- Department of Medical Pharmacology and Pharmacology/Toxicology, Colleges of Medicine and Pharmacy, University of Arizona, Tucson, AZ, United States
The blood-brain barrier (BBB) allows the brain to selectively import nutrients and energy critical to neuronal function while simultaneously excluding neurotoxic substances from the peripheral circulation. In contrast to the highly permeable vasculature present in most organs that reside outside of the central nervous system (CNS), the BBB exhibits a high transendothelial electrical resistance (TEER) along with a low rate of transcytosis and greatly restricted paracellular permeability. The property of low paracellular permeability is controlled by tight junction (TJ) protein complexes that seal the paracellular route between apposing brain microvascular endothelial cells. Although tight junction protein complexes are principal contributors to physical barrier properties, they are not static in nature. Rather, tight junction protein complexes are highly dynamic structures, where expression and/or localization of individual constituent proteins can be modified in response to pathophysiological stressors. These stressors induce modifications to tight junction protein complexes that involve de novo synthesis of new protein or discrete trafficking mechanisms. Such responsiveness of BBB tight junctions to diseases indicates that these protein complexes are critical for maintenance of CNS homeostasis. In fulfillment of this vital role, BBB tight junctions are also a major obstacle to therapeutic drug delivery to the brain. There is an opportunity to overcome this substantial obstacle and optimize neuropharmacology via acquisition of a detailed understanding of BBB tight junction structure, function, and regulation. In this review, we discuss physiological characteristics of tight junction protein complexes and how these properties regulate delivery of therapeutics to the CNS for treatment of neurological diseases. Specifically, we will discuss modulation of tight junction structure, function, and regulation both in the context of disease states and in the setting of pharmacotherapy. In particular, we will highlight how these properties can be potentially manipulated at the molecular level to increase CNS drug levels via paracellular transport to the brain.
Introduction
The neurovascular unit (NVU) is an anatomical and functional unit that responds to the needs of neuronal energy demands by precisely regulating cerebral blood flow (Iadecola, 2017). The NVU is comprised of brain endothelial cells, astrocytes, mural cells (i.e., vascular smooth muscle cells and pericytes), microglia, neurons, and extracellular matrix components (Figure 1). The microvascular endothelial cells of the NVU comprise the blood-brain barrier (BBB), a physical and metabolic barrier important for maintaining central nervous system (CNS) homeostasis and protecting the brain from potentially harmful circulating substances. The metabolic component of the BBB consists of drug metabolizing enzymes that are capable of inactivating therapeutics and/or altering their ability to enter the CNS (Agundez et al., 2014). The BBB exhibits low permeability compared to peripheral blood vessels and only lipophilic molecules with molecular weights less than 500 Da, and few hydrogen bond donors and/or acceptors are able to passively diffuse across the BBB under physiological conditions (Banks, 2009; Banks and Greig, 2019). Nutrients such as glucose, amino acids, nucleosides, and certain neurotransmitters that are important for CNS function are able to enter the brain through carrier-mediated transport (Ohtsuki and Terasaki, 2007). Influx and efflux transporters, which extrude toxic metabolites from endothelial cells and limit CNS entry of xenobiotics and therapeutics from the bloodstream, are also a prominent feature of the BBB (Abdullahi et al., 2017). While receptors and transporters expressed at the endothelial plasma membrane selectively regulate brain entry of various solutes from the blood, the major factors limiting BBB permeability are its low rate of pinocytosis and tight junction (TJ) protein complexes which limit passage through the transcellular and paracellular routes, respectively (Reese and Karnovsky, 1967).
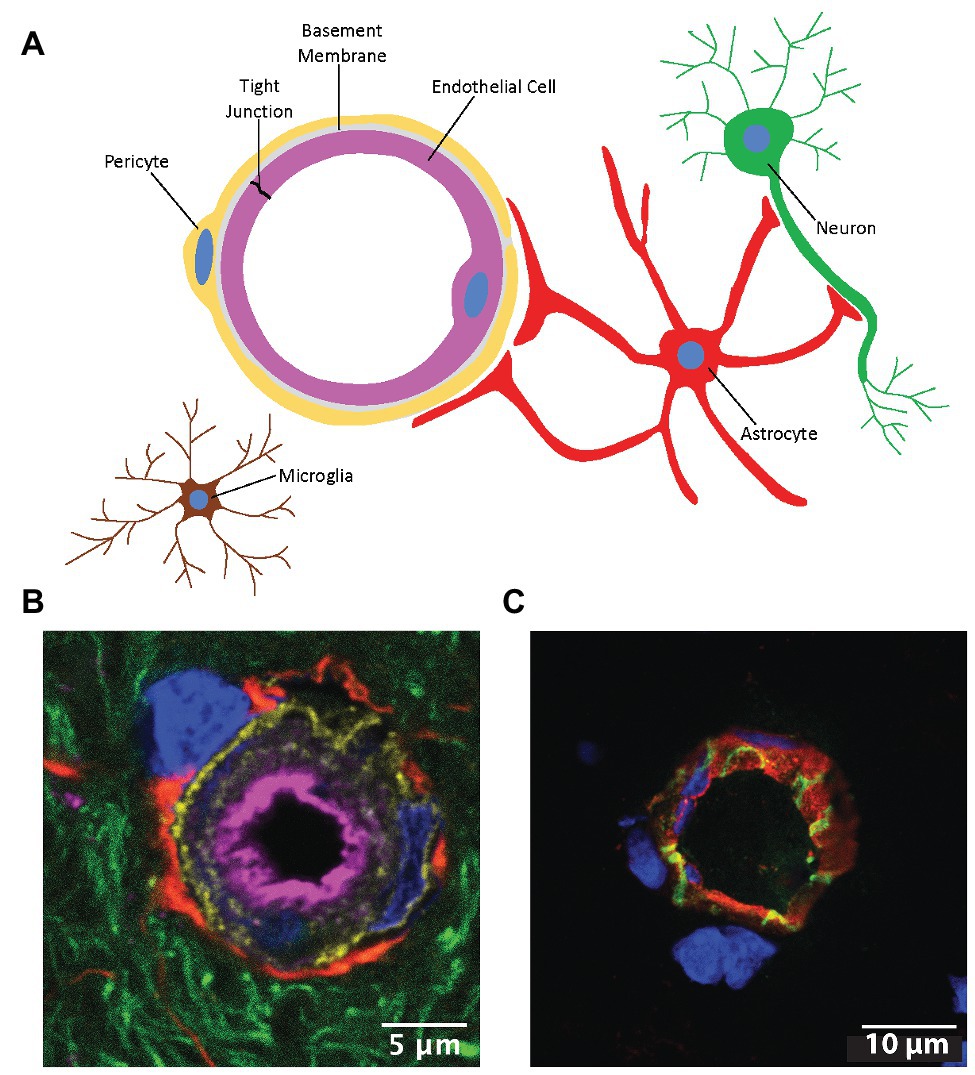
Figure 1. The neurovascular unit (NVU). (A) Cells comprising the NVU. (B) Confocal micrograph of a cerebral capillary labeled with lectin (magenta), pericytes labeled with an antibody to platelet derived growth factor beta (gold), astrocyte end feet labeled with glial fibrillary acidic protein (red), neurites labeled with Neuro-Chrom antibody (green), and nuclei labeled with 4',6-diamidino-2-phenylindole (DAPI; blue). (C) Cross-section of a microvessel labeled with lectin (red), an antibody to the tight junction (TJ) protein claudin-5 (green), and DAPI (blue). Modified with permission from Tome et al. (2018).
TJs at the BBB are protein complexes that form rows of extensive, overlapping occlusions between brain microvascular endothelial cells that greatly restrict paracellular diffusion of polar molecules and macromolecules into the CNS (Reese and Karnovsky, 1967; Brightman and Reese, 1969). The in vivo transendothelial electrical resistance (TEER) of brain microvessels is 1,500–2,000 Ω cm2, which is considerably higher than peripheral microvessels that have a TEER of 3–30 Ω cm2 (Crone and Olesen, 1982; Butt et al., 1990). Although previously considered to be static structures, the past few decades of research have revealed that TJs at the BBB are highly dynamic in nature and are dysregulated in many CNS disease states (Erdo et al., 2017; Reinhold and Rittner, 2017; Costea et al., 2019; Sweeney et al., 2019). While TJ dysregulation at the BBB can contribute to the pathogenesis of certain neurological disorders, understanding the mechanisms involved may provide an opportunity to improve CNS drug delivery through the paracellular pathway. Here, we provide an overview of the molecular composition of TJs at the BBB, disruption of TJs during CNS diseases, and strategies to manipulate TJs with the goal of improved drug delivery to the brain.
Molecular Composition and Regulation of Tjs at the BBB
In the paracellular cleft, TJs form a physical seal between adjacent brain microvascular endothelial cells (Figure 2). In conjunction with active efflux transporters and the lack of fenestrations along the apical surface of the brain microvessel, TJ protein complexes contribute to physiological functioning of the BBB, including maintenance of a stable microenvironment within the CNS. TJ functional integrity at the BBB can be evaluated by measurement of extravasation of solutes (i.e., leak) that are not transport substrates and typically remain in the vascular lumen under physiological conditions. Such vascular markers cover a wide range of molecular weights and, if used appropriately, can enable a detailed evaluation of size selectivity of vascular “leak” in the setting of pathological stressors. Commonly used tracer molecules for the study of paracellular diffusion are presented in Table 1. Using transmission electron microscopy, BBB TJ protein complexes are visualized as a series of electron dense regions (i.e., “kissing points”) between apposing membranes of adjacent endothelial cells (Chiba et al., 2008). At the molecular level, TJs are comprised of transmembrane protein complexes protruding from neighboring cells. These transmembrane TJ proteins are linked to the cytoskeleton by intracellular TJ proteins, thereby forming complex networks that are highly responsive to pathological stimuli. Indeed, many different protein constituents are reported to be present at TJs in mammalian cerebral microvasculature (Haseloff et al., 2015; Reinhold and Rittner, 2017; Berndt et al., 2019). Below, we review the specific transmembrane and intracellular proteins that have a confirmed role in maintaining TJ functional integrity in health and disease.
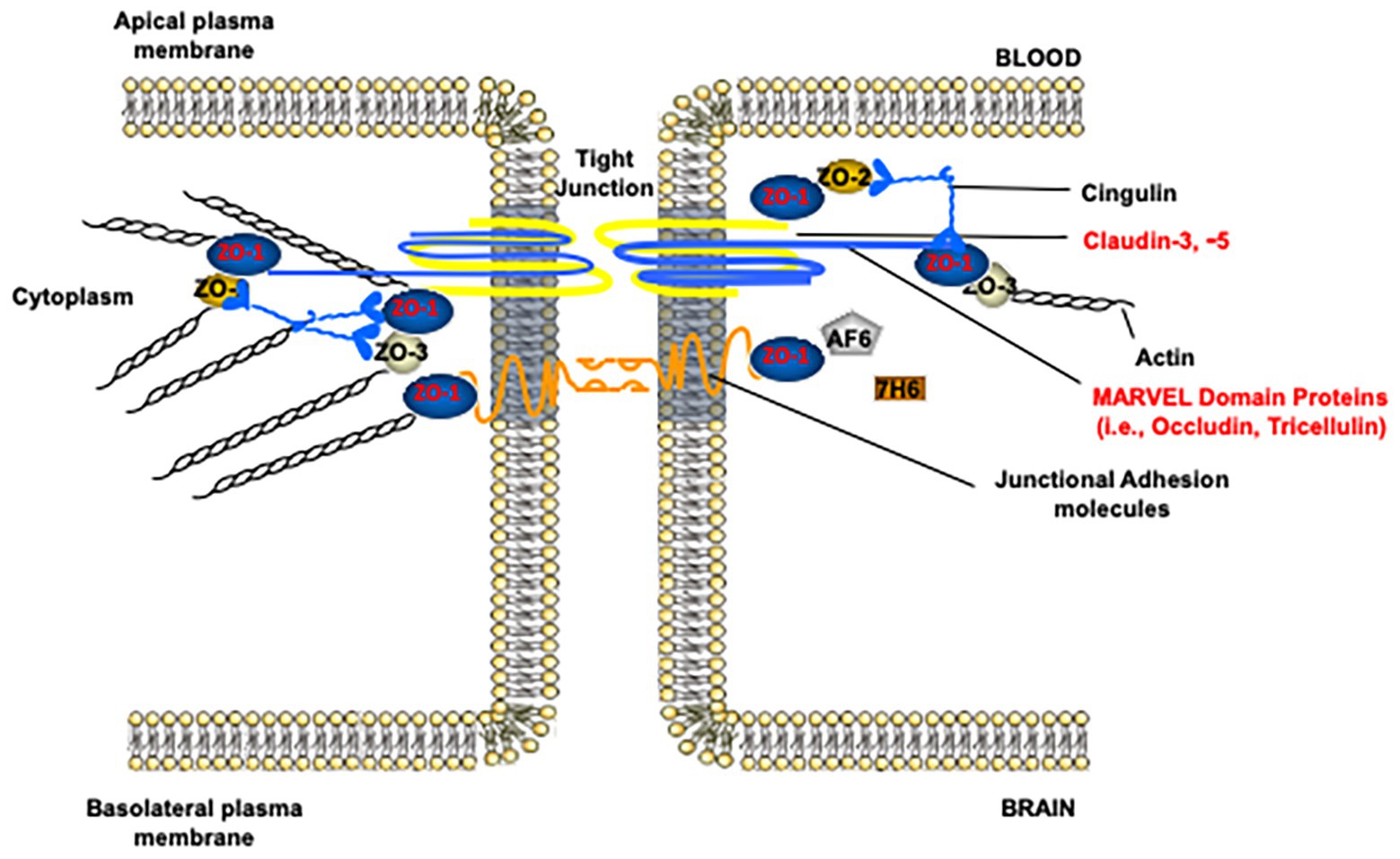
Figure 2. Molecular composition of tight junction protein complexes. Modified with permission from Abdullahi et al. (2018).
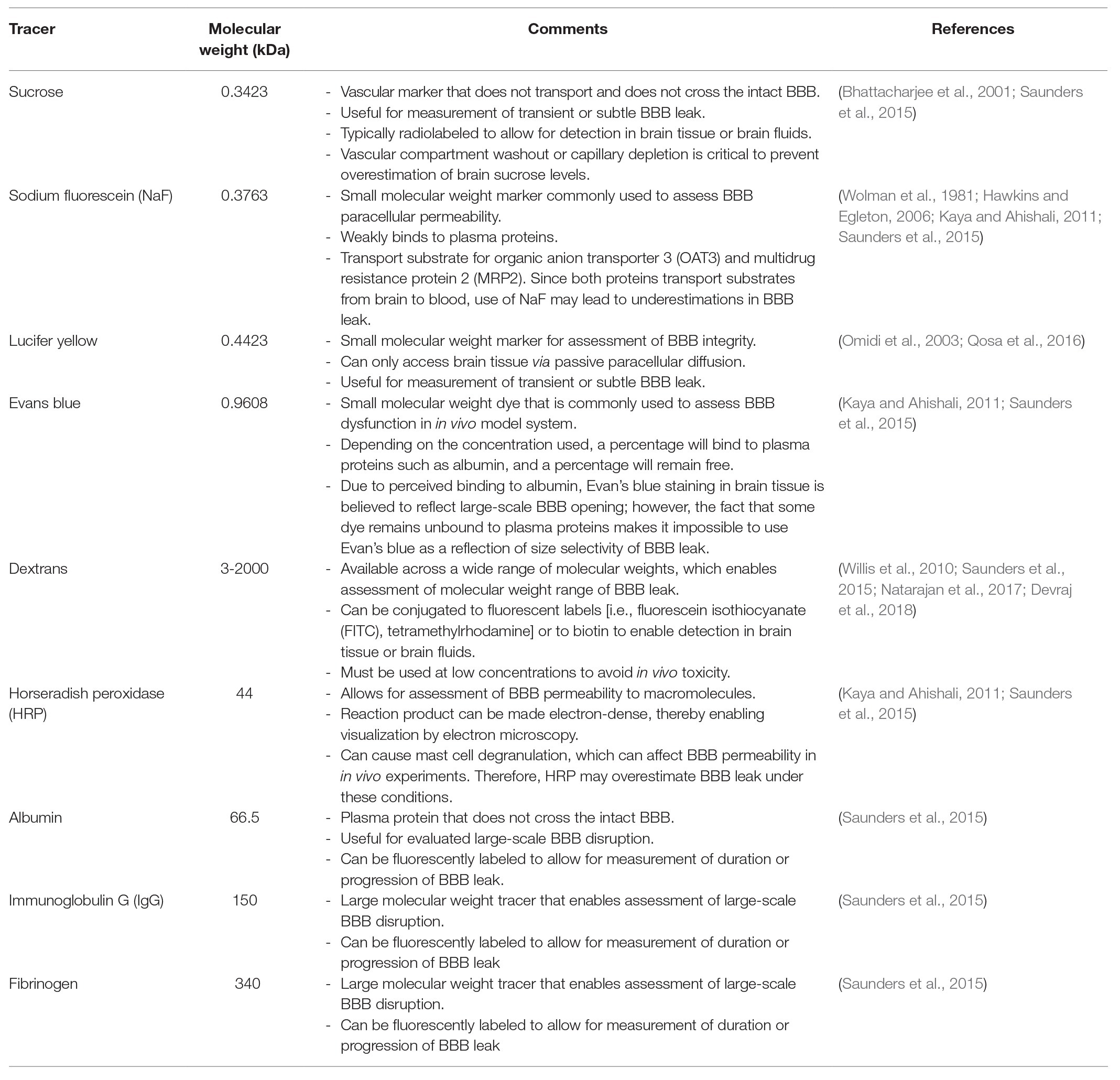
Table 1. Common blood-brain barrier (BBB) permeability markers used for measurement of paracellular leak.
Claudins are considered to be the principal proteins that establish the backbone of TJ and are critical determinants of paracellular “tightness” between adjacent BBB endothelial cells (Gunzel and Yu, 2013). The claudin family of proteins consists of 27 members that have been identified to date, and family members have an approximate molecular mass of ~26 kDa (Daneman et al., 2010; Mineta et al., 2011). They have a tissue-specific expression pattern (Hwang et al., 2014; Markov et al., 2015), which leads to different barrier properties in different tissues. In epithelial cells, claudin-3 and claudin-5 generally provide a sealing function (Amasheh et al., 2005; Milatz et al., 2010). At the BBB, claudin-5 has long been recognized as the dominant TJ protein (Greene et al., 2019). Depletion of claudin-5 in transgenic mice results in morphologically normal TJs at the BBB but also leads to detrimental brain defects and early neonatal death ~10 h after birth. Furthermore, brain microvasculature of claudin-5 knockout mice is highly permeable to molecules less than 800 Da (Nitta et al., 2003). In contrast, overexpression of claudin-5 in cultured brain microvessel endothelial cells results in elevated paracellular tightness, suggesting that expression levels of claudin-5 directly correlate with TJ function (Ohtsuki et al., 2007). Such notions have recently been challenged by the study of Schlingmann et al. (2016), which suggests that changes in TJ function are not simply the result of increased or decreased protein levels but rather arises from disruption in the overall balance of all TJ proteins (Schlingmann et al., 2016). Similar to claudin-5, expression of claudin-3 has also been reported in brain microvasculature (Ohtsuki et al., 2008; Ronaldson et al., 2009), where it may play a role in the physiological sealing function of the TJ (Winkler et al., 2020). Claudin-1 has been detected at the BBB at the messenger RNA (mRNA) level (Winkler et al., 2020); however, its expression at the BBB remains controversial in part due to possible antibody cross-reactivity between claudin-1 and claudin-3 (Bauer et al., 2014). Claudin-11 and claudin-12 have also been detected at the BBB, but claudin-12 is likely not important for barrier function (Castro Dias et al., 2019; Uchida et al., 2019).
Structurally, the claudins are tetraspan proteins with two extracellular loops (ECLs) and two cytoplasmic domains that consist of a short amino (N)-terminal sequence and a long carboxyl (C)-terminal sequence. The ECLs of claudins contribute to the formation of the TJ backbones by homo‐ or hetero-dimerization or oligomerization with neighboring claudins on the same membrane (cis-interaction) and/or with those on the apposing cell (trans-interaction; Piontek et al., 2008; Piehl et al., 2010). The ECLs are also responsible for determining charge‐ and size selectivity of the claudins (Piehl et al., 2010; Piontek et al., 2011). Therefore, interactions between claudin-5 with other claudins or with other transmembrane proteins may be necessary to properly restrict paracellular diffusion of molecules of varying charges and sizes. The C-terminus of claudins exhibits great diversity among members of the claudin family and ends with a PDZ binding motif for most claudins with the exception being claudin-12 (Piontek et al., 2011; Van Itallie and Anderson, 2018). PDZ-dependent interactions at the TJ play a prominent role in the organization and modulation of TJ proteins (Itoh et al., 1999; Poliak et al., 2002). The PDZ domain on the C-terminal tail of most claudins recruits cytoplasmic proteins, particularly zonula occludens (ZOs) proteins. Additionally, PDZ domains on the C-terminal of a claudin molecule can also promote interactions with other transmembrane TJ proteins including occludin and junction adhesion molecules (JAMs; Poliak et al., 2002). Notably, the claudin C-terminal domain contains multiple serine, threonine, and tyrosine residues (Hornbeck et al., 2015). Mass spectrometry (MS) data have revealed multiple phosphorylation sites in the C-terminal region of many claudins (Hornbeck et al., 2015; Van Itallie and Anderson, 2018). Although the regulatory role of claudin phosphorylation has not yet been directly studied at the brain microvascular endothelium, studies in other epithelial and endothelial tissues have shown roles in modulation of protein-protein interactions (Nomme et al., 2015), intracellular trafficking (Van Itallie et al., 2012; Twiss et al., 2013), and protein turnover mechanisms (Mandel et al., 2012; Hou et al., 2019). For example, Rho kinase (RhoK) has been demonstrated by Yamamoto et al. (2008) to phosphorylate claudin-5 at tyrosine 207 (T207) in mouse and human brain tissues. This observation was correlated with decreased TEER, suggesting that Rho-associated protein kinase (ROCK) signaling and associated claudin-5 phosphorylation may be a critical modulatory mechanism for TJ permeability at the BBB (Yamamoto et al., 2008).
There are three tight junction-associated marvel proteins (TAMPs) that have been identified at the mammalian BBB. These proteins are known as tricellulin, marvelD3, and occludin. Tricellulin plays a prominent role in regulating paracellular permeability to macromolecules (Krug et al., 2009; Haseloff et al., 2015) while marvelD3 appears to play a compensatory sealing role at the BBB (Raleigh et al., 2010). In contrast, occludin (molecular weight ~65 kDa), the first integral membrane protein identified at BBB TJs has been more extensively characterized. Occludin knockout mice exhibit structurally and functionally intact TJs, possibly due to compensation from other TAMPs; however, these mice display several histological abnormalities and postnatal growth retardation (Saitou et al., 2000). One study suggested that occludin’s physiological role in epithelial barriers is associated with epithelial differentiation rather than establishing barrier properties (Schulzke et al., 2005). Occludin has also been shown to be an important regulator of TJ assembly and functions (Hirase et al., 2001; Van Itallie et al., 2010; Doerfel and Huber, 2012; Buschmann et al., 2013). Additionally, occludin can assemble into higher-order oligomeric assemblies, which are essential to maintenance of TJ functional integrity (McCaffrey et al., 2008). Indeed, our laboratory has shown that disruption of occludin oligomeric structures results in BBB dysfunction characterized by an increase in paracellular permeability (Lochhead et al., 2010, 2012).
Occludin contains a tetraspanning marvel domain, two ECLs, and three cytoplasmic domains, including a short N-terminal domain, a long C-terminal domain, and an intracellular short turn. As has been demonstrated in a study with mutant occludin isoforms in MDCK cells, both ECLs together with at least one transmembrane domain serve important roles in selective permeability of TJs (Balda et al., 2000). The N-terminus of occludin contributes to maintenance of TJ integrity, as evidenced by the observation that deletion of the occludin N-terminal sequence leads to higher paracellular permeability and lower TEER (Bamforth et al., 1999). Lateral (cis-) homodimerization or oligomerization of occludin is mediated by the marvel motif, the cytoplasmic C-terminus, and the second extracellular loop (ECL2; Blasig et al., 2006; Yaffe et al., 2012). These domains are sensitive to changes in redox conditions due to the disulfide bonds between cysteine residues on ECL2 and the occludin C-terminal sequence (Walter et al., 2009; Bellmann et al., 2014). The C-terminus also interacts with ZO proteins (Li et al., 2005; Tash et al., 2012), an interaction that is essential for the trafficking of occludin to the TJ (Furuse et al., 1994). Similar to the claudins, the C-terminal domain of occludin is rich in potential phosphorylation sites and has been shown to interact with a wide range of kinases and phosphatases (Smales et al., 2003; McKenzie et al., 2006; Suzuki et al., 2009; Raleigh et al., 2011; Walsh et al., 2011). With few exceptions, in vitro experiments have repeatedly demonstrated that elevated phosphotyrosine and diminished phosphoserine/threonine are generally associated with disruptive barrier functions by interfering with occludin-ZO interactions and/or occludin trafficking (Kale et al., 2003; Elias et al., 2009; Suzuki et al., 2009; Han et al., 2010; Walsh et al., 2011). Interestingly, the phosphorylation status of the C-terminus of occludin is highly dependent on cellular localization of the protein itself; TJ-localized occludin is phosphorylated at a much higher level than those localized to the lateral membrane (Sakakibara et al., 1997), highlighting the importance of examining both cellular expression and localization when studying TJ proteins.
JAMs-1, -2, and -3 are members of the immunoglobulin superfamily that are highly enriched at TJs of the cerebral microvasculature. They are not required for TJ formation (Otani et al., 2019); however, complementary DNA (cDNA) transfection of JAMs in non-TJ forming cell cultures resulted in recruitment of occludin to regions of cell-cell contacts (Bazzoni et al., 2000). Such an observation suggests a potential role for JAMs in mediating occludin trafficking to the TJ. Additionally, loss of JAM protein expression and/or migration of JAM proteins away from the TJ directly lead to loss of BBB properties at the microvascular endothelium (Haarmann et al., 2010; Wang et al., 2014). JAMs consist of a single membrane-spanning domain, a short C-terminal tail, and an immunoglobulin G (IgG)-like extracellular domain. The extracellular domain of JAM proteins mediate homo‐ or hetero-dimerization of JAM family members in both trans‐ and cis-manners, contributing to adherence of adjacent cells at the TJs (Bazzoni et al., 2000). The cytoplasmic C-terminus contains multiple phosphorylation sites and ends with a PDZ binding motif which interacts with ZO-1 as well as other PDZ-containing proteins in the endothelial cells (Bazzoni et al., 2000; Ebnet et al., 2003). Binding affinity of JAMs to ZO-1 may be regulated through the phosphorylation of protein residues on the C-terminal sequence (Van Itallie and Anderson, 2018).
ZO-1 (225 kDa), ZO-2 (160 kDa), and ZO-3 (130 kDa) belong to the PDZ domain-containing protein and membrane-associated granulated kinase (MAGUK) family, which constitute the most prominent intracellular proteins at the TJs. ZO family members are essential for assembly of functional TJs by binding to and cross-linking a wide range of TJ proteins and tethering them to the actin cytoskeleton (Fanning et al., 1998; Shin and Margolis, 2006). The ZO proteins share considerable structural similarity except for the C-terminal sequence that is unique to each specific ZO protein (Bauer et al., 2014). Indeed, differences in these C-terminal protein sequences could explain functional differences of individual ZO family members. While ZO-1 and ZO-2 are considered indispensable for TJ assembly (Umeda et al., 2006), the specific role of ZO-3 at BBB TJs has not been not well-documented. Structurally, the ZO proteins are comprised of three PDZ domains, a Src homology 3 (SH3) domain, a guanylate kinase (GuK) domain, and a proline-rich domain. The first PDZ domain (PDZ1) interacts with the PDZ domain on the C-terminus of most of the claudin proteins (Itoh et al., 1999). The second PDZ domain (PDZ2) mediates ZO-1 heterodimerization with ZO-2 or ZO-3 (Itoh et al., 1999; Wittchen et al., 1999). Structural studies have suggested weak binding between PDZ3 and JAM-1 (Bazzoni et al., 2000). The SH3 domain is the most important element for binding of actin cytoskeleton and signaling molecules (Schmidt et al., 2004; McNeil et al., 2006). Actin cytoskeletal filaments also interact with a defined actin-binding region (ABR) at the C-terminus of the ZO-1 protein (Fanning et al., 2002). It is important to note that there are multiple organizational forms of actin that are regulated by actin-binding proteins which may affect dynamics of TJ proteins and barrier integrity (Tornavaca et al., 2015). GuK are kinases that normally catalyze ATP-dependent transformation of GMP into GDP; however, the GuK domain on the ZO subfamily of MAGUKs is likely enzymatically inactive due to a missing GMP binding-site and steric hindrance at the ATP-binding site (Lye et al., 2010; Zhu et al., 2012). Instead, the GuK domain of ZO proteins has been shown to bind occludin and PDZ motifs of the claudins (Fanning et al., 1998; Chattopadhyay et al., 2014).
Tight Junction Alterations in Neurological Disorders
BBB dysfunction is a pathological feature of several diseases affecting the CNS (Erdo et al., 2017; Reinhold and Rittner, 2017; Costea et al., 2019; Sweeney et al., 2019). BBB permeability alterations are often present before overt clinical symptoms, suggesting that BBB impairment may be a contributing factor to the pathogenesis of various neurological disorders (Figure 3). A number of studies have indicated that plasma proteins can be neurotoxic, which suggests that even if a compromised BBB does not cause these disorders it likely exacerbates them (Chodobski et al., 2011). In this section, we will review the neurological disorders most closely associated with impairments in BBB functional integrity with a focus on human data and in vivo studies.
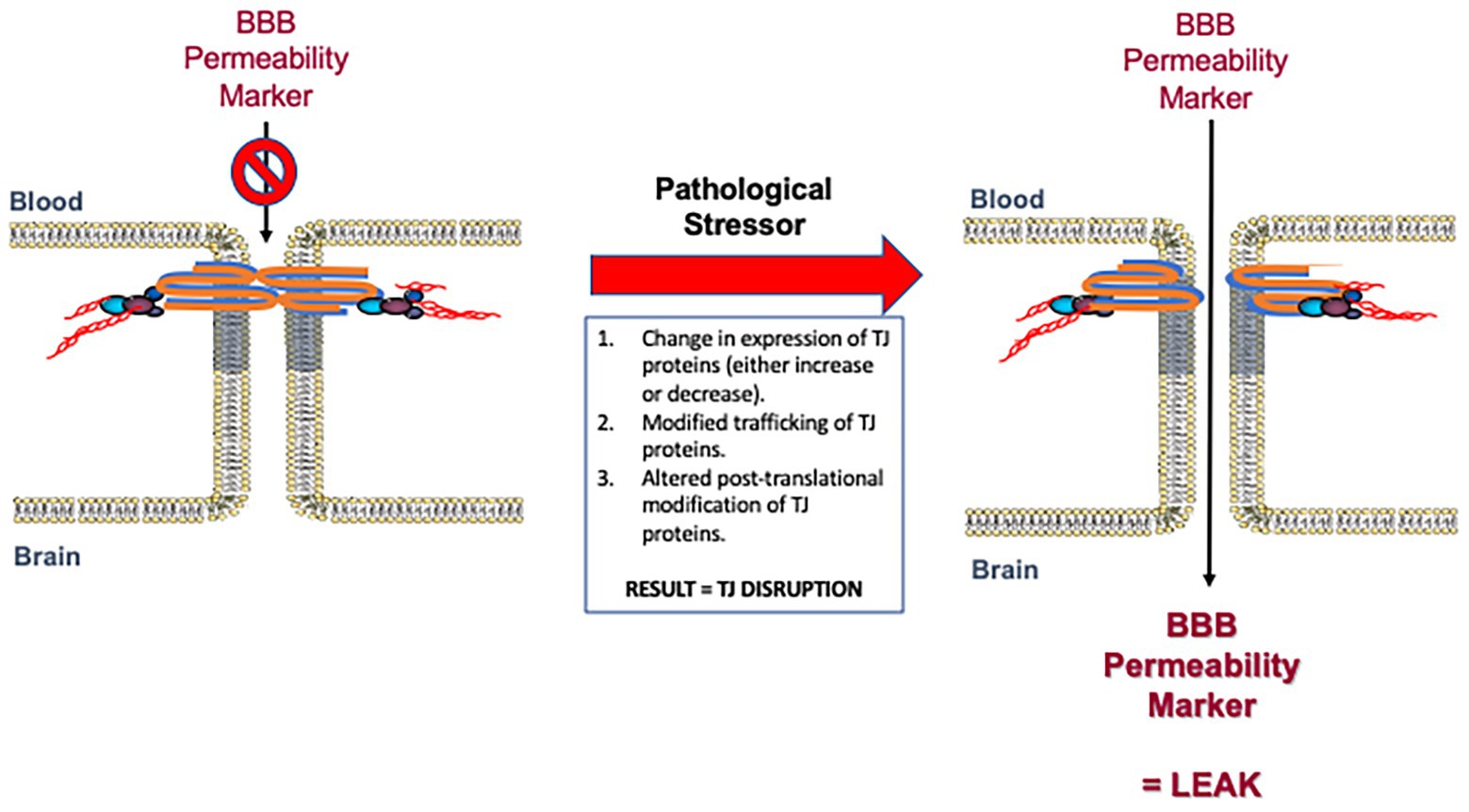
Figure 3. Disruption of blood-brain barrier (BBB) tight junction protein complexes in response to pathological stressors. Pathophysiological mechanisms can cause measurable changes in tight junction functional integrity by various mechanisms. Such mechanisms include (i) modulation in the expression of tight junction proteins as reflected by either an increase or decrease in specific protein levels; (ii) changes in trafficking of constituent proteins away from the tight junction; and/or (iii) altered post-translational modification of specific tight junction proteins. These mechanisms may occur alone or in combination to enable dynamic remodeling of the tight junction in the setting of disease. Changes in the molecular composition of tight junction protein complexes results in increased paracellular permeability (i.e., leak) to specific BBB permeability markers described in Table 1. Modified with permission from Abdullahi et al. (2018).
Alzheimer’s Disease
Alzheimer’s disease (AD) is the most common dementia disorder and is associated with substantial neurovascular dysfunction and pathology (Kisler et al., 2017). Pathological hallmarks of AD include insoluble deposits of amyloid beta (Aβ) protein in the parenchyma and within the walls of blood vessels. Additionally, AD is demarcated by neurofibrillary tangles that are comprised of hyperphosphorylated intraneuronal deposits of the microtubule-associated protein tau (Braak and Del Trecidi, 2015). A number of studies have shown increased extravasation of plasma proteins in AD brains, suggesting dysfunctional BBB properties (Paul et al., 2007; Ryu and McLarnon, 2009; Narayan et al., 2015; Llorens et al., 2019). Several postmortem studies in patients with AD have shown a significant decrease in TJ proteins claudin-5, occludin, and ZO-1 in cerebral blood vessels exhibiting cerebral amyloid angiopathy (Carrano et al., 2011, 2012; Keaney et al., 2015). In contrast, Viggars et al. (2011) showed Alzheimer-type pathology in the cortex that was not associated with downregulation of claudin-5, occludin, or ZO-1 (Viggars et al., 2011). Despite this study, it is now accepted that breakdown of the BBB is an early indicator of cognitive dysfunction and AD (Nation et al., 2019).
Studies in AD animal models display conflicting results regarding BBB permeability and TJ alterations. Tg2576 mice, which overexpress the amyloid precursor protein (APP) with the Swedish mutation (K670N and M671L) showed increased brain uptake of albumin and a fluorescein derivative following transcardial perfusion as well as increased extravasation of IgG and fibrin (Ujiie et al., 2003; Biron et al., 2011; Winkler et al., 2015). Several different laboratories have measured decreased levels of TJ proteins, such as claudin-1, claudin-5, ZO-1, and/or occludin in brain microvessels of Tg2576 mice (Biron et al., 2011; Hartz et al., 2012; Keaney et al., 2015; Winkler et al., 2015). Immunofluorescence staining of ZO-1 and occludin in Tg2576 mice displayed punctate, discontinuous, or interrupted immunoreactivity in the cerebrovasculature, suggesting compromised TJ integrity at the BBB (Biron et al., 2011). Interestingly, knockdown of claudin-5 and occludin at the BBB with small interfering RNA (siRNA) facilitated clearance of Aβ from the brain to the blood, suggesting that targeting TJ function can have beneficial effects in Tg2576 mice (Keaney et al., 2015). Ultrastructural analysis of the brain vasculature in 5XFAD mice [which express mutant human APP with the Swedish mutation, Florida mutation (I716V), and London mutation (V717I), as well as human presenilin-1 harboring two familial AD mutations (M146L and L286V)] showed TJs that were significantly shorter (Kook et al., 2012). In contrast to these findings, Bien-Ly et al. (2015) found no changes in BBB permeability to radiolabeled tracers ranging in size from 86 Da to 150 kDa in TauPS2APP mice, which express the Swedish APP mutation, the German presenilin-2 mutation (N141I), and overexpress tau (Bien-Ly et al., 2015). In these studies, no changes in claudin-5 levels were found in TauPS2APP mice or AD brain lysates. Although TJ proteins or integrity were not analyzed, studies in 3xTG-AD mice (which express the APP Swedish mutation), mutated tau (P301L), and mutated presenilin-1 (M146V) also showed no changes in BBB permeability (Bourasset et al., 2009; Mehta et al., 2013). Discrepancies in observed TJ alterations and BBB permeability in the described studies may be due to the presence and/or severity of cerebral amyloid angiopathy (CAA) in AD cases and the animal models used.
Epilepsy
A number of BBB impairments have been shown in epilepsy, which exhibits abnormal brain activity leading to seizures (Loscher and Friedman, 2020). IgG extravasation and loss of ZO-1 immunostaining have been observed postmortem in both human brain tissue derived from patients with a positive diagnosis of temporal lobe epilepsy and a rat model of limbic epilepsy. IgG leakage and ZO-1 alterations were observed early after status epilepticus (SE) as well as during latent and chronic periods (Rigau et al., 2007). As demonstrated by van Vliet et al. (2007), albumin immunoreactivity around blood vessels was observed in brain tissue from patients who died during SE (van Vliet et al., 2007). This same study showed increased BBB permeability to albumin in a rat model of SE during the latent and chronic epileptic phases, and these changes were positively correlated to seizure frequency. Hyperosmotic opening of the BBB has also been shown to lead to seizures in animals and humans, suggesting that BBB disruption may lead to progression of epilepsy (Marchi et al., 2007; van Vliet et al., 2007). In a rat model of SE, brain capillaries were more permeable to Texas Red, and seizures were associated with a decrease in the TJ proteins claudin-1, claudin-5, occludin, and ZO-1 at the BBB (Rempe et al., 2018). The same study demonstrated that seizure-induced glutamate release results in cytosolic phospholipase A2 activation, which leads to barrier leakage by activating the TJ degrading enzymes matrix metalloproteinase (MMP)-2 and MMP-9.
Hypoxia/Ischemia
Hypoxia is a central component of cerebral ischemia, which is a restriction in blood supply to the CNS typically caused by a thrombus or embolism. Both hypoxia and ischemia have been shown to exhibit an increase in BBB permeability associated with TJ alterations (Sandoval and Witt, 2008; Prakash and Carmichael, 2015; Lochhead et al., 2017; Abdullahi et al., 2018). This increase in BBB permeability may be either continuous and monophasic or biphasic with an early phase occurring during the first few hours after the onset of hypoxia/ischemia and a later phase, which occurs up to several days after the hypoxic or ischemic insult (Kuroiwa et al., 1985; Witt et al., 2008; Prakash and Carmichael, 2015; Merali et al., 2017). The time course and degree of BBB permeability alterations during ischemia are highly dependent on the species and detection methods as well as the type, degree, and duration of occlusion (Prakash and Carmichael, 2015).
We have shown in female Sprague-Dawley rats that, within 20 min of reoxygenation after an acute hypoxic insult [6% oxygen (O2) for 1 h], the permeability of the BBB increases to (14C)-sucrose (~340 Da). Of particular note, permeability to Evans blue pre-adsorbed to albumin (~80 kDa) was not altered, which suggests that acute hypoxia leads to BBB permeability changes to small molecules but not macromolecules (Witt et al., 2003; Lochhead et al., 2010). Associated with increased BBB permeability were changes in the localization of the TJ proteins occludin, claudin-5, and ZO-1 as well as disruption of high molecular weight oligomers of occludin (McCaffrey et al., 2009; Lochhead et al., 2010; Willis et al., 2010). These changes could be prevented by administration of the antioxidant tempol or the protein kinase C (PKC) inhibitor chelerythrine chloride, suggesting that generation of reactive oxygen species (ROS) and activation of PKC are involved in altering BBB paracellular permeability during acute hypoxia-reoxygenation stress (Lochhead et al., 2010; Willis et al., 2010). In mice exposed to 8% O2 for 48 h, an increase in BBB permeability to sodium fluorescein was associated with a significant reduction in occludin expression and changes in localization of occludin and ZO-1 (Bauer et al., 2010). These changes could be prevented by inhibition of vascular endothelial growth factor (VEGF) or MMP-9.
Disruption of the BBB is a well-established feature of ischemic stroke which contributes to brain injury, and increases the likelihood of hemorrhagic transformation (Sifat et al., 2017; Jiang et al., 2018). While some studies have suggested that extravasation in stroke models is due to an increase in brain endothelial transcytosis through vesicles or non-selective channels (Knowland et al., 2014; Krueger et al., 2015; De Bock et al., 2016), many other studies have demonstrated that increased BBB permeability during ischemia is associated with TJ alterations. Jiao et al. (2011) found that BBB permeability to Evans blue was increased during 3–72 h reperfusion after a 2 h middle cerebral artery occlusion (MCAO) in rats, and these changes were associated with observable gaps in TJ complexes and significant decreases in claudin-5, occludin, and ZO-1 (Jiao et al., 2011). In a permanent MCAO model, there was a biphasic increase in BBB permeability to Evans blue up to 120 h, which correlated with a significant decrease in claudin-5 and occludin (Liu et al., 2018). Recently it was shown that mRNA expression levels of claudin-1, claudin-3, claudin-12, and occludin were significantly decreased, ZO-1 mRNA levels displayed no changes, and claudin-5 mRNA was upregulated in the ipsilateral hemisphere vs. the contralateral hemisphere after 3 h of reperfusion following MCAO (Winkler et al., 2020). This same study found a significant decrease in TJ length and, interestingly, that claudin-3 knockout actually decreased the infarct size and edema formation.
Several different mechanisms have been suggested to contribute to TJ alterations and BBB disruption in experimental stroke models. A number of studies have shown that alterations in BBB integrity during ischemia are due to activation of MMPs, which are capable of degrading TJ and extracellular matrix components. MMP-9 knockout mice displayed significantly reduced Evans blue extravasation and ZO-1 downregulation, but no changes in occludin, in a transient MCAO model (Asahi et al., 2001). After transient MCAO in spontaneously hypertensive rats, an increase in BBB permeability to (14C)-sucrose and degradation of claudin-5 and ZO-1 were inhibited by the broad spectrum MMP inhibitor (BB-1101; Yang et al., 2007). Gu et al. (2012) showed that MMP activation was the result of downregulation of caveolin-1 by nitric oxide, and inhibition of nitric oxide synthase by L-NAME could reduce Evans blue leakage and ZO-1 downregulation during transient MCAO in rats (Gu et al., 2012). After photothrombotic ischemia in caveolin-1 knockout mice, Evans blue extravasation and MMP activity are significantly increased while claudin-5, occludin, and ZO-1 expression are significantly decreased (Choi et al., 2016). Progesterone or allopregnanolone has been shown to reduce Evans blue extravasation, MMP-2 and MMP-9 activation, and claudin-5 and occludin downregulation when administered 1 h post-occlusion to rats (Ishrat et al., 2010). Induction of systemic inflammation by peripheral administration of interleukin-1β has been shown to cause sustained BBB disruption, MMP-9 activation, and claudin-5, but not occludin, rearrangement in cerebral vessels after transient MCAO in mice (McColl et al., 2008). Administration of angiopoietin-1 reduced Evans blue extravasation and downregulation of occludin and ZO-1 for up to 1 week, following MCAO in rats (Yu et al., 2012).
Huntington’s Disease
Huntington’s disease (HD) is a neurodegenerative disorder caused by an autosomal dominant mutation, which results in movement, cognitive, and psychiatric problems. Mutant Huntington protein has been shown to aggregate in the blood vessels of the caudate nucleus in HD patients and in brain tissue from R6/2 mice, a preclinical HD model (Drouin-Ouellet et al., 2015). MRI studies in HD patients showed an increase BBB permeability to Gd-DTPA (~550 Da), and postmortem analysis showed an increase in fibrin deposition in the brain parenchyma associated with a significant reduction in occludin and claudin-5 expression at the BBB (Drouin-Ouellet et al., 2015). Induced pluripotent stem cell-derived brain endothelial cells from HD patients displayed lower TEER values and alterations in claudin-5 localization (Lim et al., 2017). One study in R6/2 mice showed evidence of an increase in BBB permeability through both the transcellular and paracellular pathways, wider intercellular clefts of TJs, and a reduction in occludin and claudin-5 at the BBB (Drouin-Ouellet et al., 2015). Another study showed an age-dependent increase in BBB permeability to fluorescein isothiocyanate (FITC)-albumin and a reduction in claudin-5, occludin, and ZO-1 mRNA and claudin-5 protein levels in the cortex and striatum (Di Pardo et al., 2017).
Multiple Sclerosis
Multiple sclerosis (MS) is a chronic, progressive CNS disorder, in which the immune system attacks myelinated fibers which causes inflammation, scar tissue, or lesions. BBB disruption during MS is thought to influence oligodendrocyte death, axonal damage, demyelination, and lesion development (Alvarez et al., 2011). Breakdown of the BBB identified by gadolinium-enhanced MRI has been shown to be one of the earliest detectable events in the development of most new MS lesions (Kermode et al., 1990). Vascular TJ morphological alterations and extravasation of serum proteins are frequently observed in areas of MS lesions, but are less common in normal appearing white matter and gray matter (Plumb et al., 2002; Kirk et al., 2003; van Horssen et al., 2007; Wosik et al., 2007). Abnormal tight junctions in both secondary progressive MS and primary progressive MS are most frequently observed in active white matter lesions but also persist in inactive lesions (Leech et al., 2007).
Many studies conducted in experimental autoimmune encephalomyelitis (EAE), an in vivo model of demyelinating diseases, have shown TJ alterations that correlate with changes in BBB permeability. Although imperfect, the EAE model does display some similarities in CNS pathologies with MS and, therefore, is commonly used as an animal model to study MS-associated mechanisms. Wolburg et al. (2003) showed that during EAE there was a selective loss of claudin-3, but not claudin-5 or occludin, at the BBB (Wolburg et al., 2003). In contrast to these findings, Errede et al. (2012) found that EAE causes claudin-5 to exhibit a punctate staining pattern early in the disease process while occludin moved away from the TJ and showed diffuse cytoplasmic staining (Errede et al., 2012). Another study in an EAE model observed a relocalization of ZO-1, which preceded overt clinical disease and correlated with sites of inflammatory cell accumulation (Bennett et al., 2010). Wang et al. (2016) found a significant increase in BBB permeability to Evans blue that coincided with a decrease in claudin-5, occludin, and ZO-1 levels in brain homogenates of EAE mice, and these BBB alterations could be ameliorated by resveratrol (Wang et al., 2016). Huang et al. (2017) showed similar alterations in BBB permeability, and claudin-5, occludin, and ZO-1 expression levels in cerebellar white matter could be prevented by a Kv1.3 channel blocker in EAE rats (Huang et al., 2017). Claudin-11 protein expression has also been shown to be significantly downregulated in MS patients and in EAE (Uchida et al., 2019). Differences in TJ alteration data between different groups may be due to differences in the specific EAE model, the time points examined, or the tissue in which the analysis was performed.
Pain
Pain is an unpleasant sensory and emotional experience associated with actual or potential tissue damage, or described in terms of such damage. Using in situ brain perfusion, we have shown in several models of peripheral inflammatory pain (PIP) that brain uptake increases to (14C)-sucrose, but not to Evans blue-albumin, suggesting that inflammatory pain alters BBB paracellular permeability to small molecules but not macromolecules (Huber et al., 2001; Lochhead et al., 2012). These changes are biphasic in nature and occur within the first 6 h and again at 48 in the hind paw λ-carrageenan model of PIP (Huber et al., 2002). The early phase of these alterations could be prevented or reversed by administration of the non-steroidal anti-inflammatory diclofenac, transforming growth factor-β (TGF-β), tempol, or perineural injection of bupivacaine into the right hind leg, suggesting a role for cyclooxygenase, TGF-β signaling, ROS generation, and nociceptive input in PIP-induced BBB disruption (Brooks et al., 2008; Campos et al., 2008; Ronaldson et al., 2009; Lochhead et al., 2012). Associated with the increase in BBB permeability during PIP are alterations in the expressions of claudin-3, claudin-5, occludin, and ZO-1 as well as disruption of high molecular weight occludin oligomers (Huber et al., 2002; Brooks et al., 2005, 2008; McCaffrey et al., 2008; Ronaldson et al., 2009; Lochhead et al., 2012). At 3 h after induction of PIP, we have observed ROS-mediated disruption of occludin oligomeric assemblies and altered trafficking of occludin (McCaffrey et al., 2008; Lochhead et al., 2012).
In addition to PIP, BBB paracellular permeability has been observed to increase during familial hemiplegic migraine and in animal models of migraine (Gursoy-Ozdemir et al., 2004; Dreier et al., 2005; Olah et al., 2013). In a model of cortical spreading depression (CSD), Evans blue and plasma protein extravasation was associated with an increase in MMP-9 expression and activity and a decrease in ZO-1 expression in brain microvessels (Gursoy-Ozdemir et al., 2004). These changes could be prevented by the MMP inhibitor GM6001 within 3–24 h. In another study of CSD, brain uptake of (14C)-sucrose was observed beginning at 30 min with no changes in claudin-5 or occludin expression (Cottier et al., 2018).
Parkinson’s Disease
Parkinson’s disease (PD) is a neurodegenerative disorder, which affects movement. Evidence of BBB disruption in PD includes the findings of significant increase in albumin and IgG in the cerebrospinal fluid of PD patients (Pisani et al., 2012) and an increase in extravasation of erythrocytes, hemoglobin, and fibrin in the striatum of PD patients (Gray and Woulfe, 2015). Immunofluorecence staining of ZO-1 and occludin is also significantly reduced in the substantia nigra of PD patients, and these findings correlate with an increase in parenchymal IgG (Pienaar et al., 2015). Interestingly, these changes were attenuated in patients subjected to deep brain stimulation. The 6-hydroxydopamine (6-OHDA) preclinical model of PD showed BBB leakage in the substantia nigra and striatum 10 and 34 days after injection of 6-OHDA into the striatum (Carvey et al., 2005). Also observed in the 6-OHDA model was a significant reduction in the TJ proteins ZO-1 and claudin-5 (Huang et al., 2016). In the 1-methyl-4-phenyl-1,2,3,6-tetrahydropyridine (MPTP) mouse model of PD, an increase in BBB permeability to Evans blue and FITC-albumin was observed in the striatum, but not the hippocampus (Chen et al., 2008). These changes correlated with significant decreases of occludin and ZO-1, and could be prevented by pre-administration of caffeine. Other studies in the MPTP model have shown that decreased levels of occludin or ZO-1 could be attenuated by acetyl-L-carnitine or sodium butyrate (Liu et al., 2017; Burks et al., 2019).
Traumatic Brain Injury
Traumatic brain injury (TBI) is a complex, dynamic, and heterogenous pathology which can be classified as impact or non-impact, focal or diffuse, mild, moderate, or severe. A compromised BBB is a well-established pathological feature of TBI (Saw et al., 2014; Alluri et al., 2015; Prakash and Carmichael, 2015; Rodriguez-Grande et al., 2017). Intracranial hemorrhage represents the most serious complication of TBI and can be detected in moderate to severe cases (Narayan et al., 2008; Currie et al., 2016). In pre-clinical models of TBI, BBB disruption can be monophasic or biphasic in nature and can occur within minutes to days after injury similar to what is observed in ischemic stroke models (Shapira et al., 1993; Baldwin et al., 1996; Barzo et al., 1996). Interestingly, in the mouse cerebral contusion model, BBB permeability to horseradish peroxidase (44 kDa) was seen up to 5 h post-injury while permeability to smaller molecules (~0.3–10 kDa) can be measured up to 4 days, suggesting that TBI can cause size-selective BBB leakage (Habgood et al., 2007). TJ proteins such as claudin-5, occludin, and ZO-1 are downregulated after the first few days of TBI but can be upregulated 1–2 weeks after injury when the BBB regains functional integrity (Lin et al., 2010; Wen et al., 2014). In addition to paracellular alterations, transcellular permeability across the BBB may also be increased after TBI (Castejon, 1984; Nag et al., 2007). Of particular note, Ren et al. (2013) showed increased Evan’s blue albumin extravasation at 24 h following moderate closed skull TBI in male C57Bl/6 mice, suggesting that even less severe trauma can lead to measurement BBB disruption (Ren et al., 2013). A number of different mechanisms have been suggested to contribute to BBB dysfunction during TBI including TGF-β signaling, glutamate excitotoxicity, generation of ROS, activation of MMPs, inflammation, and VEGF signaling (Chodobski et al., 2011).
Modulation of BBB Permeability to Enhance Drug Delivery
Although the BBB plays an important protective role of the CNS, modulation of TJs may allow increased brain delivery of drugs through the paracellular route (Deli, 2009; Tscheik et al., 2013; Greene and Campbell, 2016). For example, we have shown that TJ alterations during PIP are associated with improved CNS delivery of the opioid analgesic codeine, which results in enhanced analgesia (Hau et al., 2004). Ideally, TJ alterations at the BBB would be transient in nature to allow enhanced drug permeation into the CNS without causing side effects or toxicity resulting from an open barrier. Pharmacological strategies to modulate TJs at the BBB must also exhibit low systemic toxicity as their delivery to brain endothelial cells would likely be through the bloodstream. Over the past several years, a number of different strategies have been tested to increase BBB paracellular permeability with the goal of improving drug delivery to the brain in vivo.
One strategy to increase brain delivery of drugs is intracarotid infusion of hyperosmotic concentrations of arabinose or mannitol. This technique causes reversible vasodilation and shrinkage of endothelial cells, which leads to a loosening of the TJs, an increase in paracellular diffusion, and bulk fluid flow across the BBB (Rapoport and Robinson, 1986; Rapoport, 2000; Dobrogowska and Vorbrodt, 2004). While this method has been used to enhance delivery of chemotherapeutics to brain tumors, limited efficacy and severe side effects such as brain edema and seizures have prevented its widespread use in the clinic (Rapoport, 2000; Marchi et al., 2007). In addition, its invasive nature and need for patients to be anesthetized during the procedure render the technique impractical for chronic administration of therapeutics (Marchi et al., 2007; Hersh et al., 2016).
Several different attempts to pharmacologically manipulate BBB permeability to increase brain delivery of drugs have also been attempted. BBB permeability to Evans blue was reversibly increased 15 min after intracarotid infusion of oleic acid in rats (Sztriha and Betz, 1991). Administration of oleic acid or linoleic acid to cats also caused BBB disruption as measured by MRI, but these effects were associated with brain edema, necrosis, and demyelination (Kim et al., 2005). Intravenous infusion of the bradykinin B2 receptor agonist RMP-7 (Cereport®) increased lanthanum penetration through TJs of the BBB by loosening the junctional complexes (Sanovich et al., 1995). RMP-7 also showed enhanced uptake of chemotherapeutics in preclinical brain tumor models but was ineffective when used to enhance brain delivery of carboplatin to treat childhood brain tumors in a phase II trial (Emerich et al., 2001; Warren et al., 2006). Administration of alkylglycerols has also been shown to increase BBB permeability of a number of different drugs and tracers (Iannitti and Palmieri, 2010). Intracarotid administration of alkylglycerols induce a size-dependent permeability increase, suggesting that alkylglycerols increase BBB paracellular permeability by modulating TJs, but intracarotid administration is an invasive procedure, and alkylglycerol administration to increase CNS drug uptake has not been tested clinically (Erdlenbruch et al., 2003). A 12 kDa fragment of the ZO toxin (ΔG) from vibrio cholerae has been shown to increase BBB to (14C)-sucrose, likely by activating PKC (Fasano et al., 1995; Menon et al., 2005). These effects were modest without the addition of protease inhibitors, suggesting that ΔG is rapidly degraded in the bloodstream and likely not a good candidate to clinically improve drug delivery to the CNS (Menon et al., 2005).
Another strategy to transiently increase BBB paracellular permeability is by targeting specific components of the TJs with siRNA or novel peptidomimetic drugs. Campbell et al. (2008) showed that BBB paracellular permeability could be significantly increased up to 48 h after tail vein injection of siRNA targeting claudin-5 in mice (Campbell et al., 2008). This technique led to enhanced brain delivery of Gd-DTPA (742 Da), but not to FITC-dextran (4.4 kDa), showing a size-selective barrier alteration when targeting claudin-5 similar to what is seen in claudin-5 knockout mice. More recently, Dithmer et al. (2017) reported that newly engineered peptidomimetic drugs targeting claudin-5 could increase paracellular BBB permeability to various tracers across a wide molecular weight range such as lucifer yellow (457 Da), FITC-dextran (10 and 40 kDa), FITC-albumin (67 kDa), and tetramethylrhodamine-dextran (155 kDa) (Dithmer et al., 2017). These claudin-5 targeting peptides were also shown to increase CNS distribution of gadolinium 4 h following their administration, suggesting a novel approach for transient and reversal opening of the BBB for optimization of drug delivery (Dithmer et al., 2017).
Ultrasound in the presence of microbubbles is a technique that is increasingly being used to create a transient and focal opening of the BBB to deliver therapeutics to the CNS ranging in size from small molecules to antibodies, plasmids, and viral vectors. Focused ultrasound (FUS) bursts generated from an external source are able to utilize microbubbles as contrast agents that act as cavitation sites, which physically disrupt the BBB (Alonso, 2015; McMahon et al., 2019). Increased BBB permeability induced by ultrasound may involve transcytosis, opening of transendothelial channels, passage through injured endothelial cells, or opening of TJs (Sheikov et al., 2004). Studies in rats showed that FUS with microbubbles could increase BBB permeability to horseradish peroxidase and lanthanum. This BBB disruption was associated with ultrastructural alterations of claudin-5, occludin, and ZO-1, suggesting that FUS can increase BBB paracellular permeability by altering TJ proteins (Sheikov et al., 2008). Clinical trials utilizing this technique to improve CNS drug delivery are currently underway.
Conclusions
TJ protein complexes are the major determinants of paracellular permeability at the BBB. While brain microvascular endothelial TJs function to protect the CNS from potentially harmful substances in the blood, their protective role also represents a major obstacle to effectively deliver therapeutics for treatment of neurological diseases. Evidence is increasingly emerging that pathological alterations of TJs at the BBB may exacerbate or possibly initiate neurological dysfunction. A better understanding of how TJ expression, localization, and function is altered at the BBB during disease states may provide opportunities to protect the CNS from adverse consequences resulting from BBB disruption. Conversely, understanding the mechanisms involved in rapidly and transiently regulating TJ function at the BBB may allow improved delivery of therapeutics to the CNS through the paracellular route. Future research elucidating signaling pathways affecting TJ function at the BBB has the potential to both protect the CNS from a compromised barrier and more effectively treat neurological disorders by optimizing drug delivery.
Author Contributions
JL and JY wrote the paper. PR and JL created the figures and tables. PR and TD edited the paper. All authors contributed to the article and approved the submitted version.
Funding
This work was supported by the American Heart Association (18CDA34110454 to JL), and the National Institutes of Health (NS 42652-17 and DA 51812-01 to TD NS 84941-06 to PR).
Conflict of Interest
The authors declare that the research was conducted in the absence of any commercial or financial relationships that could be construed as a potential conflict of interest.
Supplementary Material
The Supplementary Material for this article can be found online at: https://www.frontiersin.org/articles/10.3389/fphys.2020.00914/full#supplementary-material.
References
Abdullahi, W., Davis, T. P., and Ronaldson, P. T. (2017). Functional expression of p-glycoprotein and organic anion transporting polypeptides at the blood-brain barrier: understanding transport mechanisms for improved CNS drug delivery? AAPS J. 19, 931–939. doi: 10.1208/s12248-017-0081-9
Abdullahi, W., Tripathi, D., and Ronaldson, P. T. (2018). Blood-brain barrier dysfunction in ischemic stroke: targeting tight junctions and transporters for vascular protection. Am. J. Phys. Cell Physiol. 315, C343–C356. doi: 10.1152/ajpcell.00095.2018
Agundez, J. A., Jimenez-Jimenez, F. J., Alonso-Navarro, H., and Garcia-Martin, E. (2014). Drug and xenobiotic biotransformation in the blood-brain barrier: a neglected issue. Front. Cell. Neurosci. 8:335. doi: 10.3389/fncel.2014.00335
Alluri, H., Wiggins-Dohlvik, K., Davis, M. L., Huang, J. H., and Tharakan, B. (2015). Blood-brain barrier dysfunction following traumatic brain injury. Metab. Brain Dis. 30, 1093–1104. doi: 10.1007/s11011-015-9651-7
Alonso, A. (2015). Ultrasound-induced blood-brain barrier opening for drug delivery. Front. Neurol. Neurosci. 36:106. doi: 10.1159/000366242
Alvarez, J. I., Cayrol, R., and Prat, A. (2011). Disruption of central nervous system barriers in multiple sclerosis. Biochim. Biophys. Acta 1812, 252–264. doi: 10.1016/j.bbadis.2010.06.017
Amasheh, S., Schmidt, T., Mahn, M., Florian, P., Mankertz, J., Tavalali, S., et al. (2005). Contribution of claudin-5 to barrier properties in tight junctions of epithelial cells. Cell Tissue Res. 321, 89–96. doi: 10.1007/s00441-005-1101-0
Asahi, M., Wang, X., Mori, T., Sumii, T., Jung, J. C., Moskowitz, M. A., et al. (2001). Effects of matrix metalloproteinase-9 gene knock-out on the proteolysis of blood-brain barrier and white matter components after cerebral ischemia. J. Neurosci. 21, 7724–7732. doi: 10.1523/JNEUROSCI.21-19-07724.2001
Balda, M. S., Flores-Maldonado, C., Cereijido, M., and Matter, K. (2000). Multiple domains of occludin are involved in the regulation of paracellular permeability. J. Cell. Biochem. 78, 85–96. doi: 10.1002/(SICI)1097-4644(20000701)78:1<85::AID-JCB8>3.0.CO;2-F
Baldwin, S. A., Fugaccia, I., Brown, D. R., Brown, L. V., and Scheff, S. W. (1996). Blood-brain barrier breach following cortical contusion in the rat. J. Neurosurg. 85, 476–481. doi: 10.3171/jns.1996.85.3.0476
Bamforth, S. D., Kniesel, U., Wolburg, H., Engelhardt, B., and Risau, W. (1999). A dominant mutant of occludin disrupts tight junction structure and function. J. Cell Sci. 112, 1879–1888.
Banks, W. A. (2009). Characteristics of compounds that cross the blood-brain barrier. BMC Neurol. 9:S3. doi: 10.1186/1471-2377-9-S1-S3
Banks, W. A., and Greig, N. H. (2019). Small molecules as central nervous system therapeutics: old challenges, new directions, and a philosophic divide. Future Med. Chem. 11, 489–493. doi: 10.4155/fmc-2018-0436
Barzo, P., Marmarou, A., Fatouros, P., Corwin, F., and Dunbar, J. (1996). Magnetic resonance imaging-monitored acute blood-brain barrier changes in experimental traumatic brain injury. J. Neurosurg. 85, 1113–1121. doi: 10.3171/jns.1996.85.6.1113
Bauer, A. T., Burgers, H. F., Rabie, T., and Marti, H. H. (2010). Matrix metalloproteinase-9 mediates hypoxia-induced vascular leakage in the brain via tight junction rearrangement. J. Cereb. Blood Flow Metab. 30, 837–848. doi: 10.1038/jcbfm.2009.248
Bauer, H. -C., Krizbai, I. A., Bauer, H., and Traweger, A. (2014). “You Shall Not Pass”-tight junctions of the blood brain barrier. Front. Neurosci. 8:392. doi: 10.3389/fnins.2014.00392
Bazzoni, G., Martinez-Estrada, O. M., Orsenigo, F., Cordenonsi, M., Citi, S., and Dejana, E. (2000). Interaction of junctional adhesion molecule with the tight junction components ZO-1, cingulin, and occludin. J. Biol. Chem. 275, 20520–20526. doi: 10.1074/jbc.M905251199
Bellmann, C., Schreivogel, S., Günther, R., Dabrowski, S., Schümann, M., Wolburg, H., et al. (2014). Highly conserved cysteines are involved in the oligomerization of occludin-redox dependency of the second extracellular loop. Antioxid. Redox Signal. 20, 855–867. doi: 10.1089/ars.2013.5288
Bennett, J., Basivireddy, J., Kollar, A., Biron, K. E., Reickmann, P., Jefferies, W. A., et al. (2010). Blood-brain barrier disruption and enhanced vascular permeability in the multiple sclerosis model EAE. J. Neuroimmunol. 229, 180–191. doi: 10.1016/j.jneuroim.2010.08.011
Berndt, P., Winkler, L., Cording, J., Breitkreuz-Korff, O., Rex, A., Dithmer, S., et al. (2019). Tight junction proteins at the blood–brain barrier: far more than claudin-5. Cell. Mol. Life Sci. 76, 1987–2002. doi: 10.1007/s00018-019-03030-7
Bhattacharjee, A. K., Nagashima, T., Kondoh, T., and Tamaki, N. (2001). Quantification of early blood-brain barrier disruption by in situ brain perfusion technique. Brain Res. Brain Res. Protoc. 8, 126–131. doi: 10.1016/S1385-299X(01)00094-0
Bien-Ly, N., Boswell, C. A., Jeet, S., Beach, T. G., Hoyte, K., Luk, W., et al. (2015). Lack of widespread BBB disruption in alzheimer’s disease models: focus on therapeutic antibodies. Neuron 88, 289–297. doi: 10.1016/j.neuron.2015.09.036
Biron, K. E., Dickstein, D. L., Gopaul, R., and Jefferies, W. A. (2011). Amyloid triggers extensive cerebral angiogenesis causing blood brain barrier permeability and hypervascularity in Alzheimer’s disease. PLoS One 6:e23789. doi: 10.1371/journal.pone.0023789
Blasig, I. E., Winkler, L., Lassowski, B., Mueller, S. L., Zuleger, N., Krause, E., et al. (2006). On the self-association potential of transmembrane tight junction proteins. Cell. Mol. Life Sci. 63, 505–514. doi: 10.1007/s00018-005-5472-x
Bourasset, F., Ouellet, M., Tremblay, C., Julien, C., Do, T. M., Oddo, S., et al. (2009). Reduction of the cerebrovascular volume in a transgenic mouse model of Alzheimer’s disease. Neuropharmacology 56, 808–813. doi: 10.1016/j.neuropharm.2009.01.006
Braak, H., and Del Trecidi, K. (2015). Neuroanatomy and pathology of sporadic Alzheimer’s disease. Adv. Anat. Embryol. Cell Biol. 215, 1–162. doi: 10.1007/978-3-540-79850-7
Brightman, M. W., and Reese, T. S. (1969). Junctions between intimately apposed cell membranes in the vertebrate brain. J. Cell Biol. 40, 648–677. doi: 10.1083/jcb.40.3.648
Brooks, T. A., Hawkins, B. T., Huber, J. D., Egleton, R. D., and Davis, T. P. (2005). Chronic inflammatory pain leads to increased blood-brain barrier permeability and tight junction protein alterations. Am. J. Physiol. Heart Circ. Physiol. 289, H738–H743. doi: 10.1152/ajpheart.01288.2004
Brooks, T. A., Nametz, N., Charles, R., and Davis, T. P. (2008). Diclofenac attenuates the regional effect of lambda-carrageenan on blood-brain barrier function and cytoarchitecture. J. Pharmacol. Exp. Ther. 325, 665–673. doi: 10.1124/jpet.107.135632
Burks, S., Raymick, J., Robinson, B., Hanig, J., and Sarkar, S. (2019). Neuroprotective effects of acetyl-L-carnitine (ALC) in a chronic MPTP-induced Parkinson’s disease mouse model: endothelial and microglial effects. Neurosci. Lett. 703, 86–95. doi: 10.1016/j.neulet.2019.03.015
Buschmann, M. M., Shen, L., Rajapakse, H., Raleigh, D. R., Wang, Y., Wang, Y., et al. (2013). Occludin OCEL-domain interactions are required for maintenance and regulation of the tight junction barrier to macromolecular flux. Mol. Biol. Cell 24, 3056–3068. doi: 10.1091/mbc.e12-09-0688
Butt, A. M., Jones, H. C., and Abbott, N. J. (1990). Electrical resistance across the blood-brain barrier in anaesthetized rats: a developmental study. J. Physiol. 429, 47–62. doi: 10.1113/jphysiol.1990.sp018243
Campbell, M., Kiang, A. S., Kenna, P. F., Kerskens, C., Blau, C., O’Dwyer, L., et al. (2008). RNAi-mediated reversible opening of the blood-brain barrier. J. Gene Med. 10, 930–947. doi: 10.1002/jgm.1211
Campos, C. R., Ocheltree, S. M., Hom, S., Egleton, R. D., and Davis, T. P. (2008). Nociceptive inhibition prevents inflammatory pain induced changes in the blood-brain barrier. Brain Res. 1221, 6–13. doi: 10.1016/j.brainres.2008.05.013
Carrano, A., Hoozemans, J. J., van der Vies, S. M., Rozemuller, A. J., van Horssen, J., and de Vries, H. E. (2011). Amyloid beta induces oxidative stress-mediated blood-brain barrier changes in capillary amyloid angiopathy. Antioxid. Redox Signal. 15, 1167–1178. doi: 10.1089/ars.2011.3895
Carrano, A., Hoozemans, J. J., van der Vies, S. M., van Horssen, J., de Vries, H. E., and Rozemuller, A. J. (2012). Neuroinflammation and blood-brain barrier changes in capillary amyloid angiopathy. Neurodegener. Dis. 10, 329–331. doi: 10.1159/000334916
Carvey, P. M., Zhao, C. H., Hendey, B., Lum, H., Trachtenberg, J., Desai, B. S., et al. (2005). 6-Hydroxydopamine-induced alterations in blood-brain barrier permeability. Eur. J. Neurosci. 22, 1158–1168. doi: 10.1111/j.1460-9568.2005.04281.x
Castejon, O. J. (1984). Increased vesicular and vacuolar transport in traumatic human brain edema. A combined electron microscopic study and theoretical approach. J. Submicrosc. Cytol. 16, 359–369. doi: 10.5114/fn.2013.35951
Castro Dias, M., Coisne, C., Baden, P., Enzmann, G., Garrett, L., Becker, L., et al. (2019). Claudin-12 is not required for blood-brain barrier tight junction function. Fluids Barriers CNS 16:30. doi: 10.1186/s12987-019-0150-9
Chattopadhyay, R., Dyukova, E., Singh, N. K., Ohba, M., Mobley, J. A., and Rao, G. N. (2014). Vascular endothelial tight junctions and barrier function are disrupted by 15(S)-hydroxyeicosatetraenoic acid partly via protein kinase C ε-mediated zona occludens-1 phosphorylation at threonine 770/772. J. Biol. Chem. 289, 3148–3163. doi: 10.1074/jbc.M113.528190
Chen, X., Lan, X., Roche, I., Liu, R., and Geiger, J. D. (2008). Caffeine protects against MPTP-induced blood-brain barrier dysfunction in mouse striatum. J. Neurochem. 107, 1147–1157. doi: 10.1111/j.1471-4159.2008.05697.x
Chiba, H., Osanai, M., Murata, M., Kojima, T., and Sawada, N. (2008). Transmembrane proteins of tight junctions. Biochim. Biophys. Acta 1778, 588–600. doi: 10.1016/j.bbamem.2007.08.017
Chodobski, A., Zink, B. J., and Szmydynger-Chodobska, J. (2011). Blood-brain barrier pathophysiology in traumatic brain injury. Transl. Stroke Res. 2, 492–516. doi: 10.1007/s12975-011-0125-x
Choi, K. H., Kim, H. S., Park, M. S., Kim, J. T., Kim, J. H., Cho, K. A., et al. (2016). Regulation of caveolin-1 expression determines early brain edema after experimental focal cerebral ischemia. Stroke 47, 1336–1343. doi: 10.1161/STROKEAHA.116.013205
Costea, L., Meszaros, A., Bauer, H., Bauer, H. C., Traweger, A., Wilhelm, I., et al. (2019). The blood-brain barrier and its intercellular junctions in age-related brain disorders. Int. J. Mol. Sci. 20:5472. doi: 10.3390/ijms20215472
Cottier, K. E., Galloway, E. A., Calabrese, E. C., Tome, M. E., Liktor-Busa, E., Kim, J., et al. (2018). Loss of blood-brain barrier integrity in a KCL-induced model of episodic headache enhances CNS drug delivery. eNeuro 5, ENEURO.0116–ENEURO.18.2018. doi: 10.1523/ENEURO.0116-18.2018
Crone, C., and Olesen, S. P. (1982). Electrical resistance of brain microvascular endothelium. Brain Res. 241, 49–55. doi: 10.1016/0006-8993(82)91227-6
Currie, S., Saleem, N., Straiton, J. A., Macmullen-Price, J., Warren, D. J., and Craven, I. J. (2016). Imaging assessment of traumatic brain injury. Postgrad. Med. J. 92, 41–50. doi: 10.1136/postgradmedj-2014-133211
Daneman, R., Zhou, L., Agalliu, D., Cahoy, J. D., Kaushal, A., and Barres, B. A. (2010). The mouse blood-brain barrier transcriptome: a new resource for understanding the development and function of brain endothelial cells. PLoS One 5:e13741. doi: 10.1371/journal.pone.0013741
De Bock, M., Van Haver, V., Vandenbroucke, R. E., Decrock, E., Wang, N., and Leybaert, L. (2016). Into rather unexplored terrain-transcellular transport across the blood-brain barrier. Glia 64, 1097–1123. doi: 10.1002/glia.22960
Deli, M. A. (2009). Potential use of tight junction modulators to reversibly open membranous barriers and improve drug delivery. Biochim. Biophys. Acta 1788, 892–910. doi: 10.1016/j.bbamem.2008.09.016
Devraj, K., Guerit, S., Macas, J., and Reiss, Y. (2018). An in vivo blood-brain barrier permeability assay in mice using fluorescently labeled tracers. J. Vis. Exp. 132:57038. doi: 10.3791/57038
Di Pardo, A., Amico, E., Scalabri, F., Pepe, G., Castaldo, S., Elifani, F., et al. (2017). Impairment of blood-brain barrier is an early event in R6/2 mouse model of Huntington disease. Sci. Rep. 7:41316. doi: 10.1038/srep41316
Dithmer, S., Staat, C., Muller, C., Ku, M. C., Pohlmann, A., Niendorf, T., et al. (2017). Claudin peptidomimetics modulate tissue barriers for enhanced drug delivery. Ann. N. Y. Acad. Sci. 1397, 169–184. doi: 10.1111/nyas.13359
Dobrogowska, D. H., and Vorbrodt, A. W. (2004). Immunogold localization of tight junctional proteins in normal and osmotically-affected rat blood-brain barrier. J. Mol. Histol. 35, 529–539. doi: 10.1007/10.1007/s10735-004-1318-3
Doerfel, M., and Huber, O. (2012). Modulation of tight junction structure and function by kinases and phosphatases targeting occludin. J. Biomed. Biotechnol. 2012:807356. doi: 10.1155/2012/807356
Dreier, J. P., Jurkat-Rott, K., Petzold, G. C., Tomkins, O., Klingebiel, R., Kopp, U. A., et al. (2005). Opening of the blood-brain barrier preceding cortical edema in a severe attack of FHM type II. Neurology 64, 2145–2147. doi: 10.1212/01.WNL.0000176298.63840.99
Drouin-Ouellet, J., Sawiak, S. J., Cisbani, G., Lagace, M., Kuan, W. L., Saint-Pierre, M., et al. (2015). Cerebrovascular and blood-brain barrier impairments in Huntington’s disease: potential implications for its pathophysiology. Ann. Neurol. 78, 160–177. doi: 10.1002/ana.24406
Ebnet, K., Aurrand-Lions, M., Kuhn, A., Kiefer, F., Butz, S., Zander, K., et al. (2003). The junctional adhesion molecule (JAM) family members JAM-2 and JAM-3 associate with the cell polarity protein PAR-3: a possible role for JAMs in endothelial cell polarity. J. Cell Sci. 116, 3879–3891. doi: 10.1242/jcs.00704
Elias, B. C., Suzuki, T., Seth, A., Giorgianni, F., Kale, G., Shen, L., et al. (2009). Phosphorylation of Tyr-398 and Tyr-402 in occludin prevents its interaction with ZO-1 and destabilizes its assembly at the tight junctions. J. Biol. Chem. 284, 1559–1569. doi: 10.1074/jbc.M804783200
Emerich, D. F., Dean, R. L., Osborn, C., and Bartus, R. T. (2001). The development of the bradykinin agonist labradimil as a means to increase the permeability of the blood-brain barrier: from concept to clinical evaluation. Clin. Pharmacokinet. 40, 105–123. doi: 10.2165/00003088-200140020-00003
Erdlenbruch, B., Alipour, M., Fricker, G., Miller, D. S., Kugler, W., Eibl, H., et al. (2003). Alkylglycerol opening of the blood-brain barrier to small and large fluorescence markers in normal and C6 glioma-bearing rats and isolated rat brain capillaries. Br. J. Pharmacol. 140, 1201–1210. doi: 10.1038/sj.bjp.0705554
Erdo, F., Denes, L., and de Lange, E. (2017). Age-associated physiological and pathological changes at the blood-brain barrier: a review. J. Cereb. Blood Flow Metab. 37, 4–24. doi: 10.1177/0271678X16679420
Errede, M., Girolamo, F., Ferrara, G., Strippoli, M., Morando, S., Boldrin, V., et al. (2012). Blood-brain barrier alterations in the cerebral cortex in experimental autoimmune encephalomyelitis. J. Neuropathol. Exp. Neurol. 71, 840–854. doi: 10.1097/NEN.0b013e31826ac110
Fanning, A. S., Jameson, B. J., Jesaitis, L. A., and Anderson, J. M. (1998). The tight junction protein ZO-1 establishes a link between the transmembrane protein occludin and the actin cytoskeleton. J. Biol. Chem. 273, 29745–29753. doi: 10.1074/jbc.273.45.29745
Fanning, A. S., Ma, T. Y., and Anderson, J. M. (2002). Isolation and functional characterization of the actin binding region in the tight junction protein ZO-1. FASEB J. 16, 1835–1837. doi: 10.1096/fj.02-0121fje
Fasano, A., Fiorentini, C., Donelli, G., Uzzau, S., Kaper, J. B., Margaretten, K., et al. (1995). Zonula occludens toxin modulates tight junctions through protein kinase C-dependent actin reorganization, in vitro. J. Clin. Invest. 96, 710–720. doi: 10.1172/JCI118114
Furuse, M., Itoh, M., Hirase, T., Nagafuchi, A., Yonemura, S., and Tsukita, S. (1994). Direct association of occludin with ZO-1 and its possible involvement in the localization of occludin at tight junctions. J. Cell Biol. 127, 1617–1626. doi: 10.1083/jcb.127.6.1617
Gray, M. T., and Woulfe, J. M. (2015). Striatal blood-brain barrier permeability in Parkinson’s disease. J. Cereb. Blood Flow Metab. 35, 747–750. doi: 10.1038/jcbfm.2015.32
Greene, C., and Campbell, M. (2016). Tight junction modulation of the blood brain barrier: CNS delivery of small molecules. Tissue Barriers 4:e1138017. doi: 10.1080/21688370.2015.1138017
Greene, C., Hanley, N., and Campbell, M. (2019). Claudin-5: gatekeeper of neurological function. Fluids Barriers CNS 16:3. doi: 10.1186/s12987-019-0123-z
Gu, Y., Zheng, G., Xu, M., Li, Y., Chen, X., Zhu, W., et al. (2012). Caveolin-1 regulates nitric oxide-mediated matrix metalloproteinases activity and blood-brain barrier permeability in focal cerebral ischemia and reperfusion injury. J. Neurochem. 120, 147–156. doi: 10.1111/j.1471-4159.2011.07542.x
Gunzel, D., and Yu, A. S. (2013). Claudins and the modulation of tight junction permeability. Physiol. Rev. 93, 525–569. doi: 10.1152/physrev.00019.2012
Gursoy-Ozdemir, Y., Qiu, J., Matsuoka, N., Bolay, H., Bermpohl, D., Jin, H., et al. (2004). Cortical spreading depression activates and upregulates MMP-9. J. Clin. Invest. 113, 1447–1455. doi: 10.1172/JCI200421227
Haarmann, A., Deiss, A., Prochaska, J., Foerch, C., Weksler, B., Romero, I., et al. (2010). Evaluation of soluble junctional adhesion molecule-a as a biomarker of human brain endothelial barrier breakdown. PLoS One 5:e13568. doi: 10.1371/journal.pone.0013568
Habgood, M. D., Bye, N., Dziegielewska, K. M., Ek, C. J., Lane, M. A., Potter, A., et al. (2007). Changes in blood-brain barrier permeability to large and small molecules following traumatic brain injury in mice. Eur. J. Neurosci. 25, 231–238. doi: 10.1111/j.1460-9568.2006.05275.x
Han, M., Pendem, S., Teh, S. L., Sukumaran, D. K., Wu, F., and Wilson, J. X. (2010). Ascorbate protects endothelial barrier function during septic insult: role of protein phosphatase type 2A. Free Radic. Biol. Med. 48, 128–135. doi: 10.1016/j.freeradbiomed.2009.10.034
Hartz, A. M., Bauer, B., Soldner, E. L., Wolf, A., Boy, S., Backhaus, R., et al. (2012). Amyloid-β contributes to blood-brain barrier leakage in transgenic human amyloid precursor protein mice and in humans with cerebral amyloid angiopathy. Stroke 43, 514–523. doi: 10.1161/STROKEAHA.111.627562
Haseloff, R. F., Dithmer, S., Winkler, L., Wolburg, H., and Blasig, I. E. (2015). Transmembrane proteins of the tight junctions at the blood-brain barrier: structural and functional aspects. Semin. Cell Dev. Biol. 38, 16–25. doi: 10.1016/j.semcdb.2014.11.004
Hau, V. S., Huber, J. D., Campos, C. R., Davis, R. T., and Davis, T. P. (2004). Effect of lambda-carrageenan-induced inflammatory pain on brain uptake of codeine and antinociception. Brain Res. 1018, 257–264. doi: 10.1016/j.brainres.2004.05.081
Hawkins, B. T., and Egleton, R. D. (2006). Fluorescence imaging of blood-brain barrier disruption. J. Neurosci. Methods 151, 262–267. doi: 10.1016/j.jneumeth.2005.08.006
Hersh, D. S., Wadajkar, A. S., Roberts, N., Perez, J. G., Connolly, N. P., Frenkel, V., et al. (2016). Evolving drug delivery strategies to overcome the blood brain barrier. Curr. Pharm. Des. 22, 1177–1193. doi: 10.2174/1381612822666151221150733
Hirase, T., Kawashima, S., Wong, E. Y. M., Ueyama, T., Rikitake, Y., Tsukita, S., et al. (2001). Regulation of tight junction permeability and occludin phosphorylation by RhoA-p160ROCK-dependent and -independent mechanisms. J. Biol. Chem. 276, 10423–10431. doi: 10.1074/jbc.M007136200
Hornbeck, P. V., Zhang, B., Murray, B., Kornhauser, J. M., Latham, V., and Skrzypek, E. (2015). PhosphoSitePlus, 2014: mutations, PTMs and recalibrations. Nucleic Acids Res. 43, D512–D520. doi: 10.1093/nar/gku1267
Hou, J., Renigunta, V., Nie, M., Sunq, A., Himmerkus, N., Quintanova, C., et al. (2019). Phosphorylated claudin-16 interacts with Trpv5 and regulates transcellular calcium transport in the kidney. Proc. Natl. Acad. Sci. 116, 19176–19186. doi: 10.1073/pnas.1902042116
Huang, L., Deng, M., He, Y., Lu, S., Ma, R., and Fang, Y. (2016). β-Asarone and levodopa co-administration increase striatal dopamine level in 6-hydroxydopamine induced rats by modulating P-glycoprotein and tight junction proteins at the blood-brain barrier and promoting levodopa into the brain. Clin. Exp. Pharmacol. Physiol. 43, 634–643. doi: 10.1111/1440-1681.12570
Huang, J., Han, S., Sun, Q., Zhao, Y., Liu, J., Yuan, X., et al. (2017). Kv1.3 channel blocker (ImKTx88) maintains blood-brain barrier in experimental autoimmune encephalomyelitis. Cell Biosci. 7:31. doi: 10.1186/s13578-017-0158-2
Huber, J. D., Hau, V. S., Borg, L., Campos, C. R., Egleton, R. D., and Davis, T. P. (2002). Blood-brain barrier tight junctions are altered during a 72-h exposure to lambda-carrageenan-induced inflammatory pain. Am. J. Physiol. Heart Circ. Physiol. 283, H1531–H1537. doi: 10.1152/ajpheart.00027.2002
Huber, J. D., Witt, K. A., Hom, S., Egleton, R. D., Mark, K. S., and Davis, T. P. (2001). Inflammatory pain alters blood-brain barrier permeability and tight junctional protein expression. Am. J. Physiol. Heart Circ. Physiol. 280, H1241–H1248. doi: 10.1152/ajpheart.2001.280.3.H1241
Hwang, I., Yang, H., Kang, H. S., Ahn, C. H., Lee, G. S., Hong, E. J., et al. (2014). Spatial expression of claudin family members in various organs of mice. Mol. Med. Rep. 9, 1806–1812. doi: 10.3892/mmr.2014.2031
Iadecola, C. (2017). The neurovascular unit coming of age: a journey through neurovascular coupling in health and disease. Neuron 96, 17–42. doi: 10.1016/j.neuron.2017.07.030
Iannitti, T., and Palmieri, B. (2010). An update on the therapeutic role of alkylglycerols. Mar. Drugs 8, 2267–2300. doi: 10.3390/md8082267
Ishrat, T., Sayeed, I., Atif, F., Hua, F., and Stein, D. G. (2010). Progesterone and allopregnanolone attenuate blood-brain barrier dysfunction following permanent focal ischemia by regulating the expression of matrix metalloproteinases. Exp. Neurol. 226, 183–190. doi: 10.1016/j.expneurol.2010.08.023
Itoh, M., Furuse, M., Morita, K., Kubota, K., Saitou, M., and Tsukita, S. (1999). Direct binding of three tight junction-associated MAGUKs, ZO-1, ZO-2, and ZO-3, with the COOH termini of claudins. J. Cell Biol. 147, 1351–1363. doi: 10.1083/jcb.147.6.1351
Jiang, X., Andjelkovic, A. V., Zhu, L., Yang, T., Bennett, M. V. L., Chen, J., et al. (2018). Blood-brain barrier dysfunction and recovery after ischemic stroke. Prog. Neurobiol. 163-164, 144–171. doi: 10.1016/j.pneurobio.2017.10.001
Jiao, H., Wang, Z., Liu, Y., Wang, P., and Xue, Y. (2011). Specific role of tight junction proteins claudin-5, occludin, and ZO-1 of the blood-brain barrier in a focal cerebral ischemic insult. J. Mol. Neurosci. 44, 130–139. doi: 10.1007/s12031-011-9496-4
Kale, G., Naren, A., Sheth, P., and Rao, R. K. (2003). Tyrosine phosphorylation of occludin attenuates its interactions with ZO-1, ZO-2, and ZO-3. Biochem. Biophys. Res. Commun. 302, 324–329. doi: 10.1016/S0006-291X(03)00167-0
Kaya, M., and Ahishali, B. (2011). Assessment of permeability in barrier type of endothelium in brain using tracers: Evans blue, sodium fluorescein, and horseradish peroxidase. Methods Mol. Biol. 763, 369–382. doi: 10.1007/978-1-61779-191-8_25
Keaney, J., Walsh, D. M., O’Malley, T., Hudson, N., Crosbie, D. E., Loftus, T., et al. (2015). Autoregulated paracellular clearance of amyloid-β across the blood-brain barrier. Sci. Adv. 1:e1500472. doi: 10.1126/sciadv.1500472
Kermode, A. G., Thompson, A. J., Tofts, P., MacManus, D. G., Kendall, B. E., Kingsley, D. P., et al. (1990). Breakdown of the blood-brain barrier precedes symptoms and other MRI signs of new lesions in multiple sclerosis. Pathogenetic and clinical implications. Brain 113, 1477–1489. doi: 10.1093/brain/113.5.1477
Kim, H. J., Pyeun, Y. S., Kim, Y. W., Cho, B. M., Lee, T. H., Moon, T. Y., et al. (2005). A model for research on the blood-brain barrier disruption induced by unsaturated fatty acid emulsion. Investig. Radiol. 40, 270–276. doi: 10.1097/01.rli.0000160488.26344.5f
Kirk, J., Plumb, J., Mirakhur, M., and McQuaid, S. (2003). Tight junctional abnormality in multiple sclerosis white matter affects all calibres of vessel and is associated with blood-brain barrier leakage and active demyelination. J. Pathol. 201, 319–327. doi: 10.1002/path.1434
Kisler, K., Nelson, A. R., Montagne, A., and Zlokovic, B. V. (2017). Cerebral blood flow regulation and neurovascular dysfunction in Alzheimer disease. Nat. Rev. Neurosci. 18, 419–434. doi: 10.1038/nrn.2017.48
Knowland, D., Arac, A., Sekiguchi, K. J., Hsu, M., Lutz, S. E., Perrino, J., et al. (2014). Stepwise recruitment of transcellular and paracellular pathways underlies blood-brain barrier breakdown in stroke. Neuron 82, 603–617. doi: 10.1016/j.neuron.2014.03.003
Kook, S. Y., Hong, H. S., Moon, M., Ha, C. M., Chang, S., and Mook-Jung, I. (2012). Aβ1-42-RAGE interaction disrupts tight junctions of the blood-brain barrier via Ca2+-calcineurin signaling. J. Neurosci. 32, 8845–8854. doi: 10.1523/JNEUROSCI.6102-11.2012
Krueger, M., Bechmann, I., Immig, K., Reichenbach, A., Hartig, W., and Michalski, D. (2015). Blood-brain barrier breakdown involves four distinct stages of vascular damage in various models of experimental focal cerebral ischemia. J. Cereb. Blood Flow Metab. 35, 292–303. doi: 10.1038/jcbfm.2014.199
Krug, S. M., Amasheh, S., Richter, J. F., Milatz, S., Günzel, D., Westphal, J. K., et al. (2009). Tricellulin forms a barrier to macromolecules in tricellular tight junctions without affecting ion permeability. Mol. Biol. Cell 20, 3713–3724. doi: 10.1091/mbc.e09-01-0080
Kuroiwa, T., Ting, P., Martinez, H., and Klatzo, I. (1985). The biphasic opening of the blood-brain barrier to proteins following temporary middle cerebral artery occlusion. Acta Neuropathol. 68, 122–129. doi: 10.1007/BF00688633
Leech, S., Kirk, J., Plumb, J., and McQuaid, S. (2007). Persistent endothelial abnormalities and blood-brain barrier leak in primary and secondary progressive multiple sclerosis. Neuropathol. Appl. Neurobiol. 33, 86–98. doi: 10.1111/j.1365-2990.2006.00781.x
Li, Y., Fanning, A. S., Anderson, J. M., and Lavie, A. (2005). Structure of the conserved cytoplasmic C-terminal domain of occludin: identification of the ZO-1 binding surface. J. Mol. Biol. 352, 151–164. doi: 10.1016/j.jmb.2005.07.017
Lim, R. G., Quan, C., Reyes-Ortiz, A. M., Lutz, S. E., Kedaigle, A. J., Gipson, T. A., et al. (2017). Huntington’s disease iPSC-derived brain microvascular endothelial cells reveal WNT-mediated angiogenic and blood-brain barrier deficits. Cell Rep. 19, 1365–1377. doi: 10.1016/j.celrep.2017.04.021
Lin, J. L., Huang, Y. H., Shen, Y. C., Huang, H. C., and Liu, P. H. (2010). Ascorbic acid prevents blood-brain barrier disruption and sensory deficit caused by sustained compression of primary somatosensory cortex. J. Cereb. Blood Flow Metab. 30, 1121–1136. doi: 10.1038/jcbfm.2009.277
Liu, J., Wang, F., Liu, S., Du, J., Hu, X., Xiong, J., et al. (2017). Sodium butyrate exerts protective effect against Parkinson’s disease in mice via stimulation of glucagon like peptide-1. J. Neurol. Sci. 381, 176–181. doi: 10.1016/j.jns.2017.08.3235
Liu, P., Zhang, R., Liu, D., Wang, J., Yuan, C., Zhao, X., et al. (2018). Time-course investigation of blood-brain barrier permeability and tight junction protein changes in a rat model of permanent focal ischemia. J. Physiol. Sci. 68, 121–127. doi: 10.1007/s12576-016-0516-6
Llorens, F., Villar-Pique, A., Schmitz, M., Diaz-Lucena, D., Wohlhage, M., Hermann, P., et al. (2019). Plasma total prion protein as a potential biomarker for neurodegenerative dementia: diagnostic accuracy in the spectrum of prion diseases. Neuropathol. Appl. Neurobiol. 46, 240–254. doi: 10.1111/nan.12573
Lochhead, J. J., McCaffrey, G., Quigley, C. E., Finch, J., DeMarco, K. M., Nametz, N., et al. (2010). Oxidative stress increases blood-brain barrier permeability and induces alterations in occludin during hypoxia-reoxygenation. J. Cereb. Blood Flow Metab. 30, 1625–1636. doi: 10.1038/jcbfm.2010.29
Lochhead, J. J., McCaffrey, G., Sanchez-Covarrubias, L., Finch, J. D., DeMarco, K. M., et al. (2012). Tempol modulates changes in xenobiotic permeability and occludin oligomeric assemblies at the blood-brain barrier during inflammatory pain. Am. J. Physiol. Heart Circ. Physiol. 302, H582–H593. doi: 10.1152/ajpheart.00889.2011
Lochhead, J. J., Ronaldson, P. T., and Davis, T. P. (2017). Hypoxic stress and inflammatory pain disrupt blood-brain barrier tight junctions: implications for drug delivery to the central nervous system. AAPS J. 19, 910–920. doi: 10.1208/s12248-017-0076-6
Loscher, W., and Friedman, A. (2020). Structural, molecular, and functional alterations of the blood-brain barrier during epileptogenesis and epilepsy: a cause, consequence, or both? Int. J. Mol. Sci. 21:591. doi: 10.3390/ijms21020591
Lye, M. F., Fanning, A. S., Su, Y., Anderson, J. M., and Lavie, A. (2010). Insights into regulated ligand binding sites from the structure of ZO-1 Src homology 3-guanylate kinase module. J. Biol. Chem. 285, 13907–13917. doi: 10.1074/jbc.M109.093674
Mandel, I., Paperna, T., Volkowich, A., Merhav, M., Glass-Marmor, L., and Miller, A. (2012). The ubiquitin-proteasome pathway regulates claudin 5 degradation. J. Cell. Biochem. 113, 2415–2423. doi: 10.1002/jcb.24118
Marchi, N., Angelov, L., Masaryk, T., Fazio, V., Granata, T., Hernandez, N., et al. (2007). Seizure-promoting effect of blood-brain barrier disruption. Epilepsia 48, 732–742. doi: 10.1111/j.1528-1167.2007.00988.x
Markov, A. G., Aschenbach, J. R., and Amasheh, S. (2015). Claudin clusters as determinants of epithelial barrier function. IUBMB Life 67, 29–35. doi: 10.1002/iub.1347
McCaffrey, G., Seelbach, M. J., Staatz, W. D., Nametz, N., Quigley, C., Campos, C. R., et al. (2008). Occludin oligomeric assembly at tight junctions of the blood-brain barrier is disrupted by peripheral inflammatory hyperalgesia. J. Neurochem. 106, 2395–2409. doi: 10.1111/j.1471-4159.2008.05582.x
McCaffrey, G., Willis, C. L., Staatz, W. D., Nametz, N., Quigley, C. A., Hom, S., et al. (2009). Occludin oligomeric assemblies at tight junctions of the blood-brain barrier are altered by hypoxia and reoxygenation stress. J. Neurochem. 110, 58–71. doi: 10.1111/j.1471-4159.2009.06113.x
McColl, B. W., Rothwell, N. J., and Allan, S. M. (2008). Systemic inflammation alters the kinetics of cerebrovascular tight junction disruption after experimental stroke in mice. J. Neurosci. 28, 9451–9462. doi: 10.1523/JNEUROSCI.2674-08.2008
McKenzie, J. A. G., Riento, K., and Ridley, A. J. (2006). Casein kinase Iε associates with and phosphorylates the tight junction protein occludin. FEBS Lett. 580, 2388–2394. doi: 10.1016/j.febslet.2006.03.048
McMahon, D., Poon, C., and Hynynen, K. (2019). Evaluating the safety profile of focused ultrasound and microbubble-mediated treatments to increase blood-brain barrier permeability. Expert Opin. Drug Deliv. 16, 129–142. doi: 10.1080/17425247.2019.1567490
McNeil, E., Capaldo, C. T., and Macara, I. G. (2006). Zonula occludens-1 function in the assembly of tight junctions in Madin-Darby canine kidney epithelial cells. Mol. Biol. Cell 17, 1922–1932. doi: 10.1091/mbc.e05-07-0650
Mehta, D. C., Short, J. L., and Nicolazzo, J. A. (2013). Altered brain uptake of therapeutics in a triple transgenic mouse model of Alzheimer’s disease. Pharm. Res. 30, 2868–2879. doi: 10.1007/s11095-013-1116-2
Menon, D., Karyekar, C. S., Fasano, A., Lu, R., and Eddington, N. D. (2005). Enhancement of brain distribution of anticancer agents using DeltaG, the 12 kDa active fragment of ZOT. Int. J. Pharm. 306, 122–131. doi: 10.1016/j.ijpharm.2005.09.006
Merali, Z., Huang, K., Mikulis, D., Silver, F., and Kassner, A. (2017). Evolution of blood-brain-barrier permeability after acute ischemic stroke. PLoS One 12:e0171558. doi: 10.1371/journal.pone.0171558
Milatz, S., Krug, S. M., Rosenthal, R., Gunzel, D., Muller, D., Schulzke, J. D., et al. (2010). Claudin-3 acts as a sealing component of the tight junction for ions of either charge and uncharged solutes. Biochim. Biophys. Acta 1798, 2048–2057. doi: 10.1016/j.bbamem.2010.07.014
Mineta, K., Yamamoto, Y., Yamazaki, Y., Tanaka, H., Tada, Y., Saito, K., et al. (2011). Predicted expansion of the claudin multigene family. FEBS Lett. 585, 606–612. doi: 10.1016/j.febslet.2011.01.028
Nag, S., Venugopalan, R., and Stewart, D. J. (2007). Increased caveolin-1 expression precedes decreased expression of occludin and claudin-5 during blood-brain barrier breakdown. Acta Neuropathol. 114, 459–469. doi: 10.1007/s00401-007-0274-x
Narayan, P. J., Kim, S. L., Lill, C., Feng, S., Faull, R. L., Curtis, M. A., et al. (2015). Assessing fibrinogen extravasation into Alzheimer’s disease brain using high-content screening of brain tissue microarrays. J. Neurosci. Methods 247, 41–49. doi: 10.1016/j.jneumeth.2015.03.017
Narayan, R. K., Maas, A. I., Servadei, F., Skolnick, B. E., Tillinger, M. N., Marshall, L. F., et al. (2008). Progression of traumatic intracerebral hemorrhage: a prospective observational study. J. Neurotrauma 25, 629–639. doi: 10.1089/neu.2007.0385
Natarajan, R., Northrop, N., and Yamamoto, B. (2017). Fluorescein isothiocyanate (FITC)-dextran extravasation as a measure of blood-brain barrier permeability. Curr. Protoc. Neurosci. 79, 9.58.1–9.58.15. doi: 10.1002/cpns.25
Nation, D. A., Sweeney, M. D., Montagne, A., Sagare, A. P., D’Orazio, L. M., Pachicano, M., et al. (2019). Blood-brain barrier breakdown is an early biomarker of human cognitive dysfunction. Nat. Med. 25, 270–276. doi: 10.1038/s41591-018-0297-y
Nitta, T., Hata, M., Gotoh, S., Seo, Y., Sasaki, H., Hashimoto, N., et al. (2003). Size-selective loosening of the blood-brain barrier in claudin-5-deficient mice. J. Cell Biol. 161, 653–660. doi: 10.1083/jcb.200302070
Nomme, J., Antanasijevic, A., Caffrey, M., Van Itallie, C. M., Anderson, J. M., Fanning, A. S., et al. (2015). Structural basis of a key factor regulating the affinity between the zonula occludens first PDZ domain and claudins. J. Biol. Chem. 290, 16595–16606. doi: 10.1074/jbc.M115.646695
Ohtsuki, S., Sato, S., Yamaguchi, H., Kamoi, M., Asashima, T., and Terasaki, T. (2007). Exogenous expression of claudin-5 induces barrier properties in cultured rat brain capillary endothelial cells. J. Cell. Physiol. 210, 81–86. doi: 10.1002/jcp.20823
Ohtsuki, S., and Terasaki, T. (2007). Contribution of carrier-mediated transport systems to the blood-brain barrier as a supporting and protecting interface for the brain; importance for CNS drug discovery and development. Pharm. Res. 24, 1745–1758. doi: 10.1007/s11095-007-9374-5
Ohtsuki, S., Yamaguchi, H., Katsukura, Y., Asashima, T., and Terasaki, T. (2008). mRNA expression levels of tight junction protein genes in mouse brain capillary endothelial cells highly purified by magnetic cell sorting. J. Neurochem. 104, 147–154. doi: 10.1111/j.1471-4159.2007.05008.x
Olah, G., Heredi, J., Menyhart, A., Czinege, Z., Nagy, D., Fuzik, J., et al. (2013). Unexpected effects of peripherally administered kynurenic acid on cortical spreading depression and related blood-brain barrier permeability. Drug Des. Devel. Ther. 7, 981–987. doi: 10.2147/DDDT.S44496
Omidi, Y., Campbell, L., Barar, J., Connell, D., Akhtar, S., and Gumbleton, M. (2003). Evaluation of the immortalised mouse brain capillary endothelial cell line, b.End3, as an in vitro blood-brain barrier model for drug uptake and transport studies. Brain Res. 990, 95–112. doi: 10.1016/S0006-8993(03)03443-7
Otani, T., Nguyen, T. P., Tokuda, S., Sugihara, K., Sugawara, T., Furuse, K., et al. (2019). Claudins and JAM-A coordinately regulate tight junction formation and epithelial polarity. J. Cell Biol. 218, 3372–3396. doi: 10.1083/jcb.201812157
Paul, J., Strickland, S., and Melchor, J. P. (2007). Fibrin deposition accelerates neurovascular damage and neuroinflammation in mouse models of Alzheimer’s disease. J. Exp. Med. 204, 1999–2008. doi: 10.1084/jem.20070304
Piehl, C., Piontek, J., Cording, J., Wolburg, H., and Blasig, I. E. (2010). Participation of the second extracellular loop of claudin-5 in paracellular tightening against ions, small and large molecules. Cell. Mol. Life Sci. 67, 2131–2140. doi: 10.1007/s00018-010-0332-8
Pienaar, I. S., Lee, C. H., Elson, J. L., McGuinness, L., Gentleman, S. M., Kalaria, R. N., et al. (2015). Deep-brain stimulation associates with improved microvascular integrity in the subthalamic nucleus in Parkinson’s disease. Neurobiol. Dis. 74, 392–405. doi: 10.1016/j.nbd.2014.12.006
Piontek, J., Fritzsche, S., Cording, J., Richter, S., Hartwig, J., Walter, M., et al. (2011). Elucidating the principles of the molecular organization of heteropolymeric tight junction strands. Cell. Mol. Life Sci. 68, 3903–3918. doi: 10.1007/s00018-011-0680-z
Piontek, J., Winkler, L., Wolburg, H., Müller, S. L., Zuleger, N., Piehl, C., et al. (2008). Formation of tight junction: determinants of homophilic interaction between classic claudins. FASEB J. 22, 146–158. doi: 10.1096/fj.07-8319com
Pisani, V., Stefani, A., Pierantozzi, M., Natoli, S., Stanzione, P., Franciotta, D., et al. (2012). Increased blood-cerebrospinal fluid transfer of albumin in advanced Parkinson’s disease. J. Neuroinflammation 9:188. doi: 10.1186/1742-2094-9-188
Plumb, J., McQuaid, S., Mirakhur, M., and Kirk, J. (2002). Abnormal endothelial tight junctions in active lesions and normal-appearing white matter in multiple sclerosis. Brain Pathol. 12, 154–169. doi: 10.1111/j.1750-3639.2002.tb00430.x
Poliak, S., Matlis, S., Ullmer, C., Scherer, S. S., and Peles, E. (2002). Distinct claudins and associated PDZ proteins form different autotypic tight junctions in myelinating Schwann cells. J. Cell Biol. 159, 361–372. doi: 10.1083/jcb.200207050
Prakash, R., and Carmichael, S. T. (2015). Blood-brain barrier breakdown and neovascularization processes after stroke and traumatic brain injury. Curr. Opin. Neurol. 28, 556–564. doi: 10.1097/WCO.0000000000000248
Qosa, H., Mohamed, L. A., Al Rihani, S. B., Batarseh, Y. S., Duong, Q. V., Keller, J. N., et al. (2016). High-throughput screening for identification of blood-brain barrier integrity enhancers: a drug repurposing opportunity to rectify vascular amyloid toxicity. J. Alzheimers Dis. 53, 1499–1516. doi: 10.3233/JAD-151179
Raleigh, D. R., Boe, D. M., Yu, D., Weber, C. R., Marchiando, A. M., Bradford, E. M., et al. (2011). Occludin S408 phosphorylation regulates tight junction protein interactions and barrier function. J. Cell Biol. 193, 565–582. doi: 10.1083/jcb.201010065
Raleigh, D. R., Marchiando, A. M., Zhang, Y., Shen, L., Sasaki, H., Wang, Y., et al. (2010). Tight junction–associated MARVEL proteins MarvelD3, Tricellulin, and Occludin have distinct but overlapping functions. Mol. Biol. Cell 21, 1200–1213. doi: 10.1091/mbc.e09-08-0734
Rapoport, S. I. (2000). Osmotic opening of the blood-brain barrier: principles, mechanism, and therapeutic applications. Cell. Mol. Neurobiol. 20, 217–230. doi: 10.1023/A:1007049806660
Rapoport, S. I., and Robinson, P. J. (1986). Tight-junctional modification as the basis of osmotic opening of the blood-brain barrier. Ann. N. Y. Acad. Sci. 481, 250–267. doi: 10.1111/j.1749-6632.1986.tb27155.x
Reese, T. S., and Karnovsky, M. J. (1967). Fine structural localization of a blood-brain barrier to exogenous peroxidase. J. Cell Biol. 34, 207–217. doi: 10.1083/jcb.34.1.207
Reinhold, A. K., and Rittner, H. L. (2017). Barrier function in the peripheral and central nervous system-a review. Pflugers Arch. 469, 123–134. doi: 10.1007/s00424-016-1920-8
Rempe, R. G., Hartz, A. M. S., Soldner, E. L. B., Sokola, B. S., Alluri, S. R., Abner, E. L., et al. (2018). Matrix metalloproteinase-mediated blood-brain barrier dysfunction in epilepsy. J. Neurosci. 38, 4301–4315. doi: 10.1523/JNEUROSCI.2751-17.2018
Ren, Z., Iliff, J. J., Yang, L., Yang, J., Chen, X., Chen, M. J., et al. (2013). ‘Hit and Run’ model of closed-skull traumatic brain injury (TBI) reveals complex patterns of post-traumatic AQP4 dysregulation. J. Cereb. Blood Flow Metab. 33, 834–845. doi: 10.1038/jcbfm.2013.30
Rigau, V., Morin, M., Rousset, M. C., de Bock, F., Lebrun, A., Coubes, P., et al. (2007). Angiogenesis is associated with blood-brain barrier permeability in temporal lobe epilepsy. Brain 130, 1942–1956. doi: 10.1093/brain/awm118
Rodriguez-Grande, B., Ichkova, A., Lemarchant, S., and Badaut, J. (2017). Early to long-term alterations of CNS barriers after traumatic brain injury: considerations for drug development. AAPS J. 19, 1615–1625. doi: 10.1208/s12248-017-0123-3
Ronaldson, P. T., Demarco, K. M., Sanchez-Covarrubias, L., Solinsky, C. M., and Davis, T. P. (2009). Transforming growth factor-beta signaling alters substrate permeability and tight junction protein expression at the blood-brain barrier during inflammatory pain. J. Cereb. Blood Flow Metab. 29, 1084–1098. doi: 10.1038/jcbfm.2009.32
Ryu, J. K., and McLarnon, J. G. (2009). A leaky blood-brain barrier, fibrinogen infiltration and microglial reactivity in inflamed Alzheimer’s disease brain. J. Cell. Mol. Med. 13, 2911–2925. doi: 10.1111/j.1582-4934.2008.00434.x
Saitou, M., Furuse, M., Sasaki, H., Schulzke, J. D., Fromm, M., Takano, H., et al. (2000). Complex phenotype of mice lacking occludin, a component of tight junction strands. Mol. Biol. Cell 11, 4131–4142. doi: 10.1091/mbc.11.12.4131
Sakakibara, A., Furuse, M., Saitou, M., Ando-Akatsuka, Y., and Tsukita, S. (1997). Possible involvement of phosphorylation of occludin in tight junction formation. J. Cell Biol. 137, 1393–1401. doi: 10.1083/jcb.137.6.1393
Sandoval, K. E., and Witt, K. A. (2008). Blood-brain barrier tight junction permeability and ischemic stroke. Neurobiol. Dis. 32, 200–219. doi: 10.1016/j.nbd.2008.08.005
Sanovich, E., Bartus, R. T., Friden, P. M., Dean, R. L., Le, H. Q., and Brightman, M. W. (1995). Pathway across blood-brain barrier opened by the bradykinin agonist, RMP-7. Brain Res. 705, 125–135. doi: 10.1016/0006-8993(95)01143-9
Saunders, N. R., Dziegielewska, K. M., Mollgard, K., and Habgood, M. D. (2015). Markers for blood-brain barrier integrity: how appropriate is Evans blue in the twenty-first century and what are the alternatives? Front. Neurosci. 9:385. doi: 10.3389/fnins.2015.00385
Saw, M. M., Chamberlain, J., Barr, M., Morgan, M. P., Burnett, J. R., and Ho, K. M. (2014). Differential disruption of blood-brain barrier in severe traumatic brain injury. Neurocrit. Care. 20, 209–216. doi: 10.1007/s12028-013-9933-z
Schlingmann, B., Overgaard, C. E., Molina, S. A., Lynn, K. S., Mitchell, L. A., Dorsainvil White, S., et al. (2016). Regulation of claudin/zonula occludens-1 complexes by hetero-claudin interactions. Nat. Commun. 7:12276. doi: 10.1038/ncomms12276
Schmidt, A., Utepbergenov, D. I., Mueller, S. L., Beyermann, M., Schneider-Mergener, J., Krause, G., et al. (2004). Occludin binds to the SH3-hinge-GuK unit of zonula occludens protein 1: potential mechanism of tight junction regulation. Cell. Mol. Life Sci. 61, 1354–1365. doi: 10.1007/s00018-004-4010-6
Schulzke, J. D., Gitter, A. H., Mankertz, J., Spiegel, S., Seidler, U., Amasheh, S., et al. (2005). Epithelial transport and barrier function in occludin-deficient mice. Biochim. Biophys. Acta 1669, 34–42. doi: 10.1016/j.bbamem.2005.01.008
Shapira, Y., Setton, D., Artru, A. A., and Shohami, E. (1993). Blood-brain barrier permeability, cerebral edema, and neurologic function after closed head injury in rats. Anesth. Analg. 77, 141–148. doi: 10.1213/00000539-199307000-00028
Sheikov, N., McDannold, N., Sharma, S., and Hynynen, K. (2008). Effect of focused ultrasound applied with an ultrasound contrast agent on the tight junctional integrity of the brain microvascular endothelium. Ultrasound Med. Biol. 34, 1093–1104. doi: 10.1016/j.ultrasmedbio.2007.12.015
Sheikov, N., McDannold, N., Vykhodtseva, N., Jolesz, F., and Hynynen, K. (2004). Cellular mechanisms of the blood-brain barrier opening induced by ultrasound in presence of microbubbles. Ultrasound Med. Biol. 30, 979–989. doi: 10.1016/j.ultrasmedbio.2004.04.010
Shin, K., and Margolis, B. (2006). ZOning out tight junctions. Cell 126, 647–649. doi: 10.1016/j.cell.2006.08.005
Sifat, A. E., Vaidya, B., and Abbruscato, T. J. (2017). Blood-brain barrier protection as a therapeutic strategy for acute ischemic stroke. AAPS J. 19, 957–972. doi: 10.1208/s12248-017-0091-7
Smales, C., Ellis, M., Baumber, R., Hussain, N., Desmond, H., and Staddon, J. M. (2003). Occludin phosphorylation: identification of an occludin kinase in brain and cell extracts as CK2. FEBS Lett. 545, 161–166. doi: 10.1016/S0014-5793(03)00525-8
Suzuki, T., Elias, B. C., Seth, A., Shen, L., Turner, J. R., Giorgianni, F., et al. (2009). PKC eta regulates occludin phosphorylation and epithelial tight junction integrity. Proc. Natl. Acad. Sci. U. S. A. 106, 61–66. doi: 10.1073/pnas.0802741106
Sweeney, M. D., Zhao, Z., Montagne, A., Nelson, A. R., and Zlokovic, B. V. (2019). Blood-brain barrier: from physiology to disease and back. Physiol. Rev. 99, 21–78. doi: 10.1152/physrev.00050.2017
Sztriha, L., and Betz, A. L. (1991). Oleic acid reversibly opens the blood-brain barrier. Brain Res. 550, 257–262. doi: 10.1016/0006-8993(91)91326-V
Tash, B. R., Bewley, M. C., Russo, M., Keil, J. M., Griffin, K. A., Sundstrom, J. M., et al. (2012). The occludin and ZO-1 complex, defined by small angle X-ray scattering and NMR, has implications for modulating tight junction permeability. Proc. Natl. Acad. Sci. U. S. A. 109, 10855–10860. doi: 10.1073/pnas.1121390109
Tome, M. E., Jarvis, C. K., Schaefer, C. P., Jacobs, L. M., Herndon, J. M., Hunn, K. C., et al. (2018). Acute pain alters P-glycoprotein-containing protein complexes in rat cerebral microvessels: implications for P-glycoprotein trafficking. J. Cereb. Blood Flow Metab. 38, 2209–2222. doi: 10.1177/0271678X18803623
Tornavaca, O., Chia, M., Dufton, N., Almagro, L. O., Conway, D. E., Randi, A. M., et al. (2015). ZO-1 controls endothelial adherens junctions, cell-cell tension, angiogenesis, and barrier formation. J. Cell Biol. 208, 821–838. doi: 10.1083/jcb.201404140
Tscheik, C., Blasig, I. E., and Winkler, L. (2013). Trends in drug delivery through tissue barriers containing tight junctions. Tissue Barriers 1:e24565. doi: 10.4161/tisb.24565
Twiss, F., Oldenkamp, M., Hiemstra, A., Zhou, H., Matheron, L., Mohammed, S., et al. (2013). HGF signaling regulates Claudin-3 dynamics through its C-terminal tyrosine residues. Tissue Barriers 1:e27425. doi: 10.4161/tisb.27425
Uchida, Y., Sumiya, T., Tachikawa, M., Yamakawa, T., Murata, S., Yagi, Y., et al. (2019). Involvement of claudin-11 in disruption of blood-brain, -spinal cord, and -arachnoid barriers in multiple sclerosis. Mol. Neurobiol. 56, 2039–2056. doi: 10.1007/s12035-018-1207-5
Ujiie, M., Dickstein, D. L., Carlow, D. A., and Jefferies, W. A. (2003). Blood-brain barrier permeability precedes senile plaque formation in an Alzheimer disease model. Microcirculation 10, 463–470. doi: 10.1038/sj.mn.7800212
Umeda, K., Ikenouchi, J., Katahira-Tayama, S., Furuse, K., Sasaki, H., Nakayama, M., et al. (2006). ZO-1 and ZO-2 independently determine where claudins are polymerized in tight-junction strand formation. Cell 126, 741–754. doi: 10.1016/j.cell.2006.06.043
van Horssen, J., Brink, B. P., de Vries, H. E., van der Valk, P., and Bo, L. (2007). The blood-brain barrier in cortical multiple sclerosis lesions. J. Neuropathol. Exp. Neurol. 66, 321–328. doi: 10.1097/nen.0b013e318040b2de
Van Itallie, C. M., and Anderson, J. M. (2018). Phosphorylation of tight junction transmembrane proteins: many sites, much to do. Tissue Barriers 6:e1382671. doi: 10.1080/21688370.2017.1382671
Van Itallie, C. M., Fanning, A. S., Holmes, J., and Anderson, J. M. (2010). Occludin is required for cytokine-induced regulation of tight junction barriers. J. Cell Sci. 123, 2844–2852. doi: 10.1242/jcs.065581
Van Itallie, C. M., Tietgens, A. J., LoGrande, K., Aponte, A., Gucek, M., and Anderson, J. M. (2012). Phosphorylation of claudin-2 on serine 208 promotes membrane retention and reduces trafficking to lysosomes. J. Cell Sci. 125, 4902–4912. doi: 10.1242/jcs.111237
van Vliet, E. A., da Costa Araujo, S., Redeker, S., van Schaik, R., Aronica, E., and Gorter, J. A. (2007). Blood-brain barrier leakage may lead to progression of temporal lobe epilepsy. Brain 130, 521–534. doi: 10.1093/brain/awl318
Viggars, A. P., Wharton, S. B., Simpson, J. E., Matthews, F. E., Brayne, C., Savva, G. M., et al. (2011). Alterations in the blood brain barrier in ageing cerebral cortex in relationship to Alzheimer-type pathology: a study in the MRC-CFAS population neuropathology cohort. Neurosci. Lett. 505, 25–30. doi: 10.1016/j.neulet.2011.09.049
Walsh, T. G., Murphy, R. P., Fitzpatrick, P., Rochfort, K. D., Guinan, A. F., Murphy, A., et al. (2011). Stabilization of brain microvascular endothelial barrier function by shear stress involves VE-cadherin signaling leading to modulation of pTyr-occludin levels. J. Cell. Physiol. 226, 3053–3063. doi: 10.1002/jcp.22655
Walter, J. K., Castro, V., Voss, M., Gast, K., Rueckert, C., Piontek, J., et al. (2009). Redox-sensitivity of the dimerization of occludin. Cell. Mol. Life Sci. 66, 3655–3662. doi: 10.1007/s00018-009-0150-z
Wang, X. S., Fang, H. L., Chen, Y., Liang, S. S., Zhu, Z. G., Zeng, Q. Y., et al. (2014). Idazoxan reduces blood-brain barrier damage during experimental autoimmune encephalomyelitis in mouse. Eur. J. Pharmacol. 736, 70–76. doi: 10.1016/j.ejphar.2014.04.034
Wang, D., Li, S. P., Fu, J. S., Zhang, S., Bai, L., and Guo, L. (2016). Resveratrol defends blood-brain barrier integrity in experimental autoimmune encephalomyelitis mice. J. Neurophysiol. 116, 2173–2179. doi: 10.1152/jn.00510.2016
Warren, K., Jakacki, R., Widemann, B., Aikin, A., Libucha, M., Packer, R., et al. (2006). Phase II trial of intravenous lobradimil and carboplatin in childhood brain tumors: a report from the children’s oncology group. Cancer Chemother. Pharmacol. 58, 343–347. doi: 10.1007/s00280-005-0172-7
Wen, J., Qian, S., Yang, Q., Deng, L., Mo, Y., and Yu, Y. (2014). Overexpression of netrin-1 increases the expression of tight junction-associated proteins, claudin-5, occludin, and ZO-1, following traumatic brain injury in rats. Exp. Ther. Med. 8, 881–886. doi: 10.3892/etm.2014.1818
Willis, C. L., Meske, D. S., and Davis, T. P. (2010). Protein kinase C activation modulates reversible increase in cortical blood-brain barrier permeability and tight junction protein expression during hypoxia and posthypoxic reoxygenation. J. Cereb. Blood Flow Metab. 30, 1847–1859. doi: 10.1038/jcbfm.2010.119
Winkler, L., Blasig, R., Breitkreuz-Korff, O., Berndt, P., Dithmer, S., Helms, H.C., et al. (2020). Tight junctions in the blood-brain barrier promote edema formation and infarct size in stroke ‐ ambivalent effects of sealing proteins. J. Cereb. Blood Flow Metab. doi: 10.1177/0271678X20904687 [Epub ahead of print]
Winkler, E. A., Nishida, Y., Sagare, A. P., Rege, S. V., Bell, R. D., Perlmutter, D., et al. (2015). GLUT1 reductions exacerbate Alzheimer’s disease vasculo-neuronal dysfunction and degeneration. Nat. Neurosci. 18, 521–530. doi: 10.1038/nn.3966
Witt, K. A., Mark, K. S., Hom, S., and Davis, T. P. (2003). Effects of hypoxia-reoxygenation on rat blood-brain barrier permeability and tight junctional protein expression. Am. J. Physiol. Heart Circ. Physiol. 285, H2820–H2831. doi: 10.1152/ajpheart.00589.2003
Witt, K. A., Mark, K. S., Sandoval, K. E., and Davis, T. P. (2008). Reoxygenation stress on blood-brain barrier paracellular permeability and edema in the rat. Microvasc. Res. 75, 91–96. doi: 10.1016/j.mvr.2007.06.004
Wittchen, E. S., Haskins, J., and Stevenson, B. R. (1999). Protein interactions at the tight junction. Actin has multiple binding partners, and ZO-1 forms independent complexes with ZO-2 and ZO-3. J. Biol. Chem. 274, 35179–35185. doi: 10.1074/jbc.274.49.35179
Wolburg, H., Wolburg-Buchholz, K., Kraus, J., Rascher-Eggstein, G., Liebner, S., Hamm, S., et al. (2003). Localization of claudin-3 in tight junctions of the blood-brain barrier is selectively lost during experimental autoimmune encephalomyelitis and human glioblastoma multiforme. Acta Neuropathol. 105, 586–592. doi: 10.1007/s00401-003-0688-z
Wolman, M., Klatzo, I., Chui, E., Wilmes, F., Nishimoto, K., Fujiwara, K., et al. (1981). Evaluation of the dye-protein tracers in pathophysiology of the blood-brain barrier. Acta Neuropathol. 54, 55–61. doi: 10.1007/BF00691332
Wosik, K., Cayrol, R., Dodelet-Devillers, A., Berthelet, F., Bernard, M., Moumdjian, R., et al. (2007). Angiotensin II controls occludin function and is required for blood brain barrier maintenance: relevance to multiple sclerosis. J. Neurosci. 27, 9032–9042. doi: 10.1523/JNEUROSCI.2088-07.2007
Yaffe, Y., Shepshelovitch, J., Nevo-Yassaf, I., Yeheskel, A., Shmerling, H., Kwiatek, J. M., et al. (2012). The MARVEL transmembrane motif of occludin mediates oligomerization and targeting to the basolateral surface in epithelia. J. Cell Sci. 125:3545. doi: 10.1242/jcs.100289
Yamamoto, M., Ramirez, S. H., Sato, S., Kiyota, T., Cerny, R. L., Kaibuchi, K., et al. (2008). Phosphorylation of claudin-5 and occludin by rho kinase in brain endothelial cells. Am. J. Pathol. 172, 521–533. doi: 10.2353/ajpath.2008.070076
Yang, Y., Estrada, E. Y., Thompson, J. F., Liu, W., and Rosenberg, G. A. (2007). Matrix metalloproteinase-mediated disruption of tight junction proteins in cerebral vessels is reversed by synthetic matrix metalloproteinase inhibitor in focal ischemia in rat. J. Cereb. Blood Flow Metab. 27, 697–709. doi: 10.1038/sj.jcbfm.9600375
Yu, H., Wang, P., An, P., and Xue, Y. (2012). Recombinant human angiopoietin-1 ameliorates the expressions of ZO-1, occludin, VE-cadherin, and PKCalpha signaling after focal cerebral ischemia/reperfusion in rats. J. Mol. Neurosci. 46, 236–247. doi: 10.1007/s12031-011-9584-5
Keywords: blood-brain barrier, claudins, occludin, tight junctions, paracellular permeability, drug delivery
Citation: Lochhead JJ, Yang J, Ronaldson PT and Davis TP (2020) Structure, Function, and Regulation of the Blood-Brain Barrier Tight Junction in Central Nervous System Disorders. Front. Physiol. 11:914. doi: 10.3389/fphys.2020.00914
Edited by:
Juan R. Viña, University of Valencia, SpainReviewed by:
Salah Amasheh, Freie Universität Berlin, GermanyPeter S. Reinach, Wenzhou Medical University, China
Copyright © 2020 Lochhead, Yang, Ronaldson and Davis. This is an open-access article distributed under the terms of the Creative Commons Attribution License (CC BY). The use, distribution or reproduction in other forums is permitted, provided the original author(s) and the copyright owner(s) are credited and that the original publication in this journal is cited, in accordance with accepted academic practice. No use, distribution or reproduction is permitted which does not comply with these terms.
*Correspondence: Thomas P. Davis, davistp@email.arizona.edu