- 1Biosystems & Integrative Sciences Institute, Faculty of Sciences, University of Lisbon, Lisbon, Portugal
- 2Laboratory of Pulmonary Investigation, Carlos Chagas Filho Biophysics Institute, Federal University of Rio de Janeiro, Rio de Janeiro, Brazil
- 3National Institute of Science and Technology for Regenerative Medicine, Rio de Janeiro, Brazil
- 4Rio de Janeiro Innovation Network in Nanosystems for Health-NanoSAÚDE/FAPERJ, Rio de Janeiro, Brazil
- 5COVID-19 Virus Network, Ministry of Science, Technology and Innovation, Brasília, Brazil
- 6COVID-19 Virus Network, Brazilian Council for Scientific and Technological Development, Brasília, Brazil
- 7COVID-19 Virus Network, Fundação de Amparo à Pesquisa do Estado do Rio de Janeiro – FAPERJ, Rio de Janeiro, Brazil
- 8Anesthesia and Intensive Care, San Martino Policlinico Hospital, IRCCS for Oncology and Neuroscience, Genoa, Italy
- 9Department of Surgical Sciences and Integrated Diagnostic, University of Genoa, Genoa, Italy
- 10Laboratory of Cellular and Molecular Physiology, Carlos Chagas Filho Biophysics Institute, Federal University of Rio de Janeiro, Rio de Janeiro, Brazil
- 11Laboratory of Biochemistry and Cell Signaling, Carlos Chagas Filho Institute of Biophysics, Federal University of Rio de Janeiro, Rio de Janeiro, Brazil
Severe acute respiratory disease coronavirus 2 (SARS-CoV-2, formerly 2019-nCoV) is a novel coronavirus that has rapidly disseminated worldwide, causing the coronavirus disease 2019 (COVID-19) pandemic. As of January 6th, 2021, there were over 86 million global confirmed cases, and the disease has claimed over 1.87 million lives (a ∼2.2% case fatality rate). SARS-CoV-2 is able to infect human cells by binding its spike (S) protein to angiotensin-conversing enzyme 2 (ACE2), which is expressed abundantly in several cell types and tissues. ACE2 has extensive biological activities as a component of the renin-angiotensin-aldosterone system (RAAS) and plays a pivotal role as counter-regulator of angiotensin II (Ang II) activity by converting the latter to Ang (1-7). Virion binding to ACE2 for host cell entry leads to internalization of both via endocytosis, as well as activation of ADAM17/TACE, resulting in downregulation of ACE2 and loss of its protective actions in the lungs and other organs. Although COVID-19 was initially described as a purely respiratory disease, it is now known that infected individuals can rapidly progress to a multiple organ dysfunction syndrome. In fact, all human structures that express ACE2 are susceptible to SARS-CoV-2 infection and/or to the downstream effects of reduced ACE2 levels, namely systemic inflammation and injury. In this review, we aim to summarize the major features of SARS-CoV-2 biology and the current understanding of COVID-19 pathogenesis, as well as its clinical repercussions in the lung, heart, kidney, bowel, liver, and brain. We also highlight potential therapeutic targets and current global efforts to identify safe and effective therapies against this life-threatening condition.
Introduction
In late 2019, a cluster of infections by a novel coronavirus – the severe acute respiratory syndrome coronavirus 2 (SARS-CoV-2, formerly 2019-nCoV) – was epidemiologically linked to a large seafood market in Wuhan, China (Wang C. et al., 2020; Zhou P. et al., 2020). This outbreak has rapidly disseminated domestically and internationally, becoming a global pandemic1. SARS-CoV-2 has demonstrated to be much more infectious than SARS-CoV and Middle East Respiratory Syndrome coronavirus (MERS-CoV), which were responsible for two previous large-scale outbreaks (Table 1). As of January 6th, 2020, there were over 86 million global confirmed cases of coronavirus disease 2019 (COVID-19) and over 1.87 million fatalities2 3.
Human coronaviruses usually attach to cell-surface ectopeptidases, such as dipeptidyl peptidase 4 (DPP4), aminopeptidase N, and angiotensin-conversing enzyme 2 (ACE2), for host cell entry (Fehr and Perlman, 2015; Cui et al., 2019). Both SARS-CoV and SARS-CoV-2 were found to use ACE2, which has extensive biological activities (Santos et al., 2018), as their key cell entry receptor (Hoffmann et al., 2020a; Walls et al., 2020; Zhou P. et al., 2020). The distribution of ACE2 in the host organism appears to be an important factor associated with organ injury (Figure 1). There is also evidence that individual variation in expression and/or polymorphisms in ACE2 may influence susceptibility to SARS-CoV-2 infection and COVID-19 phenotype (Calcagnile et al., 2020; Cao et al., 2020a; Chen J. et al., 2020; Devaux et al., 2020; Hou et al., 2020; Hussain et al., 2020; Leung et al., 2020).
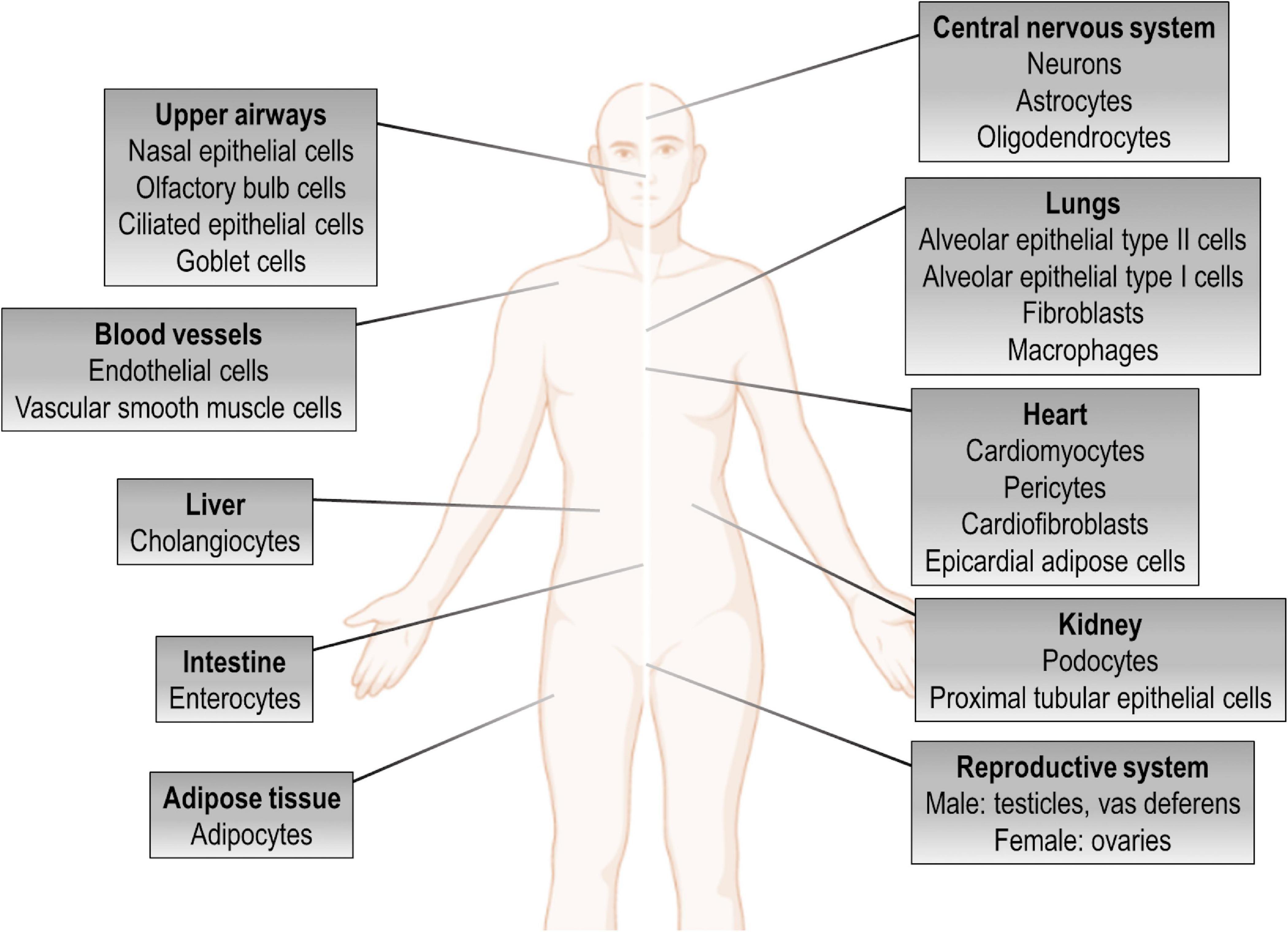
Figure 1. ACE2 expression in the human body. Tissue distribution of angiotensin-converting enzyme 2 (ACE2) expression and potential targets for direct cytotoxicity induced by SARS-CoV-2 infection.
The clinical spectrum of COVID-19 is very heterogeneous (Williamson et al., 2020). Many individuals infected with SARS-CoV-2 are asymptomatic or develop a mild illness with non-specific symptoms, such as fever, fatigue, dry cough, and headache (Huang C. et al., 2020; Sakurai et al., 2020; Wang C. et al., 2020). However, ∼20% of individuals require hospitalization, and ∼25% of these (∼5% of all cases) experience a rapid progression of their symptoms to severe pneumonia/acute respiratory distress syndrome (ARDS), requiring invasive mechanical ventilation (Guan et al., 2020; Huang C. et al., 2020; Wang D. et al., 2020). Individuals with a more severe phenotype usually have a high viral load and long virus-shedding period (He et al., 2020; Liu et al., 2020b). Some risk factors for the development of severe COVID-19 and poor prognosis include advanced age and presence of certain comorbidities, such as chronic obstructive pulmonary disease, coronary heart disease, diabetes mellitus, and hypertension (Guan et al., 2020; Leung et al., 2020; Wu and McGoogan, 2020; Williamson et al., 2020; Zhou F. et al., 2020), although the latter remains controversial (Iaccarino et al., 2020). Multivariable parameters such as higher sequential organ failure assessment score and D-dimer >1 μg/mL on hospital admission have also been associated with higher risk of fatality (Chen N. et al., 2020; Guan et al., 2020; Tang et al., 2020; Zhou F. et al., 2020).
Although SARS-CoV-2 infection was initially described as causing severe respiratory disease, it is now known that infected individuals can rapidly progress to a multiple organ dysfunction syndrome (MODS); therefore, multi-target therapeutic approaches are warranted, and a wide range of distinct therapeutic protocols have been investigated (Gupta et al., 2020; Li H. et al., 2020b; Robba et al., 2020a, b; Zhang et al., 2020b). In this review, we summarize major features of SARS-CoV-2 biology and the current understanding of COVID-19 pathogenesis, as well as its clinical repercussions in the lung, heart, kidney, bowel, liver, and brain. We also shed light on potential therapeutic targets and the current global efforts to identify effective therapies against this devastating condition.
Structure of SARS-CoV-2 and Host Cell Infection
The SARS-CoV-2 Genome
Coronaviruses are named for the crown-shaped spikes on their outer surface. The novel coronavirus (SARS-CoV-2) is an enveloped, 29.9 kb-long, positive-sense, single-stranded RNA virus belonging to the β-coronavirus genus (Figure 2A). The open-reading frames (ORFs) 1a and 1b represent ∼70% of the complete viral genome and possess several conserved non-structural protein sequences (Chan et al., 2020; Kim et al., 2020; Wu A. et al., 2020). A frameshift between these ORFs encodes two polypeptides (1a and 1ab) that are processed by viral proteases to produce non-structural proteins, which are involved in viral replication and suppression of host innate immune defenses (Chan et al., 2020; Wu A. et al., 2020). Among all known coronavirus sequences, SARS-CoV-2 shares the highest genetic similarity with the bat coronavirus RATG13 (∼96%) and the Malayan pangolin coronavirus (∼91%), although it also has considerable genetic similarity with the human coronaviruses SARS-CoV (∼79%) and MERS-CoV (∼50%) (Andersen et al., 2020; Coronaviridae Study Group of the International Committee on Taxonomy of Viruses, 2020; Lam et al., 2020; Zhang T. et al., 2020).
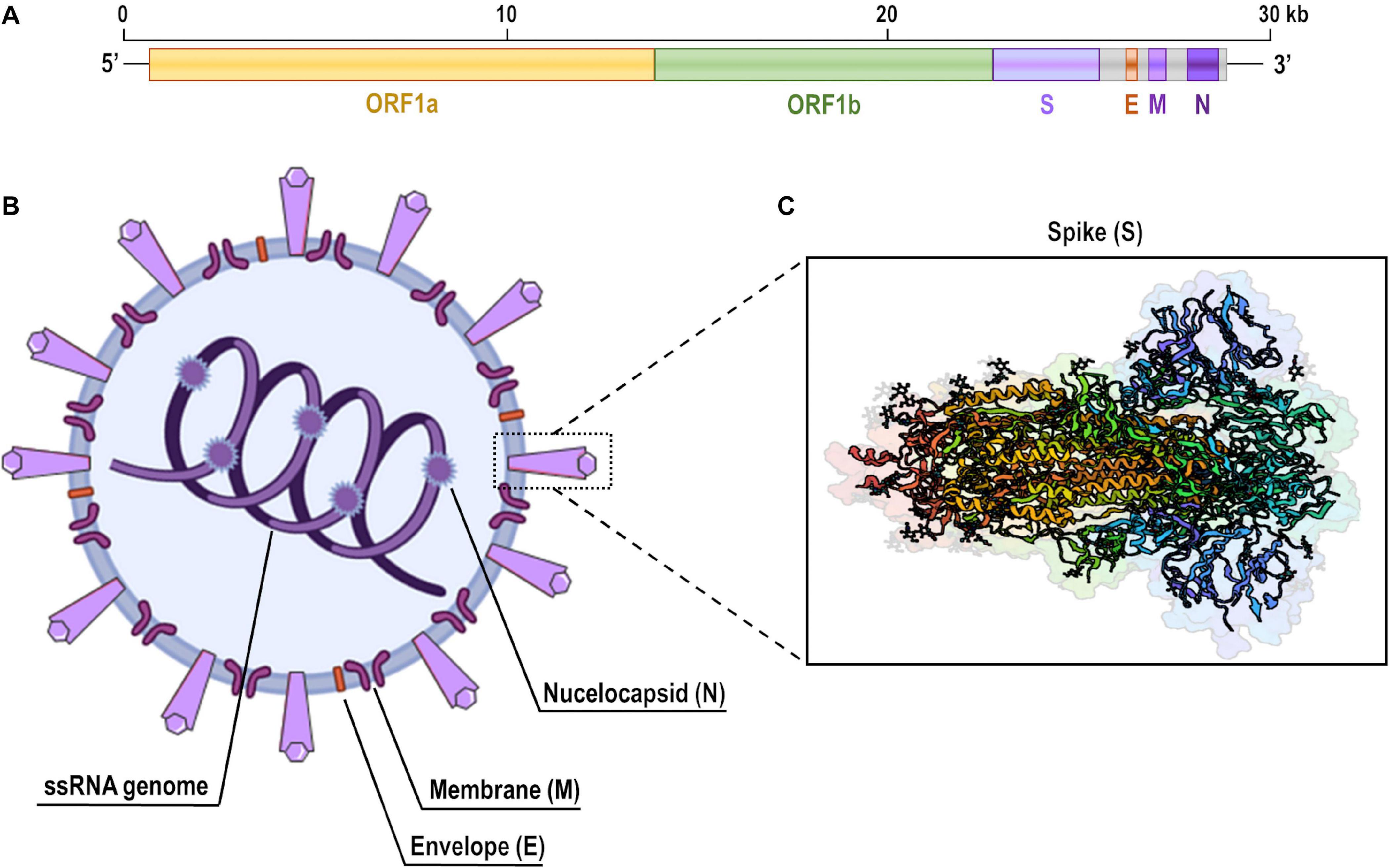
Figure 2. Genome and structure of SARS-CoV-2. (A) Full-length single-strand (ss)RNA genome of SARS-CoV-2 demonstrating open-reading frames (ORFs) 1a and 1b, which encode non-structural proteins, and the position of genes that encode main structural proteins: spike (S), envelope (E), membrane (M), and nucleocapsid (N). (B) Schematic representation of a SARS-CoV-2 viral particle. (C) Ribbon diagram of the open conformation of SARS-CoV-2 spike protein. This structure has been deposited on the Protein Data Bank under accession number 6VYB (SARS-CoV-2 spike ectodomain structure).
The SARS-CoV-2 Structure
The structure of SARS-CoV-2 is composed of four main structural proteins: the spike, envelope, and membrane glycoproteins, and the nucleocapsid protein (Figure 2B). The nucleocapsid is a phosphorylated protein which consists of the structure that directly binds to viral RNA and plays multiple critical roles during the viral life cycle. The envelope glycoprotein is a small, integral membrane structure involved in the maturation and pathogenesis of coronaviruses. The membrane glycoprotein is the most abundant component of the virus structure and plays a central role in viral assembly by interconnecting with all main structural proteins of the viral particle. This protein also delineates the shape of the viral envelope (Fehr and Perlman, 2015; Cui et al., 2019).
The spike glycoprotein is a transmembrane structure present in the outer surface of the viral particle (Figure 2C). It has two subunits (S1 and S2) that are cleaved by the host cell proteases. Interestingly, the furin protease-like cleavage spot is present in SARS-CoV-2, MERS-CoV, and human coronavirus OC43, but absent in SARS-CoV (Andersen et al., 2020; Coutard et al., 2020; Wrapp et al., 2020). The S1 subunit consists of an N-terminal domain and a receptor-binding domain (RBD), which determine host range and cellular tropism of the virus. This subunit is released during the fusion process, thus inducing a conformational change in the S2 subunit, which is the viral membrane-anchored fraction and consists of a hydrophobic fusion peptide and two heptad repeated domains (Cui et al., 2019; Wu A. et al., 2020).
The Host Cell Receptor
ACE2 is a zinc-dependent carboxypeptidase and component of the renin-angiotensin-aldosterone system (RAAS), a complex network that plays a pivotal role in maintaining fluid and electrolyte homeostasis, thus affecting function of multiple organs (Figure 3). Briefly, the protease renin cleaves angiotensinogen to angiotensin I (Ang I), which is subsequently cleaved to Ang II by ACE. Ang II can bind to angiotensin type 1 receptors (AT1-R) and induce vasoconstriction as well as pro-inflammatory, oxidant, and fibrotic responses (Forrester et al., 2018; Danser et al., 2020). On the other hand, ACE2 can convert Ang II to Ang (1-7), which binds to the Mas receptor and counteracts the aforementioned actions mediated by Ang II/AT1-R. ACE2 can also convert Ang I to Ang (1-9), which is subsequently cleaved to Ang (1-7) by ACE. However, the former pathway is more common due to higher affinity between ACE and Ang I (Santos et al., 2018; Gheblawi et al., 2020).
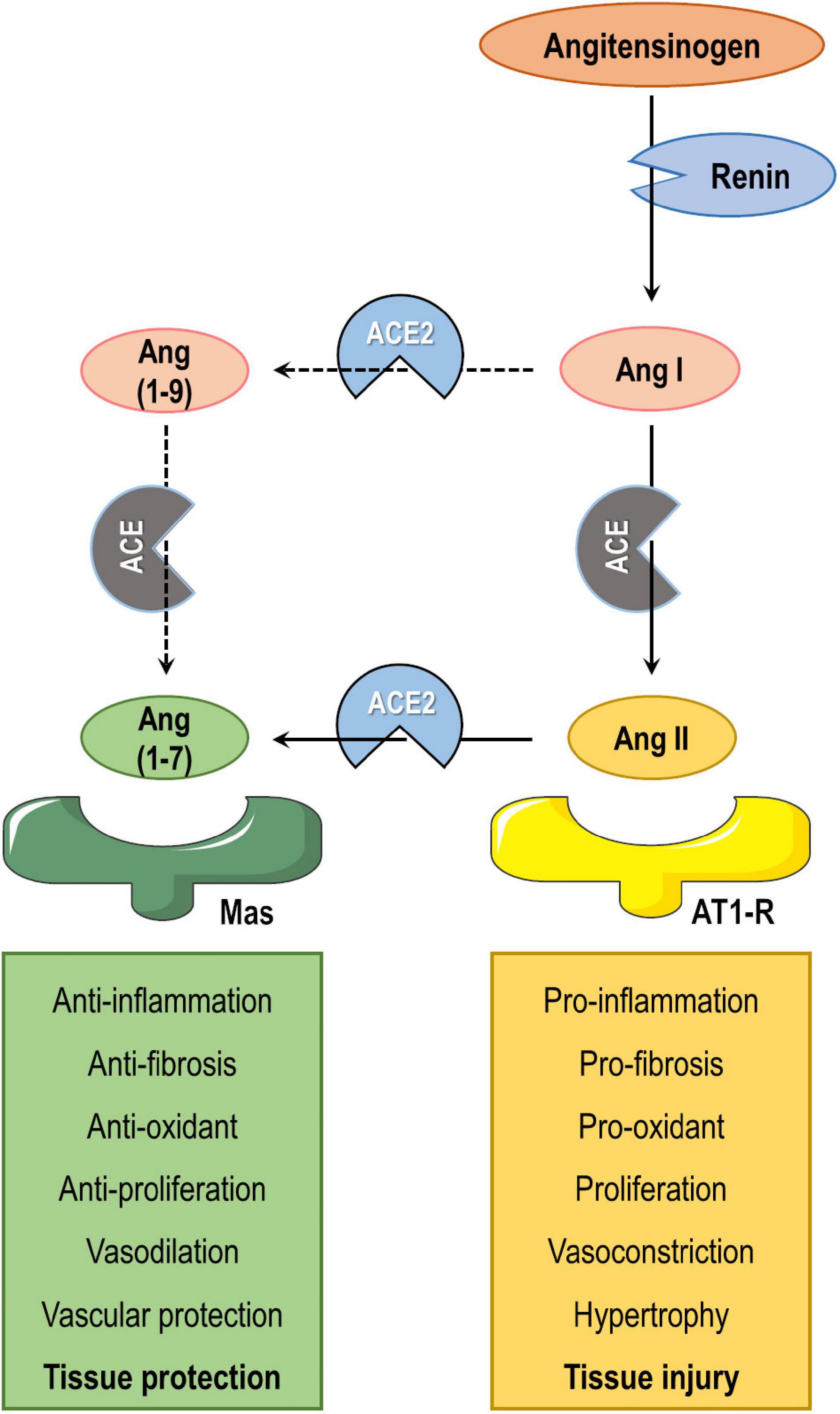
Figure 3. Simplified diagram of the renin-angiotensin-aldosterone system. Angiotensinogen is cleaved by renin to angiotensin I (Ang I). Ang I is then converted by angiotensin-converting enzyme (ACE) to Ang II, which interacts with the AT1 receptor (AT1-R), or is cleaved by ACE2 to Ang (1-7), which interacts with Mas receptor. The ACE2/Ang (1-7)/Mas axis exerts counter-regulatory effects to the ACE/Ang II/AT1-R axis in multiple organs. ACE2 can also convert Ang I to Ang (1-9), which is then cleaved to Ang (1-7) by ACE. However, ACE2 converts Ang II to Ang (1-7) with higher efficiency than it converts Ang I to Ang (1-9).
SARS-CoV-2 Infection and Replication
SARS-CoV-2 RBD attaches to ACE2 for host cell entry (Figure 4), a proteolytic process that is facilitated by TMPRSS2 priming and involves cathepsin L (Hoffmann et al., 2020a; Ou et al., 2020; Wrapp et al., 2020; Zhou P. et al., 2020). This interaction then triggers endocytosis of ACE2 together with the SARS-CoV-2 virion and fusion of the viral membrane and host cell. Interestingly, SARS-CoV-2 not only reduces surface tissue expression of ACE2, but also inhibits its messenger RNA expression after infection (Kuba et al., 2005; Walls et al., 2020). Virus-host receptor interactions also activate ADAM17/TACE, a disintegrin and metalloprotease component, which leads to cleavage and shedding of ACE2 from the cell surface and local production of hyaluronan (Haga et al., 2010; Xu et al., 2017). Concomitantly, the viral spike protein is exposed to endosomal cysteine proteases that lead to its cleavage at two different sites: the first removes the S1 subunit, while the second occurs within the S2 subunit and results in exposure of the fusion peptide (Wan et al., 2020; Walls et al., 2020). Neutrophil elastase was recently found to exert an important role in SARS-CoV-2 infection, as it possesses a cleavage site near the S1-S2 subunits (Bhattacharyya et al., 2020). The viral package is released into the host cytoplasm, where it usurps the cellular machinery to produce new viral particles. As SARS-CoV-2 is a single-stranded RNA virus, its own genetic material serves as messenger RNA, thus driving the synthesis of viral proteins by host cell ribosomes. The uncoated viral RNA encodes the 1a and 1ab polypeptides, which are processed into non-structural proteins. These form a complex with viral genomic RNA to continuously synthesize subgenomic products that encode structural proteins (Jiang et al., 2020). TMPRSS2 also appears to participate in the SARS-CoV-2 replication process, although the mechanisms are unclear (Matsuyama et al., 2020). In fact, protein-protein mapping has identified 332 high-confidence SARS-CoV-2/human protein-protein interactions involved with the virus life cycle (Gordon D.E. et al., 2020). Once all viral components are produced and assembled in the endoplasmic reticulum–Golgi intermediate compartment (ERGIC), the new viruses are released via exocytosis into the extracellular compartment (Jiang et al., 2020).
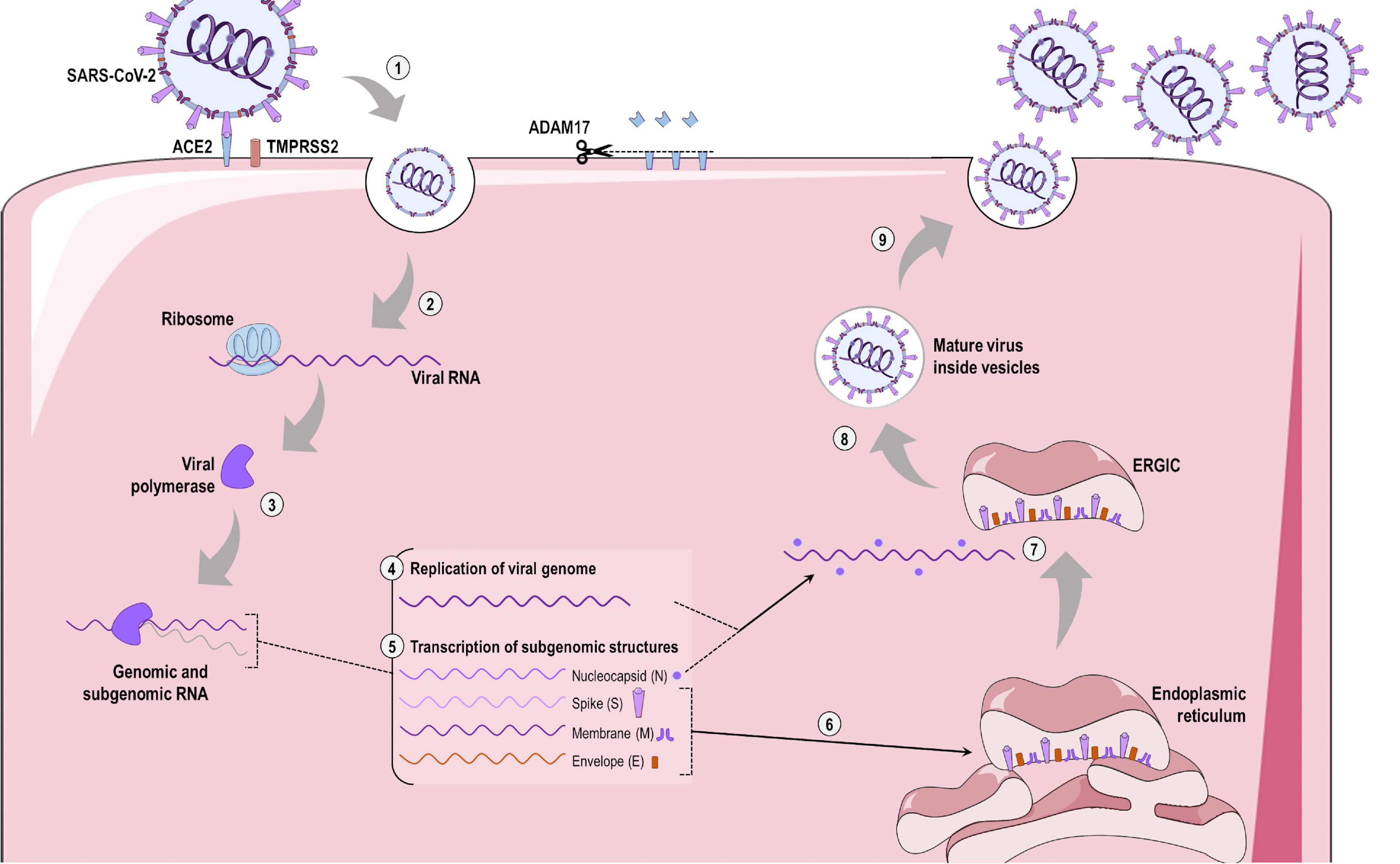
Figure 4. SARS-CoV-2 infection and replication. (1) SARS-CoV-2 spike protein is primed by the type II transmembrane serine protease (TMPRSS2) and interacts with the angiotensin-converting enzyme 2 (ACE2) expressed on the surface of host cells, allowing binding and entry of the virus via clathrin-dependent endocytosis. Concomitantly, ADAM17 is activated and leads to cleavage of ACE2 in its soluble form. (2) The SARS-CoV-2 virion uses host ribosomes to produce viral RNA-dependent RNA polymerase (3). This viral polymerase then initiates replication of the SARS-CoV-2 genome (4) and transcription of subgenomic structures (5). The spike, membrane, and envelope subgenomic transcripts use the endoplasmic reticulum for translation of these viral structural proteins (6), while the viral genome joins the nucleocapsid. (7) All viral structures are exported to the endoplasmic-reticulum-Golgi intermediated compartment (ERGIC), where new viral particles are assembled. (8) After the formation of mature virions, these are exported inside Golgi vesicles to the extracellular compartment via exocytosis. (9) These new viruses are now able to infect adjacent ACE2-expressing cells or enter the bloodstream and infect other tissues.
Pathogenesis and Multiple Organ Injury in COVID-19
Many features of virus-host interactions involving SARS-CoV-2 remain unknown, but several of these have been demonstrated to recapitulate the infection process of other human coronaviruses. COVID-19 development and progression consist of five major pathological mechanisms (Figure 5): (1) direct virus-induced cytotoxicity in ACE2-expressing cells; (2) dysregulation of the RAAS as a result of virus-mediated ACE2 downregulation; (3) dysregulation of immune responses; (4) endothelial cell injury and thrombo-inflammation; and (5) tissue fibrosis (Gupta et al., 2020).
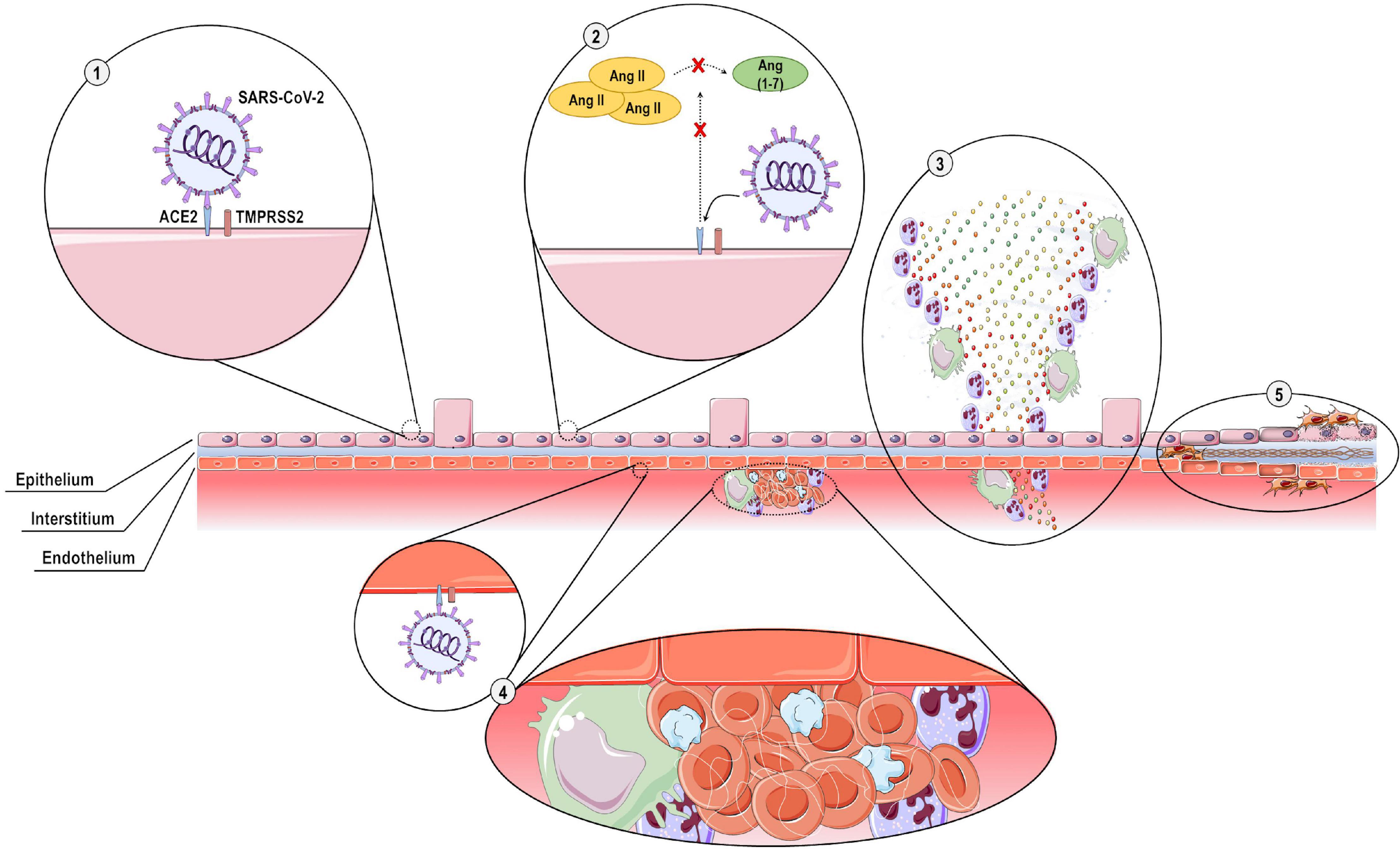
Figure 5. Pathogenesis of COVID-19. Five main pathological mechanisms are implicated in COVID-19 development and progression: (1) SARS-CoV-2 spike protein is primed by the type II transmembrane serine protease (TMPRSS2) and interacts with angiotensin-converting enzyme 2 (ACE2) expressed on the cell surface of epithelial cells, inducing direct cytotoxicity; (2) The interaction of SARS-CoV-2 with ACE2 causes downregulation of ACE2 expression, thus preventing cleavage of angiotensin II (Ang II) to Ang (1-7) and resulting in dysregulation of the renin-angiotensin-aldosterone system; (3) excessive acute inflammatory responses are elicited, with overproduction of pro-inflammatory cytokines and chemokines; (4) SARS-CoV-2 exerts direct cytotoxic effects on endothelial cells, leading to recruitment of pro-inflammatory cells and activation of the coagulation cascade, with intravascular thrombus formation; and (5) extensive tissue destruction with interstitial thickening, fibroblast proliferation, and fibrosis.
The lungs possess several features that facilitate their role as an initial reservoir for viral replication and human-to-human transmission. Lung tissue has a large surface area, making it highly susceptible to inhaled viruses. It is also highly vascularized, which allows rapid dissemination of viral particles to other organs. Furthermore, ACE2 is expressed in several pulmonary cell types (Hamming et al., 2004), with alveolar epithelial type II cells being the major ACE2-expressing cells (Zhao Y. et al., 2020). Gene ontology enrichment analysis has demonstrated that alveolar epithelial type II cells express multiple viral life cycle-associated functional genes, including those related to virus internalization, genome replication, assembly, and transmission (Zhao Y. et al., 2020).
The production and exocytosis of new viral particles can induce the host cell to undergo pyroptosis and release damage-associated molecular pattern signals. In lung tissue, these are recognized by adjacent epithelial and endothelial cells that are primed to activate certain transcription factors, such as nuclear factor-κB (NF-κB) and interferon regulatory factor 3 (IRF3), to produce and secrete pro-inflammatory mediators (Li G. et al., 2020). Concomitantly, secretion of type I interferons occurs and induces antiviral actions by multiple mechanisms. In an immunocompetent response, these initial inflammatory signals are recognized by antigen-presenting cells—such as resident macrophages and dendritic cells—that present the foreign antigen to CD4+ T-cells, which then prime other CD4+ T-cells, CD8+ T-cells, and B-cells (Channappanavar et al., 2014). CD8+ T-cells are cytotoxic and directly kill virus-infected cells, whereas B-cells produce neutralizing antibodies against the nucleocapsid and spike protein. Phagocytes remove apoptotic cells and neutralized viruses, resulting in well-coordinated clearance of infection with recovery and minimal lung tissue injury (Channappanavar et al., 2014; Li G. et al., 2020). On the other hand, during a faulty immune response occurs an intense inflammation with inefficient virus clearance, as well as overactivation of innate immunity with overproduction of pro-inflammatory cytokines and chemokines, including interleukin (IL)-1β, IL-6, tumor necrosis factor (TNF)-α, and others. This uncontrolled inflammatory process leads to alveolar-capillary barrier disruption; viral particles, along with the emerging cytokine storm, can then circulate to other organs, resulting in multiple organ dysfunction (Zhang et al., 2020b). Neutrophil extravasation into the alveolar space, hyaline membrane formation, and acute capillaritis are major features observed in histopathological analysis of lung cells in cases of severe COVID-19 (Barnes et al., 2020; Fox et al., 2020; Tian S. et al., 2020; BR236). The thromboembolic events observed in severe COVID-19 cases may also be associated with the inflammatory response triggered by the host-virus interaction, including activation of the coagulation cascade, formation of neutrophil extracellular traps (NETs), and leakage of fluid in the subendothelial compartment (Barnes et al., 2020; Helms et al., 2020; Tang et al., 2020; Varga et al., 2020).
The heterogeneous clinical manifestations in COVID-19 may be attributed to individual variation in expression not only of ACE2 and TMPRSS2 receptors, but also of genes related to inflammation and immune responses (Elhabyan et al., 2020; Ellinghaus et al., 2020; Russo et al., 2020; Zhao J. et al., 2020). Accordingly, the excessive acute inflammatory responses seen in individuals with severe COVID-19 may lead to MODS and death (Li H. et al., 2020b; Wang D. et al., 2020). Compared to healthy individuals, those infected with SARS-CoV-2 have demonstrated increased serum concentrations of many pro-inflammatory mediators, including IL-1β, IL-2, IL-6, IL-7, IL-8, interferon (IFN)-γ-induced protein 10 (IP-10), granulocyte colony-stimulating factor (G-CFS), monocyte chemoattractant protein 1 (MCP1), macrophage inflammatory protein (MIP)-1α, platelet-derived growth factor (PDGF), TNF-α, vascular endothelial growth factor (VEGF), and others (Ackermann et al., 2020; Chen N. et al., 2020; Huang C. et al., 2020; Liu J. et al., 2020; Zhang et al., 2020b). This cytokine storm is even more evident in individuals admitted to the intensive care unit (ICU), as demonstrated by greater increases in serum concentration of several of these pro-inflammatory mediators, which suggests a correlation with disease severity (Chen N. et al., 2020; Huang C. et al., 2020; Liu J. et al., 2020; Zhang et al., 2020b). Aberrant pathogenic CD4+ T-cells co-expressing IFN-γ and G-CFS have also been observed in severe COVID-19 (Zhou Y. et al., 2020). A high neutrophil-to-lymphocyte ratio has also been reported as a risk factor for mortality in hospitalized individuals with COVID-19 (Liu et al., 2020a). Nevertheless, individuals with severe COVID-19 may also present with lymphopenia, characterized by a significant reduction in peripheral CD4+ T-cell and CD8+ T-cell counts. Such countervailing effects may prevent complete clearance of SARS-CoV-2 or lead to secondary infections by opportunistic pathogens, thus resulting in a sepsis-like state (Guan et al., 2020; Liu J. et al., 2020; Zhou F. et al., 2020). Below, we summarize the main features of SARS-CoV-2 infection and its potential repercussions on multiple organs (Figure 6).
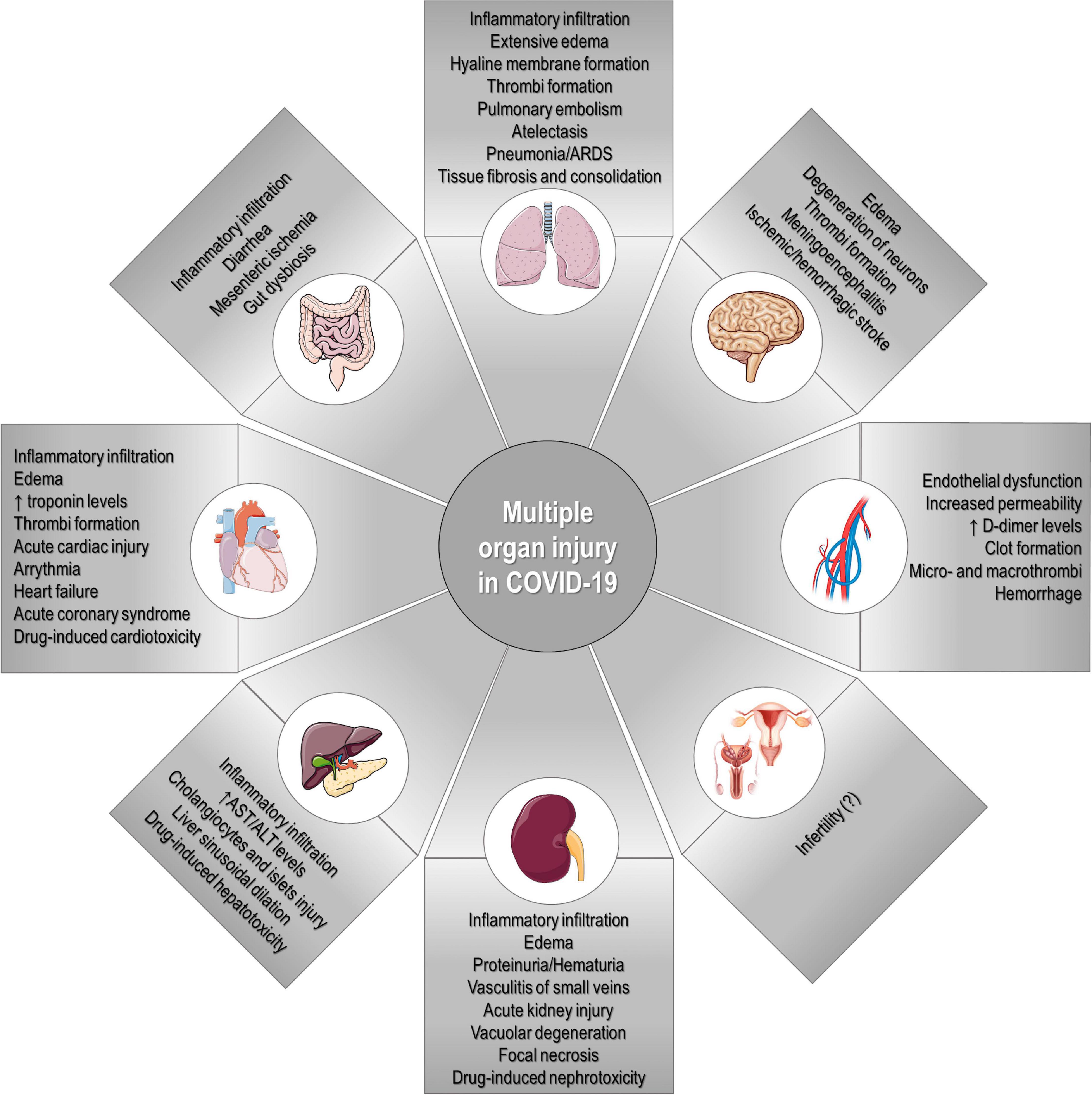
Figure 6. Multiple organ injury in COVID-19. SARS-CoV-2 infection can potentially cause multiple clinical manifestations and affect several organs, including the lungs, heart, blood vessels, kidney, intestine, liver, brain, and reproductive system.
Respiratory System
The lung is undoubtedly the organ most vulnerable to, and most affected by, SARS-CoV-2 infection. Accordingly, severe pneumonia is the most common and serious clinical manifestation observed in severe COVID-19 cases (Huang C. et al., 2020; Zhou Y. et al., 2020). As ACE2 is expressed in various cell types along the respiratory tract, SARS-CoV-2 may either enter through mucosal membranes in the upper respiratory tract or directly infect bronchial and alveolar epithelial cells in the lower respiratory tract (Sungnak et al., 2020; Wölfel et al., 2020). Interestingly, nasal expression of ACE2 was found to be lower in children compared to adults, which might partially explain age-related differences in the risk of developing COVID-19 (Bunyavanich et al., 2020; Sharif-Askari et al., 2020). SARS-CoV-2 RNA has also been detected in the sputum of infected individuals before the onset of clinical symptoms (Zhang W. et al., 2020), and in some cases, even 2 weeks after recovery (Lauer et al., 2020; Wölfel et al., 2020). Current and former smokers, as well as individuals with chronic obstructive pulmonary disease, overexpress ACE2 in airway cells compared to non-smokers and healthy individuals, which may explain at least partly the increased risk of severe COVID-19 in these individuals (Cai, 2020; Leung et al., 2020; Sharif-Askari et al., 2020).
ACE2 acts as a double-edged sword in both SARS-CoV and SARS-CoV-2 infection; it not only serves as the functional receptor for virus entry into host cells, but also has a pivotal role in protecting lung tissue from injury by counter-regulating the vasoconstrictive, pro-inflammatory, and pro-fibrotic effects of Ang II on pulmonary vascular and epithelial cells (Kuba et al., 2005; Uhal et al., 2011). Virus-receptor interactions lead to virion-ACE2 internalization (Wang et al., 2008) or cleavage and shedding of ACE2 (Haga et al., 2010; Xu et al., 2017), thus reducing its expression while promoting accumulation of Ang II, production of TNF-α and IL-6 receptor, and activation of macrophages to a pro-inflammatory state (Jia et al., 2009; Glowacha et al., 2010; Haga et al., 2010; Gheblawi et al., 2020). Furthermore, the virus nucleocapsid protein may interact with Smad3 to prevent apoptosis of infected host cells, while promoting transforming growth factor (TGF)-β-mediated tissue fibrosis (Zhao et al., 2008). As alveolar epithelial type II cells are self-renewing and express high levels of ACE2, they may be continuously targeted for viral entry and replication, which induces a vicious cycle of tissue injury and repair that may ultimately result in replacement of gas-exchange areas to non-functional fibrotic tissue. Lung stem/progenitor cells also express ACE2, and active virus replication in these cells may impair lung tissue repair (Ling et al., 2006).
In addition to the pivotal role of ACE2 in COVID-19 pathogenesis, a genome-wide association analysis identified other host genetic factors that may contribute to the development of COVID-19–induced respiratory failure. Although there were no single nucleotide polymorphism (SNP) associations in the human leukocyte antigen (HLA) complex, SNPs in a cluster of six genes on chromosome 3p21.31 (SLC6A20, ZTFL1, CCR9, FYCO1, CXCR6, and XCR1) were found to be potentially influential (Ellinghaus et al., 2020). Furthermore, ABO blood group has been suggested to be involved in susceptibility to SARS (Cheng et al., 2005a) and COVID-19 (Ellinghaus et al., 2020; Zhao J. et al., 2020; Zietz and Tatonetti, 2020).
Lung histopathological findings have demonstrated considerable similarities among ARDS, SARS, and COVID-19 (Tian S. et al., 2020; Xu Z. et al., 2020; Zhang H. et al., 2020). These consist of an increased neutrophil and mononuclear cell count, diffuse alveolar injury with proteinaceous alveolar exudates, and hyperplasia of epithelial type II cells. In more severe lung injury, thickened alveolar septa, hyaline membrane formation, and thrombus formation have been observed, as well as consolidation with fibroblast proliferation and fibrosis. Multinucleated giant cells in alveoli have also been found in some cases (Tian S. et al., 2020; Xu Z. et al., 2020; Zhang H. et al., 2020). An increased rate of deep venous thrombosis and pulmonary embolism was found in COVID-19 cases admitted to the ICU, despite prophylactic use of anticoagulation agents (Helms et al., 2020; Klok et al., 2020; Lodigiani et al., 2020); this can rapidly worsen lung function and lead to respiratory failure.
Based on chest computed tomography findings, COVID-19–induced lung impairment can be grouped into three main phenotypes: (1) multiple, focal, potently overperfused ground-glass opacities; (2) inhomogeneously dispersed atelectasis; and (3) typical moderate-to-severe ARDS, with alveolar edema and low compliance (Robba et al., 2020a). As these phenotypes may be related to different pathological mechanisms and disease progression, personalized mechanical ventilation approaches should be implemented in order to allow more efficient clinical recovery for each individual.
Circulatory System
Coagulation dysfunction has been found in a high proportion of COVID-19 cases, as evidenced by increasing D-dimer levels and prolonged prothrombin time, as well as overt thrombotic manifestations (Guan et al., 2020; Helms et al., 2020; Huang C. et al., 2020; Tang et al., 2020; Wang D. et al., 2020). SARS-CoV-2 RNA has been detected in blood specimens of individuals with COVID-19 (Huang C. et al., 2020; Wölfel et al., 2020); as ACE2 is highly expressed in smooth muscle as well as arterial and venous endothelium in virtually all organs, these tissues can be directly targeted by SARS-CoV-2, with induction of endothelial dysfunction (Hamming et al., 2004; Ackermann et al., 2020; Varga et al., 2020). COVID-19–associated hypoxia resulting from pulmonary dysfunction leads to reduced blood flow and vasoconstriction, which also contribute to endothelial dysfunction (Helms et al., 2020; Levi et al., 2020; Varga et al., 2020). Furthermore, the excessive production of pro-inflammatory mediators leads to an imbalance between the amount of pro- vs. anti-coagulant factors and induction of platelet aggregation (Tang et al., 2020; Zuo et al., 2020). An increase in levels of thrombin, tissue factor V and VIII, and fibrinogen, alongside NET formation, results in a hypercoagulable state with increased risk of systemic macro- and micro-thrombosis (Barnes et al., 2020; Levi et al., 2020; Tang et al., 2020; Zuo et al., 2020). Indeed, fibrinous exudates and thrombi have been observed in histopathological specimens obtained from individuals with COVID-19 (Ackermann et al., 2020; Tian S. et al., 2020; Zhang H. et al., 2020). Therefore, laboratory monitoring of D-dimer levels, fibrinogen, platelet count and prothrombin time is crucial in all hospitalized and severe cases of COVID-19.
An increased incidence of cardiovascular complications has been observed in severe COVID-19, with a high incidence of clinical symptoms of heart disease (palpitations and chest tightness), elevated cardiac biomarker levels tests (especially cardiac troponin I or T), and abnormalities in electro- or echocardiography (Huang C. et al., 2020; Shi et al., 2020; Wang D. et al., 2020). In autopsy series of COVID-19 cases, most patients were found to have cardiomegaly, right ventricular dilatation (Fox et al., 2020), and mild fibrosis (Tian S. et al., 2020). The heart may be susceptible to direct SARS-CoV-2–mediated cytotoxicity, as ACE2 is highly expressed in cardiomyocytes (Hamming et al., 2004; Varga et al., 2020). Nevertheless, single-cell RNA sequencing has demonstrated that pericytes express higher levels of ACE2 than cardiomyocytes, and thus may also be targeted by SARS-CoV-2, leading to capillary endothelial dysfunction upon viral infection (Chen L. et al., 2020; Robba et al., 2020b). Heterozygosis for loss of ACE2 activity is believed to be sufficient to increase the risk of heart disease (Wang et al., 2014). The role of circulating soluble ACE2 is not well understood, but its levels are significantly increased in the presence of cardiovascular dysfunction and/or SARS-CoV-2 infection, and may be used as a biomarker (Úri et al., 2016; Xu et al., 2017; Sama et al., 2020; Wang K. et al., 2020). Furthermore, cytokine storm, compounded by a hypoxic state resulting from pulmonary dysfunction, may lead to direct myocardial injury. Early acute myocardial injury has been associated with a higher risk of in-hospital mortality in COVID-19 (Ni et al., 2020). Pre-existing cardiovascular diseases have also been associated with a worse prognosis, as COVID-19 may aggravate cardiac tissue injury and dysfunction (Chen N. et al., 2020; Wu and McGoogan, 2020; Zhou F. et al., 2020). Monitoring of cardiac function and standard biomarkers is recommended.
Urogenital System
A balance between the effects of ACE2/Ang (1-7) and Ang II is tightly regulated to maintain normal kidney function. As ACE2 is expressed in kidney tissue, mainly in the brush border of the proximal cells, these cells are susceptible to direct injury caused by SARS-CoV-2 infection (Hamming et al., 2004; Fan C. et al., 2020). Laboratory tests have frequently revealed evidence of kidney dysfunction in individuals with COVID-19, including proteinuria, hematuria, and elevated levels of serum creatinine and blood urea nitrogen (Cheng et al., 2020; Hirsch et al., 2020; Li Z. et al., 2020). Renal abnormalities suggestive of inflammation and edema have also been observed on computed tomography (Li Z. et al., 2020). COVID-19–induced acute kidney injury has been associated with a markedly elevated risk of in-hospital mortality—approximately 5 times higher than that of COVID-19 patients without acute kidney injury (Chen N. et al., 2020; Cheng et al., 2020; Guan et al., 2020; Li Z. et al., 2020; Wang D. et al., 2020). A substantial number of individuals who develop COVID-19–induced acute kidney injury also require dialysis (Hirsch et al., 2020). SARS-CoV-2 viral antigens have been found in urine and in kidney tissue, mainly tubular epithelial cells and podocytes, at autopsy (Diao et al., 2020; Pan X. et al., 2020; Su et al., 2020); histopathological studies showed diffuse tubular injury with loss of brush border integrity, vacuolar degeneration, and necrosis (Su et al., 2020). Intraluminal erythrocyte aggregation in capillaries, without the presence of fibrinoid material, has also been reported (Su et al., 2020). The potential for direct viral damage to the kidney notwithstanding, inflammation and antiviral agents can also induce nephrotoxicity; therefore, kidney biomarkers and fluid and electrolyte status should be closely monitored to prevent kidney injury.
Despite a similar prevalence across genders, a higher case fatality rate has been observed in men with COVID-19, regardless of age (Jin et al., 2020). It is worth noting that testicular and vas deferens cells have a much higher expression of ACE2 than ovaries (Fan C. et al., 2020; Shastri et al., 2020; Wang and Xu, 2020), which could partially explain differences in disease severity and, consequently, fatality. Furthermore, sex-chromosome genes and sex hormones may contribute to the differential regulation of immunological responses between genders (Bukowska et al., 2017; Taneja, 2018). A high density of immune-related genes is located on the X chromosome, as is ACE2. This functional mosaic could influence innate and adaptive immune responses or disease severity (Taneja, 2018). In this context, women have been found to mount a more robust T-cell activation response than men during SARS-CoV-2 infection, which may contribute to a more efficient virus clearance (Takahashi et al., 2020).
Gastrointestinal System
A considerable proportion of patients with COVID-19 present with abdominal pain, nausea, diarrhea, and vomiting, suggesting that SARS-CoV-2 infection may cause gastrointestinal dysfunction (Guan et al., 2020; Huang C. et al., 2020; Robba et al., 2020b; Wang D. et al., 2020; Zhou F. et al., 2020). Indeed, ACE2 is abundantly expressed in the luminal surface of intestinal epithelial cells (Hamming et al., 2004), where it acts as a co-receptor for amino acid uptake (Hashimoto et al., 2012). SARS-CoV-2 nucleocapsid protein has been found along multiple gastrointestinal structures (Lamers et al., 2020; Xiao et al., 2020), as well as in stool specimens (Holshue et al., 2020; Wölfel et al., 2020; Zhang et al., 2020a), which suggests that oral-fecal transmission might occur. Diffuse endothelial inflammation of the small intestine and mesenteric ischemia were observed in histopathological examination of COVID-19 cases (Varga et al., 2020). Although gastrointestinal symptoms have not been associated with increased risk of mortality, they appear to correlate with a longer duration of illness (Mao R. et al., 2020; Pan L. et al., 2020). Furthermore, altered liver function tests have been observed in several individuals with COVID-19, including elevated levels of transaminases (aspartate aminotransferase and alanine aminotransferase), total bilirubin (Guan et al., 2020; Huang C. et al., 2020; Wang D. et al., 2020), and γ-glutamyl transferase (Fan Z. et al., 2020; Zhang et al., 2020a). At autopsy, mild lobular infiltration by lymphocytes, central sinusoidal dilation, and patchy necrosis of the liver were reported in a case series of COVID-19 (Tian S. et al., 2020; Xu Z. et al., 2020). Kupffer cell proliferation and chronic hepatic congestion were also observed in another case series (Lax et al., 2020). As cholangiocytes express ACE2, they are susceptible to direct virus-induced cytotoxicity (Chai et al., 2020). However, it remains unclear whether liver injury in COVID-19 is due to SARS-CoV-2 infection, systemic inflammation, drug-related, or multifactorial. As most drugs under investigation as therapies for COVID-19 are metabolized in the liver, its function should be monitored periodically.
Nervous System
Clinical manifestations consistent with neurological dysfunction, such as headache, confusion, and sudden loss of olfaction and gustation, as well as visual impairment, have been reported with increasing frequency in cases of COVID-19 (Butowt and Bilinska, 2020; Chen N. et al., 2020; Mao L. et al., 2020; Robba et al., 2020b; Wu P. et al., 2020). As ACE2 is expressed in olfactory bulb cells, neurons, astrocytes, and oligodendrocytes, the virus may rapidly disseminate through important brain areas once the olfactory epithelium is infected (Chen R. et al., 2020). SARS-CoV-2 may also infect the cerebral vascular endothelium and cross the blood–brain barrier via infected leukocytes, thus migrating into the central nervous system (Li Y. et al., 2020). Although no evidence of SARS-CoV-2 infection was observed in a first report of brain autopsies (Solomon et al., 2020), the presence of SARS-CoV-2 RNA in cerebrospinal fluid has been reported in patients with meningoencephalitis, suggesting that neurological manifestations might be due to direct invasion by SARS-CoV-2 (Huang Y.H. et al., 2020; Moriguchi et al., 2020). An autopsy series also reported cerebral edema and partial neuronal degeneration in individuals with COVID-19 (Xu Z. et al., 2020). Further evidence of SARS-CoV-2 neurotropism has been observed in other autopsies of COVID-19 cases, with such manifestations as lymphocytic encephalitis, meningitis, and massive intracranial hemorrhage (Von Weyhern et al., 2020). Furthermore, SARS-CoV-2 infection of the brainstem may be at least partially responsible for respiratory and cardiovascular failure (Li Y. et al., 2020).
Potential Therapeutic Approaches
The identification of similarities among human coronaviruses has provided some clues for the development of effective therapies (Table 1), especially for specific vaccines or effective antivirals against SARS-CoV-2. Several therapeutic approaches are under experimental and clinical investigation for COVID-19, but most are existing drugs approved for other disease indications. At least 66 human proteins or host factors can be targeted by clinically approved drugs in order to potentially inhibit SARS-CoV-2/host cell interactions (Gordon D.E. et al., 2020). Drug repurposing offers an advantage, as these drugs are already available in the clinic and have undergone extensive toxicological studies before marketing approval (Pushpakom et al., 2019; Battaglini et al., 2020; Lopes-Pacheco, 2020; Rocco et al., 2020). Therefore, the time frame to acquire an indication for COVID-19 may be reduced if safety and efficacy are demonstrated in late-stage clinical trials. Nevertheless, as COVID-19 is a multifaceted disease (Li H. et al., 2020b; Zhang B. et al., 2020b), multi-target therapeutic approaches are required to reduce or even prevent SARS-CoV-2 infection and its downstream effects—namely, systemic inflammation and multiple organ injury. To date, over 4,000 clinical trials of COVID-19 therapies have been registered in the U.S. National Institutes of Health Clinical Trials Platform4 (Figure 7). In this section, we collected a wide number of therapeutics under investigations and some that, despite being extensively evaluated in the clinic, demonstrated no efficacy against COVID-19.
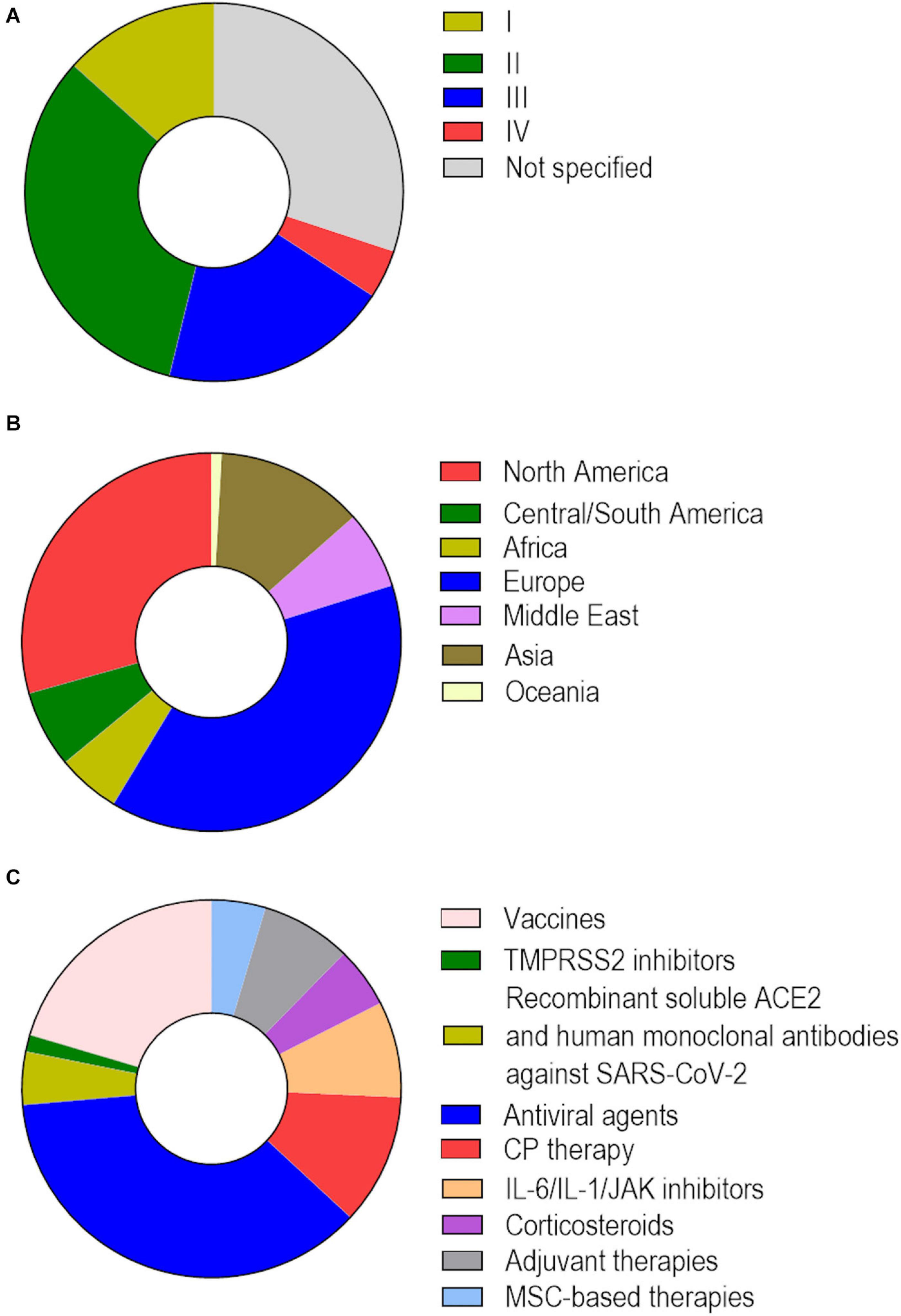
Figure 7. Clinical trials of COVID-19 therapeutics registered on the U.S. National Institutes of Health Clinical Trials Platform. As of December 7th, 2020, there were 4,094 registered clinical trials to evaluate the safety and efficacy of therapeutic approaches for COVID-19. (A) Distribution based on study phase: I (13.3%), II (32.9%), III (19.6%), and IV (4.1%). (B) Distribution based on region: North America (28.9%), Central/South America (6.5%), Africa (5.2%), Europe (37.9%), Middle East (6.5%), Asia (12.5%), Oceania (0.9%). (C) Distribution of 1,520 clinical trials based on therapeutic approaches under investigation: vaccines (20.4%), TMPRSS2 inhibitors (1.4%), recombinant soluble ACE2 and human monoclonal antibodies against SARS-CoV-2 (4.5%), agents with known or purported antiviral activity (ribavirin, favipiravir, remdesivir, lopinavir, umifenovir, ivermectin, nitazoxanide, chloroquine/hydroxychloroquine) (36.7%), convalescent plasma (CP) therapy (11.2%), IL-6/IL-1/JAK inhibitors (8.2%), corticosteroids (5.2%), adjuvant therapies (low-molecular-weight heparin, bevacizumab, recombinant human DNase) (7.7%), and mesenchymal stromal cell (MSC)-based therapies (4.6%).
Vaccine Development
The best long-term strategy to stop SARS-CoV-2 transmission and prevent new infections is the development of an effective vaccine. There are many efforts in progress from both the scientific community and the pharmaceutical industry to develop vaccines against SARS-CoV-2; the current pipeline has over 200 candidates, of which 45 are in clinical trials and five have received interim or conditional approval for emergency use in different countries. These include the traditional inactivated and attenuated vaccines, as well as novel DNA- and RNA-based vaccines. Major advantages and limitations of each vaccine type have been discussed in detail elsewhere (Badgujar et al., 2020; Rawat et al., 2020). Among these, five have demonstrated promising results in preliminary phase III trials (Table 2). AZD1222 (formerly ChAdOx1-S, from Oxford/AstraZeneca) is a non-replicating viral vector vaccine comprising the SARS-CoV-2 spike protein that has demonstrated protective effects in rhesus macaques after a single dose (Van Doremalen et al., 2020), and 62–90% efficacy when given in two-dose regimen in humans (Voysey et al., 2020). Vaccines developed by Moderna and Pfizer/BioNTech (mRNA-based vaccines) (Jackson et al., 2020; Polack et al., 2020), Gamaleya (recombinant viral vector-based vaccine) (Logunov et al., 2020), and Sinovac (inactivated viral-based vaccine) (Zhang Y. et al., 2020) were also reported to have high efficacy. The importance of vaccine development notwithstanding, alternative therapeutic approaches are being concomitantly investigated to identify effective therapies against COVID-19 with the necessary urgency.
Therapeutic Approaches Aimed at Preventing Cell Entry and Replication of SARS-CoV-2
TMPRSS2 Inhibitors
Camostat mesilate (FoipanTM) is a serine protease inhibitor clinically approved for squamous cell carcinoma and chronic pancreatitis in Japan. It significantly reduces viral load of SARS-CoV, human coronavirus NL63, and SARS-CoV-2 in vitro by partially blocking TMPRSS2 activity (Kawase et al., 2012; Hoffmann et al., 2020a). Nafamostat mesilate (BuipelTM) is another serine protease inhibitor used in Japan that has been demonstrated to inhibit MERS-CoV and SARS-CoV-2 entry into host cells by targeting TMPRSS2 (Hoffmann et al., 2020b; Yamamoto et al., 2020). Nafamostat was even more effective at inhibiting SARS-CoV-2 infection in vitro than camostat (Yamamoto et al., 2016, 2020; Hoffmann et al., 2020b). Furthermore, nafamostat is approved for disseminated intravascular coagulation in Japan due to its anticoagulant properties, which is an additional advantage for the treatment of COVID-19, given the high prevalence of coagulation disturbances described above (Tang et al., 2020; Zhou F. et al., 2020).
Cathepsin Inhibitors
In vitro studies demonstrated that E64d, a non-selective cysteinyl cathepsin inhibitor, was able to limit both SARS-CoV and SARS-CoV-2 infection in human epithelial cells, while the combination of E64d with a TMPRSS2 inhibitor completely abrogated viral entry (Hoffmann et al., 2020a, b). The investigational compound K11777 and three of its analogs demonstrated strong antiviral activity against SARS-CoV pseudotypes in vitro (Zhou et al., 2015). Oxocarbazate was also effective at inhibiting SARS-CoV and Ebola virus entry into cells (Shah et al., 2010). These agents, all cathepsin inhibitors, have potential therapeutic utility in COVID-19.
RAAS Modulators
Some concerns have been raised regarding the long-term use of ACE inhibitors or angiotensin receptor blockers (e.g., captopril, losartan) for individuals with pre-existing cardiovascular diseases during the COVID-19 pandemic, as these drugs might upregulate ACE2 and could theoretically enhance susceptibility to SARS-CoV-2 infection and COVID-19 severity. However, multiple studies have found no correlation between use of RAAS inhibitors and likelihood of testing positive for SARS-CoV-2 infection, nor with COVID-19 severity in those infected (Iaccarino et al., 2020; Khera et al., 2020; Mancia et al., 2020; Reynolds et al., 2020). In fact, in a retrospective study, a reduction in COVID-19–related mortality was observed in hospitalized individuals with hypertension who had been treated with ACE inhibitors or angiotensin receptor blockers compared to those not using any of these drugs (Zhang P. et al., 2020). Furthermore, abrupt discontinuation of RAAS inhibitors in individuals with cardiovascular disease and on long-term therapy is not recommended, as it may cause clinical decompensation (Danser et al., 2020). Based on the protective role of ACE2 as a counter-regulator of Ang II/AT1-R effects, therapeutic approaches that restore the balance between ACE and ACE2 would be ideal to mitigate COVID-19–induced multiple organ injury in individuals without pre-existing medical conditions, preferably in combination with an effective antiviral agent.
Recombinant Soluble ACE2 and Human Anti-SARS-CoV-2 Monoclonal Antibodies
It has been proposed that a recombinant soluble form of ACE2 (rhACE2), administered exogenously, may competitively bind to the SARS-CoV-2 RBD and thus prevent its interaction with native ACE2 for host-cell entry. Such an approach not only would neutralize the virus but also preserve ACE2 activity, thus protecting lung and cardiovascular tissues from injury (Monteil et al., 2020). In a pilot study, one rhACE2 formulation (known as GSK2586881) demonstrated no safety concerns and was able to decrease levels of Ang II with a trend toward decreasing IL-6 levels in serum (Kahn et al., 2017). This rhACE2 also inhibited SARS-CoV-2 infection in both engineered human blood vessels and kidney organoids in vitro (Monteil et al., 2020). A clinical trial is ongoing to evaluate the effects of rhACE2 in individuals with COVID-19 (NCT04335136). Alternatively, CR3022 is a SARS-CoV-specific human monoclonal antibody that was able to bind to SARS-CoV-2 RBD and neutralize viral actions. CR3022 may be a promising candidate to prevent or significantly reduce SARS-CoV-2 infection (Tian X. et al., 2020). A monoclonal antibody obtained from B-cells of individuals recently recovered from COVID-19 has also demonstrated ability to inhibit SARS-CoV-2 RBD and ACE2 interactions (Chen X. et al., 2020).
Antiviral Drugs
An ideal antiviral agent should target factors that are highly conserved among coronaviruses and essential for viral pathogenesis. A number of antivirals under investigation for COVID-19 are nucleoside analogs used for other viruses. These molecules are incorporated into nascent DNA/RNA chains during viral replication, and lead to premature termination of nucleic acid synthesis or insertion of mutations in the viral genome that prevent subsequent viral replication. Ribavirin is a guanosine analog used for the treatment of hepatitis C virus, respiratory syncytial virus, and certain viral hemorrhagic fevers. A recent in vitro study demonstrated that high-dose ribavirin can reduce SARS-CoV-2 infection (Wang M. et al., 2020). It was also tested with and without IFN-α in individuals infected with SARS-CoV and MERS-CoV, but whether it has any therapeutic benefit remains controversial (Zhao et al., 2003; Arabi et al., 2020). Severe adverse effects were also reported, including hepatotoxicity and hemolysis.
Favipiravir (AviganTM) is another guanosine analog that selectively inhibits the influenza viral RNA-dependent RNA polymerase (RdRp). It has been approved against influenza virus but also demonstrated antiviral activity against Ebola virus, yellow fever virus, and other viruses in experimental models (Furuta et al., 2017). As with ribavirin, a high dose of favipiravir was able to reduce SARS-CoV-2 infection in vitro (Wang M. et al., 2020). In an early clinical study, favipiravir demonstrated better clinical outcomes compared to umifenovir (see below), with a significant decrease in fever and cough in individuals with COVID-19 (Chen N. et al., 2020). In a similar study, favipiravir therapy was associated with a shorter time to viral clearance and higher improvement rate in chest imaging of individuals with COVID-19 (Cai et al., 2020), resulting in extension of its approved indications by the National Medical Products Administration of China.
Remdesivir (VekluryTM) is an adenosine analog with a broad spectrum of antiviral activity against several RNA viruses. It blocks RdRp, thus preventing an early step of viral replication (Gordon C.J. et al., 2020; Sheahan et al., 2020). Remdesivir has been shown to inhibit viral replication of Ebola virus, MERS-CoV, and SARS-CoV-2 in experimental studies, with higher efficacy compared to other antiviral agents (Gordon C.J. et al., 2020; Sheahan et al., 2020; Wang M. et al., 2020). Furthermore, it demonstrated a favorable benefit-risk profile compared to placebo in early-stage clinical study in individuals with severe COVID-19 (Davies et al., 2020). In a compassionate-use setting, remdesivir was used in hospitalized individuals with severe COVID-19 and 68% of the cohort demonstrated an improvement in oxygen-support class and 25% were discharged during 18 days follow-up (Grein et al., 2020). In phase III multicenter randomized clinical trials, remdesivir appeared to shorten the recovery time of some patients, although therapeutic benefits were not clearly demonstrated in terms of reducing fatality rate of individuals with severe COVID-19 (Beigel et al., 2020; Wang et al., 2020b). Despite its inefficiency in improving survival (Dyer, 2020), final reports of the multicenter clinical trial indicated that remdesivir might shorten the time to recovery of hospitalized adults with COVID-19 and reduce lower respiratory tract infection, which culminated in its FDA approval.
Besides nucleoside analogs, antiviral agents may block viral replication through distinct mechanisms, including inhibition of endosomal acidification and inhibition of proteases essential for intracellular assembly. The fixed-dose combination lopinavir/ritonavir (KaletraTM) is an FDA-approved therapy for human immunodeficiency virus (HIV). Lopinavir is an inhibitor of HIV-1 protease, while ritonavir prevents the rapid metabolism of lopinavir by inhibiting CYP3A isoenzymes. This combination has demonstrated antiviral activity against SARS-CoV and MERS-CoV, reducing viral load in experimental studies (Chu et al., 2004; Chan et al., 2015), and was associated with modest clinical improvement of MERS in a case report (Kim et al., 2016). Furthermore, a milder disease course was observed in individuals infected with SARS-CoV who received lopinavir/ritonavir (Chu et al., 2004). Initially, lopinavir/ritonavir appeared to accelerate the recovery process from COVID-19, shortening ICU length of stay compared to standard therapy alone; however, it has since proven unable to reduce viral load or mortality in adults hospitalized with severe COVID-19 (Cao et al., 2020; Osborne et al., 2020). Darunavir is a second-generation protease inhibitor used in combination with ritonavir or cobicistat for HIV therapy. It was also considered as a potential therapeutic alternative in COVID-19; however, darunavir demonstrated no antiviral activity against SARS-CoV-2 in vitro (De Meyer et al., 2020).
Umifenovir (ArbidolTM) is a dual-acting antiviral/host-targeting agent approved for treatment of influenza in Russia and China. It inhibits membrane fusion of viral envelope and host cell by preventing clathrin-mediated endocytosis and has been demonstrated to prevent in vitro infection with several common pathogenic viruses (Pécheur et al., 2016). In an early clinical study of COVID-19, umifenovir therapy was associated with a trend toward reductions in viral load and mortality rate, albeit not statistically significant (Wang Z. et al., 2020). In a retrospective study, most umifenovir-treated individuals demonstrated SARS-CoV-2 negativity and improvement in chest imaging after 14 days of therapy (Deng et al., 2020). However, there is no additional evidence to support that umifenovir may improve clinical outcomes of individuals with COVID-19.
Two investigational antiviral drug candidates (11a and 11b) have been developed by structure-based design methods to target 3CL protease (Mpro), the main SARS-CoV-2 protease. Both demonstrated good pharmacokinetic properties and low toxicity and were able to significantly reduce viral load in vitro (Dai et al., 2020). Further development is ongoing.
Despite some promising results in experimental and early clinical studies, large-scale randomized trials are still in progress and their results on safety and efficacy will provide a better guidance for the potential use of these drugs in the treatment of COVID-19.
Other Anti-infectives
Ivermectin and nitazoxanide are two clinically approved antiparasitic agents that have demonstrated significant antiviral activity against SARS-CoV-2 infection in vitro (Caly et al., 2020; Rocco et al., 2020; Wang Z. et al., 2020). A recent study (Rocco et al., 2020) confirmed that early administration of nitazoxanide (1-3 days after onset of symptoms) reduced the viral load in individuals with mild COVID-19 with a good safety profile, even though more studies are required to evaluate its efficacy in individuals with both mild and severe COVID-19. Clinical safety and efficacy of ivermectin in COVID-19 have yet to be confirmed.
Chloroquine and hydroxychloroquine are aminoquinoline antimalarials also used in the treatment of several autoimmune diseases. Both drugs have demonstrated a broad spectrum of antiviral activity in vitro—including against coronaviruses—through various mechanisms, such as blocking ACE2 terminal glycosylation and hindering endosome–lysosome fusion (De Wilde et al., 2014; Mauthe et al., 2018; Wang M. et al., 2020). In early clinical studies, chloroquine and hydroxychloroquine were suggested to reduce viral load and improve clinical outcomes in individuals with COVID-19 (Gao et al., 2020; Gautret et al., 2020) with an even better effect when combined with azithromycin (Gautret et al., 2020). However, such findings were not confirmed in later, well-designed clinical trials; both chloroquine and its hydroxy analog appear ineffective, whether used therapeutically or for postexposure prophylaxis, with conflicting evidence regarding their safety and toxicity (Borba et al., 2020; Boulware et al., 2020; Cavalcanti et al., 2020; Geleris et al., 2020; Lane et al., 2020; Molina et al., 2020).
Convalescent Plasma Therapy
Convalescent plasma (CP) is plasma rich in neutralizing antibodies which has been extracted from individuals who have recovered from an infection. This plasma is processed and then administered to infected individuals. CP has been demonstrated to reduce viral load and improve clinical outcomes in other coronavirus infections, specifically SARS and MERS (Cheng et al., 2005b; Ko et al., 2018). In early-stage clinical studies, CP therapy was well tolerated and improved clinical outcomes by neutralizing viremia in severe COVID-19 (Duan et al., 2020; Shen et al., 2020; Ye et al., 2020). Improvements in clinical scales as well as discharge and survival rate in individuals with severe COVID-19 were observed 14 days after CP therapy (Salazar et al., 2020; Zhang et al., 2020a). Several clinical trials of CP are in progress. Based on previous experience with influenza A (H1N1), some practical limitations may apply, and should be borne in mind when seeking to implement an effective CP therapy regimen for COVID-19. These limitations have been discussed elsewhere (Wong et al., 2010). Antibodies produced in humanized mouse or equine serum have been investigated as alternative strategies to neutralized SARS-CoV-2 with some promising results – up to 100 times more potent than convalescent plasma from COVID-19-recovered individuals (Cunha et al., 2020; Hansen et al., 2020).
Therapeutic Approaches Aimed at Immunomodulation and Tissue Repair
IL-6 Inhibitors
IL-6 is acutely induced by inflammatory stimuli and mediates a number of immune responses. Individuals with COVID-19 demonstrate an increase in serum levels of IL-6 within 3 days after disease onset, with even higher levels in severe cases compared to mild ones (Liu J. et al., 2020). A further increase in IL-6 levels has also been associated with increased risk of respiratory failure and death (Herold et al., 2020; Quartuccio et al., 2020; Ruan et al., 2020).
Tocilizumab (ActemraTM) is a recombinant humanized monoclonal antibody that binds and inhibits IL-6 receptor activity. It is indicated for the treatment of autoimmune disorders and cytokine release syndrome and has been suggested as a potential therapeutic option for COVID-19-induced hyperinflammation (Zhang et al., 2020a). In early clinical studies, tocilizumab significantly improved several outcomes in severe and critical COVID-19 cases, including supplemental oxygen utilization and C-reactive protein and D-dimer levels (Sciascia et al., 2020; Xu X. et al., 2020). In a subsequent study, tocilizumab therapy was associated with clinical improvements, as well as rapid and sustained benefits in ICU and non-ICU patients with COVID-19 (Toniati et al., 2020). Alternatively, siltuximab (SylvantTM) is a chimeric monoclonal antibody that directly bind to IL-6. Siltuximab provides an advantage compared to tocilizumab as it neutralizes circulating IL-6, which could contribute to neurotoxic effects. In an early clinical study, siltuximab therapy at the onset of mechanical ventilation was associated with reduced occurrence of respiratory failure and death in severe COVID-19 cases (Gritti et al., 2020). Sarilumab is another IL-6R inhibitor that appears to be a potential therapy against severe COVID-19 (Gremese et al., 2020).
IL-1 Inhibitors
The ORF3a protein of coronaviruses can activate NF-κB signaling and the NLRP3 inflammasome. The inflammasome activates cleavage of pro-IL-1β by caspase-1 into active IL-1β, which mediates lung inflammation and fibrosis (Siu et al., 2019). Anakinra (KineretTM), a recombinant IL-1 receptor antagonist, was evaluated in individuals with severe COVID-19 and demonstrated effective reductions in need for mechanical ventilation and fatality rate in an early clinical study (Huet et al., 2020). A retrospective study also indicated that high-dose anakinra was safe and associated with clinical improvements in over 70% of individuals with severe COVID-19 (Cavalli et al., 2020). At least 10 additional clinical trials are ongoing with the aim of evaluating the effects of anakinra in targeting hyperinflammation in COVID-19 (King et al., 2020). Colchicine is another drug known to reduce neutrophil recruitment and IL-1β levels, and widely used for the treatment of gout. In hospitalized individuals with COVID-19, colchicine did not affect C-reactive protein or cardiac troponin levels, although an improved time to clinical deterioration was reported (Deftereos et al., 2020).
Janus Kinase/Signal Transducer and Activators of Transcription (JAK/STAT) Inhibitors
Targeting the JAK/STAT pathway appears to be a promising approach, given its role in cytokine receptors on immune cells. JAK/STAT inhibitors have also been used for the treatment of cytokine release syndrome. Several clinical trials evaluating the effects of JAK/STAT inhibitors for COVID-19 are ongoing. Fedratinib, a JAK2 inhibitor, has been hypothesized to inhibit SARS-CoV-2 and Th17-induced inflammation without modulating IFN signaling, but efficacy remains to be investigated (Wu and Yang, 2020). In a recent clinical study of individuals with COVID-19, ruxolitinib, a JAK1/2 inhibitor, was not significantly superior to placebo in terms of clinical parameters, although faster recovery from lymphopenia was observed (Cao et al., 2020b). On the other hand, baricitinib, a high-affinity JAK1/2 inhibitor, has been shown to improve some clinical and laboratory parameters (including C-reactive protein levels) in a pilot study of COVID-19 cases (Cantini et al., 2020).
Corticosteroids
There is some controversy regarding corticosteroid therapy in COVID-19 (Mattos-Silva et al., 2020). Although corticosteroids are widely used to suppress lung inflammation, they were associated with delayed viral clearance and no improvement in fatality rate during the SARS and MERS epidemics (Li et al., 2020a). Nevertheless, methylprednisolone therapy has been shown to improve chest imaging, reduce fatality rate, and shorten hospital stay in individuals with severe COVID-19 (Ramiro et al., 2020; Wang et al., 2020a).
Recent preliminary results of low-dose dexamethasone therapy also demonstrated a significant reduction in fatality rate (up to one-third) in critically ill individuals with COVID-19, although such benefits were not observed for the cohort of individuals who did not require oxygen therapy at admission (Horby et al., 2020). It is possible that corticosteroid therapy may be beneficial in certain phases of COVID-19, such as the hyperinflammatory stage, but certainly should be combined with an effective antiviral or antibiotic agent to reduce the risk of superinfection. Further clinical studies are necessary to better understand the effects of corticosteroid therapy in COVID-19.
Adjuvant Therapy
Unfractionated or low-molecular-weight heparin has been used prophylactically in hospitalized individuals with COVID-19 to prevent the occurrence of thromboembolic events. Heparin can also induce immunomodulatory effects and protect endothelial cells from oxidative stress, thus preventing increased vascular permeability, microthrombus formation, and leukocyte extravasation. In an observational study, anticoagulant therapy with low-molecular-weight heparin was associated with better prognosis in severe COVID-19 cases with high levels of D-dimer (Tang et al., 2020). Despite current recommendations for the prophylactic use of low-molecular-weight heparin in all hospitalized COVID-19 patients (except those with contraindications) (Thachil et al., 2020), individuals admitted to the ICU remain at high risk of pulmonary embolism (Klok et al., 2020).
The use of recombinant human DNase (dornase) to disrupt NETs has been proposed, as SARS-CoV-2 was found to induce excessive production of NETs, which may contribute to thromboembolism events, cytokine storm, and tissue injury (Barnes et al., 2020; Veras et al., 2020). Dornase alfa (PulmozymeTM) has been long used as an inhalation solution for mucus clearance in individuals with cystic fibrosis; however, its effects on COVID-19 remain to be investigated. Bevacizumab (AvastinTM/ZaribevTM) is an anti-VEGF humanized monoclonal antibody that has been investigated to reduce lung edema in COVID-19 (Samudrala et al., 2020). Finally, mepolizumab (NucalaTM), an anti-CD147 humanized antibody used for the treatment of severe asthma, demonstrated to improve the recovery of individuals with COVID-19-induced severe pneumonia in a small-scale clinical study (Bian et al., 2020).
Mesenchymal Stromal Cell (MSC)-Based Therapies
Mesenchymal stromal cells and their biologically active products, such as extracellular vesicles, are known to induce immunomodulatory and reparative effects, reducing lung and distal organ injury and improving survival in several preclinical models of ARDS and sepsis (Matthay et al., 2017; Silva et al., 2018, 2019; Lopes-Pacheco et al., 2020). In early clinical studies, MSC administration was well tolerated and caused no obvious safety concerns in critically ill individuals (McIntyre et al., 2018; Matthay et al., 2019; Khoury et al., 2020). Notably, SARS-CoV-2 is unable to infect MSCs, as these cells do not express ACE2 (Leng et al., 2020). In one case report of compassionate use in severe COVID-19, administration of Wharton’s jelly-derived MSCs reduced plasma levels of IL-6, TNF-α, and C-reactive protein and improved lung function (Zhang Y. et al., 2020). In another early clinical study, bone marrow-derived MSCs were administered intravenously to seven individuals with severe COVID-19. MSC administration was safe and significantly reduced inflammation, resulting in improvements in symptoms and lung function (Leng et al., 2020). Alternatively, administration of bone marrow MSC-derived exosomes was evaluated in an early clinical study of individuals with severe COVID-19 and demonstrated no adverse events (Sengupta et al., 2020). Several other clinical trials are evaluating MSC-based therapies for COVID-19. Despite the great promise of MSCs for the treatment of COVID-19 complications, such as ARDS and sepsis, several open questions remain, including the best source, dose, route of administration, frequency, and timing (De Castro et al., 2019; Khoury et al., 2020; Lopes-Pacheco et al., 2020). Further understanding of the effects of MSCs on COVID-19 pathogenesis and their underlying mechanisms are also needed in order to translate MSC-based therapy into clinical practice with safety and effectiveness.
Outlook and Considerations
COVID-19 can cause not only severe lung injury but also multiple organ dysfunction with potential long-term effects on survivors. The downregulation of membrane-bound active ACE2 induced by SARS-CoV-2 infection can be detrimental to everyone, but is particular so for individuals whose baseline ACE2 expression is already deficient. Although infected individuals with certain pre-existing medical conditions are more prone to developing severe COVID-19, the disease is not exclusively restricted to this population, and a combination of multiple direct and indirect pathogenic factors contribute to disease severity and a broad spectrum of phenotypes. Even though vaccination has initiated in multiple countries, the production of vaccines on a global scale will take some time, despite having different vaccines approved against SARS-CoV-2. Accordingly, implementation of preventive measures remains crucial to limit rapid dissemination of the virus and potential COVID-19-induced organ injuries, as well as prevent saturation of national healthcare systems.
It is imperative to continuously monitor genetic modifications of coronaviruses, as any gain-of-function mutation affecting the life-cycle pathways of SARS-CoV-2 (and other viruses in this family) can make them more infectious and lethal to humans. In this context, a SARS-CoV-2 subtype with the D614G mutation in the spike protein was recently found to confer increased infectivity (Bhattacharyya et al., 2020; Hou et al., 2020); further investigations are ongoing to elucidate the lethality rate of this and other subtypes. More strict regulations against the trade of wild animals for domestication or food should also be implemented to prevent potential future pandemics.
Over the last two decades, we have witnessed three coronavirus outbreaks. The unprecedented consequences of COVID-19 pandemic have prompted a massive global research effort to better understand the pathological mechanisms underlying SARS-CoV-2 infection and—at an accelerated pace—develop safe and efficient therapies against this devastating condition. Although a number of these therapies have demonstrated promising results in early clinical studies, safety and efficacy remain to be demonstrated in large-scale clinical trials. A greater understanding of the clinical features has provided better guidance in managing the disease to prevent further complications. Furthermore, the phenotypical differences in COVID-19 manifestations suggest that personalized therapeutic regimens should be considered on a case-by-case basis, ranging from prevention of viral cell entry and replication to supportive, immunomodulatory and tissue reparative approaches. Therefore, multi-target therapeutic protocols may be the best option to achieve a higher number of individuals with severe COVID-19 and significantly reduce or even prevent multiple organ dysfunction.
Author Contributions
ML-P contributed to the design and conceptualization, original draft, editing, and review for intellectual content. PS, FC, DB, CR, PP, MM, and CCN reviewed the intellectual content. PR did the conceptualization and edited and reviewed the intellectual content. All authors read and approved the final version of the manuscript.
Funding
This study was supported by the Brazilian Council for Scientific and Technological Development (CNPq) and Carlos Chagas Filho Rio de Janeiro State Research Foundation (FAPERJ). ML-P is a recipient of the 2018 Gilead Sciences Research Scholars for Cystic Fibrosis.
Conflict of Interest
The authors declare that the research was conducted in the absence of any commercial or financial relationships that could be construed as a potential conflict of interest.
Acknowledgments
We express our gratitude to Mrs. Moira Elizabeth Schottler and Mr. Filippe Vasconcellos for their assistance in editing the manuscript.
Footnotes
- ^ WHO Director-General’s opening remarks at the media briefing on COVID-19 – 11 March 2020: https://www.who.int/dg/speeches/detail/who-director-general-s-opening-remarks-at-the-media-briefing-on-covid-19—11-march-2020
- ^ WHO Coronavirus Disease (COVID-19) Dashboard: https://covid19.who.int/
- ^ Johns Hopkins University & Medicine – Coronavirus Resource Center: https://coronavirus.jhu.edu/map.html
- ^ http://clinicaltrials.gov
References
Ackermann, M., Verleden, S. E., Kuehnel, M., Haverich, A., Welte, T., Laenger, F., et al. (2020). Pulmonary Vascular Endothelialitis, Thrombosis, and Angiogenesis in Covid-19. N. Eng. J. Med. 383, 120–128. doi: 10.1056/nejmoa2015432
Andersen, K. G., Rambaut, A., Lipkin, W. I., Holmes, E. C., and Garry, R. F. (2020). The Proximal Origin of SARS-CoV-2. Nat. Med. 26, 450–452. doi: 10.1038/s41591-020-0820-9
Arabi, Y. M., Shalhoub, S., Mandourah, Y., Al-Hammed, F., Al-Omari, A., Al Qasim, E., et al. (2020). Ribavirin and Interferon Therapy for Critically Ill Patients with Middle East Respiratory Syndrome: A Multicenter Observation Study. Clin. Infect. Dis. 70, 1837–1844. doi: 10.1093/cid/ciz544
Badgujar, K. C., Badgujar, V. C., and Badgujar, S. B. (2020). Vaccine development agains coronavirus (2003 to present): An orverview, recent advances, current scenario, opportunities and challenges. Diabetes Metab. Syndr. 14, 1361–1376. doi: 10.1016/j.dsx.2020.07.022
Barnes, N. J., Adrover, J. M., Baxter-Stoltzfus, A., Borczuk, A., Cools-Lartigue, J., Crawford, J. M., et al. (2020). Targeting Potential Driver of COVID-19: Neutrophil Extracellular Traps. J. Exp. Med. 217:e20200652.
Battaglini, D., Robba, C., Ball, L., Cruz, F. F., Silva, P. L., Pelosi, P., et al. (2020). Emerging therapies for COVID-19 pneumonia. Expert Opin. Investig. Drugs 29, 633–637.
Beigel, J. H., Tomashek, K. D., Dodd, L. E., Mehta, A. K., Zingman, B. S., Kalil, A. C., et al. (2020). Remdesivir for the Treatment of Covid-19 – Final Report. N. Engl. J. Med. 383, 1813–1826.
Bhattacharyya, C., Das, C., Ghosg, A., Singh, A. K., Mukherjee, S., Majumder, P. P., et al. (2020). Global Spread of SARS-CoV-2 Subtype with Spyke Protein Mutation D614G is Shaped by Human Genomic Variations that Regulate Expression of TMPRSS2 and MXI Genes. bioRxiv 2020:75911. doi: 10.1101/2020.05.04.075911
Bian, H., Zheng, Z.-H., Wei, D., Zhang, Z., Kang, W.-Z., Hao, C.-Q., et al. (2020). Meplazumab treats COVID-19 pneumonia: an open-labelled, concurrent controlled add-on clinical trial. medRxiv Preprint. doi: 10.1101/2020.03.21.20040691
Borba, M. G. S., Val, F. F. A., Sampaio, V. S., Alexandre, M. A. A., Melo, G. C., Brito, M., et al. (2020). Effect of High vs Low Doses of Chloroquine Diphosphate as Adjunctive Therapy for Patients with Severe Acute Respiratory Syndrome Coronavirus 2 (SARS-CoV-2) Infection: A Randomized Clinical Trial. JAMA Netw. Open 3:e208857.
Boulware, D. R., Pullen, M. F., Bangdiwala, A. S., Pastick, K. A., Lofgern, S. M., Ofagor, E. C., et al. (2020). A Randomizes Trial of Hydroxychloroquine as Postexposure Prophylaxis for Covid-19. N. Eng. J. Med. 383, 517–525.
Bukowska, A., Spiller, L., Wolke, C., Lendeckel, U., Weinert, S., Hoffmann, J., et al. (2017). Protective Regulation of the ACE2/ACE Gene Expression by Estrogen in Human Atrial Tissue from Elderly Men. Exp. Biol. Med. 242, 1412–1423. doi: 10.1177/1535370217718808
Bunyavanich, S., Do, A., and Vicencio, A. (2020). Nasal Gene Expression of Angiotensin-Converting Enzyme 2 in Children and Adults. JAMA 323, 2427–2429. doi: 10.1001/jama.2020.8707
Butowt, R., and Bilinska, K. (2020). SARS-CoV-2: Olfaction, Brain Infection, and the Urgent Need for Clinical Samples Allowing Earlier Virus Detection. ACS Chem. Neurosci. 11, 1200–1203. doi: 10.1021/acschemneuro.0c00172
Cai, G. (2020). Bulk and single-cell transcriptomics identify tobacco-use disparity in lung gene expression of ACE2, the receptor of 2019-nCov. medRxiv Preprint. doi: 10.1101/2020.02.05.20020107
Cai, Q., Yang, M., Liu, D., Chen, J., Shu, D., Xia, J., et al. (2020). Experimental Treatment with Favipiravir for COVID-19: An Open-Label Control Study. Engineering 6, 1192–1198. doi: 10.1016/j.eng.2020.03.007
Calcagnile, M., Forgez, P., Iannelli, A., Bucci, C., Alifano, M., and Alifano, P. (2020). ACE2 Polymorphisms and Individual Susceptibility to SARS-CoV-2 Infection: Inseghts from an In Silico Study. bioRxiv Preprint. doi: 10.1101/2020.04.23.057042
Caly, L., Druce, J. D., Catton, M. G., Jans, D. A., and Wagstaff, K. M. (2020). The FDA-approved Drug Ivermectin Inhibits the Replication of SARS-CoV.2 in Vitro. Antiviral Res. 178:104787. doi: 10.1016/j.antiviral.2020.104787
Cantini, F., Niccoli, L., Matarrese, D., Nicastri, E., Stobbione, and Goletti, D. (2020). Baracitinib Therapy in COVID-19: A Pilot Study on Safety and Clinical Impact. J. Infect. 81, 318–356. doi: 10.1016/j.jinf.2020.04.017
Cao, B., Wang, Y., Wen, D., Liu, W., Wang, J., Fan, G., et al. (2020). A Trial of Lapinavir-Ritonavir in Adults Hospitalized with Several Covid-19. N. Eng. J. Med. 382, 1787–1799.
Cao, Y., Li, L., Feng, Z., Wan, S., Huang, P., Sun, X., et al. (2020a). Comparative Genetic Analysis of the Novel Coronavirus (2019-nCov/SARS-CoV-2) Receptors ACE2 in Different Populations. Cell Discov. 6:11.
Cao, Y., Wei, J., Zou, L., Jiang, T., Wang, G., Chen, L., et al. (2020b). Ruxolitinib in Treatment of Severe Coronavirus Dosease 2019 (COVID-19): A Multicenter, Single-Blind, Randomized Controlled Trial. J. Allergy Clin. Immunol. 146, 137.e–146.e.
Cavalcanti, A. B., Zampieri, F. G., Rosa, R. G., Azevedo, L. C. P., Veiga, V. C., Avezum, A., et al. (2020). Hydroxychloroquine with or without Azithromycin in Mild-to-Moderate Covid-19. N. Eng. J. Med. 383, 2041–2052.
Cavalli, G., de Luca, G., Campochiaro, C., Della-Torre, E., Ripa, M., Canetti, D., et al. (2020). Interleukin-1 blockade with high-dose anakinra in patients with COVID-19, acute respiratory distress syndrome, and hyperinflammation: a retrospective cohort study. Lancet Rheumatol. 2, 325–331e.
Chai, X., Hu, L., Zhang, Y., Han, W., Lu, Z., Ke, A., et al. (2020). Specific ACE2 Expression in Cholangiocytes May Cause Liver Damage After 2019-nCov Infection. bioRxiv Preprint. doi: 10.1101/2020.02.03.931766
Chan, J. F.-W., Kok, K.-H., Zheng, Z., Chu, H., To, K. K.-W., Yuan, S., et al. (2020). Genomic Characterization of the 2019 Novel Human-Pathogenic Coronavirus Isolated From a Patients with Atypical Pneumonia After Visiting Wuhan. Emerg. Microbes Infect. 9, 221–236. doi: 10.1080/22221751.2020.1719902
Chan, J. F.-W., Yao, Y., Yeung, M.-L., Deng, W., Bao, L., Jia, L., et al. (2015). Treatment with Lopinavir/Ritonavir or Interferon-β1b Outcome of MERS-CoV Infection in a Nonhuman Primate Model of Common Marmoset. J. Infect. Dis. 212, 1904–1913. doi: 10.1093/infdis/jiv392
Channappanavar, R., Zhao, J., and Perlman, S. (2014). T Cell-Mediated Immune Response to Respiratory Coronaviruses. Immunol. Res. 59, 118–128. doi: 10.1007/s12026-014-8534-z
Chen, J., Jiang, Q., Xia, X., Liu, K., Yu, Z., Tao, W., et al. (2020). Individual Variation of the SARS-CoV-2 Receptor ACE 2 Expression and Regulation. Aging Cell 19:e13168.
Chen, L., Li, X., Chen, M., Feng, Y., and Xiong, C. (2020). The ACE2 Expression in Human Heart Indicates New Potential Mechanism of Heart Injury Among Patients Infected with SARS-CoV-2. Cardiovasc. Res. 116, 1097–1100. doi: 10.1093/cvr/cvaa078
Chen, N., Zhou, M., Dong, X., Qu, J., Gong, F., Han, Y., et al. (2020). Epidemiological and Clinical Characteristics of 99 Cases of 2019 Novel Coronavirus Pneumonia in Wuhan, China: A Descriptive Study. Lancet 395, 507–513. doi: 10.1016/s0140-6736(20)30211-7
Chen, R., Wang, K., Yu, J., Chen, Z., Wen, C., Xu, Z., et al. (2020). The spatial and cell-type distribution of SARS-CoV-2 receptor ACE2 in human and mouse brain. bioRxiv Preprint. doi: 10.1101/2020.04.07.030650
Chen, X., Li, R., Pan, Z., Qian, C., Yang, Y., You, R., et al. (2020). Human monoclonal antibodies block the binding of SARS-CoV-2 spike protein to angiotensin converting enzyme 2 receptor. Cell. Mol. Immunol. 17, 647–649. doi: 10.1038/s41423-020-0426-7
Chen, Y., Guo, Y., Pan, Y., and Zhao, Z. J. (2020). Structure Analysis of the Receptor Binding of 2019-nCov. Biochem. Biophys. Res. Commun. 525, 135–140. doi: 10.1016/j.bbrc.2020.02.071
Cheng, Y., Cheng, G., Chui, C. H., Lau, F. Y., Chan, P. K. S., Ng, M. H. L., et al. (2005a). ABO blood group and susceptibility to severe acute respiratory syndrome. JAMA 293, 1450–1451.
Cheng, Y., Luo, R., Wang, K., Zhang, M., Wang, Z., Dong, L., et al. (2020). Kidney impairment is associated with in-hospital death of COVID-19 patients. medRxiv Preprint. doi: 10.1101/2020.02.18.20023242
Cheng, Y., Wong, R., Soo, Y. O. Y., Wong, W. S., Lee, C. K., Ng, M. H. L., et al. (2005b). Use of Convalescent Plasma Therapy in SARS Patients in Hong Kong. Eur. J. Clin. Microbiol. Infect. Dis. 24, 44–46. doi: 10.1007/s10096-004-1271-9
Chu, C. M., Cheng, V. C. C., Hung, I. F. N., Wong, M. M. L., Chan, K. H., Kao, R. Y. T., et al. (2004). Role of lopinavir/ritonavir in the Treatment of SARS: Initial Virological and Clinical Findings. Thorax 59, 252–256. doi: 10.1136/thorax.2003.012658
Coronaviridae Study Group of the International Committee on Taxonomy of Viruses (2020). The Species Severe Acute Respiratory Syndrome-Related Coronavirus: Classifying 2019-nCoV and Naming It SARS-CoV-2. Nat. Microbiol. 5, 536–544. doi: 10.1038/s41564-020-0695-z
Coutard, B., Valle, C., de Lamballerie, X., Canard, B., Seidah, N. G., and Decroly, E. (2020). The Spike Glycoprotein of the New Coronavirus 2019-nCoV Contains a Furin-Like Clevage Site Absent in Cov of the same clade. Antiviral Res. 176:104742. doi: 10.1016/j.antiviral.2020.104742
Cui, J., Li, F., and Shi, Z.-L. (2019). Origin and Evolution of Pathogenic Coronaviruses. Nat. Rev. Microbiol. 17, 181–192. doi: 10.1038/s41579-018-0118-9
Cunha, L. E. R., Stolet, A. A., Strauch, M. A., Pereira, V. A. R., Dumard, C. H., Gomes, A. M. O., et al. (2020). Potent neutralizing equine antibodies raised against recombinant SARS-CoV-2 spike protein for COVID-19 passive immunization therapy. bioRxiv Preprint. doi: 10.1101/2020.08.17254375
Dai, W., Zhang, B., Jiang, X.-M., Su, H., Li, J., Zhao, Y., et al. (2020). Structure-based Design of Antiviral Candidates Targeting the SARS-CoV-2 Main Protease. Science 368, 1331–1335.
Danser, A. H. J., Epstein, M., and Battle, D. (2020). Renin-Angiotensin System Blockers and the COVID-19 Pandemic: At Present There Is No Evidence to Abandon Renin-Angiotensin System Blockers. Hypertension 75, 1382–1385. doi: 10.1161/hypertensionaha.120.15082
Davies, M., Osborne, V., Lane, S., Roy, D., Dhanda, S., Evans, A., et al. (2020). Remdesivir in Treatment of COVID-19: A Systematic Benefit-Risk Assessment. Drug Saf. 43, 645–656. doi: 10.1007/s40264-020-00952-1
De Castro, L. L., Lopes-Pacheco, M., Weiss, D. J., Cruz, F. F., and Rocco, P. R. M. (2019). Current Understanding of the Immunosuppressive Properties of Mesenchymal Stromal Cells. J. Mol. Med. 97, 605–618. doi: 10.1007/s00109-019-01776-y
De Meyer, S., Bojkova, D., Cinatl, J., Van Damme, E., Buyck, C., Van Loock, M., et al. (2020). Lack of Antiviral Activity of Darunavir Against SARS-CoV-2. Int. J. Infect. Dis. 97, 7–10. doi: 10.1016/j.ijid.2020.05.085
De Wilde, A. H., Jochmans, D., Posthuma, C. C., Zevenhoven-Dobbe, J. C., van Nieuwkoop, S., Bestebroer, T. M., et al. (2014). Screening of an FDA-approved Compound Library Identifies Four Small-Molecules Inhibitors of Middle East Respiratory Syndrome Coronavirus Replication in Cell Cultures. Antimicrob. Agents Chemother. 58, 4875–4884. doi: 10.1128/aac.03011-14
Deftereos, S. P., Giannopoulos, G., Vrachatis, D. A., Siasos, G. D., Giotaki, S. G., Gargalianos, P., et al. (2020). Effect of Colchicine vs Standard Care on Cardiac and Inflammarion Biomarkers and Clinical Outcomes in Patients Hospitalized with Coronavirus Disease 2019: The GRECCO-19 Randomized Clinical Trial. JAMA Netw. Open 3:e2013136. doi: 10.1001/jamanetworkopen.2020.13136
Deng, L., Li, C., Zeng, W., Liu, X., Li, X., Zhang, H., et al. (2020). Arbidol Combined with LPV/r versus LPV/r Alone Agains Corona Virus Disease 2019: A Retrospective Cohort Study. J. Infect. 81, 1–5e.
Devaux, C. A., Rolain, J.-M., and Raoult, D. (2020). ACE2 receptor polymorphism: Susceptibility to SARS-CoV-2, hypertension, multi-organ failure, and COVD-19 disease outcome. J. Microbiol. Immunol. Infect. 53, 425–435. doi: 10.1016/j.jmii.2020.04.015
Diao, B., Wang, C., Wang, R., Feng, Z., Tan, Y., Wang, H., et al. (2020). Human Kidney is a Target for Novel Severe Acute Respiratory Syndrome Coronavirus 2 (SARS-CoV-2) Infection. medRxiv Preprint. doi: 10.1101/2020.03.04.20031120
Duan, K., Liu, B., Li, C., Zhang, H., Yu, T., Qu, J., et al. (2020). Effectiveness of Convalescent Plasma Therapy in Severe COVID-19 Patients. Proc. Natl. Acad. Sci. U S A. 177, 9490–9496.
Dyer, O. (2020). Covid-19: Remdesivir has little or no impact on survival, WHO trial show. BMJ 371:m4057. doi: 10.1136/bmj.m4057
Elhabyan, A., Elyaacoub, S., Sanad, E., Abukhadra, A., Elhabyan, A., and Dinu, V. (2020). The role of host genetics in susceptibility to severe viral infections in human and insights into host genetic of severe COVID-19: A systematic review. Virus Res. 289:198163. doi: 10.1016/j.virusres.2020.198163
Ellinghaus, D., Degenhardt, F., Bujanda, L., Buti, M., Albillos, A., Invernizzi, P., et al. (2020). Gonemowide Association Study of Severe Covid-19 with Respiratory Failure. N. Engl. J. Med. 383, 1522–1534. doi: 10.1056/nejmoa2020283
Fan, C., Li, K., Ding, Y., Lu, W. L., and Wang, J. (2020). ACE2 Expression in Kidney and Testis May Cause Kidney and Testis Damage after 2019-nCov Infection. medRxiv Preprint. doi: 10.1101/2020.02.12.20022418
Fan, Z., Chen, L., Li, J., Cheng, X., Yang, H., Tian, C., et al. (2020). Clinical Features of COVID-19-Related Liver Functional Abnormality. Clin. Gastroenterol. Hepatol. 18, 1561–1566. doi: 10.1016/j.cgh.2020.04.002
Fehr, A. R., and Perlman, S. (2015). Coronaviruses: Na Overview of Their Replication and Pathogenesis. Methods Mol. Biol. 1282, 1–23. doi: 10.1007/978-1-0716-0900-2_1
Forrester, S., Booz, G. W., Sigmund, C. D., Coffman, T. M., Kawai, T., Rizzo, V., et al. (2018). Angiotensin II Signal Transduction: An Update on Mechanisms of Physiology and Pathophysiology. Physiol. Rev. 98, 1627–1738. doi: 10.1152/physrev.00038.2017
Fox, S. E., Akmatbekov, A., Harbert, J. L., Li, G., Brown, Q., and Heide, R. S. V. (2020). Pulmonary and Cardiac Pathology in Covid-19: The First Autopsy Series from New Orleans. medRxiv Preprint. doi: 10.1101/2020.04.06.20050575
Furuta, Y., Komeno, T., and Nakamura, T. (2017). Favipiravir (T-705), a Broad-Spectrum Inhibitor of Viral RNA Polymerase. Proc. Jpn. Acad. Ser. B Phys. Biol. Sci. 93, 449–463. doi: 10.2183/pjab.93.027
Gao, J., Tian, Z., and Yang, X. (2020). Breakthrough: Chloroquine Phosphate Has Shown Apparent Efficacy in Treatment of COVID-19 Associated Pneumonia in Clinical Studies. Biosci. Trends 14, 72–73. doi: 10.5582/bst.2020.01047
Gautret, P., Lagier, J.-C., Parola, P., Hoang, V. T., Meddeb, L., Maihle, M., et al. (2020). Hydroxychloroquine and Azithromycin as a Treatment of COVID-19: Results of an Open-Label Non-Randomized Clinical Trial. Int. J. Antimicrob. Agents 56:105949.
Geleris, J., Sun, Y., Platt, J., Zucker, J., Baldwin, M., Hripcsak, G., et al. (2020). Observational Study of Hydroxychloroquine in Hospitalized Patients with COVID-19. N. Eng. J. Med. 382, 2411–2418.
Gheblawi, M., Wang, K., Viveiros, A., Nguyen, Q., Zhong, J.-C., Turner, A. J., et al. (2020). Angiotensin-Converting Enzyme 2: SARS-CoV-2 Receptor and Regulator of the Renin-Angiotensin System: Celebrating the 20th Anniversary of the Discovery of ACE2. Circ. Res. 126, 1245–1274.
Glowacha, I., Bertran, S., Herzog, P., Pfefferle, S., Steffen, I., Muench, M. O., et al. (2010). Differential Downregulation of ACE2 by the Spike Protein of Severe Acute Respiratory Syndrom Coronavirus and Human Coronavirus NL63. J. Virol. 84, 1198–1205. doi: 10.1128/jvi.01248-09
Gordon, C. J., Tchesnokiv, E. P., Woolner, E., Perry, J. K., Feng, J. Y., Porter, D. P., et al. (2020). Remdesivir Is a Direct-Acting Antiviral That Inhibits RNA-dependent RNA Polymerase From Severe Acute Respiratory Syndrome Coronavirus 2 with High Potency. J. Biol. Chem. 295, 6785–6797. doi: 10.1074/jbc.ra120.013679
Gordon, D. E., Jan, G. M., Bouhaddou, M., Xu, J., Obernier, K., White, K. M., et al. (2020). A SARS-CoV-2 Protein Interaction Map Reveals Targets for Drug Repurposing. Nature 583, 459–468.
Grein, J., Ohmagar, N., Shin, D., Diaz, G., Asperges, E., Castagna, A., et al. (2020). Compassionate Use of Remdesivir for Patients with Severe Covid-19. N. Engl. J. Med. 382, 2327–2336.
Gremese, E., Cingolani, A., Bosello, S. L., Alivernini, S., Tolusso, B., Perniola, S., et al. (2020). Sarilumab use in severe SARS-CoV-2 pneumonia. EClinicalMedicine 27:100553. doi: 10.1016/j.eclinm.2020.100553
Gritti, G., Raimondi, F., Ripamonti, D., Riva, I., Landi, F., Alborghetti, L., et al. (2020). IL-6 signalling pathway inactivation with siltuximab in patients with COVID-19 respiratory failure: an observational cohort study. medRxiv Preprint. doi: 10.1101/2020.04.01.20048561
Guan, W.-J., Ni, Z.-Y., Hu, Y., Liang, W.-H., Ou, C.-Q., He, J.-X., et al. (2020). Clinical Characteristics of Coronavirus Disease 2019 in China. N. Engl. J. Med. 382, 1708–1720.
Gupta, A., Madhavan, M. V., Sehgal, K., Nair, N., Mahajan, S., Sehrawat, T. S., et al. (2020). Extrapulmonary manifestations of COVID-19. Nat. Med. 26, 1017–1032.
Haga, S., Nagata, N., Okamura, T., Yamamoto, N., Sata, T., Yamamoto, N., et al. (2010). TACE Antagonists Blocking ACE2 Shedding Caused by the Spike Protein of SARS-CoV Are Candidate Antiviral Compounds. Antiviral Res. 85, 551–555. doi: 10.1016/j.antiviral.2009.12.001
Hamming, I., Timens, W., Bulthuis, M. L. C., Lely, A. T., Navis, G. J., and van Good, H. (2004). Tissue Distribution of ACE2 Protein, the Functional Receptor for SARS Coronavirus. A First Step in Understandin SARS Pathogenesis. J. Pathol. 203, 631–637. doi: 10.1002/path.1570
Hansen, J., Baum, A., Pascal, K. E., Russo, V., Giordano, S., Wloga, E., et al. (2020). Studies in humanized mice and convalescente humans yield a SARS-CoV-2 antibody cocktail. Science 369, 1010–1014.
Hashimoto, T., Perlot, T., Rehman, A., Trichereau, J., Ishiguro, H., Paolino, M., et al. (2012). ACE2 links amino acid malnutrition to microbial ecology and intestinal inflammation. Nature 487, 477–481. doi: 10.1038/nature11228
He, X., Lau, E. H. Y., Wu, P., Deng, X., Wang, J., Hao, X., et al. (2020). Temporal dynamics in viral shedding and transmissibility of COVID-19. Nat. Med. 26, 672–675. doi: 10.1038/s41591-020-0869-5
Helms, J., Tacquard, C., Severac, F., Leonard-Lorant, I., Ohana, M., et al. (2020). High Risk of Thrombosis in Patients with Severe SARS-CoV-2 Infection: A Multicenter Prospective Cohort Study. Intensive Care Med. 46, 1089–1098. doi: 10.1007/s00134-020-06062-x
Herold, T., Jurinovic, V., Arnreich, C., Lipworth, B. J., Hellmuth, J. C., von Bergwelt-Baildon, M., et al. (2020). Elevated levels of IL-6 and CRP predict the need for mechanical ventilation in COVID-19. J. Allergy Clin. Immunol. 146, 128–136–e4.
Hirsch, J. S., Ng, J. H., Ross, D. W., Sharma, P., Shah, H. H., Barnett, R. L., et al. (2020). Acute kidney injury in patients hospitalized with COVID-19. Kidney Int. 98, 209–218.
Hoffmann, M., Kleine-Weber, H., Schroeder, S., Krüger, N., Herrler, T., Erichsen, S., et al. (2020a). SARS-CoV-2 Cell Entry Dependes on ACE2 and TMPRSS2 and Is Blocked by a Clinically Proven Protease Inhibitor. Cell 181, 271–280. doi: 10.1016/j.cell.2020.02.052
Hoffmann, M., Schroeder, S., Kleine-Weber, H., Müller, M. A., Drosten, C., and Pöhlmann, S. (2020b). Nafamostat Mesylate Blocks Activation of SARS-CoV-2: New Treatment Option for COVID-19 Antimicrob. Agents Chemother. 64, 754–720e.
Holshue, M. L., DeBolt, C., Lindquist, S., Lofy, K. H., Wiesman, J., Bruce, H., et al. (2020). Fisrt Case of 2019 Novel Coronavirus in the United States. N. Eng. J. Med. 382, 929–936.
Horby, P., Lim, W. S., Emberson, J., Mafham, M., Bell, J., Linsell, L., et al. (2020). Dexamethasone in Hospitalized Patients with Covid-19 – Preliminary Report. N. Eng. J. Med. 2020:NEJMoa2021436. doi: 10.1056/NEJMoa2021436
Hou, Y. J., Chiba, S., Halfmann, P., Ehre, C., Kuroda, M., Dinnon, 3rd, et al. (2020). SARS-CoV-2 D614G variant exhibits efficient replication ex vivo and transmission in vivo. Science 370, 1464–1468.
Huang, C., Wang, Y., Li, X., Ren, L., Zhao, J., Hu, Y., et al. (2020). Clinical Features of Patients Infected with 2019 Novel Coronavirus in Wuahn. China. Lancet 395, 497–506.
Huang, Y. H., Jiang, D., and Huang, J. T. (2020). SARS-CoV-2 Detected in Cerebrospinal Fluid by PCR in a Case of COVID-19 Encephalitis. Crain Behav. Immun. 87:149. doi: 10.1016/j.bbi.2020.05.012
Huet, T., Beaussier, H., Voisin, O., Jouveshomme, S., Dauriat, G., Lazareth, I., et al. (2020). Anakinra for severe forms of COVID-19: a cohort study. Lancet Rheum. 2, 393–400e. doi: 10.1016/S2665.9913(20)30164-8
Hussain, M., Jabeen, N., Raza, F., Shabbir, S., Baig, A. A., Amanullah, A., et al. (2020). Structural Variations in Human ACE2 May Influence Its Binding With SARS-CoV-2 Spike Protein. J. Med. Virol. 92, 1580–1586. doi: 10.1002/jmv.25832
Iaccarino, G., Grassi, G., Borghi, C., Ferri, C., Salvetti, M., Volpe, M., et al. (2020). Age and Multimorbidity Predict Death Among COVID-19 Patients: Results of the SARS-RAS Study of the Italian Society of Hypertension. Hypertension 76, 366–372.
Jackson, L. A., Anderson, E. J., Rouphael, N. G., Roberts, P. C., Makhene, M., Coler, R. N., et al. (2020). An mRNA Vaccine against SARS-CoV-2 – Preliminary Report. N. Engl. J. Med. 383, 1920–1931. doi: 10.1056/NEJMoa2022483
Jia, H. P., Look, D. C., Tan, P., Shi, L., Hickey, M., Gakhar, L., et al. (2009). Ectodomain Shedding of Angiotensin Converting Enzyme 2 in Human Airway Epithelia. Am. J. Physiol. Lung Cell. Mol. Physiol. 297, L84–L96.
Jiang, S., Hillyer, C., and Du, L. (2020). Neutralizing Antibodies Against SARS-CoV-2 and Other Human Coronaviruses. Trends Immunol. 41, 355–359. doi: 10.1016/j.it.2020.03.007
Jin, J.-M., Bai, P., He, W., Wu, F., Liu, X.-F., Han, D.-M., et al. (2020). Gender Differences in Patients with COVID-19: Focus on Severity and Mortality. Front. Public Health 8:152. doi: 10.3389/fpubh.2020.00152
Kahn, A., Benthin, C., Zeno, B., Albertson, T. E., Boyd, J., Christie, J. D., et al. (2017). A Pilot Clinical Trial of Recombinant Human Angiotensin-Converting Enzyme 2 in Acute Respiratory Distress Syndrome. Crit. Care 21:234.
Kawase, M., Shirato, K., van der Hoek, L., Taguchi, F., and Matsuyama, S. (2012). Simultaneous Treatment of Human Bronchial Epithelial Cells with Serine and Cysteine Protease Inhibitors Prevents Severe Acute Respiratory Syndrome Coronavirus Entry. J. Virol. 86, 6537–6545. doi: 10.1128/jvi.00094-12
Khera, R., Clark, C., Lu, Y., Guo, Y., Ren, S., Truax, B., et al. (2020). Association of Angiotensin-Converting Enzyme Inhibitors and Angiotensin Receptor Blockers with the Risk of Hospitalization and Death in Hypertensive Patients with Coronavirus Doseiase-19. medRxiv Preprint. doi: 10.1101/2020.05.17.20104943
Khoury, M., Cuenca, J., Cruz, F. F., Figueroa, F. E., Rocco, P. R. M., and Weiss, D. J. (2020). Current status of cell-based therapies for respiratory virus infections: applicability to COVID-19. Eur. Respir. J. 55:2000858. doi: 10.1183/13993003.00858-2020
Kim, D., Lee, J.-Y., Yang, J.-S., Kim, J. W., Kim, V. N., and Chang, H. (2020). The Architecture of SARS-CoV-2 Transcriptome. Cell 181, 914–921.
Kim, U. J., Won, E.-J., Kee, S.-J., Jung, S.-I., and Jang, H.-C. (2016). Combination Therapy with Lopinavir/Ritonavir, Ribavirin and Interferon-α for Middle East Respiratory Syndrome. Antivir. Ther. 21, 455–459. doi: 10.3851/imp3002
King, A., Vail, A., O’Leary, C., Hannan, C., Brough, D., Patel, H., et al. (2020). Anakinra in COVID-19: important considerations for clinical trials. Lancet Rheum. 2, 30160–30160.
Klok, F. A., Kruip, M. J. H. A., van der Meer, N. J. M., Arbous, M. S., Gommers, D. A. M. P. J., Kant, K. M., et al. (2020). Incidence of Thrombotic Complications in Critically Ill ICU Patients with COVID-19. Thromb. Res. 191, 145–147. doi: 10.1016/j.thromres.2020.04.013
Ko, J.-H., Seok, H., Cho, S. Y., Ha, Y. E., Baek, J. Y., Kim, S. H., et al. (2018). Challenges of Convalescent Plasma Infusion Therapy in Middle East Respiratory Coronavirus Infection: A Single Centre Experience. Antivir. Ther. 23, 617–622. doi: 10.3851/imp3243
Kuba, K., Imai, Y., Rao, S., Gao, H., Guo, F., Guan, B., et al. (2005). A Crucial Role of Angiotensins Converting Enzyme 2 (ACE2) in SARS Coronavirus-Induced Lung Injury. Nat. Med. 11, 875–879. doi: 10.1038/nm1267
Lam, T. T.-Y., Jia, N., Zhang, Y.-W., Shum, M. H.-H., Jiang, J.-F., Zhu, H.-C., et al. (2020). Identifying SARS-CoV-2-related Coronaviruses in Malayan Pangolins. Nature 583, 282–285.
Lamers, M. M., Beumer, J., van der Vaart, J., Knoops, K., Puschhof, J., Breugen, T. I., et al. (2020). SARS-CoV-2 productively infects human gut enterocytes. Science 369, 50–54. doi: 10.1126/science.abc1669
Lane, J. C. E., Weaver, J., Kostka, K., Duarte-Salle, T., Abrahao, M. T. F., Alghoul, H., et al. (2020). Safety of hydroxychloroquine, alone and in combination with azithromycin, in light of rapid wide-spread use for COVID-19: a multinational, network cohort and self-controlled case series study. Preprint. doi: 10.1101/2020.04.08.20054551
Lauer, S. A., Grantz, K. H., Bi, Q., Jones, F. K., Zheng, Q., Meredith, H. R., et al. (2020). The Incubation Periodo of Coronavirus Disease 2019 (COVID-19) From Publicly Reported Confirmed Cases: Estimation and Application. Ann. Intern. Med. 172, 577–582. doi: 10.7326/m20-0504
Lax, S. F., Skok, K., Zechner, P., Kessler, H. H., Kaufmann, N., Koelblinger, C., et al. (2020). Pulmonary Arterial Thrombosis in COVID-19 with Fatal Outcome: Results from a Prospective, Single-Center, Clinicopathologic Case Series. Ann. Intern. Med. 173, M20–M2566.
Leng, Z., Zhu, R., Hou, W., Feng, Y., Yang, Y., Han, Q., et al. (2020). Transplantation of ACE2- Mesenchymal Stem Cells Improves the Outcomes of Patients with COVID-19 Pneumonia. Aging Dis. 11, 216–228.
Leung, J. M., Yang, C. X., Tam, A., Shaipanich, T., Hackett, T.-L., Singhera, G. K., et al. (2020). ACE-2 Expression in the Small Airway Epithelial of Smokers and COPD Patients: Implications for COVID-19. Eur. Respir. J. 55:2000688. doi: 10.1183/13993003.00688-2020
Levi, M., Thachil, J., Iba, T., and Levy, J. H. (2020). Coagulation Abnormalities and Thrombosis in Patients with COVID-19. Lancet Haematol. 7, 438–440e.
Li, G., Fan, Y., Lai, Y., Han, T., Li, Z., Zhou, P., et al. (2020). Coronavirus Infections and Immune Responses. J. Med. Virol. 92, 424–432.
Li, H., Chen, C., Hu, F., Wang, J., Zhao, Q., Gale, R. P., et al. (2020a). Impact of Corticosteriod Therapy on Outcomes of Persons with SARS-CoV-2, SARS-CoV, or MERS-CoV Infection: A Systematic Review and Meta-Analysis. Leukemia 34, 1503–1511. doi: 10.1038/s41375-020-0848-3
Li, H., Liu, L., Zhang, D., Xu, J., Dai, H., Tang, N., et al. (2020b). SARS-CoV-2 and Viral Sepsis: Observations and Hypotheses. Lancet 395, 1517–1520. doi: 10.1016/s0140-6736(20)30920-x
Li, Y.-C., Bai, W.-Z., and Hashikawa, T. (2020). The neuroinvasive potential of SARS-CoV2 may play a role in the respiratory failure of COVID-19 patients. J. Med. Virol. 92, 552–555. doi: 10.1002/jmv.25728
Li, Z., Wi, M., Yao, J., Guo, J., Liao, X., Song, S., et al. (2020). Caution on Kidney Dysfunctions of COVID-19 Patients. medRxiv Preprint. doi: 10.1101/2020.02.08.20021212
Ling, T.-Y., Kuo, M.-D., Li, C.-L., Yu, A. L., Huang, Y.-H., Wu, T.-J., et al. (2006). Identification pf Pulmonary Oct-4+ stem-progenitor Cells and Demonstration of Their Susceptibility to SARS Coronavirus (SARS-CoV) Infection in Vitro. Proc. Natl. Acad. Sci. U S A. 103, 9530–9535. doi: 10.1073/pnas.0510232103
Liu, J., Li, S., Liu, J., Liang, B., Wang, X., Wang, H., et al. (2020). Longitudinal characteristics of lymphocyte responses and cytokine profile in the peripheral blood of SARS-CoV-2 infected patients. EBioMedicine 55:102763.
Liu, Y., Du, X., Chen, J., Jin, Y., Peng, L., Wang, H. H. X., et al. (2020a). Neutrophil-to-lymphocyte ratio as an independent risk factor for mortality in hospitalized patients with COVID-19. J. Infect. 81, 6–12e.
Liu, Y., Yan, L.-M., Wan, L., Xiang, T.-X., Le, A., Liu, J.-M., et al. (2020b). Viral Dynamics in Mild and Severe Cases of COVID-19. Lancet Infect. Dis. 20, 656–657. doi: 10.1016/s1473-3099(20)30232-2
Lodigiani, C., Iapichino, G., Carenzo, L., Cecconi, M., Ferrazzi, P., Sebastian, T., et al. (2020). Venous and arterial thromboembolic complications in COVID-19 patients admitted to an academic hospital in Milan. Italy. Thromb. Res. 191, 9–14. doi: 10.1016/j.thromres.2020.04.024
Logunov, D. Y., Dolzhikova, I. V., Zubkova, O. V., Tukhvatullin, A. I., Skcheblyakov, D. V., Dzharullaeva, A. S., et al. (2020). Safety and immunogenicity of ah rAD26 and rAd5 vactor-based heterologous prime-boost COVID-19 vaccine in two formulations: two open, non-randomised phase 1/2 studies from Russia. Lancet 396, 887–897. doi: 10.1016/S0140-6736(20)31866-3
Lopes-Pacheco, M. (2020). CFTR Modulators: The Changing Face of Cystic Fibrosis in the Era of Precision Medicine. Front. Pharmacol. 10:1662. doi: 10.3389/fphar.2019.01662
Lopes-Pacheco, M., Robba, C., Rocco, P. R. M., and Pelosi, P. (2020). Current Understanding of the Therapeutic Benefits of Mesenchymal Stem Cells in Acute Respiratory Distress Syndrome. Cell. Biol. Toxicol. 36, 83–102. doi: 10.1007/s10565-019-09493-5
Mancia, G., Rea, F., Ludergnani, M., Apolone, G., and Corrao, G. (2020). Renin-Angiotensin-Aldosterone System Blockers and the Risk of Covid-19. N. Engl. J. Med. 382, 2431–2440. doi: 10.1056/nejmoa2006923
Mao, L., Wang, M., Chen, S., He, Q., Chang, J., Hong, C., et al. (2020). Neurological Manifestations of Hospitalized Patients with COVID-19 in Wuhan, China: a retrospective case series study. medRxiv Preprint. doi: 10.1101/2020.02.22.20026500
Mao, R., Qiu, Y., Tan, J.-Y., Li, X.-H., Liang, J., Shen, J., et al. (2020). Manifestations and prognosis of gastrointestinal and liver involvement in patients with COVID-19: a systematic review and meta-analysis. Lancet Gastroenterol. Hepatol. 5, 667–678. doi: 10.1016/s2468-1253(20)30126-6
Matsuyama, S., Nao, N., Shirato, K., Kawasa, M., Saito, S., Takayama, I., et al. (2020). Enhanced Isolation of SARS-CoV-2 by TMPRSS2-expressing Cells. Proc. Natl. Acad. Sci. U S A. 117, 7001–7003. doi: 10.1073/pnas.2002589117
Matthay, M. A., Calfee, C. S., Zhuo, H., Thompsom, B. T., Wilson, J. G., Levitt, J. E., et al. (2019). Treatment with Allogeneic Mesenchymal Stromal Cells for Moderate to Severe Acute Respiratory Distress Syndrom (START Study): A Randomised Phase 2a Safety Trial. Lancet Respir. Med. 7, 154–162. doi: 10.1016/s2213-2600(18)30418-1
Matthay, M. A., Pati, S., and Lee, J.-W. (2017). Concise Review: Mesenchymal Stem (Stromal) Cells: Biology and Preclinical Evidence for Therapeutic Potential for Organ Dysfunction Following Trauma os Sepsis. Stem Cells 35, 316–324. doi: 10.1002/stem.2551
Mattos-Silva, P., Felix, N. S., Silva, P. L., Rocca, C., Battaglini, D., Pelosi, P., et al. (2020). Pros and cons of corticosteriod therapy for COVID-19 patients. Respir. Physiol. Neurobiol. 280:103492.
Mauthe, M., Orhon, I., Rocchi, C., Zhou, X., Luhr, X., Luhr, M., et al. (2018). Chloroquine Inhibits Autophagic Flux by Decreasing Autophagosome-Lysosome Fusion. Autophagy 14, 1435–1455. doi: 10.1080/15548627.2018.1474314
McIntyre, L. A., Stewart, D. J., Mei, S. H. J., Courtman, D., Watpool, I., Granton, J., et al. (2018). Cellular Immunotherapy for Septic Shock. A Phase I Clinical Trial. Am. J. Respir. Crit. Care Med. 197, 337–347. doi: 10.1164/rccm.201705-1006oc
Molina, J. M., Celaugerre, C., Goff, J. L., Mela-Lima, B., Ponscarme, D., Goldwirt, L., et al. (2020). No Evidence of Rapid Antiviral Clearance or Clinical Benefit with the Combination of Hydroxychloroquine and Azithromycin in Patients with Severe COVID-19. Med. Mal. Infect. 50:384. doi: 10.1016/j.medmal.2020.03.006
Monteil, V., Kwon, H., Prado, P., Hagelkrüys, A., Wimmer, R. A., Stahl, M., et al. (2020). Inhibition of SARS-CoV-2 Infections in Engineered Human Tissues Using Clinical-Grade Soluble Human ACE2. Cell 181, 905–913. doi: 10.1016/j.cell.2020.04.004
Moriguchi, T., Harii, N., Goto, J., Harada, D., Sugawara, H., Takamino, J., et al. (2020). A first case of meningitis/enchephalitis associated with SARS-Coronavirus-2. Int. J. Infect. Dis. 94, 55–58.
Ni, W., Yang, X., Liu, J., Bao, J., Li, R., Xu, Y., et al. (2020). Acute Myocardical Injury at Hospital Admission Is Associated with All-Cause Mortality in COVID-19. J. Am. Coll. Cardiol. 76, 124–125. doi: 10.1016/j.jacc.2020.05.007
Osborne, V., Davies, M., Lane, S., Evans, A., Denyer, J., Dhanda, S., et al. (2020). Lopanavir-Ritonavir in the Treatment of COVID-19: A Dynamic Systematic Benefit-Risk Assessment. Drug Saf. 43, 809–821. doi: 10.1007/s40264-020-00966-9
Ou, X., Liu, Y., Lei, X., Li, P., Mi, D., Ren, L., et al. (2020). Characterization of Spike Glycoprotein of SARS-CoV-2 on Virus Entry and Its Immune Cross- Reactivity with SARS-CoV. Nat. Commun. 11:1620.
Pan, L., Mu, M., Yang, P., Sun, Y., Wang, R., Yan, J., et al. (2020). Clinical Characteristics of COVID-19 Patients with Digestive Symptoms in Hubei, China: A Descriptive, Cross-Sectional, Multicenter Study. Am. J. Gastroenterol. 115, 766–773. doi: 10.14309/ajg.0000000000000620
Pan, X.-W., Xu, D., Zhang, H., Zhou, W., Wang, L.-H., and Cui, X.-G. (2020). Identification of a Potential Mechanims of Acute Kidney Injury During the COVID-19 Outbreak: A Study Based on Single-Cell Transcriptome Analysis. Intesive Care Med. 46, 1114–1116. doi: 10.1007/s00134-020-06026-1
Pécheur, E.-I., Borisevich, V., Halfmann, P., Morrey, J. D., Smee, D. F., Prichard, M., et al. (2016). The Synthetic Antiviral Drug Arbidol Inhibits Globally Prevalent Pathogenic Viruses. J. Virol. 90, 3086–3092. doi: 10.1128/jvi.02077-15
Polack, F. P., Thomas, S. J., Kitchin, N., Absalon, J., Gurtman, A., Locklart, S., et al. (2020). Safety and Efficacy of the BNT162b2 mRNA Covid-19 Vaccine. N. Engl. J. Med. 383:2034577. doi: 10.1056/NEJMoa2034577
Pushpakom, S., Iorio, F., Eyers, P. A., Escott, K. J., Hopper, S., Wells, A., et al. (2019). Drug Repurposing: Progress, Challenges and Recommendations. Nat. Rev. Drug Discov. 18, 41–58. doi: 10.1038/nrd.2018.168
Quartuccio, L., Sonaglia, A., Pecori, D., Peghin, M., Fabris, M., Tascini, C., et al. (2020). Higher levels of IL-6 early after toxilizumab distinguish survivors from nonsurvivors in COVID-19 pneumonia: A possible indication for deeper targeting of IL-6. J. Med. Virol. 92, 2852–2856. doi: 10.1002/jmv.26149
Ramiro, S., Mostard, R. L. M., Magro-Checa, C., van Dongen, C. M. P., Dormans, T., Buijs, J., et al. (2020). Historically controlled comparison of glucocorticoids with and without tocilizumab versus supportive care only in patients with COIVD-19-associated cytokine storm syndrome: results of the CHIC study. Ann. Rheum. Dis. 79, 1143–1151. doi: 10.1136/annrheumdis-2020-218479
Rawat, K., Kumari, P., and Saha, L. (2020). COVID-19 vaccine: A recent update in pipeline vaccines, their design and development strategies. Eur. J. Pharmacol. 892:173751. doi: 10.1016/j.ejphar.2020.173751
Reynolds, H. R., Adhihari, S., Pulgarin, C., Troxel, A. B., Iturrate, E., Johnson, S. B., et al. (2020). Renin-Angiotensin-Aldosterone System Inhibitos and Risk of Covid-19. N. Engl. J. Med. 382, 2441–2448.
Robba, C., Battaglini, D., Ball, L., Patroniti, N., Loconte, M., Brunetti, I., et al. (2020a). Distinct Phenotypes Require Distinct Respiratory Management Strategies in Severe COVID-19. Respir. Physiol. Neurobiol. 279:103455. doi: 10.1016/j.resp.2020.103455
Robba, C., Battaglini, D., Pelosi, P., and Rocco, P. R. M. (2020b). Multiple organ dysfunction in SARS-CoV-2: MODS-CoV-2. Expert Rev. Respir. Med. 14, 865–868. doi: 10.1080/17476348.2020.1778470
Rocco, P. R., Silva, P. L., Cruz, F. F., Junior, M. A. C. M., Tierno, P. F. G. M. M., Moura, M. A., et al. (2020). Early use of nitazoxanide in mild Covid-19 disease: randomised, placebo-controlled trial. Eur. Respir. J. 2020:2003725. doi: 10.1183/13993003.03725-2020
Ruan, Q., Yang, K., Wang, W., Jiang, L., and Song, J. (2020). Clinical Predictors of Mortality Due to COVID-19 Based on an Analysis of Data of 150 Patients From Wuhan, China. Intensive Care Med. 46, 846–848. doi: 10.1007/s00134-020-05991-x
Russo, R., Andolfo, I., Lasorca, V. A., Iolascon, A., and Capasso, M. (2020). Genetic Analysis of the Coronavirus SARS-CoV-2 Host Protease TMPRSS2 in Different Populations. Front. Genet. 11:872. doi: 10.3389/fgene.2020.00872
Sakurai, A., Sasaki, T., Kato, S., Hayashi, M., Tsuzuki, S.-I., Ishihara, T., et al. (2020). Natural History of Asymptomatic SARS-CoV.2 Infection. N. Engl. J. Med. 383, 885–886.
Salazar, E., Perez, K. K., Ashraf, M., Chen, J., Castillo, B., Christensen, P. A., et al. (2020). Treatment of Coronavirus Disease 2019 (COVID-19) Patients with Convalescent Plasma. Am. J. Pathol. 190, 1680–1690.
Sama, I. E., Ravera, A., Santema, B. T., van Goor, H., Ter Maaten, J. M., Cleland, J. G. F., et al. (2020). Circulating Plasma Concentrations of Angiotensin-Converting Enzyme 2 in Men and Women with Heart Failure and Effects of Renin-Angiotensin-Aldosterone Inhibitors. Eur. Heart J. 41, 1810–1817.
Samudrala, P. K., Kumar, P., Choudhary, K., Thakur, N., Wadekar, G. S., Dayaramani, R., et al. (2020). Virology, pathogenesis, diagnosis and in-line treatment of COVID-19. Eur. J. Pharmacol. 883:173375. doi: 10.1016/j.ejphar.2020.173375
Santos, R. A. S., Sampaio, W. O., Alzamora, A. C., Motta-Santos, D., Alenina, N., Bader, M., et al. (2018). The ACE2/Angiotension-(1-7)/MAS Axis of the Renin-Angiotensin-System: Focus on Angiotensin-(1-7). Physiol. Rev. 98, 505–553. doi: 10.1152/physrev.00023.2016
Sciascia, S., Aprà, F., Baffa, A., Baldovino, S., Boaro, D., Boero, R., et al. (2020). Pilot Prospective Open, Single-Arm Multicentre Study on Off-Label Use of Tocilizumab in Patients with Severe COVID-19. Clin. Exp. Rheumatol. 38, 529–532.
Sengupta, V., Sengupta, S., Lazo, A., Woods, P., Nolan, A., and Bremer, N. (2020). Exosomes Derived From Bone Marrow Mesenchymal Stem Cells as Treatment for Severe COVID-19. Stem Cells Dev. 29, 747–754. doi: 10.1089/scd.2020.0080
Shah, P. P., Wang, T., Kaletsky, R. L., Myers, M. C., Purvir, J. E., Jing, H., et al. (2010). A Small-Molecule Oxocarbazate Inhibitor of Human Cathepsin L Blocks Severe Acute Respiratory Syndrome and Ebola Pseudotype Virus Infection Into Human Embryonic Kidney 293T Cells. Mol. Pharmacol. 78, 319–324. doi: 10.1124/mol.110.064261
Sharif-Askari, N. D., Sharif-Askari, F. S., Alabed, M., Temsah, M.-H., Heialy, S. A., Hamid, Q., et al. (2020). Airways Expression of SARS-CoV-2 Receptor, ACE2, and TMPRSS2 is Lower in Children than Adults and Increases with Smoking and COPD. Mol. Ther. Methods Clin. Dev. 18, 1–6. doi: 10.1016/j.omtm.2020.05.013
Shastri, A., Wheat, J., Agrawal, S., Chaterjee, N., Pradhan, K., Goldfinger, M., et al. (2020). Delayed clearance of SARS-CoV2 in male compared to female patients: High ACE2 expression in testes suggests possible existence of gender-specific viral reservoirs. medRxiv Preprint. doi: 10.1101/2020.04.16.20060566
Sheahan, T. P., Sims, A. C., Leist, S. R., Schäfer, A., Won, J., Brown, A. J., et al. (2020). Comparative Therapeutic Efficact of Remdesivir and Combination Lopinavir, Ritonavir, and Interferon Beta Against MERS-CoV. Nat. Commun. 11:222.
Shen, C., Wang, Z., Zhao, F., Yang, Y., Li, Z., Yuan, J., et al. (2020). Treatment of 5 Critically Ill Patients with COVID-19 with Convalescent Plasma. JAMA 323, 1582–1589.
Shi, S., Qin, M., Shen, B., Cai, Y., Liu, T., Yang, F., et al. (2020). Association of Cardiac Injury with Mortality in Hospitalized Patients with COVID-19 in Wuhan, China. JAMA Cardiol. 5, 802–810. doi: 10.1001/jamacardio.2020.0950
Silva, J. D., de Castro, L. L., Braga, C. L., Oliveira, G. P., Trivelin, A. S., Barbosa-Junior, C. M., et al. (2019). Mesenchymal Stromal Cells Are More Effective Than Theirs Extracellular Vesicles at Reducing Lung Injury of Acute Respiratory Distress Syndrome Etiology. Stem Cells Int. 2019:8262849.
Silva, J. D., Lopes-Pacheco, M., Paz, A. H. R., Cruz, F. F., Melo, E. B., de Oliveira, M. V., et al. (2018). Mesenchymal Stem Cells From Bone Marrow, Adipose Tissue, and Lung Tissue Differentially Mitigate Lung and Distal Organ Damage in Experimental Acute Respiratory Distress Syndrome. Crit. Care Med. 46, 132–140e.
Siu, K.-L., Yuen, K.-S., Castaño-Rodriguez, C., Ye Zi-Wei, Yeung, M.-L., et al. (2019). Severe Acute Respiratory Syndrome Coronavirus ORF3a Protein Activates the NLRP3 Inflammasome by Promoting TRAF3-dependent Ubiquitination of ASC. FASEB J. 33, 8865–8877. doi: 10.1096/fj.201802418r
Solomon, I. H., Normandin, E., Bhattacharyya, S., Mukerji, S., Keller, K., Ali, A. S., et al. (2020). Neuropathological Features of Covid-19. N. Eng. J. Med. 383, 989–992.
Su, H., Yang, M., Wan, G., Yi, L.-X., Tang, F., Zhu, H.-Y., et al. (2020). Renal Histopathological Analysis of 26 Postmortem Findings of Patients with COVID-19 in China. Kidney Int. 98, 219–227. doi: 10.1016/j.kint.2020.04.003
Sungnak, W., Huang, N., Bécavin, C., Berg, M., Queen, R., Litvinukova, M., et al. (2020). SARS-CoV-2 Entry Factors Are Highly Expressed in Nasal Epithelial Cells Together with Innate Immune Genes. Nat. Med. 26, 681–687. doi: 10.1038/s41591-020-0868-6
Takahashi, T., Wong, P., Ellingson, M., Lucas, C., Klein, J., Israelow, B., et al. (2020). Sex differences in immune responses to SARS-CoV-2 that underlie disease outcomes. medRxiv Preprint. doi: 10.1101/2020.06.06.20123414
Taneja, V. (2018). Sex Hormones Determine Immune Response. Front. Immunol. 9:1931. doi: 10.3389/fimmu.2018.01931
Tang, N., Bai, H., Chen, X., Gong, J., Li, D., and Sun, Z. (2020). Anticoagulant Treatment Is Associated with Decreased Mortality in Severe Coronavirus Disease 2019 Patients with Coagulopathy. J. Thromb. Harmost. 18, 1095–1099.
Thachil, J., Tang, N., Gando, S., Falanga, A., Cattaneo, M., Levi, M., et al. (2020). ISTH Interim Guidance on Recognition and Management of Coagulopathy in COVID.19. J. Thromb. Haemost. 18, 1023–1026. doi: 10.1111/jth.14810
Tian, S., Xiong, Y., Liu, H., Niu, L., Guo, J., Miao, M., et al. (2020). Pathological Study of the 2019 Novel Coronavirus Disease (COVID-19) Through Postmortem Core Biopsies. Mol. Pathol. 33, 1007–1014. doi: 10.1038/s41379-020-0536-x
Tian, X., Li, C., Huang, A., Xia, S., Lu, S., Shi, Z., et al. (2020). Potent Binding of 2019 Novel Coronavirus Spike Protein by a SARS Coronavirus-Specific Human Monoclonal Antibody. Emerg. Microbes Infect. 9, 382–385. doi: 10.1080/22221751.2020.1729069
Toniati, P., Piva, S., Cattalini, M., Garrafa, E., Regola, F., Castelli, F., et al. (2020). Toxilizumab for the treatment of severe COVID-19 pneumonia with hyperinflammatory syndrome and acute respiratory failure: A single center study of 100 patients in Brescia, Italy. Autoimmun. Rev. 19:102568.
Uhal, B. D., Li, X., Xue, A., Gao, X., and Abdul-Hafez, A. (2011). Regulation of Alveolar Epithelial Cell Survival by the ACE-2/angiotensin1-7/Mas Axis. Am. J. Physiol. Lung Cell. Mol. Physiol. 301, L269–L274.
Úri, K., Fagyas, M., Kertész, Borbély, A., Jenei, C., Bene, O., et al. (2016). Circulating ACE2 Activity Correlates with Cardiovascular Disease Development. J. Renin Angiotensin Aldosterone Syst. 17:1470320316668435.
Van Doremalen, N., Lambe, T., Spencer, A., Belij-Rammerstorfer, S., Purushotham, J. N., Port, J. R., et al. (2020). ChAdOx1 nCov-19 vaccination prevents SARS-CoV-2 pneumonia in rhesus macaques. bioRxiv Preprint. doi: 10.1101/2020.05.13.093195
Varga, Z., Flammer, A. J., Steiger, P., Haberecker, M., Andermatt, R., Zinkernagel, A. S., et al. (2020). Endothelial Cell Infection and Endotheliitis in COVID-19. Lancet 395, 1417–1418.
Veras, F. P., Pontelli, M., Silva, C., Toller-Kawahisa, J., de Lima, M., Nascimento, D., et al. (2020). SARS-CoV-2 triggered neutrophil extracelular traps (NETs) mediated COVID-19 pathology. medRxiv Preprint. doi: 10.1101/2020.06.08.20125823
Von Weyhern, C. H., Kaufmann, I., Neff, F., and Kremer, M. (2020). Early evidence of pronounced brain involvement in fatal COVID-19 outcomes. Lancet 395:e109. doi: 10.1016/s0140-6736(20)31282-4
Voysey, M., Clemens, S. A. C., Madhi, S. A., Weckx, L. Y., Folegatti, P. M., Aley, P. K., et al. (2020). Safety and efficacy of ChAdOx1 nCov-19 vaccine (AZD1222) against SARS-CoV-2: an interim analysis of four randomized controlled trials in Brazil, South Africa, and the UK. Lancet 397, 99–111. doi: 10.1016/S0140-3736(20)32661-1
Walls, A. C., Park, Y.-J., Tortorici, M. A., Wall, A., McGuire, A. T., and Veesler, D. (2020). Structure, Function and Antigenicity of the SARS-CoV-2 Spike Glycoprotein. Cell 181, 281–292. doi: 10.1016/j.cell.2020.02.058
Wan, Y., Shang, J., Graham, R., Baric, R. S., and Li, F. (2020). Receptor Recognition by the Novel Coronavirus From Wuhan: An Analysis Based on Decade-Long Structural Studies of SARS Coronavirus. J. Virol. 94, 127–120e.
Wang, C., Horby, P. W., Hayden, F. G., and Gao, G. F. (2020). A Novel Coronavirus Outbreak of Global Health Concern. Lancet 395, 470–473. doi: 10.1016/s0140-6736(20)30185-9
Wang, D., Hu, B., Hu, C., Zhu, F., Liu, X., Zhang, J., et al. (2020). Clinical Characteristics of 138 Hospitalized Patients with 2019 Novel Coronavirus-Infected Pneumonia in Wuhan. China. JAMA 323, 1061–1069. doi: 10.1001/jama.2020.1585
Wang, K., Gheblawi, M., and Oudit, G. Y. (2020). Angiotensin Converting Enzyme 2: A Double-Edged Sword. Circulation 142, 426–428. doi: 10.1161/CIRCULATIONAHA.120.047049
Wang, M., Cao, R., Zhang, L., Yang, X., Liu, J., Xu, M., et al. (2020). Remdesivir and Chloroquine Effectively Inhibit the Recently Emerged Novel Coronavirus (2019-nCoV) in Vitro. Cell Res. 30, 269–271. doi: 10.1038/s41422-020-0282-0
Wang, S., Guo, F., Liu, K., Wang, H., Rao, S., Yang, P., et al. (2008). Endocytosis of the Receptor-Binding Domain of SARS-CoV Spike Protein Together with Virus Receptor ACE2. Virus Res. 136, 8–15. doi: 10.1016/j.virusres.2008.03.004
Wang, W., Patel, V. B., Parajuli, N., Fan, D., Basu, R., Wang, Z., et al. (2014). Heterozygote loss of ACE2 is sufficient to increae the susceptibility to heart disease. J. Mol. Med. 92, 847–858. doi: 10.1007/s00109-014-1149-y
Wang, Y., Jiang, W., He, Q., Wang, C., Wang, B., Zhou, P., et al. (2020a). A retrospective cohort study of methylprednisolone therapt in severe patients with COVID-19 pneumonia. Signal Transduct. Target Ther. 5:57.
Wang, Y., Zhang, D., Du, G., Du, R., Zhao, J., Jin, Y., et al. (2020b). Remdesivir in Adults with Severe COVID-19: A Randomised, Double-Blind, Placebo-Controlled, Multicentre Trial. Lancet 395, 1569–1579.
Wang, Z., and Xu, X. (2020). scRNA-seq Profiling of Human Testes Reveals the Presence of ACE2 Receptor, A Target for SARS-CoV-2 Infection in Spermatogonia, Leydig and Sertoli Cells. Cells 9:920. doi: 10.3390/cells9040920
Wang, Z., Yang, B., Li, Q., Wen, L., and Zhang, R. (2020). Clinical Features of 69 Cases with Coronavirus Disease 2019 in Wuhan, China. Clin. Infect. Dis. 71, 769–777. doi: 10.1096/cid/ciaa272
Williamson, E., Walker, A. J., Bhaskaran, K., Bacon, S., Bates, C., Morton, C. E., et al. (2020). OpenSAFELY: factors associated with COVID-19 death in 17 million patients. Nature 584, 430–436. doi: 10.1038/s41586-020-2521-4
Wölfel, R., Corman, V. M., Guggemos, W., Seilmaier, M., Zange, S., Müller, M. A., et al. (2020). Virological assessment of hospitalized patients with COVID-19. Nature 581, 465–469.
Wong, H. K., Lee, C. K., Hung, I. F. N., Leung, J. N. S., Hong, J., Yuen, K. Y., et al. (2010). Practical limitations of Convalescent Plasma Collection: A Case Scenation in Pandemic Preparation for Influenza A (H1N1) Infection. Transfusion 50, 1967–1971. doi: 10.1111/j.1537-2995.2010.02651.x
Wrapp, D., Wang, N., Corbett, K., Goldsmith, J. A., Hsieh, C.-L., Abiona, O., et al. (2020). Cryo-EM Structure of the 2019-nCoV Spike in the Prefusion Conformation. Science 367, 1260–1263.
Wu, A., Peng, Y., Huang, B., Ding, X., Wang, X., Niu, P., et al. (2020). Genome Composition and Divergence of the Novel Coronavirus (2019-nCoV) Originating in China. Cell Host Microbe 27, 325–328. doi: 10.1016/j.chom.2020.02.001
Wu, D., and Yang, X. O. (2020). Th17 Responses in Cytokine Storm of COVID-19: An Emerging Target of JAK2 Inhibitor Fedratinib. J. Microbiol. Immunol. Infect. 53, 368–370. doi: 10.1016/j.jmii.2020.03.005
Wu, P., Duan, F., Luo, C., Liu, Q., Qu, X., Liang, L., et al. (2020). Characteristics of Ocular Findings of Patients with Coronavirus Disease 2019 (COVID-19) in Hubei Province, China. JAMA Ophthalmol. 138, 575–578. doi: 10.1001/jamaophthalmol.2020.1291
Wu, Z., and McGoogan, J. M. (2020). Characteristics of and Important Lessons From the Coronavirus Disease 2019 (COVID-19) Outbreak in China: Summary of a Report of 72314 Cases From Chinese Center for Disease Control and Prevention. JAMA 323, 1239–1242. doi: 10.1001/jama.2020.2648
Xiao, F., Tang, M., Zheng, X., Liu, Y., Li, X., and Shan, H. (2020). Evidence for Gastrointestinal Infection of SARS-CoV-2. Gastroenterology 158, 1831–1833. doi: 10.1053/j.gastro.2020.02.055
Xu, J., Sriramula, S., Xia, H., Moreno-Walton, L., Culicchia, F., Domenig, O., et al. (2017). Clinical Relevance and Role of Neuronal AT1 Receptors in ADAM17-Mediated ACE2 Shedding in Neurogenic Hypertension. Circ. Res. 121, 43–55.
Xu, X., Han, M., Li, Y., Sun, W., Wang, D., Fu, B., et al. (2020). Effective Treatment of Severe COVID-19 Patients with Tocilizumab. Proc. Natl. Acad. Sci. U S A. 117, 10970–10975.
Xu, Y., Sriramula, S., Xia, H., Moreno-Walton, L., Culicchia, F., Domening, O., et al. (2017). Clinical Relevance and Role of Neuronal AT1 Receptor in ADAM17-Mediated ACE2 Shedding in Neurogenic Hypertension. Circ. Res. 121, 43–55. doi: 10.1161/circresaha.116.310509
Xu, Z., Shi, L., Wang, Y., Zhang, J., Huang, L., Zhang, C., et al. (2020). Pathological Findings of COVID-19 Associated with Acute Respiratory Distress Syndrome. Lancet Respir. Med. 8, 420–422. doi: 10.1016/s2213-2600(20)30076-x
Yamamoto, M., Kiso, M., Sakai-Tagawa, Y., Iwatsuki-Horimoto, K., Imai, M., Takeda, M., et al. (2020). The anticoagulante nafamostat potently inhibits SARS-CoV-2 infection in vitro: an existing drug with multiple possible therapeutic effects. bioRxiv Preprint. doi: 10.1101/2020.04.22.054981
Yamamoto, M., Matsuyama, S., Li, X., Takeda, M., Kawaguchi, Y., Inoue, J.-I., et al. (2016). Identification of Nafamostat as a Potent Inhibitor of Middle East Respiratory Syndrome Coronavirus S Protein-Mediated Membrane Fusion Using the Split-Protein-Based Cell-Cell Fusion Assay. Antimicrob. Agents Chemother. 60, 6532–6539. doi: 10.1128/aac.01043-16
Ye, M., Fu, D., Ren, Y., Wang, F., Wang, D., Zhang, F., et al. (2020). Treatment with convalescent plasma for COVID-19 patients in Wuhan, China. J. Med. Virol. 92, 1890–1901. doi: 10.1002/jmv.25882
Zhang, B., Liu, S., Tan, T., Huang, W., Dong, Y., Chen, L., et al. (2020a). Treatment with Convalescent Plasma for Critically Ill Patients with Severe Acute Respiratory Syndrome Coronavirus 2 Infection. Chest 158, 9–13e.
Zhang, B., Zhou, X., Qiu, Y., Feng, F., Feng, J., Jia, Y., et al. (2020b). Clinical characteristics of 82 death cases with COVID-19. medRxiv Preprint. doi: 10.1101/2020.02.26.20028191
Zhang, C., Shi, L., and Wang, F.-S. (2020a). Liver Injury in COVID-19: Management and Challenges. Lancet Gastroenterol. Hepatol. 5:430.
Zhang, C., Wu, Z., Li, J.-W., Zhao, H., and Wang, G.-Q. (2020b). Cytokine Release Syndrome in Severe COVID-19: Interleukin-6 Receptor Antagonist Tocilizumab May Be the Key to Reduce Mortality. Int. J. Antimicrob. Agents 55:105954. doi: 10.1016/j.ijantimicag.2020.105954
Zhang, H., Zhou, P., Wei, Y., Yue, H., Wang, Y., Hu, M., et al. (2020). Histopathologic Changes and SARS-CoV-2 Immunostaining in the Lung of a Patients with COVID-19. Ann. Intern. Med. 172, 629–632. doi: 10.7326/m20-0533
Zhang, P., Zhu, L., Cai, J., Lei, F., Qin, J.-J., Xie, J., et al. (2020). Association of Inpatient Use of Angiotensin-Converting Enzyme Inhibitors and Angiotensin II Receptor Blockers with Mortality Among Patients with Hypertension Hospitalized with COVID-19. Circ. Res. 126, 1671–1681.
Zhang, T., Wu, Q., and Zhang, Z. (2020). Probable Pangolin Origin of SARS-CoV-2 Associated with the COVID-19 Outbreak. Corr. Biol. 30, 1346–1351. doi: 10.1016/j.cub.2020.03.022
Zhang, W., Du, R.-H., Li, B., Zheng, X.-S., Yang, X.-L., Hu, B., et al. (2020). Molecular and serological investigation of 2019-nCoV infected patients: implication of multiple shedding routes. Emerg. Microbes Infect. 9, 386–389.
Zhang, Y., Zheng, G., Pan, H., Li, C., Hu, Y., Chu, K., et al. (2020). Safety, tolerability, and immunogenicity of an inactivated SARS-CoV-2 vaccine in healthy adults aged 18-59 years: a randomized, double-blind, placebo- controlled, phase 1/2 clinical trial. Lancet Infect. Dis. 2020:4. doi: 10.1016/S1473-3099(20)30843-4
Zhao, J., Yang, Y., Huang, H., Li, D., Gu, D., Li, X., et al. (2020). Relationship between the ABO blood groups and the COVID-19 susceptibility. Preprint doi: 10.1101/2020.03.11.20031096
Zhao, X., Nichills, J. M., and Chen, Y.-G. (2008). Severe acute respiratory syndrom-associated coronavirus nucleocapsid protein interacts with Smad3 and modulates transforming growth factor-beta signaling. J. Biol. Chem. 283, 3272–3280.
Zhao, Y., Zhao, Z., Wang, Y., Zhou, Y., Ma, Y., and Zuo, W. (2020). Single-cell RNA expression profiling of ACE2, the receptor of SARS-CoV-2. Preprint doi: 10.1101/2020.01.26.919985
Zhao, Z., Zhang, F., Xu, M., Huang, K., Zhong, W., Cai, W., et al. (2003). Description and clinical treatment of an early outbreak of severe acute respiratory syndrome (SARS) in Guangzhou. PR China. J. Med. Microbiol. 52, 715–720.
Zhou, F., Yu, T., Fan, G., Liu, Y., Liu, Z., Xiang, J., et al. (2020). Clinical course and risk factor for mortality of adult inpatients with COVID-19 in Wuhan, China: a retrospective cohort study. Lancet 395, 1054–1062.
Zhou, P., Yang, X.-L., Wang, X.-G., Hu, B., Zhang, L., Zhang, W., et al. (2020). A pneumonia outbreak associated with a new coronavirus of probable bat origin. Nature 579, 270–273.
Zhou, Y., Fu, B., Zheng, X., Wang, D., Zhao, C., Qi, Y., et al. (2020). Pathogenic T cells and inflammatory monocytes incite inflammatory storm in severe COVID-19 patients. Natl. Sci. Rev. nwaa041. doi: 10.1093/nsr/nwaa041
Zhou, Y., Vedantham, P., Kai, L., Agudelo, J., Carrion, R. Jr., Nunneley, J. W., et al. (2015). Protease inhibitors targeting coronavirus and filovirus entry. Antiviral Res. 116, 76–84.
Zietz, M., and Tatonetti, N. P. (2020). Testing the association between blood type and COVID-19 infection, intubation and death. Preprint doi: 10.1101/2020.04.08.20058073
Keywords: ACE2, coronavirus, lung, multiple organ dysfunction, pathophysiology, SARS-CoV-2, therapy, viral infection
Citation: Lopes-Pacheco M, Silva PL, Cruz FF, Battaglini D, Robba C, Pelosi P, Morales MM, Caruso Neves C and Rocco PRM (2021) Pathogenesis of Multiple Organ Injury in COVID-19 and Potential Therapeutic Strategies. Front. Physiol. 12:593223. doi: 10.3389/fphys.2021.593223
Received: 11 August 2020; Accepted: 08 January 2021;
Published: 28 January 2021.
Edited by:
Ravi Nistala, University of Missouri, United StatesReviewed by:
Massimo Volpe, Sapienza University of Rome, ItalySavneet Kaur, The Institute of Liver and Biliary Sciences (ILBS), India
Copyright © 2021 Lopes-Pacheco, Silva, Cruz, Battaglini, Robba, Pelosi, Morales, Caruso Neves and Rocco. This is an open-access article distributed under the terms of the Creative Commons Attribution License (CC BY). The use, distribution or reproduction in other forums is permitted, provided the original author(s) and the copyright owner(s) are credited and that the original publication in this journal is cited, in accordance with accepted academic practice. No use, distribution or reproduction is permitted which does not comply with these terms.
*Correspondence: Miquéias Lopes-Pacheco, mlopes0811@gmail.com; Patricia Rieken Macedo Rocco, prmrocco@gmail.com