- Centre for Regenerative Medicine and Stem Cell Research, Aga Khan University, Karachi, Pakistan
Transfusion Medicine is facing mounting challenges, including but not limited to donor availability, blood supply shortages, and transfusion-associated complications, such as immunogenicity and transmission of viral infections. ‘Blood Pharming’, for in vitro Red Blood Cells (RBC) synthesis, offers a potentially effective approach to addressing the challenges and risks associated with the transfusion of blood and related products. This innovative approach employs cells from variable sources such as Hematopoietic stem cells (HSCs), induced pluripotent stem cells (iPSCs), or immortalized progenitor cell lines, directing their differentiation towards erythropoiesis in an in-vitro environment that mimics the normal bone marrow niche required for erythropoiesis. This review article provides a comprehensive analysis of the progress and hurdles in blood pharming, emphasizing in vitro RBC synthesis for clinical application. In-vitro large-scale production of RBCs offers cutting-edge advantages, such as consistent scalability, the capacity to acquire desired blood phenotypes, and a significant reduction in transfusion-related infections, however, substantial molecular and methodological challenges still need to be addressed before the transfer of this approach from bench to bedside. The review discusses the challenges in ensuring scalability that matches demand and supply, the structural and functional integrity of in-vitro synthesized RBCs compared to their in-vivo counterparts, and the cost-effective methods of RBC synthesis in vitro. It also highlights the importance of implementing thorough characterization and testing protocols to comply with regulatory standards. Additionally, it delves into the ethical concerns associated with commercializing such products. In summary, this review examines the progress and obstacles in the field of in-vitro blood pharming. Through a comprehensive analysis of the present state of the discipline, ongoing scholarly investigations, and prospective avenues of inquiry, our objective is to contribute to a more profound comprehension of the potential impact of synthetic RBCs on the transformation of transfusion medicine.
Introduction
Blood transfusion is a cornerstone therapeutic intervention in medical emergencies and for individuals grappling with conditions such as inherited anemias, hemoglobinopathies, myelodysplasias, cancers, and chronic diseases of RBCs. Lifelong transfusion, in some patients, comes with the increased risk of immunization against the donated RBCs, which can lead to severe transfusion-related complications, occasionally culminating in life threatening outcomes (1–4).
The continuous requirement for blood and blood products relies primarily on blood donations by volunteers worldwide. According to the World Health Organization (WHO), approximately 118 million blood donations are made annually worldwide. The increasing gap in the demand and supply of blood and blood products is further predicted to exacerbate and pose a global threat to blood services (5). The essential nature of transfusions is challenged by overwhelming demand for blood, inadequate blood supplies and the potential for adverse reactions, highlighting a significant concern for the global healthcare system (1–4). Numerous efforts are underway to explore alternative approaches for ex-vivo generation and expansion of erythrocytes that may serve as a novel and renewable source of transfusion grade RBCs (6, 7).
In recent years, the field of regenerative medicine has witnessed remarkable strides in the development of alternative approaches to traditional blood transfusions. Among these advancements, the concept of in-vitro RBC production, often referred to as “blood pharming,” has emerged as a promising avenue with profound implications for therapeutic applications. Blood pharming has led to the development of protocols that can yield large numbers of laboratory grown, transfusion grade RBCs termed as “manufactured RBCs” (mRBCs) or cultured RBCs (cRBCs) (4).
The in vitro generation of mRBCs has emerged as a vital area of transfusion research. This review aims to comprehensively explore the progress in the field and persistent challenges encountered in harnessing the potential of mRBCs for therapeutic application. Concentrated research efforts have pivoted towards using multiple cell sources as starting material, including peripheral blood (PB), cord blood (CB), bone marrow (BM), human adult HSCs, erythroblast progenitor cell lines and iPSCs. Amongst these, iPSCs and erythroblast progenitor cell lines have received prominence due to their unparalleled potential for unlimited expansion of mRBCs proposing a viable and scalable alternative to conventional blood transfusions (8). Several protocols are still under investigation to explore the possibilities of generating transfusion grade mRBCs to overcome the hurdles of scarcity of donors, availability of blood for rare blood types and frequency of transfusions.
While we navigate the multifaceted landscape of blood pharming and mRBC synthesis, a breakthrough in the field of transfusion medicine was reported through the “RESTORE (Recovery and Survival of Stem Cell Originated Red Cells) trial”, an initiative by the “NHS Blood and Transplant, University of Bristol, National Institute for Health and Care Research Cambridge Clinical Facility”. The trial was aimed to compare the post transfusion longevity of mRBCs which were developed from donor−derived stem cells compared to the standard RBC transfusion from the same donor (9, 10).
As we delve into the intricacies of in-vitro RBC production, it is essential to address not only the scientific achievements but also the ethical, regulatory, and translational aspects that govern the transition of these technologies from the laboratory to the clinic.
This review aims to provide a holistic understanding of the progress achieved and the challenges that lie ahead in realizing the full potential of in-vitro mRBCs for therapeutic applications. This review aims to navigate the normal physiological process of erythropoiesis, challenging landscape of blood pharming, exploring its scientific underpinnings, current progress, and obstacles still to overcome within the ethical framework and guidelines. For anyone interested in the future of healthcare and biotechnology, understanding blood pharming becomes imperative as we enter a new era of transfusion medicine.
Erythropoiesis
Erythropoiesis is a multifaceted process that entails the migration of cells from the embryonic yolk sac to the fetal liver, and eventually to the BM during the course of embryonic development (11). Within the BM, erythroid progenitor cells undergo multiple stages culminating in the production of reticulocytes, which then enter the bloodstream. This process is under the control of several regulatory factors (12). Erythropoietin (EPO), produced by the kidneys, acts as a stimulatory agent, while hepcidin, produced by the liver, serves to regulate iron mobilization (13, 14). Key transcription factors, notably GATA binding protein 1 (GATA) and Krüppel-like factors (KLF), are integral to the regulation of erythropoiesis (15) (see Figure 1). Any interference with these regulatory elements can cause disturbances in erythropoiesis, potentially leading to an overproduction or deficiency of red cells, or to defects in their morphological functionality (16).
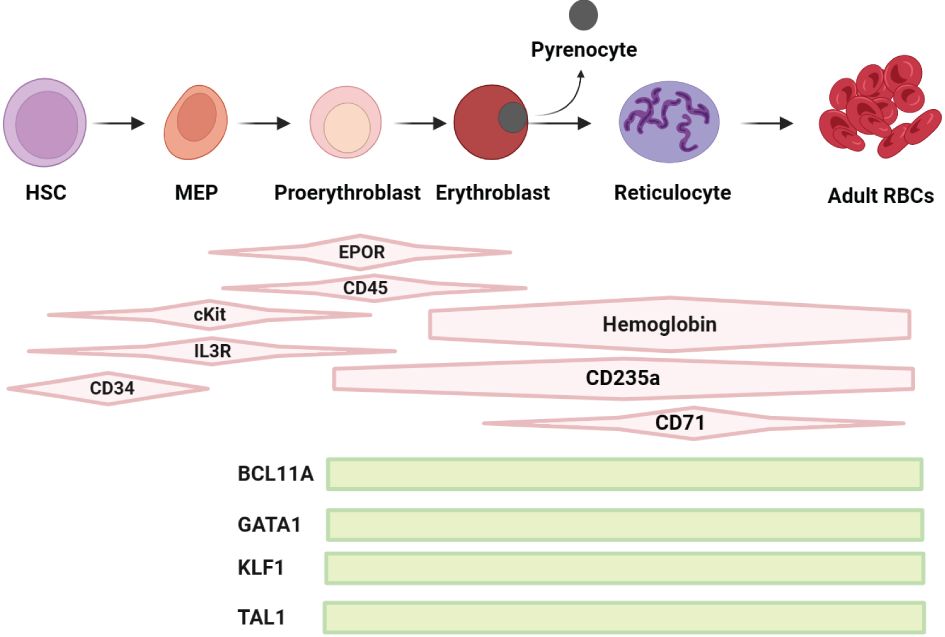
Figure 1 Schematic representation of the regulation of erythropoiesis: Early phase of erythropoiesis starts from HSCs and ends with the proerythroblast stage, the late phase of erythropoiesis starts with proerythroblasts to erythroblasts (E) towards enucleation with generation of reticulocytes and expulsion of pyrencoytes, maturating into RBCs. The differentiation and maturation of these cells are regulated by broadly acting hematopoietic cytokines, including erythropoietin (EPO), stem cell factor (SCF) and interleukin-3 (IL-3) and their receptors (R), the SCF receptor cKIT, EPO receptor EPO-R and IL-3-R, which are required for the biosynthesis of heme and the production of hemoglobin (Hb). Key factors such as BCL11A, GATA1, KLF1 and TAL1 are pivotal in the regulation and development of erythropoiesis. BCL11A plays a crucial role in fetal/adult hemoglobin switch and erythroid progenitor maturation. GATA1 is essential for the survival and differentiation of erythroid lineage cells. KLF1, also known as EKLF, is vital for erythrocyte development and the regulation of several erythroid-specific genes. TAL1, in conjunction with GATA1, contributes to the control of erythroid and megakaryocytic differentiation. These factors collectively orchestrate the complex process of erythropoiesis, ensuring the efficient production of RBCs.
Erythropoiesis takes place within the BM through a series of tightly regulated steps of erythroid progenitor cell differentiation. This process ensures the supply of RBCs, which are essential for oxygen transport. Recent scientific advancements have deepened our understanding of the molecular underpinnings of erythropoiesis, paving the way for novel treatments for related disorders.
Erythropoiesis in embryos
In the embryo, erythropoiesis begins in the yolk sac, transitioning to the liver and spleen, and eventually establishing in the BM post-birth (11, 17, 18). Primarily, yolk sac is the site of primitive progenitor cells forming blood islands to produce embryonic hemoglobin before birth (19). Erythropoiesis then moves to the liver and spleen at around 6–8 weeks of gestation. By the 10–28-week, liver becomes the main site for producing EPO (20, 21). By the second trimester’s end, the BM takes over, producing fetal hemoglobin (HbF), which has a high oxygen affinity, facilitating oxygen transport across the placenta (20).
Fetal hematopoiesis is influenced by factors such as ferritin Iron regulatory protein 1 and 2 (IRP1 and IRP2), which regulates iron transfer from the placenta (22), and Erythropoietin receptor (EpoR), which extends through early erythropoiesis (23). In order to suffice the adaptations to changing oxygen needs after birth, the switch from fetal liver to BM erythropoiesis facilitates the transition of fetal globin to adult globin (11).
Erythropoiesis in bone marrow
BM erythropoiesis involves progenitor cells maturing into RBCs, with about 20 billion new erythrocytes formed daily (24). Cytokines and growth factors like EPO and stem cell factor (SCF) modulate this process, with the marrow’s microenvironment providing additional support (16). Osteoblasts, endothelial cells, and stromal cells within this niche contribute to erythropoiesis regulation through cytokine production and cellular interactions (25).
Erythroblast islands in the marrow, with a central macrophage surrounded by erythroblasts, create a microenvironment essential for erythropoiesis. Macrophages provide support with nutrients, adhesion molecules, and phagocytosis of cellular debris (26).
Erythropoiesis spans over approximately 14 days, beginning with HSCs producing megakaryocytes, Burst Forming Unit Erythroid (BFU-E), and Colony Forming Unit-Erythroid (CFU-E), and progressing through a series of erythroid precursors to reticulocytes (27, 28). This maturation process involves reduced cell size and increased hemoglobin content and expression of surface markers (17). Erythroid progenitors, BFU-E and CFU-E, marked by CD34, CD105, CD36, CD71, CD45RA and glycophorin A (CD235a) are abundant in the umbilical cord (UC), BM, and PB (17, 27, 29). Human erythroid precursors show distinctive surface markers at different stages of maturation.
Driven by the activity of erythropoietin, erythroblasts divide and undergo a series of cell division, which results in transcriptional and morphological changes: i.e., degradation of intracellular organelles, increases hemoglobin content, restructure of cellular membrane and chromatin condensation which ultimately leads to reduce volume and expulsion of nuclei. Enucleation is essential as it transforms erythroblasts into reticulocytes, which then mature into erythrocytes (RBCs). The challenges in replicating this process effectively in vitro, particularly with iPSCs and immortalized cell lines, hinder the large-scale production of functional RBCs for therapeutic purposes (30, 31).
Blood pharming; cell sources for in vitro erythropoiesis
Studies have employed adult and CB derived HSCs and hematopoietic progenitor cells (HSPCs) to generate RBCs in vitro (32–34) (see Figure 2). However, the accessibility of HSCs and/or HSPCs derived from adult and CB is limited, and the production of RBCs from these sources is not sustainable (8).
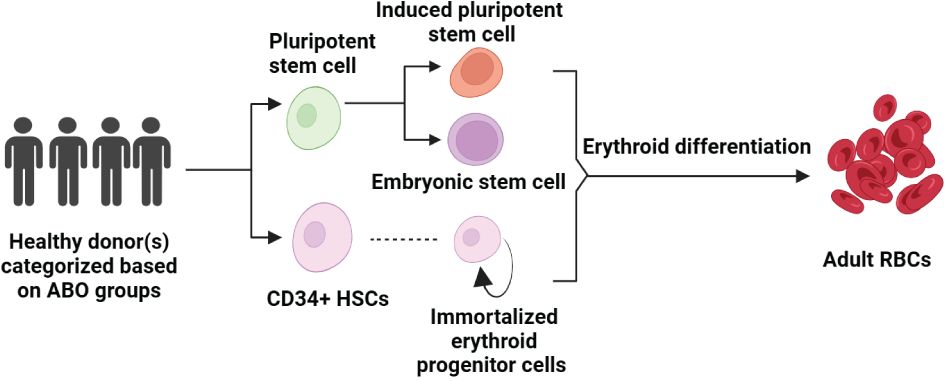
Figure 2 Pathways to Erythropoiesis from Stem Cells: This schematic illustrates the process of generating adult RBCs from various stem cell sources, starting with healthy donors who are selected based on ABO blood groups. Pluripotent stem cells can differentiate into iPSCs or be derived from embryonic stem cells (ESCs), both of which have the capacity to enter the erythroid differentiation pathway. CD34+ HSCs, isolated from these pluripotent sources or directly from donors, can be cultured to produce immortalized erythroid progenitor cells, which further differentiate into mature RBCs. The arrow pathways denote the progression from undifferentiated stem cells to fully differentiated erythroid cells, highlighting the potential for both research and therapeutic applications in erythropoiesis.
Human pluripotent stem cells (hPSCs); including both embryonic stem cells (ESCs) and iPSCs, offer a promising solution by potentially serving as a plentiful source for RBCs owing to their capacity for self-renewal (35–37). The process of differentiating RBCs from hPSCs closely mirrors the key stages of erythropoiesis, in which HSCs and HSPCs are committed to generating cells of the erythroid lineage under a tightly regulated microenvironment through growth factors and molecules that mimic the natural phenomenon of erythropoiesis (8).
Although cells from these varied sources exhibit similarity to human erythrocytes, their therapeutic potential for clinical application is still under investigation.
Using HSC or HPSC as starting material for generation of RBCs
Research endeavors focusing on the generation of mRBCs date back to the late 1980s (38–40). Differentiation protocols, involving CD34+ HSPCs, have been developed and comprehensively employed (8, 41).
A widely adopted approach involves a 3-step method of liquid culture of erythroid cells. In the first step of RBC synthesis, HSCs are expanded using EPO, SCF, and interleukin-3 (IL-3) within a base medium such as Iscove’s modified Dulbecco’s medium (IMDM) or serum-free expansion medium. In the second step, erythroblast expansion is induced in the presence of SCF, EPO, and transferrin. The third step involves subsequent terminal differentiation, either with or without EPO. While SCF and EPO are essential for the expansion of erythroblasts, cells in the terminal differentiation phase gradually lose receptors for these cytokines. Since enucleated reticulocytes retain ferritin receptors, it is recommended to supplement transferrin, the supplier of iron to erythroid cells, until the final maturation phase is completed (42).
Amongst those, expansion and differentiation of stem cells harvested from BM, PB and CB, to erythroid lineage was extensively facilitated through development of a liquid culture system (43, 44). Various protocols used for generating RBCs from CD34+ HSPCs and HSCs harvested from PB, BM and CB have been extended to hPSCs to determine their RBC differentiation potential (11). These methodologies have been employed and optimized using different approaches for step-wise generation of RBCs involving varied combinations of essential molecules (45–48).
A study by Giarratana et al., demonstrated that approximately 90% of CD34+ HSCs and HSPCs harvested from human BM, CB, and PB exhibited characteristics resembling RBCs with the ability of oxygen transport, post differentiation (49).
The significance of microenvironmental factors in the terminal differentiation process was effectively demonstrated by the work of Neildez-Nguyen et al., They developed a culture system utilizing CD34+ HSPCs sources form human CB (50). Although in vitro this culture system led to a remarkable 200,000-fold increase in the number of pure populations of erythroid precursor cells, however, the precursor cells did not undergo terminal differentiation into RBCs until they were transfused to a mouse model, where presence of the microenvironment and niche led to complete maturation yielding functional enucleated RBCs.
The evidence for involvement of the microenvironment was further supported by the work of Fujimi et al., They used CD34+ HSPCs human CB and integrated various approaches to generate RBCs (51). In one of their studies, they utilized human telomerase reverse transcriptase gene (hTERT) stroma (telomerase gene-transduced human stromal cells) of human origin to culture CD34+ HSPCs. Subsequently, a liquid culture system was employed to eliminate the hTERT stroma, followed by co-culturing erythroblasts with macrophages. Compared to previously employed protocols, a higher rate of enucleation was observed using this method.
Miharada et al., introduced a further innovation to conventional protocols through a feeder cell-free method for efficient enucleated RBC production, challenging the established notion that cell-to-extracellular matrix adhesion and cell to cell connections in the niche or erythroblastic islands (EBIs) are the primary determinants of enucleation (52). Their findings confirmed that EBIs alone are sufficient to initiate paracrine factors for efficient enucleation in terminal erythropoiesis.
While this multi-faceted approach has been proven to be efficient for in vitro RBC production from CD34+ HSPCs of diverse origins; however, using HSCs or HPSCs for RBC generation faces certain limitations such as challenge in acquiring sufficient sample with minimal batch to batch variation, availability of quality CD34+ HSPCs, and compromised enucleation efficiency. Addressing these concerns is imperative to establishing a sustainable and reliable source for RBC generation in vitro.
Using PSCs as starting material for generation of RBCs
The advent of hPSCs offers a distinct opportunity to address the existing challenges in terms of the choice of starting material for RBC synthesis in vitro (8, 36). Comprising of ESCs and iPSCs; hPSCs demonstrate the ability to regenerate and transform into the three germ layers including erythroid lineage. A study has shown that both PB and CB-derived iPSCs are reliable sources for the clinical production of RBCs in vitro. However, PB-derived iPSCs may have advantages over CB-derived iPSCs due to the limited availability and large amount of CB required for iPSC production (53).
Studies have shown that upon inducing erythroid differentiation, PSCs undergo an intermediary phase where they express HSCs markers such as CD34 or CD43 (54). Three distinct methodologies are conventionally employed for the differentiation of iPSCs into HSCs: a) a co-culture approach involving BM stromal cells, b) a two-dimensional (2D) paradigm implemented on a monolayer culture platform, and c) a three-dimensional (3D) strategy revolving around the formation of embryonic bodies (EBs) (55).
A study in 2009 validated that co-culturing iPSCs with OP9 cells enhanced their differentiation into CD34 + and CD45 + hematopoietic progenitors, which subsequently gave rise to various types of hematopoietic colonies (55). In the presence of OP9 mouse BM stromal cells as feeder cells, human hiPSCs exhibit the phenotype of CD34-positive and CD45-positive hematopoietic cells.
Tisdale’s group employed a two-step approach where they differentiated hPSCs into human yolk sac-like sacs and subsequently into RBCs using medium containing vascular endothelial growth factor (VEGF), SCF, EPO, thrombopoietin (TPO), Interleukin-3 (IL3), Fms-like tyrosine kinase 3 ligand (FLT3) and Bone Morphogenetic Protein 4 (BMP4) (56, 57).
Differentiating hPSCs into hematopoietic stem cells (HSCs) via feeder cells, monolayer cultures or embryoid body (EB) formation has been a preferred approach by many researchers. In one such approach, human ESCs were expanded in IL3, FLT3 ligand, BMP4, hemin, Insulin- like growth factor 1 (IGF1), insulin and transferrin supplemented serum free media. Followed by subsequent co-culture with murine MS5 feeder cells. The protocol generated hemoglobinized erythroblasts expressing embryonic and fetal globin in 24 days. However, adult globin was not expressed (58). A similar approach was used by Dias et al., who utilized feeder cells with a relatively simpler but different cocktail of factors including colony-stimulating factor 3 (CSF3), TPO, IL6, EPO, SCF and IL3 for differentiation of PSCs into RBCs (59).
Dorn et al., established a protocol where CD34+ HSCs were generated from EBs and were subsequently differentiated into enucleated RBCs using a representative cytokine combination consisting of SCF, IL3, and EPO (60). Notably, these RBCs were enucleated and expressed fetal and embryonic hemoglobin.
The Kim group pursued erythroid lineage differentiation through EB formation with varied factor combinations in two different studies from the same group (61). In the first step, EBs were formed with a cocktail of glycogen synthase kinase 3_ inhibitor VIII (GSK3_ inhibitor VIII), BMP4, VEGF, wingless-related integration site 3A (WNT3A) and activin A. Subsequently, Fibroblast growth factor 1 (FGF1), SCF, and β-oestradiol were added for 24 hours followed by 11 days incubation with a combination of BMP4, VEGF, IGF2, FGF1, TPO, heparin, SCF, β-oestradiol, and IBMX to differentiate Embroyed bodies towards HSC lineage. After 11 days a reduced combo consisting of hydrocortisone, SCF, IL3, and EPO was used which was further reduced to poloxamer 188 to achieve terminal erythropoiesis.
The same group utilized another cocktail comprising of VEGF, FGF2, BMP4, WNT2A and activin A for EB formation (62) followed by another cocktail including SCF, EPO, heparin, poloxamer 188, IL3and human serum for terminal differentiation of HSCs to RBCs.
Cerdan, Rouleau, and Bhatia explored selective promotion of hESC differentiation into early erythroblasts through EB formation by adding VEGF to a combination of BMP4, IL6, EPO, IL3, SCF and FLT3 ligand (63). However, these erythroblasts expressed only embryonic globin.
Another innovative four-step approach was employed by Lu et al., aiming to generate RBCs from hESCs (64). Formation and expansion of hematopoietic cells was aimed in the first two steps by utilizing a cytokine cocktail and serum free medium was supplemented with FLT3 ligand, TPO, FGF2, SCF, BMP4, VEGF and triple protein transduction domain-homeobox B4 (tPTD-HoxB4) fusion protein. Differentiation and enrichment of RBCs was achieved in the third and fourth steps where only SCF and EPO were added to the medium. The protocol yielded adult globin expressing enucleated RBCs. This was the first study demonstrating generation of RBC with normal physiological function where comparable oxygen equilibrium curves were observed in PSC-derived RBCs and adult RBCs.
To address challenges associated with culture-to-culture variability and labor intensity, some protocols have adopted monolayer culture systems without EB formation (65–67).
Using immortalized cell lines as starting material for generation of RBCs
Utilizing immortalized erythroid progenitor cell lines for RBC synthesis is another approach. Notably, Akimov et al., generated a cord blood CD34+ cell line through lentiviral co-transduction of HPV16 E6, E7, and hTERT, extending the cell line’s lifespan without tumorigenic activity (54).
Kurita et al., established Human iPS-TAL1 cell-derived erythroid progenitor (HiDEP) and human umbilical cord blood-derived erythroid progenitor (HUDEP) cell lines derived from human iPS cells and umbilical cord blood CD34+ HSCs, respectively. The lines showed erythroid differentiation and gene expression similar to in vitro -cultured CB erythroid cells (68).
Additionally, Trakarnsanga et al. (69) developed Bristol Erythroid Line - Adult (BEL-A) from BM CD34+ cells transduced with HPV16 E6/E7, resulting in up to 30% enucleated cells after differentiation. The cell line closely resembled normal adult erythropoiesis by expressing surface markers akin to control cells and predominantly synthesizing hemoglobin A (HbA) (69). The differentiated cells lacked nuclei, thereby eliminating the tumorigenic potential of oncogene-mediated immortalization.
More recently, detailed characterization of these cell lines has further shown that they accurately recapitulate their primary cell equivalents, thereby providing a molecular signature for immortalization (70).
Wong et al. (71) used three lentiviral factors SV40 large T antigen (SV40T), hTERT, and HPV16-E6/E7 genes to immortalize CD36+ erythroid progenitor cells to generate immortalized CD36+ erythroblast (CD36E) cell line. HPV16-E6/E7 expression, alone or with hTERT, increased CD36+ EPC proliferation, resulting in elevated hemoglobin-producing cells (27% increase) and a shift from HbA to HbF. However, this transformation led to chromosomal alterations, loss of CD34, and changes in gene expression favoring lymphoid-associated factors over erythroid differentiation markers (71, 72).
Using peripheral blood mononuclear cells as starting material for generation of RBCs
Heshusius et al., utilized peripheral blood mononuclear cells without prior CD34+ isolation (73). They developed a GMP-grade medium and erythroid culture protocol which resulted in over 90% enucleation of RBCs. This method holds great potential for cost-effective large-scale production of RBCs. Notably, the expanded erythroblast cultures differentiated into mature RBCs, and their deformability and oxygen-binding capacity were comparable to in vivo reticulocytes.
Present research endeavors also focus on replicating the BM microenvironment using 3D culture systems that simulate optimum conditions for erythroid differentiation. Severn et al., introduced porous polyurethane scaffolds for PB CD34+ cell inoculation, demonstrating superior HPC egress compared to human bone scaffolds (74). Erythroid progenitor proliferation on polymerized high internal phase emulsion (polyHIPE) scaffolds, mimicking the structural characteristics of human BM, resulted in significantly augmented cell density and erythroid cell production. Lee et al., investigated microporous micro-carriers for erythroid cell culture. Larger diameter carriers facilitated increased cell density and high enucleated RBC yields, whereas smaller pore sizes limited cell interaction, leading to reduced cell viability (75).
Hence, diverse strategies have been employed for generating RBCs from different starting materials, exploring a range of differentiation approaches, factor combinations, and culture systems, however, much remains to be explored to effectively assess their therapeutic potential for clinical application.
Current challenges and future directions
The ultimate objective of generating transfusion grade RBCs poses several key challenges, such as achieving effective terminal differentiation and enucleation, sustainability, increased yield, scalability, and cost efficiency (31, 73). These factors are discussed briefly:
Concerns regarding cell of origin
The initial research on mRBCs primarily focused on human HSCs. Despite demonstrating multiple sources of erythroid precursors capable of terminal differentiation with varying degrees of efficiency, the prevailing methods rely heavily on utilizing primary HSCs extracted from BM, CB or PB. This involves expanding a specific subset of stem cells, initially enriched through CD34+ cell isolation or direct expansion from peripheral blood mononuclear cells, by employing a combination of growth factors.
These processes allow for the generation of a large quantity of proerythroblasts, which can then undergo differentiation to produce enucleated reticulocytes, resembling young RBCs. These artificially produced reticulocytes display similar traits to those derived in vivo, undergo maturation into biconcave erythrocytes post-transfusion, and have shown promising circulatory lifespans in early-stage human clinical trials (31).
Despite success in generating enucleated RBCs, these cells tend to generate fetal hemoglobin (Hb) which has a greater propensity to denature and cause membrane damage in contrast to adult Hb. Moreover, there are limitations to their long-term proliferation and the necessity for repeated transductions in successive cultures from different donor sources (31).
The process of generating RBCs from hPSCs mirrors the key developmental stages of erythropoiesis, involving the commitment of HSCs and/or HSPCs to the erythroid lineage (8). However, achieving efficient differentiation of HSPCs from iPSCs remains a substantial challenge. Equally significant is the establishment of a standardized protocol to guide HSPC differentiation towards erythroid lineages. Despite these advancements, these cells exhibit limited enucleation potential and minimal expression of adult hemoglobin, if any.
Mass scale production of transfusion grade RBCs
The primary challenge in mRBC synthesis lies in achieving a production scale to meet standard therapeutic transfusion doses for adults, necessitating over 2×1012 RBCs per unit (42). However, currently employed culture protocols have the potential to generate 3-50 units of packed RBCs from one cord blood donation. Optimized culture conditions are required to mitigate this challenge, particularly ensuring > 90% enucleation for higher yields of adult hemoglobin-expressing erythrocytes, accurately expressing blood group antigens, such as O Rh-negative, and establishing a universal blood type (72). Furthermore, the primary hurdle in clinical application lies in the efficacy of differentiation protocols. Existing methodologies fall short in generating ample functional RBCs due to ineffective RBC differentiation. Terminal maturation issues, especially sustained β-globin expression and enucleation, hinders the process of mRBC synthesis in vitro. Besides quantity, the focus extends to functional and uniform RBC populations, urging the need for extensive research and identification of robust protocols (8).
The use of 3D culture methods and bioreactors is being explored to overcome the challenge and to improve the manufacturing efficiencies of mRBCs. This approach includes leveraging different cell sources, bioreactors, and 3-dimensional materials. However, there’s a need for further research to optimize these methods for clinical application (42). Heshusius et al., engineered a scalable culture methodology utilizing a G-Rex bioreactor, resulting in the generation of pure erythroid cultures from PBMCs without prior CD34+ isolation with a 3x107-fold increase in erythroblast production within 25 days (73). Remarkably, the cultured RBCs showed a strong functional and morphological resemblance to in vivo RBCs. However, the cost involved in RBCs synthesis through bioreactors makes it unlikely that mRBCs can be produced in clinically required numbers in near future. Significant advances are required in protocols for erythroblast expansion and bioreactor technologies to achieve sufficient production of mRBCs.
Almost all the methods currently employed for RBC synthesis have challenges such as culture heterogeneity and complex-labor intensive protocols. Establishing adaptable protocols which will yield consistent product and functionally relevant RBCs is indispensable for clinical application of this innovative technology. However, further understanding of these techniques is still in process (4).
Cost evaluation
Industrial preparation of mRBC synthesis for clinical application is expensive and complex. A single unit of blood containing approximately 2 X 1012 RBCs costs around US$200-300. However, it is estimated that mRBCs would be more expensive, costing approximately between US$1000-$15,000 for the same number of cells. Several variables contribute to the high production costs of mRBCs. One such significant factor is the choice of starting material. The process of culture, expansion and differentiation to RBCs is undertaken in vitro with the help of certain commercial media components and growth factors that may increase the cost significantly. The cost may also increase significantly because multiple batches and flasks would be required to produce this large number of cells available for transfusion. Additionally, structural, and functional characterization of these mRBCs again involves commercial products and services that will increase the overall production expense of the product, hence increasing the cost of the product (3).
Current research is also emphasized in the development of cost-effective protocols for this process. Several studies are focusing on creating an “economically friendly” protocol, aiming to minimize the reliance on expensive recombinant growth factors, especially erythropoietin (EPO), or exploring alternative strategies to reduce costs. Considering the potential need for large-scale production of RBCs for future clinical applications, the development of protocols that minimize costly resources holds significant importance (55).
Methods of reprograming; integration method/non-integrating method/small molecules
Cellular reprogramming of somatic cells to a pluripotent state requires expression of specific transcription factors (TFs) termed as OSKM factors. The famous OSKM factor combination, consisting of Oct4, Sox2, Klf4, and c-Myc, was demonstrated by Yamanaka’s lab (76). Although varied combination for reprogramming have been identified, OSKMs factors are commonly used for reprogramming. While further research is ongoing to determine the precise mechanisms through which somatic cells transition from their mature state to a pluripotent state (See Supplementary Table 1).
Reprogramming techniques have advanced concurrently our understanding of transcription factors. Initially, viral integrating techniques were used to achieve TF expression, but these methods are not suitable for clinical therapeutics (3).
Advancements in lentiviral vector design have been pursued with the aim of enhancing both safety and efficiency; however, persisting challenges include the risks associated with off-target effects, immune responses, and the potential for random mutagenesis (77–81). Despite improving the methodology, concerns regarding insertional mutagenesis persist, as lentiviral integration may activate oncogenes or silence tumor suppressor genes, fostering clonal expansion and the development of malignancies (81). Ongoing investigations continue to scrutinize the integration profile of lentiviral vectors in efforts to mitigate these risks. Additionally, the phenomenon of off-target effects poses a significant concern, as unintended vector integration into the genome may disrupt gene function or regulatory networks, potentially culminating in cellular dysfunction or neoplastic transformations (80). The introduction of genetically modified cells into the body may incite immune responses, leading to the rejection of transduced cells or systemic immunological reactions, a critical consideration particularly in the context of chronic administration of lentivirally-derived red blood cells for therapeutic purposes (77). Ensuring efficient transduction and directed differentiation of stem cells into fully functional red blood cells presents another obstacle, with inefficiencies potentially compromising the yield and functionality of the resultant cells, thereby impacting therapeutic efficacy (M.-C. 78). Moreover, the ethical and regulatory landscape surrounding the use of genetic modification technologies in human subjects necessitates careful consideration, encompassing informed consent procedures, ethical concerns regarding genetic alterations, and the negotiation of regulatory approvals, all of which are imperative for the advancement of lentiviral red blood cell generation toward clinical applications (79). To avoid aboe mentioned challenges associated with lentiviral ectors researchers have been working on developing non-integrative techniques to express reprogramming factors.
Another approach for immortalizing erythroid cells primarily involves utilizing human papillomavirus (HPV)-based in vitro systems. These lines require stringent assays aimed at detecting any residual nucleated cells in the final product, a necessity for all erythroid cultures from diverse stem cell sources (69, 72) (see Figure 3).
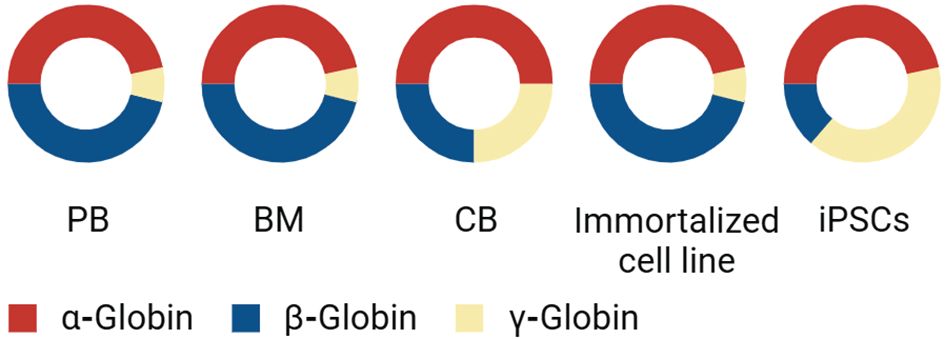
Figure 3 Comparative Analysis of Globin Expression in Different Cell Sources: This diagram presents the proportion of α-globin, β-globin, and γ-globin expression across various cell types. ‘PB’ represents peripheral blood, ‘BM’ stands for bone marrow, and ‘CB’ indicates cord blood, each exhibiting a unique composition of globin chains. In addition to these primary sources, the graph also compares globin expression in immortalized erythroid cell lines and iPSCs. The red segment denotes the presence of α-globin, the blue segment represents β-globin, and the yellow segment indicates γ-globin. This distribution provides insights into the globin expression profiles and the potential use of these cells in therapeutic applications or research studies related to hemoglobinopathies and other blood disorders.
Using red blood substitutes
Red blood cell substitutes are being developed as an alternative to blood transfusions. These substitutes aim to mimic the oxygen-carrying capability of blood and are designed to behave similarly to natural blood in microcirculation. There are two main types of red blood cell substitutes: hemoglobin-based oxygen carriers (HBOCs) and perfluorocarbon-based oxygen carriers (PFCs). However, no oxygen-carrying blood substitutes have been approved for use by the US FDA due to side effects and short half-life. Scientists are actively working to overcome these challenges and develop real blood substitutes, such as red blood cells obtained through differentiation of stem cells (82, 83). Research on artificial blood substitutes has focused on creating alternatives for specific functions of blood, particularly oxygen transport by red blood cells and hemostasis by platelets. Hemoglobin-based substitutes use hemoglobin from various sources, while perfluorocarbon-based substitutes are completely synthetic compounds. These substitutes have shown increased safety levels, do not require blood typing, and do not seem to cause immunosuppression in recipients. Despite the progress, current red cell and platelet substitutes have a short duration of action and can interfere with clinical laboratory testing (83, 84). The development of a universal blood substitute remains elusive despite numerous attempts over the years. While there have been advancements in hemoglobin-based oxygen carriers showing promise in early clinical trials, no FDA-approved products are available yet. Challenges in developing blood substitutes include the need for compatibility testing with donor blood, sterilization methods, and ensuring efficient oxygen transport to tissues.In summary, the quest for effective blood substitutes continues as researchers strive to overcome existing challenges and develop safe alternatives to traditional blood transfusions (85).
Structural and functional integrity of in-vitro synthesized RBCs
Due to the increasing demand for safe alternatives to blood transfusions, there has been significant interest in mRBCs synthesis in vitro. However, before implementing this innovative idea in medical practice, mRBCs need thorough evaluation as they must not only mirror the morphological properties such as enucleated cells with biconcave shape switching hemoglobin and a particular membrane morphology of natural RBCs but also mimic the biological functions of their natural counterparts including immunological response, exchange of gases, oxygen-carrying capacity, and lifetime of mRBCs.
The biconcave structure provides sufficient area for gaseous exchange and provides flexibility to the cells to pass through narrow blood capillaries (86). Since extensive research is focused on generating functional mRBCs, we have a limited number of studies where the ultimate objective has been achieved. Efforts are still underway to completely understand the molecular details of the underlying process and to achieve it in vitro.
Enucleation is a key characteristic feature indicating terminal differentiation of mature RBCs. However, mRBC synthesis is largely hampered by the low enucleation efficiency of cultures in vitro and the maximum enucleation efficiency as reported by research groups around the world has been 42% (8). Studies of the molecular details to understand the enucleation process are at their early stages.
Stable expression of β-globin is amongst the biggest challenges in generating transfusion-grade mRBCs. During the process of terminal differentiation of enucleated RBC, embryonic and fetal globin are naturally repressed, and expression of β-globin is kickstarted in adult RBCs. Although extensive efforts have been made to overcome the challenge of β-globin switch in mRBCs, the field is still an area of active investigation (8).
The oxygen-carrying capacity, longevity in circulation, and immunogenicity are key structural and functional characteristics that mRBCs must possess to be deemed appropriate for transfusion as RBCs. Although significant efforts have been made in the area, it is still a subject of ongoing research.
Ethical concerns associated with the commercialization of RBC products
Blood pharming offers a promising avenue not only for substituting donor blood products but also for potentially augmenting the therapeutic efficacy of these blood cells through genetic modification. However, universally recognized ethical concerns regarding the utilization of human stem cells (hSC), coupled with subsequent adjustments in legislative regulations, significantly restrict the application of ESCs in research. Similarly, generating pluripotent stem cell lines from oocytes and embryos triggers the controversy regarding the definition of human personhood (87).
Conversely, ethical considerations surrounding ESC procurement have impeded research progress, steering attention toward alternative resources. iPSCs, introduced by Shinya Yamanaka and Kazutoshi Takahashi, offer an ethically uncomplicated alternative resembling ESC properties. iPSCs have become a primary focus in developing efficient and scalable protocols for blood cell production as they possess similar characteristics to ESCs with minimal or conditional ethical concerns. Successful reports have demonstrated the derivation of RBCs from iPSCs, showcasing terminal maturation and enucleation in vitro (8, 59, 88). However, stringent regulations are required for bedside applications of iPSCs. These regulations include ethical, legal, and social considerations of cellular therapy including but not limited to (i) extensive characterization of clinical-grade cells for the absence of potential of acquiring secondary disease-causing mutations, (ii) adherence to GMP grade manufacturing applications, (iii) genetic manipulations of cells (89).
Umbilical cord blood shows great promise to produce RBCs in vitro. Cord blood cells, typically discarded after birth, offer a readily available source. With proper consent, utilizing umbilical cord blood avoids complicating critical or ethical concerns, presenting a valuable resource for RBC production (90).
Clinical trials
The “RESTORE (Recovery and Survival of Stem Cell Originated Red Cells) trial” is a pioneering effort in transfusion medicine by introducing a novel approach involving the infusion of laboratory-cultivated RBCs into recipients requiring blood transfusions. It is a randomized control trial by the “NHS Blood and Transplant, University of Bristol, National Institute for Health and Care Research Cambridge Clinical Facility,” in collaboration with other universities (10).The ultimate goal of the trial is to be able to produce rare blood types. The trial involves transferring laboratory-grown RBCs into an individual requiring blood transfusion (9).
Donor derived stem cells were differentiated to RBCs followed by transfer of these cells to volunteers. The methodology of trial is based upon a culture system established by Cogan et al. and Griffith et al. where mature reticulocytes were produced in 20 days from CD34+ adult PB derived cells (91, 92). The methodology delineated in the trial manuscript lacks explicit clarity; however, pertinent protocol details are inferred through referenced sources suggesting that a three-stage method of Douay and Giarratana was used to culture the cells. IMDM containing AB Serum, fatal bovine serum, Insulin, heparin and Transferrin based media was used for culture. The media was supplemented with SCF, IL-3 and EPO in the first stage (days 0-8). During the second stage (days 8-11) SCF, EPO and iron saturated transferrin were supplemented followed by the final stage (days 11-20) where cells were incubated with media containing EPO and 800μg/ml iron saturated transferrin. After 20 days, mature reticulocytes and erythrocytes were isolated and cultured without the use of feeder layers where up to 90% enucleation efficiency was seen. The cells contain normal glycosylation profile and expressed adult hemoglobin with comparable capacity to oxygen,
The life span of this mini blood transfusion (10 ml) during the trials will be compared to the RBC injection with an equal quantity of regular RBCs obtained from the same donor. The volunteers received two mini transfusions with a gap of 4 months. The study is still ongoing. Volunteer’s blood sample will be collected after six months of the first injection of lab grown RBCs and will be tested for the presence of tracer labeled laboratory synthesized RBCs. The trial holds high expectations in the scientific community as it offers several advantages: it will offer an opportunity to synthesize blood for rare blood types, hence lifting the requirement of blood donors for every patient. It may reduce the frequencies of blood transfusions. Although this one trial may not answer all our questions, it can be a breakthrough scientific innovation and will facilitate future endeavors in the field.
Conclusion
This comprehensive review endeavors to delve into the current advancements and persistent challenges within the domain of ex-vivo synthesis of functional and clinically viable mRBCs. The pursuit of artificial substitutes for RBCs commenced nearly half a century ago, yet attaining a successful outcome has remained elusive, with no immediate substitute yet. This protracted quest underscores the intricate nature of in vitro RBC culture, underscoring the complexities inherent in replicating blood tissue and the existing technological constraints.
The identification of HSCs, iPSCs, immortalized erythroid cells and ESCs offered a significant breakthrough to varied areas in regenerative medicine approach including mRBC synthesis. Research initiatives have diversified into various cell sources as discussed in this review. Particularly noteworthy are iPSCs and erythroblast progenitor cells, which have gained prominence due to their unparalleled capacity for unlimited expansion, representing a transformative stride toward a viable and scalable alternative to traditional blood transfusions. As we navigate the complexities of in vitro RBC production, this review amalgamates insights from diverse studies, encapsulating advancements in stem cell biology, bioprocessing techniques, and biomaterial engineering. These collective efforts contribute to the intricate process of generating RBCs outside the human body.
Ex vivo expansion of erythrocytes is a potential approach for RBC synthesis for the last few years. The review by Migliaccio et al. provides an in-depth analysis of the current landscape surrounding the ex vivo expansion of red blood cells (RBCs) and its potential for clinical transfusion applications in humans. (93). The authors extensively explore the methodologies employed in RBC expansion, emphasizing the critical role of identifying proper sources of HSCs and optimizing culture conditions to support efficient erythropoiesis. They discuss the diverse strategies utilized for stimulating HSC differentiation into mature RBCs, including the use of growth factors, cytokines, and various culture systems. The potential clinical implications of ex vivo expanded RBCs are extremely relevant in scenarios where conventional blood transfusions are not feasible, such as in patients who refuse allogeneic transfusions due to religious beliefs, as in the case of Jehovah’s Witnesses. However, the ex vivo expansion of RBCs still faces significant challenges in transfusion medicine such as the concerns related to the safety, scalability, and cost-effectiveness of the expansion process. The application of this technique needs continued research efforts aimed at addressing these challenges and optimizing ex vivo RBC expansion technologies for practical clinical use.
Ongoing basic research is constantly setting the groundwork for transitioning scientific information to industrial-scale production, thereby facilitating clinical trials with an objective to establish an industrial technology for the manufacturing of mRBCs, analogous to the mass production of pharmaceuticals, hence “blood pharming”. This necessitates the establishment of a prototype for large-scale production under pharmaceutical-grade conditions, not only for conducting clinical trials but also to lay the foundation for broader applications of this transformative technology. Each milestone achieved on this road has heightened interest and research in this field, expanding the horizons of possibilities for stem cell technology and its practical applications within the ethical framework.
This perspective underscores a pivotal challenge in regenerative medicine developing large-scale bioengineering solutions for clinical trials to substantiate the efficacy of novel approaches. The aspiration is that such advancements, including the mass production of cultured RBCs, will soon become a reality.
Author contributions
HH: Writing – original draft, Writing – review & editing, Conceptualization. SR: Conceptualization, Writing – original draft, Writing – review & editing.
Funding
The author(s) declare financial support was received for the research, authorship, and/or publication of this article. The project was funded by Centre for Regenerative Medicine and Stem Cell Research at the Aga Khan University (AKU) and philanthropic contributors.
Conflict of interest
The authors declare that the research was conducted in the absence of any commercial or financial relationships that could be construed as a potential conflict of interest.
Publisher’s note
All claims expressed in this article are solely those of the authors and do not necessarily represent those of their affiliated organizations, or those of the publisher, the editors and the reviewers. Any product that may be evaluated in this article, or claim that may be made by its manufacturer, is not guaranteed or endorsed by the publisher.
Supplementary material
The Supplementary Material for this article can be found online at: https://www.frontiersin.org/articles/10.3389/frhem.2024.1373408/full#supplementary-material
References
1. Adams RJ, McKie VC, Hsu L, Files B, Vichinsky E, Pegelow C, et al. Prevention of a first stroke by transfusions in children with sickle cell anemia and abnormal results on transcranial Doppler ultrasonography. N Engl J Med. (1998) 339:5–11. doi: 10.1056/nejm199807023390102
2. Lucarelli G, Gaziev J. Advances in the allogeneic transplantation for thalassemia. Blood Rev. (2008) 22:53–63. doi: 10.1016/j.blre.2007.10.001
3. Lim ZR, Vassilev S, Leong YW, Hang JW, Rénia L, Malleret B, et al. Industrially compatible transfusable iPSC-derived RBCs: progress, challenges and prospective solutions. Int J Mol Sci. (2021) 22:9808. doi: 10.3390/ijms22189808
4. Pellegrin S, Severn CE, Toye AM. Towards manufactured red blood cells for the treatment of inherited anemia. Haematologica. (2021) 106:2304. doi: 10.3324/haematol.2020.268847
6. Migliaccio AR, Palis J. Blood in a dish: In vitro synthesis of red blood cells. Drug Discovery Today Dis Mech. (2011) 8:e3–8. doi: 10.1016/j.ddmec.2011.10.002
7. Sivalingam J, SuE Y, Lim ZR, Lam ATL, Lee AP, Lim HL, et al. A scalable suspension platform for generating high-density cultures of universal red blood cells from human induced pluripotent stem cells. Stem Cell Rep. (2021) 16:182–97. doi: 10.1016/j.stemcr.2020.11.008
8. Lee SJ, Jung C, Oh JE, Kim S, Lee S, Lee JY, et al. Generation of red blood cells from human pluripotent stem cells-an update. Cells. (2023) 12. doi: 10.3390/cells12111554
9. Kale SSS, Kode R, Kuchana SK, Kutikuppala LVS. Breakthrough in the scientific world: Lab-grown red blood cells used in transfusions. Asian J Transfus Sci. (2023) 17:143–4. doi: 10.4103/ajts.ajts_148_22
10. Kutikuppala LVS, Ponnaganti SVK, Kale SSS, Kode R, Kuchana SK. Transfusions with laboratory-grown red blood cells: a new development in science. Exp Hematol. (2023) 119-120:1–2. doi: 10.1016/j.exphem.2023.01.004
11. Dzierzak E, Philipsen S. Erythropoiesis: development and differentiation. Cold Spring Harb Perspect Med. (2013) 3:a011601. doi: 10.1101/cshperspect.a011601
12. Stevens-Hernandez CJ, Flatt JF, Kupzig S, Bruce LJ. Reticulocyte maturation and variant red blood cells. Front Physiol. (2022) 13:834463. doi: 10.3389/fphys.2022.834463
13. Collins JF, Wessling-Resnick M, Knutson MD. Hepcidin regulation of iron transport. J Nutr. (2008) 138:2284–8. doi: 10.3945/jn.108.096347
15. Siatecka M, Bieker JJ. The multifunctional role of EKLF/KLF1 during erythropoiesis. Blood. (2011) 118:2044–54. doi: 10.1182/blood-2011-03-331371
16. Zivot A, Lipton JM, Narla A, Blanc L. Erythropoiesis: insights into pathophysiology and treatments in 2017. Mol Med. (2018) 24:11. doi: 10.1186/s10020-018-0011-z
17. Nandakumar SK, Ulirsch JC, Sankaran VG. Advances in understanding erythropoiesis: evolving perspectives. Br J Haematol. (2016) 173:206–18. doi: 10.1111/bjh.13938
18. Yamane T. Cellular basis of embryonic hematopoiesis and its implications in prenatal Erythropoiesis. Int J Mol Sci. (2020) 21. doi: 10.3390/ijms21249346
19. Popescu DM, Botting RA, Stephenson E, Green K, Webb S, Jardine L, et al. Decoding human fetal liver haematopoiesis. Nature. (2019) 574:365–71. doi: 10.1038/s41586-019-1652-y
20. Baron MH, Vacaru A, Nieves J. Erythroid development in the mammalian embryo. Blood Cells Mol Dis. (2013) 51:213–9. doi: 10.1016/j.bcmd.2013.07.006
21. Palis J. Interaction of the macrophage and primitive erythroid lineages in the mammalian embryo. (Amsterdam Netherlands: Elsevier Science) Front Immunol. (2016) 7:669. doi: 10.3389/fimmu.2016.00669
22. Chakraborty S, Andrieux G, Kastl P, Adlung L, Altamura S, Boehm ME, et al. Erythropoietin-driven dynamic proteome adaptations during erythropoiesis prevent iron overload in the developing embryo. Cell Rep. (2022) 40:111360. doi: 10.1016/j.celrep.2022.111360
23. Hidalgo D, Bejder J, Pop R, Gellatly K, Hwang Y, Maxwell Scalf S, et al. EpoR stimulates rapid cycling and larger red cells during mouse and human erythropoiesis. Nat Commun. (2021) 12:7334. doi: 10.1038/s41467-021-27562-4
24. Iolascon A, Andolfo I, Russo R. Congenital dyserythropoietic anemias. Blood. (2020) 136:1274–83. doi: 10.1182/blood.2019000948
25. Morrison SJ, Scadden DT. The bone marrow niche for haematopoietic stem cells. Nature. (2014) 505:327–34. doi: 10.1038/nature12984
26. Klei TRL, Meinderts SM, van den Berg TK, van Bruggen R. From the cradle to the grave: the role of macrophages in erythropoiesis and erythrophagocytosis. Front Immunol. (2017) 8:73. doi: 10.3389/fimmu.2017.00073
27. Le Goff S, Boussaid I, Floquet C, Raimbault A, Hatin I, Andrieu-Soler C, et al. p53 activation during ribosome biogenesis regulates normal erythroid differentiation. Blood. (2021) 137:89–102. doi: 10.1182/blood.2019003439
28. Caulier AL, Sankaran VG. Molecular and cellular mechanisms that regulate human erythropoiesis. Blood. (2022) 139:2450–9. doi: 10.1182/blood.2021011044
29. Yan H, Ali A, Blanc L, Narla A, Lane JM, Gao E, et al. Comprehensive phenotyping of erythropoiesis in human bone marrow: Evaluation of normal and ineffective erythropoiesis. Am J Hematol. (2021) 96:1064–76. doi: 10.1002/ajh.26247
30. Palis J. Primitive and definitive erythropoiesis in mammals. Front Physiol. (2014) 5:3. doi: 10.3389/fphys.2014.00003
31. Satchwell TJ. Generation of red blood cells from stem cells: Achievements, opportunities and perspectives for malaria research. Front Cell Infect Microbiol. (2022) 12:1039520. doi: 10.3389/fcimb.2022.1039520
32. Douay L, Andreu G. Ex vivo production of human red blood cells from hematopoietic stem cells: what is the future in transfusion? Transfus Med Rev. (2007) 21:91–100. doi: 10.1016/j.tmrv.2006.11.004
33. Migliaccio AR, Whitsett C, Migliaccio G. Erythroid cells in vitro: from developmental biology to blood transfusion products. Curr Opin Hematol. (2009) 16:259–68. doi: 10.1097/MOH.0b013e32832bcaa2
34. Vinjamur DS, Bauer DE. Growing and genetically manipulating human umbilical cord blood-derived erythroid progenitor (HUDEP) cell lines. Methods Mol Biol 1698. (2018), 275–84. doi: 10.1007/978-1-4939-7428-3_17
35. Thomson JA, Itskovitz-Eldor J, Shapiro SS, Waknitz MA, Swiergiel JJ, Marshall VS, et al. Embryonic stem cell lines derived from human blastocysts. Science. (1998) 282:1145–7. doi: 10.1126/science.282.5391.1145
36. Takahashi K, Tanabe K, Ohnuki M, Narita M, Ichisaka T, Tomoda K, et al. Induction of pluripotent stem cells from adult human fibroblasts by defined factors. Cell. (2007) 131:861–72. doi: 10.1016/j.cell.2007.11.019
37. Yu J, Vodyanik MA, Smuga-Otto K, Antosiewicz-Bourget J, Frane JL, Tian S, et al. Induced pluripotent stem cell lines derived from human somatic cells. Science. (2007) 318:1917–20. doi: 10.1126/science.1151526
38. Fibach E, Manor D, Oppenheim A, Rachmilewitz EA. Proliferation and maturation of human erythroid progenitors in liquid culture. Blood. (1989) 73:100–3. doi: 10.1182/blood.V73.1.100.100
39. Wada H, Suda T, Miura Y, Kajii E, Ikemoto S, Yawata Y. Expression of major blood group antigens on human erythroid cells in a two phase liquid culture system. Blood. (1990) 75:505–11. doi: 10.1182/blood.V75.2.505.505
40. Fibach E, Rachmilewitz EA. A two-step liquid culture–a novel culture procedure for studying erythroid cell development. Haematologia (Budap). (1991) 24:211–20.
41. Singh VK, Saini A, Tsuji K, Sharma PB, Chandra R. Manufacturing blood ex vivo: a futuristic approach to deal with the supply and safety concerns. Front Cell Dev Biol. (2014) 2:26. doi: 10.3389/fcell.2014.00026
42. Kweon S, Kim S, Baek EJ. Current status of red blood cell manufacturing in 3D culture and bioreactors. Blood Res. (2023) 58:S46–51. doi: 10.5045/br.2023.2023008
43. Wright DE, Wagers AJ, Gulati AP, Johnson FL, Weissman IL. Physiological migration of hematopoietic stem and progenitor cells. Science. (2001) 294:1933–6. doi: 10.1126/science.1064081
44. Massberg S, Schaerli P, Knezevic-Maramica I, Köllnberger M, Tubo N, Moseman EA, et al. Immunosurveillance by hematopoietic progenitor cells trafficking through blood, lymph, and peripheral tissues. Cell. (2007) 131:994–1008. doi: 10.1016/j.cell.2007.09.047
45. Leary A, Ikebuchi K, Hirai Y, Wong G, Yang Y, Clark S, et al. Synergism between interleukin-6 and interleukin-3 in supporting proliferation of human hematopoietic stem cells: comparison with interleukin-1 alpha. Blood. (1988) 71:1759–63. doi: 10.1182/blood.V71.6.1759.1759
46. Kieran MW, Perkins AC, Orkin SH, Zon LI. Thrombopoietin rescues in vitro erythroid colony formation from mouse embryos lacking the erythropoietin receptor. Proc Natl Acad Sci U.S.A. (1996) 93:9126–31. doi: 10.1073/pnas.93.17.9126
47. Wu H, Klingmüller U, Acurio A, Hsiao JG, Lodish HF. Functional interaction of erythropoietin and stem cell factor receptors is essential for erythroid colony formation. Proc Natl Acad Sci U.S.A. (1997) 94:1806–10. doi: 10.1073/pnas.94.5.1806
48. Lyman SD, Jacobsen SEW. c-kit ligand and Flt3 ligand: stem/progenitor cell factors with overlapping yet distinct activities. Blood. (1998) 91:1101–34. doi: 10.1182/blood.V91.4.1101
49. Giarratana MC, Kobari L, Lapillonne H, Chalmers D, Kiger L, Cynober T, et al. Ex vivo generation of fully mature human red blood cells from hematopoietic stem cells. Nat Biotechnol. (2005) 23:69–74. doi: 10.1038/nbt1047
50. Neildez-Nguyen TMA, Wajcman H, Marden MC, Bensidhoum M, Moncollin V, Giarratana M-C, et al. Human erythroid cells produced ex vivo at large scale differentiate into red blood cells. vivo. Nat Biotechnol. (2002) 20:467–72. doi: 10.1038/nbt0502-467
51. Fujimi A, Matsunaga T, Kobune M, Kawano Y, Nagaya T, Tanaka I, et al. Ex vivo large-scale generation of human red blood cells from cord blood CD34+ cells by co-culturing with macrophages. Int J Hematol. (2008) 87:339–50. doi: 10.1007/s12185-008-0062-y
52. Miharada K, Hiroyama T, Sudo K, Nagasawa T, Nakamura Y. Efficient enucleation of erythroblasts differentiated in vitro from hematopoietic stem and progenitor cells. Nat Biotechnol. (2006) 24:1255–6. doi: 10.1038/nbt1245
53. Cho YK, Kim HK, Kwon SS, Jeon SH, Cheong JW, Nam KT, et al. In vitro erythrocyte production using human-induced pluripotent stem cells: determining the best hematopoietic stem cell sources. Stem Cell Res Ther. (2023) 14:106. doi: 10.1186/s13287-023-03305-8
54. Akimov SS, Ramezani A, Hawley TS, Hawley RG. Bypass of senescence, immortalization, and transformation of human hematopoietic progenitor cells. Stem Cells. (2005) 23:1423–33. doi: 10.1634/stemcells.2005-0390
55. Han H, Rim YA, Ju JH. Recent updates of stem cell-based erythropoiesis. Hum Cell. (2023) 36:894–907. doi: 10.1007/s13577-023-00872-z
56. Uchida N, Haro-Mora JJ, Fujita A, Lee DY, Winkler T, Hsieh MM, et al. Efficient generation of β-globin-expressing erythroid cells using stromal cell-derived induced pluripotent stem cells from patients with sickle cell disease. Stem Cells. (2017) 35:586–96. doi: 10.1002/stem.2517
57. Haro-Mora JJ, Uchida N, Demirci S, Wang Q, Zou J, Tisdale JF. Biallelic correction of sickle cell disease-derived induced pluripotent stem cells (iPSCs) confirmed at the protein level through serum-free iPS-sac/erythroid differentiation. Stem Cells Transl Med. (2020) 9:590–602. doi: 10.1002/sctm.19-0216
58. Olivier EN, Qiu C, Velho M, Hirsch RE, Bouhassira EE. Large-scale production of embryonic red blood cells from human embryonic stem cells. Exp Hematol. (2006) 34:1635–42. doi: 10.1016/j.exphem.2006.07.003
59. Dias J, Gumenyuk M, Kang H, Vodyanik M, Yu J, Thomson JA, et al. Generation of red blood cells from human induced pluripotent stem cells. Stem Cells Dev. (2011) 20:1639–47. doi: 10.1089/scd.2011.0078
60. Isabel D, Katharina K, Marcos JA-B, Martina R, Simeon S, Foued G, et al. Erythroid differentiation of human induced pluripotent stem cells is independent of donor cell type of origin. Haematologica. (2015) 100:32–41. doi: 10.3324/haematol.2014.108068
61. Park YJ, Jeon S-H, Kim H-K, Suh EJ, Choi SJ, Kim S, et al. Human induced pluripotent stem cell line banking for the production of rare blood type erythrocytes. J Trans Med. (2020) 18:236. doi: 10.1186/s12967-020-02403-y
62. Roh J, Kim S, Cheong J-W, Jeon S-H, Kim H-K, Kim MJ, et al. Erythroid differentiation of induced pluripotent stem cells co-cultured with OP9 cells for diagnostic purposes. Ann Lab Med. (2022) 42:457–66. doi: 10.3343/alm.2022.42.4.457
63. Cerdan C, Rouleau A, Bhatia M. VEGF-A165 augments erythropoietic development from human embryonic stem cells. Blood. (2004) 103:2504–12. doi: 10.1182/blood-2003-07-2563
64. Lu SJ, Feng Q, Park JS, Vida L, Lee BS, Strausbauch M, et al. Biologic properties and enucleation of red blood cells from human embryonic stem cells. Blood. (2008) 112:4475–84. doi: 10.1182/blood-2008-05-157198
65. Smith BW, Rozelle SS, Leung A, Ubellacker J, Parks A, Nah SK, et al. The aryl hydrocarbon receptor directs hematopoietic progenitor cell expansion and differentiation. Blood. (2013) 122:376–85. doi: 10.1182/blood-2012-11-466722
66. Olivier EN, Zhang S, Yan Z, Suzuka S, Roberts K, Wang K, et al. PSC-RED and MNC-RED: Albumin-free and low-transferrin robust erythroid differentiation protocols to produce human enucleated red blood cells. Exp hematology. (2019) 75:31–52.e15. doi: 10.1016/j.exphem.2019.05.006
67. Netsrithong R, Suwanpitak S, Boonkaew B, Trakarnsanga K, Chang LJ, Tipgomut C, et al. Multilineage differentiation potential of hematoendothelial progenitors derived from human induced pluripotent stem cells. Stem Cell Res Ther. (2020) 11:481. doi: 10.1186/s13287-020-01997-w
68. Kurita R, Suda N, Sudo K, Miharada K, Hiroyama T, Miyoshi H, et al. Establishment of immortalized human erythroid progenitor cell lines able to produce enucleated red blood cells. PloS One. (2013) 8:e59890. doi: 10.1371/journal.pone.0059890
69. Trakarnsanga K, Griffiths RE, Wilson MC, Blair A, Satchwell TJ, Meinders M, et al. An immortalized adult human erythroid line facilitates sustainable and scalable generation of functional red cells. Nat Commun. (2017) 8:14750. doi: 10.1038/ncomms14750
70. Daniels DE, Ferguson DCJ, Griffiths RE, Trakarnsanga K, Cogan N, MacInnes KA, et al. Reproducible immortalization of erythroblasts from multiple stem cell sources provides approach for sustainable RBC therapeutics. Mol Ther Methods Clin Dev. (2021) 22:26–39. doi: 10.1016/j.omtm.2021.06.002
71. Wong S, Keyvanfar K, Wan Z, Kajigaya S, Young NS, Zhi N. Establishment of an erythroid cell line from primary CD36+ erythroid progenitor cells. Exp Hematol. (2010) 38:994–1005.e1001-1002. doi: 10.1016/j.exphem.2010.07.012
72. Cervellera CF, Mazziotta C, Di Mauro G, Iaquinta MR, Mazzoni E, Torreggiani E, et al. Immortalized erythroid cells as a novel frontier for in vitro blood production: current approaches and potential clinical application. Stem Cell Res Ther. (2023) 14:139. doi: 10.1186/s13287-023-03367-8
73. Heshusius S, Heideveld E, Burger P, Thiel-Valkhof M, Sellink E, Varga E, et al. Large-scale in vitro production of red blood cells from human peripheral blood mononuclear cells. Blood Adv. (2019) 3:3337–50. doi: 10.1182/bloodadvances.2019000689
74. Severn CE, Macedo H, Eagle MJ, Rooney P, Mantalaris A, Toye AM. Polyurethane scaffolds seeded with CD34(+) cells maintain early stem cells whilst also facilitating prolonged egress of haematopoietic progenitors. Sci Rep. (2016) 6:32149. doi: 10.1038/srep32149
75. Lee E, Han SY, Choi HS, Chun B, Hwang B, Baek EJ. Red blood cell generation by three-dimensional aggregate cultivation of late erythroblasts. Tissue Eng Part A. (2015) 21:817–28. doi: 10.1089/ten.TEA.2014.0325
76. Yamanaka S. Induction of pluripotent stem cells from mouse fibroblasts by four transcription factors. Cell Prolif. (2008) 41 Suppl 1:51–6. doi: 10.1111/j.1365-2184.2008.00493.x
77. Modlich U, Navarro S, Zychlinski D, Maetzig T, Knoess S, Brugman MH, et al. Insertional transformation of hematopoietic cells by self-inactivating lentiviral and gammaretroviral vectors. Mol Ther. (2009) 17:1919–28. doi: 10.1038/mt.2009.179
78. Giarratana M-C, Rouard H, Dumont A, Kiger L, Safeukui I, Le Pennec P-Y, et al. Proof of principle for transfusion of in vitro–generated red blood cells. Blood J Am Soc Hematol. (2011) 118:5071–9. doi: 10.1182/blood-2011-06-362038
79. Hill R, Moran M, Cook T, Jones R, Sharkey N, Sturgis P, et al. Emerging biotechnologies: technology, choice and the public good. (2012). doi: 10.13140/2.1.1031.8086
80. Biffi A, Montini E, Lorioli L, Cesani M, Fumagalli F, Plati T, et al. Lentiviral hematopoietic stem cell gene therapy benefits metachromatic leukodystrophy. Science. (2013) 341:1233158. doi: 10.1126/science.1233158
81. Kvaratskhelia M, Sharma A, Larue RC, Serrao E, Engelman A. Molecular mechanisms of retroviral integration site selection. Nucleic Acids Res. (2014) 42:10209–25. doi: 10.1093/nar/gku769
82. Moradi S, Jahanian-Najafabadi A, Roudkenar MH. Artificial blood substitutes: first steps on the long route to clinical utility. Clin Med Insights: Blood Disord. (2016) 9:S38461. doi: 10.4137/CMBD.S38461
83. Lanza R, Langer R, Vacanti JP, Atala A. Principles of tissue engineering. Amsterdam Netherlands: Academic press (2020).
84. Hohenhaus AE. Chapter 24 - Blood Transfusion and Blood Substitutes. In DiBartola SP (Ed.) Fluid, Electrolyte, and Acid-Base Disorders in Small Animal Practice (Fourth Edition) (2012) 585-604. Saint Louis: W.B. Saunders.
85. Khan F, Singh K, Friedman MT. Artificial blood: the history and current perspectives of blood substitutes. Discoveries. (2020) 8. doi: 10.15190/d.2020.1
86. Diez-Silva M, Dao M, Han J, Lim CT, Suresh S. Shape and Biomechanical Characteristics of Human Red Blood Cells in Health and Disease MRS Bull. (2010) 35(5):382–388. doi: 10.1557/mrs2010.571
87. Lo B, Parham L. Ethical issues in stem cell research. Endocr Rev. (2009) 30:204–13. doi: 10.1210/er.2008-0031
88. Focosi D, Amabile G. Induced pluripotent stem cell-derived red blood cells and platelet concentrates: from bench to bedside. Cells. (2017) 7. doi: 10.3390/cells7010002
89. Moradi S, Mahdizadeh H, Šarić T, Kim J, Harati J, Shahsavarani H, et al. Research and therapy with induced pluripotent stem cells (iPSCs): social, legal, and ethical considerations. Stem Cell Res Ther. (2019) 10:341. doi: 10.1186/s13287-019-1455-y
90. Kim HO. In-vitro stem cell derived red blood cells for transfusion: are we there yet? Yonsei Med J. (2014) 55:304–9. doi: 10.3349/ymj.2014.55.2.304
91. Cogan N, Kupzig S, Parsons SF, Anstee DJ. A robust ex vivo culture system for production of mature human reticulocytes from a single donor. Am Soc Hematol. (2011) 118. doi: 10.1182/blood.V118.21.2096.2096
92. Griffiths RE, Kupzig S, Cogan N, Mankelow TJ, Betin VM, Trakarnsanga K, et al. Maturing reticulocytes internalize plasma membrane in glycophorin A–containing vesicles that fuse with autophagosomes before exocytosis. Blood J Am Soc Hematol. (2012) 119:6296–306. doi: 10.1182/blood-2011-09-376475
Keywords: erythropoiesis, in-vitro RBCs, hematopoietic stem cells, transfusion medicine, blood supply shortages, scalability
Citation: Hassan H and Rajput S (2024) Blood pharming: exploring the progress and hurdles in producing in-vitro red blood cells for therapeutic applications. Front. Hematol. 3:1373408. doi: 10.3389/frhem.2024.1373408
Received: 19 January 2024; Accepted: 05 March 2024;
Published: 28 March 2024.
Edited by:
Kristbjorn Orri Gudmundsson, National Cancer Institute at Frederick (NIH), United StatesReviewed by:
Sophie D. Lefevre, Université Paris Cité, FranceElspeth Payne, University College London, United Kingdom
Copyright © 2024 Hassan and Rajput. This is an open-access article distributed under the terms of the Creative Commons Attribution License (CC BY). The use, distribution or reproduction in other forums is permitted, provided the original author(s) and the copyright owner(s) are credited and that the original publication in this journal is cited, in accordance with accepted academic practice. No use, distribution or reproduction is permitted which does not comply with these terms.
*Correspondence: Hammad Hassan, hammad.hassan@aku.edu; Sheerien Rajput, sheerien.rajput@aku.edu
†These authors have contributed equally to this work