- 1Animal Reproduction, Gynaecology and Obstetrics, ICAR-National Dairy Research Institute, Karnal, India
- 2Molecular Reproduction Lab, Animal Biotechnology Center, ICAR-National Dairy Research Institute, Karnal, India
- 3Proteomics and Cell Biology Lab, Animal Biotechnology Center, ICAR-National Dairy Research Institute, Karnal, India
- 4Division of Proteomics of Stem Cells and Cancer, German Cancer Research Center (DKFZ), Heidelberg, Germany
- 5Animal Biotechnology Centre, ICAR-National Dairy Research Institute, Karnal, India
Accurate determination of estrus is essentially required for efficient reproduction management of farm animals. Buffalo is a shy breeder and does not manifest overt signs of estrus that make estrus detection difficult resulting in a poor conception rate. Therefore, identifying estrus biomarkers in easily accessible biofluid such as saliva is of utmost interest. In the current study, we generated saliva proteome profiles during proestrus (PE), estrus (E), metestrus (ME), and diestrus (DE) stages of the buffalo estrous cycle using both label-free quantitation (LFQ) and labeled (TMT) quantitation and mass spectrometry analysis. A total of 520 proteins were identified as DEPs in LFQ; among these, 59 and four proteins were upregulated (FC ≥ 1.5) and downregulated (FC ≤ 0.5) during E vs. PE, ME, and DE comparisons, respectively. Similarly, TMT-LC-MS/MS analysis identified 369 DEPs; among these, 74 and 73 proteins were upregulated and downregulated during E vs. PE, ME, and DE stages, respectively. Functional annotations of GO terms showed enrichment of glycolysis, pyruvate metabolism, endopeptidase inhibitor activity, salivary secretion, innate immune response, calcium ion binding, oocyte meiosis, and estrogen signaling. Over-expression of SERPINB1, HSPA1A, VMO1, SDF4, LCN1, OBP, and ENO3 proteins during estrus was further confirmed by Western blotting. This is the first comprehensive report on differential proteome analysis of buffalo saliva between estrus and non-estrus stages. This study generated an important panel of candidate proteins that may be considered buffalo estrus biomarkers which can be applied in the development of a diagnostic kit for estrus detection in buffalo.
1 Introduction
Saliva is an important diagnostic body fluid since it contains explicit biomolecules such as metabolites, hormones, mRNA, miRNA, DNA, and proteins that may be a good source of biomarkers of health and disease (Levine, 1993). Saliva consists of secretion from serous and mucous acinar cells of salivary glands and a small portion originating from the blood (Edgar, 1992; Pedersen et al., 2002). Dietary and physiological variations bring changes in the salivary composition (Prout and Hopps., 1970; Mass et al., 2002). Furthermore, its stable nature, high biological half-life, and non-invasive approach make it preferable to other body fluids for biomarker discovery (Li et al., 2004; Archunan et al., 2014; Shashikumar et al., 2017). Proteomics using a mass spectrometer enables the identification of new biomarker proteins in a variety of bodily fluids, including saliva (Almeida et al., 2021). In recent past years, salivary proteomics has led to the discovery of biomarkers for several disease conditions such as oral squamous cell carcinoma (OSCC) (Jou et al., 2014; Wang et al., 2015), autoimmune disease (Castagnola et al., 2017), and genetic diseases such as Down’s syndrome (Cabras et al., 2013) in humans. Unlike human saliva, the characterization of salivary proteins of farm animals has been little studied. A global proteome analysis of bovine saliva was carried out by Ang et al. (2011) using both non-targeted and targeted proteomics techniques to identify only extracellular proteins. They identified 402 proteins and 45 N-linked glycoproteins in bovine saliva with enrichment of low-abundance proteins.
Buffalo (Bubalus bubalis) is a premier livestock species that contributes immensely to milk and meat production (20th Livestock Census, 2019). Nevertheless, specific inherent reproductive problems such as delayed puberty, silent or poor expression of estrus signs, and seasonal anestrus compromise its reproductive efficiency and production potential. Accurate and efficient identification of estrus is essential for successful conception and efficient reproduction management of farm animals. However, estrus detection is difficult in buffaloes because of non-manifestation of overt signs of estrus and higher incidences (29%) of silent estrus, especially during the summer season (Singh et al., 2000; Roy and Prakash, 2009). This leads to difficulty in estrus detection, improper insemination time, and conception failure in buffaloes (Sharma et al., 2008; Das and Khan, 2010). In addition, various estrus detection tools, such as teaser bull parading, tail painting, KaMar heat mounting detector, behavioral monitoring using a closed circuit television, and activity monitor using a pedometer, were used in buffaloes that are not very efficient since their sensitivity and specificity vary widely (Selvam and Archunan 2017). Therefore, discovering estrus biomarkers in easily accessible body fluids such as saliva is of utmost importance for developing an easy, reliable, and accurate estrus detection method for buffaloes.
Saliva possesses biomarker potential to detect fertile periods in mammals. Saibaba et al. (2021) reported mass spectrometric analysis of salivary proteome profiles of women during the fertile phase of the menstruation cycle as characterized by mass spectrometry. They identified 16 unique and differentially expressed proteins during the ovulatory phase; among them, cystatin-S offers a biomarker potential. Similarly, Muthukumar et al. (2014a) reported proteome profiling of buffalo saliva during the estrous cycle by employing in-gel digestion followed by LC-MS/MS analysis. They identified 179 proteins collectively during proestrus (PE), estrus (E), and diestrus (DE) stages, and 37 proteins are found exclusively during estrus. Among estrus-specific proteins, β-enolase, toll-like receptor (TLR)-4, clusterin, lactoperoxidase, serotransferrin, TGM3, and UBA6 proteins are the most predominant. Our previous study reported global proteome profiling of buffalo saliva during the estrous cycle (Shashikumar et al., 2018) and identified 275, 371, 304, and 565 proteins with ≥2 peptides during PE, E, ME, and DE stages, respectively. Among these, 62 proteins are identified exclusively during the estrus stage, and heat shock 70-kDa protein 1A, 17-beta-hydroxysteroid dehydrogenase type 1, inhibin beta A chain, and testin proteins are found to be the most predominant during estrus. These two studies concluded that salivary secretion contains specific proteins, and their expression varies according to the estrous cycle stages. However, differential proteome analysis using labeled quantitation {tandem mass tag (TMT)} and label-free quantitation (LFQ) coupled to mass spectrometry for estrus biomarker discovery, which is lacking in buffaloes. Therefore, the aim of the present study was to identify candidate estrus biomarkers in saliva of buffaloes. We hypothesized that identifying differentially expressed proteins (DEPs) associated with estrus could lead to the identification of estrus biomarkers in buffaloes. Thus, for the first time, we identified differentially expressed estrus-associated proteins in buffalo saliva by employing both label-free quantitation (LFQ) and labeled quantitation (TMT) coupled to high-resolution mass spectrometry (LC-MS/MS) and their validation using Western blotting.
2 Materials and Methods
2.1 Experimental Animals
The study was carried out at the Livestock Research Centre, ICAR-National Dairy Research Institute, Karnal, Haryana. Healthy pluriparous Murrah buffaloes (n = 15) of 2–4 parity maintained under iso-managerial conditions were included for the study. The nutrient requirement of the animals was provided as per National Research Council (NRC) standards. After completing the voluntary waiting period, all animals underwent gynecological examination using per-rectal examination and transrectal ultrasonography (Model UST-5820-5). For estrus induction, buffaloes with functional corpus luteum (CL) on the ovary are administered with PGF2α (Vetmate; 500 µg I/M). Samples were collected from the next spontaneous estrous cycle, and stages of the estrous cycle were categorized as proestrus (PE; day 2 to 1 before the onset of estrus), estrus (E; day 0), metestrus (ME; day+3), and diestrus (DE; day +10). All the sampling was completed during the breeding season, that is, from November to February. All the experiments conducted in this study were approved by the Institute Animal Ethics Committee (42-IAEC-18-9).
2.2 Estrus Detection and Confirmation
Estrus in buffaloes was detected by physical observation of estrus signs. Teaser bull parading was carried out twice a day, that is, daily in the morning 5–6 AM and evening 5–6 PM for exhibition of estrus signs. The following estrus signs were observed: standing to be mounted, sniffing/licking the vulva, chin resting, flehmen’s reaction, mounting on or by other buffaloes, restlessness, bellowing, and frequent micturition. The onset of estrus is marked by expression of a typical estrus sign, that is, standing to be mounted behavior (standing estrus). The onset of estrus was further confirmed by reproductive tract examination (uterine horn tonicity, cervical relaxation, tumefaction of vulva, hyperemia, or reddening of the vulvar mucous membrane), biochemical parameters (cervicovaginal mucus crystallization, fluidity, and spinnbarkeit value (Verma et al., 2014)), and progesterone hormone estimation in blood serum. Transrectal ultrasonography (USG) using a real-time B-mode scanner equipped with a 7.5 MHz rectal probe was used to confirm further presence of dominant follicle (DF; ≥ 10 mm) and absence of CL during the follicular phase (proestrus and estrus) and the onset of ovulation and presence of CL during the luteal phase. Progesterone hormone concentration was estimated in serum samples using an ELISA kit (Cayman Chemical, United States) to confirm the stages of the estrous cycle.
2.3 Sample Collection and Processing
Saliva samples were collected from the lower jaw of the mouth of the animal by the direct aspiration method using a 20-ml syringe without a needle in sterile polypropylene tubes (Thermo Fisher Scientific, NY). All the sampling was performed in the early morning (6–7 AM) before feeding, and samples after collection were immediately transported to the laboratory on ice. Samples were centrifuged at 12,000 rpm at 4°C for 30 min to eliminate any particulate matter or feed debris. Supernatant was collected in another 1.5-ml Eppendorf tube, and protease and phosphatase inhibitor (1 × MS-SAFE; Sigma-Aldrich, United States) was added to prevent protein degradation and stored at −80°C until further analysis. Saliva samples from animals wherein estrus and non-estrus stages confirmed through various methods were selected and processed further for proteomics analysis.
2.4 Quantification and SDS-PAGE Profiling of Saliva Proteins During Different Stages of the Estrous Cycle
Out of 15 buffaloes, seven animals showing all the cardinal signs of estrus confirmed by gynecological examination, USG, cervicovaginal mucus (CVM) parameters, and progesterone estimation were selected for further proteomics study. The protein concentration of neat saliva samples from PE (n = 7), E (n = 7), ME (n = 7), and DE (n = 7) stages were determined with a Bradford assay. Then, salivary proteins, that is, 25 µg from all stages of the estrous cycle, were taken, and initial protein profiling was carried using SDS-PAGE. Initially, proteins were resolved on a 4% stacking and 12% separating acrylamide–bis-acrylamide gel at 15 mA for 3 h using the mini-protean vertical electrophoresis system (Bio-Rad, United States). The gels were stained with Coomassie brilliant blue G (CBB) at room temperature for 1 h, de-stained for 2 h, and scanned using an Epson scanner (GE, Healthcare, United States). The initial protein profiles were identified by running the saliva proteins with a reference protein marker (RTU-BLUeye prestained protein ladder). Based on the intactness and uniformity of the bands, saliva samples from PE, E, ME, and DE stages of five animals were selected for further in-solution digestion and label-free quantification (LFQ) and labeled quantitation using TMT labels and LC-MS/MS analysis.
2.5 Label-Free Quantitation
2.5.1 Trypsin In-Solution Digestion of Proteins for LC-MS/MS Analysis
Neat saliva protein of 50 µg from PE, E, ME, and DE stages were taken from five animals, and all samples were processed separately for in-sol digestion. In brief, samples were dissolved in dissolution buffer (50 mM ammonium bicarbonate) and reduced with 50 mM dithiothreitol (Sigma-Aldrich, United States) at 50°C for 45 min. Further alkylation was carried out using 100 mM IAA at room temperature in the dark for 15 min. Trypsin digestion (Promega, Madison, WI) at 1:20 (enzyme: protein) of protein samples was carried out at 37°C for overnight, followed by quenching with 10% trifluoroacetic acid. Furthermore, tryptic-digested samples were vacuum-dried (SpeedVac concentrator, Thermo Fisher Scientific, United States) and desalted using Pierce® C18 Spin Columns (Thermo Fisher Scientific, United States). Desalted peptide samples were vacuum-dried using SpeedVac and reconstituted in 0.1% formic acid. Finally, all 20 samples from five animals of four stages were subjected to LC-MS/MS analysis.
2.6 Labeled Quantitation Using TMT Labels
Saliva proteins (75 µg) from each stages of the estrous cycle, that is, PE, E, ME, and DE from five animals were pooled. Proteins were dissolved in dissolution buffer (50 mM ammonium bicarbonate), followed by reduction (50 mM dithiothreitol) at 50°C for 45 min s. Alkylation of cysteine residues was carried out using 100 mM IAA at room temperature in the dark for 15 min s, followed by trypsin digestion at 1:20 (enzyme: protein) at 37°C for overnight. Peptides of PE, E, ME, and DE stages were labeled with 126, 127, 128, and 129 TMT labels, respectively, using TMT 6-plex (Thermo Fisher Scientific, Germany), according to the manufacturer’s protocol. Peptides labeled with TMT tags were incubated for 3 h, quenched (5% hydroxylamine), vacuum-dried, and fractionated into eight fractions using a Pierce™ high pH reversed-phase peptide fractionation kit (Thermo Fisher Scientific, China), according to the manufacturer’s protocol. Furthermore, the fractions were vacuum-dried and reconstituted in 0.1% formic acid and subjected to LC-MS/MS analysis.
2.7 ESI–LC-MS/MS Analysis
Peptide samples were analyzed using an EASY-nLC 1,000 mass spectrometer (Thermo Fisher Scientific, United States) coupled with Thermo Fisher Q Exactive equipped with nano-ESI. The peptide mixture of 1 µg was resolved using a 25-cm PicoFrit column (360 µm outer diameter, 75 µm inner diameter, and 10 µm tip) filled with 1.8 µm of C18 resin (Dr. Maisch, Germany). The peptides were loaded with buffer A and eluted with a 0–40% gradient of buffer B (95% ACN and 0.1% formic acid) at a 300 nl/min flow rate for 100 min. MS data were acquired using a data-dependent top 10 method dynamically choosing the most abundant precursor ions from the survey scan.
2.8 Data Processing
The MS/MS data were searched and analyzed with Proteome Discoverer (v2.2) against the UniProt Bos taurus reference proteome database. In Proteome Discoverer, the Sequest search engine was used; the precursor and fragment mass tolerances were set at 10 ppm and 0.5 Da, respectively. The protease used to generate peptides, that is, enzyme specificity, was set at trypsin/P (cleavage at the C terminus of “K/Rˮ: unless followed by “Pˮ) along with a maximum missed cleavages value of two. The “ion score cutoff” was set to 20, thereby eliminating the lowest quality matches and minimum peptide length as six amino acid residues. Carbamidomethyl on cysteine was set at fixed modification and oxidation of methionine, and N-terminal acetylation was considered variable modifications for database search. The maximal number of modifications per peptide was set at 6. The minimum peptide length parameter was set to 6, and the “peptide” re-quantification function was enabled. Both peptide spectra match, and the protein false discovery rate (FDR) was set to 0.01. The decoy-reversed sequences database was included for the calculation of the FDR.
2.9 Bioinformative Analysis and Network Generation
Protein class analysis of the differentially expressed proteins based on molecular function, biological process, and cellular component was performed using Cytoscape ClueGo plug-in (v.3.7.2). A protein–protein interaction (PPI) network was constructed with the Search Tool for the Retrieval of Interacting Genes (STRING) database (v.11.5) and Cytoscape. A hypergeometric test (p < 0.05) was used in statistical analysis in the ClueGO tool. Furthermore, the online KEGG (Kyoto Encyclopedia of Genes and Genomes) pathway database was used for biological interpretation of higher-level systemic functions.
2.10 Western Blot Analysis
A quantity of 40 µg salivary proteins from four stages was loaded in a 12% SDS-PAGE gel and then transferred onto a polyvinylidene difluoride (PVDF) membrane using a semidry Western blot transfer system (Invitrogen, United States). The membrane was incubated with 5% BSA in TBST buffer (10 mM Tris, 150 mM NaCl, 0.05% Tween 20, and 5% BSA, pH 7.60) as blocking solution overnight at 4°C. After blocking, the membrane was incubated separately with seven primary antibodies: SERPINB1 (Aviva Systems Biology, San Diego, United States; 1:5,000), HSPA1A (Sigma-Aldrich, United States; 1:5,000), ENO3 (Sigma-Aldrich, MO, United States; 1:1,250), LCN1 (MyBiosource, CA, United States; 1:1,000), SDF4 (Aviva Systems Biology, San Diego, United States; 1:5,000), VMO1 (Thermo Fischer Scientific, United States; 1:5,000), and beta-actin (Sigma-Aldrich, MO, United States; 1:1,667) at room temperature for 1 h. Subsequently, the membranes were washed three times with TBST followed by incubation with horseradish peroxidase–conjugated secondary antibody (diluted 1:4,000, Sigma-Aldrich, United States) for 1 h at room temperature. The membranes were dried and developed using an X-ray film with Clarity Western ECL substrate (Bio-Rad, United States). The band intensity of the protein expression was normalized with beta-actin as an internal control, and values were analyzed using one way ANOVA with SPSS Inc (2007) after normalization.
3 Results
3.1 Estrus Detection and Confirmation
Accurate and efficient identification of estrus is essential for successful conception and efficient breeding management of farm animals including cattle and buffaloes. Hence, the onset of estrus in buffaloes was determined by physical signs and confirmed by gynecological examination of reproductive tract, transrectal USG, biochemical parameters (CVM fern pattern, spinnbarkeit value, and other parameters), and progesterone hormone estimation in blood serum. Behavioral signs of estrus are listed in Table 1; Figure 1. Major estrus signs exhibited by female buffaloes were cervicovaginal mucus discharge (89.9%), standing to be mounted (83.3%), bellowing (77.7%), frequent micturition (65.1%), hyperemia of the vulval mucous membrane (89.9%), and vulva tumefaction (72.2%). Further male behavior toward estrus buffaloes such as flehmen’s reaction (81.1%), sniffing the vulva (71.1%), and chin resting (68.2%) were also considered for estrus identification. Other estrus signs such as uterine horn tonicity, CVM crystallization, and spinnbarkeit value were considered for confirmation of estrus. Tonicity of uterine horns (94%) was mostly intense during the estrus stage (Table 2). The CVM crystallization/fern pattern was typical with scores 3 and 4 in 83.3% during estrus (Figures 2, 3). The spinnbarkeit value of CVM was the highest (21.30 ± 1.56 cm) during estrus (Figure 4). Transrectal USG revealed the presence of dominant follicle (DF) during the follicular phase, and the size of DF was 12.1 ± 0.03 mm and 13.5 ± 0.04 mm during PE and E, respectively (Figure 5). The absence of preovulatory follicle confirmed ovulation during the ME stage and presence of CL during the DE stage (Figure 5). The serum progesterone level was found to be lowest during E (0.35 ± 0.04 ng/ml, p < 0.05) and PE (0.54 ± 0.08 ng/ml) than that in ME (1.56 ± 0.14 ng/ml) and DE (3.79 ± 0.09 ng/ml) stages (Figure 6).
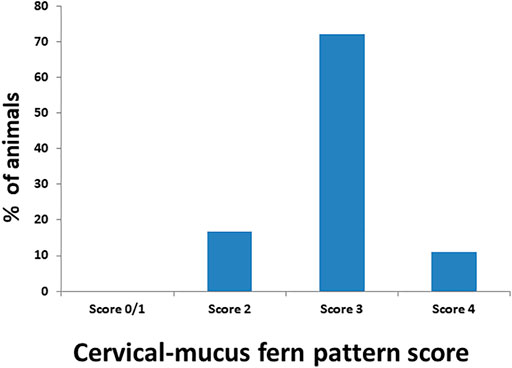
FIGURE 2. Estimation of the crystallization/fern pattern score of cervicovaginal mucus during estrus in buffaloes.
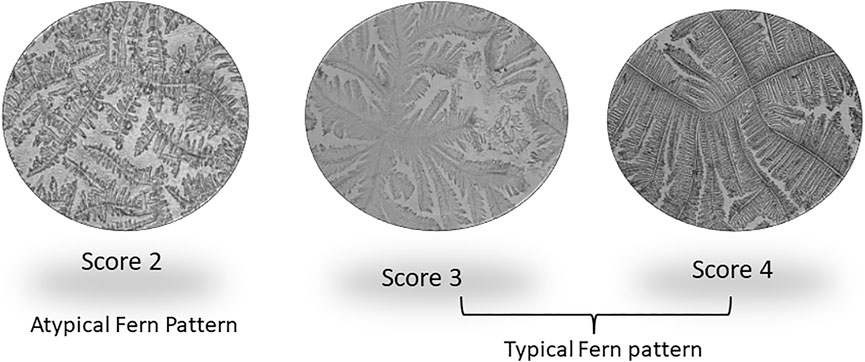
FIGURE 3. Different crystallization/fern patterns of cervicovaginal mucus observed during estrus in buffaloes.
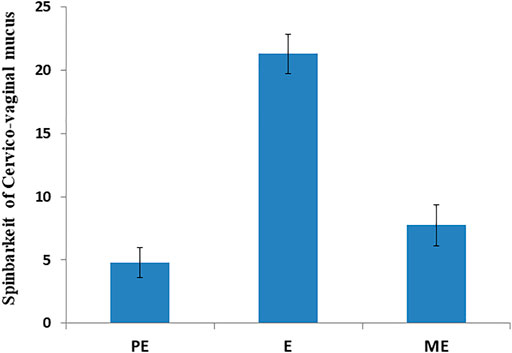
FIGURE 4. Spinnbarkeit value of cervicovaginal mucus during proestrus (PE), estrus (E), and metestrus (ME) stages of the buffalo estrous cycle.
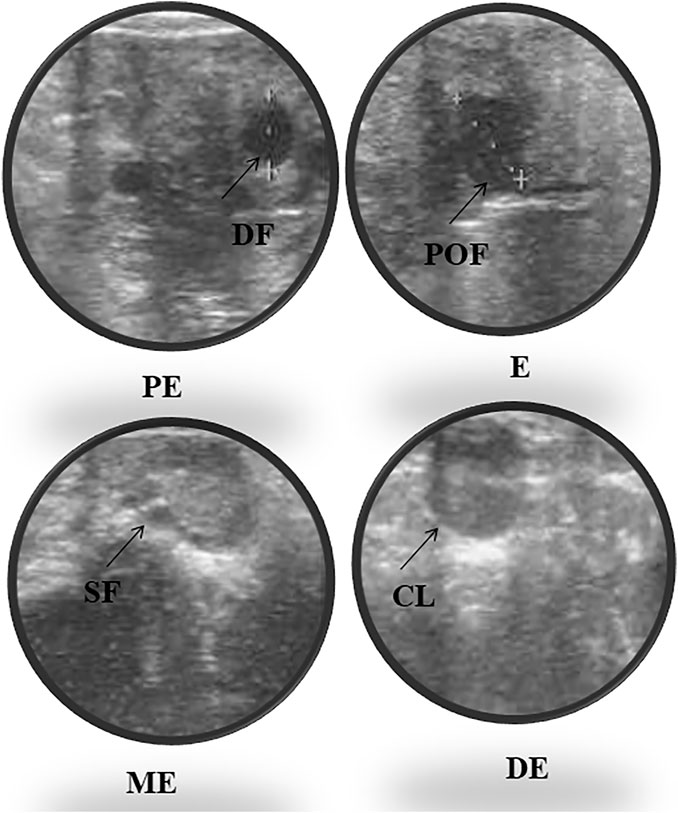
FIGURE 5. Ultrasonography of ovarian follicles and corpus luteum (CL) during proestrus (PE), estrus (E), metestrus (ME), and diestrus (DE) stages of the buffalo estrous cycle (DF, dominant follicle; SF, small follicle; POF, preovulatory follicle; CL, corpus luteum).
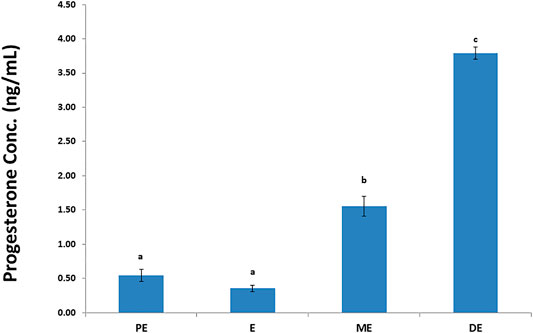
FIGURE 6. Progesterone hormone concentration (ng/ml) during proestrus (PE), estrus (E), metestrus (ME), and diestrus (DE) stages of the buffalo estrous cycle.
3.2 Quantification and SDS-PAGE of Salivary Proteins
The salivary proteins were quantified, and the concentration was in the range of 500–1,000, 400–1,000, 400–900, and 466–800 μg/ml during PE, E, ME, and DE stage, respectively. Furthermore, salivary proteins were separated using SDS-PAGE. A total of seven major and several minor bands were exhibited in the CBB-stained gel invariably among four stages of the estrous cycle and their molecular mass ranged from 17 to 245 kDa (Figure 7).
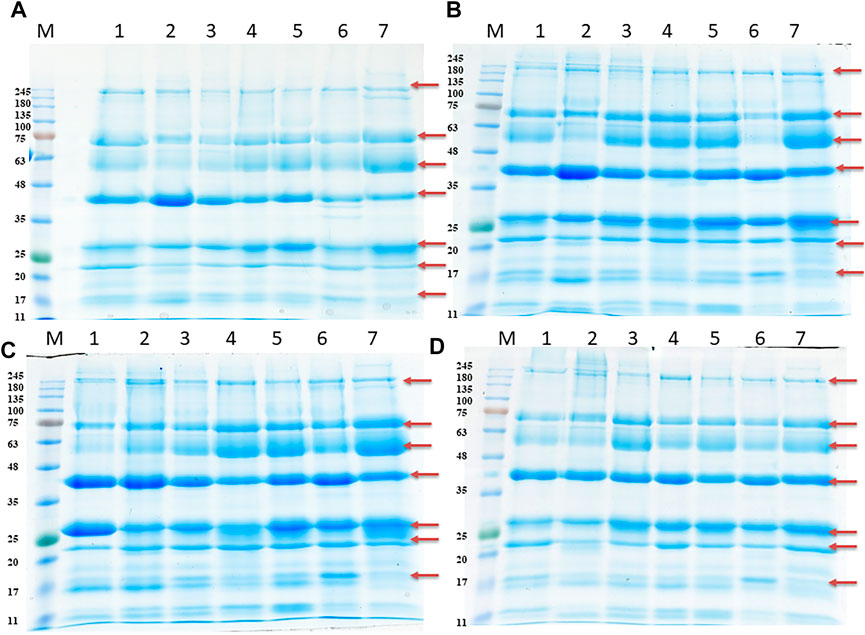
FIGURE 7. SDS-PAGE of salivary proteins during proestrus (PE) (A), estrus (E) (B), metestrus (ME) (C), and diestrus (DE) (D) stages of the buffalo estrous cycle. M, protein molecular markers; Lane 1-7 contains 25 μg protein from 7 individual buffaloes. Arrow indicates presence of 7 major bands.
3.3 LC-MS/MS Analysis and DEPs Comparisons
The LFQ-LC-MS/MS analysis identified 520 DEPs with 1% protein and peptide FDR cutoff. The detailed information related to accession, description, protein FDR confidence, peptide score, coverage %, peptides, PSM, unique peptides, protein groups, MW, calculated pI, Sequest score, gene name, and abundance of the identified proteins is shown in Supplementary Table S1. The number of upregulated and downregulated proteins was distinctly identified based on the fold-change criteria between E, PE, ME, and DE stages. The fold-change values were set between 0.5 and 1.5; the proteins expressed with values greater than 1.5 were upregulated, and the proteins expressed with values less than 0.5 were downregulated. The list of upregulated and downregulated proteins during E compared to other non-estrus stages (PE, ME, and DE) is listed in Table 3. By considering the fold-change range, a total of 59 proteins and four proteins were found to be upregulated and downregulated during E compared to PE, ME, and DE stages, respectively. Among the DEPs, few proteins with higher abundance at E than all other non-estrus stages and identified in all the biological replicate samples were gastric triacylglycerol lipase (LIPF; E/P: 33-fold, E/M: 38.8-fold, and E/D: 19-fold), peptidyl-prolyl cis-trans isomerase E (PPIE; E/P: 21-fold, E/M: 15-fold, and E/D: 25-fold), fatty acid–binding protein, (FABP3; E/P: 2.4-fold, E/M: 6.2-fold, and E/D: 10.8-fold), leukocyte elastase inhibitor (SERPINB1; E/P: 3-fold, E/M: 2.3-fold, and E/D: 3.4-fold), apolipoprotein A-I (APOA1; E/P: 1.9-fold, E/M: 9.7-fold, and E/D: 6.8-fold), heat shock 70-kDa protein 1A (HSPA1A; E/P: 1.5-fold, E/M: 2.3-fold, and E/D: 3.6-fold), and pyruvate kinase (PKLR; E/P: 5-fold, E/M: 2-fold, and E/D: 4.7-fold) were highly abundant proteins during E vs. PE, ME, and DE comparisons.
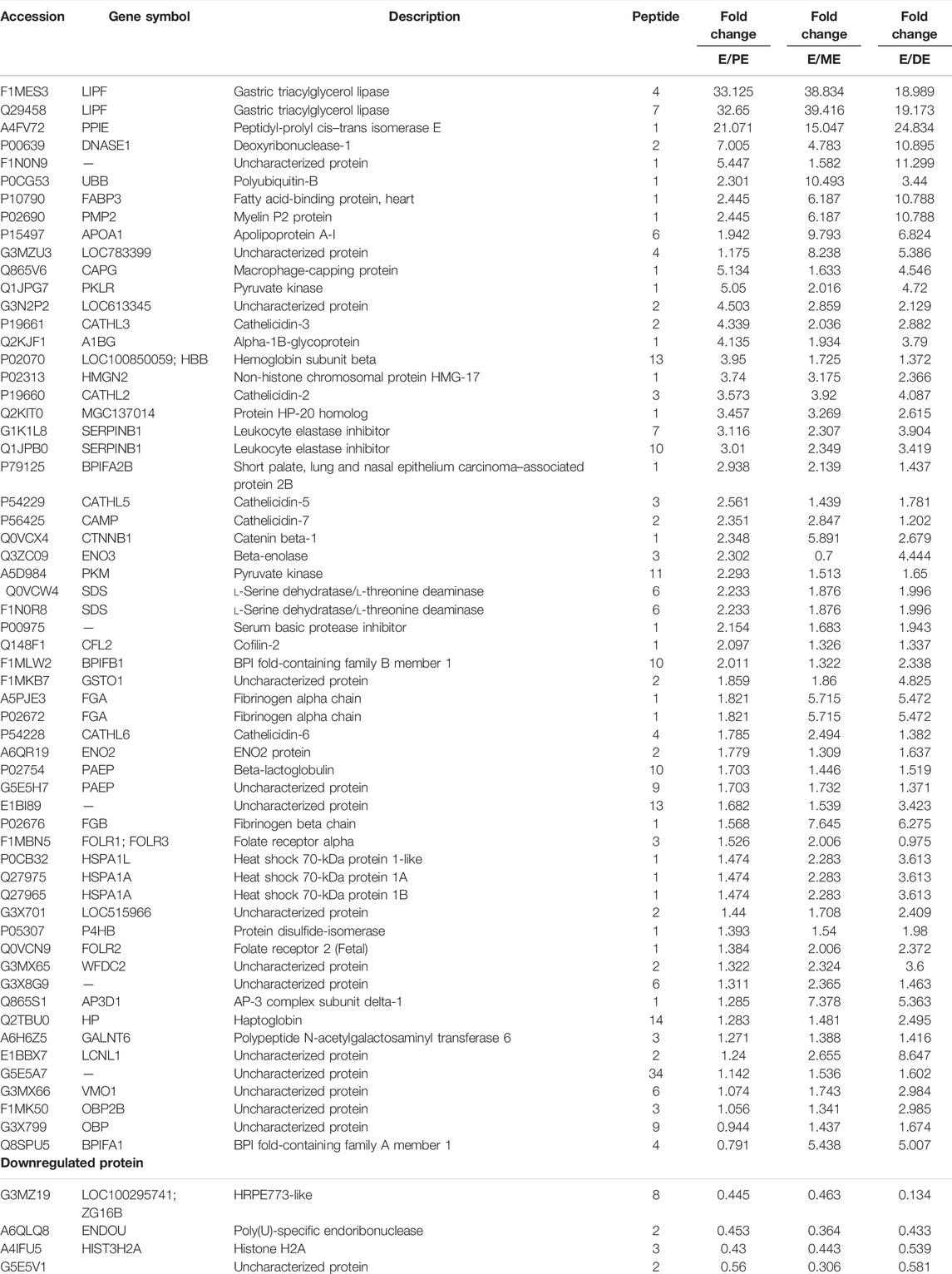
TABLE 3. List of differentially expressed proteins (DEPs) during estrus (E) vs. proestrus (PE), metestrus (ME), and diestrus (DE) stages using LFQ-LC-MS/MS analysis.
Similarly, TMT-LC-MS/MS analysis identified a total of 369 DEPs; among these 74 and 73 proteins were upregulated and downregulated during E compared to PE, ME, and DE stages, respectively. The detail information of identified proteins is listed in Supplementary Table S2. The list of upregulated and downregulated proteins during E than other non-estrus stages (PE, ME, and DE) is presented in Tables 4, 5, respectively. Among the upregulated DEPs, odorant-binding protein (OBP; E/P: 2.5-fold, E/M: 2.5-fold, and E/D: 69-fold), lipocalin 1 (LCN1; E/P: 7.3-fold, E/M: 14.3-fold, and E/D: 11.3-fold), odorant-binding protein-like (MGC151921; E/P: 1.8-fold, E/M: 2.8-fold, and E/D: 24-fold), lipocln_cytosolic_FA-bd_dom domain-containing protein (LOC104969973; E/P: 11.3-fold, E/M: 14.6-fold, and E/D: 5.8-fold), heat shock protein beta-1 (HSPB1; E/P: 12.5-fold, E/M: 15.5-fold, and E/D: 7.3-fold), galectin (LGALS7B; E/P: 5.3-fold, E/M: 6.6-fold, and E/D: 4.6-fold), metalloproteinase inhibitor 2 (TIMP2; E/P: 11.2-fold, E/M: 11.9-fold, and E/D: 2.7-fold), 45-kDa calcium-binding protein (SDF4; E/P: 62.6-fold, E/M: 88.6-fold, and E/D: 19.2-fold), peptidyl-prolyl isomerase (LOC526524; E/P: 13.6-fold, E/M: 17.3-fold, and E/D: 37.9-fold), and vitelline membrane outer layer 1 (VMO1; E/P: 62.6-fold, E/M: 88.6-fold, and E/D: 19.2-fold) were highly abundant proteins during E than other non-estrus stages, and few of these were also discovered in our previous investigation.
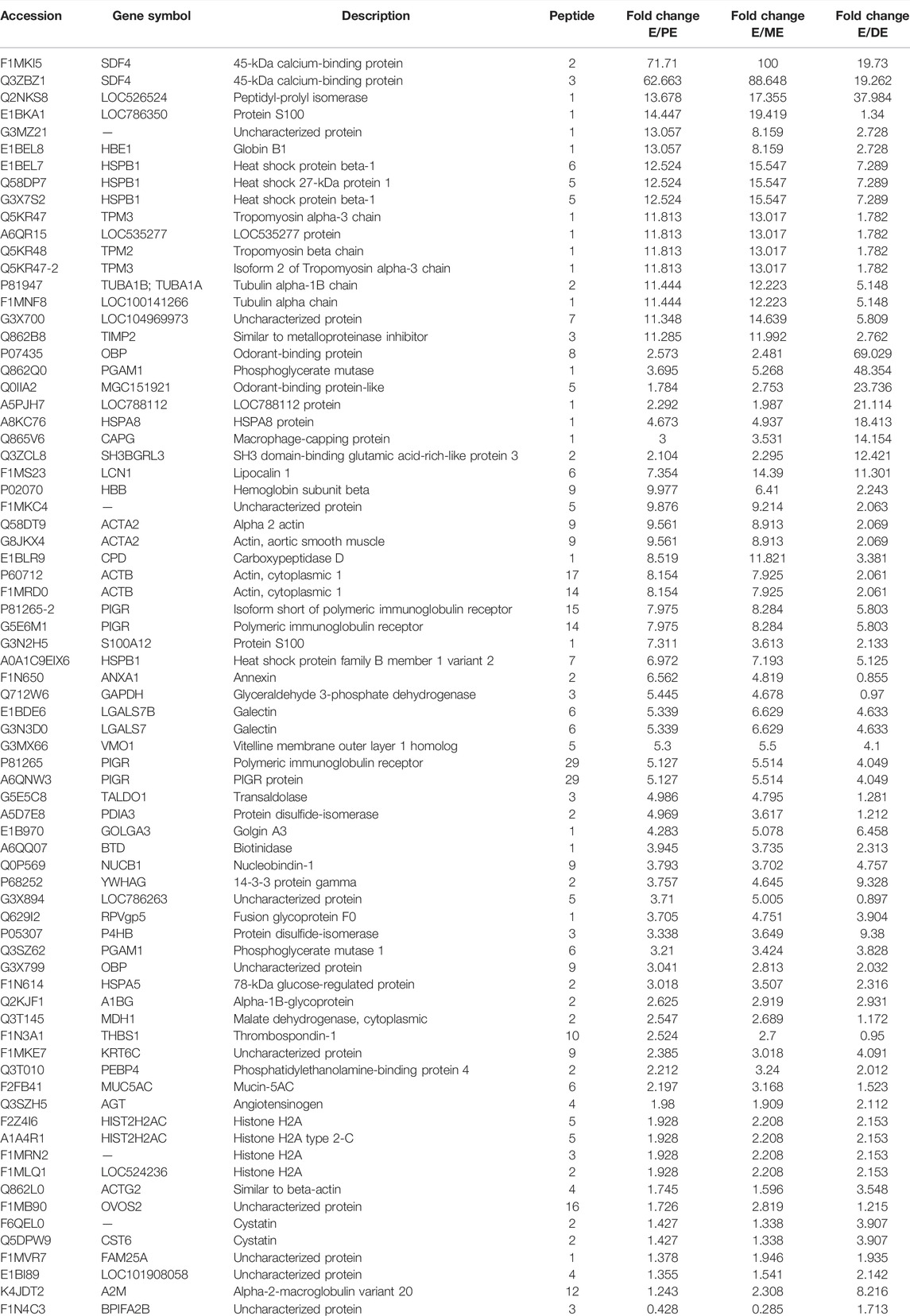
TABLE 4. List of upregulated proteins during estrus (E) vs. proestrus (PE), metestrus (ME), and diestrus (DE) stages using TMT-LC-MS/MS analysis.
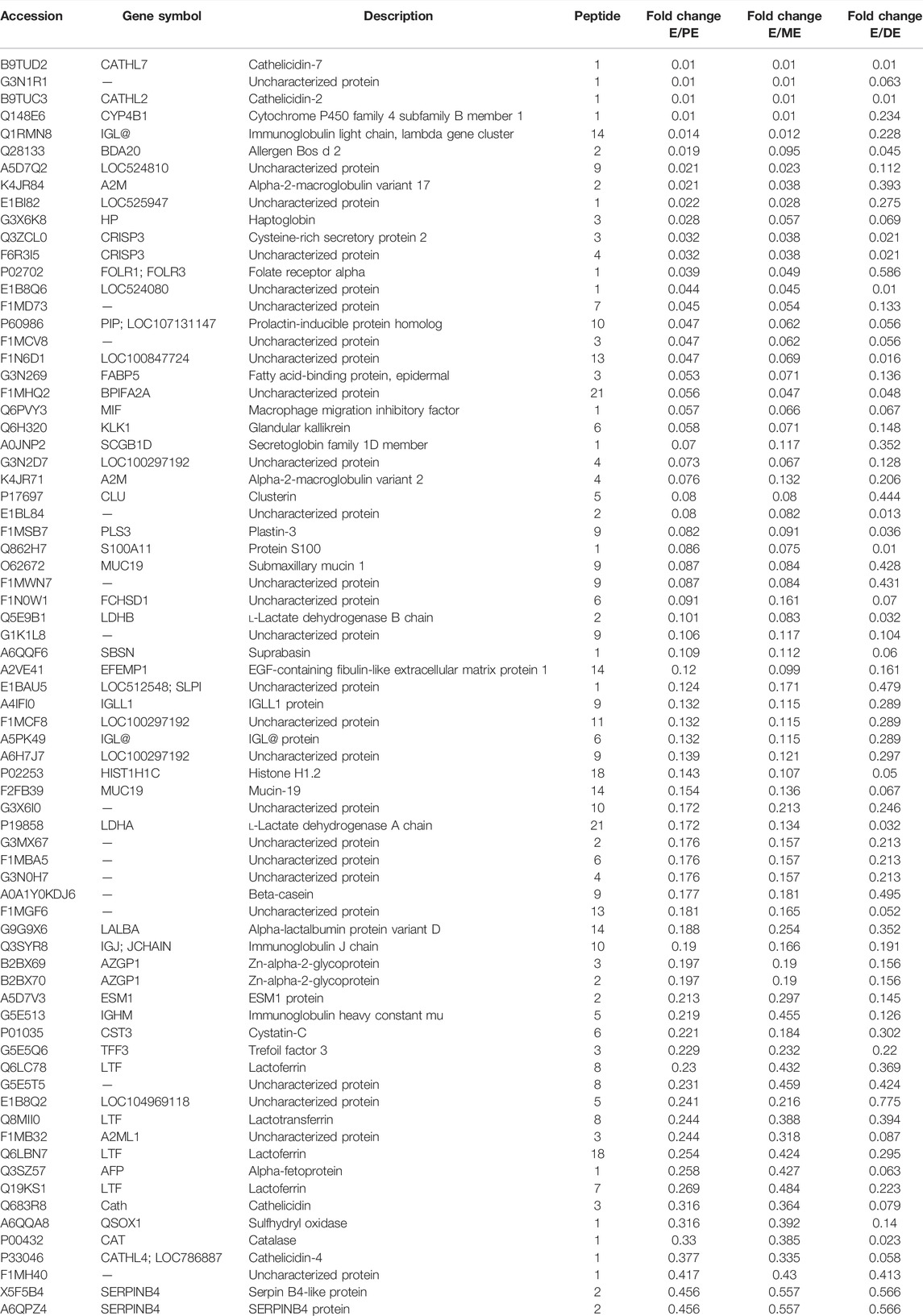
TABLE 5. List of downregulated proteins during estrus (E) vs. proestrus (PE), metestrus (ME), and diestrus (DE) stages using TMT-LC-MS/MS analysis.
Hierarchical clustering (Figure 8) and principal component analysis (PCA) (Figure 9) of proteins identified during four stages depicts clear cut differences in the expression of proteins among four stages. A Venn diagram analysis revealed that 214 proteins were commonly identified by both techniques, that is, TMT and LFQ; however, 223 and 78 proteins were specifically identified using LFQ and TMT methods, respectively (Figure 10).
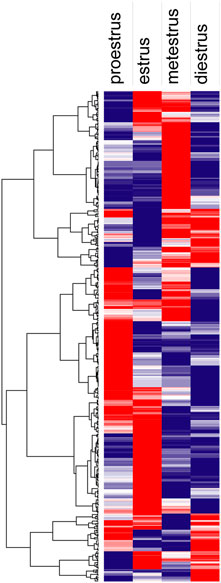
FIGURE 8. Hierarchical clustering analysis shows proteins identified during proestrus, estrus, metestrus, and diestrus stages (red to blue indicates highest to lowest abundance).
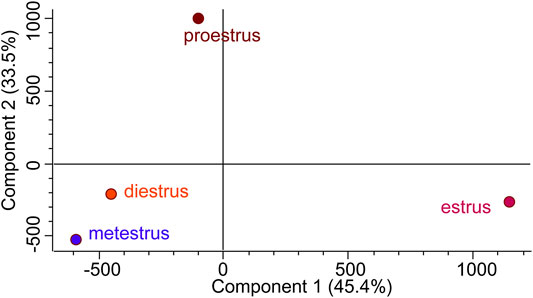
FIGURE 9. Principal component analysis (PCA) attributes the more difference between estrus and other non-estrus stages (proestrus, metestrus, and diestrus).
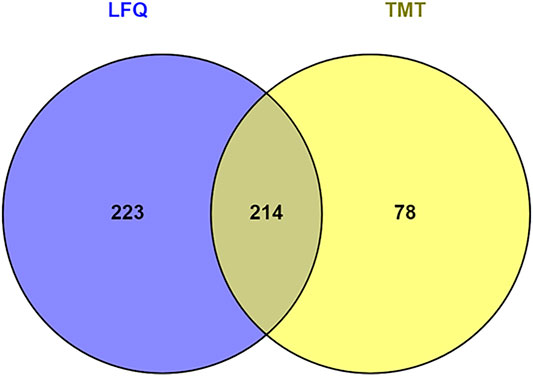
FIGURE 10. Venn diagram represents number of proteins identified by tandem mass tag (TMT) and label-free quantitation (LFQ).
3.4 Validation of DEPs Using Western Blotting
A total of six DEPs were selected for validation using Western blot analysis based on their involvement in different signaling pathways and their over-expression during E compared to all other non-estrus stages: SERPINB1, HSPA1A, SDF4, VMO1, ENO3, and LCN1. Interestingly, Western blot analysis confirmed the significantly higher expression of these proteins during E than PE, ME, and DE stages. The intensity of the protein expression was normalized with beta-actin as an internal control (Figure 11).
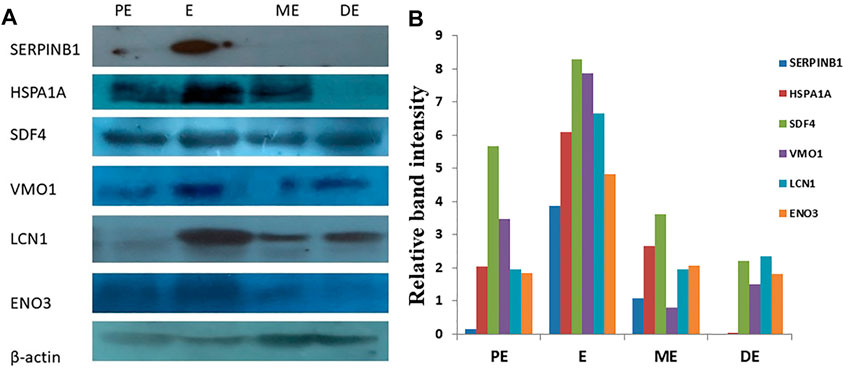
FIGURE 11. Western blot analysis for validation of differentially expressed upregulated proteins. (A) Over-expression of SERPINB1, HSPA1A, SDF4, VMO1, LCN1, and ENO3, during estrus (E) compared to proestrus (PE), metestrus (ME), and diestrus (DE) stages of the buffalo estrous cycle. (B) Band intensity was normalized against beta-actin as an internal control.
3.5 Gene Ontology Annotation of DEPs
Gene Ontology annotation of DEPs was performed on the Cytoscape ClueGo plug-in tool and presented in Figure 12. Based on the molecular function (Figure 12A, Supplementary Figure S1), the DEPs were found to be mainly enriched in cell adhesion molecule binding, endopeptidase regulator activity, protein heterodimerization activity, cadherin binding, cytoskeletal protein binding, oxidoreductase activity, hydrolase activity, transferase activity, fatty acid-binding, cis–trans isomerase activity, lysozyme activity, calcium ion binding, ion binding, antioxidant activity, and protein folding chaperone. Based on biological process (Figure 12B, Supplementary Figure S2), the DEPs were enriched in response to external biotic stimulus, metabolic process, regulation of endopeptidase activity, innate immune response, cytoskeleton organization, tissue homeostasis, generation of precursor metabolites and energy, ATP metabolic process, glycolytic process, chromatin assembly or disassembly, acute inflammatory response, cell redox homeostasis, and mucosal immune response. Based on the GO terms for cellular component (Figure 12C, Supplementary Figure S3), the DEPs belonged to endoplasmic reticulum lumen, plasma membrane, actin cytoskeleton, endoplasmic reticulum chaperone complex, myelin sheath, nucleosome, extracellular matrix, adherens junction, exosome, organelle, vesicle, and extracellular space.
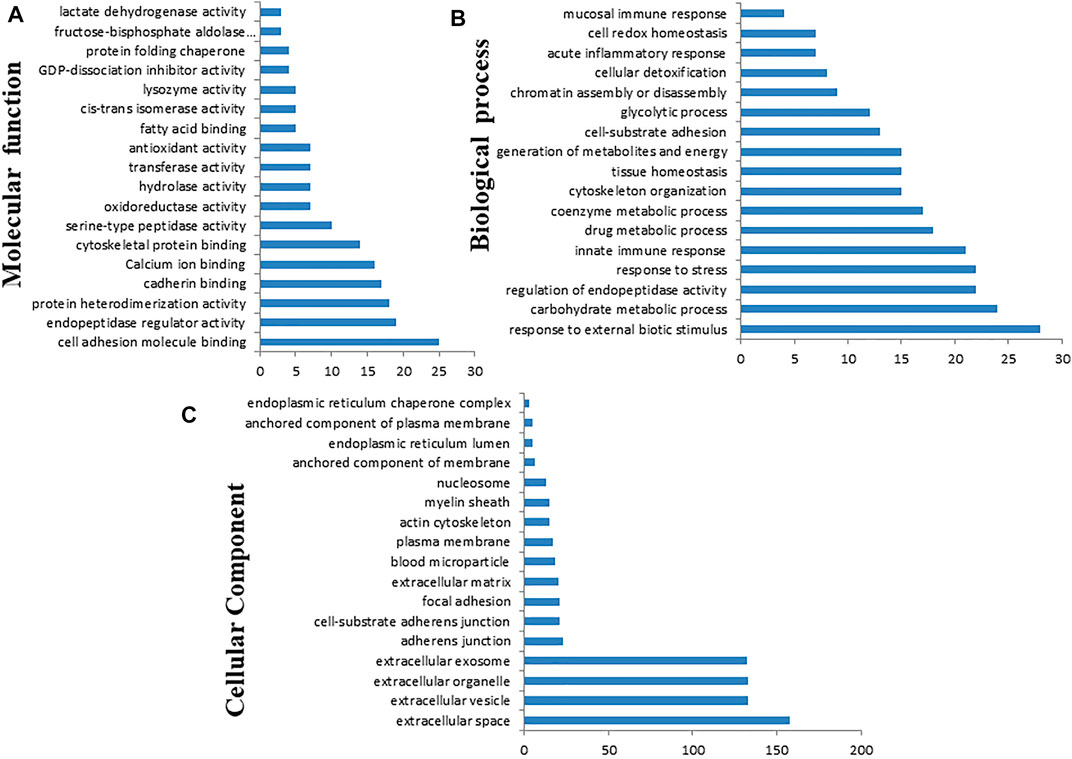
FIGURE 12. Gene Ontology (GO) classification of the differentially expressed proteins based on molecular function (A), biological process (B), and cellular component (C) using Cytoscape software with ClueGO plug-in.
3.6 Protein–Protein Interaction and Network Visualization
We performed protein–protein interaction (PPI) network analysis for DEPs using the STRING tool (v.11.5) with the Bos taurus database. The highly significant protein–protein interaction network (p < 1.0E-16) was created with 378 nodes, 810 edges, 4.29 average node degree, and 0.357 average local clustering coefficient (Figure 13A). A total of 31 clusters were found with the highest degree of connectivity among proteins. Furthermore, to explore the interactions between DEPs, we constructed protein networks using Cytoscape ClueGo plug-in tool (Figure 13B). A total 28 KEGG pathways were significantly enriched (p < 0.05), including glycolysis/gluconeogenesis, carbon metabolism, biosynthesis of amino acids, salivary secretion, fluid shear stress and atherosclerosis, glutathione metabolism, pentose phosphate pathway, innate immune response, antigen processing and presentation, oocyte meiosis, cell cycle, and estrogen signaling pathway (Table 6).
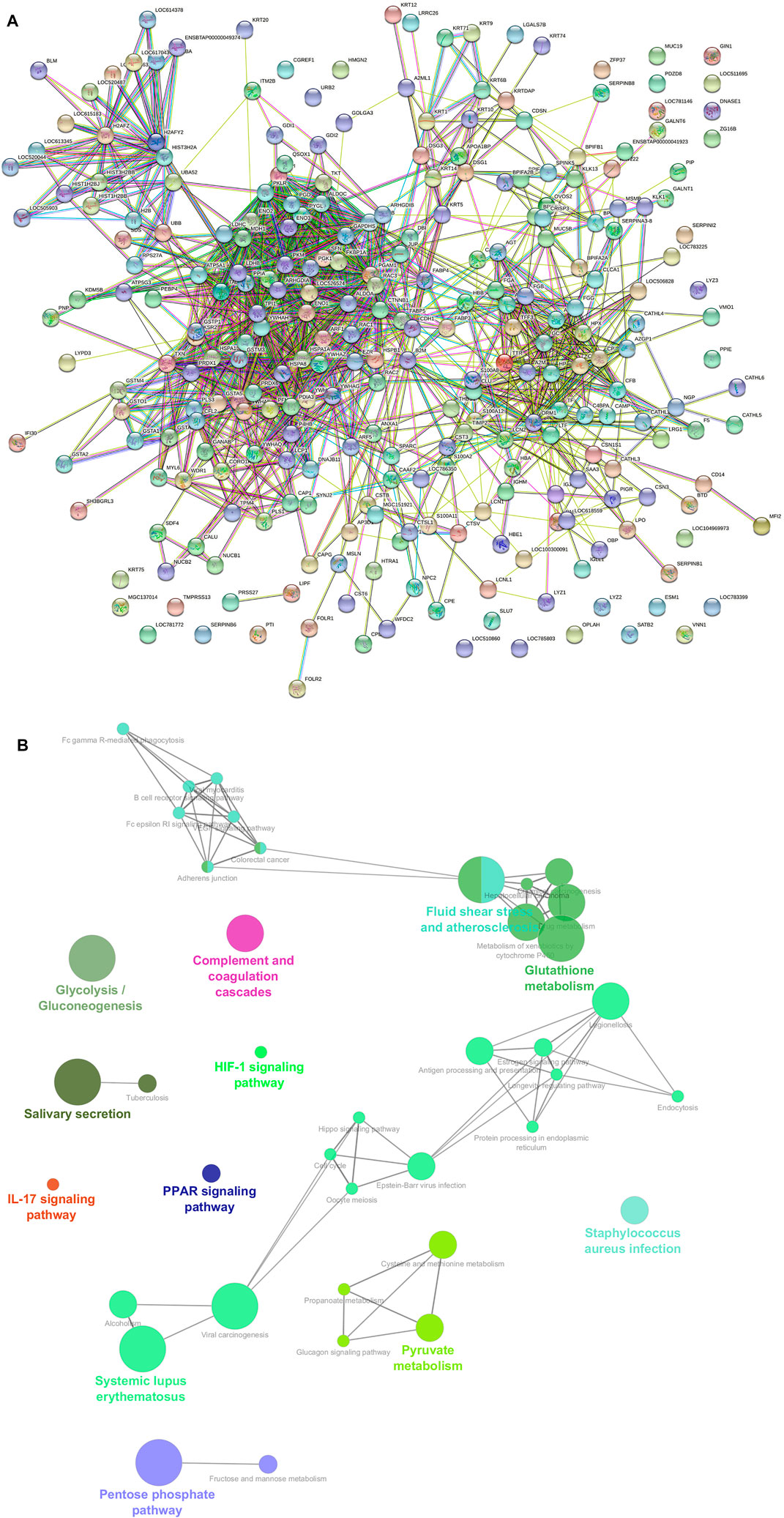
FIGURE 13. (A) Protein–protein interaction (PPI) network constructed by the STRING tool using combined score of 0.9–0.4. A PPI network with high combined score was created (p < 1.0E-16) with 378 nodes, 810 edges, 4.29 average node degree, and 0.357 average local clustering coefficient. (B) Functional annotation of a protein–protein interacting hub using Cytoscape software with ClueGO plug-in.
4 Discussion
Due to the difficulty of detecting estrus in buffalo, the finding of an estrus biomarker in readily accessible bodily fluids such as saliva is critical for the development of a simple, reliable estrus detection tool. Earlier investigation has confirmed the salivary proteins as a potential candidate to detect fertile periods in woman (Alagendran et al., 2013; Saibaba et al., 2021) and estrus (Muthukumar et al., 2014b; Shashikumar et al., 2018) in buffaloes. However, estrus biomarker discovery in buffalo saliva by differential proteome analysis using TMT and LFQ coupled to mass spectrometry is lacking. Comparative proteome profiling using LFQ-LC-MS/MS and TMT-LC-MS/MS analysis in buffalo saliva identified a total of 520 and 369 proteins as DEPs, respectively. These findings may be helpful for further understanding of buffalo estrus biology and development of an easy, reliable estrus detection method for buffalo.
4.1 Proteins Involved in Regulation of Endopeptidase Activity
Our study found the over-expression of leukocyte elastase inhibitor (SERPINB1) during estrus followed by proestrus and almost negligible expression during metestrus and diestrus stages of the estrous cycle. SERPINB1 and several other DEPs (A2M, A2ML1, C3, CST3, CST6, CSTB, LOC404103, LOC786263, LTF, OVOS2, PTI, S100A8, SERPINA3-8, SERPINB1, SERPINB6, SERPINB8, SERPINI2, SFN, SPINK5, THBS1, TIMP2, and WFDC2) in the present study were involved in the regulation of endopeptidase inhibitor activity. SERPINB1 and other protease inhibitors inactivate serine proteases and cysteine proteases and mediate important functions in fibrinolysis, coagulation, inflammation, cell mobility, cellular differentiation, apoptosis, and protein C pathways (Law et al., 2006), thus regulating the innate immune response, inflammation, and cellular homeostasis (Choi et al., 2019). The previous study demonstrated altered expression of three SERPINs, SERPINB2, SERPINE1, and SERPINE2 during follicular development in bovine ovarian follicles (Dow et al., 2002; Bedard et al., 2003). Expression of SERPINE2 gene in preovulatory follicles was markedly upregulated immediately after the LH surge and then decreased to the lowest level toward ovulation (Hasan et al., 2002; Cao et al., 2006). Similarly, over-expression of SERPINE2 in granulosa cells of mature follicles compared to that in small and medium follicles has also been observed (Cao et al., 2004). Previous studies also demonstrated over-expression of SERPINB6 in healthy follicles compared to that in the atretic ones, suggesting follicular development and atresia in bovine ovarian follicles are mediated through SERPINs (Hayashi et al., 2011). The increased abundance of SERPINB1 during the estrus stage may be a protective mechanism of follicular cells against several proteases and intrinsic for follicular development and final follicular maturation and steroid production by ovarian follicles.
4.2 Proteins Involved in Glycolysis and Pyruvate Metabolism
We observed increased expression of beta-enolase (ENO3) during E compared to PE, ME, and DE stages. ENO3 is a glycolytic enzyme and regulates the glycolysis process by catalyzing the reversible conversion of 2-phosphoglyceric acid to phosphoenolpyruvic acid (Giallongo et al., 1993). In addition, we observed 14 other DEPs (ALDOA, ALDOB, ALDOC, ENO1, ENO2, GAPDHS, GPI, LDHA, LDHB, LDHC, PGAM1, PGK1, PKLR, and TPI1) and five DEPs (LDHA, LDHB, LDHC, MDH1, and PKLR) were significantly involved in the glycolysis or gluconeogenesis metabolic pathway and pyruvate metabolism, respectively. It is speculated that these proteins associated with active synthesis of energy may support follicular development, steroidogenesis, final follicular maturation, and ovulation process. In a previous study, pyruvate metabolism has been essential for the completion of oogenesis, serving as a vital source of energy during the meiotic maturation of murine oocytes (Johnson et al., 2007). It has also been observed that the mature cumulus oocyte complex (COC) uses two times more glucose and pyruvate than the immature ones (Sutton et al., 2003), indicating high energy demand supported by upregulation of glycolysis by ovarian follicles during the process of final maturation of oocyte. The previous study has demonstrated that increased enolase expression is responsible for higher expression of the FSH gene in granulosa cells during the follicular phase (Hermann and Heckert, 2007). FSH has a significant role in reproduction by regulating granulosa cell differentiation, proliferation and ovarian steroidogenesis (Richards, 1994), and controlling follicular development and fertility in females (Hermann and Heckert, 2007). Specific expression of ENO3 in buffalo saliva, particularly during estrus stage has also been observed by an earlier study (Muthukumar et al. (2014b). The increased expression of ENO3 during E compared to other stages of the estrous cycle suggests it has a defined role in follicular development, final follicular maturation, and steroid production by follicular granulosa cells that leads to the onset of estrus in buffaloes.
4.3 Proteins Involved in Estrogen Signaling
This study also found a higher abundance of the heat shock protein family A (HSPA1A/HSP70) during estrus than other stages of the estrous cycle. HSPA1A is a molecular chaperon and plays a role in the estrogen signaling pathway. The present study also found other molecular chaperons such as HSPA1L, HSPA2, and HSPA8 as DEPs. Chaperones are stress-response molecules and involved in housekeeping of the cells and facilitating the transport, folding, unfolding, assembly, and disassembly of multi-structured protein units and degradation of misfolded or aggregated proteins (Sorensen et al., 2003). HSPA1A plays a role in the assembly and trafficking of steroid hormone receptors, acts as a co-activator for the nuclear estrogen receptor-α activity, and regulates steroid hormone synthesis by ovarian follicles (Khanna et al., 1995; Liu and Stocco, 1997; Hurd et al., 2000). HSPA1A also mediates luteal regression in murine corpus luteum (Khanna et al., 1995). A previous study also identified a higher abundance of HSPA1A protein in cervical mucus during E than the DE stage of the buffalo estrous cycle (Muthukumar et al., 2014a). Our previous study also observed estrus-specific expression of HSPA1A in buffalo saliva (Shashikumar et al., 2018). Furthermore, our direct saliva transcript analysis also demonstrated a higher level of HSP70 transcript in saliva during estrus than the diestrus stage, suggesting it as a good indicator of estrus in buffaloes (Onteru et al., 2016). HSPA1A protects cells from the negative effect of stress by promoting the folding of proteins and correcting the misfolding of denatured proteins (Lindquist and Craig 1988). Steroid hormone, particularly estradiol plays a key role in inducing estrus signs in farm animals, and its level increased from 22.4–35 pg/ml (Roy and Prakash 2009; Mondal et al., 2010) just before the onset of estrus in buffaloes. An earlier study demonstrated estradiol treatment-induced stress or injury in rat brain vasculature and estradiol-induced heat shock protein expression occurred as a protective mechanism to prevent cellular damage (Lu et al., 2002; Raval et al., 2013). Suggestive increased abundance of HSPA1A protein during estrus could be a protective mechanism of granulosa cells against estrogen-induced stress and its possible role in follicular development and steroidogenesis process.
4.4 Calcium Ion Binding Activity
Increased expression of 45-kDa calcium-binding protein (SDF4) was observed during E compared to PE, ME and DE stages of the estrous cycle. SDF4 is a calcium-binding protein and regulates calcium-dependent activities in the lumen of the endoplasmic reticulum and is involved in calcium-ion regulated exocytosis. Calcium-binding proteins are mainly localized in the cytosol and various parts of the secretory pathway (Honore, 2009). They have diversified functions, including secretory process, chaperone activity, and signal transduction (Honore, 2009). Although its exact role in estrous physiology is unknown, its higher expression during estrus suggests its possible involvement in chaperon-mediated steroidogenesis and cellular protection against estradiol-induced stress.
4.5 Proteins Involved in Innate Immune Response
The present study also identified several DEPs (PIGR, S100A12, BPIFA2B, B2M, A2M, BPIFB1, BPIFA1, CATHL3, CATHL5, FGB, and FGA) involved in innate immune response. The whole estrous cycle is regulated by the differential level of steroid hormones; estradiol is predominant during follicular phase and progesterone during the luteal phase. These steroid hormones modulate the immune system depending on the estrous cycle stage. Binding with specific hormone receptors, steroid hormone induces genomic and non-genomic actions in immune cells (Gilliver, 2010; Kovacs et al., 2010; Bellavance and Rivest, 2014). In general, estradiol is immune-enhancing by stimulating functions of innate immune cells, Th1 responses, and antibody production from B cells. However, progesterone is immune-suppressing by down-regulating functions of immune cells such as macrophages (Su et al., 2009), natural killer cells (Arruvito et al., 2008), or dendritic cells (Butts et al., 2007; Hughes et al., 2008). In addition, progesterone antagonizes the immune-enhancing function of estrogens on T-cell cycle progression (McMurray et al., 2001) and proliferation of T cells (Butts et al., 2007). Importance of antimicrobial peptides (S100A12, CATHL3, and CATHL5)(Diamond et al., 2009), BPI fold-containing family proteins (BPIFA2B, BPIFB1, and BPIFA1) (Li et al., 2020), polymeric immunoglobulin receptor (PIGR) (Wei and Wang, 2021), alpha-2-macroglobulin (A2M), and beta-2-macroglobulin (B2M) (Vandooren and Itoh, 2021) in mucosal immunity is well established. Mucosal immunity protects the internal genital tract against pathogens. During the estrus period, physical barriers between external genitalia and uterus breached, making pathogens to enter into the uterus. Hence, the estrogen-dominant estrus phase modulates the function of innate immune cells and secretes several antimicrobial peptides and proteins that protect the mucosa of the female reproductive tract against external pathogens.
4.6 Proteins Involved in Cytoskeleton Organization
The present study also depicted several DEPs involved in the cytoskeleton organization process (APOA1, ARF1, CAP1, CAPG, CFL1, CFL2, CORO1A, CSN1S1, EZR, FKBP1A, HSPA1A, KRT14, KRT20, KRT71, KRT9, LCP1, PFN1, PLS1, PLS3, RAC1, RAC2, and WDR1). It is during estrus that an oocyte that has been diplotene-restricted restarts meiosis before ovulation. Oocyte meiosis entails a number of intricate processes, including change of the mitotic cell cycle, chromosomal segregation, spindle reorganization, and accomplishment of oocyte asymmetry and polar body extrusion. These activities need reorganization of the cytoskeleton, coordination of cytoplasmic dynamics, and regulation of the cortex’s tension forces (Brunet and Verlhac, 2011; Yi et al., 2011). These findings and our results support the role of cytoskeletal proteins in follicular and oocyte maturation during estrus.
4.7 Proteins With Miscellaneous Functions
Notably, we noticed an elevated expression of vitelline membrane outer layer protein 1 (VMO1) in comparisons between E and PE, ME, and DE. It is the first report of VMO1 protein in saliva during the estrous cycle. Its precise involvement in controlling folliculogenesis and estrous physiology is unknown. VMO1 protein was initially found in chicken as an important component of the egg’s vitelline membrane’s outer layer (Kido et al., 1992) and plays a critical function in oviductal recrudescence in laying hens (Lim and Song, 2015). It is anticipated to be a new estrogen-induced gene in laying hens and a possible diagnostic biomarker for ovarian cancer (Lim and Song, 2015). As a result, it is probable that the estradiol peak that occurs during estrus is the cause for the over-expression of VMO1 and its significant relationship with estrus physiology. Additional investigations in large animals using powerful genetic engineering technologies such as CRISPR-based knockout are required to characterize the mechanism of action (Singh et al., 2000). Another protein, nucleobindin-1 (NUCB1), was shown to be significantly increased during estrus compared to other non-estrus phases. Nucleobindins are multidomain Ca2+ and DNA-binding proteins that have a variety of roles in vertebrates. NUCB1 is converted from NUCB2 to biologically active NUCB1 by the action of prohormone convertase (Rajeswari et al., 2020). NUCB2 is claimed to operate as a local regulator in the mouse ovary, controlling steroidogenesis and energy balance through autocrine and paracrine signaling (Kim and Yang, 2012). Additionally, NUCB2 has been shown to bind predominantly to epithelial cells of uterine glands and neutrophils of the endometrium during the mouse estrous cycle, suggesting that its expression in the uterus may be regulated by estrogen secreted by the ovary but not by gonadotropin released by the pituitary gland (Kim et al., 2011). The precise involvement of NUCB1 in estrous physiology in farm animals is unknown and requires additional research. Additionally, the current research showed that two proteins, lipocalin 1 (LCN1) and odorant-binding protein-2B (OBP2B), were overexpressed during estrus compared to other non-estrus phases. Lipocalin one is a globular protein that interacts with and transports a variety of ligands including volatile pheromones/odorants, fatty acids, lipids, steroids, bilins, and retinol. Lipocalin has been extensively studied as a chemosignal in a variety of animals, including rats and pigs (Scaloni et al., 2001; Muthukumar et al., 2013; Cerna et al., 2017). It was more abundant in rodent urine during estrus than during diestrus (Muthukumar et al., 2013; Cerna et al., 2017). Additionally, it was isolated from bovine olfactory mucosa (Pevsner et al., 1986). Lipocalin is connected with chemical communication during estrus, that is, a unique volatile signal sent by urine from a female to male partner as a mating call (Brennan and Keverne, 2004). Lipocalin has also been identified as a significant progesterone-dependent protein released into the uterine lumen in mares (Crossett et al., 1996). Similarly, over-expression of odorant-binding protein during estrus compared to other stages of the estrous cycle was observed. Odorant-binding protein (OBP) plays a role in odor perception, olfactory stimulus, and chemical communication, especially in insects and mammals through body fluids (Pelosi and Maida, 1990). Cerna et al. (2017) also identified OBP as a pheromone-binding protein in the vaginal fluid of mice and observed its highest concentration before ovulation. OBP is an essential protein for the communication signal in rodents (Cavaggioni and Mucignat-Caretta, 2000; Rajkumar et al., 2009). A previous study also identified this protein in buffalo saliva ((Rajkumar et al., 2010; Muthukumar et al., 2018). Increased expression of LCN1 and OBP2B during estrus compared to other non-estrus phases implies that they play a critical role in mediating chemosignaling during estrus in buffalo.
Conclusion
This is the first comprehensive report on differential proteome analysis of buffalo saliva between the stages of estrus and non-estrus by employing both label-free and TMT-based labeled quantitation and mass spectrometry analysis. Numerous proteins involved in endopeptidase activity, glycolysis, pyruvate metabolism, calcium ion binding, estrogen signaling, and pheromone signaling were identified as differentially expressed between estrus and non-estrus phases. The extensive saliva proteome data set obtained in this work will provide insights into the cellular processes behind buffalo estrous biology. Taken together, this work identified a critical panel of potential proteins in saliva that may be used as a biomarker for buffalo estrus and paves the way for the development of a diagnostic kit for buffalo estrus detection.
Data Availability Statement
The datasets presented in this study can be found in online repositories. The names of the repository/repositories and accession number(s) can be found in the article/Supplementary Material.
Ethics Statement
The animal study was reviewed and approved by the Institute Animal Ethics Committee, NDRI, Karnal [42-IAEC-18-9].
Author Contributions
RB, TKM, AKM and AK conceptualized the study and arranged the funds. LKS, SP, and Neeraj carried out the sampling. RB, AKM, and MP designed the experiments. MP and LKS performed all experiments. MP, AF, SP, Neeraj, and LKS performed Western blotting. RB and SA performed bioinformatics and statistical analysis. RB, MP, and LS wrote the manuscript, and all other authors reviewed the manuscript.
Funding
The study was supported by the Department of Biotechnology (DBT) under the research project entitled “Genomic and proteomics approaches to develop a specific diagnostic assay for detection of estrus/silent estrus in buffaloes” under Grant No. BT/PR23365/AAQ/1/688/2017.
Conflict of Interest
The authors declare that the research was conducted in the absence of any commercial or financial relationships that could be construed as a potential conflict of interest.
Publisher’s Note
All claims expressed in this article are solely those of the authors and do not necessarily represent those of their affiliated organizations, or those of the publisher, the editors, and the reviewers. Any product that may be evaluated in this article, or claim that may be made by its manufacturer, is not guaranteed or endorsed by the publisher.
Acknowledgments
The authors thank the Director, ICAR-National Dairy Research Institute, Karnal, Haryana, for facilitating the work.
Supplementary Material
The Supplementary Material for this article can be found online at: https://www.frontiersin.org/articles/10.3389/fgene.2022.867909/full#supplementary-material
References
20th Livestock Census (2019). 20th Livestock Census. Available at: https://dahd.nic.in/division/provisional-key-results-20th-livestock-census.
Alagendran, S., Saibaba, G., Muthukumar, S., Rajkumar, R., Guzman, R. G., and Archunan, G. (2013). Characterization of Salivary Protein during Ovulatory Phase of Menstrual Cycle through MALDI-TOF/MS. Indian J. Dent. Res. 24, 157–163. doi:10.4103/0970-9290.116669
Almeida, A. M., Ali, S. A., Ceciliani, F., Eckersall, P. D., Hernández-Castellano, L. E., Han, R., et al. (2021). Domestic Animal Proteomics in the 21st century: A Global Retrospective and Viewpoint Analysis. J. Proteomics 241, 104220. doi:10.1016/j.jprot.2021.104220
Ang, C.-S., Binos, S., Knight, M. I., Moate, P. J., Cocks, B. G., and McDonagh, M. B. (2011). Global Survey of the Bovine Salivary Proteome: Integrating Multidimensional Prefractionation, Targeted, and Glycocapture Strategies. J. Proteome Res. 10, 5059–5069. doi:10.1021/pr200516d
Archunan, G., Rajanarayanan, S., and Karthikeyan, K. (2014). Cattle Pheromones. Neurobiology of Chemical Communication. 1st edn. New York: CRC Press, 461–479.
Arruvito, L., Giulianelli, S., Flores, A. C., Paladino, N., Barboza, M., Lanari, C., et al. (2008). NK Cells Expressing a Progesterone Receptor Are Susceptible to Progesterone-Induced Apoptosis. J. Immunol. 180, 5746–5753. doi:10.4049/jimmunol.180.8.5746
Bédard, J., Brûlé, S., Price, C. A., Silversides, D. W., and Lussier, J. G. (2003). Serine Protease Inhibitor-E2 (SERPINE2) Is Differentially Expressed in Granulosa Cells of Dominant Follicle in Cattle. Mol. Reprod. Dev. 64, 152–165. doi:10.1002/mrd.10239
Bellavance, M.-A., and Rivest, S. (2014). The HPA €" Immune Axis and the Immunomodulatory Actions of Glucocorticoids in the Brain. Front. Immunol. 5, 136. doi:10.3389/fimmu.2014.00136
Brennan, P. A., and Keverne, E. B. (2004). Something in the Air? New Insights into Mammalian Pheromones. Curr. Biol. 14, R81–R89. doi:10.1016/j.cub.2003.12.052
Brunet, S., and Verlhac, M. H. (2011). Positioning to Get Out of Meiosis: The Asymmetry of Division. Hum. Reprod. Update 17, 68–75. doi:10.1093/humupd/dmq044
Butts, C. L., Shukair, S. A., Duncan, K. M., Bowers, E., Horn, C., Belyavskaya, E., et al. (2007). Progesterone Inhibits Mature Rat Dendritic Cells in a Receptor-Mediated Fashion. Int. Immunol. 19, 287–296. doi:10.1093/intimm/dxl145
Cabras, T., Pisano, E., Montaldo, C., Giuca, M. R., Iavarone, F., Zampino, G., et al. (2013). Significant Modifications of the Salivary Proteome Potentially Associated with Complications of Down Syndrome Revealed by Top-Down Proteomics. Mol. Cell Proteomics 12, 1844–1852. doi:10.1074/mcp.m112.026708
Cao, M., Nicola, E., Portela, V. M., and Price, C. A. (2006). Regulation of Serine Protease Inhibitor-E2 and Plasminogen Activator Expression and Secretion by Follicle Stimulating Hormone and Growth Factors in Non-luteinizing Bovine Granulosa Cells In Vitro. Matrix Biol. 25, 342–354. doi:10.1016/j.matbio.2006.05.005
Cao, M., Sahmi, M., Lussier, J. G., and Price, C. A. (2004). Plasminogen Activator and Serine Protease Inhibitor-E2 (Protease Nexin-1) Expression by Bovine Granulosa Cells In Vitro1. Biol. Reprod. 71, 887–893. doi:10.1095/biolreprod.104.029702
Castagnola, M., Scarano, E., Passali, G. C., Messana, I., Cabras, T., Iavarone, F., et al. (2017). Salivary Biomarkers and Proteomics: Future Diagnostic and Clinical Utilities. Acta Otorhinolaryngol. Ital. 37, 94–101. doi:10.14639/0392-100x-1598
Cavaggioni, A., and Mucignat-Caretta, C. (2000). Major Urinary Proteins, α2U-globulins and Aphrodisin. Biochim. Biophys. Acta (Bba) - Protein Struct. Mol. Enzymol. 1482, 218–228. doi:10.1016/s0167-4838(00)00149-7
Černá, M., Kuntová, B., Talacko, P., Stopková, R., and Stopka, P. (2017). Differential Regulation of Vaginal Lipocalins (OBP, MUP) during the Estrous Cycle of the House Mouse. Sci. Rep. 7, 11674–11710. doi:10.1038/s41598-017-12021-2
Choi, Y. J., Kim, S., Choi, Y., Nielsen, T. B., Yan, J., Lu, A., et al. (2019). SERPINB1-mediated Checkpoint of Inflammatory Caspase Activation. Nat. Immunol. 20, 276–287. doi:10.1038/s41590-018-0303-z
Crossett, B., Allen, W. R., and Stewart, F. (1996). A 19 kDa Protein Secreted by the Endometrium of the Mare Is a Novel Member of the Lipocalin Family. Biochem. J. 320, 137–143. doi:10.1042/bj3200137
Das, G. K., and Khan, F. A. (2010). Summer Anoestrus in buffalo--a Review. Reprod. Domest. Anim. 45, e483–94. doi:10.1111/j.1439-0531.2010.01598.x
Diamond, G., Beckloff, N., Weinberg, A., and Kisich, K. (2009). The Roles of Antimicrobial Peptides in Innate Host Defense. Cpd 15 (21), 2377–2392. doi:10.2174/138161209788682325
Dow, M. P. D., Bakke, L. J., Cassar, C. A., Peters, M. W., Pursley, J. R., and Smith, G. W. (2002). Gonadotropin Surge-Induced Up-Regulation of the Plasminogen Activators (Tissue Plasminogen Activator and Urokinase Plasminogen Activator) and the Urokinase Plasminogen Activator Receptor within Bovine Periovulatory Follicular and Luteal Tissue1. Biol. Reprod. 66, 1413–1421. doi:10.1095/biolreprod66.5.1413
Edgar, W. M. (1992). Saliva: its Secretion, Composition and Functions. Br. Dent. J. 172, 305–312. doi:10.1038/sj.bdj.4807861
Giallongo, A., Venturella, S., Oliva, D., Barbieri, G., Rubino, P., and Feo, S. (1993). Structural Features of the Human Gene for Muscle-specific Enolase. Differential Splicing in the 5'-untranslated Sequence Generates Two Forms of mRNA. Eur. J. Biochem. 214, 367–374. doi:10.1111/j.1432-1033.1993.tb17932.x
Gilliver, S. C. (2010). Sex Steroids as Inflammatory Regulators. J. Steroid Biochem. Mol. Biol. 120, 105–115. doi:10.1016/j.jsbmb.2009.12.015
Hasan, S., Hosseini, G., Princivalle, M., Dong, J.-C., Birsan, D., Cagide, C., et al. (2002). Coordinate Expression of Anticoagulant Heparan Sulfate Proteoglycans and Serine Protease Inhibitors in the Rat Ovary: A Potent System of Proteolysis Control1. Biol. Reprod. 66, 144–158. doi:10.1095/biolreprod66.1.144
Hayashi, K. G., Ushizawa, K., Hosoe, M., and Takahashi, T. (2011). Differential Gene Expression of Serine Protease Inhibitors in Bovine Ovarian Follicle: Possible Involvement in Follicular Growth and Atresia. Reprod. Biol. Endocrinol. 9, 72–79. doi:10.1186/1477-7827-9-72
Hermann, B. P., and Heckert, L. L. (2007). Transcriptional Regulation of the FSH Receptor: New Perspectives. Mol. Cell Endocrinol. 260-262, 100–108. doi:10.1016/j.mce.2006.09.005
Honoré, B. (2009). The Rapidly Expanding CREC Protein Family: Members, Localization, Function, and Role in Disease. Bioessays 31, 262–277. doi:10.1002/bies.200800186
Hughes, G. C., Thomas, S., Li, C., Kaja, M.-K., and Clark, E. A. (2008). Cutting Edge: Progesterone Regulates IFN-α Production by Plasmacytoid Dendritic Cells. J. Immunol. 180, 2029–2033. doi:10.4049/jimmunol.180.4.2029
Hurd, A. M., Schiff, R., Parra, I., Friedrichs, W. E., Osborne, C. K., Morimoto, R. I., et al. (2000). Heat Shock Protein 70 Can Modulate Estrogen Receptor Activity in Breast Cancer Cells. Proc. Am. Assoc. Cancer Res. 41, 73.
Johnson, M. T., Freeman, E. A., Gardner, D. K., and Hunt, P. A. (2007). Oxidative Metabolism of Pyruvate Is Required for Meiotic Maturation of Murine Oocytes In Vivo1. Biol. Reprod. 77, 2–8. doi:10.1095/biolreprod.106.059899
Jou, Y.-J., Hua, C.-H., Lin, C.-D., Lai, C.-H., Huang, S.-H., Tsai, M.-H., et al. (2014). S100A8 as Potential Salivary Biomarker of Oral Squamous Cell Carcinoma Using nanoLC-MS/MS. Clinica Chim. Acta 436, 121–129. doi:10.1016/j.cca.2014.05.009
Khanna, A., Aten, R. F., and Behrman, H. R. (1995). Heat Shock Protein-70 Induction Mediates Luteal Regression in the Rat. Mol. Endocrinol. 9, 1431–1440. doi:10.1210/mend.9.11.8584020
Kido, S., Morimoto, A., Kim, F., and Doi, Y. (1992). Isolation of a Novel Protein from the Outer Layer of the Vitelline Membrane. Biochem. J. 286, 17–22. doi:10.1042/bj2860017
Kim, J. H., Lee, K. R., Kim, H. K., No, S. H., Yoo, H. M., Moon, C. I., et al. (2011). 17beta-estradiol Regulates the Expression of nesfatin-1/Nucb2 in Mouse Uterus. Dev. Reprod. 15, 349–357. doi:10.12717/DR.2020.24.1.43
Kim, J., and Yang, H. (2012). Nesfatin-1 as a New Potent Regulator in Reproductive System. Dev. Reprod. 16, 253–264. doi:10.12717/dr.2012.16.4.253
Kovacs, P., Kovats, T., and Kaali, S. G. (2010). Results with Early Follicular Phase Recombinant Luteinizing Hormone Supplementation during Stimulation for In Vitro Fertilization. Fertil. Sterility 93, 475–479. doi:10.1016/j.fertnstert.2008.12.010
Kumar, G. (2015). “Comparative Proteome Analysis of Saliva in Pregnant and Non-pregnant Sahiwal Cows,” (Karnal, Haryana, India: National Dairy Research Institute). M.Tech. Thesis.
Law, R. H., Zhang, Q., McGowan, S., Buckle, A. M., Silverman, G. A., Wong, W., et al. (2006). An Overview of the Serpin Superfamily. Genome Biol. 7, 216–311. doi:10.1186/gb-2006-7-5-216
Levine, M. J. (1993). Salivary Macromolecules. Ann. NY Acad. Sci. 694, 11–16. doi:10.1111/j.1749-6632.1993.tb18337.x
Li, J., Xu, P., Wang, L., Feng, M., Chen, D., Yu, X., et al. (2020). Molecular Biology of BPIFB1 and its Advances in Disease. Ann. Transl. Med. 8 (10), 651. doi:10.21037/atm-20-3462
Li, Y., St. John, M. A. R., Zhou, X., Kim, Y., Sinha, U., Jordan, R. C. K., et al. (2004). Salivary Transcriptome Diagnostics for Oral Cancer Detection. Clin. Cancer Res. 10, 8442–8450. doi:10.1158/1078-0432.ccr-04-1167
Lim, W., and Song, G. (2015). Differential Expression of Vitelline Membrane Outer Layer Protein 1: Hormonal Regulation of Expression in the Oviduct and in Ovarian Carcinomas from Laying Hens. Mol. Cell Endocrinol. 399, 250–258. doi:10.1016/j.mce.2014.10.015
Lindquist, S., and Craig, E. A. (1988). The Heat-Shock Proteins. Annu. Rev. Genet. 22 (1), 631–677. doi:10.1146/annurev.ge.22.120188.003215
Liu, Z., and Stocco, D. M. (1997). Heat Shock-Induced Inhibition of Acute Steroidogenesis in MA-10 Cells Is Associated with Inhibition of the Synthesis of the Steroidogenic Acute Regulatory Protein1. Endocrinology 138, 2722–2728. doi:10.1210/endo.138.7.5278
Lu, A., Ran, R.-q., Clark, J., Reilly, M., Nee, A., and Sharp, F. R. (2002). 17-β-Estradiol Induces Heat Shock Proteins in Brain Arteries and Potentiates Ischemic Heat Shock Protein Induction in Glia and Neurons. J. Cereb. Blood Flow Metab. 22, 183–195. doi:10.1097/00004647-200202000-00006
Mariani, M., Souto, M., Fanelli, M., and Ciocca, D. (2000). Constitutive Expression of Heat Shock Proteins HSP25 and HSP70 in the Rat Oviduct during Neonatal Development, the Oestrous Cycle and Early Pregnancy. Reproduction 120, 217–223. doi:10.1530/jrf.0.1200217
Mass, E., Gadoth, N., Harell, D., and Wolff, A. (2002). Can Salivary Composition and High Flow Rate Explain the Low Caries Rate in Children with Familial Dysautonomia? Pediatr. Dent. 24, 581–586.
McMurray, R. W., Suwannaroj, S., Ndebele, K., and Jenkins, J. K. (2001). Differential Effects of Sex Steroids on T and B Cells: Modulation of Cell Cycle Phase Distribution, Apoptosis and Bcl-2 Protein Levels. Pathobiology 69, 44–58. doi:10.1159/000048757
Mondal, S., Suresh, K. P., and Nandi, S. (2010). Endocrine Profile of Oestrous Cycle in Buffaloes: A Meta-Analysis. Asian Aust. J. Anim. 23, 169–174. doi:10.5713/ajas.2010.90193
Muthukumar, S., Rajesh, D., Saibaba, G., Alagesan, A., Rengarajan, R. L., and Archunan, G. (2013). Urinary Lipocalin Protein in a Female Rodent with Correlation to Phases in the Estrous Cycle: an Experimental Study Accompanied by In Silico Analysis. PLoS One 8, e71357. doi:10.1371/journal.pone.0071357
Muthukumar, S., Rajesh, D., Selvam, R. M., Saibaba, G., Suvaithenamudhan, S., Akbarsha, M. A., et al. (2018). Buffalo Nasal Odorant-Binding Protein (bunOBP) and its Structural Evaluation with Putative Pheromones. Sci. Rep. 8, 9323–9414. doi:10.1038/s41598-018-27550-7
Muthukumar, S., Rajkumar, R., Karthikeyan, K., Liao, C. C., Singh, D., Akbarsha, M. A., et al. (2014a). Buffalo Cervico-Vaginal Fluid Proteomics with Special Reference to Estrous Cycle: Heat Shock Protein (HSP)-70 Appears to Be an Estrus Indicator. Biol. Reprod. 90, 97–101. doi:10.1095/biolreprod.113.113852
Muthukumar, S., Rajkumar, R., Rajesh, D., Saibaba, G., Liao, C. C., Archunan, G., et al. (2014b). Exploration of Salivary Proteins in buffalo: an Approach to Find Marker Proteins for Estrus. FASEB j. 28, 4700–4709. doi:10.1096/fj.14-252288
Onteru, S. K., Baddela, V. S., Ravinder, R., Kaipa, O., Nayan, V., Singh, P., et al. (2016). Direct Saliva Transcript Analysis as a Novel Non-invasive Method for Oestrus Marker Detection in Buffaloes. Biomarkers 21, 99–101. doi:10.3109/1354750x.2015.1118549
Pedersen, A., Bardow, A., Jensen, S. B., and Nauntofte, B. (2002). Saliva and Gastrointestinal Functions of Taste, Mastication, Swallowing and Digestion. Oral Dis. 8, 117–129. doi:10.1034/j.1601-0825.2002.02851.x
Pelosi, P., and Maida, R. (1990). Odorant-binding Proteins in Vertebrates and Insects: Similarities and Possible Common Function. Chem. Senses. 15, 205–215. doi:10.1093/chemse/15.2.205
Pevsner, J., Sklar, P. B., and Snyder, S. H. (1986). Odorant-binding Protein: Localization to Nasal Glands and Secretions. Proc. Natl. Acad. Sci. U.S.A. 83, 4942–4946. doi:10.1073/pnas.83.13.4942
Prout, R. E. S., and Hopps, R. M. (1970). A Relationship between Human Oral Bacteria and the Menstrual Cycle. J. Periodontol. 41, 98–101. doi:10.1902/jop.1970.41.2.98
Rajeswari, J. J., Hatef, A., and Unniappan, S. (2020). Nesfatin-1-like Peptide Suppresses Hypothalamo-Pituitary-Gonadal mRNAs, Gonadal Steroidogenesis, and Oocyte Maturation in Fish. Biol. Reprod. 103, 802–816. doi:10.1093/biolre/ioaa106
Rajkumar, R., Ilayaraja, R., Mucignat, C., Cavaggioni, A., and Archunan, G. (2009). Identification of Alpha2u-Globulin and Bound Volatiles in the Indian Common House Rat (Rattus rattus). Indian J. Biochem. Biophys. 46, 319–324.
Rajkumar, R., Karthikeyan, K., Archunan, G., Huang, P. H., Chen, Y. W., Ng, W. V., et al. (2010). Using Mass Spectrometry to Detect buffalo Salivary Odorant-Binding Protein and its post-translational Modifications. Rapid Commun. Mass. Spectrom. 24, 3248–3254. doi:10.1002/rcm.4766
Raval, A. P., Borges-Garcia, R., Javier Moreno, W., Perez-Pinzon, M. A., and Bramlett, H. (2013). Periodic 17β-Estradiol Pretreatment Protects Rat Brain from Cerebral Ischemic Damage via Estrogen Receptor-β. PLoS One 8, e60716. doi:10.1371/journal.pone.0060716
Richards, J. S. (1994). Hormonal Control of Gene Expression in the Ovary. Endocr. Rev. 15, 725–751. doi:10.1210/edrv-15-6-725
Roy, K. S., and Prakash, B. S. (2009). Plasma Progesterone, Oestradiol-17β and Total Oestrogen Profiles in Relation to Oestrous Behaviour during Induced Ovulation in Murrah buffalo Heifers. J. Anim. Physiol. Anim. Nutr. 93, 486–495. doi:10.1111/j.1439-0396.2008.00830.x
Saibaba, G., Rajesh, D., Muthukumar, S., Sathiyanarayanan, G., Aarthy, A. P., and Archunan, G. (2021). Salivary Proteome Profile of Women during fertile Phase of Menstrual Cycle as Characterized by Mass Spectrometry. Gynecol. Minim. Invasive Ther. 10 (4), 226–234. doi:10.4103/GMIT.GMIT_78_20
Scaloni, A., Paolini, S., Brandazza, A., Fantacci, M., Bottiglieri, C., Marchese, S., et al. (2001). Purification, Cloning and Characterisation of Odorant- and Pheromone-Binding Proteins from Pig Nasal Epithelium. Cmls, Cel. Mol. Life Sci. 58, 823–834. doi:10.1007/pl00000903
Selvam, R. M., and Archunan, G. (2017). A Combinatorial Model for Effective Estrus Detection in Murrah buffalo. Vet. World 10 (2), 209–213. doi:10.14202/vetworld.2017.209-213
Sharma, H. C., Dhami, A. J., Sharma, S. K., Sarvaiya, N. P., and Kavani, F. S. (2008). Assessment of Estrus Detection and Insemination Efficiency of AI Workers in Buffaloes through Plasma Progesterone Profile under Field Conditions. Indian J. Anim. Sci. 27, 706–709.
Shashikumar, N. G., Baithalu, R. K., Bathla, S., Ali, S. A., Rawat, P., Kumaresan, A., et al. (2018). Global Proteomic Analysis of Water buffalo (Bubalus Bubalis) Saliva at Different Stages of Estrous Cycle Using High Throughput Mass Spectrometry. Theriogenology 110, 52–60. doi:10.1016/j.theriogenology.2017.12.046
Shashikumar, N. G. (2017). “Identification of Estrus Specific Proteins in buffalo Saliva,” (Karnal, Haryana, India: National Dairy Research Institute). M.V.Sc.Thesis.
Singh, J., Nanda, A. S., and Adams, G. P. (2000). The Reproductive Pattern and Efficiency of Female Buffaloes. Anim. Reprod. Sci. 60-61, 593–604. doi:10.1016/s0378-4320(00)00109-3
Skalova, I., Fedorova, T., and Brandlova, K. (2013). Saliva Crystallization in Cattle: New Possibility for Early Pregnancy Diagnosis? Agricultura Tropica Et Subtropica 46, 102–104. doi:10.2478/ats-2013-0018
Sorensen, J. G., Kristensen, T. N., and Loeschcke, V. (2003). The Evolutionary and Ecological Role of Heat Shock Proteins. Ecol. Lett. 6, 1025–1037. doi:10.1046/j.1461-0248.2003.00528.x
Su, L., Sun, Y., Ma, F., Lü, P., Huang, H., and Zhou, J. (2009). Progesterone Inhibits Toll-like Receptor 4-mediated Innate Immune Response in Macrophages by Suppressing NF-Κb Activation and Enhancing SOCS1 Expression. Immunol. Lett. 125, 151–155. doi:10.1016/j.imlet.2009.07.003
Sutton, M., Cetica, P., Beconi, M., Kind, K., Gilchrist, R., and Thompson, J. (2003). Influence of Oocyte-Secreted Factors and Culture Duration on the Metabolic Activity of Bovine Cumulus Cell Complexes. Reprod 126, 27–34. doi:10.1530/rep.0.1260027
Tsiligianni, T., Karagiannidis, A., Brikas, P., and Saratsis, P. (2001). Physical Properties of Bovine Cervical Mucus during normal and Induced (Progesterone And/or PGF2α) Estrus. Theriogenology 55, 629–640. doi:10.1016/s0093-691x(01)00431-9
Vandooren, J., and Itoh, Y. (2021). Alpha-2-macroglobulin in Inflammation, Immunity and Infections. Front. Immunol. 12, 803244. doi:10.3389/fimmu.2021.803244
Verma, K. K., Prasad, S., Kumaresan, A., Mohanty, T. K., Layek, S. S., Patbandha, T. K., et al. (2014). Characterization of Physico-Chemical Properties of Cervical Mucus in Relation to Parity and conception Rate in Murrah Buffaloes. Vet. World 7, 467–471. doi:10.14202/vetworld.2014.467-471
Wang, Z., Niu, W., Wang, Y., Teng, Z., Wen, J., Xia, G., et al. (2015). Follistatin288 Regulates Germ Cell Cyst Breakdown and Primordial Follicle Assembly in the Mouse Ovary. PLoS One 10, e0129643. doi:10.1371/journal.pone.0129643
Wei, H., and Wang, J.-Y. (2021). Role of Polymeric Immunoglobulin Receptor in IgA and IgM Transcytosis. Ijms 22, 2284. doi:10.3390/ijms22052284
Keywords: biomarker, estrus, label-free quantitation, LC-MS/MS, tandem mass tag, saliva
Citation: Singh LK, Pandey M, Baithalu RK, Fernandes A, Ali SA, Jaiswal L, Pannu S, Neeraj , Mohanty TK, Kumaresan A, Datta TK, Kumar S and Mohanty AK (2022) Comparative Proteome Profiling of Saliva Between Estrus and Non-Estrus Stages by Employing Label-Free Quantitation (LFQ) and Tandem Mass Tag (TMT)-LC-MS/MS Analysis: An Approach for Estrus Biomarker Identification in Bubalus bubalis. Front. Genet. 13:867909. doi: 10.3389/fgene.2022.867909
Received: 01 February 2022; Accepted: 05 April 2022;
Published: 13 May 2022.
Edited by:
Olanrewaju B. Morenikeji, University of Pittsburgh at Bradford, United StatesReviewed by:
Mihir Sarkar, Indian Council of Agricultural Research (ICAR), IndiaCinzia Marchitelli, Research Centre for Animal Production and Aquaculture (CREA), Italy
Copyright © 2022 Singh, Pandey, Baithalu, Fernandes, Ali, Jaiswal, Pannu, Neeraj, Mohanty, Kumaresan, Datta, Kumar and Mohanty. This is an open-access article distributed under the terms of the Creative Commons Attribution License (CC BY). The use, distribution or reproduction in other forums is permitted, provided the original author(s) and the copyright owner(s) are credited and that the original publication in this journal is cited, in accordance with accepted academic practice. No use, distribution or reproduction is permitted which does not comply with these terms.
*Correspondence: Rubina Kumari Baithalu, rbaithalu@gmail.com, orcid.org/0000-0003-0681-7209
†These authors have contributed equally to this work and share first authorship