, R., Ciacci, C., Vergani, L., Roch, P., et al. (2010). Effects of vibrio challenge on digestive gland biomarkers and antioxidant gene expression in Mytilus galloprovincialis. Comp. Biochem. Physiol. Part C Toxicol. Pharmacol. 152, 399–406. doi: 10.1016/j.cbpc.2010.06.008
Cantó, C., Gerhart-Hines, Z., Feige, J. N., Lagouge, M., Noriega, L., Milne, J. C., et al. (2009). AMPK regulates energy expenditure by modulating NAD+ metabolism and SIRT1 activity. Nature 458, 1056–1060. doi: 10.1038/nature07813
Cao, R., Wang, Q., Yang, D., Liu, Y., Ran, W., Qu, Y., et al. (2018). CO2-induced ocean acidification impairs the immune function of the Pacific oyster against Vibrio splendidus challenge: an integrated study from a cellular and proteomic perspective. Sci. Total Environ. 625, 1574–1583. doi: 10.1016/j.scitotenv.2018.01.056
Carregosa, V., Velez, C., Soares, A. M., Figueira, E., and Freitas, R. (2014). Physiological and biochemical responses of three Veneridae clams exposed to salinity changes. Comp. Biochem. Physiol. B Biochem. Mol. Biol. 17, 1–9. doi: 10.1016/j.cbpb.2014.08.001
Castillo, N., Saavedra, L. M., Vargas, C. A., Gallardo-Escárate, C., and Détrée, C. (2017). Ocean acidification and pathogen exposure modulate the immune response of the edible mussel Mytilus chilensis. Fish Shellfish Immunol. 70, 149–155. doi: 10.1016/j.fsi.2017.08.047
Chandel, N. S., and Schumacker, P. T. (2000). Cellular oxygen sensing by mitochondria: old questions, new insight. J. Appl. Physiol. 88, 1880–1889.
Chen, S., Gao, K., and Beardall, J. (2015). Viral attack exacerbates the susceptibility of a bloom-forming alga to ocean acidification. Glob. Change Biol. 21, 629–636. doi: 10.1111/gcb.12753
Ciacci, C., Betti, M., Canonico, B., Citterio, B., Roch, P., and Canesi, L. (2010). Specificity of anti-Vibrio immune response through p38 MAPK and PKC activation in the hemocytes of the mussel Mytilus galloprovincialis. J. Invertebr. Pathol. 105, 49–55. doi: 10.1016/j.jip.2010.05.010
Dahlke, F. T., Leo, E., Mark, F. C., Pörtner, H.-O., Bickmeyer, U., Frickenhaus, S., et al. (2016). Effects of ocean acidification increase embryonic sensitivity to thermal extremes in Atlantic cod, Gadus morhua. Glob. Change Biol. 23, 1499–1510. doi: 10.1111/gcb.13527
Dailianis, S., Piperakis, S. M., and Kaloyianni, M. (2005). Cadmium effects on ROS production and DNA damage via adrenergic receptors stimulation: role of Na+/H+ exchanger and PKC. Free Radic. Res. 39, 1059–1070. doi: 10.1080/10715760500243765
de Lorgeril, J., Zenagui, R., Rosa, R. D., Piquemal, D., and Bachère, E. (2011). Whole transcriptome profiling of successful immune response to vibrio infections in the oyster Crassostrea gigas by digital gene expression analysis. PLoS One 6:e23142. doi: 10.1371/journal.pone.0023142
Dickson, A. G. (1990). Standard potential of the reaction: AgCl(s) + 12H2(g) = Ag(s) + HCl(aq), and the standard acidity constant of the ion HSO4- in synthetic sea water from 273.15 to 318.15 K. J. Chem. Thermodyn. 22, 113–127. doi: 10.1016/0021-9614(90)90074-Z
Dineshram, R., Chandramouli, K., Ko, G. W. K., Zhang, H., Qian, P.-Y., Ravasi, T., et al. (2016). Quantitative analysis of oyster larval proteome provides new insights into the effects of multiple climate change stressors. Glob. Change Biol. 22, 2054–2068. doi: 10.1111/gcb.13249
Dittman, D. E., Ford, S. E., and Padilla, A. (2001). Effects of Perkinsus marinus on reproduction and condition of the eastern oyster, Crassostrea virginica, depend on timing. Mar. Ecol. Prog. Ser. 20, 1025–1034. doi: 10.3354/dao02964
Doney, S. C., Mahowald, N., Lima, I., Feely, R. A., Mackenzie, F. T., Lamarque, J.-F., et al. (2007). Impact of anthropogenic atmospheric nitrogen and sulfur deposition on ocean acidification and the inorganic carbon system. Proc. Natl. Acad. Sci. U.S.A. 104, 14580–14585. doi: 10.1073/pnas.0702218104
Dong, Y., Han, G., and Huang, X. (2014). Stress modulation of cellular metabolic sensors: interaction of stress from temperature and rainfall on the intertidal limpet Cellana toreuma. Mol. Ecol. 23, 4541–4554. doi: 10.1111/mec.12882
Dong, Y., and Zhang, S. (2015). Ecological relevance of energy metabolism: transcriptional responses in energy sensing and expenditure to thermal and osmotic stresses in an intertidal limpet. Funct. Ecol. 30, 1539–1548. doi: 10.1111/1365-2435.12625
Duckworth, C. G., Picariello, C. R., Thomason, R. K., Patel, K. S., and Bielmyer-Fraser, G. K. (2017). Responses of the sea anemone, Exaiptasia pallida, to ocean acidification conditions and zinc or nickel exposure. Aquat. Toxicol. 182, 120–128. doi: 10.1016/j.aquatox.2016.11.014
Dumbauld, B. R., Ruesink, J. L., and Rumrill, S. S. (2009). The ecological role of bivalve shellfish aquaculture in the estuarine environment: a review with application to oyster and clam culture in West Coast (USA) estuaries. Aquaculture 290, 196–223. doi: 10.1016/j.aquaculture.2009.02.033
Duperthuy, M., Binesse, J., Le Roux, F., Romestand, B., Caro, A., Got, P., et al. (2010). The major outer membrane protein OmpU of Vibrio splendidus contributes to host antimicrobial peptide resistance and is required for virulence in the oyster Crassostrea gigas. Environ. Microbiol. 12, 951–963. doi: 10.1111/j.1462-2920.2009.02138.x
Ellis, R. P. (2013). The Impact of Ocean Acidification, Increased Seawater Temperature and a Bacterial Challenge on the Immune Response and Physiology of the Blue Mussel, Mytilus edulis. Plymouth: University of Plymouth.
Ellis, R. P., Spicer, J. I., Byrne, J. J., Sommer, U., Viant, M. R., White, D. A., et al. (2014). 1H NMR metabolomics reveals contrasting response by male and female mussels exposed to reduced seawater pH, increased temperature, and a pathogen. Environ. Sci. Technol. 48, 7044–7052. doi: 10.1021/es501601w
Ellis, R. P., Widdicombe, S., Parry, H., Hutchinson, T. H., and Spicer, J. I. (2015). Pathogenic challenge reveals immune trade-off in mussels exposed to reduced seawater pH and increased temperature. J. Exp. Mar. Biol. Ecol. 462, 83–89. doi: 10.1016/j.jembe.2014.10.015
Fernie, A. R., Carrari, F., and Sweetlove, L. J. (2004). Respiratory metabolism: glycolysis, the TCA cycle and mitochondrial electron transport. Curr. Opin. Plant Biol. 7, 254–261. doi: 10.1016/j.pbi.2004.03.007
Ferreira, N. G. C., Morgado, R., Santos, M. J. G., Soares, A. M., and Loureiro, S. (2015). Biomarkers and energy reserves in the isopod Porcellionides pruinosus: the effects of long-term exposure to dimethoate. Sci. Total Environ. 502, 91–102. doi: 10.1016/j.scitotenv.2014.08.062
Flye-Sainte-Marie, J., Pouvreau, S., Paillard, C., and Jean, F. (2007). Impact of brown ring disease on the energy budget of the Manila clam Ruditapes philippinarum. J. Exp. Mar. Biol. Ecol. 349, 378–389. doi: 10.1016/j.jembe.2007.05.029
Forster, P., Ramaswamy, V., Artaxo, P., Berntsen, T., Betts, R., Fahey, D. W., et al. (2007). “Changes in atmospheric constituents and in radiative forcing,” in Climate Change 2007: The Physical Science Basis. Contribution of Working Group I to the Fourth Assessment Report of the Intergovernmental Panel on Climate Change, eds S. Solomon, D. Qin, M. Manning, Z. Chen, M. Marquis, K. B. Averyt, et al. (Cambridge: Cambridge University Press), 129–234.
Gay, M., Renault, T., Pons, A.-M., and Roux, F. L. (2004). Two Vibrio splendidus related strains collaborate to kill Crassostrea gigas: taxonomy and host alterations. Dis. Aquat. Organ. 62, 65–74.
Gazeau, F., Parker, L. M., Comeau, S., Gattuso, J.-P., O’Connor, W. A., Martin, S., et al. (2013). Impacts of ocean acidification on marine shelled molluscs. Mar. Biol. 160, 2207–2245. doi: 10.1007/s00227-013-2219-3
Giacomin, M., Jorge, M. B., and Bianchini, A. (2014). Effects of copper exposure on the energy metabolism in juveniles of the marine clam Mesodesma mactroides. Aquat. Toxicol. 152, 30–37. doi: 10.1016/j.aquatox.2014.03.025
Giarratano, E., Gil, M. N., and Malanga, G. (2014). Biomarkers of environmental stress in gills of ribbed mussel Aulacomya atra atra (Nuevo Gulf, Northern Patagonia). Ecotoxicol. Environ. Saf. 107, 111–119. doi: 10.1016/j.ecoenv.2014.05.003
Gran, G. (1952). Determination of the equivalence point in potentiometric titrations. Part II. Analyst 77, 661–670.
Greenway, S. C., and Storey, K. B. (1999). The effect of prolonged anoxia on enzyme activities in oysters (Crassostrea virginica) at different seasons. J. Exp. Mar. Biol. Ecol. 242, 259–272. doi: 10.1016/S0022-0981(99)00103-3
Gunderson, A. R., Armstrong, E. J., and Stillman, J. H. (2016). Multiple stressors in a changing world: the need for an improved perspective on physiological responses to the dynamic marine environment. Annu. Rev. Mar. Sci. 8, 357–378. doi: 10.1146/annurev-marine-122414-033953
Halliwell, B., and Gutteridge, J. M. C. (1986). Oxygen free radicals and iron in relation to biology and medicine: some problems and concepts. Arch. Biochem. Biophys. 246, 501–514. doi: 10.1016/0003-9861(86)90305-X
Han, G., Zhang, S., Marshall, D. J., Ke, C., and Dong, Y. (2013). Metabolic energy sensors (AMPK and SIRT1), protein carbonylation and cardiac failure as biomarkers of thermal stress in an intertidal limpet: linking energetic allocation with environmental temperature during aerial emersion. J. Exp. Biol. 216, 3273–3282. doi: 10.1242/jeb.084269
Hardie, D. G., Schaffer, B. E., and Brunet, A. (2016). AMPK: an energy-sensing pathway with multiple inputs and outputs. Trends Cell Biol. 26, 190–201. doi: 10.1016/j.tcb.2015.10.013
Hemre, G.-I., Mommsen, T. P., and Krogdahl, Å. (2002). Carbohydrates in fish nutrition: effects on growth, glucose metabolism and hepatic enzymes. Aquac. Nutr. 8, 175–194. doi: 10.1046/j.1365-2095.2002.00200.x
Hernroth, B., Baden, S., Tassidis, H., Hörnaeus, K., Guillemant, J., Bergström Lind, S., et al. (2016). Impact of ocean acidification on antimicrobial activity in gills of the blue mussel (Mytilus edulis). Fish Shellfish Immunol. 55, 452–459. doi: 10.1016/j.fsi.2016.04.007
Hernroth, B., Baden, S., Thorndyke, M., and Dupont, S. (2011). Immune suppression of the echinoderm Asterias rubens (L.) following long-term ocean acidification. Aquat. Toxicol. 103, 222–224. doi: 10.1016/j.aquatox.2011.03.001
Hooper, C., Day, R., Slocombe, R., Handlinger, J., and Benkendorff, K. (2007). Stress and immune responses in abalone: limitations in current knowledge and investigative methods based on other models. Fish Shellfish Immunol. 22, 363–379. doi: 10.1016/j.fsi.2006.06.009
Houtkooper, R. H., Pirinen, E., and Auwerx, J. (2012). Sirtuins as regulators of metabolism and healthspan. Nat. Rev. Mol. Cell Biol. 13, 225–238. doi: 10.1038/nrm3293
Hu, X., and Cai, W.-J. (2013). Estuarine acidification and minimum buffer zone—A conceptual study. Geophys. Res. Lett. 40, 5176–5181. doi: 10.1002/grl.51000
Intergovernmental Panel on Climate Change (Ed.). (2014). Climate Change 2013 - The Physical Science Basis: Working Group I Contribution to the Fifth Assessment Report of the Intergovernmental Panel on Climate Change. Cambridge: Cambridge University Press.
Ivanina, A. V., Hawkins, C., and Sokolova, I. M. (2014). Immunomodulation by the interactive effects of cadmium and hypercapnia in marine bivalves Crassostrea virginica and Mercenaria mercenaria. Fish Shellfish Immunol. 37, 299–312. doi: 10.1016/j.fsi.2014.02.016
Ivanina, A. V., and Sokolova, I. M. (2015). Interactive effects of metal pollution and ocean acidification on physiology of marine organisms. Curr. Zool. 61, 653–668. doi: 10.1093/czoolo/61.4.653
Jost, J. A., Keshwani, S. S., and Abou-Hanna, J. J. (2015). Activation of AMP-activated protein kinase in response to temperature elevation shows seasonal variation in the zebra mussel, Dreissena polymorpha. Comp. Biochem. Physiol. A Mol. Integr. Physiol. 182, 75–83. doi: 10.1016/j.cbpa.2014.11.025
Lacoste, A., Jalabert, F., Malham, S., Cueff, A., Gélébart, F., Cordevant, C., et al. (2001). A Vibrio splendidus strain is associated with summer mortality of juvenile oysters Crassostrea gigas in the Bay of Morlaix (North Brittany, France). Dis. Aquat. Organ. 46, 139–145.
Lannig, G., Eilers, S., Pörtner, H. O., Sokolova, I. M., and Bock, C. (2010). Impact of ocean acidification on energy metabolism of oyster, Crassostrea gigas—changes in metabolic pathways and thermal response. Mar. Drugs 8, 2318–2339. doi: 10.3390/md8082318
Lawrence, R. A., and Burk, R. F. (1976). Glutathione peroxidase activity in selenium-deficient rat liver. Biochem. Biophys. Res. Commun. 71, 952–958. doi: 10.1016/0006-291X(76)90747-6
Leiniö, S., and Lehtonen, K. K. (2005). Seasonal variability in biomarkers in the bivalves Mytilus edulis and Macoma balthica from the northern Baltic Sea. Comp. Biochem. Physiol. Part C Toxicol. Pharmacol. 140, 408–421. doi: 10.1016/j.cca.2005.04.005
Lesser, M. P. (2016). Climate change stressors cause metabolic depression in the blue mussel, Mytilus edulis, from the Gulf of Maine. Limnol. Oceanogr. 61, 1705–1717. doi: 10.1002/lno.10326
Li, Y., Li, M., Shi, J., Yang, X., and Wang, Z. (2012). Hepatic antioxidative responses to PCDPSs and estimated short-term biotoxicity in freshwater fish. Aquat. Toxicol. 120–121, 90–98. doi: 10.1016/j.aquatox.2012.04.016
Li, Y., Song, X., Wang, W., Wang, L., Yi, Q., Jiang, S., et al. (2017). The hematopoiesis in gill and its role in the immune response of Pacific oyster Crassostrea gigas against secondary challenge with Vibrio splendidus. Dev. Comp. Immunol. 71, 59–69. doi: 10.1016/j.dci.2017.01.024
Liu, S., Shi, W., Guo, C., Zhao, X., Han, Y., Peng, C., et al. (2016). Ocean acidification weakens the immune response of blood clam through hampering the NF-kappa β and toll-like receptor pathways. Fish Shellfish Immunol. 54, 322–327. doi: 10.1016/j.fsi.2016.04.030
Liu, X., Ji, C., Zhao, J., and Wu, H. (2013). Differential metabolic responses of clam Ruditapes philippinarum to Vibrio anguillarum and Vibrio splendidus challenges. Fish Shellfish Immunol. 35, 2001–2007. doi: 10.1016/j.fsi.2013.09.014
Livak, K. J., and Schmittgen, T. D. (2001). Analysis of relative gene expression data using Real-Time quantitative PCR and the 2-ΔΔCT method. Methods 25, 402–408. doi: 10.1006/meth.2001.1262
Matoo, O. B., Ivanina, A. V., Ullstad, C., Beniash, E., and Sokolova, I. M. (2013). Interactive effects of elevated temperature and CO2 levels on metabolism and oxidative stress in two common marine bivalves (Crassostrea virginica and Mercenaria mercenaria). Comp. Biochem. Physiol. A Mol. Integr. Physiol. 164, 545–553. doi: 10.1016/j.cbpa.2012.12.025
Michaelidis, B., Ouzounis, C., Paleras, A., and Pörtner, H. O. (2005). Effects of long-term moderate hypercapnia on acid–base balance and growth rate in marine mussels Mytilus galloprovincialis. Mar. Ecol. Prog. Ser. 293, 109–118.
Millero, F. J., Graham, T. B., Huang, F., Bustos-Serrano, H., and Pierrot, D. (2006). Dissociation constants of carbonic acid in seawater as a function of salinity and temperature. Mar. Chem. 100, 80–94. doi: 10.1016/j.marchem.2005.12.001
Moreira, A., Figueira, E., Soares, A. M., and Freitas, R. (2016). The effects of arsenic and seawater acidification on antioxidant and biomineralization responses in two closely related Crassostrea species. Sci. Total Environ. 545–546, 569–581. doi: 10.1016/j.scitotenv.2015.12.029
Ohkawa, H., Ohishi, N., and Yagi, K. (1979). Assay for lipid peroxides in animal tissues by thiobarbituric acid reaction. Anal. Biochem. 95, 351–358. doi: 10.1016/0003-2697(79)90738-3
Paillard, C., Allam, B., and Oubella, R. (2004). Effect of temperature on defense parameters in Manila clam Ruditapes philippinarum challenged with Vibrio tapetis. Dis. Aquat. Organ. 59, 249–262. doi: 10.3354/dao059249
Pörtner, H. O., Langenbuch, M., and Reipschläger, A. (2004). Biological impact of elevated ocean CO2 concentrations: lessons from animal physiology and earth history. J. Oceanogr. 60, 705–718. doi: 10.1007/s10872-004-5763-0
Qu, R., Feng, M., Wang, X., Qin, L., Wang, C., Wang, Z., et al. (2014). Metal accumulation and oxidative stress biomarkers in liver of freshwater fish Carassius auratus following in vivo exposure to waterborne zinc under different pH values. Aquat. Toxicol. 150, 9–16. doi: 10.1016/j.aquatox.2014.02.008
Queirós, A. M., Fernandes, J. A., Faulwetter, S., Nunes, J., Rastrick, S. P. S., Mieszkowska, N., et al. (2015). Scaling up experimental ocean acidification and warming research: from individuals to the ecosystem. Glob. Change Biol. 21, 130–143. doi: 10.1111/gcb.12675
Radi, R., Beckman, J. S., Bush, K. M., and Freeman, B. A. (1991). Peroxynitrite-induced membrane lipid peroxidation: the cytotoxic potential of superoxide and nitric oxide. Arch. Biochem. Biophys. 288, 481–487. doi: 10.1016/0003-9861(91)90224-7
Ricevuto, E., Lanzoni, I., Fattorini, D., Regoli, F., and Gambi, M. C. (2016). Arsenic speciation and susceptibility to oxidative stress in the fanworm Sabella spallanzanii (Gmelin) (Annelida, Sabellidae) under naturally acidified conditions: an in situ transplant experiment in a Mediterranean CO2 vent system. Sci. Total Environ. 544, 765–773. doi: 10.1016/j.scitotenv.2015.11.154
Robinson, H. W., and Hogden, C. (1940). The biuret reaction in the determination of serum proteins. 1. A study of the conditions necessary for the production of a stable color which bears a quantitative relationship to the protein concentration. J. Biol. Chem. 135, 707–725. doi: 10.1016/0003-9861(91)90224-7
Sabine, C. L., Feely, R. A., Gruber, N., Key, R. M., Lee, K., Bullister, J. L., et al. (2004). The oceanic sink for anthropogenic CO2. Science 305, 367–371. doi: 10.1126/science.1097403
Sanchez, W., Burgeot, T., and Porcher, J.-M. (2013). A novel “Integrated Biomarker Response” calculation based on reference deviation concept. Environ. Sci. Pollut. Res. 20, 2721–2725. doi: 10.1007/s11356-012-1359-1
Simon, E. S., Plante, R., and Whitesides, G. M. (1989). D-lactate dehydrogenase. Appl. Biochem. Biotechnol. 22, 169–179. doi: 10.1007/BF02921743
Sokolova, I. M., Frederich, M., Bagwe, R., Lannig, G., and Sukhotin, A. A. (2012). Energy homeostasis as an integrative tool for assessing limits of environmental stress tolerance in aquatic invertebrates. Mar. Environ. Res. 79, 1–15. doi: 10.1016/j.marenvres.2012.04.003
Sui, Y., Hu, M., Huang, X., Wang, Y., and Lu, W. (2015). Anti-predatory responses of the thick shell mussel Mytilus coruscus exposed to seawater acidification and hypoxia. Mar. Environ. Res. 109, 159–167. doi: 10.1016/j.marenvres.2015.07.008
Tomanek, L., Zuzow, M. J., Ivanina, A. V., Beniash, E., and Sokolova, I. M. (2011). Proteomic response to elevated PCO2 level in eastern oysters, Crassostrea virginica: evidence for oxidative stress. J. Exp. Biol. 214, 1836–1844. doi: 10.1242/jeb.055475
Valerio-García, R. C., Carbajal-Hernández, A. L., Martínez-Ruíz, E. B., Jarquín-Díaz, V. H., Haro-Pérez, C., and Martínez-Jerónimo, F. (2017). Exposure to silver nanoparticles produces oxidative stress and affects macromolecular and metabolic biomarkers in the goodeid fish Chapalichthys pardalis. Sci. Total Environ. 583, 308–318. doi: 10.1016/j.scitotenv.2017.01.070
Vega-López, A., Galar-Martínez, M., Jiménez-Orozco, F. A., García-Latorre, E., and Domínguez-López, M. L. (2007). Gender related differences in the oxidative stress response to PCB exposure in an endangered goodeid fish (Girardinichthys viviparus). Comp. Biochem. Physiol. A Mol. Integr. Physiol. 146, 672–678. doi: 10.1016/j.cbpa.2006.04.022
Velez, C., Figueira, E., Soares, A. M., and Freitas, R. (2016a). Combined effects of seawater acidification and salinity changes in Ruditapes philippinarum. Aquat. Toxicol. 176, 141–150. doi: 10.1016/j.aquatox.2016.04.016
Velez, C., Figueira, E., Soares, A. M., and Freitas, R. (2016b). Native and introduced clams biochemical responses to salinity and pH changes. Sci. Total Environ. 566–567, 260–268. doi: 10.1016/j.scitotenv.2016.05.019
Wang, C., Rong, H., Liu, H., Wang, X., Gao, Y., Deng, R., et al. (2018). Detoxification mechanisms, defense responses, and toxicity threshold in the earthworm Eisenia foetida exposed to ciprofloxacin-polluted soils. Sci. Total Environ. 612, 442–449. doi: 10.1016/j.scitotenv.2017.08.120
Wang, Q., Cao, R., Ning, X., You, L., Mu, C., Wang, C., et al. (2016). Effects of ocean acidification on immune responses of the Pacific oyster Crassostrea gigas. Fish Shellfish Immunol. 49, 24–33. doi: 10.1016/j.fsi.2015.12.025
Wang, X., Wang, M., Jia, Z., Qiu, L., Wang, L., Zhang, A., et al. (2017). A carbonic anhydrase serves as an important acid-base regulator in Pacific oyster Crassostrea gigas exposed to elevated CO2: implication for physiological responses of mollusk to ocean acidification. Mar. Biotechnol. 19, 22–35. doi: 10.1007/s10126-017-9734-z
Wang, Y., Li, L., Hu, M., and Lu, W. (2015). Physiological energetics of the thick shell mussel Mytilus coruscus exposed to seawater acidification and thermal stress. Sci. Total Environ. 514, 261–272. doi: 10.1016/j.scitotenv.2015.01.092
Wikstrom, M. (1977). Proton pump coupled to cytochrome c oxidase in mitochondria. Nature 266, 271–273.
Xu, P., Zeng, G., Huang, D., Liu, L., Zhao, M., Lai, C., et al. (2016). Metal bioaccumulation, oxidative stress and antioxidant defenses in Phanerochaete chrysosporium response to Cd exposure. Ecol. Eng. 87, 150–156. doi: 10.1016/j.ecoleng.2015.11.029
Zhang, Y.-L., Guo, H., Zhang, C.-S., Lin, S.-Y., Yin, Z., Peng, Y., et al. (2013). AMP as a low-energy charge signal autonomously initiates assembly of AXIN-AMPK-LKB1 complex for AMPK activation. Cell Metab. 18, 546–555. doi: 10.1016/j.cmet.2013.09.005
Zhao, X., Shi, W., Han, Y., Liu, S., Guo, C., Fu, W., et al. (2017). Ocean acidification adversely influences metabolism, extracellular pH and calcification of an economically important marine bivalve, Tegillarca granosa. Mar. Environ. Res. 125, 82–89. doi: 10.1016/j.marenvres.2017.01.007
Keywords: ocean acidification, Crassostrea gigas, Vibrio splendidus, oxidative stress, energy metabolism, physiological response
Citation: Cao R, Liu Y, Wang Q, Yang D, Liu H, Ran W, Qu Y and Zhao J (2018) Seawater Acidification Reduced the Resistance of Crassostrea gigas to Vibrio splendidus Challenge: An Energy Metabolism Perspective. Front. Physiol. 9:880. doi: 10.3389/fphys.2018.00880
Received: 03 April 2018; Accepted: 19 June 2018;
Published: 12 July 2018.
Edited by:
Xiaotong Wang, Ludong University, ChinaReviewed by:
Wenguang Liu, South China Sea Institute of Oceanology (CAS), ChinaHongjun Li, National Marine Environmental Monitoring Center, China
Camille Détrée, Universidad Austral de Chile, Chile
Copyright © 2018 Cao, Liu, Wang, Yang, Liu, Ran, Qu and Zhao. This is an open-access article distributed under the terms of the Creative Commons Attribution License (CC BY). The use, distribution or reproduction in other forums is permitted, provided the original author(s) and the copyright owner(s) are credited and that the original publication in this journal is cited, in accordance with accepted academic practice. No use, distribution or reproduction is permitted which does not comply with these terms.
*Correspondence: Jianmin Zhao, jmzhao@yic.ac.cn