- 1Department of Medical Biology, Amsterdam University Medical Centers, University of Amsterdam, Amsterdam, Netherlands
- 2Department of Experimental Cardiology, Amsterdam University Medical Centers, University of Amsterdam, Amsterdam, Netherlands
- 3Netherlands Heart Institute, Utrecht, Netherlands
- 4ICREC Research Program, Germans Trias i Pujol Health Science Research Institute, Badalona, Spain
- 5CIBERCV, Instituto de Salud Carlos III, Madrid, Spain
Genome Wide Association Studies (GWAS) have provided an enormous amount of data on genomic loci associated with cardiac electrophysiology and arrhythmias. Clinical relevance, however, remains unclear since GWAS do not provide a mechanistic explanation for this association. Determining the electrophysiological relevance of variants for arrhythmias would aid development of risk stratification models for patients with arrhythmias. In this review, we give an overview of genetic variants related to ECG intervals and arrhythmogenic pathologies and discuss how these variants may influence cardiac electrophysiology and the occurrence of arrhythmias.
Introduction
Genome Wide Association Studies (GWAS) can identify genetic variants associated with phenotypic traits, such as electrocardiographic (ECG) intervals (Table 1). Interpretation of GWAS data relies on identification of the target gene affected by the novel discovered variant. Variants can be present in coding DNA, causing amino acid changes affecting protein function – or in non-coding DNA, altering the behavior of regulatory elements, thereby changing expression levels of its target gene(s) (Figure 1A). For many highly significant GWAS loci the gene causing the association with ECG intervals has not been identified yet. This is mostly because genes in or near these loci do not have a proven association with the phenotype. Overlaying such loci with publicly available genome wide data sets of gene expression (GTEx Consortium, 2013; Heinig, 2018), cardiac transcriptional elements (van Duijvenboden et al., 2016), genomic conformation Hi-C data sets (Montefiori et al., 2018), and other GWAS results will likely provide more insight into potential novel candidate genes regulating cardiac electrophysiology (van Ouwerkerk et al., 2019).
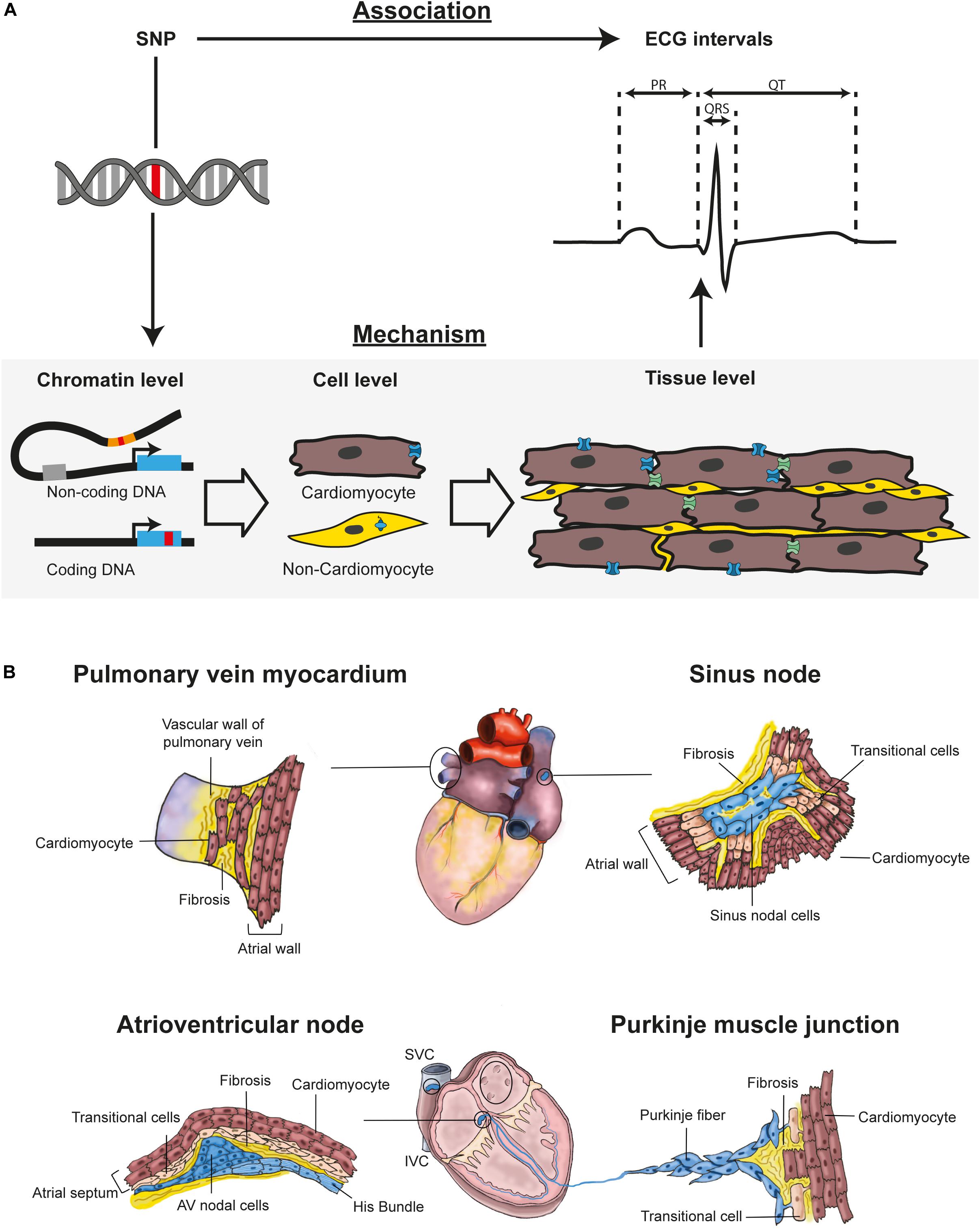
Figure 1. In GWAS, (A) SNPs are associated with ECG intervals, like PR interval, QRS complex, and QT interval. To understand physiological relevance of a SNP three biological levels of organization should be studied (1) Chromatin level: Determine which gene(s) is affected by the SNP. The SNP (red mark) can be located in non-coding or coding DNA. For SNPs in non-coding DNA, it is important to determine which coding genes are affected. (2) Cell level: Determine in which cell types the gene is expressed. The cell type in which the affected gene is expressed is relevant for cellular function of the gene. (3) Tissue level: Determine how gene expression in a cell type affects tissue electrophysiology. Tissue electrophysiology can be related to ECG intervals. (B) Tissue structure is essential for proper conduction. The pulmonary vein has an outer sleeve of cardiomyocytes. These pulmonary vein cardiomyocytes are not as densely packed as the atrial cardiomyocytes – resulting in less coupling. Small reentry circuits or ectopic activity can therefore occur in pulmonary vein myocardium. The sinus node – where it is not insulated by fibrosis – connects with the atrial cardiomyocytes through transitional cells. In the atrioventricular junction, AV nodal cells are electrically connected with the atrial myocardium through a layer of transitional cells. In the Purkinje muscle junction, Purkinje cells connect with ventricular cardiomyocytes through transitional cells. AV, atrioventricular; IVC, inferior vena cava; SNP, Single nucleotide polymorphism; SVC, superior vena cava.
Upon discovery, the expression of the target gene is often modified in isolated cardiomyocytes (from an animal model or human induced pluripotent stem cells). These cellular models can be used to assess any electrophysiological phenotypes associated with the potential GWAS candidate. However, ECG intervals reflect the complex electrophysiological interaction of the cardiac cells and tissue structures, complicating extrapolation of single cell measurements to ECG intervals (Opthof et al., 1987). Additionally, discovered variants may affect expression of genes in non-cardiomyocytes, further complicating the interpretation of such experiments in isolated cardiomyocytes (Stroud et al., 2016; Veerman et al., 2016). To overcome these limitations, in vivo models such as transgenic mice are most commonly used to investigate the relation between GWAS variants and electrophysiological phenotypes. However, large electrophysiological differences exist between mice and larger mammals such as human (Boukens et al., 2014), which need to be taken into account when interpreting the resulting data.
In this review, we give an overview of genomic loci related to abnormal cardiac electrophysiology and discuss how these variants may directly or indirectly influence ECG intervals and the occurrence of arrhythmias through their expression pattern and regulation within the heart.
GWAS Loci Related to Heart Rate
The sinus node is located at the border between intercaval area and the right atrium. Action potentials of sinus node cardiomyocytes have low upstroke velocities and occur spontaneously (West, 1955). The mechanism underlying these spontaneous depolarizations is based on the funny current, mediated by the Hyperpolarization-Activated Cyclic Nucleotide-gated channel 4 (HCN4), and the calcium clock mediated by e.g., Ryanodine Receptor 2 (RYR2) and Phospholamban (PLN) (Lakatta and DiFrancesco, 2009). Accordingly, associations between the genetic loci of HCN4 and PLN and heart rate found by GWAS is most likely mediated through variants impacting on the expression and or function of these genes (Eijgelsheim et al., 2010; den Hoed et al., 2013; Nolte et al., 2017). Unfortunately, for the other GWAS loci, the association is not as straightforward. In the proximity of these loci, no genes with a known function in the SAN have been identified. These loci therefore potentially affect SAN function by impacting on other parameters, e.g., electrical coupling with the rest of the atrium and levels of fibrosis (Glukhov et al., 2013).
In smaller mammals – e.g., mice and rabbits – the sinus node is transmural (Bleeker et al., 1980; van Eif et al., 2019), whereas in large mammals – e.g., humans and dogs – it is not (Fedorov et al., 2009, 2010). The geometry of the sinus node affects coupling with atrial myocardium, which influences its electrophysiological behavior (Kirchhof et al., 1987). The delicate interaction between the sinus node and the surrounding atrial myocardium is established by fibrous tissue providing up to five exit pathways in large mammals (Figure 1B; Unudurthi et al., 2014; Csepe et al., 2015; Li et al., 2017). A transitional layer of cells within the exit pathways (Figure 1B) allows for spontaneous depolarization within the sinus node (Joyner and van Capelle, 1986; Kléber and Rudy, 2004). The identity of the transitional cells may depend on the expression of transcription factor Nkx2-5 and GWAS has found variants near NKX2-5 associating with heart rate (Mommersteeg et al., 2007b; den Hoed et al., 2013; Li et al., 2019). Within the exit pathways, high conductance connexins CX43 and CX40 (GJA1 and GJA5) are lower expressed toward the sinus node, whereas the low conductance CX45 is expressed higher toward the sinus node (Chandler et al., 2009, 2011; Allah et al., 2011). This gradual increase of conductance from sinus node toward atrial myocardium enables a proper current-to-load match. Therefore, genes involved in sinus node insulation and exit pathway formation are candidates for being associated with sinus node function (or heart rate) in GWAS. Accordingly, a locus near GJA1 is associated with heart rate in GWAS (den Hoed et al., 2013).
The electrotonic influence of atrial myocardium on sinus node function is important to consider when investigating the relevance of genes provided by GWAS. For instance, common and rare variants in MYH6 – a component of the sarcomere – are associated with heart rate and sick sinus syndrome (Holm et al., 2010, 2011). However, MYH6 is more abundantly expressed in the atrial myocardium than in the sinus node (van Eif et al., 2019). In vitro experiments in atrial-like cells indicate that mutations in MYH6 affect conduction velocity (Ishikawa et al., 2015). It is possible that conduction slowing in the atrium underlies the association between MYH6 and abnormal function of the sinus node. That genes expressed in the atrium but not in the sinus node can affect sinus is further illustrated by Cx40 knock-out mice in which the dominant pacemaker is not always the SAN (Bagwe et al., 2005). Moreover, mutations in SCN5A can result in sick sinus syndrome (Benson et al., 2003) despite the lack of SCN5A expression in the sinus node.
GWAS Loci Related to PR Interval
The PR interval is mainly determined by conduction through the atrioventricular junction (Meijler and Janse, 1988). The atrioventricular junction is a complex anatomical structure which was first described in 1906 by Sunao Tawara, who found cells forming a compact complex network but also small cells that joined into bundles (Tawara, 1906). These cells were later called compact nodal cells and transitional cells, respectively, and both have distinct electrophysiological properties (de Carvalho and de Almeida, 1960; Greener et al., 2011). A layer of transitional cells can be found in rings around the orifices of the AV valves where expression of Cx40 and SCN5A is low (Aanhaanen et al., 2010; Fedorov et al., 2011). This transitional ring lies on top of an atrioventricular nodal ring providing two conducting pathways with different electrophysiological characteristics both connecting the atria with the His bundle (Denes et al., 1973; Figure 1B). The presence of two conduction pathways illustrates the challenge of relating gene expression of single atrioventricular nodal cells to the electrophysiological phenotype of the atrioventricular node. Moreover, in the atrioventricular junction, not only the compact atrioventricular node contributes to atrioventricular delay but also all other cells present within the AV junction – e.g., transitional cells, fibroblasts and macrophages (Hulsmans et al., 2017). Similar to the sinus node, the function of the atrioventricular node depends on the interaction with these different cell-types.
The cells of the atrioventricular junction find their origin in the atrioventricular canal of the embryonic heart. Impulse conduction delay occurs in both the transitional cells and the compact atrioventricular node, therefore, normal development of the atrioventricular canal is crucial for atrioventricular delay in adult hearts (Meijler and Janse, 1988). Accordingly, GWAS for PR interval showed association with 18 of 44 loci which are related to heart development (van Setten et al., 2018), indicating that proper embryonic development is a crucial factor for adult AV conduction. Some of these loci are located near TBX2 and TBX3 – essential transcription factors controlling patterning of the atrioventricular canal during development (Holm et al., 2010; Pfeufer et al., 2010; Aanhaanen et al., 2011; van Setten et al., 2018; Table 1). Other loci are located nearby genes related to electrical function of the adult cardiomyocyte, like SCN5A and CAMK2D or cardiomyocyte contraction, like TTN and MYH6, however, the significance of these associations with PR interval await further investigation (Holm et al., 2010; van Setten et al., 2018).
GWAS Loci Related to QRS Duration
Total ventricular activation time is visualized on the ECG as the duration of the QRS complex, which comprises conduction in the His-Purkinje system and in the ventricular myocardium. The cardiac sodium channel Nav1.5 – encoded by SCN5A – is the major determinant of conduction in these tissues and, accordingly, QRS duration is associated with loci near SCN5A (Papadatos et al., 2002; Sotoodehnia et al., 2010). Variants in the SCN10A gene – encoding the neuronal sodium channel Nav1.8 – associate with QRS duration as well (Sotoodehnia et al., 2010). These variants are located within an enhancer region that modulates expression of SCN5A which could explain a relation with QRS duration (Sotoodehnia et al., 2010; van den Boogaard et al., 2014). Although variants in these region do not always associate with QRS prolongation (Behr et al., 2015). Other variants related to QRS duration are those near or within genes involved in bundle branch development and working myocardial phenotype, like TBX3, TBX5, TBX20, HAND1, DKK1, and NFIA (Moskowitz et al., 2007; Bakker et al., 2008; Singh et al., 2009; Sotoodehnia et al., 2010). In addition variants in calcium handling genes such as PLN, CACNA1D, STRN, PRKCA, and CASQ2, ATP2A2/ANAPC7 are associated with QRS duration (Sotoodehnia et al., 2010; van Setten et al., 2019). These variants could affect calcium homeostasis resulting in reduced sodium current and slow conduction and thereby prolong QRS duration (King et al., 2013).
The Purkinje network activates the ventricular myocardium via Purkinje muscle junctions composed of transitional cells connecting the Purkinje fibers to ventricular cardiomyocytes (Figure 1B; Martinez-Palomo et al., 1970; Tranum-Jensen et al., 1991). Similar to the AV node and the SAN, the connection of Purkinje cardiomyocytes with ventricular cardiomyocytes requires high resistance – preventing current-to-load mismatch (Rohr et al., 1997). We expect that reduction of electrical coupling in these junctions – by e.g., lower expression of CX40 – will delay ventricular activation, which prolongs QRS duration. Purkinje fibers develop from embryonic trabeculae (Jensen et al., 2012) where e.g., Cx40, Scn5a, CnTn2 are abundantly present. The primordial Purkinje trabeculae require further specialization after birth under influence of Nkx2-5 and Irx3 expression and Notch signaling (Zhang et al., 2011; Rentschler et al., 2012). Homozygous Irx3 loss-of-function mice have slowed conduction in Purkinje fibers (Koizumi et al., 2016). We expect that genetic variations affecting expression of these factors – that are involved in development and maturation of the Purkinje fibers – will relate to total ventricular activation time and thereby QRS duration in GWAS.
GWAS Loci Related to QT Interval
The QT interval is a measure of ventricular repolarization and reflects the time between the first moment of activation to the last moment of repolarization. Accordingly, the QT interval relates to action potential duration (APD) but also to differences in regional conduction velocity. Conduction slowing in regions that repolarize late prolongs QT interval whereas conduction slowing in areas that repolarize early may not affect QT interval. The QT interval has an inverse relation with heart rate. This inverse relation results from shortening of the APD at higher rates due to activation of the slowly delayed rectifier current IKs (Boyett and Jewell, 1978; Carmeliet, 2006). QT intervals corrected for heart rate – QTc – or QT interval measured at similar heart rates may increase sensitivity for finding variants related to repolarization (Bazett, 1920). In mice, however, QT interval does not depend on heart rate. Therefore, QT interval – not QTc – should be used as measure for ventricular repolarization in mice (Speerschneider and Thomsen, 2013).
Genome Wide Association Studies for QT interval identified 22 loci (Table 1) of which many are in or near genes encoding for potassium channels or involved in calcium handling (Arking et al., 2014). Increased potassium current shortens QT interval whereas increased calcium current prolongs QT interval (Wemhöner et al., 2015; Landstrom et al., 2016). Nitric Oxide Synthase 1 Adaptor Protein (NOS1AP) – regulating calcium current – is associated with QT interval in GWAS (Arking et al., 2006, 2014). Genetic variation within the NOS1AP gene affects QT interval and is related to arrhythmogenesis in patients with the long QT syndrome (Crotti et al., 2009; Tomás et al., 2010). The effect of NOS1AP on QT interval, however, could also have an extracardiac pathway as its expression is high in brain (Xu et al., 2005) providing the possibility of NOS1AP to affect autonomic modulation of the QT interval (Yagishita et al., 2015).
GWAS Loci Related to Arrhythmias
Atrial Arrhythmias
Atrial fibrillation (AF) is the most common arrhythmia and the prevalence increases with age (Krijthe et al., 2013). AF results from an interplay between electrical (triggers and reentry), structural and hemodynamic remodeling (Schotten et al., 2011). The combination of these pathophysiological changes sets the stage for AF.
The trigger for AF is commonly near the connection between the left atrium and pulmonary veins (PVs) (Haïssaguerre et al., 1998). In humans – at this connection – the four PVs are enclosed by an outer sleeve of myocardium (Saito et al., 2000). PV cardiomyocytes are morphologically similar to atrial cardiomyocytes, but have a different developmental history (Verheule et al., 2002; Mommersteeg et al., 2007a). The formation of PV myocardium in mice highly depends on expression of Pitx2 and Nkx2-5 during development (Mommersteeg et al., 2007a). GWAS in more than 65 thousand AF patients identified 97 loci (Roselli et al., 2018) – including PITX2 and NKX2-5, suggesting a relation of AF with PV formation during development. The myocardial sleeves of the PVs are thinner distal to the left atrium and end in single cardiomyocyte protrusions in the PV (Figure 1B; Verheule et al., 2002). These PV myocytes have a high resting membrane potential and low expression of inward rectifier channels (Ehrlich et al., 2003; Melnyk et al., 2005), setting the stage for spontaneous activity. Accordingly, variants near genes encoding inward rectifier channels – e.g., KCNJ2, KCNJ5 – are associated with AF (Christophersen et al., 2017; Roselli et al., 2018).
Electrical remodeling is a cause and effect of reentry circuits that maintain AF. Reentry is facilitated by slow conduction and short APD (Wiener and Rosenblueth, 1946). Accordingly, GWAS for AF identified six genes encoding for potassium channels all involved in atrial APD: KCND3, KCNH2, KCNN2, and KCNN3 (Roselli et al., 2018). Genes encoding channels involved in conduction – e.g., GJA1, GJA5, SCN5A, and SCN10A are also associated with AF (Christophersen et al., 2017; Roselli et al., 2018). Moreover, transcription factors involved in spatiotemporal expression of these ion channels – TBX3, TBX5, and PITX2 – associate with AF as well (Tao et al., 2014; Nadadur et al., 2016). Novel findings have indicated that not only genes encoding ion channel are related to AF. Titin (TTN) – a large sarcomere protein – is associated with early onset AF (Choi et al., 2018). TTN dysfunction may predispose to AF by increasing myocardial fibrosis and prolonging PR interval, which are both associated with increased risk for AF (Ahlberg et al., 2018).
Ventricular Arrhythmias
Up to 80% of sudden cardiac arrests (SCA) are caused by acute ischemia resulting from coronary artery disease (Huikuri et al., 2001; Fishman et al., 2010). GWAS in patients with coronary artery disease identified 11 variants related to SCA of which eight were near or within genes related to long QT syndrome (Marsman et al., 2014). It is unclear whether these variants predispose to arrhythmias in general or only in the setting of coronary artery disease. A gene that does specifically relate to arrhythmias in the setting of acute myocardial ischemia is CXADR which encodes the CXADR Ig-like cell adhesion molecule (previously named Coxsackie and adenovirus receptor, CAR) (Bezzina et al., 2010). Reduced expression of CAR lowers sodium channel availability – thereby reducing conduction velocity – and facilitates reentry arrhythmias in the setting of ischemia (Marsman et al., 2014). Non-ischemia induced arrhythmias explain 20% of SCA and comprise a variety of arrhythmogenic syndromes which can be related to structural abnormal myocardium or genetic mutations (John et al., 2012). GWAS for SCA identified several genes which all associate with risk factors for SCA such as QRS duration and QT interval (Adabag et al., 2010; Arking et al., 2011; Milano et al., 2016; Ashar et al., 2018).
Genome Wide Association Studies for syncope – which is a common symptom of many arrhythmogenic syndromes – identified a genetic variant close in proximity to the gene zinc finger protein 804a (ZNF804A) (Hadji-Turdeghal et al., 2020). Whether a role of ZNF804A in cardiac arrhythmias or e.g., blood pressure regulation explains this association remains to be investigated.
Ventricular fibrillation is associated with IRX3 (Koizumi et al., 2016), which plays a role in conduction in Purkinje fibers. Ablation of Purkinje muscle junctions is a successful treatment for a subset of patients with idiopathic ventricular fibrillation indicating a role of Purkinje cells in these arrhythmias (Haïssaguerre et al., 2002).
Understanding arrhythmogenic mechanisms can guide interpretation of GWAS derived data. This is exemplified by the Brugada syndrome, which is characterized by ST segment elevation in the right precordial leads and ventricular arrhythmias. Initially, the Brugada syndrome was considered an ion channel disease mainly resulting from dysfunction of the cardiac sodium channel. However, only 20% of patients present with mutations in SCN5A (Antzelevitch et al., 2005). A GWAS in Brugada syndrome patients identified several variants in regulatory DNA controlling SCN5A expression (Bezzina et al., 2013). In order to discover causal variants in GWAS with limited number of patients, knowledge on the arrhythmogenic mechanism of the disease is helpful. Mechanistic studies in hearts from Brugada syndrome patients have suggested that arrhythmias occur due to conduction block in the presence of subtle structural abnormalities (Coronel et al., 2005; Hoogendijk et al., 2010). This suggests that variants near genes involved in the formation of fibrosis (TGFB2) or genes affecting safety factor of cardiac conduction (e.g., SCN5A, GJA1, KCHIP1) are important variants to further investigate.
Conclusion
Most of the variants provided by GWAS lie near or within expected candidate genes potentially explaining the phenotype they are associated with. However, the electrophysiological characterization of the vast majority of associated genes has not shown direct effects on ECG intervals nor on arrhythmia susceptibility. In this review, we emphasize that mechanistic knowledge of the structure-function relations underlying ECG intervals and arrhythmias should be considered when interpreting experimental characterization of these variants, in order to guide clinical applicability of GWAS data.
Author Contributions
KS and BB designed and wrote the manuscript. VM, CG-M, and EL critically revised the manuscript.
Funding
This work was supported by the Dutch Heart Foundation (2016T047 to BB), the Dutch Research Council (NWO Talent Scheme VIDI-91718361 to EL), and the CVON (Dutch cardiovascular allowance RESCUED project to EL).
Conflict of Interest
The authors declare that the research was conducted in the absence of any commercial or financial relationships that could be construed as a potential conflict of interest.
References
Aanhaanen, W. T. J., Mommersteeg, M. T. M., Norden, J., Wakker, V., de Gier-de Vries, C., Anderson, R. H., et al. (2010). Developmental origin, growth, and three-dimensional architecture of the atrioventricular conduction axis of the mouse heart. Circ. Res. 107, 728–736. doi: 10.1161/CIRCRESAHA.110.222992
Aanhaanen, W. T. J. J., Boukens, B. J. D. D., Sizarov, A., Wakker, V., de Gier-de Vries, C., van Ginneken, A. C., et al. (2011). Defective Tbx2-dependent patterning of the atrioventricular canal myocardium causes accessory pathway formation in mice. J. Clin. Invest. 121, 534–544. doi: 10.1172/JCI44350
Adabag, A. S., Luepker, R. V., Roger, V. L., and Gersh, B. J. (2010). Sudden cardiac death: epidemiology and risk factors. Nat. Rev. Cardiol. 7, 216–225. doi: 10.1038/nrcardio.2010.3
Ahlberg, G., Refsgaard, L., Lundegaard, P. R., Andreasen, L., Ranthe, M. F., Linscheid, N., et al. (2018). Rare truncating variants in the sarcomeric protein titin associate with familial and early-onset atrial fibrillation. Nat. Commun. 9:4316. doi: 10.1038/s41467-018-06618-y
Allah, E. A., Tellez, J. O., Yanni, J., Nelson, T., Monfredi, O., Boyett, M. R., et al. (2011). Changes in the expression of ion channels, connexins and Ca2+-handling proteins in the sino-atrial node during postnatal development. Exp. Physiol. 96, 426–438. doi: 10.1113/expphysiol.2010.055780
Amend, N., Thiermann, H., Worek, F., and Wille, T. (2019). The arrhythmogenic potential of nerve agents and a cardiac safety profile of antidotes – A proof-of-concept study using human induced pluripotent stem cells derived cardiomyocytes (hiPSC-CM). Toxicol. Lett. 308, 1–6. doi: 10.1016/j.toxlet.2019.03.003
Antzelevitch, C., Brugada, P., Borggrefe, M., Brugada, J., Brugada, R., Corrado, D., et al. (2005). Brugada syndrome: report of the second consensus conference: endorsed by the heart rhythm society and the European heart rhythm association. Circulation 111, 659–670. doi: 10.1161/01.CIR.0000152479.54298.51
Arking, D. E., Junttila, M. J., Goyette, P., Huertas-Vazquez, A., Eijgelsheim, M., Blom, M. T., et al. (2011). Identification of a sudden cardiac death susceptibility locus at 2q24.2 through genome-wide association in European ancestry individuals. PLoS Genet. 7:e1002158. doi: 10.1371/journal.pgen.1002158
Arking, D. E., Pfeufer, A., Post, W., Kao, W. H. L., Newton-Cheh, C., Ikeda, M., et al. (2006). A common genetic variant in the NOS1 regulator NOS1AP modulates cardiac repolarization. Nat. Genet. 38, 644–651. doi: 10.1038/ng1790
Arking, D. E., Pulit, S. L., Crotti, L., van der Harst, P., Munroe, P. B., Koopmann, T. T., et al. (2014). Genetic association study of QT interval highlights role for calcium signaling pathways in myocardial repolarization. Nat. Genet. 46, 826–836. doi: 10.1038/ng.3014
Ashar, F. N., Mitchell, R. N., Albert, C. M., Newton-Cheh, C., Brody, J. A., Müller-Nurasyid, M., et al. (2018). A comprehensive evaluation of the genetic architecture of sudden cardiac arrest. Eur. Heart J. 39, 3961–3969. doi: 10.1093/eurheartj/ehy474
Bagwe, S., Berenfeld, O., Vaidya, D., Morley, G. E., and Jalife, J. (2005). Altered right atrial excitation and propagation in connexin40 knockout mice. Circulation 112, 2245–2253. doi: 10.1161/CIRCULATIONAHA.104.527325
Bai, Y., Jones, P. P., Guo, J., Zhong, X., Clark, R. B., Zhou, Q., et al. (2013). Phospholamban knockout breaks arrhythmogenic Ca2+ waves and suppresses catecholaminergic polymorphic ventricular tachycardia in mice. Circ. Res. 113, 517–526. doi: 10.1161/CIRCRESAHA.113.301678
Bakker, M. L., Boukens, B. J., Mommersteeg, M. T. M. M., Brons, J. F., Wakker, V., Moorman, A. F. M. M., et al. (2008). Transcription factor Tbx3 is required for the specification of the atrioventricular conduction system. Circ. Res. 102, 1340–1349. doi: 10.1161/CIRCRESAHA.107.169565
Barbuti, A., Scavone, A., Mazzocchi, N., Terragni, B., Baruscotti, M., and Difrancesco, D. (2012). A caveolin-binding domain in the HCN4 channels mediates functional interaction with caveolin proteins. J. Mol. Cell. Cardiol. 53, 187–195. doi: 10.1016/j.yjmcc.2012.05.013
Barefield, D. Y., Puckelwartz, M. J., Kim, E. Y., Wilsbacher, L. D., Vo, A. H., Waters, E. A., et al. (2017). Experimental modeling supports a role for MyBP-HL as a novel myofilament component in arrhythmia and dilated cardiomyopathy. Circulation 136, 1477–1491. doi: 10.1161/CIRCULATIONAHA.117.028585
Barwe, S. P., Jordan, M. C., Skay, A., Inge, L., Rajasekaran, S. A., Wolle, D., et al. (2009). Dysfunction of ouabain-induced cardiac contractility in mice with heart-specific ablation of Na,K-ATPase beta1-subunit. J. Mol. Cell. Cardiol. 47, 552–560. doi: 10.1016/j.yjmcc.2009.07.018
Bazett, H. C. (1920). An analysis of the time-relations of electrocardiograms. Heart 7, 353–370. doi: 10.1111/j.1542-474X.1997.tb00325.x
Behr, E. R., Savio-Galimberti, E., Barc, J., Holst, A. G., Petropoulou, E., Prins, B. P., et al. (2015). Role of common and rare variants in SCN10A: results from the Brugada syndrome QRS locus gene discovery collaborative study. Cardiovasc. Res. 106, 520–529. doi: 10.1093/cvr/cvv042
Bender, K., Wellner-Kienitz, M. C., Inanobe, A., Meyer, T., Kurachi, Y., and Pott, L. (2001). Overexpression of monomeric and multimeric GIRK4 subunits in rat atrial myocytes removes fast desensitization and reduces inward rectification of muscarinic K(+) current (I(K(ACh))). Evidence for functional homomeric GIRK4 channels. J. Biol. Chem. 276, 28873–28880. doi: 10.1074/jbc.M102328200
Benson, D. W., Wang, D. W., Dyment, M., Knilans, T. K., Fish, F. A., Strieper, M. J., et al. (2003). Congenital sick sinus syndrome caused by recessive mutations in the cardiac sodium channel gene (SCN5A). J. Clin. Invest. 112, 1019–1028. doi: 10.1172/JCI200318062
Berecki, G., Wilders, R., de Jonge, B., van Ginneken, A. C. G., and Verkerk, A. O. (2010). Re-evaluation of the action potential upstroke velocity as a measure of the Na+ current in cardiac myocytes at physiological conditions. PLoS One 5:e15772. doi: 10.1371/journal.pone.0015772
Bezzina, C. R., Barc, J., Mizusawa, Y., Remme, C. A., Gourraud, J.-B., Simonet, F., et al. (2013). Common variants at SCN5A-SCN10A and HEY2 are associated with Brugada syndrome, a rare disease with high risk of sudden cardiac death. Nat. Genet. 45, 1044–1049. doi: 10.1038/ng.2712
Bezzina, C. R., Pazoki, R., Bardai, A., Marsman, R. F., de Jong, J. S. S. G., Blom, M. T., et al. (2010). Genome-wide association study identifies a susceptibility locus at 21q21 for ventricular fibrillation in acute myocardial infarction. Nat. Genet. 42, 688–691. doi: 10.1038/ng.623
Bleeker, W. K., Mackaay, A. J. C., Masson-Pevet, M., Bouman, L. N., and Becker, A. E. (1980). Functional and morphological organization of the rabbit sinus node. Circ. Res. 46, 11–22. doi: 10.1161/01.RES.46.1.11
Boukens, B. J., Rivaud, M. R., Rentschler, S., and Coronel, R. (2014). Misinterpretation of the mouse ECG: “Musing the waves of Mus musculus. J. Physiol. 592, 4613–4626. doi: 10.1113/jphysiol.2014.279380
Boyett, M. R., and Jewell, B. R. (1978). A study of the factors responsible for rate-dependent shortening of the action potential in mammalian ventricular muscle. J. Physiol. 285, 359–380. doi: 10.1113/jphysiol.1978.sp012576
Caballero, R., Utrilla, R. G., Amorós, I., Matamoros, M., Pérez-Hernández, M., Tinaquero, D., et al. (2017). Tbx20 controls the expression of the KCNH2 gene and of hERG channels. Proc. Natl. Acad. Sci. U.S.A. 114, E416–E425. doi: 10.1073/pnas.1612383114
Carmeliet, E. (2006). Action potential duration, rate of stimulation, and intracellular sodium. J. Cardiovasc. Electrophysiol. 17(Suppl. 1), S2–S7. doi: 10.1111/j.1540-8167.2006.00378.x
Chandler, N., Aslanidi, O., Buckley, D., Inada, S., Birchall, S., Atkinson, A., et al. (2011). Computer three-dimensional anatomical reconstruction of the human sinus node and a novel paranodal area. Anat. Rec. (Hoboken). 294, 970–979. doi: 10.1002/ar.21379
Chandler, N. J., Greener, I. D., Tellez, J. O., Inada, S., Musa, H., Molenaar, P., et al. (2009). Molecular architecture of the human sinus node insights into the function of the cardiac pacemaker. Circulation 119, 1562–1575. doi: 10.1161/CIRCULATIONAHA.108.804369
Cheng, W.-L., Kao, Y.-H., Chao, T.-F., Lin, Y.-K., Chen, S.-A., and Chen, Y.-J. (2019). MicroRNA-133 suppresses ZFHX3-dependent atrial remodelling and arrhythmia. Acta Physiol. (Oxf). 227:e13322. doi: 10.1111/apha.13322
Choi, S. H., Weng, L.-C., Roselli, C., Lin, H., Haggerty, C. M., Shoemaker, M. B., et al. (2018). Association between titin loss-of-function variants and early-onset atrial fibrillation. JAMA 320, 2354–2364. doi: 10.1001/jama.2018.18179
Christophersen, I. E., Rienstra, M., Roselli, C., Yin, X., Geelhoed, B., Barnard, J., et al. (2017). Large-scale analyses of common and rare variants identify 12 new loci associated with atrial fibrillation. Nat. Genet. 49, 946–952. doi: 10.1038/ng.3843
Coronel, R., Casini, S., Koopmann, T. T., Wilms-Schopman, F. J. G., Verkerk, A. O., de Groot, J. R., et al. (2005). Right ventricular fibrosis and conduction delay in a patient with clinical signs of Brugada syndrome: a combined electrophysiological, genetic, histopathologic, and computational study. Circulation 112, 2769–2777. doi: 10.1161/CIRCULATIONAHA.105.532614
Crotti, L., Monti, M. C., Insolia, R., Peljto, A., Goosen, A., Brink, P. A., et al. (2009). NOS1AP is a genetic modifier of the long-QT syndrome. Circulation 120, 1657–1663. doi: 10.1161/CIRCULATIONAHA.109.879643
Csepe, T. A., Kalyanasundaram, A., Hansen, B. J., Zhao, J., and Fedorov, V. V. (2015). Fibrosis: a structural modulator of sinoatrial node physiology and dysfunction. Front. Physiol. 6:37. doi: 10.3389/fphys.2015.00037
Dai, W., Laforest, B., Tyan, L., Shen, K. M., Nadadur, R. D., Alvarado, F. J., et al. (2019). A calcium transport mechanism for atrial fibrillation in Tbx5-mutant mice. eLife 8:e41814. doi: 10.7554/eLife.41814
de Carvalho, A., and de Almeida, D. (1960). Spread of activity through the atrioventricular node. Circ. Res. 8, 801–809. doi: 10.1161/01.RES.8.4.801
den Hoed, M., Eijgelsheim, M., Esko, T., Brundel, B. J. J. M., Peal, D. S., Evans, D. M., et al. (2013). Identification of heart rate-associated loci and their effects on cardiac conduction and rhythm disorders. Nat. Genet. 45, 621–631. doi: 10.1038/ng.2610
Denes, P., Wu, D., Dhingra, R. C., Chuquimia, R., and Rosen, K. M. (1973). Demonstration of dual A-V nodal pathways in patients with paroxysmal supraventricular tachycardia. Circulation 48, 549–555. doi: 10.1161/01.CIR.48.3.549
DiFrancesco, D., and Borer, J. S. (2007). The funny current: cellular basis for the control of heart rate. Drugs 67(Suppl. 2), 15–24. doi: 10.2165/00003495-200767002-00003
Drici, M. D., Arrighi, I., Chouabe, C., Mann, J. R., Lazdunski, M., Romey, G., et al. (1998). Involvement of IsK-associated K+ channel in heart rate control of repolarization in a murine engineered model of Jervell and Lange-Nielsen syndrome. Circ. Res. 83, 95–102. doi: 10.1161/01.res.83.1.95
Dustrude, E. T., Wilson, S. M., Ju, W., Xiao, Y., and Khanna, R. (2013). CRMP2 protein SUMOylation modulates NaV1.7 channel trafficking. J. Biol. Chem. 288, 24316–24331. doi: 10.1074/jbc.M113.474924
Ehrlich, J. R., Cha, T.-J., Zhang, L., Chartier, D., Melnyk, P., Hohnloser, S. H., et al. (2003). Cellular electrophysiology of canine pulmonary vein cardiomyocytes: action potential and ionic current properties. J. Physiol. 551, 801–813. doi: 10.1113/jphysiol.2003.046417
Eijgelsheim, M., Newton-Cheh, C., Sotoodehnia, N., de Bakker, P. I. W., Müller, M., Morrison, A. C., et al. (2010). Genome-wide association analysis identifies multiple loci related to resting heart rate. Hum. Mol. Genet. 19, 3885–3894. doi: 10.1093/hmg/ddq303
Eldabah, N., Nembo, E. N., Penner, M., Semmler, J., Swelem, R., Hassab, A., et al. (2018). Altered functional expression of β-Adrenergic receptors in rhesus monkey embryonic stem cell-derived cardiomyocytes. Stem Cells Dev. 27, 336–346. doi: 10.1089/scd.2017.0053
Fedorov, V. V., Ambrosi, C. M., Kostecki, G., Hucker, W. J., Glukhov, A. V., Wuskell, J. P., et al. (2011). Anatomic localization and autonomic modulation of atrioventricular junctional rhythm in failing human hearts. Circ. Arrhythm. Electrophysiol. 4, 515–525. doi: 10.1161/CIRCEP.111.962258
Fedorov, V. V., Glukhov, A. V., Chang, R., Kostecki, G., Aferol, H., Hucker, W. J., et al. (2010). Optical mapping of the isolated coronary-perfused human sinus node. J. Am. Coll. Cardiol. 56, 1386–1394. doi: 10.1016/j.jacc.2010.03.098
Fedorov, V. V., Schuessler, R. B., Hemphill, M., Ambrosi, C. M., Chang, R., Voloshina, A. S., et al. (2009). Structural and functional evidence for discrete exit pathways that connect the canine sinoatrial node and atria. Circ. Res. 104, 915–923. doi: 10.1161/CIRCRESAHA.108.193193
Fishman, G. I., Chugh, S. S., Dimarco, J. P., Albert, C. M., Anderson, M. E., Bonow, R. O., et al. (2010). Sudden cardiac death prediction and prevention: report from a national heart, lung, and blood institute and heart rhythm society workshop. Circulation 122, 2335–2348. doi: 10.1161/CIRCULATIONAHA.110.976092
Glukhov, A. V., Hage, L. T., Hansen, B. J., Pedraza-Toscano, A., Vargas-Pinto, P., Hamlin, R. L., et al. (2013). Sinoatrial node reentry in a canine chronic left ventricular infarct model: role of intranodal fibrosis and heterogeneity of refractoriness. Circ. Arrhythm. Electrophysiol. 6, 984–994. doi: 10.1161/CIRCEP.113.000404
Goldfarb, R. D., Glock, D., Johnson, K., Creasey, A. A., Carr, C., McCarthy, R. J., et al. (1998). Randomized, blinded, placebo-controlled trial of tissue factor pathway inhibitor in porcine septic shock. Shock 10, 258–264. doi: 10.1097/00024382-199810000-00005
Greener, I. D., Monfredi, O., Inada, S., Chandler, N. J., Tellez, J. O., Atkinson, A., et al. (2011). Molecular architecture of the human specialised atrioventricular conduction axis. J. Mol. Cell. Cardiol. 50, 642–651. doi: 10.1016/j.yjmcc.2010.12.017
GTEx Consortium (2013). The genotype-tissue expression (GTEx) project. Nat. Genet. 45, 580–585. doi: 10.1038/ng.2653
Hadji-Turdeghal, K., Andreasen, L., Hagen, C. M., Ahlberg, G., Ghouse, J., Bækvad-Hansen, M., et al. (2020). Genome-wide association study identifies locus at chromosome 2q32.1 associated with syncope and collapse. Cardiovasc. Res. 116, 138–148. doi: 10.1093/cvr/cvz106
Haïssaguerre, M., Jaïs, P., Shah, D. C., Takahashi, A., Hocini, M., Quiniou, G., et al. (1998). Spontaneous initiation of atrial fibrillation by ectopic beats originating in the pulmonary veins. N. Engl. J. Med. 339, 659–666. doi: 10.1056/NEJM199809033391003
Haïssaguerre, M., Shah, D. C., Jaïs, P., Shoda, M., Kautzner, J., Arentz, T., et al. (2002). Role of Purkinje conducting system in triggering of idiopathic ventricular fibrillation. Lancet 359, 677–678. doi: 10.1016/S0140-6736(02)07807-8
Hattori, T., Makiyama, T., Akao, M., Ehara, E., Ohno, S., Iguchi, M., et al. (2012). A novel gain-of-function KCNJ2 mutation associated with short-QT syndrome impairs inward rectification of Kir2.1 currents. Cardiovasc. Res. 93, 666–673. doi: 10.1093/cvr/cvr329
Heinig, M. (2018). Using gene expression to annotate cardiovascular GWAS loci. Front. Cardiovasc. Med. 5:59. doi: 10.3389/fcvm.2018.00059
Herron, T. J., Devaney, E., Mundada, L., Arden, E., Day, S., Guerrero-Serna, G., et al. (2010). Ca2+-independent positive molecular inotropy for failing rabbit and human cardiac muscle by alpha-myosin motor gene transfer. FASEB J. 24, 415–424. doi: 10.1096/fj.09-140566
Holm, H., Gudbjartsson, D. F., Arnar, D. O., Thorleifsson, G., Thorgeirsson, G., Stefansdottir, H., et al. (2010). Several common variants modulate heart rate, PR interval and QRS duration. Nat. Genet. 42, 117–122. doi: 10.1038/ng.511
Holm, H., Gudbjartsson, D. F., Sulem, P., Masson, G., Helgadottir, H. T., Zanon, C., et al. (2011). A rare variant in MYH6 is associated with high risk of sick sinus syndrome. Nat. Genet. 43, 316–323. doi: 10.1038/ng.781
Hoogendijk, M. G., Potse, M., Linnenbank, A. C., Verkerk, A. O., den Ruijter, H. M., van Amersfoorth, S. C. M., et al. (2010). Mechanism of right precordial ST-segment elevation in structural heart disease: excitation failure by current-to-load mismatch. Hear. Rhythm 7, 238–248. doi: 10.1016/j.hrthm.2009.10.007
Howden, R., Cooley, I., Van Dodewaard, C., Arthur, S., Cividanes, S., Leamy, L., et al. (2013). Cardiac responses to 24 hrs hyperoxia in Bmp2 and Bmp4 heterozygous mice. Inhal. Toxicol. 25, 509–516. doi: 10.3109/08958378.2013.808287
Huang, X., Li, Z., Hu, J., Yang, Z., Liu, Z., Zhang, T., et al. (2019). Knockout of Wdr1 results in cardiac hypertrophy and impaired cardiac function in adult mouse heart. Gene 697, 40–47. doi: 10.1016/j.gene.2019.02.023
Huikuri, H. V., Castellanos, A., and Myerburg, R. J. (2001). Sudden death due to cardiac arrhythmias. N. Engl. J. Med. 345, 1473–1482. doi: 10.1056/NEJMra000650
Hulsmans, M., Clauss, S., Xiao, L., Aguirre, A. D., King, K. R., Hanley, A., et al. (2017). Macrophages facilitate electrical conduction in the heart. Cell 169, 510–522.e20. doi: 10.1016/j.cell.2017.03.050
Inoue, T., Lin, X., Kohlmeier, K. A., Orr, H. T., Zoghbi, H. Y., and Ross, W. N. (2001). Calcium dynamics and electrophysiological properties of cerebellar Purkinje cells in SCA1 transgenic mice. J. Neurophysiol. 85, 1750–1760. doi: 10.1152/jn.2001.85.4.1750
Ishikawa, T., Jou, C. J., Nogami, A., Kowase, S., Arrington, C. B., Barnett, S. M., et al. (2015). Novel mutation in the α-myosin heavy chain gene is associated with sick sinus syndrome. Circ. Arrhythmia Electrophysiol. 8, 400–408. doi: 10.1161/CIRCEP.114.002534
Jensen, B., Boukens, B. J. D., Postma, A. V., Gunst, Q. D., van den Hoff, M. J. B., Moorman, A. F. M., et al. (2012). Identifying the evolutionary building blocks of the cardiac conduction system. PLoS One 7:e44231. doi: 10.1371/journal.pone.0044231
John, R. M., Tedrow, U. B., Koplan, B. A., Albert, C. M., Epstein, L. M., Sweeney, M. O., et al. (2012). Ventricular arrhythmias and sudden cardiac death. Lancet 380, 1520–1529. doi: 10.1016/S0140-6736(12)61413-5
Joyner, R. W., and van Capelle, F. J. (1986). Propagation through electrically coupled cells. How a small SA node drives a large atrium. Biophys. J. 50, 1157–1164. doi: 10.1016/S0006-3495(86)83559-7
Kao, Y.-H., Hsu, J.-C., Chen, Y.-C., Lin, Y.-K., Lkhagva, B., Chen, S.-A., et al. (2016). ZFHX3 knockdown increases arrhythmogenesis and dysregulates calcium homeostasis in HL-1 atrial myocytes. Int. J. Cardiol. 210, 85–92. doi: 10.1016/j.ijcard.2016.02.091
Kapoor, A., Sekar, R. B., Hansen, N. F., Fox-Talbot, K., Morley, M., Pihur, V., et al. (2014). An enhancer polymorphism at the cardiomyocyte intercalated disc protein NOS1AP locus is a major regulator of the QT interval. Am. J. Hum. Genet. 94, 854–869. doi: 10.1016/j.ajhg.2014.05.001
Kelly, R. G., Buckingham, M. E., and Moorman, A. F. (2014). Heart fields and cardiac morphogenesis. Cold Spring Harb. Perspect. Med. 4:a015750. doi: 10.1101/cshperspect.a015750
King, J. H., Wickramarachchi, C., Kua, K., Du, Y., Jeevaratnam, K., Matthews, H. R., et al. (2013). Loss of Nav1.5 expression and function in murine atria containing the RyR2-P2328S gain-of-function mutation. Cardiovasc. Res. 99, 751–759. doi: 10.1093/cvr/cvt141
Kirchhof, C. J., Bonke, F. I., Allessie, M. A., and Lammers, W. J. (1987). The influence of the atrial myocardium on impulse formation in the rabbit sinus node. Pflugers Arch. 410, 198–203. doi: 10.1007/bf00581916
Kléber, A. G., and Rudy, Y. (2004). Basic mechanisms of cardiac impulse propagation and associated arrhythmias. Physiol. Rev. 84, 431–488. doi: 10.1152/physrev.00025.2003
Knollmann, B. C., Casimiro, M. C., Katchman, A. N., Sirenko, S. G., Schober, T., Rong, Q., et al. (2004). Isoproterenol exacerbates a long QT phenotype in Kcnq1-deficient neonatal mice: possible roles for human-like Kcnq1 isoform 1 and slow delayed rectifier K+ current. J. Pharmacol. Exp. Ther. 310, 311–318. doi: 10.1124/jpet.103.063743
Koizumi, A., Sasano, T., Kimura, W., Miyamoto, Y., Aiba, T., Ishikawa, T., et al. (2016). Genetic defects in a His-Purkinje system transcription factor, IRX3, cause lethal cardiac arrhythmias. Eur. Heart J. 37, 1469–1475. doi: 10.1093/eurheartj/ehv449
Krijthe, B. P., Kunst, A., Benjamin, E. J., Lip, G. Y. H., Franco, O. H., Hofman, A., et al. (2013). Projections on the number of individuals with atrial fibrillation in the European Union, from 2000 to 2060. Eur. Heart J. 34, 2746–2751. doi: 10.1093/eurheartj/eht280
Lakatta, E. G., and DiFrancesco, D. (2009). What keeps us ticking: a funny current, a calcium clock, or both? J. Mol. Cell. Cardiol. 47, 157–170. doi: 10.1016/j.yjmcc.2009.03.022
Landstrom, A. P., Boczek, N. J., Ye, D., Miyake, C. Y., De la Uz, C. M., Allen, H. D., et al. (2016). Novel long QT syndrome-associated missense mutation, L762F, in CACNA1C-encoded L-type calcium channel imparts a slower inactivation tau and increased sustained and window current. Int. J. Cardiol. 220, 290–298. doi: 10.1016/j.ijcard.2016.06.081
Li, A., Ahsen, O. O., Liu, J. J., Du, C., McKee, M. L., Yang, Y., et al. (2013). Silencing of the Drosophila ortholog of SOX5 in heart leads to cardiac dysfunction as detected by optical coherence tomography. Hum. Mol. Genet. 22, 3798–3806. doi: 10.1093/hmg/ddt230
Li, H., Li, D., Wang, Y., Huang, Z., Xu, J., Yang, T., et al. (2019). Nkx2-5 defines a subpopulation of pacemaker cells and is essential for the physiological function of the sinoatrial node in mice. Development 146:dev178145. doi: 10.1242/dev.178145
Li, N., Hansen, B. J., Csepe, T. A., Zhao, J., Ignozzi, A. J., Sul, L. V., et al. (2017). Redundant and diverse intranodal pacemakers and conduction pathways protect the human sinoatrial node from failure. Sci. Transl. Med. 9:eaam5607. doi: 10.1126/scitranslmed.aam5607
Li, N., Timofeyev, V., Tuteja, D., Xu, D., Lu, L., Zhang, Q., et al. (2009). Ablation of a Ca2+-activated K+ channel (SK2 channel) results in action potential prolongation in atrial myocytes and atrial fibrillation. J. Physiol. 587, 1087–1100. doi: 10.1113/jphysiol.2008.167718
Liu, G.-Z., Hou, T.-T., Yuan, Y., Hang, P.-Z., Zhao, J.-J., Sun, L., et al. (2016). Fenofibrate inhibits atrial metabolic remodelling in atrial fibrillation through PPAR-α/sirtuin 1/PGC-1α pathway. Br. J. Pharmacol. 173, 1095–1109. doi: 10.1111/bph.13438
Liu, Z., Hutt, J. A., Rajeshkumar, B., Azuma, Y., Duan, K. L., and Donahue, J. K. (2017). Preclinical efficacy and safety of KCNH2-G628S gene therapy for postoperative atrial fibrillation. J. Thorac. Cardiovasc. Surg. 154, 1644–1651.e8. doi: 10.1016/j.jtcvs.2017.05.052
Lübkemeier, I., Requardt, R. P., Lin, X., Sasse, P., Andrié, R., Schrickel, J. W., et al. (2013). Deletion of the last five C-terminal amino acid residues of connexin43 leads to lethal ventricular arrhythmias in mice without affecting coupling via gap junction channels. Basic Res. Cardiol. 108:348. doi: 10.1007/s00395-013-0348-y
Lugenbiel, P., Govorov, K., Rahm, A.-K., Wieder, T., Gramlich, D., Syren, P., et al. (2018). Inhibition of histone deacetylases induces K+ channel remodeling and action potential prolongation in HL-1 atrial cardiomyocytes. Cell. Physiol. Biochem. 49, 65–77. doi: 10.1159/000492840
Mahida, S., Mills, R. W., Tucker, N. R., Simonson, B., Macri, V., Lemoine, M. D., et al. (2014). Overexpression of KCNN3 results in sudden cardiac death. Cardiovasc. Res. 101, 326–334. doi: 10.1093/cvr/cvt269
Marsman, R. F. J., Bezzina, C. R., Freiberg, F., Verkerk, A. O., Adriaens, M. E., Podliesna, S., et al. (2014). Coxsackie and adenovirus receptor is a modifier of cardiac conduction and arrhythmia vulnerability in the setting of myocardial ischemia. J. Am. Coll. Cardiol. 63, 549–559. doi: 10.1016/j.jacc.2013.10.062
Martinez-Palomo, A., Alanis, J., and Benitez, D. (1970). Transitional cardiac cells of the conductive system of the dog heart, distinguishing morphological and electrophysiological features. J. Cell Biol. 47, 1–17. doi: 10.1083/jcb.47.1.1
Masuda, Y., and Kawamura, A. (2003). Acetylcholinesterase inhibitor (donepezil hydrochloride) reduces heart rate variability. J. Cardiovasc. Pharmacol. 41(Suppl. 1), S67–S71.
Mazhari, R., Nuss, H. B., Armoundas, A. A., Winslow, R. L., and Marbán, E. (2002). Ectopic expression of KCNE3 accelerates cardiac repolarization and abbreviates the QT interval. J. Clin. Invest. 109, 1083–1090. doi: 10.1172/JCI15062
Meijler, F. L., and Janse, M. J. (1988). Morphology and electrophysiology of the mammalian atrioventricular node. Physiol. Rev. 68, 608–647. doi: 10.1152/physrev.1988.68.2.608
Melnyk, P., Ehrlich, J. R., Pourrier, M., Villeneuve, L., Cha, T.-J., and Nattel, S. (2005). Comparison of ion channel distribution and expression in cardiomyocytes of canine pulmonary veins versus left atrium. Cardiovasc. Res. 65, 104–116. doi: 10.1016/j.cardiores.2004.08.014
Milano, A., Blom, M. T., Lodder, E. M., van Hoeijen, D. A., Barc, J., Koopmann, T. T., et al. (2016). Sudden cardiac arrest and rare genetic variants in the community. Circ. Cardiovasc. Genet. 9, 147–153. doi: 10.1161/CIRCGENETICS.115.001263
Mommersteeg, M. T. M., Brown, N. A., Prall, O. W. J., De Gier-De Vries, C., Harvey, R. P., Moorman, A. F. M., et al. (2007a). Pitx2c and Nkx2-5 are required for the formation and identity of the pulmonary myocardium. Circ. Res. 101, 902–909. doi: 10.1161/CIRCRESAHA.107.161182
Mommersteeg, M. T. M., Hoogaars, W. M. H., Prall, O. W. J., de Gier-de Vries, C., Wiese, C., Clout, D. E. W., et al. (2007b). Molecular pathway for the localized formation of the sinoatrial node. Circ. Res. 100, 354–362. doi: 10.1161/01.RES.0000258019.74591.b3
Montefiori, L. E., Sobreira, D. R., Sakabe, N. J., Aneas, I., Joslin, A. C., Hansen, G. T., et al. (2018). A promoter interaction map for cardiovascular disease genetics. eLife 7:e35788. doi: 10.7554/eLife.35788
Moreno, C., Oliveras, A., de la Cruz, A., Bartolucci, C., Muñoz, C., Salar, E., et al. (2015). A new KCNQ1 mutation at the S5 segment that impairs its association with KCNE1 is responsible for short QT syndrome. Cardiovasc. Res. 107, 613–623. doi: 10.1093/cvr/cvv196
Moskowitz, I. P. G., Kim, J. B., Moore, M. L., Wolf, C. M., Peterson, M. A., Shendure, J., et al. (2007). A molecular pathway including Id2, Tbx5, and Nkx2-5 required for cardiac conduction system development. Cell 129, 1365–1376. doi: 10.1016/j.cell.2007.04.036
Myers, E. J., Yuan, L., Felmlee, M. A., Lin, Y.-Y., Jiang, Y., Pei, Y., et al. (2016). A novel mutant Na+ /HCO3- cotransporter NBCe1 in a case of compound-heterozygous inheritance of proximal renal tubular acidosis. J. Physiol. 594, 6267–6286. doi: 10.1113/JP272252
Nadadur, R. D., Broman, M. T., Boukens, B., Mazurek, S. R., Yang, X., Van Den Boogaard, M., et al. (2016). Pitx2 modulates a Tbx5-dependent gene regulatory network to maintain atrial rhythm. Sci. Transl. Med. 8: 354ra115. doi: 10.1126/scitranslmed.aaf4891
Newton-Cheh, C., Eijgelsheim, M., Rice, K. M., De Bakker, P. I. W., Yin, X., Estrada, K., et al. (2009). Common variants at ten loci influence QT interval duration in the QTGEN Study. Nat. Genet. 41, 399–406. doi: 10.1038/ng.364
Nolte, I. M., Munoz, M. L., Tragante, V., Amare, A. T., Jansen, R., Vaez, A., et al. (2017). Genetic loci associated with heart rate variability and their effects on cardiac disease risk. Nat. Commun. 8:15805. doi: 10.1038/ncomms15805
Noureldin, M., Chen, H., and Bai, D. (2018). Functional characterization of novel atrial fibrillation-linked GJA5 (Cx40) mutants. Int. J. Mol. Sci. 19:977. doi: 10.3390/ijms19040977
Opthof, T., VanGinneken, A. C., Bouman, L. N., and Jongsma, H. J. (1987). The intrinsic cycle length in small pieces isolated from the rabbit sinoatrial node. J. Mol. Cell. Cardiol. 19, 923–934. doi: 10.1016/s0022-2828(87)80621-1
Orvos, P., Kohajda, Z., Szlovák, J., Gazdag, P., Árpádffy-Lovas, T., Tóth, D., et al. (2019). Evaluation of possible proarrhythmic potency: comparison of the effect of dofetilide, cisapride, sotalol, terfenadine, and verapamil on hERG and native IKr currents and on cardiac action potential. Toxicol. Sci. 168, 365–380. doi: 10.1093/toxsci/kfy299
Papadatos, G. A., Wallerstein, P. M. R., Head, C. E. G., Ratcliff, R., Brady, P. A., Benndorf, K., et al. (2002). Slowed conduction and ventricular tachycardia after targeted disruption of the cardiac sodium channel gene Scn5a. Proc. Natl. Acad. Sci. U.S.A. 99, 6210–6215. doi: 10.1073/pnas.082121299
Park, D. S., Woodman, S. E., Schubert, W., Cohen, A. W., Frank, P. G., Chandra, M., et al. (2002). Caveolin-1/3 double-knockout mice are viable, but lack both muscle and non-muscle caveolae, and develop a severe cardiomyopathic phenotype. Am. J. Pathol. 160, 2207–2217. doi: 10.1016/S0002-9440(10)61168-6
Pfeiffer, M. J., Quaranta, R., Piccini, I., Fell, J., Rao, J., Röpke, A., et al. (2018). Cardiogenic programming of human pluripotent stem cells by dose-controlled activation of EOMES. Nat. Commun. 9:440. doi: 10.1038/s41467-017-02812-6
Pfeufer, A., van Noord, C., Marciante, K. D., Arking, D. E., Larson, M. G., Smith, A. V., et al. (2010). Genome-wide association study of PR interval. Nat. Genet. 42, 153–159. doi: 10.1038/ng.517
Posokhova, E., Ng, D., Opel, A., Masuho, I., Tinker, A., Biesecker, L. G., et al. (2013). Essential role of the m2R-RGS6-IKACh pathway in controlling intrinsic heart rate variability. PLoS One 8:e76973. doi: 10.1371/journal.pone.0076973
Posokhova, E., Wydeven, N., Allen, K. L., Wickman, K., and Martemyanov, K. A. (2010). RGS6/Gβ5 complex accelerates IKACh gating kinetics in atrial myocytes and modulates parasympathetic regulation of heart rate. Circ. Res. 107, 1350–1354. doi: 10.1161/CIRCRESAHA.110.224212
Pott, C., Ren, X., Tran, D. X., Yang, M.-J., Henderson, S., Jordan, M. C., et al. (2007). Mechanism of shortened action potential duration in Na+-Ca2+ exchanger knockout mice. Am. J. Physiol. Cell Physiol. 292, C968–C973. doi: 10.1152/ajpcell.00177.2006
Purohit, A., Rokita, A. G., Guan, X., Chen, B., Koval, O. M., Voigt, N., et al. (2013). Oxidized Ca(2+)/calmodulin-dependent protein kinase II triggers atrial fibrillation. Circulation 128, 1748–1757. doi: 10.1161/CIRCULATIONAHA.113.003313
Rentschler, S., Yen, A. H., Lu, J., Petrenko, N. B., Lu, M. M., Manderfield, L. J., et al. (2012). Myocardial Notch signaling reprograms cardiomyocytes to a conduction-like phenotype. Circulation 126, 1058–1066. doi: 10.1161/CIRCULATIONAHA.112.103390
Rocher, A. B., Crimins, J. L., Amatrudo, J. M., Kinson, M. S., Todd-Brown, M. A., Lewis, J., et al. (2010). Structural and functional changes in tau mutant mice neurons are not linked to the presence of NFTs. Exp. Neurol. 223, 385–393. doi: 10.1016/j.expneurol.2009.07.029
Roder, K., Werdich, A. A., Li, W., Liu, M., Kim, T. Y., Organ-Darling, L. E., et al. (2014). RING finger protein RNF207, a novel regulator of cardiac excitation. J. Biol. Chem. 289, 33730–33740. doi: 10.1074/jbc.M114.592295
Rohr, S., Kucera, J. P., Fast, V. G., and Kléber, A. G. (1997). Paradoxical improvement of impulse conduction in cardiac tissue by partial cellular uncoupling. Science 275, 841–844. doi: 10.1126/science.275.5301.841
Roselli, C., Chaffin, M. D., Weng, L.-C., Aeschbacher, S., Ahlberg, G., Albert, C. M., et al. (2018). Multi-ethnic genome-wide association study for atrial fibrillation. Nat. Genet. 50, 1225–1233. doi: 10.1038/s41588-018-0133-9
Sah, R., Mesirca, P., Van den Boogert, M., Rosen, J., Mably, J., Mangoni, M. E., et al. (2013). Ion channel-kinase TRPM7 is required for maintaining cardiac automaticity. Proc. Natl. Acad. Sci. U.S.A. 110, E3037–E3046. doi: 10.1073/pnas.1311865110
Saito, T., Waki, K., and Becker, A. E. (2000). Left atrial myocardial extension onto pulmonary veins in humans: anatomic observations relevant for atrial arrhythmias. J. Cardiovasc. Electrophysiol. 11, 888–894. doi: 10.1111/j.1540-8167.2000.tb00068.x
Sapra, G., Tham, Y. K., Cemerlang, N., Matsumoto, A., Kiriazis, H., Bernardo, B. C., et al. (2014). The small-molecule BGP-15 protects against heart failure and atrial fibrillation in mice. Nat. Commun. 5:5705. doi: 10.1038/ncomms6705
Sato, P. Y., Musa, H., Coombs, W., Guerrero-Serna, G., Patiño, G. A., Taffet, S. M., et al. (2009). Loss of plakophilin-2 expression leads to decreased sodium current and slower conduction velocity in cultured cardiac myocytes. Circ. Res. 105, 523–526. doi: 10.1161/CIRCRESAHA.109.201418
Schotten, U., Verheule, S., Kirchhof, P., and Goette, A. (2011). Pathophysiological mechanisms of atrial fibrillation: a translational appraisal. Physiol. Rev. 91, 265–325. doi: 10.1152/physrev.00031.2009
Shin, J.-H., Bostick, B., Yue, Y., Hajjar, R., and Duan, D. (2011). SERCA2a gene transfer improves electrocardiographic performance in aged mdx mice. J. Transl. Med. 9:132. doi: 10.1186/1479-5876-9-132
Singh, R., Horsthuis, T., Farin, H. F., Grieskamp, T., Norden, J., Petry, M., et al. (2009). Tbx20 interacts with smads to confine tbx2 expression to the atrioventricular canal. Circ. Res. 105, 442–452. doi: 10.1161/CIRCRESAHA.109.196063
Sinner, M. F., Tucker, N. R., Lunetta, K. L., Ozaki, K., Smith, J. G., Trompet, S., et al. (2014). Integrating genetic, transcriptional, and functional analyses to identify 5 novel genes for atrial fibrillation. Circulation 130, 1225–1235. doi: 10.1161/CIRCULATIONAHA.114.009892
Smith, J. G., Magnani, J. W., Palmer, C., Meng, Y. A., Soliman, E. Z., Musani, S. K., et al. (2011). Genome-wide association studies of the PR interval in African Americans. PLoS Genet. 7:e1001304. doi: 10.1371/journal.pgen.1001304
Song, L., Alcalai, R., Arad, M., Wolf, C. M., Toka, O., Conner, D. A., et al. (2007). Calsequestrin 2 (CASQ2) mutations increase expression of calreticulin and ryanodine receptors, causing catecholaminergic polymorphic ventricular tachycardia. J. Clin. Invest. 117, 1814–1823. doi: 10.1172/JCI31080
Sotoodehnia, N., Isaacs, A., de Bakker, P. I. W., Dörr, M., Newton-Cheh, C., Nolte, I. M., et al. (2010). Common variants in 22 loci are associated with QRS duration and cardiac ventricular conduction. Nat. Genet. 42, 1068–1076. doi: 10.1038/ng.716
Speerschneider, T., and Thomsen, M. B. (2013). Physiology and analysis of the electrocardiographic T wave in mice. Acta Physiol. (Oxf). 209, 262–271. doi: 10.1111/apha.12172
Stroud, D. M., Yang, T., Bersell, K., Kryshtal, D. O., Nagao, S., Shaffer, C., et al. (2016). Contrasting Nav1.8 activity in Scn10a-/- ventricular myocytes and the intact heart. J. Am. Heart Assoc. 5:e002946. doi: 10.1161/JAHA.115.002946
Suarez, J., Gloss, B., Belke, D. D., Hu, Y., Scott, B., Dieterle, T., et al. (2004). Doxycycline inducible expression of SERCA2a improves calcium handling and reverts cardiac dysfunction in pressure overload-induced cardiac hypertrophy. Am. J. Physiol. Heart Circ. Physiol. 287, H2164–H2172. doi: 10.1152/ajpheart.00428.2004
Sugiyama, K., Sasano, T., Kurokawa, J., Takahashi, K., Okamura, T., Kato, N., et al. (2016). Oxidative stress induced ventricular arrhythmia and impairment of cardiac function in Nos1ap deleted mice. Int. Heart J. 57, 341–349. doi: 10.1536/ihj.15-471
Syeda, F., Holmes, A. P., Yu, T. Y., Tull, S., Kuhlmann, S. M., Pavlovic, D., et al. (2016). PITX2 modulates atrial membrane potential and the antiarrhythmic effects of sodium-channel blockers. J. Am. Coll. Cardiol. 68, 1881–1894. doi: 10.1016/j.jacc.2016.07.766
Tao, Y., Zhang, M., Li, L., Bai, Y., Zhou, Y., Moon, A. M., et al. (2014). Pitx2, an atrial fibrillation predisposition gene, directly regulates ion transport and intercalated disc genes. Circ. Cardiovasc. Genet. 7, 23–32. doi: 10.1161/CIRCGENETICS.113.000259
Tomás, M., Napolitano, C., De Giuli, L., Bloise, R., Subirana, I., Malovini, A., et al. (2010). Polymorphisms in the NOS1AP gene modulate QT interval duration and risk of arrhythmias in the long QT syndrome. J. Am. Coll. Cardiol. 55, 2745–2752. doi: 10.1016/j.jacc.2009.12.065
Tranum-Jensen, J., Wilde, A. A. M., Vermeulen, J. T., and Janse, M. J. (1991). Morphology of electrophysiologically identified junctions between Purkinje fibers and ventricular muscle in rabbit and pig hearts. Circ. Res. 69, 429–437. doi: 10.1161/01.RES.69.2.429
Tucker, N. R., Dolmatova, E. V., Lin, H., Cooper, R. R., Ye, J., Hucker, W. J., et al. (2017). Diminished PRRX1 expression is associated with increased risk of atrial fibrillation and shortening of the cardiac action potential. Circ. Cardiovasc. Genet. 10:e001902. doi: 10.1161/CIRCGENETICS.117.001902
Tuomi, J. M., Tyml, K., and Jones, D. L. (2011). Atrial tachycardia/fibrillation in the connexin 43 G60S mutant (Oculodentodigital dysplasia) mouse. Am. J. Physiol. Heart Circ. Physiol. 300, H1402–H1411. doi: 10.1152/ajpheart.01094.2010
Unudurthi, S. D., Wolf, R. M., and Hund, T. J. (2014). Role of sinoatrial node architecture in maintaining a balanced source-sink relationship and synchronous cardiac pacemaking. Front. Physiol. 5:446. doi: 10.3389/fphys.2014.00446
van den Boogaard, M., Smemo, S., Burnicka-Turek, O., Arnolds, D. E., van de Werken, H. J. G., Klous, P., et al. (2014). A common genetic variant within SCN10A modulates cardiac SCN5A expression. J. Clin. Invest. 124, 1844–1852. doi: 10.1172/JCI73140
van den Hoogenhof, M. M. G., Beqqali, A., Amin, A. S., van der Made, I., Aufiero, S., Khan, M. A. F., et al. (2018). RBM20 mutations induce an arrhythmogenic dilated cardiomyopathy related to disturbed calcium handling. Circulation 138, 1330–1342. doi: 10.1161/CIRCULATIONAHA.117.031947
van Duijvenboden, K., de Boer, B. A., Capon, N., Ruijter, J. M., and Christoffels, V. M. (2016). EMERGE: a flexible modelling framework to predict genomic regulatory elements from genomic signatures. Nucleic Acids Res. 44:e42. doi: 10.1093/nar/gkv1144
van Eif, V. W. W., Stefanovic, S., van Duijvenboden, K., Bakker, M., Wakker, V., de Gier-de Vries, C., et al. (2019). Transcriptome analysis of mouse and human sinoatrial node cells reveals a conserved genetic program. Development 146:dev173161. doi: 10.1242/dev.173161
van Ouwerkerk, A. F., Bosada, F. M., van Duijvenboden, K., Hill, M. C., Montefiori, L. E., Scholman, K. T., et al. (2019). Identification of atrial fibrillation associated genes and functional non-coding variants. Nat. Commun. 10:4755. doi: 10.1038/s41467-019-12721-5
van Setten, J., Brody, J. A., Jamshidi, Y., Swenson, B. R., Butler, A. M., Campbell, H., et al. (2018). PR interval genome-wide association meta-analysis identifies 50 loci associated with atrial and atrioventricular electrical activity. Nat. Commun. 9:2904. doi: 10.1038/s41467-018-04766-9
van Setten, J., Verweij, N., Mbarek, H., Niemeijer, M. N., Trompet, S., Arking, D. E., et al. (2019). Genome-wide association meta-analysis of 30,000 samples identifies seven novel loci for quantitative ECG traits. Eur. J. Hum. Genet. 27, 952–962. doi: 10.1038/s41431-018-0295-z
Veerman, C. C., Mengarelli, I., Guan, K., Stauske, M., Barc, J., Tan, H. L., et al. (2016). hiPSC-derived cardiomyocytes from Brugada syndrome patients without identified mutations do not exhibit clear cellular electrophysiological abnormalities. Sci. Rep. 6:30967. doi: 10.1038/srep30967
Verheule, S., Wilson, E. E., Arora, R., Engle, S. K., Scott, L. R., and Olgin, J. E. (2002). Tissue structure and connexin expression of canine pulmonary veins. Cardiovasc. Res. 55, 727–738. doi: 10.1016/S0008-6363(02)00490-X
Vikram, A., Lewarchik, C. M., Yoon, J.-Y., Naqvi, A., Kumar, S., Morgan, G. M., et al. (2017). Sirtuin 1 regulates cardiac electrical activity by deacetylating the cardiac sodium channel. Nat. Med. 23, 361–367. doi: 10.1038/nm.4284
Wang, H., Qin, J., Gong, S., Feng, B., Zhang, Y., and Tao, J. (2014). Insulin-like growth factor-1 receptor-mediated inhibition of A-type K(+) current induces sensory neuronal hyperexcitability through the phosphatidylinositol 3-kinase and extracellular signal-regulated kinase 1/2 pathways, independently of Akt. Endocrinology 155, 168–179. doi: 10.1210/en.2013-1559
Wemhöner, K., Friedrich, C., Stallmeyer, B., Coffey, A. J., Grace, A., Zumhagen, S., et al. (2015). Gain-of-function mutations in the calcium channel CACNA1C (Cav1.2) cause non-syndromic long-QT but not Timothy syndrome. J. Mol. Cell. Cardiol. 80, 186–195. doi: 10.1016/j.yjmcc.2015.01.002
West, T. C. (1955). Ultramicroelectrode recording from the cardiac pacemaker. J. Pharmacol. Exp. Ther. 115, 283–290.
Wiener, N., and Rosenblueth, A. (1946). The mathematical formulation of the problem of conduction of impulses in a network of connected excitable elements, specifically in cardiac muscle. Arch. Inst. Cardiol. Mex 16, 205–265.
Xu, B., Wratten, N., Charych, E. I., Buyske, S., Firestein, B. L., and Brzustowicz, L. M. (2005). Increased expression in dorsolateral prefrontal cortex of CAPON in schizophrenia and bipolar disorder. PLoS Med. 2:e263. doi: 10.1371/journal.pmed.0020263
Yagishita, D., Chui, R. W., Yamakawa, K., Rajendran, P. S., Ajijola, O. A., Nakamura, K., et al. (2015). Sympathetic nerve stimulation, not circulating norepinephrine, modulates T-peak to T-end interval by increasing global dispersion of repolarization. Circ. Arrhythm. Electrophysiol. 8, 174–185. doi: 10.1161/CIRCEP.114.002195
Yamaguchi, T., Suzuki, T., Sato, T., Takahashi, A., Watanabe, H., Kadowaki, A., et al. (2018). The CCR4-NOT deadenylase complex controls Atg7-dependent cell death and heart function. Sci. Signal. 11:eaan3638. doi: 10.1126/scisignal.aan3638
Yang, J.-M., Shen, C.-J., Chen, X.-J., Kong, Y., Liu, Y.-S., Li, X.-W., et al. (2019). erbb4 deficits in chandelier cells of the medial prefrontal cortex confer cognitive dysfunctions: implications for schizophrenia. Cereb. Cortex 29, 4334–4346. doi: 10.1093/cercor/bhy316
Yang, K.-C., Breitbart, A., De Lange, W. J., Hofsteen, P., Futakuchi-Tsuchida, A., Xu, J., et al. (2018). Novel adult-onset systolic cardiomyopathy due to MYH7 E848G mutation in patient-derived induced pluripotent stem cells. JACC Basic Transl. Sci. 3, 728–740. doi: 10.1016/j.jacbts.2018.08.008
Yi, F., Ling, T.-Y., Lu, T., Wang, X.-L., Li, J., Claycomb, W. C., et al. (2015). Down-regulation of the small conductance calcium-activated potassium channels in diabetic mouse atria. J. Biol. Chem. 290, 7016–7026. doi: 10.1074/jbc.M114.607952
Zaritsky, J. J., Redell, J. B., Tempel, B. L., and Schwarz, T. L. (2001). The consequences of disrupting cardiac inwardly rectifying K(+) current (I(K1)) as revealed by the targeted deletion of the murine Kir2.1 and Kir2.2 genes. J. Physiol. 533, 697–710. doi: 10.1111/j.1469-7793.2001.t01-1-00697.x
Zhang, S., Kim, K., Rosen, A., Smyth, J. W., Sakuma, R., Delgado-Olguín, P., et al. (2011). Iroquois homeobox gene 3 establishes fast conduction in the cardiac His-Purkinje network. Proc. Natl. Acad. Sci. U.S.A. 108, 13576–13581. doi: 10.1073/pnas.1106911108
Zhang, T., Yong, S. L., Tian, X.-L., and Wang, Q. K. (2007). Cardiac-specific overexpression of SCN5A gene leads to shorter P wave duration and PR interval in transgenic mice. Biochem. Biophys. Res. Commun. 355, 444–450. doi: 10.1016/j.bbrc.2007.01.170
Zhang, X.-D., Timofeyev, V., Li, N., Myers, R. E., Zhang, D.-M., Singapuri, A., et al. (2014). Critical roles of a small conductance Ca2+-activated K+ channel (SK3) in the repolarization process of atrial myocytes. Cardiovasc. Res. 101, 317–325. doi: 10.1093/cvr/cvt262
Zhang, Z., He, Y., Tuteja, D., Xu, D., Timofeyev, V., Zhang, Q., et al. (2005). Functional roles of Cav1.3(alpha1D) calcium channels in atria: insights gained from gene-targeted null mutant mice. Circulation 112, 1936–1944. doi: 10.1161/CIRCULATIONAHA.105.540070
Zheng, Z., Wang, Z.-M., and Delbono, O. (2002). Charge movement and transcription regulation of L-type calcium channel alpha(1S) in skeletal muscle cells. J. Physiol. 540, 397–409. doi: 10.1113/jphysiol.2001.013464
Keywords: GWAS, cardiac electrophysiology, arrhythmias, gene expression, genetics
Citation: Scholman KT, Meijborg VMF, Gálvez-Montón C, Lodder EM and Boukens BJ (2020) From Genome-Wide Association Studies to Cardiac Electrophysiology: Through the Maze of Biological Complexity. Front. Physiol. 11:557. doi: 10.3389/fphys.2020.00557
Received: 26 February 2020; Accepted: 04 May 2020;
Published: 27 May 2020.
Edited by:
Marcel van der Heyden, University Medical Center Utrecht, NetherlandsReviewed by:
Marina Cerrone, New York University School of Medicine, United StatesMorten Salling Olesen, Rigshospitalet, Denmark
Copyright © 2020 Scholman, Meijborg, Gálvez-Montón, Lodder and Boukens. This is an open-access article distributed under the terms of the Creative Commons Attribution License (CC BY). The use, distribution or reproduction in other forums is permitted, provided the original author(s) and the copyright owner(s) are credited and that the original publication in this journal is cited, in accordance with accepted academic practice. No use, distribution or reproduction is permitted which does not comply with these terms.
*Correspondence: Bastiaan J. Boukens, b.j.boukens@amsterdamumc.nl