- 1Université Paris 1 Panthéon-Sorbonne, Paris, France
- 2Laboratoire de Géographie Physique, UMR 8591 CNRS, Meudon, France
- 3Institut Universitaire de France, Paris, France
- 4Department of Geography, University of Cambridge, Cambridge, United Kingdom
- 5CNRS, UMR 8215 Trajectoires, Paris, France
- 6Ministry of Land and Natural Resources, Natural Resources Division, Nuku’alofa, Tonga
- 7Université Clermont Auvergne, CNRS, IRD, OPGC, Laboratoire Magmas et Volcans, Clermont-Ferrand, France
- 8Faculty of Geography, Universitas Gadjah Mada, Yogyakarta, Indonesia
- 9Geocare and Petroleum Consult Ltd., Nuku’alofa, Tonga
- 10Laboratoire d’analyse des sols EOST, Université de Strasbourg, Strasbourg, France
- 11Laboratory of Sediment Hazards and Disaster Risk, Kobe University, Kobe, Japan
The pre-colonial history (i.e. before the 16th century) of Tonga and West Polynesia still suffers from major gaps despite significant scientific advances in recent years, particularly in the field of archaeology. By the 14th century, the powerful Tu’i Tonga kingdom united the islands of the Tongan archipelago under a centralised authority and, according to tradition, extended its influence to neighbouring island groups in the Central Pacific. However, some periods of deep crisis were identified, e.g. in the mid- 15th century, marked by an abrupt cessation of inter-archipelago migration on the deep seas in the Pacific, significant cultural changes, and a decrease in accessible natural resources. The origins of these disturbances are still debated, and they are usually assigned to internal political problems or loss of external influence vis-à-vis neighboring chiefdoms. However, the hypothesis of a major natural disaster was rarely suggested up to now, while field evidence points to the occurrence of a very large tsunami in the past, including the presence of numerous megablocks that were deposited by a “red wave” (or peau kula, which also mean tsunami in the Tongan language) according to a local myth. Drawing on a body of new evidence from sedimentary signatures and radiocarbon dating of charcoal and marine bioclasts, geomorphology, and sedimentology, in support of previously published archaeological data, we argue that a large tsunami inundated large areas of Tongatapu island in the mid-15th century with runup heights up to 30 m, and that the Tu’i Tonga kingdom was severely impacted by this event. We also discuss the likely sources of this tsunami.
1 Introduction
Located in the South Pacific Ocean, the archipelago of Tonga has almost 170 islands, 36 of them inhabited, and divided into three main groups, namely Vava’u, Ha’apai, and Tongatapu archipelagos (Figure 1). The pre-colonial history of Tonga and West Polynesia still suffers from major gaps despite significant scientific advances in recent years, particularly in the field of archaeology. By the 13th century, the Tongan kings named the Tu’i Tonga ruled several nations over the Southwest and South Central Pacific for more than 300 years, sparking historians to refer to a “Tongan Maritime Empire” (Clark et al., 2014). By the 14th century, a powerful chiefdom united the islands of Tonga under a centralised authority and, according to tradition, extended its influence to neighbouring island groups in the Central Pacific (Dickinson et al., 1999; Barnes and Hunt, 2005; Clark and Reepmeyer, 2014; Cochrane and Rieth, 2016; Burley and Addison, 2018). However, some periods of deep crisis were identified, especially in the middle of the 15th century, marked by an abrupt cessation of inter-archipelago migration on the deep seas in the Pacific (Goff and Nunn, 2016) and significant cultural changes. The origins of these disturbances are still debated, e.g. internal political problems (Burley, 1998), or loss of external influence vis-à-vis neighboring states such as Samoa. While the hypothesis of a major natural disaster was never suggested, a local legend in Tonga refers to a gigantic peau kula or “red wave” (which is also the Tongan word for tsunami) that covered the whole island of Tongatapu in the Past (Morton, 2003).
Based upon a combination of palaeotsunami and archaeological data out of Tonga, several scholars have already evidenced the occurrence of a “region-wide single, high-energy coastal event” over the southwest Pacific Ocean around the 15th Century (Goff et al., 2011a). In New Zealand, a minimum runup of 11–15 m a.s.l. was estimated at the Kapiti Island, near Wellington, and a maximum of 65 m a.s.l. along Ngararahae Bay on the west coast of the North Island (Goff and Chagué-Goff, 2015). This widespread tsunami strongly affected the prehistoric Maori settlements in this country (McFadgen, 2007; Goff et al., 2012), where they were installed since the end of the 13th Century after a mass migration event (Walter et al., 2017). In the Great Barrier Island and several other sites, archaeological records and Maori oral traditions (pūrākau: King and Goff, 2010) show a significant break in the occupation, either temporary or permanent, around the mid-15th century CE (Goff and McFadgen, 2001).
Evidence of a large-scale tsunami was also reported from the east coast of Australia, where several coastal midden sites reworked by seawater in the Sydney area also date back to the mid-15th century (Bryant et al., 1992; Nott, 1997). Further North, sedimentary evidence in coastal sediments indicates significant tsunami inland inundation and runup all around the island of Futuna, dated ca. 1,450 to 1500 CE (Goff et al., 2011a). Other unequivocal sedimentary evidence of a similar event is found in Rurutu (Austral Islands: Bollt, 2008). This region-wide tsunami might have highly contributed to landward movement from bays and coastal platforms to hills and inland sites in the mid-15th century (Leach and Leach, 1979).
In order to corroborate–or deny-the “red wave” legend in Tonga, we carried out a field survey with the aim to investigate sedimentary features at five sites in Tongatapu. This island is made up of up to 250 m of Pliocene and Pleistocene coral reef limestone (Cunningham and Anscombe, 1985), which rises at its highest point to some 65 m above sea level (a.s.l.) at the southern end of the island (Harrison, 1993). The limestone is covered by tephra deposits, which decrease in thickness from the west (up to 5.5 m) to the east (<1.5 m), indicating that they were deposited from volcanic sources west of Tongatapu against the prevailing winds (Spennemann, 1997). Drawing on a robust body of new compelling evidence from radiocarbon dates, geomorphology and sedimentology, we argue that the Tu’i Tonga kingdom was also severely impacted by a large tsunami in the mid-15th century.
2 Materials and Methods
2.1 Field Investigation
We investigated five sites in Tongatapu where tsunami signatures were identified (Figure 2). One of these sites, at Fahefa village, displays huge coral boulders located between 10 and 22 m asl which were already studied by Frohlich et al. (2009). The second site, called Haveluliku, was the subject of an unpublished preliminary study by a Japanese team, which measured the size of some blocks. The three other sites, namely Kolovai, Alaki and Anahulu cave were discovered and investigated by our team during a field trip in October 2018.
Sedimentary deposits were studied at natural or man-made outcrops (cliffs, embankments) and in hand shovelled trenches, including one trench in the Anahulu cave. Several sandy deposits were found and sampled beneath some megablocks suspected to have been deposited by a tsunami. These deposits cannot therefore be unambiguously more recent than the block above them. The faces of the trenches were refreshed and levelled with the help of a narrow trowel. For all sites, the stratigraphic units were identified, thoroughly described and sampled for: 1) sedimentological analysis: grain size characterization to infer hydrodynamic conditions prevailing during deposition; petrographic nature of the sediments; micro-fossils determination; 2) radiocarbon dating on shells, foraminiferal samples and charcoals. For the Anahulu cave site, preliminary coring was carried out to assess the depth of sediment trapped in the cave to determine the location with the most significant sediment filling. A 128 cm deep pit was excavated down to the limestone bedrock using a shovel and a crowbar. For the tsunami boulders, which may have been bulldozed by the turbulent tsunami front (Maui rock, Haveluliku and Alaki sites), investigations focused on their landward side under which marine sediments would remain trapped. Excavations were thus carried out to clear the base of the boulders. In addition, the morphometrical component of the roughly ellipsoidal boulders were measured (i.e. length, width, and height) in order to calculated approximate volume (π.Le.Wi.H/6) and masses, using a density of ∼2.0 g/cm3 for these massive coral limestone boulders (Spiske et al., 2008).
2.2 Laboratory Analysis
Several types of analyses were carried out in laboratory. Protocol details of the following analyses are provided in the SI Appendix—Material and methods. Grain size measurement of 12 sand and finer particles were performed at the Laboratory of Physical Geography in Meudon, France, using a Beckman Coulter laser diffraction particle size analyser LS 13 320. It measures particles size over a single range of 0.04–2000 µm. The determination of foraminifera was carried out at the University of Strasbourg from 100 g of sediment taken from samples collected in the field (details in SI Supplementary Appendix S1.1). The composition of major and trace elements of volcanic material (pumice fragments) was performed in three laboratories: analysis of major, minor, and trace elements were made on pumice samples by ICP-AES and ICP-MS at the Laboratoire Magma et Volcans, Clermont-Ferrand, France, and the SARM-CRPG (Centre Pétrographique et Géochimique), Nancy, France (details in SI Supplementary Appendix S1.2). Analysis of melt inclusions, matrix glasses and fluid inclusions was performed at the Department of Geography, University of Cambridge (details in SI Supplementary Appendix S1.2).
Twenty-one charcoal and bioclasts (marine shells, foraminifera) samples were dated using Accelerator Mass Spectrometer (AMS) and radiometric methods at the DirectAMS Radiocarbon Dating Laboratory in Seattle, United States (details in SI Supplementary Appendix S1.3). An important difficulty with respect to dating a tsunami deposit is that during run-up, erosional processes incorporate previously deposited material into the sediment mix, e.g., old shells and foraminifera samples that have previously stagnated at the bottom of the sea or on the beach up to several centuries after the death of the animal (Ishizawa et al., 2020). Therefore, the results conducted using bulk sediment samples merely represent a maximum age of deposition. Another issue for the age estimation of tsunami deposits is that they are not commonly found within a sedimentary sequence. Therefore, it is usually difficult to obtain the required number of 14C ages for Bayesian modelling (Ishizawa et al., 2020).
2.3 Tsunami Numerical Modelling
We performed numerical modelling of tsunamis triggered by earthquakes, caldera-forming volcanic eruptions and volcano flank collapses (details in SI Supplementary Appendix S1.4), and meteorite impact. The simulations were performed using two simulation codes already tested and recognised by the scientific community, i.e. VolcFlow (Kelfoun and Druitt, 2005) and Comcot (Wang and Power, 2011). VolcFlow, which is based on a depth-averaged approach of the equations of mass and momentum balance, has been already used for all types of tsunamis. The tectonic tsunamis were computed by imposing several amplitudes and wavelengths at the boundaries of the calculation domain. To generate the caldera tsunamis, a downward vertical velocity of the caldera floor is imposed (Nomikou et al., 2016). For volcano destabilizations, the mass is released immediately and the velocities of the destabilized rocks and, consequently, the waves characteristics are controlled by the rheology assumed (Kelfoun et al., 2010; Giachetti et al., 2012; Paris et al., 2017). The tsunamis from meteorite impact were simulated for a large range of initial wave amplitudes and related radii (methods detailed in Costard et al., 2017).
3 Results: Evidence of a Large Tsunami in Tongatapu in the 15th Century
Our results are presented following the same West-East geographic transect of this island.
3.1 Kolovai
In the north-western peninsula of Tongatapu, Duphorn (1981) described a widespread sandy deposit termed “Pumice Terrace” (because it contains rounded pumice), covered by a layer of dark grey compact ash fallout deposit. This “Pumice Terrace” has been interpreted as a relict beach related to a +2–3 m mid-Holocene sea level highstand (Roy, 1990). In the same area, we sampled a deposit located 6 m a.s.l. and 70 m away from the Kolovai Beach (Figure 2). This deposit displays typical features of a tsunami deposit (Goff et al., 2011a), e.g.: 1) poorly sorted white sand, which is not consistent with a palaeobeach; 2) abundant individual shells and shell-rich sub-units, and coral fragments; overtopped by 3) a 15 cm-thick layer of rounded dacitic pumice (SiO2 > 63 wt%, Na2O + K20 < 5 wt%: SI Appendix, Supplementary Figure S1, Supplementary Table S1 and Supplementary Table S2), which may have been floated by a flow. This composition is representative of the volcanoes along the Tonga-Kermadec Trench (Bryan et al., 1972). These rocks differ from most other circum-Pacific andesite-dacite suites in their very low content of alkali, especially low K2O (almost always <0.8%). Light differences between the samples indicate that the pumice have been eroded from the Kolovai beach, either by a tsunami or a storm surge similar or higher than the one that have been generated by typhon Harold in April 2020 The altitude of this pumice layer may indicate the maximum runup of the tsunami wave, i.e. 6 m. A charcoal embedded in this layer is dated 1,467 ± 20 years CE (Table 1), which would indicate a mid-15th century tsunami event.
3.2 Fahefa Village, Site of the Maui Rock
A line of seven massive coral limestone boulders is located at Fahefa village, 100–400 m from the reef edge of the western shore of Tongatapu (Figure 2). The largest boulder, called Tsunami Rock or Maui Rock (Maka Tolo ‘a Maui in the local language) reaches 15 m long, 9 m high, and may weights up to 1,600 tons (Figure 3A and Supplementary Table S3). According to Tongan tradition, there are two tales about this Rock (Gifford, 1924, https://www.kanivatonga.nz/2017/09/tongan-legends-portray-maui-as-a-scientist-but-in-poetic-language-say-scholars). One of them relates that the god Maui used this boulder together with a magical rope from the hair of the goddess Hina to anchor the Sun and slow it down from racing across the sky. Another legend tells that Maui hurled the boulders ashore in an attempt to kill a giant man-eating fowl and saved people, the giant fowl referring to the waves—as also suggested by the “Maui throwing stones” legend encountered in Haveluliku (see below). This second tale could be interpreted as a metaphoric reference to a tsunami, which brought these massive boulders to the surface of Tongatapu. Based on scientific evidence, these boulders are considered as the largest known tsunami erratic in the world (Frohlich et al., 2009). All lying from 10 to 20 m a.s.l., they could not have rolled downhill from elsewhere because the island is flat. They are located hundreds of meters from the reef and made of the same coral limestone reef material offshore, but cannot under any circumstances have been deposited by a cyclone.
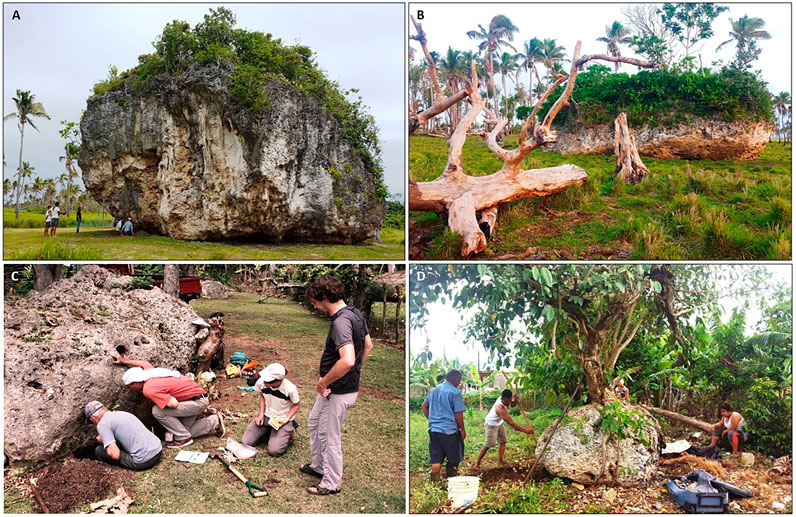
FIGURE 3. Tsunami boulders in Tongatapu. (A) The sacred Maui Rock in Faheha, 10 m a.s.l. (∼780 m3); (B) Second largest megablock at Fahefa, 13.5 m a.s.l. (∼230 m3); (C) Boulder at Haveluliku, 30 m asl; (D) The sacred Masila boulder at Alaki (Mu’a town).
Using the methodology of Nandasena et al. (2011), we calculated the minimum wave heights required to transport the boulders along the west and southeast coast of Tongatapu (Supplementary Table S3). The equation we used for a free boulder displacement is based on four parameters, i.e. size, density, and distance from shore of the boulder, considering two scenarios of Froude number (F). Along the Fahefa coast, the minimum wave height required to transport the largest boulder, i.e. the so-called Tsunami Rock, ranges from 23 m (F = 0.75) to 13 m (F = 1).
On the basis of 230Th ages, Frohlich et al. (2009) argue that the Maui rock must have been deposited either within the past 7,000 years or ca. 122,000 years, rather than at intermediate times when sea level was 15–120 m lower than present. However, there is no trace of limestone dissolution at the bottom of the boulders, which suggests an age of a few thousands or even hundreds years at most. Furthermore, the volcanic soil surrounding the boulders in the lowlands is much thinner than that found at higher elevations (Cowie, 1980), even in remote areas not subject to anthropogenic erosion (due to agricultural or tourist pressure like at the Maui rock). Despite the absence of datable material within the remaining thin surficial soil, we argue that the volcanic soil could have been eroded by the tsunami very recently, a few centuries at most. A large cavity under the Maui rock has trapped thick sandy deposits containing sea shells (e.g. MAU1b2 sample in Table 1), probably carried by the wind during cyclones. This natural cavity, due to the shape of the boulder, could have rapidly served as a shelter against heavy rains soon after the boulder deposition. This could explain the presence of a hearth at the base of the sandy deposit in the cavity, in contact with the basal coral limestone. We excavated this hearth for radiocarbon dating, which gave an age around the 15th or 16th century (Table 1).
3.3 Haveluliku Boulders
On the eastern coast of Tongatapu, a group of eight coral limestone boulders are located in the village of Haveluliku at 30 m a.s.l. and 500 m from the sea shore (Figures 2, 3C and Supplementary Table S3). The largest boulder is 8 × 3 × 3.20 m, i.e. about 70 m3, and may weights about 140 tons. Some of the local residents we interviewed reported a higher number of boulders decades ago, that were gradually dismantled by the local community and used as building material. According to a local myth, these blocks were thrown up to Haveluliku by Maui, a giant chief, from the neighbouring island of Eua. As Maui was annoyed at being woken up by a rooster every morning, he picked up stones to throw them at the rooster, which fled from Eua to Tongatapu where he ended up wounded. In the old Tongan poem by Tufui displaying this legend, the rooster is fowl (Gifford, 1923, p.12). Some Haveluliku inhabitants refer to the blocks as the “Maui throwing stones” deposited while chasing chicken(s). Interestingly, as the term “white chicken” means “foaming waves” in Tongan, this legend might be a metaphor for a tsunami. However, this interpretation is tenuous as roosters are very widely associated in Polynesian mythology with voracious human or semi-divine warriors or enemies (Richter-Gravier, 2019), and not the sea specifically; it is more likely that this myth refers to the destruction of a dominant community (maybe by a tsunami).
The boulders lay on a surface formation mainly formed by a 25–30 cm thick layer composed of coarse and slightly pedogenized marine sand mixed with marine shells and occasional rounded pumices (Figure 4). Some erosional figures within the deposit makes the case for a transport by a landward turbulent flow, which cannot be a storm surge at this altitude (up to 30 m a.s.l.). The anthropic origin being ruled out, this deposit can only be attributed to a large tsunami that hit the southern coast of Tongatapu.
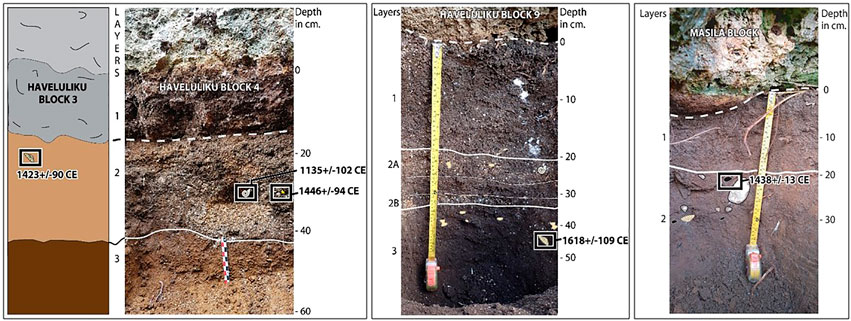
FIGURE 4. Sediment layers interpreted as tsunami deposits below the boulders at Haveluliku and Alaki.
As an additional argument for this interpretation, the deposits below the boulders are poorly sorted as attested by the S0 that evolves between 4.246 and 2.663 (while 1 corresponds to a good sorting: SI Appendix, Supplementary Figure S2). The spreading and multimodal shape of the granulometric curves, in addition to some erosional figures within the deposits, reflect an en-masse deposition of the sediment transported by a highly turbulent flow, which cannot be a storm surge at this altitude (30 m a.s.l.). Such a particle size distribution cannot be associated with either an aeolian-type deposit (e.g. from a cyclone) or an anthropogenic origin. This deposit can therefore only be attributed to a large tsunami. Based on the model of Nandasena et al. (2011), the minimum wave heights required to transport the largest boulder at Haveluliku ranges from 11 m (F = 0.75) to 6.2 m (F = 1) (Supplementary Table S3).
A confirmation of the high turbulence during sediment transport from the sea to Haveluliku is reflected by the rate of foraminifera wear and tear. A comparative study of the foraminiferal content of the beach closest to Haveluliku and the sands trapped beneath the boulders shows that the wide variety of species present on the beach is reduced to the clear dominance of a single species in the deposit: Baculogypsina sphaerulata (Parker and Jones 1860). When alive, this species prefers shallow conditions, i.e. <5 m. These habitats are characterized by coral sand and constant wave disturbance (Hallock, 1984; Hohenegger et al., 1999). In this shallow zone, these star-like Large Bentic Foraminifera (LBF) display 3 to 7 sharp points. On the beach, the attrition due to the permanent movement of the sea reduces the sharpness of the branches which are smaller, and sometimes reduced to small bulges. The turbulent transport by the tsunami waves on a 500 m distance from the seashore to Haveluliku village has produced an intense friction and the Baculogypsina sphaerulata found in large number under the boulders therefore exhibit spherical shapes. The lack within this deposit of all the other foraminifera present on the beach must be related to their weak resistance to shocks and attrition.
Radiocarbon dating of four shells and 1 foraminifera sample (Baculogypsina sphaerulata) within the sand deposits below three boulders at Haveluliku (HAV 3, HAV4, and HAV9 display a wide range of ages, from the ninth to post-1950 (Figure 4). According to testimonies of local inhabitants, the largest and most recent shells are leftovers of food consumed and then buried under the Block 7. Excluding these recent shells, the other bioclasts that have been dated are considered part of the tsunami deposits. The oldest bioclasts, dated to several centuries before the event, were eroded near the shoreline and then transported and deposited by the tsunami. The most recent one believed to have been deposited by the tsunami have been dated to the 15th century CE.
3.4 Anahulu Cave
About 500 m away from the Haveluliku boulders in the southern direction, we identified at least one but probably two tsunami deposits at the bottom the Anahulu cave (Figure 5A), which entrance is facing the sea at 9 m altitude. Its name “Anahulu” means « cave of dried leaves” (Gifford, 1923), because it was so dark in the cave that the Tongans had to burn torches of dried leaves to penetrate and explore it. Along a 130 cm deep trench realised inside the cave, we identified 25 layers above the calcareous bedrock (Figure 5B). The basal sequence (1–3) is underlain by limestone bedrock and is characterized by predominantly sandy terrigenous sediments resulting from soil erosion above the cave. This sequence is overlain by a layer of greenish volcanic ash (4) whose facies evolves upward by enrichment in terrigenous silt (5). Resting on these deposits with a slightly erosive base, silty layers regularly alternate with layers of slightly coarser material (6–14). This sequence seems to correspond to periodic entries of eroded material from the slope upstream of the cave through fractures in its ceiling.
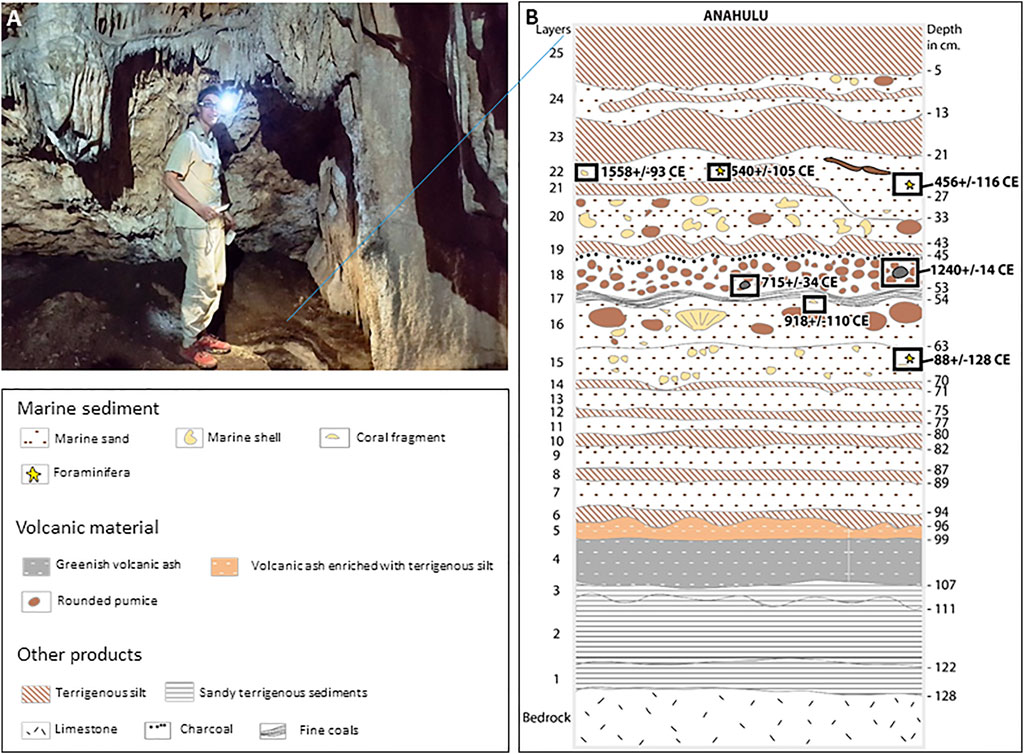
FIGURE 5. Sedimentary sequence within the Anahulu cave, showing two sequences of tsunami deposits. 1–3: Predominantly sandy terrigenous sediments. 4: Greenish volcanic ash. 5: Volcanic ash enriched with terrigenous silt. 6–14: silty layers alternating with layers of slightly coarser material, interpreted as periodic entries of eroded material from the slope upstream of the cave through fractures. 15: Coarse marine bioclastic sand, with numerous shell fragments and clasts of coral limestone. 16: Coarse marine sand with abundant large shells, rounded pumice up to 9 cm in diameter and terrigenous cave bottom material. 17: 2-cm layer of fine coals. 18: 8-cm layer of small well rounded pumice. 19: Thin layer of brown compact silt. 20: White bioclastic sand mixed with terrigenous silty material, numerous coral debris, shells and pumice. 21: Brown silts. 22: Marine sand sometimes enriched with terrigenous silts. Presence of rip-up clasts. 23–24: alternating terrigenous elements and stretched marine sands. 25: Blackish silty layer containing recent anthropic artifacts.
Layer 15, weakly erosive on layer 14, marks a clear change in the origin of the sedimentation. Composed of coarse marine bioclastic sands, it contains numerous shell fragments and clasts of coral limestone. Its top part (16) contains abundant large whole shells, isolated rounded pumice up to 9 cm in diameter and is enriched in terrigenous cave bottom material. This coarse heterometric material is covered by an 8 cm layer of small well rounded pumice (18). The dacitic composition of these pumices (63–69 wt% SiO2, 3.4–4.6 wt% Na2O + K2O: Supplementary Table S2 (geochimie glasses) differs from the basalt-andesite ignimbrites formed during the latest paroxysmal (VEI 5–6) explosive eruption of Tofua volcano ca. 1,000 years BP, located ca. 160 km from Tongatapu (SI Appendix, Supplementary Figure S2 and Supplementary Table S1). The top of this marine sequence 18) corresponds to a 2 cm thick layer of fine coals. The tsunami origin of this marine sequence (15–19) is beyond doubt, because the entrance of the cave is too high to be reached by the sea during the largest cyclones (i.e., of Cat. Five on the Saffir-Simpson scale) like Harold in 2020 or Gita in February 2018, which is considered as the strongest to have hit Tonga in its history. This first marine sequence is separated from a more recent one by a thin layer of brown terrigenous silt (19).
This second marine sequence (20–24) more than 35 cm thick rests on the silt layer (19) with an undulating contact. At the base, layer 20 is composed of white bioclastic sands mixed with terrigenous silty material. It contains numerous coral debris, foraminifers, sea shells and pumice. Layer 22 also corresponds to pure marine sands sometimes enriched with terrigenous silts. Its contact is erosive on the underlying layers (20 and 21) which it locally cuts in unconformity. The high energy of the water during the deposition of this layer is attested at the base by the presence of silty rip-up clasts typical of highly turbulent flows with a high capacity to erode the terrigenous substratum. The top of the marine sequence (23–24) shows alternating terrigenous elements and stretched marine sand that indicates a back and forth movement of sediment-laden water in the confined context of the cave at the end of a high energy marine flooding. The entire deposit is fossilized by a blackish silty layer (25) containing more or less recent anthropic artifacts. For the same reasons as for the first marine sequence (15–19), this second marine sequence (20–24) is interpreted as a tsunami deposit.
Seven samples were radiocarbon dated, including two charcoals, two shells and three foraminifera, at different depths of the stratigraphic sequence in the cave (Figure 5B and Table 1). The youngest radiocarbon date calculated for the lower sequence is 1,240± 14 years CE. It was obtained on a charcoal within the pumice layer (18), 48 cm deep. The youngest radiocarbon date of the stratigraphic sequence in the cave was obtained on a sea shell in the upper tsunami sequence (layer 22). The calculated age of 1,558 ± 93 CE is consistent with the most recent one obtained for the Haveluliku boulders and at Kolovai beach, i.e. around the 15th century.
The presence of a charcoal layer overlain by a terrigenous silty deposit shows that the two marine sequences were separated by a short period. It is not clear however, whether the whole succession refers to two different tsunamis, or to a single tsunami composed of two main waves. In the former case, the dates obtained suggest that the two different tsunamis were separated by no more than three centuries. However, while the charcoal may have an intrinsic age older than the tsunami deposit they are found (e.g., older inner rings of a tree: Ishizawa et al., 2020), the lower marine sequence could belong to the first wave of a single tsunami, and be the same age as the upper marine sequence, i.e., 15th century. Under this hypothesis, the terrigenous silty layer (19) that separates the two units may have formed rapidly by erosion of the cave roof during the interval between the two tsunami waves.
3.5 The Masila Boulder at Alaki, Mu’a City
Along the Fanga ‘Uta lagoon, located in the northern part of this island, we found only one coral boulder of about 3 cubic metres in the town of Mu’a. Locally called Masila, this sacred boulder for the inhabitants is topped by a tree (Figure 3D). As there are a large number of undisturbed archaeological sites dated to around 2,800–2,700 years in the coastal deposits of the lagoon, it is unlikely that this isolated boulder was deposited by a tsunami a few centuries ago, even if a charcoal located at a 20 cm depth beneath this boulder was dated 15th century (Table 1).
4 Discussion
Using stratigraphic data, sedimentological analyses, and radiocarbon dates, this study argues that a large tsunami struck and flooded the lowlands of Tongatapu Island up to over 30 m a.s.l. around the mid-15th century. The timing and size of this event are consistent with a region-wide tsunami identified elsewhere in the southwestern Pacific Ocean, which raises the question of its origin and of the human impacts of this tsunami.
4.1 Lack of Evidence for the Origin of the Tsunami
Based on geomorphological field data and numerical tsunami propagation models, we review here the different sources and processes that may have caused the mid-15th century tsunami.
4.1.1 Earthquake Without Co-seismic Slope Failure
Based on the Global Historical Tsunami Database (NCEI-NOAA), there are actually no historical record describing tsunamis exceeding 8–10 m runup anywhere in Tonga produced by either the largest historically known regional earthquakes (up to MW 8.4) or by far-sources teletsunamis. Computer simulations of earthquake-triggered tsunami along the Tonga-Kermadec Trench (TKT: Figure 1) were performed by Frohlich et al. (2009). The authors selected a worst case earthquake scenario of Mw 9, with a slip of ∼30 m along faults and dimensions ∼120 km × 1,000 km. Simulations provided peak-to-trough amplitudes of ∼12 m on the west side of Tongatapu. This value is at the lower limit of the wave heights needed to move the largest Fahefa boulders, e.g. Maui Rock, making this source possible, but quite unlikely without a local coseismic submarine landslide. Accordingly, while acknowledging that there may be numerous unknown sources for the large tsunami identified in New Zealand at the same period, Goff et al. (2011a) and Goff and Chagué-Goff (2015) suggested that two different tsunami events may have occurred: one of them resulting from a large earthquake triggered along the Tonga-Kermadec Trench (TKT); and another one due to a local fault rupture, with or without co-seismic flank collapse, off the New Zealand west coast.
4.1.2 Pyroclastic Density Currents (PDCs) Entering the Sea
Located north of Tongatapu, Tofua volcano (Figure 1) displays a 5 km-wide caldera with steep inner walls rising up to 200 m above sea level. This island has been mantled by thick ignimbrite, which form high pumice cliffs in the south slope of the volcano. Radiocarbon dating of a carbonised tree trunk recovered from the base of the ignimbrite sequence gave an age of 970 ± 50 years BP (Caulfield et al., 2011) corresponding to a calendar age range of 1,044–1158 CE (using CALIB 8.2), which is inconsistent with a 15th century tsunamigenic eruption. In addition, numerical modelling performed by one of the coauthors (KK) using VOLCFLOW code show that voluminous PDCs or debris avalanche entering the sea from the south flank of Tofua do not provide tsunami waves higher than 15 m at Tongatapu and only 5 m at the SE shore where the highest boulders have been described (SI Appendix, Supplementary Figure S3). Furthermore, the basalt-andesitic composition of the Tofua ignimbrite (Caulfield et al., 2011) is not consistent with the Low-K dacite composition of the pumice fragments found within the mid-15th century tsunami deposits ((SI Appendix, Supplementary Figure S1).
4.1.3 Eruption-Triggered Flank-Collapse
Slope failures several kilometres wide were reported to have generated megatsunamis due to the entry into the sea of large-scale debris avalanches (Giachetti et al., 2012). In such case, the closer the volcano is, the higher the runup on the exposed shore, with maximum values being reached in the axis of the collapse. Scarps resulting from flank collapses are common morphological landforms on the submarine stratovolcanoes along the Tonga Ridge (Massoth et al., 2007). Computer simulations of tsunamis generated by flank collapses of volcano #2 performed by Frohlich et al. (2009) were successful to produce peak to-trough amplitudes of 14 m at 100 m water depths off western Tongatapu, which suggests a higher tsunami wave and runup at the coast. In this study, new computer simulations of an immediate collapse of 15 km3 from Volcano 2 reproduces similar waves of 15 m on the southeast coast of Tongatapu (SI Appendix, Supplementary Figure S3), which could be consistent with a 30 m runup height. However, the amplitude of tsunami waves decreases rapidly with distance when triggered by a volcanic flank collapse (Walters et al., 2006), making the generation of a tsunami affecting the entire southwest Pacific unlikely. Interestingly, the position of the proposed tsunamigenic submarine volcano, as well as that of the other potential volcanic sources presented in the next section, fits well with the Maui myth documented by Gifford (1923). Indeed, he identifies the “flying stones” that chased the giant moa as coming from the direction of Eua Island, specifically striking the southern coast of Tongatapu.
4.1.4 Collapse of a Volcanic Island During a Caldera-forming Eruption
It is recognised that calderas formed rapidly and “en masse” during explosive eruptions of silicic magmas (e.g. dacite) might trigger a tsunami (Cas and Wright, 1991). However, tsunamigenic processes capable of generating high tsunami runup during caldera-forming eruptions are still debated, as different processes may be involved (Paris, 2015): the caldera collapse itself (which is poorly constrained and may vary widely in terms of duration), pyroclastic flows entering the sea, underwater explosions, earthquakes, slope instabilities and shock waves. With runup higher than 30 m a.s.l., the tsunami that has been identified ca. 1450 CE in Tonga looks as strong as the one generated by the Krakatoa eruption in 1883 CE (Simkin and Fiske, 1983). Therefore, the tsunamigenic caldera-forming eruption would probably have had a Volcanic Explosivity Index (VEI) ≥ 5 or even 6 (Self, 2006).
The strong bipolar sulfate spikes identified in polar ice cores from Greenland and Antarctica point to two tropical eruptions, in 1,452–53 CE (Gao et al., 2006; Cole-Dai et al., 2013; Esper et al., 2017) and 1,457–58 CE (Sigl et al., 2013). The identification of sulfate aerosols in polar ice discards the hypothesis of submarine eruptions, as it would have prevented sulfate gases to reach the stratosphere. The source of these eruptions remains to be identified. Kuwae volcano in Vanuatu was the first to be proposed as the most likely source of one of these large eruptions in the mid-15th century (Witter and Self, 2007; Goff and Chagué-Goff, 2015). According to marine and field observations, its 12 × 6 km wide submarine caldera was formed during a single eruption supposed to have ejected the equivalent of 30–60 km3 DRE (dense rock equivalent) in the form of pyroclastic flows and ashfall deposits (Robin et al., 1994; Witter and Self, 2007). However, the Kuwae eruption was initially dated 1,425–1430 CE (Monzier et al., 1994), and further correlated—with doubtful scientific argument—to bipolar sulfate spikes identified by polar ice cores. Indeed, this link is mainly based on a local myth suggesting the collapse of this volcano in the sea, but without providing any precision concerning the date of the eruption. Furthermore, the limits of the underwater caldera are hypothetical (Nemeth et al., 2007; Caulfield et al., 2011), and the most proximal palaeotsunami deposit in Vanuatu is very small, both in elevation above sea level and thickness (Goff et al., 2008; Goff et al., 2011b). More recently, geochemical analysis of 1,457 cryptotephra found in the South Pole ice core definitely dismisses Kuwae as the only source of the 1458 CE unidentified eruption (Hartmann et al., 2019).
Shallow-water calderas are ubiquitous along the Tonga Ridge (SI Appendix, Supplementary Figure S4: Massoth et al., 2007), and none of them have been dated yet. The good state of conservation of the walls of some of them and the apparent weakness of erosion on certain slopes pleads in favour of a rather young age, possibly a few centuries. Therefore, some of the “vanished islands” of the Pacific reported in Polynesian legends (Nunn and Pastorizo, 2007; Nunn, 2008) could correspond to ancient collapsed volcanoes of Tonga. Observations during recent submarine eruptions in Tonga along the Tofua arc (Brandl et al., 2020), and computed drift trajectories of sea-rafted pumice using numerical models, indicate that pumice rafts are always directed westward from their source, in accordance with the southwest Pacific surface wind fields and ocean currents. Therefore, considering that at least part of these pumice clasts have been expelled by a tsunamigenic volcanic eruption, the volcanic source should have been located quite close from Tongatapu. With the aim to identify the former volcanic islands before their total collapse, we reconstructed the precaldera altitude of all submarine volcanoes located along the south part of the Tonga ridge, from volcano #1 to volcano #19 (SI Appendix, Supplementary Figure S4). As plotted in Fig. S4, only 7 out of 19 calderas resulted from the total collapse of a former emerged volcano (SI Appendix, Supplementary Figure S5), whereas the other volcanoes were already submarine at the time of the caldera–forming eruption (SI Appendix, Supplementary Figure S6). Volcanoes #1 and #2, which are the closest from Tongatapu, were identified as candidates for a possible mid-15th century stratospheric eruption. However, difficulties in calibrating caldera formation models due to the lack of in situ observation make assessments of the speed of caldera collapses doubtful. It would seem, however, that the rate of caldera collapses is too slow to generate significant tsunamis, except if the total collapse of the volcanic island is preceded by one or more flank collapses, which appear to be much more tsunamigenic (Paris, 2015).
4.1.5 An Unidentified Meteorite?
The ability of bolide impacts to generate tsunamis capable of leaving long-term traces on coastal areas has been extensively discussed (Crawford and Mader, 1998; Wünnemann et al., 2007). It has been shown that Indigenous oral traditions and legends contain historical accounts of actual meteoritic events from across Australia, particularly Queensland and Victoria (Hamacher, 2013). Based on Aboriginal and Maori stories, Bryant (2001) suggested that the southeastern coast of Australia was struck by a tsunami induced by a cosmic impact in the Tasman Sea within the last 600—years. A 40-km wide crater candidate for a remarkable tsunami event that could have affected the Tongan archipelago was identified by David Sandwell 450 km away from maps of gravity gradients derived from satellite altimetry (Dallas Abbott, pers. comm.). Based on this hypothetical source located along the Tonga Trench (Figure 1), we performed numerical simulations using COMCOT (SI Appendix Supplementary Figure S7). A vertical water surface displacement of 165 m at the impact (following Kharif and Pelinovsky, 2005) may have only generated a ∼6 m high wave at a water depth of 220 m off Tongatapu, due to a rapid dissipation of its energy. The maximum wave height recorded at the western coast of this island is around 10–15 m, whereas it does not exceed a few meters along the coasts of New Zealand.
In summary, it is very unlikely that a single event of very high magnitude, whether seismic or cosmic in origin, would have generated a tsunami that affected the entire southwest Pacific coastline, from New Zealand to Wallis and Futuna through Tonga archipelago, in the 15th century. This implies that this region of the world must have been subjected to multiple telluric events at this time, some near New Zealand as suggested by Goff and Chagué-Goff (2015), and another near Tongatapu, possibly a volcanic eruption with flank collapse.
4.2 Tracking the Societal Impacts of the Mid-15th Century Tsunami in Tonga and Beyond
The hypothesis of a devastating tsunami in Tongatapu in the 15th century has never been mentioned in the literature. Could several facts that have been hotly debated by historians and/or archaeologists for years find a scientific explanation in a natural disaster caused by a tsunami? We focus this discussion on two main issues.
4.2.1 The Exceptionally High Number of Mounds on the Island
The island of Tongatapu is covered with nearly 10,000 tombs and mounds (revealed through LiDAR survey: Freeland et al., 2016), the origin of which is still under debate. Relatively little is known about the people who built and used the majority of mounds, with only a handful having been excavated (Davidson, 1969; Spennemann, 1989). Tongan traditions differ significantly on the issue of mounds and tomb age, as well as to who built the tombs and who was buried in them. Clark et al. (2008) suggested that it might be the result of a deliberate or inadvertent hiding of tomb history. On the basis of their sheer numbers, most “generic” mounds in Tonga are assumed to be burial places for subordinate chiefly and commoner lineages (Burley, 1998). One could think that the exceptionally high number of funeral structures could be linked—at least for some of them - to the construction of mass graves following a major disaster in order to limit epidemics, as was done in Aceh, Indonesia during the 2004 tsunami. Several arguments, however, plaid against of this highly speculative hypothesis. First, as so few mounds have been excavated, we have actually no idea if these are generally burial features or not (E. Cochrane, pers. comm.). Second, if the 10,000 mounds were largely burial mounds, one would think such a catastrophic population loss would be recorded in oral tradition and perhaps even reflected in population numbers and demography when Europeans arrived two centuries later. This, however, is not the case (Burley, 2007). Third, the construction of mass graves following a major disaster seems highly unlikely in the absence of a modern state coordinating the retrieval of victims’ bodies. Gathering and identifying bodies, to say nothing of organising formal burials in large-scale earthworks, is likely to have been beyond the capacity of survivors.
4.2.2 The Construction of Ha’amonga ‘a Maui or Trilithon, and the Relocation of the Tu’i Tonga Capital
Some of the tombs are monumental, especially in the sites of the two former capitals of the Tu’i Tonga Empire. The site of the oldest capital, Heketā, at the eastern edge of Tongatapu (Figure 2), displays nine stone structures spread over 350 m of land that gently slopes towards the exposed limestone coast (Clark and Reepmeyer, 2014). The principal structure of this site, and the most famous monument in Tonga, is the sacred Ha’amonga ‘a Maui (“burden of Maui”), a unique megalithic trilithon, which comprises three coral limestone slabs (SI Appendix, Supplementary Figure S8). In Tongan tradition, the trilithon is believed to have been built by the god Maui, as the stones would be too huge for mortals to handle. However, Clark and Reepmeyer (2014) have recently shown that the coral limestone of the trilithon come from the nearby coast, where it was carved on site. Radiocarbon dating of three marine samples that have been collected beside the west upright of the trilithon provide ages of the monument construction between 1,320 and 1460 CE (Clark and Reepmeyer, 2014), i.e. soon before the relocation of the Tu’i Tonga capital from Heketā to Lapaha (Figure 2), that occurred around the mid-15th century (Clark et al., 2008). The reason of this relocation remains unclear. Campbell (2015) suggests that for a political power that had to rely on long-distance travel, Lapaha was a superior location, providing a safe anchorage where large voyaging canoes could be brought safely ashore. Nunn (2007) considers that the political instability, the abrupt end of long-distance travel and the shift of settlements from the coasts to inlands across the Pacific (which may include the move from Heketa to Lapaha) may have originated in the effects of the Little Ice Age that began around 1300 AD. For a few centuries indeed, lower temperatures and stormier weather were disruptive, especially as the resulting 70–80 cm drop in sea level profoundly affected the food resources available in coastal areas (Nunn, 2007). However, the hypothesis of a widespread settlement shift has been challenged by Fitzpatrick (2010, 2011) who claims that it is not supported by data across the Pacific (cf. Allen, 2006). Could the occurrence of a tsunami have helped motivate this choice to relocate to a safer area? The bracket date of the trilithon’s construction does not exclude the possibility that the monument was erected soon after the tsunami, as a means of resilience in order to assert the great power of paramount chiefs over nature, as observed in other civilizations. It should be borne in mind, however, that this hypothesis remains highly speculative and is not based on any proven fact, but only on a date agreement.
5 Conclusion
In the Tongan traditions, several legends related to the god Maui are related to huge blocks of coral limestone perched at an altitude up to 30 m a.s.l. along the southeast coast of Tongatapu and up to 20 m a.s.l. along the west coast. But so far, no one has linked these legends to scientific data. The largest one weighs ∼1,600 tons and is considered as the largest boulder deposited by a tsunami worldwide, previously dated to 120,000 BP or late Holocene. Based on radiocarbon dating of organic material collected under some of these boulders, and within other sandy tsunami deposits recently discovered on the west and southeast part of Tongatapu, we can confidently claim that a large tsunami occurred during the 15th century. However, it is unlikely that this tsunami destroyed the Tui’ Tonga kingdom, as it mainly affected the sparsely populated southern coast of Tongatapu, while most people lived around the lagoon along the northern coast. Despite the lack of compelling evidence, we state that this event possibly triggered some cultural upheavals, such as the relocation of the capital of the Tui’i Tonga empire or the emergence of monumental funerary architecture at the aftermath of the disaster.
Although the origin of this large tsunami still remains uncertain, we provide a series of arguments in favour of a caldera-forming eruption that would have caused the total collapse of an ancient island volcano, probably located along the Tonga ridge less than 150 km southwest off Tongatapu. The majority of the large explosive eruptions of the Common Era and beyond remain unidentified, partly because some of them are related to former volcanic islands which disappeared underwater. In order to identify the volcanic source of the mid-15th southern Pacific tsunami, further investigation should focus on the identification and characterisation of submarine active and dormant volcanoes and extensive tsunami modelling. A catastrophic tsunami at the same period has also been identified in other islands of the Southwest Pacific such as New Zealand or Wallis and Futuna, but numerical modelling show that a single gigantic tsunami generated by a mega-earthquake, a caldera-forming eruption or even a big meteorite is unlikely.
Data Availability Statement
The original contributions presented in the study are included in the article/Supplementary Material, further inquiries can be directed to the corresponding author.
Ethics Statement
Written informed consent was obtained from the individual(s) for the publication of any potentially identifiable images or data included in this article.
Author Contributions
FL designed the study and wrote the paper in collaboration with JM, PW, RP, and KK, FL, JM, PW, OW, AM, TT, and FK conducted fieldwork in Tonga. Laboratory analyses were made by SS-C and MT (sedimentology), FM, MB, and CV (geochemistry). RP and KK run tsunami modelling. MM developed the geomorphological model of submarine volcanoes. AF drew the figures on the geochemical data and interpreted them. JM and OW collected Tongan tales and legends. TK provided administrative permissions and information on the Tonga geology and History. CG provided information on tsunami events in the Western Pacific.
Funding
The field trip of the French team was carried out in the frame of the Cluster of Excellence (LabEx) “DynamiTe”, with reference ANR11-LABX-0046 (SarDyn Program TongaPag), which is funded by the French National Agency for Research. This field trip was co-funded by the Laboratory of Physical Geography, UMR 8591 CNRS-Paris 1-UPEC. Field work of the Tongan team was funded by the Tongan Ministry of Land and Natural Resources, Natural Resources Division. Laboratory analysis was funded by the Institut Universitaire de France in the frame of the IUF program of FL CV was funded by VEILA project H2020-MSCA-IF-2017.
Conflict of Interest
FK was employed by the company Geocare Petroleum Consult Ltd.
The remaining authors declare that the research was conducted in the absence of any commercial or financial relationships that could be construed as a potential conflict of interest.
Publisher’s Note
All claims expressed in this article are solely those of the authors and do not necessarily represent those of their affiliated organizations, or those of the publisher, the editors and the reviewers. Any product that may be evaluated in this article, or claim that may be made by its manufacturer, is not guaranteed or endorsed by the publisher.
Acknowledgments
A special thanks to the LabEx DynatiTe which made this project possible. All samples analyzed in the study were collected under a Research Permit issued to DVB by the Prime Minister’s Office, Government of Tonga, Nuku’alofa, Tonga. We acknowledge the people of Tonga who provided useful information in the field. Dallas Abbott for her information concerning the potential meteor crater in the Southwestern Pacific.
Supplementary Material
The Supplementary Material for this article can be found online at: https://www.frontiersin.org/articles/10.3389/feart.2021.748755/full#supplementary-material
References
Allen, M. S. (2006). New Ideas about Late Holocene Climate Variability in the Central Pacific. Curr. Anthropol. 47 (3), 521–535. doi:10.1086/504168
Barnes, S. S., and Hunt, T. L. (2005). Samoa's Pre-contact Connections in West Polynesia and beyond. J. Polynesian Soc. 114 (3), 227–266.
Bollt, R. (2008). Excavations in Peva Valley, Rurutu, Austral Islands (East Polynesia). Asian Perspect. 47, 156–187. doi:10.1353/asi.2008.0006
Bounoure, G. (2009). Hostile Shores. Catastrophic Events in Prehistoric New Zealand and Their Impact on Maori Coastal Communities. Auckland: Hostile Shores, 174–175. doi:10.4000/jso.5835
Brandl, P. A., Schmid, F., Augustin, N., Grevemeyer, I., Arculus, R. J., Devey, C. W., et al. (2020). The 6-8 Aug 2019 Eruption of 'Volcano F' in the Tofua Arc, Tonga. J. Volcanology Geothermal Res. 390, 106695. doi:10.1016/j.jvolgeores.2019.106695
Bryan, W. B., Stice, G. D., and Ewart, A. (1972). Geology, Petrography, and Geochemistry of the Volcanic Islands of Tonga. J. Geophys. Res. 77, 1566–1585. doi:10.1029/jb077i008p01566
Bryant, E. A. (2001). Tsunami : The Underrated Hazard. Cambridge: Spinger. Cambridge University Press.
Bryant, E. A., Young, R. W., and Price, D. M. (1992). Evidence of Tsunami Sedimentation on the southeastern Coast of Australia. J. Geology. 100, 753–765. doi:10.1086/629626
Burley, D. V. (2007). “Archaeological Demography and Population Growth in the Kingdom of Tonga,” in The Growth and Collapse of Pacific Island Societies. Editors P. V. KirchJ-L. Rallu (Honolulu: University of Hawaii Press), 177–202.
Burley, D. V., and Addison, D. J. (2014). “Tonga and Sāmoa in Oceanic Prehistory,” in The Oxford Handbook of Prehistoric Oceania (New York: Oxford University Press), 231–251. doi:10.1093/oxfordhb/9780199925070.013.017
Burley, D. V. (1998). Tongan Archaeology and the Tongan Past, 2850 – 150 BP. J. World Prehistory 12, 337–392. doi:10.1023/a:1022322303769
Campbell, I. C. (2015). Island Kingdom: Tonga Ancient and Modern. Christchurch: Canterbury University Press.
Cas, R. A., and Wright, J. V. (1991). Subaqueous Pyroclastic Flows and Ignimbrites: an Assessment. Bull. Volcanol. 53, 357–380. doi:10.1007/BF00280227
Caulfield, J. T., Cronin, S. J., Turner, S. P., and Cooper, L. B. (2011). Mafic Plinian Volcanism and Ignimbrite Emplacement at Tofua Volcano, Tonga. Bull. Volcanol. 73, 1259–1277. doi:10.1007/s00445-011-0477-9
Clark, G., Burley, D., and Murray, T. (2008). Monumentality and the Development of the Tongan Maritime Chiefdom. Antiquity 82, 994–1008. doi:10.1017/S0003598X00097738
Clark, G., and Reepmeyer, C. (2014). Stone Architecture, Monumentality and the Rise of the Early Tongan Chiefdom. Antiquity 88, 1244–1260. doi:10.1017/S0003598X00115431
Clark, G. R., Reepmeyer, C., Melekiola, N., Woodhead, J., Dickinson, W. R., and Martinsson-Wallin, H. (2014). Stone Tools from the Ancient Tongan State Reveal Prehistoric Interaction Centers in the Central Pacific. Proc. Natl. Acad. Sci. 111 (29), 10491–10496. doi:10.1073/pnas.1406165111
Cochrane, E. E., and Rieth, T. M. (2016). Sāmoan Artefact Provenance Reveals Limited Artefact Transfer within and beyond the Archipelago. Archaeology in Oceania 51 (2), 150–157. doi:10.1002/arco.5090
Cole-Dai, J., Ferris, D. G., Lanciki, A. L., Savarino, J., Thiemens, M. H., and McConnell, J. R. (2013). Two Likely Stratospheric Volcanic Eruptions in the 1450s C.E. Found in a Bipolar, Subannually Dated 800 Year Ice Core Record. J. Geophys. Res. Atmos. 118, 7459–7466. doi:10.1002/jgrd.50587
Costard, F., Séjourné, A., Kelfoun, K., Clifford, S., Lavigne, F., Di Pietro, I., et al. (2017). Modeling Tsunami Propagation and the Emplacement of Thumbprint Terrain in an Early Mars Ocean. J. Geophys. Res. Planets 122, 633–649. doi:10.1002/2016JE005230
Cowie, J. (1980). Soils from Andesitic Tephra and Their Variability, Tongatapu, Kingdom of tonga. Soil Res. 18, 273–284. doi:10.1071/SR9800273
Crawford, D. A., and Mader, C. L. (1998). Modeling Asteroid Impact and Tsunami. Sci. Tsunami Hazards 16, 21–30. Available at: http://tsunamisociety.org/STHVol16N1Y1998.pdf.
Cunningham, J. K., and Anscombe, K. J. (1985). “Geology of’Eua and Other Islands, Kingdom of Tonga,” in Geology and Offshore Resources of Pacific Island Arcs —Tonga Region. Editors D. W. Scholl, and T. L. Vallier (Houston: CircumPacific Council for Energy and Mineral Resources), 221–257.
Davidson, J. M. (1969). Archaeological Excavations in Two Burial mounds at ‘Atele, Tongatapu. Rec. Auckl. Inst. Mus. 6, 251–286.
Dickinson, W. R., Burley, D. V., and Richard, S. (1999). Holocene Paleoshoreline Record in Tonga: Geomorphic Features and Archaeological Implications. J. Coastal Res. 15 (3), 682–700.
Duphorn, K. (1981). Interim Report on Applied Coral Sand Investigations in and off Tongatapu. Suva: Report of the Institute of Marine Resources.
Esper, J., Büntgen, U., Hartl-Meier, C., Oppenheimer, C., and Schneider, L. (2017). Northern Hemisphere Temperature Anomalies during the 1450s Period of Ambiguous Volcanic Forcing. Bull. Volcanol. 79, 41. doi:10.1007/s00445-017-1125-9
Fitzpatrick, S. M. (2010). A Critique of the ‘AD 1300 Event’, with Particular Reference to Palau. J. Pac. Archaeology 1 (2), 168–173.
Fitzpatrick, S. M. (2011). Defending the Defensible or Offending the Sensible? A Response to Nunn & Hunter-Anderson. J. Pac. Archaeology 2 (1), 100–105.
Freeland, T., Heung, B., Burley, D. V., Clark, G., and Knudby, A. (2016). Automated Feature Extraction for Prospection and Analysis of Monumental Earthworks from Aerial LiDAR in the Kingdom of Tonga. J. Archaeological Sci. 69, 64–74. doi:10.1016/j.jas.2016.04.011
Frohlich, C., Hornbach, M. J., Taylor, F. W., Shen, C.-C., Moala, A., Morton, A. E., et al. (2009). Huge Erratic Boulders in Tonga Deposited by a Prehistoric Tsunami. Geology 37, 131–134. doi:10.1130/G25277A.1
Gao, C., Robock, A., Self, S., Witter, J. B., Steffenson, J. P., Clausen, H. B., et al. (2006). The 1452 or 1453 A.D. Kuwae Eruption Signal Derived from Multiple Ice Core Records: Greatest Volcanic Sulfate Event of the Past 700 Years. J. Geophys. Res. 111, 1–11. doi:10.1029/2005JD006710
Giachetti, T., Paris, R., Kelfoun, K., and Ontowirjo, B. (2012). Tsunami hazard Related to a Flank Collapse of Anak Krakatau Volcano, Sunda Strait, Indonesia. Geol. Soc. Lond. Spec. Publications 361, 79–90. doi:10.1144/SP361.7
Goff, J., Chagué-Goff, C., Dominey-Howes, D., McAdoo, B., Cronin, S., Bonté-Grapetin, M., et al. (2011a). Palaeotsunamis in the Pacific Islands. Earth-Science Rev. 107, 141–146. doi:10.1016/j.earscirev.2010.10.005
Goff, J., Chagué-Goff, C., and Dominey-Howes, D. (2011b). “Tracking the Extent of the Kuwae Tsunami,” in Geophysical Research Abstracts (Vienna: EGU2011-85).
Goff, J., and Chagué-Goff, C. (2015). Three Large Tsunamis on the Non-subduction, Western Side of New Zealand over the Past 700years. Mar. Geology. 363, 243–260. doi:10.1016/j.margeo.2015.03.002
Goff, J., Charley, D., Haruel, C., and Bonté-Grapentin, M. (2008). “Preliminary Findings of the Geological Evidence and Oral History O F Tsunamis in Vanuatu,” in South Pacific Applied Geoscience Commission (SOPAC) Technical Report (Suva: SOPAC). 416.
Goff, J., McFadgen, B. G., Chagué-Goff, C., and Nichol, S. L. (2012). Palaeotsunamis and Their Influence on Polynesian Settlement. The Holocene 22, 1067–1069. doi:10.1177/0959683612437873
Goff, J., and Nunn, P. D. (2016). Rapid Societal Change as a Proxy for Regional Environmental Forcing: Evidence and Explanations for Pacific Island Societies in the 14-15th Centuries. Isl. Arc 25, 305–315. doi:10.1111/iar.12117
Goff, J. R., and McFadgen, B. G. (2001). “Nationwide Tsunami during Prehistoric Maori Occupation, New Zealand,” in Proceedings of the International Tsunami Symposium 2001 (Seattle: NOAA/PEML), 469–476.
Hallock, P. (1984). Distribution of Selected Species of Living Algal Symbiont-Bearing Foraminifera on Two Pacific Coral Reefs. J. Foraminiferal Res. 14, 250–261. doi:10.2113/gsjfr.14.4.250
Hamacher, D. W. (2013). Recorded Accounts of Meteoric Events in the Oral Traditions of Indigenous Australians. Archaeoastronomy 25, 99–111.
Harrison, D. (1993). The limestone Resources of Tongatapu and Vava’u, Kingdom of Tonga. Nottingham: British Geological Survey.
Hartmann, M., Blunier, T., Brügger, S. O., Schmale, J., Schwikowski, M., Vogel, A., et al. (2019). Variation of Ice Nucleating Particles in the European Arctic over the Last Centuries. Geophys. Res. Lett. 46, 4007–4016. doi:10.1029/2019GL082311
Hohenegger, J., Yordanova, E., Nakano, Y., and Tatzreiter, F. (1999). Habitats of Larger Foraminifera on the Upper Reef Slope of Sesoko Island, Okinawa, Japan. Mar. Micropaleontology 36, 109–168. doi:10.1016/S0377-8398(98)00030-9
Ishizawa, T., Goto, K., Yokoyama, Y., and Goff, J. (2020). Dating Tsunami Deposits: Present Knowledge and Challenges. Earth-Science Rev. 200, 102971. doi:10.1016/j.earscirev.2019.102971
Kelfoun, K., and Druitt, T. H. (2005). Numerical Modeling of the Emplacement of Socompa Rock Avalanche, Chile. J. Geophys. Res. 110, 1–13. doi:10.1029/2005JB003758
Kelfoun, K., Giachetti, T., and Labazuy, P. (2010). Landslide-generated Tsunamis at Réunion Island. J. Geophys. Res. 115, 1381. doi:10.1029/2009JF001381
Kharif, C., and Pelinovsky, E. (2005). Asteroid Impact Tsunamis. Comptes Rendus Physique 6, 361–366. doi:10.1016/j.crhy.2004.12.016
King, D. N., and Goff, J. R. (2010). Benefitting from Differences in Knowledge, Practice and Belief: Māori Oral Traditions and Natural Hazards Science. Nat. Hazards Earth Syst. Sci. 10, 1927–1940. doi:10.5194/nhess-10-1927-2010
Leach, B. F., and Leach, H. M. (1979). Prehistoric Man in Palliser Bay. Wellington: National Museum of New Zealand.
Massoth, G., Baker, E., Worthington, T., Lupton, J., De Ronde, C., Arculus, R., et al. (2007). Multiple Hydrothermal Sources along the South Tonga Arc and Valu Fa Ridge. Geochem. Geophys. Geosyst. 8, a–n. doi:10.1029/2007GC001675
Monzier, M., Robin, C., and Eissen, J.-P. (1994). Kuwae (≈ 1425 A.D.): the Forgotten Caldera. J. Volcanology Geothermal Res. 59, 207–218. doi:10.1016/0377-0273(94)90091-4
Morton, A. E. (2003). “Evidence for Large Tsunami in the Tongan Islands,” in 99th Annual Cordilleran Section Meeting - Session 1 Geomorphology and Quaternary Geology, 4–6 Apr 2003 (Mexico: Puerto Vallarta, Jalisco).
Nandasena, N. A. K., Paris, R., and Tanaka, N. (2011). Reassessment of Hydrodynamic Equations: Minimum Flow Velocity to Initiate boulder Transport by High Energy Events (Storms, Tsunamis). Mar. Geology. 281, 70–84. doi:10.1016/j.margeo.2011.02.005
Nemeth, K., Cronin, S. J., and White, J. D. L. (2007). Kuwae Caldera and Climate Confusion. Togeoj 1, 7–11. doi:10.2174/1874262900701010007
Nomikou, P., Druitt, T. H., Hübscher, C., Mather, T. A., Paulatto, M., Kalnins, L. M., et al. (2016). Post-eruptive Flooding of Santorini Caldera and Implications for Tsunami Generation. Nat. Commun. 7, 13332. doi:10.1038/ncomms13332
Nott, J. (1997). Extremely High-Energy Wave Deposits inside the Great Barrier Reef, Australia: Determining the Cause-Tsunami or Tropical Cyclone. Mar. Geology. 141, 193–207. doi:10.1016/S0025-3227(97)00063-7
Nunn, P. D., and Pastorizo, R. (2007). Geological Histories and Geohazard Potential of Pacific Islands Illuminated by Myths. Geol. Soc. Lond. Spec. Publications 273, 143–163. doi:10.1144/GSL.SP.2007.273.01.13
Nunn, P. D. (2007). The A.D. 1300 Event in the Pacific Basin. Geographical Rev. 97, 1–23. doi:10.1111/j.1931-0846.2007.tb00277.x
Nunn, P. D. (2008). Vanished Islands and Hidden Continents of the Pacific. Honolulu: University of Hawaï Press.
Paris, R., Bravo, J. J. C., González, M. E. M., Kelfoun, K., and Nauret, F. (2017). Explosive Eruption, Flank Collapse and Megatsunami at Tenerife Ca. 170 Ka. Nat. Commun. 8, 1–8. doi:10.1038/ncomms15246
Paris, R. (2015). Source Mechanisms of Volcanic Tsunamis. Phil. Trans. R. Soc. A. 373, 20140380. doi:10.1098/rsta.2014.0380
Petchey, F., and Clark, G. (2011). Tongatapu Hardwater: Investigation into the 14C marine Reservoir Offset in Lagoon, Reef and Open Ocean Environments of a limestone Island. Quat. Geochronol. 6, 539–549. doi:10.1016/j.quageo.2011.08.001
Richter-Gravier, R. (2019). “Manu Narratives of Polynesia: a Comparative Study of Birds in 300 Traditional Polynesian Stories,” (New Zealand: University of Otago). PhD Thesis. Available at: http://hdl.handle.net/10523/9739.
Robin, C., Monzier, M., and Eissen, J.-P. (1994). Formation of the Mid-fifteenth century Kuwae Caldera (Vanuatu) by an Initial Hydroclastic and Subsequent Ignimbritic Eruption. Bull. Volcanol. 56, 170–183. doi:10.1007/BF00279602
Roy, P. S. (1990). The Morphology and Surface Geology of the Islands of Tongatapu and Vava’u, Kingdom of Tonga. Bull. Volcanol. 51, 170–183.
Self, S. (2006). The Effects and Consequences of Very Large Explosive Volcanic Eruptions. Phil. Trans. R. Soc. A. 364, 2073–2097. doi:10.1098/rsta.2006.1814
Sigl, M., McConnell, J. R., Layman, L., Maselli, O., McGwire, K., Pasteris, D., et al. (2013). A New Bipolar Ice Core Record of Volcanism from WAIS Divide and NEEM and Implications for Climate Forcing of the Last 2000 Years. J. Geophys. Res. Atmos. 118, 1151–1169. doi:10.1029/2012JD018603
Simkin, T., and Fiske, R. (1983). Krakatau 1883 : The Volcanic Eruption and its Effects. Smithsonia. Washington D.C: Smithsonian Institution Press.
Spennemann, D. H. (1997). “A Holocene Sea-Level History for Tongatapu, Kingdom of Tonga,” in Coastal and Environmental Geoscience Studies of the Southwest Pacific Islands. Editor A. M. Sherwood, 115–152.
Spennemann, D. H. (1989). “’Ata ’a Tonga Mo ’ata ’o Tonga: Early and Later Pre-history of the Tongan Islands,” (Camberra: Australian National University). PhD dissertation.
Spiske, M., Böröcz, Z., and Bahlburg, H. (2008). The Role of Porosity in Discriminating between Tsunami and hurricane Emplacement of Boulders - A Case Study from the Lesser Antilles, Southern Caribbean. Earth Planet. Sci. Lett. 268, 384–396. doi:10.1016/j.epsl.2008.01.030
Walter, R., Buckley, H., Jacomb, C., and Matisoo-Smith, E. (2017). Mass Migration and the Polynesian Settlement of New Zealand. J. World Prehist 30, 351–376. doi:10.1007/s10963-017-9110-y
Walters, A. L., Phillips, J. C., Brown, R. J., Field, M., Gernon, T., Stripp, G., et al. (2006). The Role of Fluidisation in the Formation of Volcaniclastic Kimberlite: Grain Size Observations and Experimental Investigation. J. Volcanology Geothermal Res. 155, 119–137. doi:10.1016/j.jvolgeores.2006.02.005
Wang, X., and Power, W. L. (2011). COMCOT : A Tsunami Generation Propagation and Run-Up Model. Lower Hutt: GNS Science, 121.
Witter, J. B., and Self, S. (2006). The Kuwae (Vanuatu) Eruption of AD 1452: Potential Magnitude and Volatile Release. Bull. Volcanol. 69, 301–318. doi:10.1007/s00445-006-0075-4
Keywords: tsunami, megablock, sedimentology, radiocarbon dating, bioclasts, legend, southwest Pacific
Citation: Lavigne F, Morin J, Wassmer P, Weller O, Kula T, Maea AV, Kelfoun K, Mokadem F, Paris R, Malawani MN, Faral A, Benbakkar M, Saulnier-Copard S, Vidal CM, Tu’I’afitu T, Kitekei’aho F, Trautmann M and Gomez C (2021) Bridging Legends and Science: Field Evidence of a Large Tsunami that Affected the Kingdom of Tonga in the 15th Century. Front. Earth Sci. 9:748755. doi: 10.3389/feart.2021.748755
Received: 28 July 2021; Accepted: 09 November 2021;
Published: 20 December 2021.
Edited by:
Felix Riede, Aarhus University, DenmarkReviewed by:
Paul William Taylor, Independent researcher, East Maitland, NSW, AustraliaChris Ballard, Australian National University, Australia
Copyright © 2021 Lavigne, Morin, Wassmer, Weller, Kula, Maea, Kelfoun, Mokadem, Paris, Malawani, Faral, Benbakkar, Saulnier-Copard, Vidal, Tu’I’afitu, Kitekei’aho, Trautmann and Gomez. This is an open-access article distributed under the terms of the Creative Commons Attribution License (CC BY). The use, distribution or reproduction in other forums is permitted, provided the original author(s) and the copyright owner(s) are credited and that the original publication in this journal is cited, in accordance with accepted academic practice. No use, distribution or reproduction is permitted which does not comply with these terms.
*Correspondence: Franck Lavigne, ZnJhbmNrLmxhdmlnbmVAdW5pdi1wYXJpczEuZnI=