- 1Department of Internal Medicine, School of Medicine, Wake Forest University, Winston Salem, NC, United States
- 2Department of Physiology and Pharmacology, School of Medicine, Wake Forest University, Winston Salem, NC, United States
- 3Department of Physiology, Medical College of Wisconsin, Milwaukee, WI, United States
- 4Department of Psychiatry and Behavioral Sciences, Feinberg School of Medicine, Northwestern University, Chicago, IL, United States
We previously identified Keratinocyte-associated protein 3, Krtcap3, as a novel adiposity gene, but subsequently found that its impact on adiposity may depend on environmental stress. To more thoroughly understand the connection between Krtcap3, adiposity, and stress, we exposed wild-type (WT) and Krtcap3 knock-out (KO) rats to chronic stress then measured adiposity and behavioral outcomes. We found that KO rats displayed lower basal stress than WT rats under control conditions and exhibited metabolic and behavioral responses to chronic stress exposure. Specifically, stress-exposed KO rats gained more weight, consumed more food when socially isolated, and displayed more anxiety-like behaviors relative to control KO rats. Meanwhile, there were minimal differences between control and stressed WT rats. At study conclusion stress-exposed KO rats had increased corticosterone (CORT) relative to control KO rats with no differences between WT rats. In addition, KO rats, independent of prior stress exposure, had an increased CORT response to removal of their cage-mate (psychosocial stress), which was only seen in WT rats when exposed to chronic stress. Finally, we found differences in expression of the glucocorticoid receptor, Nr3c1, in the pituitary and colon between control and stress-exposed KO rats that were not present in WT rats. These data support that Krtcap3 expression affects stress response, potentially via interactions with Nr3c1, with downstream effects on adiposity and behavior. Future work is necessary to more thoroughly understand the role of Krtcap3 in the stress response.
Introduction
Obesity continues to be a national and global health crisis. Data from 2021 show that over 40% of adults are characterized as having obesity (Stierman et al., 2021), which is commonly attributed to decreases in diet quality and increases in sedentary lifestyles. Obesity is a complex disease that results from interaction of genetic and environmental factors. The genetic architecture of obesity remains poorly understood, despite the hundreds of genetic loci that have been identified (Abadi et al., 2017; Yengo et al., 2018; Pulit et al., 2019; Rohde et al., 2019). Importantly, environmental factors include more than diet and exercise but are not as frequently investigated. For example, stress can have profound effects on eating behavior, fat deposition, and mental health, which may induce or propagate the well-established vicious cycle between obesity, dysregulated metabolic health, and altered mental health (Lee et al., 2000; Torres and Nowson, 2007; Calabrese et al., 2009; Peckett et al., 2011; Scott et al., 2012; van der Valk et al., 2018; Lahdepuro et al., 2019; Kahan, 2020; Xiao et al., 2020; Wang et al., 2022). Though the connection between obesity and stress is well-known, only a small amount of research has specifically investigated the genetic connections between obesity and stress. Prior work has shown that there is a genetic component to the stress response (Ising and Holsboer, 2006; Solberg et al., 2006; van den Bos et al., 2009; Henckens et al., 2016; Flati et al., 2020), which raises the possibility that genetic variation in stress reactivity may connect to variation in adiposity.
We first identified Keratinocyte-associated protein 3 (Krtcap3) as a candidate gene for adiposity using a genome-wide association study (GWAS) in rats (Keele et al., 2018). Initial work in an in vivo Krtcap3 knock-out (KO) model supported these GWAS findings, demonstrating that female KO rats had increased food intake and adiposity compared to wild-type (WT) controls. These data initially suggested that increased expression of Krtcap3 had a protective effect on adiposity in rats. However, we were unable to replicate these initial results in a subsequent study, which may have been due to changes in environmental stress (Szalanczy et al., 2023). COVID-19 shut-downs caused large environmental differences in rat housing conditions between the two studies, including decreased foot traffic and the completion of a nearby construction project, that may have decreased the stress of the rats. We found that WT rats ate more, had increased adiposity, and had decreased serum corticosterone (CORT) in the second study, supporting a decrease in stress. The same phenotypes were not altered in KO rats between the two studies. This suggests that decreased Krtcap3 expression may either protect against the effects of low-grade, chronic stress or that environmental stress alters KO behavior to favor increased eating and adiposity. We thus proposed that Krtcap3 may play a role in stress response with secondary effects on adiposity (Szalanczy et al., 2023).
In the current study, we sought to investigate the relationship between Krtcap3 expression, adiposity, and stress. We expected that under stressful conditions, WT rats would show decreased food intake and adiposity relative to KO rats. When naïve to stress, we anticipated that WT and KO rats would have similar adiposity measures. We also expected that environmental stress would induce increased eating and adiposity in KO rats compared to controls. We also included several behavioral tests as chronic stress has been shown to worsen mental health in humans (Calabrese et al., 2009; Lahdepuro et al., 2019) and alter emotion-like behavior in rodents (Sequeira-Cordero et al., 2019; Atrooz et al., 2021). We initially sought to induce stress by mimicking the increased noise conditions from the first study by exposing rats to experimentally controlled, loud auditory stimuli. When we did not see increases in serum corticosterone or changes in adiposity, we began a second arm of stress delivery using a modified unpredictable chronic mild stress (UCMS) paradigm (Willner, 2017) where rats were exposed to a variety of mild stressors 6 days of the week.
In the current study we found that stress more strongly increased adiposity and heightened anxiety-like behaviors in KO rats, without corresponding changes in WT. This could be explained by the fact that serum CORT increased only in the KO rats as opposed to WT, in contrast to our previous work where CORT was higher in the WT rats (Szalanczy et al., 2023). Although we are unable to make direct comparisons between the studies, that decreased expression of Krtcap3 leads to increased eating and adiposity under conditions of chronic stress is consistent with the previous findings (Szalanczy et al., 2022; Szalanczy et al., 2023) and further supports a role of Krtcap3 in the stress response. Whether the role of Krtcap3 in the stress response is protective or deleterious, however, is still an open question and future studies will be needed to address this.
Materials and methods
Animals
We previously generated a whole-body in vivo Krtcap3-KO on the WKY (WKY/NCrl; RGD_1358112) inbred rat strain (WKY-Krtcap3em3Mcwi) and established a breeding colony at Wake Forest University School of Medicine (WFUSOM) in 2019 (Szalanczy et al., 2022). At Building A of WFUSOM, rats were housed in ventilated cages (46 cm × 24 cm × 20 cm) at 22°C in a 12 h light/dark cycle (dark from 18:00 to 6:00) at standard temperature and humidity conditions, and given ad libitum access to water. Bedding was 0.25 in corn cob with a paper puck for enrichment. Breeder rats and experimental rats prior to high-fat diet (HFD) start were given ad libitum standard chow diet (Lab Diet, Prolab RMH 3000, Catalog #5P00). At 6 weeks of age, experimental WT and KO rats were placed on experimental diet as described below (n = 8 female rats per genotype and stress condition).
To briefly describe the postnatal period of the experimental rats, litters were between eight to twelve rats and only rats from the first litter were used in the current study. Dam and sire were housed together during the postnatal period. Rats were not disturbed for the first 5 days after birth, and after were handled once per week by animal care staff during cage cleanings. In addition, pups were handled once by experimenters to collect ear punches for genotyping before weaning. Pups were away from their dam for no more than 10 min and tools were cleaned between each pup. This method of ear punch collection was also used in previous studies with the WKY-Krtcap3em3Mcwi strain (Szalanczy et al., 2022; Szalanczy et al., 2023). Pups were exposed to the same lighting, noise, and traffic conditions of Building A as all other rats.
Experimental animals in the stress study were weaned at three weeks of age and housed as two rats of the same genotype per cage. Animals were housed at Building A in the same room as the breeding colony up until approximately 23 weeks of age, at which point they were transferred to the nearby Building B, which is better set up for rodent behavioral testing. At Building B, rats were housed in a male/female room in non-ventilated, open-air wire top cages (26.5 cm × 48 cm x 20 cm) with aspen shavings. Rats were maintained on the same 12 h light/dark cycle (dark from 18:00 to 6:00) and access to food and water remained ad libitum. Building B is an older building that does not allow temperature and humidity to be as tightly controlled as Building A, but conditions are appropriately managed with animal care health observation and veterinary oversight.
We also sought to evaluate Krtcap3 expression in multiple tissues between adolescent WT and KO rats from the colony without diet or stress exposure. Female WT and KO (n = 4 per genotype) rats were weaned at 3 weeks of age and placed into mixed-genotype cages with littermates, with two to four rats per cage. Rats were housed in Building A and maintained on the same chow diet as breeders. One week later, rats were euthanized, described below.
All experiments were performed using a protocol approved by the Institutional Animal Care and Use Committee at WFUSOM.
Genotyping
Experimental rats were genotyped at the Medical College of Wisconsin (MCW) as described elsewhere (Szalanczy et al., 2023).
Stress study design
Female experimental rats were weaned at 3 weeks of age, weighed, and placed two per cage in same-sex, same-genotype cages. Rats were randomly assigned to either a control or stress group, as described in detail below. Stress study rats were initially maintained on the same diet as breeders (see above), and body weight was recorded weekly starting at 4 weeks of age. To remain consistent with our previous studies (Szalanczy et al., 2022; Szalanczy et al., 2023), all rats began a HFD (60% kcal fat; ResearchDiet D12492) at 6 weeks of age. Rats were allowed access to diet ad libitum. Cage-wide food intake was recorded weekly from the time of HFD start until the end of UCMS.
From the time of weaning, the study lasted for 22 weeks (Figure 1). For rats assigned into the stress group, a mild stress exposure began at 22 days of age and lasted for approximately 12 weeks. Control rats underwent the same metabolic and behavioral phenotyping as stress rats, but were not exposed to the stress protocols. The first EchoMRI analysis was conducted 3 weeks after stress onset, at HFD start, while an acute restraint test took place 10 weeks after stress onset. The first two behavioral tests were the novelty suppressed feeding test (NSF) conducted 11.5 weeks after stress onset and the forced swim test (FST) conducted a week later. Another EchoMRI analysis was run 13 weeks after stress onset, leading immediately into a week-long period of individual housing to assess food intake. Following return to cage-mate, UCMS began 15 weeks after initial stress onset, and continued for 4 weeks, concluding with another EchoMRI analysis and an intraperitoneal glucose tolerance test (IPGTT). After rats were transferred to Building B they were given an open field test (OFT) 20.5 weeks after initial stress onset. 22 weeks after stress onset rats were euthanized (Sac). Blood was collected at three points to measure CORT responses: at the acute restraint test, immediately prior to UCMS, and at study termination.
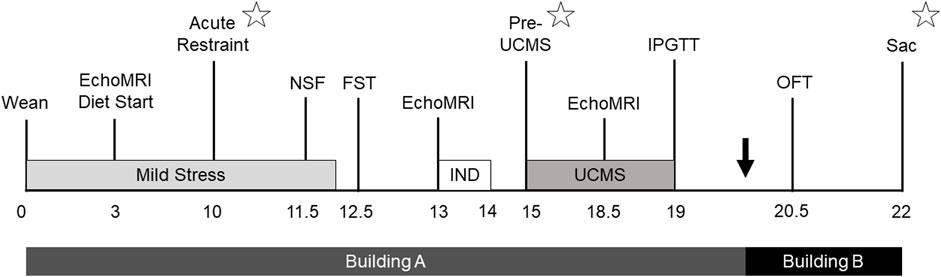
FIGURE 1. Study timeline. Timeline outlining study design, with weeks relative to stress start shown. Rats were housed in Building A from birth to approximately 20 weeks from stress start, and then Building B until study completion. Mild stress was administered starting after weaning and continued for 12 weeks, while 4 weeks of unpredictable chronic mild stress (UCMS) started 15 weeks after initial stress exposure. Metabolic phenotyping included EchoMRI analysis (EchoMRI), a week of individual housing (IND) to measure individual food intake, an intraperitoneal glucose tolerance test (IPGTT), and euthanasia (Sac). Behavioral tests included the novelty suppressed feeding test (NSF), forced swim test (FST), and open field test (OFT). Corticosterone was assessed 10 weeks after stress start at an acute restraint test, prior to UCMS 15 weeks into the study, and then at euthanasia (represented by star symbols).
Stress protocol designs
Mild stress protocol
We first started with a mild stress protocol that emphasized noise exposure in an effort to mimic the increased noise in the vivarium prior to COVID-19 shut-downs (Szalanczy et al., 2022). White noise exposure has previously been shown to increase CORT in rodents (Burow et al., 2005; Samson et al., 2007). We modified other stress-delivery protocols that exposed rodents to white noise (Campeau et al., 2002; Burow et al., 2005; Samson et al., 2007) by placing rats in a small, well-lit, enclosed room, 12 in away from a noise machine (Serene Evolution White Noise Machine, Amazon ASIN B08FZSGFSK) set to max volume (70 dB) that was low enough to avoid hearing loss (Escabi et al., 2019). In order to minimize acclimatization (Masini et al., 2008), the noise was cycled through different types of noise, different intervals, and different times of the day. The types of noise included white noise, brown noise, thunder, vacuum, and crowd. Noise exposure lasted from 1 to 3 h, delivered anytime between 8:00 to 17:00, and was randomly broken into the following intervals: straight, 1 h on and 30 min off, 30 min on and 30 min off, 40 min on and 20 min off, 15 min on and 15 min off. The noise machine could be turned on and off from outside the room.
In our first in vivo study conducted from 2019 to 2020, KO rats were larger than WT rats by 6 weeks of age (Szalanczy et al., 2022). In the current study, we did not see the anticipated changes to body weight at 6 weeks of age (HFD start) and concluded that the noise procedure described above was too mild to causes increases in CORT. We then altered the stress design by decreasing exposure to white noise to 2 days a week but adding exposure to an additional stressor one time per week, modified from those used in the UCMS paradigm (Willner, 2017): 30 min of restraint, a 5 min swim in 22°C water, a cage tilted at 45° for 2 hours, 5 min exposure to a cool air stream from a hair dryer, or 8 hours in a flooded cage (500 mL water added to bedding). This mild stress protocol lasted for 9 weeks after diet start, through the first round of behavioral tests discussed below. Noise and the additional stressors were administered variably between 8:00 to 17:00.
Unpredictable chronic mild stress (UCMS)
UCMS is an established, validated protocol for generating stress in rodents, inducing behavioral changes that correlate with increased anxiety-like and depressive-like symptoms (Willner, 2017; Willner, 2017). The UCMS procedure that we used was taken from the literature (Isingrini et al., 2010; Frisbee et al., 2015; Monteiro et al., 2015; Willner, 2017; Burstein and Doron, 2018; Alqurashi et al., 2022), with some modifications: rats remained pair-housed during the duration of stress delivery and stressors were administered 6 days per week instead of seven. Stressors were administered in either the housing room or different procedure rooms and included: a 2-h restraint in a flat-bottomed tube (see below), 15-min exposure to a cotton ball soaked in fox urine, a 5 min swim in water approximately 15°C, overnight exposure to soiled breeder bedding, 5 min exposure to a cool air stream from a hair dryer, 3–8 h in a flooded cage, and 2–3 h in a cage tilted at approximately 45°. UCMS began on Monday and ended just over 4 weeks later with an overnight fast for a glucose tolerance test (discussed below). The exact schedule of procedures for UCMS is given in Table 1. As with other UCMS protocols (Isingrini et al., 2010; Frisbee et al., 2015; Monteiro et al., 2015; Willner, 2017; Alqurashi et al., 2022) rats did not see the same stressor 2 days in a row, and timing of stress delivery varied from day to day. With the exception of overnight exposure to soiled bedding, all other procedures were given variably from 8:00 to 17:00. Four researchers contributed to UCMS stress delivery.
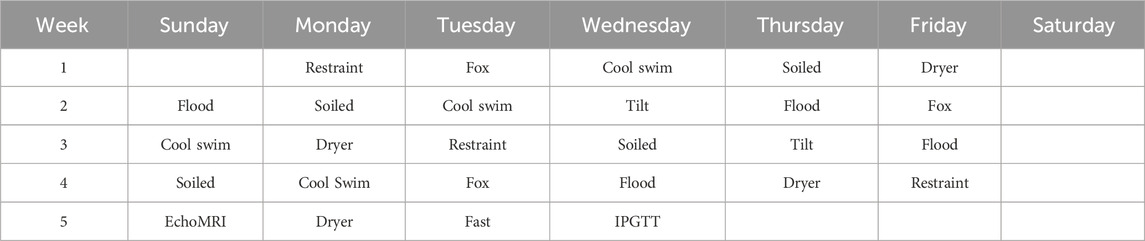
TABLE 1. Sequence of unpredictable chronic mild stress (UCMS) procedures. UCMS stressors included a two-hour restraint in a plastic tube (Restraint), a 15-min exposure to fox urine (Fox), a 5-min swim in cool (15°C) water (Cool Swim), overnight exposure to soiled bedding from breeder cages (Soiled), 5-min exposure to a cool air stream from a hair dryer (Dryer), 3 to 8 h exposed to a flooded cage (Flood), and 2–3 h exposed to a cage tilt at 45° (Tilt). UCMS began on a Monday, and stressors were delivered Sunday through Monday with Saturdays off. Stressors, excepting overnight exposure to soiled bedding, were administered randomly from 8:00 to 17:00, and rats did not see the same stressors twice in a row. On the last Sunday of the UCMS period rats had a third EchoMRI analysis, and UCMS concluded with an overnight fast and an intraperitoneal glucose tolerance test (IPGTT).
Metabolic phenotyping
Throughout the course of the study, body weight, and cage-wide food intake were measured weekly. EchoMRI analysis (EchoMRI LLC, Houston, TX) was conducted at three, 13, and 18.5 weeks after first stress exposure (Szalanczy et al., 2022). Immediately following the EchoMRI analysis 13 weeks after stress onset, rats began a seven day-long period of individual housing to measure food intake as previously described (Szalanczy et al., 2023). 19 weeks after stress onset, rats were fasted overnight before being administered an intraperitoneal glucose tolerance test (IPGTT) as performed elsewhere (Szalanczy et al., 2022; Szalanczy et al., 2023). We measured blood glucose (Contour Next EZ) at fasting and 15, 30, 60, 90, and 120 min after a 1 mg/kg glucose injection and calculated glucose area-under-the-curve (AUC) (Szalanczy et al., 2022; Szalanczy et al., 2023).
Blood collection for measurement of basal and restraint CORT
Acute restraint test
To determine if there were differences in basal CORT due to the mild stress protocol, we collected blood 10 weeks after mild stress onset. Rats were also subjected to a 30 min acute restraint test to investigate if there were different CORT responses by genotype to an acute physical stressor. The test was performed in the housing room in the morning (9:00–10:00) with two researchers. Rats were removed from the cage at the same time, placed in either a plastic flat-bottomed restraint tube (Braintree Scientific Cat #FB-ML or Cat #FB-L) or a restraint bag (Fisher Scientific Cat #14370112), and their tails nicked to collect serum (Sarstedt Inc. MicrovetteCB 300 LH). Basal collection was completed within 90 s of the rats being removed from the cage. After completing the basal collection, tails were taped to the surface for further restraint. 10 min later, rats were again bled from the tail, still restrained. After 30 min total, rats were bled a final time then removed from the restraint and returned to the home cage. Two cages could be run in the same 30 min chunk, staggered 5 min apart.
Pre-UCMS
We collected blood approximately 15 weeks after stress onset to obtain a basal CORT measurement prior to starting UCMS. Starting in the morning (9:00–10:00), rats were briefly removed from their cage, their tail nicked, and serum collected. This was performed in the housing room with two researchers—both rats were removed from the cage at the same time, and blood collection was completed within 90 s of removal. Rats were then either returned to the home cage (control rats) or remained restrained for another 90 min as the first stressor of UCMS (stressed rats).
Post-UCMS
We collected blood for basal CORT at euthanasia, described below under Tissue Harvest.
Behavioral analyses
Novelty suppressed feeding test (NSF)
About 11 weeks after mild stress exposure began, rats were administered the NSF to measure anxiety-like behaviors such as hyponeophagia (Dulawa and Hen, 2005) and exploratory behaviors. Rats were fasted for 24 h before the test began, then brought to a procedure room to acclimate for 30 min before the test started. A large box (67 cm × 61 cm × 46 cm) was placed in the center of the room under an overhead light with a camera positioned at one edge to look down over the box. The sides of the box were covered in black construction paper to minimize outside distractions. The bottom of the box was marked in red tape with two concentric circles (diameters 16 and 47 cm) and six lines radiating at 60° from the edge of the inner circle, dividing the box into 13 sections (McAuley et al., 2009). A small dish with three pellets of HFD was positioned in the center of the box and taped down to prevent movement. The video camera was started, a rat was removed from its cage and placed in the left corner facing the walls of the box, and then the researcher left the room and started a timer. The rat was left alone in the box for 15 min, at which point the researcher returned to the room to return the rat to its home cage. The box was cleaned with 70% ethanol between rats.
Researchers scoring these measures were blinded to the genotype and stress exposure of the rats, and the same researcher scored the same phenotype across all rats. Latency to feed, or how long it took the rat to begin eating, was defined as the rat chewing for at least four consecutive seconds. From that point, total time spent feeding was measured based on the total time the rat spent chewing. The number of line crossings, the number of center approaches, and time spent in the center were also measured. A line crossing was defined as all four paws crossing from one block into another. The smaller circle was considered the center of the box, and due to the blockage of the food dish, a rat was considered to have entered the center when both front paws were on or over the line. Time in center began when two front paws were in the center and ended when at least one paw left the center. Eating within the center was also counted within the center time. Increased latency to feed, decreased total feeding time, fewer line crossings, and decreased center time are all considered anxiety-like behaviors in rodents (Dulawa and Hen, 2005; Seibenhener and Wooten, 2015).
Forced swim test (FST)
Three days after the NSF, rats participated in a FST to measure passive coping to stress (Commons et al., 2017) and/or depressive-like behavior (Redei et al., 2022). Rats were placed in a tank of water (25°C ± 2, diameter 28.8 cm, height 49.8 cm, water depth 39 cm) for 15 min on Day 1 and 5 min on Day 2 as previously described (Solberg et al., 2004). Video recording of the first 5 min of Day 1 and the full 5 min of Day 2 were used to manually score movements made by the rat at 5 s intervals: immobility (floating or bracing) and mobility (swimming, climbing, or diving). Increased immobility is associated with increased passive coping (Commons et al., 2017) and/or depressive-like behavior (Redei et al., 2022).
Open field test (OFT)
20.5 weeks after stress exposure began, and 1.5 weeks after the conclusion of UCMS, an OFT was administered to measure locomotor activity and anxiety-like behaviors in the rats driven by the competing urges of exploring new environments but avoiding open, well-lit spaces (Seibenhener and Wooten, 2015). The test was conducted in Plexiglas chambers (41.5 cm × 41.5 cm × 30 cm). The center of the box was measured as a 20.5 cm × 20.5 cm square. At the start of the test, rats were placed in the chambers equipped with Omnitech Superflex Sensors (Omnitech Electronics, Inc., Columbus, OH), which utilize arrays on infrared photodetectors located at regular intervals along each way of the chambers. The chamber walls are solid and contained within sound-attenuating boxes with a 7.5-W white light to illuminate the arena. Exploratory activity in this environment was measured for 30 min and the data analyzed in 5-min time bins. The following activities were recorded: total distance moved, total time spent moving, number of rears and time spent rearing, number of center approaches, time spent in the center of the box, and distance traveled in the center of the box.
Tissue Harvest
Stress study
After 22 weeks of stress exposure and beginning at 8:00, rats were euthanized via decapitation after a 4 h fast. Rats were transferred to the anteroom of the necropsy suite 15 min prior to the start of the euthanasia protocol and were euthanized one at a time. Weight gain was calculated as the difference between the final body weight of the rats following the fast and the body weight at study start. Plasma was collected from trunk blood (Fisher Scientific Cat #02-657-32) and saved at −80 C. Body length from nose to anus and tail length from anus to tail tip were measured with a ruler. The brain, retroperitoneal (RetroFat), and liver were dissected, weighed, and snap-frozen. The pituitary and adrenal glands as well as the ovaries and sections of the ileum and colon were dissected and snap-frozen without being weighed. Adrenal glands were later weighed on a more precise scale. Kidneys, parametrial fat (ParaFat), and omental/mesenteric fat (OmenFat) were weighed but not saved.
Adolescent rats for Krtcap3 expression
To confirm the Krtcap3-KO in multiple tissues, four week-old control rats were transferred to the anteroom of the necropsy suite 30 min prior to euthanasia, without fasting. Rats were euthanized with CO2 for 5 min, then decapitated. The brain, RetroFat, liver, pituitary gland, adrenal gland, ovaries, a section of the ileum, and a section of the colon were dissected and immediately snap-frozen in liquid nitrogen.
Adrenocorticotropic hormone and corticosterone
To measure adrenocorticotropic hormone (ACTH) in plasma collected at euthanasia, we used a sandwich ELISA kit (AbCam Ref #ab263880). Plasma samples were diluted 1:4 in 1X Cell Extraction Buffer PTR. The plate was incubated for 15 min in the TMB substrate, then Stop Solution was added, and the plate was analyzed at 450 nm against a 4-parameter standard curve.
We used a CORT competitive ELISA kit (ThermoFisher Ref # EIACORT) to analyze serum/plasma corticosterone collected throughout the study. As guided by the manufacturer’s instructions, samples were diluted at least 1:100, and analyzed at 450 nm against a 4-parameter standard curve.
RNA extraction
RNA was extracted from fatty tissues using the RNeasy Lipid Tissue Mini Kit (Qiagen Cat # 74804). Non-fatty tissue, such as liver or intestine, were extracted by Trizol.
Real time quantitative PCR
Pro-opiomelanocortin (Pomc) expression from the pituitary was measured between rats from the stress study to determine if there were genotype or stress exposure-driven differences on expression of the precursor to ACTH. We also examined expression of the glucocorticoid receptor (GR) nuclear receptor subfamily 3 group C member 1 (Nr3c1) in liver, colon, and pituitary between females of the main study to assess changes in CORT receptor expression by genotype and stress exposure. Colon and pituitary have high Krtcap3 expression (Supplementary Figure S1) while we previously investigated Nr3c1 expression in the liver (Szalanczy et al., 2023). We also investigated expression of hydroxysteroid 11-beta dehydrogenase 2 (Hsd11β2), responsible for catalyzing CORT deactivation, in the colon to determine if there were differences in CORT processing.
We then measured Krtcap3 expression between control and stress-exposed WT females in pituitary, adrenal, and colon to determine if expression had changed following exposure to the stress protocols. Results from the adolescent rats (described below) confirmed KO rats did not have Krtcap3 expression in these tissues, and they were excluded from this analysis.
We assessed Krtcap3 expression in adolescent females to confirm that Krtcap3 expression was significantly knocked-out in multiple tissues. We examined expression in tissues pertinent to metabolism or stress response: pituitary, adrenal, liver, whole hypothalamus, ileum, ovaries, and RetroFat.
To measure gene expression, either Gapdh or β-actin were used as housekeeping genes. Primers for all genes are found in Table 2. Fold change of the corresponding gene of interest transcript was calculated by the following equation:
Where ΔCt was the difference between the crossing threshold (Ct) of the gene of interest and the housekeeping gene, and ΔΔCt the difference between each sample ΔCt and the average control ΔCt. For analysis of the adolescent rats, the average control ΔCt was the mean ΔCt of WT rats. In gene expression analyses in the main study, the average control ΔCt was the mean ΔCt of control WT rats.
Statistical analysis
All data were analyzed in R (1.4.1103). Outliers were assessed either by Grubb’s test or by 1.5 * the interquartile range for phenotypes where n < 6 and were removed. Distribution was assessed by the Shapiro-Wilks test and data were transformed to reflect a normal distribution if necessary. Homogeneity of variance was assessed by Levene’s test.
For adiposity, behavioral, and gene expression results, data were first analyzed considering all four groups together. If ANOVA analysis indicated relevant interactions, the data were deconstructed appropriately. However, several phenotypes showed a visually stronger effect in one genotype relative to the other, even if ANOVA could not detect an interaction. In these cases, according to our a priori hypothesis that WT and KO rats would respond differently to stress, we split the data by genotype and assessed the effect of stress.
Single point adiposity and behavioral measurements were first assessed by a two-way ANOVA, where one factor was genotype (WT v KO) and the other was stress exposure (control v stress). Growth curves and cage-wide food intake were analyzed by a two-way repeated measures ANOVA, where the effect of genotype and stress exposure were examined over time. We measured cage-wide food intake from diet start until the end of UCMS (weeks 4–19 of stress). Food consumed during the week of individual housing was calculated as the average food consumed per day and the total food consumed during the week for each rat. If there was an interaction, or visually WT and KO rats responded differently, we split the data by genotype to evaluate the effect of stress or the effect of stress and time together.
Based on our previous study (Szalanczy et al., 2023) we anticipated there being three factors to consider when analyzing CORT and ACTH data from euthanasia: genotype, stress exposure, and euthanasia order (if the rat was euthanized first or second within a cage). We first examined CORT and ACTH from euthanasia using a two-way ANOVA, with only data from Rat 1 which we presumed to represent the basal condition, removing the consideration for the factor of order. The data were then analyzed by a three-way ANOVA and split according to significant interactions. We did not include rat order as a factor in analyses of CORT collected from the acute restraint test or prior to the UCMS protocol as both rats were handled at the same time. We used a mixed effects model to analyze CORT during the acute restraint, with the factors of genotype, stress exposure, and time restrained; Tukey’s multiple comparisons test compared CORT between the time points. Given the large effect of time, we then also assessed CORT at each time point separately with a two-way ANOVA. To evaluate CORT prior to UCMS exposure, we used a two-way ANOVA.
In the NSF, latency to feed and total time spent feeding could not be analyzed by a two-way ANOVA because there was a significant ceiling effect, as over one-quarter of the rats did not participate in the test in the given timeframe. Instead, we chose to re-conceptualize the latency to feed and time spent feeding phenotypes as participation in the test, and to then analyze by methods similar to those for survival analyses. Rats who participated in the test (that is, had a latency to feed less than 15 min) were considered “death events” while those who did not participate “survived”. We then analyzed this by the Kaplan-Meier estimator and log-rank test to examine the effect of stress exposure between each genotype.
Krtcap3 expression between WT and KO adolescents in multiple tissues was assessed by a t-test per tissue, and p-values for multiple comparisons were adjusted via the Holm-Sidak method. This same method was applied to comparing Krtcap3 expression between WT control and stress animals from the main study. Expression analyses of the other genes, which included KO rats, were first analyzed by a two-way ANOVA, and then appropriately deconstructed to examine effects of stress per genotype and effects of genotype per stress condition.
Results
Verification of the Krtcap3 knock-out in multiple tissues
We verified the Krtcap3-KO in multiple tissues in female adolescent rats (Supplementary Figure S1). Compared to WT, KO rats had significantly lower Krtcap3 expression in all tissues that were assessed: pituitary (T3.13 = 11.95, p = 6.17e-3), adrenal (T4.77 = 11.03, p = 9.95e-4), liver (T3.02 = 6.94, p = 0.024), hypothalamus (T3.33 = 4.84, p = 0.026), ileum (T3.05 = 8.34, p = 0.017), ovary (T3.03 = 3.55, p = 0.038), and RetroFat (T5.46 = 4.35, p = 0.024) tissues.
Chronic stress exposure increased early fat mass and cage-wide food intake in both genotypes, but effects on body weight over time, individual food intake, and final adiposity are seen only in KO rats
As expected, there were no differences in body weight at weaning (3 weeks of age) between WT or KO rats, and no differences in weight between animals assigned to the control or the stress groups (Supplementary Figure S2A). Surprisingly, despite exposure to noise stress for 3 weeks, there were no differences in body weight by genotype nor by stress exposure at 6 weeks of age at HFD start (Supplementary Figure S2B). There was, however, a modest increase in total fat mass in noise-exposed rats compared to control rats for both genotypes (F1, 26 = 5.38, p = 0.03; Supplementary Figure S2C).
Contrary to what we had hypothesized, the entirety of the stress protocol described here increased the body weight of the rats over time (F2.42, 67.89 = 3.13, p = 0.041) rather than decrease it. When WT and KO growth curves were assessed separately, however, stress exposure ultimately did not impact WT body weight over time (Figure 2A), while it did significantly increase KO body weight over time (F22, 308 = 2.03, p = 0.005; Figure 2A). Similarly, from the time of HFD start until the end of UCMS, stress led to an increase in cage-wide food intake over time (F1, 12 = 7.5, p = 0.018) as well as a stress by week interaction (F14, 168 = 1.88, p = 0.031) where food intake increased over time only in the stress-exposed rats. Although this effect appears to be driven mainly by the KO rats (Figure 2B), thereby explaining their increase in body weight over time, a larger n is needed to pick up an interaction and/or determine significant differences within each genotype separately (with two rats per cage the n is only 4 for each genotype/stress group).
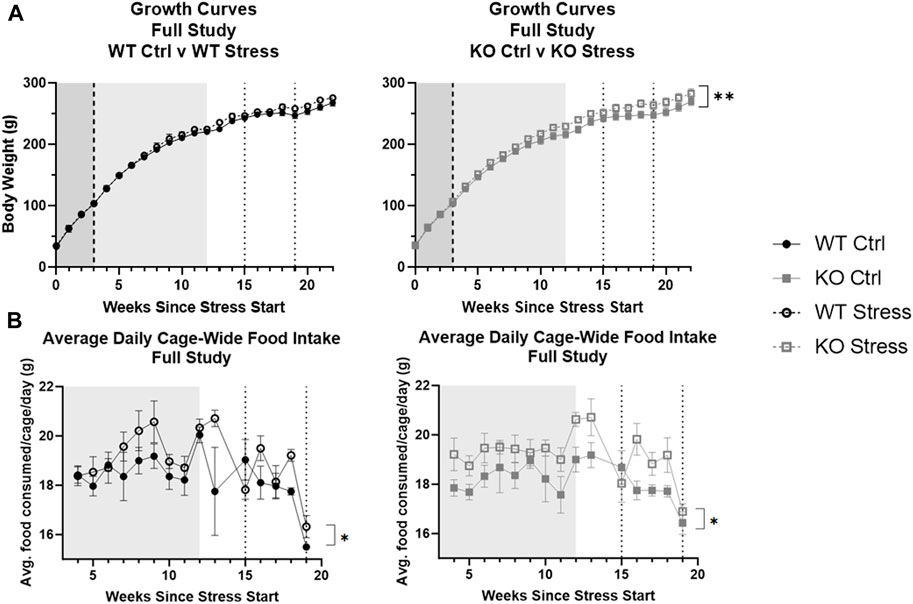
FIGURE 2. Stress exposure increased body weight (A) and food intake (B) over time, with a larger effect in KO rats. Data are split by genotype to assess differences due to stress exposure respective for WT and KO rats. Increases in body weight were found in Krtcap3 knock-out (KO, gray square) rats but not in wild-type (WT, black circle) rats. While increases in food intake also appear to be driven by KO rats, a larger n is needed. The mild stress period is demarcated by the shaded region from weeks 0–3 (white noise, dark gray) and from weeks 3–12 (white noise + additional mild stress, light gray), while the dotted lines at weeks 15 and 19 indicate the beginning and end of UCMS. High fat diet start is shown by the dashed line 3 weeks after the start of stress. Cage-wide food intake was measured from time of diet start to end of UCMS (4–19 weeks of stress exposure). **p < 0.01 represents an interaction between body weight and time only in the KO rats and *p < 0.05 is for the interaction between food intake and time based on the 2-way repeated measures ANOVA.
EchoMRI analysis performed 13 weeks after mild stress initiation showed that stress-exposed rats of both genotypes had increased total fat mass relative to control counterparts (F1, 27 = 16.18, p = 4.17e-4; Figure 3A) as well as increased total lean mass (F1, 27 = 8.74, p = 6.4e-3; Figure 3B).
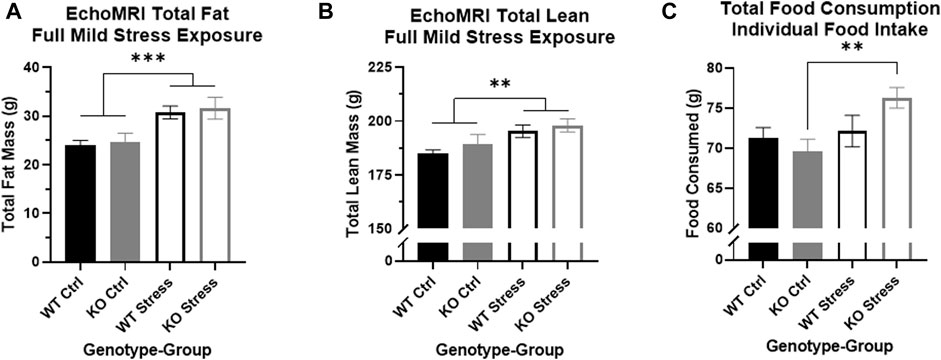
FIGURE 3. Mild stress exposure resulted in increased fat and lean mass in both genotypes, but increases in individual food intake are found only in the KO rats. (A) At the end of the mild stress protocol, EchoMRI analysis assessed total fat mass, and found that mild stress exposure had significantly increased total fat mass in both WT (black) and KO (gray) rats. ***p < 0.001 represents main effect of stress. (B) Similarly, mild stress exposure significantly increased total lean mass in both WT and KO rats. **p < 0.01 represents main effect of stress. (C) During the week of individual housing, stress-exposed KO rats consumed a greater quantity of food during the week, with no differences in WT eating behavior related to stress exposure. **p < 0.01 represents effect of stress in KO rats.
Within the single week of individual housing, 13 weeks after mild stress onset, there was a main effect of stress exposure on the sum of food consumed per rat (F1, 27 = 5.53, p = 0.026) but also a nearly significant interaction between genotype and stress exposure (F1, 27 = 3.44, p = 0.075). Between WT rats there were no differences in the total amount of food consumed during the week, but stress-exposed KO rats consumed a significantly larger quantity of food compared to control counterparts (T13 = 3.3, p = 5.53e-3; Figure 3C), supporting the visual difference seen for cage-wide food intake (Figure 2).
Four weeks of UCMS exposure did not alter the patterns we had seen during the earlier mild stress protocol. From start to end of UCMS (weeks 15-19), stress-exposed WT and KO rats gained more weight than control counterparts (F1, 28 = 435.1, p = 1.61e-5). They also ate more over time (F4, 48 = 6.80, p = 0.02) than control rats (Figure 2). EchoMRI analysis conducted at the conclusion of UCMS exposure determined that stress exposure significantly increased total fat mass (F1, 28 = 5.58, p = 0.025), which was driven by differences in the KO rats (T14 = 2.34, p = 0.035; Figure 4A). Stress exposure also increased total lean mass in both genotypes (F1, 27 = 4.34, p = 0.047; data not shown).
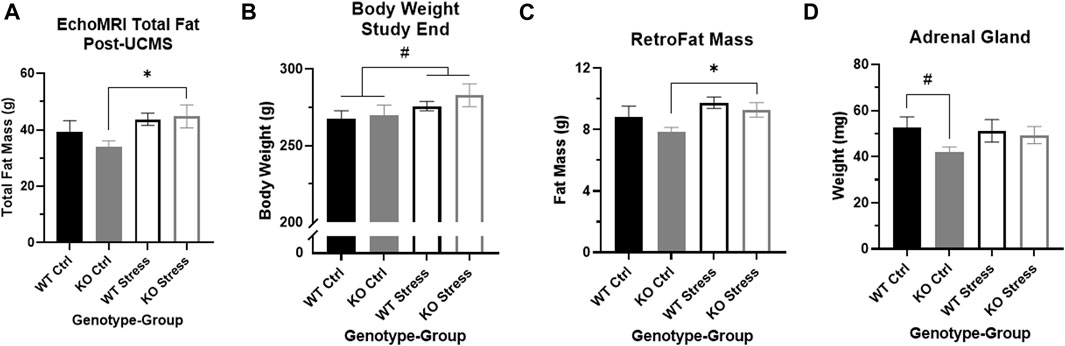
FIGURE 4. Stress exposure increased fat mass in Krtcap3 knock-out (KO, grey) rats but not wild-type (WT, black) rats. (A) Stress-exposed (empty) KO rats had significantly greater total fat mass relative to KO control (filled) rats, with no differences between WT rats. *p < 0.05 represents effect of stress for KO rats. (B) Stress-exposed rats of both genotypes had a slightly greater body weight at study conclusion compared to control rats. #p < 0.1 represents main effect of stress. (C) Stress-exposed KO rats had increased RetroFat mass compared to control counterparts, with no differences between WT rats. *p < 0.05 represents effect of stress for KO rats. (D) KO rats naive to stress had slightly smaller adrenal glands compared to control WT rats. #p < 0.1 represents effect of genotype for control rats.
At the end of the study, stress-exposed rats had a trend toward increased body weight compared to control rats, for both genotypes (F1, 28 = 3.46, p = 0.074; Figure 4B). Similar to the post-UCMS findings, there was a main effect of stress for RetroFat mass (F1, 26 = 5.43, p = 0.028), that was driven by the KO rats (T12 = 2.58, p = 0.024; Figure 4C) with no differences in WT rats. Stress had a modest impact on ParaFat (F1, 27 = 3.52, p = 0.071) and OmenFat (F1, 28 = 3.52, p = 0.071; data not shown). There were no differences in body length, tail length, or organ weight by genotype nor by stress exposure (data not shown). Control KO rats had a trend toward smaller adrenal glands compared to control WT rats (T13 = 2.07, p = 0.059; Figure 4D), although there were no differences in adrenal gland weight between stress-exposed WT and KO rats. While there is a visual increase in adrenal gland weight between control and stress-exposed KO rats, due to large variation this was not statistically significant.
No differences in fasting glucose or glucose tolerance by genotype nor by stress exposure
There were no differences in fasting glucose or glucose response to a glucose challenge, neither by genotype nor by stress exposure (data not shown).
Initial mild stress increased NSF anxiety-like behaviors in KO, but not WT
There was a significant interaction between genotype and stress exposure regarding the overall movement of the rat during the NSF, as measured by the number of line crossings (F1, 28 = 13.22, p = 1.1e-3), where stress-exposed KO rats moved much less than their control counterparts (T14 = 3.35, p = 4.8e-3; Figure 5A), with little difference in activity between WT groups (T14 = 1.78, p = 0.096; Figure 5A). There were similar results in the number of center approaches, with a significant interaction between genotype and stress exposure (F1, 26 = 6.23, p = 0.019) where stress-exposed KO rats approached much less frequently than control KO rats (T13 = 3.78, p = 2.3e-3; Figure 5B), with no significant differences between WT rats. When measuring the amount of time spent in the center there was a main effect of stress group, where control rats of both genotypes were more willing to spend time in the center of the box compared to stress-exposed rats (F1, 26 = 7.88, p = 9.4e-3; Figure 5C).
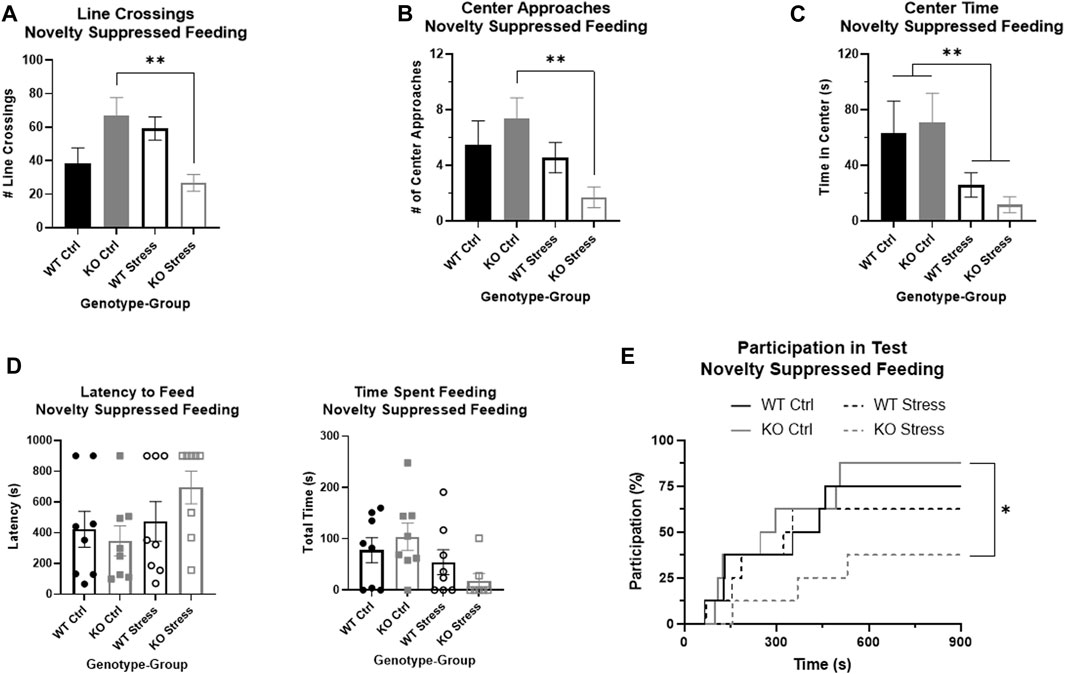
FIGURE 5. Novelty suppressed feeding test demonstrates that stress exposure did not greatly alter anxiety in wild-type (WT, black) rats, but did significantly increase anxiety in Krtcap3 knock-out (KO, gray) rats. (A) There was a significant interaction between genotype and group for number of line crossings where stress-exposed (empty) KO rats moved much less than control counterparts (filled) with no changes in WT rats. **p < 0.01 represents effects of stress respective to each genotype. (B) There were no differences in frequency of center approaches between control and stress WT rats, but stress-exposed KO rats approached the center fewer times than controls. **p < 0.01 represents effect of stress for KO rats. (C) For both WT and KO rats, stress-exposed rats spent much less time in the center of the field compared to controls. **p < 0.01 represents a main effect of stress. (D) When directly evaluating latency to feed and time spent feeding, there were ceiling and floor effects that precluded statistical analysis by a two-way ANOVA. Visually, there is little difference between control and stress-exposed WT rats (circle), but a large difference in KO rats (squares). Instead, we re-conceptualized these phenotypes as test participation instead. (E) Stress exposure (dashed line) had little effect on the probability of participation of WT rats compared to control counterparts (solid line), but KO rats exposed to stress were much less likely to consume food during the test compared to controls. *p < 0.05 represents effect of stress for KO rats.
Because of ceiling and floor effects, we could not accurately measure statistical differences in latency to feed or the total time a rat spent feeding (Figure 5D). We instead compiled these phenotypes into one that examined participation in the test and used modified survival curves to analyze the likelihood of a rat participating given its genotype and stress exposure. There were no significant differences in WT participation in the test due to stress exposure, but stress-exposed KO rats had a significantly lower likelihood of participating in the NSF than controls (Χ2 = 5.3, p = 0.02; Figure 5E).
Initial mild stress increased FST stress response only in KO rats
We evaluated immobility and mobility in the FST on Day 2, plus the change in immobility from the first 5 min of Day 1 to Day 2, as measures of passive coping response to stress. There were main effects by stress exposure for both immobility and mobility on Day 2: as expected, stress exposure increased immobility in the test (F1, 27 = 10.21, p = 3.5e-3; Figure 6A) and decreased mobility (F1, 27 = 10, p = 3.8e-3; Figure 6B). In addition, there was a strong effect of stress exposure on the change in immobility between the days (F1, 26 = 24.04, p = 4.34e-5; Figure 6C), where stress-exposed rats had a smaller change between the days. Importantly, there was a main effect of genotype (F1, 26 = 4.25, p = 0.049) driven by differences in the stress-exposed rats (T13 = 3.19, p = 7.2e-3; Figure 6C): KO rats had significantly greater immobility on Day 2 relative to Day 1, while WT rats had a slight decrease in immobility on Day 2 compared to Day 1. These differences support an increased response to FST stress in the KO rats exposed to mild stress relative to WT rats.
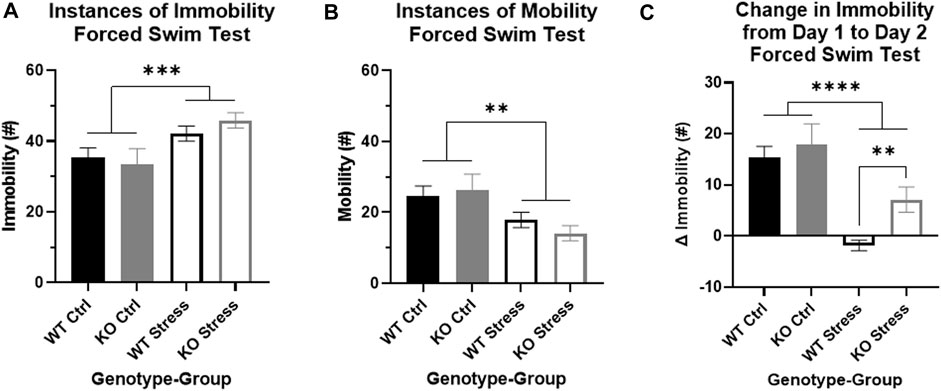
FIGURE 6. Forced swim test indicates that stress exposure more greatly alters stress coping in Krtcap3 knock-out (KO, gray) rats compared to wild-type (WT, black) rats. The FST is a 2-day test: Day 1 is a 15-min training swim while Day 2 is a 5-min test swim. (A) When compared to control rats (filled), stress-exposed rats (empty) of both genotypes had increased immobility and (B) decreased mobility compared to control rats (filled) on Day 2 of the test. **p < 0.01 represents a main effect of stress. (C) The change in immobility from Day 1 to Day 2 was significantly greater in control rats compared to stress-exposed rats, regardless of genotype. However, within the stress-exposed rats, immobility did not change in WT rats but had continued to increase between the days in KO rats, indicating differences in the stress coping response. **p < 0.01 represents effect of genotype respective to stress exposure; ****p < 0.0001 represents a main effect of stress.
KO rats are more exploratory in OFT relative to WT, and show decreased center entries in response to stress relative to control, with no stress-related changes in WT
After UCMS exposure, we used an OFT to measure locomotor and anxiety-like behavior in the rats. Over the full course of the test, there was a main effect of both genotype (F1, 27 = 4.23, p = 0.049; Figure 7A) and stress (F1, 27 = 4.23, p = 0.049; Figure 7A), where KO rats spent more time moving than WT rats and stress exposed rats spent more time moving than controls. KO rats also reared significantly more relative to WT rats (F1, 28 = 4.49, p = 0.043), though this was primarily in the control rats (T14 = 2.65, p = 0.019; Figure 7B) rather than the stress-exposed rats. KO rats also trended toward travelling a greater distance (F1, 27 = 4.09, p = 0.053; Figure 7C) than WT counterparts under both control and stress conditions.
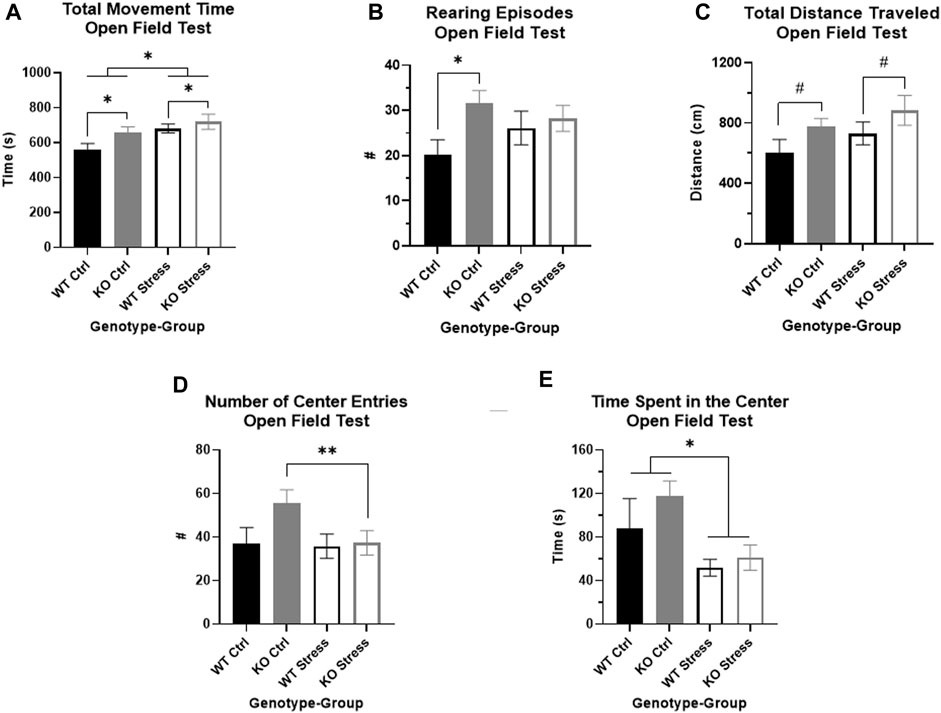
FIGURE 7. Open field test indicates that Krtcap3 knock-out (KO, gray) rats are more exploratory than wild-type (WT, black) rats. (A) KO rats spent more time moving compared to WT counterparts, plus stress-exposed (empty) rats of both genotypes spent more time moving compared to respective control counterparts (filled). *p < 0.05 represents a main effect of genotype and a main effect of stress. (B) Control KO rats reared more frequently than WT counterparts, demonstrating increased vertical exploration, while there were no differences in stress-exposed rats. *p < 0.05 represents an effect of genotype respective to stress condition. (C) Additionally, KO rats of both stress conditions travelled a slightly greater distance in the field compared to WT rats, indicating increased horizontal exploration. #p < 0.1 represents a main effect of genotype. (D) While the two-way ANOVA did not pick up an interaction between genotype and stress exposure, an examination of the genotypes separately revealed that stress exposure did not alter WT willingness to approach the center of the box, but stress-exposed KO rats approached much less frequently than control counterparts. **p < 0.01 represents an effect of stress for KO rats. This is consistent with results from the novelty suppressed feeding test, signifying that stress exposure more strongly impacted anxiety-related behaviors in KO rats than WT rats. (E) Also consistent with prior behavioral results, stress exposure for both genotypes did decrease amount of time spent in the center of the box. *p < 0.05 represents main effect of stress.
While the two-way ANOVA did not show an interaction between genotype and stress exposure that impacted number of center entries, we previously saw in the NSF that stress affected willingness to approach the center in KO rats but not WT. When we examined each genotype separately in the OFT, we found that there were no differences by stress exposure in WT rats, but stress-exposed KO rats entered the center of the box significantly less frequently than control counterparts (T13 = 3.78, p = 2.3e-3; Figure 7D). As we had previously seen in the NSF, stress exposure affected amount of time spent in the center, where stress-exposed rats of both genotypes spent significantly less time in the center than controls (F1, 27 = 4.41, p = 0.045; Figure 7E). Neither genotype nor stress exposure affected distance traveled in the center of the box, however (data not shown).
Mild stress exposure did not increase CORT in stress-exposed rats relative to control counterparts in both WT and KO rats
After 10 weeks of mild stress exposure, rats were administered an acute restraint test. There were no statistically significant differences in basal CORT by genotype nor by stress exposure (Figure 8A), although KO rats visually have a slightly lower basal CORT than WT. As restraint continued, CORT increased in all rats regardless of genotype or stress exposure (F2, 40 = 317.23, p = 2.9e-25), both after 10 (Q25 = 20.07, p < 1e-4; Figure 8B) and 30 (Q27 = 20.61, p < 1e-4; Figure 8B) min of restraint. When we examined the effect of genotype and stress exposure separately for each time point, there was a significant interaction after 10 min of restraint (F1, 26 = 4.73, p = 0.039) where stress-exposed KO rats had a lower CORT than control counterparts (T13 = 2.29, p = 0.039; Figure 8C), with no differences in WT rats. Although stress-exposed KO rats maintain a visually lower CORT after 30 min of restraint compared to control KO rats, there was no longer a statistically significant difference.
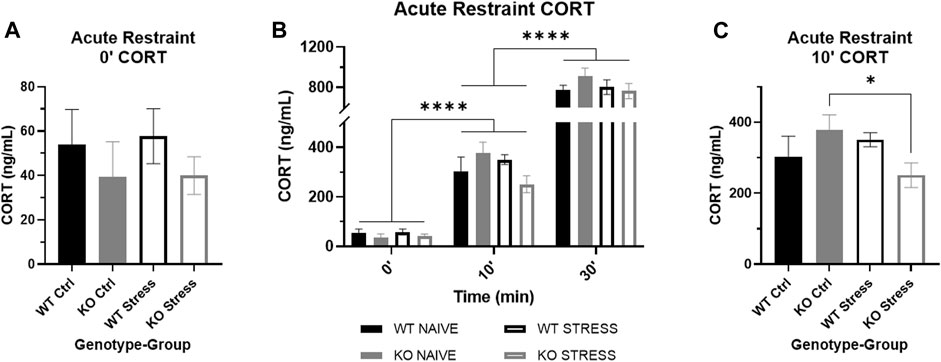
FIGURE 8. Corticosterone (CORT) response to acute restraint stress in wild-type (WT, black) and Krtcap3 knock-out (KO, gray) rats. (A) Serum basal CORT was measured immediately prior to acute restraint after 10 weeks of the mild stress protocol. Basal CORT was not increased in stress-exposed rats (empty) relative to control counterparts (filled). (B) Serum CORT measurements were also taken after 10 and 30 min of restraint. CORT significantly increased as restraint continued in all rats. ****p < 0.0001 represents effect of time for all groups. (C) After 10 min of restraint, there were no differences in CORT between WT rats, but stress-exposed KO rats had a lower CORT than control KO rats. *p < 0.05 represents effect of stress exposure in KO rats.
Prior to UCMS, at week 15, we measured serum CORT in the rats and saw no differences by genotype nor by stress exposure (Supplementary Figure S3). This confirmed that the mild stress protocol did not increase basal CORT in WT rats and indicates that the changes in adiposity and behavior in KO rats occurred despite lack of changes in basal CORT.
At the end of the study, CORT is lower in KO vs. WT under control conditions, and increases in response to stress in KO but not WT rats
We also measured CORT collected at euthanasia. Given prior results (Szalanczy et al., 2023), we expected that there would be an effect of euthanasia order on CORT, so we initially examined only the first rat of each cage, presumed to be the basal measurement. There was a significant interaction between genotype and stress exposure (F1, 12 = 9.93, p = 0.008), where stress-exposed KO rats had significantly elevated CORT relative to control counterparts (T4.67 = 2.91, p = 0.036; Figure 9A) yet contrary to expectation stress-exposed WT rats had a trend toward lower CORT relative to control counterparts (T5.98 = 2.11, p = 0.08). We do not fully understand why basal CORT was so high in control WT rats and these results may be confounded by an unknown stress at the time of sac. Similar to our previous work (Szalanczy et al., 2023), we also found that control KO rats have significantly lower serum CORT relative to control WT rats at the time of euthanasia (T3.59 = 3.31, p = 0.035; Figure 9A), indicating KO rats have lower basal stress compared to WT rats, supporting the behavioral studies described above. We show serum CORT over time for both rats in the cage and just rat 1 (Supplementary Figures S3A, B). Note that because conditions of serum CORT collection differed at each time-point, time-points may not be directly comparable.
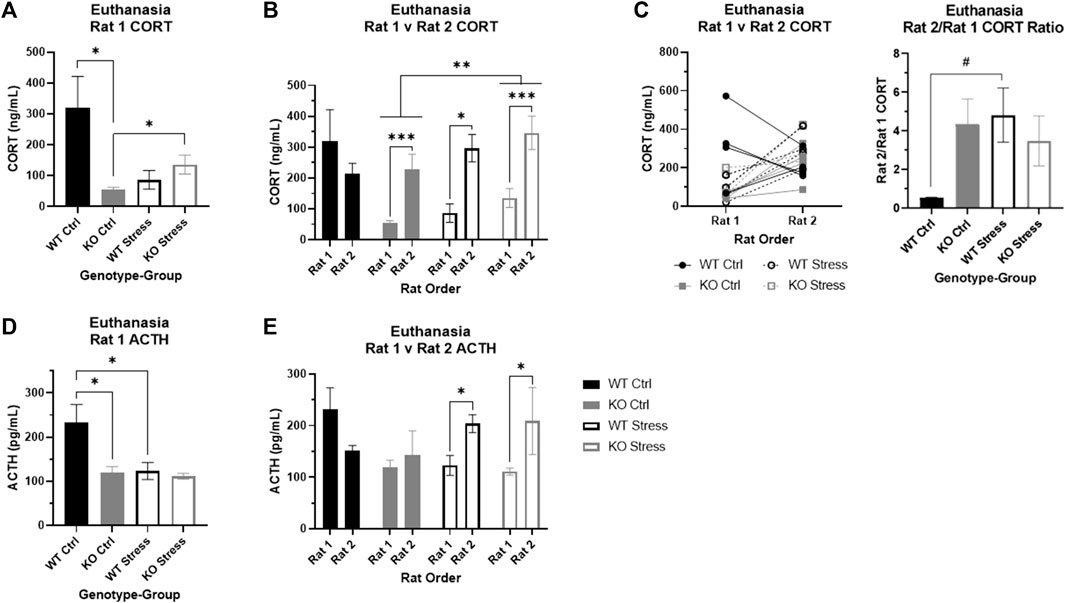
FIGURE 9. Corticosterone (CORT) and adrenocorticotropic hormone (ACTH) at euthanasia in wild-type (WT, black) and Krtcap3 knock-out (KO, gray) rats. Serum was collected from trunk blood at euthanasia, and rats were euthanized within the cage one at a time, with Rat 1 euthanized first and Rat 2 euthanized second. (A) Control (filled) KO rats had lower basal CORT compared to control WT rats. Additionally, CORT of KO Rat 1 was greater in those that were stress-exposed (empty) compared to control rats, but not for WT rats. *p < 0.05 represents effect of genotype in control rats and effect of stress exposure in KO rats. (B) We confirmed that stress exposure increased CORT in KO rats, for both Rat 1 and Rat 2. **p < 0.01 represents effect of stress exposure in KO rats. Additionally, we found that KO rats show a significant increase in CORT when their cage-mate is removed at euthanasia regardless of stress exposure; we found the same pattern in stress-exposed WT rats, but not control WT rats. *p < 0.05 represents effect of euthanasia order in WT rats, ***p < 0.001 represents effect of euthanasia order in KO rats. (C) The line graph demonstrates the difference in CORT between Rat 1 and Rat 2 of each cage, where WT rats are represented by black circles and KO rats by gray squares. Control rats are marked with filled symbols and a solid line, while stress-exposed rats have empty symbols and dashed lines. The bar graph examines the CORT ratio between Rat 1 and Rat 2 for each genotype and stress condition confirmed that stress exposure does not alter this ratio in KO rats, but does increase the ratio in WT rats. #p < 0.1 represents effect of stress for WT rats. (D) ACTH was lower in control KO Rat 1 compared to control WT rats. Further, ACTH was lower in stress-exposed WT Rat 1 relative to control counterparts. *p < 0.05 represents effect of genotype in control rats and effect of stress exposure in WT rats. (E) We found that ACTH is significantly increased in Rat 2 for stress-exposed rats, whether WT or KO. There were no differences by order in control rats. *p < 0.05 represents effect of order in stress-exposed rats.
Euthanasia order alters serum CORT in both control and stress-exposed KO rats as well as stress-exposed WT rats
We next assessed the impact of euthanasia order in addition to genotype and stress exposure. Similar to our previous work (Szalanczy et al., 2023), there was a significant effect of order (F1, 24 = 21.01, p = 1.2e-4), with rats euthanized second showing higher serum CORT. There was still an interaction between genotype and stress exposure (F1, 24 = 8.65, p = 7e-3), but there was also a three-way interaction between genotype, stress exposure, and euthanasia order (F1, 24 = 6.6, p = 0.017). We split the data by genotype to confirm the impact of stress exposure and order on CORT, and found a significant main effects of stress (F1, 12 = 9.53, p = 9e-3; Figure 9B) and order (F1, 12 = 29.72, p = 1.47e-4; Figure 9B) in KO rats, demonstrating that chronic stress exposure increased CORT in the KO rats and that CORT rises in the second rat of the cage regardless of prior stress exposure. In WT rats, we found an interaction between stress exposure and order (F1, 12 = 6.28, p = 0.028) where order did not impact CORT in control WT rats but CORT was significantly elevated in the second rat of the cage in stress-exposed WT rats (T5.25 = 3.91, p = 0.01; Figure 9B).
These findings were supported by additional analyses of the CORT ratio between the first and second rat of the cage for each genotype and stress condition. There was a nearly significant interaction between genotype and stress exposure (F1, 11 = 4.21, p = 0.065) where WT control rats had a much lower ratio relative to the other three groups (Figure 9C). These data indicate a stronger social stress response to removal of cage-mate in KO rats, which is seen only in WT rats when they have previously been exposed to chronic stress.
Basal ACTH is lower in KO vs. WT rats under control conditions
Given that there was a significant effect of euthanasia order on CORT, we initially examined ACTH in only the first rats of the cage. There were main effects of genotype (F1, 12 = 5.83, p = 0.033) and stress exposure (F1, 12 = 5.94, p = 0.031) where control WT rats had greater basal ACTH than control KO rats (T4.98 = 3.02, p = 0.03; Figure 9D) and stress-exposed WT rats (T5.99 = 2.46, p = 0.049; Figure 9D).
ACTH increases in response to separation at euthanasia only in stress-exposed rats, regardless of genotype
When we incorporated data from the second rats and the factor of euthanasia order into the analyses, there was an interaction between stress exposure and order (F1, 24 = 5.76, p = 0.025) separate from genotype. Specifically, in control rats there were no differences in ACTH between the two rats of the cage, whereas in stress-exposed rats the second rat of the cage had elevated ACTH compared to the first rat (T1, 14 = 8.71, p = 0.011; Figure 9E).
Expression of the GR Nr3c1 differs between genotypes and stress exposure in pituitary, liver, and colon
We investigated Nr3c1 expression in the pituitary, colon, and liver. We looked in the pituitary and colon because both tissues have high Krtcap3 expression (Szalanczy et al., 2023) and each are involved in stress response (Herman et al., 2016; Wiley et al., 2016), indicating that they may be the tissues of action for Krtcap3. Further, we also sought to verify previously reported changes in Nr3c1 expression in the liver in response to stress (Szalanczy et al., 2023). We found a very nearly significant main effect of stress-exposure on Nr3c1 expression in the pituitary (F1, 27 = 4.15, p = 0.052), where stress-exposed KO rats had greater Nr3c1 expression in the pituitary than control counterparts (T14 = 2.33, p = 0.035; Figure 10A) with no differences in expression between WT rats. Similarly, there was a near interaction in colon Nr3c1 expression (F1, 25 = 4.2, p = 0.051) where there was no difference by stress exposure in WT rats, but stress exposure resulted in a trend toward decreased Nr3c1 expression in KO rats (T13 = 2.06, p = 0.06; Figure 10B). In the control condition, KO rats also had significantly higher colon Nr3c1 expression than WT rats (T13 = 2.2, p = 0.047; Figure 10B). These findings mimic the metabolic and behavioral findings, where differences are seen between control and stress-exposed KO rats but not between stress conditions in WT rats, further supporting an important role for Krtcap3 in the stress axis. As expected, Nr3c1 expression was also significantly increased in the liver tissue of stress-exposed rats (F1, 27 = 4.51, p = 0.043), but the difference here was driven by the WT rats (T13 = 3.11, p = 8.2e-3; Figure 10C) with no changes in the KO rats. Similar to our findings in the colon, we also identified a difference in liver Nr3c1 expression between control WT and KO rats, where KO rats had significantly higher expression (T13 = 2.26, p = 0.041; Figure 10C). On the other hand, there were no differences in pituitary Pomc expression or colon Hsd11β2 by genotype or by stress exposure (data not shown).
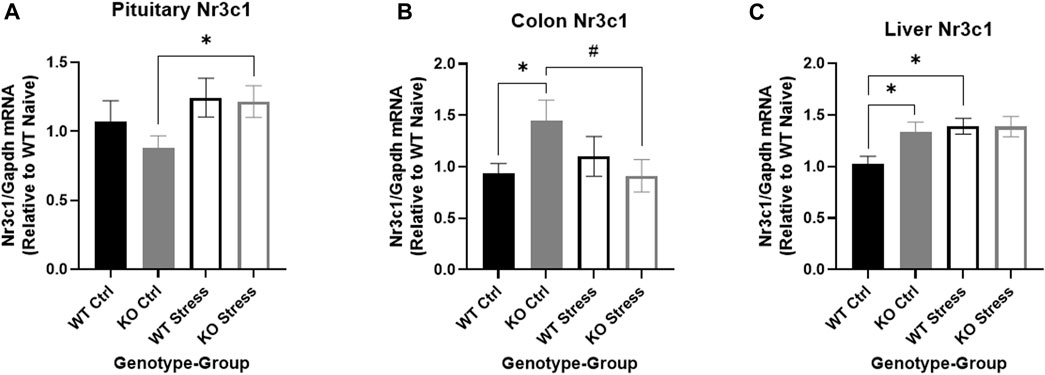
FIGURE 10. Differential expression of the glucocorticoid receptor Nr3c1 by genotype and stress exposure in pituitary, colon, and liver. We compared Nr3c1 expression between wild-type (WT, black) and Krtcap3 knock-out (KO, gray) control rats (filled) and exposed to stress (empty) in multiple tissues. (A) Stress-exposed KO rats had greater pituitary Nr3c1 expression than control counterparts, with no significant difference in WT rats. *p < 0.05 represents effect of stress for KO rats. (B) In the colon, control KO rats had greater Nr3c1 expression than control WT and greater expression than stress-exposed KO rats. #p < 0.1 represents effect of stress for KO rats, *p < 0.05 represents effect of genotype for control rats. (C) In the liver control WT rats had lower Nr3c1 expression than control KO rats and stress-exposed WT rats. Stress exposure did not alter expression in KO rats. *p < 0.05 represents effect of stress for WT rats and effect of genotype for control rats.
Krtcap3 mRNA expression does not change between control and stress-exposed WT rats
We measured Krtcap3 expression between control and stress-exposed WT rats in key tissues to evaluate if stress exposure altered expression. Ultimately, there were no differences in Krtcap3 expression between control and stress-exposed WT rats in the pituitary, the adrenal, or the colon (data not shown).
Discussion
We demonstrate here that chronic stress impacted both metabolic and behavioral phenotypes to a greater extent in KO rats than in WT rats. Although the differences were mild, stress-exposed KO rats gained more weight over time, ate more food when isolated, and had greater fat mass relative to control counterparts. Stress-exposed KO rats also exhibited increased anxiety-like behaviors relative to control KO rats and had an increased response to FST stress than stress-exposed WT rats. In contrast, stress-exposed WT rats showed minimal differences in all these measures compared to control WT rats. These data support an important role for Krtcap3 in the stress response and endorse that its impact on adiposity is influenced by stress, specifically that rats with low Krtcap3 expression favor increased eating when stressed. Similar to previous work (Szalanczy et al., 2023), KO rats in either stress condition demonstrate a strong CORT response when their cage-mate is removed at euthanasia, indicating that KO rats are more sensitive to psychosocial stress. In addition, we found that chronic stress altered Nr3c1 expression in pituitary and colon only in the KO rats, while control KO rats have higher Nr3c1 expression in the colon and liver compared to WT. While more work remains to be done to elucidate the role of Krtcap3 in the stress pathway, this work is the first to demonstrate that Krtcap3 plays a role in the stress response of WKY rats and that this has downstream impacts on adiposity and behavior.
It is important to note that serum CORT increased in KO rats exposed to the stress paradigm, but not in WT. These findings indicate that the stress protocol in this study differs from conditions we have previously reported where we saw increased CORT only in the WT rats (Szalanczy et al., 2022; Szalanczy et al., 2023). Despite these differences, however, we have demonstrated that Krtcap3 KO rats consistently exhibit increased eating and adiposity when exposed to stress. In the current work, we initially used a noise stress and this may not have been powerful enough to recapitulate construction vibrations (Reynolds et al., 2018; Terashvili et al., 2020) that were likely present in the first study. Because early measures of serum CORT did not increase in response to the noise stress in either genotype, we chose to employ a standard stress protocol, UCMS, which is well known for inducing depression and anxiety-like behaviors in rats (Willner, 2017). It is important to acknowledge, however, that the earlier noise stress may have altered the rats’ response to the later UCMS stress, possibly even protecting them against this later stress, as shown for other models of early life stress (Meaney et al., 1991; Bilbo et al., 2008; Xu et al., 2011; Genty et al., 2018; Genty et al., 2018; Tran and Gellner, 2023). We therefore propose that the lack of metabolic, behavioral, and CORT differences between WT control and stress-exposed rats are because:
1) Early mild stress led to desensitization of subsequent stressors (in our case, UCMS) only in WT rats (Xu et al., 2011; Mileva et al., 2017; Morera et al., 2020; Tran and Gellner, 2023),
2) WT rats could not distinguish between the chronic stress procedure and the stress of the study design. While control rats were not exposed to the noise and UCMS stress protocols, they participated in numerous metabolic and behavior tests as well as repeated blood collections that would have induced stress. Or,
3) Some combination of both.
Given the literature supporting early mild stress as potentially protective and that WKY rats are known to be stress-sensitive (Redei et al., 2022), it is likely a combination of both. Upcoming work from the Redei lab also suggests that WKY rats are chronically stressed when administered multiple behavioral tests despite long periods of rest in between (personal communication). If WT rats were not able to distinguish between the stress protocol and the study protocol, this work may indicate that low Krtcap3 expression levels “normalize” HPA axis function and stress response in WKY rats. This is supported by 1) low basal stress, 2) the expected behavioral, metabolic, and CORT responses to the chronic stress protocol, and 3) the expected changes in pituitary and colon Nr3c1 expression levels in KO, but not WT, rats. Future work is necessary to better clarify how WT and KO rats respond to stressors, and to compare the Krtcap3-KO rats to other strains in order to test this compelling hypothesis.
Stress induced greater changes in adiposity and eating behavior in KO rats than WT
As opposed to the suspected decrease in food intake and adiposity in response to construction noise in WT rats from our previous study (Szalanczy et al., 2023), the chronic stress protocol here increased body weight over time only in the KO rats, with no effect in WT rats. Although stress exposure increased adiposity in both WT and KO rats early in the study, by the end the difference was only maintained in KO rats. Our findings here are consistent with the hypothesis that stress is necessary to increase eating and adiposity in rats with low Krtcap3 expression. The increased adiposity in response to stress in the KO rats from the current study more closely aligns with changes seen following psychosocial stress rather than physical stress (Patterson and Abizaid, 2013) and may result from the number of times cage-mates were separated during the study. In support of this, when all rats were socially isolated, the stress-exposed KO rats ate significantly more food compared to control KO rats. We posit that prior stress-exposure increased susceptibility to the psychosocial stress of social isolation in KO rats, who may have increased food intake to ameliorate the anxiety-inducing effects of the separation (Maniam and Morris, 2010; Kistenmacher et al., 2018; Hyldelund et al., 2022), while WT rats were unaffected. Overall, we show that KO rats responded differently to stress than WT rats and that these differences influenced adiposity. Fully elucidating the difference in response between WT and KO rats to different stressors and understanding the impact on adiposity is a key goal of future studies.
Stress exposure increased anxiety-like behaviors and passive coping only in KO rats
As we saw larger adiposity differences between control and stress-exposed KO rats, so too did we see larger behavioral changes in KO rats compared to WT, with greater increases in anxiety following stress exposure. Importantly, control KO rats exhibited lower anxiety measures in the OFT compared to WT rats, supportive of a lower basal stress state. In response to chronic stress, KO rats showed the expected increases in thigmotaxis in the OFT as well as hyponeophagia and significant decreases in exploratory activity in the NSF. WT rats, on the other hand, displayed only minimal differences in these measures after stress exposure, in contrast to findings after chronic restraint stress in WKYs (Jung et al., 2020). We also found that immobility in the FST increased in stress-exposed KO rats from Day 1 to Day 2 of the test, with minimal changes between days in stress-exposed WT rats. This suggests a greater passive coping response (Commons et al., 2017; Molendijk and de Kloet, 2019) or greater depressive-like behavior (Redei et al., 2022) in KO rats following early life stress. As the WKY rat is hyper-sensitive to stress (Redei et al., 2022), these findings support that WT rats may have been unable to distinguish between the stress of the study design (control rats) and the chronic stress procedures (stress-exposed rats): it is possible that both groups of rats were chronically stressed. The fact that KO rats show the expected changes in emotional behaviors in response to chronic stress suggests that low Krtcap3 expression may normalize the stress response in WKY rats, although more work is needed to test this hypothesis. If low Krtcap3 expression normalizes the stress response in WKY rats, then this may also explain why only KO rats consumed more HFD when stressed, as occurred during social isolation. Taken together, findings in the NSF, OFT, FST, and food intake under social isolation indicate that stress exposure leads to the expected increases in anxiety-like and depression-like behaviors when Krtcap3 expression is low.
KO rats exhibit lower basal CORT and increased CORT response to both chronic stress and an acute psychosocial stress relative to WT rats
Differences in plasma CORT and ACTH between WT and KO rats at euthanasia indicate that Krtcap3 expression likely plays a role in HPA axis function. As before (Szalanczy et al., 2023), we found that control KO rats had lower basal plasma CORT compared to control WT rats, supported by lower basal ACTH and slightly smaller adrenal glands. At the end of the study, stress-exposed KO rats had greater CORT than control counterparts with no differences in basal ACTH and a mild increase in adrenal gland weight. These data support that KO rats may have a more normal HPA axis response to chronic stress compared to WKY rats: increased basal CORT following stress may in turn lead to increased passive coping and anxiety-like behaviors, including increased food intake. Contrary to our initial expectations, stress-exposed WT rats did not have greater CORT than control WT rats and in fact had lower basal ACTH than control WT rats, which was surprising as prolonged stress is known to increase ACTH in WKYs (Pardon et al., 2003; Malkesman et al., 2006). Furthermore, there were no differences in adrenal gland weight between control and stress-exposed WT rats. These findings potentially support the hypothesis that control WT were chronically stressed due to the study design, although we are unable to distinguish between this hypothesis and the possibility that the early noise stress had a protective effect on later UCMS stress in these rats.
We also confirmed here that KO rats of either stress condition experienced a spike in CORT when their cage-mate is removed at euthanasia (Szalanczy et al., 2023), supporting that KO rats are more sensitive to psychosocial stress. Interestingly, we identified this same pattern in stress-exposed WT rats, but not control WT rats. It is possible that prior stress exposure sensitized the HPA axis to a novel acute stress (Franco et al., 2016) in WT rats, although the adiposity and behavioral data suggest this is not the case.
It is unclear why control WT rats had such a high basal CORT at euthanasia compared to the other groups. One explanation for this finding is that control WT rats had not fully adjusted to the room change for euthanasia, and the room change stress is responsible for the differences in basal CORT between control WT and KO rats at euthanasia. A second possibility is that the large change in housing environment to a different building coupled with a behavioral test in the final weeks of the study may have more greatly affected control WT rats than KO rats. This also supports that WT and KO rats respond to different stressors differently, as supported by work in other models (Singh et al., 1999; Kogler et al., 2015). Future studies to evaluate the neurocircuitry between WT and KO rats, before and after stress, are warranted to fully understand these differences in response (Bangasser et al., 2013).
Nr3c1 expression differs in control and stress-exposed KO rats, with no differences in WT
The pattern of expression for the GR Nr3c1 may provide insight into the differences seen between WT and KO rats in the current study. We measured Nr3c1 expression in the pituitary, colon, and liver of WT and KO rats; while we only explored changes in gene expression here, the connection between Krtcap3 and Nr3c1 is also supported by evidence of protein-protein interactions elsewhere (Lievens et al., 2016).
The pituitary has high Krtcap3 expression in female WKY rats and is key to the HPA axis. The exact regulation of the GR under chronic stress conditions is unclear. Foundational in vitro work demonstrated that GR expression decreases following GC exposure in a mouse corticotrope or pituitary cell line (Sheppard et al., 1991; Williams et al., 1991) which aligns with findings in humans that early life stress is associated with methylation of NR3C1 in saliva or blood, leading to decreased gene expression and dysregulated HPA axis function (van der Knaap et al., 2015; Alexander et al., 2018; Holmes et al., 2019; Chatzittofis et al., 2021; Lewis et al., 2021; Chubar et al., 2023). However, in vivo work where animals are subjected to up to a week of stress has shown that expression of the GR increases in the pituitary (Sheppard et al., 1990; Nishimura et al., 2004; Noguchi et al., 2010). Similarly, in the current study we found that pituitary Nr3c1 expression increased when KO rats were exposed to stress, with no changes in WT rats. These findings further support that low Krtcap3 expression may “normalize” the HPA axis of the WKY rat. A change in pituitary Nr3c1 expression after stress exposure may be related to negative feedback regulation pathway of the HPA axis (Gjerstad et al., 2018) and could clarify the role of Krtcap3.
Krtcap3 expression is also high along the gastrointestinal tract of female WKY rats and can also be connected to the stress response (Wiley et al., 2016). Other work has shown that chronic stress decreases colonic Nr3c1 (Zheng et al., 2017; Muir et al., 2023), similar to our findings in the KO rats, lending further credence to the hypothesis that low Krtcap3 expression “normalizes” the HPA axis of WKY rats. In the colon we found that control KO rats have greater Nr3c1 expression than control WT rats and that stress exposure decreased Nr3c1 expression in KO rats to levels comparable to the WT rats. These data may indicate that control KO rats have improved intestinal integrity over WT rats due to the comparatively high Nr3c1 expression, but upon exposure to chronic stress and a HFD, Nr3c1 expression may decrease to minimize damage (Aranda et al., 2019; Shukla et al., 2022). The lack of change in Nr3c1 expression in WT rats after stress exposure supports that WT and KO rats responded to the study design differently. Krtcap3 may be acting in either the pituitary, the gastrointestinal tract, or both to influence GR expression and the effect of glucocorticoids on metabolism and behavior.
In the current study, we found that chronic stress increased liver Nr3c1 expression only in the WT rats, where previous work demonstrated increases in both WT and KO rats (Szalanczy et al., 2023). This highlights that the stress administered in this study did not recapitulate the stress in our previous work and that the role of Krtcap3 in stress response, and its relationship with Nr3c1 expression, is tissue-dependent (Costello et al., 2022). There remains a need to better understand how different types of stress regulate expression of the GR in different tissues and to identify downstream effects on health.
We did not see changes in Krtcap3 expression in the pituitary, adrenal, or colon between control and stress-exposed rats. Given that the control and stress-exposed WT rats had similar responses throughout the study, this finding is not surprising. Future work will therefore be needed to determine if stress alters Krtcap3 expression among these tissues.
Conclusion
This work confirms that Krtcap3 expression affects the stress response, with indirect effects on adiposity and behavior. We found here that Krtcap3-KO rats were susceptible to the current stress paradigm, with corresponding increases in adiposity and anxiety-like behavior. Despite exposure to the same stressors, there were minimal differences in these measures between control and stress-exposed WT rats. The data here demonstrate that decreased Krtcap3 expression, when combined with environmental stress, predisposes rats towards increased eating and adiposity. While we also suspect that control WT rats were chronically stressed due to study design, future work is needed to test this. This could indicate that KO rats are better able to distinguish between and adapt to stressors than WT rats, and that they respond to stress in an obesity-promoting manner. That Nr3c1 expression increases in the pituitary and decreases in the colon in the KO, but not WT rats, supports the hypothesis that low Krtcap3 expression may “normalize” the HPA axis of WKY rats, although again, future studies are needed to test this. Importantly, the potential interaction between Krtcap3 and Nr3c1—either at the mRNA level or at protein level—may explain the connection between Krtcap3 expression, stress, metabolism, and behavior and will be key to further explorations.
Data availability statement
The raw data supporting the conclusion of this article will be made available by the authors, without undue reservation.
Ethics statement
The animal study was approved by the Wake Forest Institutional Animal Care and Use Committee. The study was conducted in accordance with the local legislation and institutional requirements.
Author contributions
AMS designed the study, conducted experiments, oversaw assisting researchers, ran statistical analysis, analyzed results, created figures, and wrote the manuscript. LCSW designed study, oversaw experimental work, and edited manuscript. MF, TB, SE, and JL assisted with experiments. MF also assisted with statistical analysis. AB scored the FST; JL and CS scored the NSF test. CD coordinated rat care at Building B and ran the OFT; JLW consulted on behavioral data. MG, JK, and AMG created the rat knock-out model. EER consulted on stress hypotheses. All authors contributed to the article and approved the submitted version.
Funding
R01 DK106386, T32 DA041349, and R01 DK120667.
Acknowledgments
As always, the authors thank the MCW Genotyping Core for their assistance in genotyping the rats.
Conflict of interest
The authors declare that the research was conducted in the absence of any commercial or financial relationships that could be construed as a potential conflict of interest.
Publisher’s note
All claims expressed in this article are solely those of the authors and do not necessarily represent those of their affiliated organizations, or those of the publisher, the editors and the reviewers. Any product that may be evaluated in this article, or claim that may be made by its manufacturer, is not guaranteed or endorsed by the publisher.
Supplementary material
The Supplementary Material for this article can be found online at: https://www.frontiersin.org/articles/10.3389/fgene.2023.1247232/full#supplementary-material
References
Abadi, A., Alyass, A., Robiou du Pont, S., Bolker, B., Singh, P., Mohan, V., et al. (2017). Penetrance of polygenic obesity susceptibility loci across the body mass index distribution. Am. J. Hum. Genet. 101 (6), 925–938. doi:10.1016/j.ajhg.2017.10.007
Alexander, N., Kirschbaum, C., Wankerl, M., Stauch, B. J., Stalder, T., Steudte-Schmiedgen, S., et al. (2018). Glucocorticoid receptor gene methylation moderates the association of childhood trauma and cortisol stress reactivity. Psychoneuroendocrinology 90, 68–75. doi:10.1016/j.psyneuen.2018.01.020
Alqurashi, G. K., Hindi, E. A., Zayed, M. A., Abd El-Aziz, G. S., Alturkistani, H. A., Ibrahim, R. F., et al. (2022). The impact of chronic unpredictable mild stress-induced depression on spatial, recognition and reference memory tasks in mice: behavioral and histological study. Behav. Sci. (Basel) 12 (6), 166. doi:10.3390/bs12060166
Aranda, C. J., Arredondo-Amador, M., Ocon, B., Lavin, J. L., Aransay, A. M., Martinez-Augustin, O., et al. (2019). Intestinal epithelial deletion of the glucocorticoid receptor NR3C1 alters expression of inflammatory mediators and barrier function. FASEB J. 33 (12), 14067–14082. doi:10.1096/fj.201900404RR
Atrooz, F., Alkadhi, K. A., and Salim, S. (2021). Understanding stress: insights from rodent models. Curr. Res. Neurobiol. 2, 100013. doi:10.1016/j.crneur.2021.100013
Bangasser, D. A., Lee, C. S., Cook, P. A., Gee, J. C., Bhatnagar, S., and Valentino, R. J. (2013). Manganese-enhanced magnetic resonance imaging (MEMRI) reveals brain circuitry involved in responding to an acute novel stress in rats with a history of repeated social stress. Physiol. Behav. 122, 228–236. doi:10.1016/j.physbeh.2013.04.008
Bilbo, S. D., Yirmiya, R., Amat, J., Paul, E. D., Watkins, L. R., and Maier, S. F. (2008). Bacterial infection early in life protects against stressor-induced depressive-like symptoms in adult rats. Psychoneuroendocrinology 33 (3), 261–269. doi:10.1016/j.psyneuen.2007.11.008
Burow, A., Day, H. E., and Campeau, S. (2005). A detailed characterization of loud noise stress: intensity analysis of hypothalamo-pituitary-adrenocortical axis and brain activation. Brain Res. 1062 (1-2), 63–73. doi:10.1016/j.brainres.2005.09.031
Burstein, O., and Doron, R. (2018). The unpredictable chronic mild stress protocol for inducing anhedonia in mice. J. Vis. Exp. 140, 58184. doi:10.3791/58184
Calabrese, F., Molteni, R., Racagni, G., and Riva, M. A. (2009). Neuronal plasticity: a link between stress and mood disorders. Psychoneuroendocrinology 34 (Suppl. 1), S208–S216. doi:10.1016/j.psyneuen.2009.05.014
Campeau, S., Dolan, D., Akil, H., and Watson, S. J. (2002). c-fos mRNA induction in acute and chronic audiogenic stress: possible role of the orbitofrontal cortex in habituation. Stress 5 (2), 121–130. doi:10.1080/10253890290027895
Chatzittofis, A., Bostrom, A. D. E., Ciuculete, D. M., Oberg, K. G., Arver, S., Schioth, H. B., et al. (2021). HPA axis dysregulation is associated with differential methylation of CpG-sites in related genes. Sci. Rep. 11 (1), 20134. doi:10.1038/s41598-021-99714-x
Chubar, V., Vaessen, T., Noortgate, W. V. D., Lutin, E., Bosmans, G., Bekaert, B., et al. (2023). Mild daily stress, in interaction with NR3C1 DNA methylation levels, is linked to alterations in the HPA axis and ANS response to acute stress in early adolescents. Psychoneuroendocrinology 150, 106045. doi:10.1016/j.psyneuen.2023.106045
Commons, K. G., Cholanians, A. B., Babb, J. A., and Ehlinger, D. G. (2017). The rodent forced swim test measures stress-coping strategy, not depression-like behavior. ACS Chem. Neurosci. 8 (5), 955–960. doi:10.1021/acschemneuro.7b00042
Costello, H. M., Krilis, G., Grenier, C., Severs, D., Czopek, A., Ivy, J. R., et al. (2022). High salt intake activates the hypothalamic-pituitary-adrenal axis, amplifies the stress response, and alters tissue glucocorticoid exposure in mice. Cardiovasc Res. 119, 1740–1750. doi:10.1093/cvr/cvac160
Dulawa, S. C., and Hen, R. (2005). Recent advances in animal models of chronic antidepressant effects: the novelty-induced hypophagia test. Neurosci. Biobehav Rev. 29 (4-5), 771–783. doi:10.1016/j.neubiorev.2005.03.017
Escabi, C. D., Frye, M. D., Trevino, M., and Lobarinas, E. (2019). The rat animal model for noise-induced hearing loss. J. Acoust. Soc. Am. 146 (5), 3692. doi:10.1121/1.5132553
Flati, T., Gioiosa, S., Chillemi, G., Mele, A., Oliverio, A., Mannironi, C., et al. (2020). A gene expression atlas for different kinds of stress in the mouse brain. Sci. Data 7 (1), 437. doi:10.1038/s41597-020-00772-z
Franco, A. J., Chen, C., Scullen, T., Zsombok, A., Salahudeen, A. A., Di, S., et al. (2016). Sensitization of the hypothalamic-pituitary-adrenal Axis in a male rat chronic stress model. Endocrinology 157 (6), 2346–2355. doi:10.1210/en.2015-1641
Frisbee, J. C., Brooks, S. D., Stanley, S. C., and d'Audiffret, A. C. (2015). An unpredictable chronic mild stress protocol for instigating depressive symptoms, behavioral changes and negative health outcomes in rodents. J. Vis. Exp. 106, 53109. doi:10.3791/53109
Genty, J., Tetsi Nomigni, M., Anton, F., and Hanesch, U. (2018). The combination of postnatal maternal separation and social stress in young adulthood does not lead to enhanced inflammatory pain sensitivity and depression-related behavior in rats. PLoS One 13 (8), e0202599. doi:10.1371/journal.pone.0202599
Genty, J., Tetsi Nomigni, M., Anton, F., and Hanesch, U. (2018). Neonatal maternal separation leads to a dampening of inflammation-related mechanical and thermal hypersensitivity in juvenile rats. Neurosci. Lett. 674, 117–122. doi:10.1016/j.neulet.2018.03.044
Gjerstad, J. K., Lightman, S. L., and Spiga, F. (2018). Role of glucocorticoid negative feedback in the regulation of HPA axis pulsatility. Stress 21 (5), 403–416. doi:10.1080/10253890.2018.1470238
Henckens, M. J., Klumpers, F., Everaerd, D., Kooijman, S. C., van Wingen, G. A., and Fernandez, G. (2016). Interindividual differences in stress sensitivity: basal and stress-induced cortisol levels differentially predict neural vigilance processing under stress. Soc. Cogn. Affect Neurosci. 11 (4), 663–673. doi:10.1093/scan/nsv149
Herman, J. P., McKlveen, J. M., Ghosal, S., Kopp, B., Wulsin, A., Makinson, R., et al. (2016). Regulation of the hypothalamic-pituitary-adrenocortical stress response. Compr. Physiol. 6 (2), 603–621. doi:10.1002/cphy.c150015
Holmes, L., Shutman, E., Chinaka, C., Deepika, K., Pelaez, L., and Dabney, K. W. (2019). Aberrant epigenomic modulation of glucocorticoid receptor gene (NR3C1) in early life stress and major depressive disorder correlation: systematic review and quantitative evidence synthesis. Int. J. Environ. Res. Public Health 16 (21), 4280. doi:10.3390/ijerph16214280
Hyldelund, N. B., Dalgaard, V. L., Byrne, D. V., and Andersen, B. V. (2022). Why being “stressed” is “desserts” in reverse-the effect of acute psychosocial stress on food pleasure and food choice. Foods 11 (12), 1756. doi:10.3390/foods11121756
Ising, M., and Holsboer, F. (2006). Genetics of stress response and stress-related disorders. Dialogues Clin. Neurosci. 8 (4), 433–444. doi:10.31887/DCNS.2006.8.4/mising
Isingrini, E., Camus, V., Le Guisquet, A. M., Pingaud, M., Devers, S., and Belzung, C. (2010). Association between repeated unpredictable chronic mild stress (UCMS) procedures with a high fat diet: a model of fluoxetine resistance in mice. PLoS One 5 (4), e10404. doi:10.1371/journal.pone.0010404
Jung, S. H., Meckes, J. K., Schipma, M. J., Lim, P. H., Jenz, S. T., Przybyl, K., et al. (2020). Strain differences in responsiveness to repeated restraint stress affect remote contextual fear memory and blood transcriptomics. Neuroscience 444, 76–91. doi:10.1016/j.neuroscience.2020.07.052
Kahan, L. G. M. R. (2020). Environmental factors related to the obesity epidemic. Glob. Impact Epidemiol. R. Mehrzad, 117–139. doi:10.1016/B978-0-12-818839-2.00010-7
Keele, G. R., Prokop, J. W., He, H., Holl, K., Littrell, J., Deal, A., et al. (2018). Genetic fine-mapping and identification of candidate genes and variants for adiposity traits in outbred rats. Obes. (Silver Spring) 26 (1), 213–222. doi:10.1002/oby.22075
Kistenmacher, A., Goetsch, J., Ullmann, D., Wardzinski, E. K., Melchert, U. H., Jauch-Chara, K., et al. (2018). Psychosocial stress promotes food intake and enhances the neuroenergetic level in men. Stress 21 (6), 538–547. doi:10.1080/10253890.2018.1485645
Kogler, L., Muller, V. I., Chang, A., Eickhoff, S. B., Fox, P. T., Gur, R. C., et al. (2015). Psychosocial versus physiological stress - meta-analyses on deactivations and activations of the neural correlates of stress reactions. Neuroimage 119, 235–251. doi:10.1016/j.neuroimage.2015.06.059
Lahdepuro, A., Savolainen, K., Lahti-Pulkkinen, M., Eriksson, J. G., Lahti, J., Tuovinen, S., et al. (2019). The impact of early life stress on anxiety symptoms in late adulthood. Sci. Rep. 9 (1), 4395. doi:10.1038/s41598-019-40698-0
Lee, A., Cardel, M., and Donahoo, W. T. (2000). “Social and environmental factors influencing obesity,” in Endotext K. R. Feingold, A. B. Anawalt, M. R. Blackman, A. Boyce, G. Chrousos, and E. Corpas, (South Dartmouth (MA): MDText.com, Inc.).
Lewis, C. R., Breitenstein, R. S., Henderson, A., Sowards, H. A., Piras, I. S., Huentelman, M. J., et al. (2021). Harsh parenting predicts novel HPA receptor gene methylation and NR3C1 methylation predicts cortisol daily slope in middle childhood. Cell Mol. Neurobiol. 41 (4), 783–793. doi:10.1007/s10571-020-00885-4
Lievens, S., Van der Heyden, J., Masschaele, D., De Ceuninck, L., Petta, I., Gupta, S., et al. (2016). Proteome-scale binary interactomics in human cells. Mol. Cell Proteomics 15 (12), 3624–3639. doi:10.1074/mcp.M116.061994
Malkesman, O., Braw, Y., Maayan, R., Weizman, A., Overstreet, D. H., Shabat-Simon, M., et al. (2006). Two different putative genetic animal models of childhood depression. Biol. Psychiatry 59 (1), 17–23. doi:10.1016/j.biopsych.2005.05.039
Maniam, J., and Morris, M. J. (2010). Palatable cafeteria diet ameliorates anxiety and depression-like symptoms following an adverse early environment. Psychoneuroendocrinology 35 (5), 717–728. doi:10.1016/j.psyneuen.2009.10.013
Masini, C. V., Day, H. E., and Campeau, S. (2008). Long-term habituation to repeated loud noise is impaired by relatively short interstressor intervals in rats. Behav. Neurosci. 122 (1), 210–223. doi:10.1037/0735-7044.122.1.210
McAuley, J. D., Stewart, A. L., Webber, E. S., Cromwell, H. C., Servatius, R. J., and Pang, K. C. (2009). Wistar-Kyoto rats as an animal model of anxiety vulnerability: support for a hypervigilance hypothesis. Behav. Brain Res. 204 (1), 162–168. doi:10.1016/j.bbr.2009.05.036
Meaney, M. J., Mitchell, J. B., Aitken, D. H., Bhatnagar, S., Bodnoff, S. R., Iny, L. J., et al. (1991). The effects of neonatal handling on the development of the adrenocortical response to stress: implications for neuropathology and cognitive deficits in later life. Psychoneuroendocrinology 16 (1-3), 85–103. doi:10.1016/0306-4530(91)90072-2
Mileva, G. R., Rooke, J., Ismail, N., and Bielajew, C. (2017). Corticosterone and immune cytokine characterization following environmental manipulation in female WKY rats. Behav. Brain Res. 316, 197–204. doi:10.1016/j.bbr.2016.09.004
Molendijk, M. L., and de Kloet, E. R. (2019). Coping with the forced swim stressor: current state-of-the-art. Behav. Brain Res. 364, 1–10. doi:10.1016/j.bbr.2019.02.005
Monteiro, S., Roque, S., de Sa-Calcada, D., Sousa, N., Correia-Neves, M., and Cerqueira, J. J. (2015). An efficient chronic unpredictable stress protocol to induce stress-related responses in C57BL/6 mice. Front. Psychiatry 6, 6. doi:10.3389/fpsyt.2015.00006
Morera, L. P., Gallea, J. I., Trogolo, M. A., Guido, M. E., and Medrano, L. A. (2020). From work well-being to burnout: a hypothetical phase model. Front. Neurosci. 14, 360. doi:10.3389/fnins.2020.00360
Muir, R. Q., Klocke, B. J., Jennings, M. S., Molina, P. A., Hsu, J. S., Kellum, C. E., et al. (2023). Early life stress in mice leads to impaired colonic corticosterone production and prolonged inflammation following induction of colitis. Inflamm. Bowel Dis. 29, 960–972. doi:10.1093/ibd/izac280
Nishimura, K., Makino, S., Tanaka, Y., Kaneda, T., and Hashimoto, K. (2004). Altered expression of p53 mRNA in the brain and pituitary during repeated immobilization stress: negative correlation with glucocorticoid receptor mRNA levels. J. Neuroendocrinol. 16 (1), 84–91. doi:10.1111/j.1365-2826.2004.01131.x
Noguchi, T., Makino, S., Matsumoto, R., Nakayama, S., Nishiyama, M., Terada, Y., et al. (2010). Regulation of glucocorticoid receptor transcription and nuclear translocation during single and repeated immobilization stress. Endocrinology 151 (9), 4344–4355. doi:10.1210/en.2010-0266
Pardon, M. C., Ma, S., and Morilak, D. A. (2003). Chronic cold stress sensitizes brain noradrenergic reactivity and noradrenergic facilitation of the HPA stress response in Wistar Kyoto rats. Brain Res. 971 (1), 55–65. doi:10.1016/s0006-8993(03)02355-2
Patterson, Z. R., and Abizaid, A. (2013). Stress induced obesity: lessons from rodent models of stress. Front. Neurosci. 7, 130. doi:10.3389/fnins.2013.00130
Peckett, A. J., Wright, D. C., and Riddell, M. C. (2011). The effects of glucocorticoids on adipose tissue lipid metabolism. Metabolism 60 (11), 1500–1510. doi:10.1016/j.metabol.2011.06.012
Pulit, S. L., Stoneman, C., Morris, A. P., Wood, A. R., Glastonbury, C. A., Tyrrell, J., et al. (2019). Meta-analysis of genome-wide association studies for body fat distribution in 694 649 individuals of European ancestry. Hum. Mol. Genet. 28 (1), 166–174. doi:10.1093/hmg/ddy327
Redei, E. E., Udell, M. E., Solberg-Woods, L. C., and Chen, H. (2022). The Wistar Kyoto rat: a model of depression traits. Curr. Neuropharmacol. doi:10.2174/1570159X21666221129120902
Reynolds, R. P., Li, Y., Garner, A., and Norton, J. N. (2018). Vibration in mice: a review of comparative effects and use in translational research. Anim. Model Exp. Med. 1 (2), 116–124. doi:10.1002/ame2.12024
Rohde, K., Keller, M., la Cour Poulsen, L., Bluher, M., Kovacs, P., and Bottcher, Y. (2019). Genetics and epigenetics in obesity. Metabolism 92, 37–50. doi:10.1016/j.metabol.2018.10.007
Samson, J., Sheeladevi, R., Ravindran, R., and Senthilvelan, M. (2007). Stress response in rat brain after different durations of noise exposure. Neurosci. Res. 57 (1), 143–147. doi:10.1016/j.neures.2006.09.019
Scott, K. A., Melhorn, S. J., and Sakai, R. R. (2012). Effects of chronic social stress on obesity. Curr. Obes. Rep. 1 (1), 16–25. doi:10.1007/s13679-011-0006-3
Seibenhener, M. L., and Wooten, M. C. (2015). Use of the Open Field Maze to measure locomotor and anxiety-like behavior in mice. J. Vis. Exp. 96, e52434. doi:10.3791/52434
Sequeira-Cordero, A., Salas-Bastos, A., Fornaguera, J., and Brenes, J. C. (2019). Behavioural characterisation of chronic unpredictable stress based on ethologically relevant paradigms in rats. Sci. Rep. 9 (1), 17403. doi:10.1038/s41598-019-53624-1
Sheppard, K. E., Roberts, J. L., and Blum, M. (1990). Differential regulation of type II corticosteroid receptor messenger ribonucleic acid expression in the rat anterior pituitary and hippocampus. Endocrinology 127 (1), 431–439. doi:10.1210/endo-127-1-431
Sheppard, K. E., Roberts, J. L., and Blum, M. (1991). Adrenocorticotropin-releasing factor down-regulates glucocorticoid receptor expression in mouse corticotrope tumor cells via an adenylate cyclase-dependent mechanism. Endocrinology 129 (2), 663–670. doi:10.1210/endo-129-2-663
Shukla, P. K., Meena, A. S., Pierre, J. F., and Rao, R. (2022). Central role of intestinal epithelial glucocorticoid receptor in alcohol- and corticosterone-induced gut permeability and systemic response. FASEB J. 36 (1), e22061. doi:10.1096/fj.202101424R
Singh, A., Petrides, J. S., Gold, P. W., Chrousos, G. P., and Deuster, P. A. (1999). Differential hypothalamic-pituitary-adrenal axis reactivity to psychological and physical stress. J. Clin. Endocrinol. Metab. 84 (6), 1944–1948. doi:10.1210/jcem.84.6.5746
Solberg, L. C., Baum, A. E., Ahmadiyeh, N., Shimomura, K., Li, R., Turek, F. W., et al. (2004). Sex- and lineage-specific inheritance of depression-like behavior in the rat. Mamm. Genome 15 (8), 648–662. doi:10.1007/s00335-004-2326-z
Solberg, L. C., Baum, A. E., Ahmadiyeh, N., Shimomura, K., Li, R., Turek, F. W., et al. (2006). Genetic analysis of the stress-responsive adrenocortical axis. Physiol. Genomics 27 (3), 362–369. doi:10.1152/physiolgenomics.00052.2006
Stierman, B. A., Carroll, M. D., Chen, T.-C., Davy, O., Fink, S., Fryar, C. D., et al. (2021). National health and nutrition examination survey 2017–march 2020 prepandemic data files development of files and prevalence estimates for selected health outcomes. United States: National Center for Health Statistics.
Szalanczy, A. M., Giorgio, G., Goff, E., Seshie, O., Grzybowski, M., Klotz, J., et al. (2023). Changes in environmental stress over COVID-19 pandemic likely contributed to failure to replicate adiposity phenotype associated with Krtcap3. Physiol. Genomics 55 (10), 452–467. doi:10.1152/physiolgenomics.00019.2023
Szalanczy, A. M., Goff, E., Seshie, O., Deal, A., Grzybowski, M., Klotz, J., et al. (2022). Keratinocyte-associated protein 3 plays a role in body weight and adiposity with differential effects in males and females. Front. Genet. 13, 942574. doi:10.3389/fgene.2022.942574
Terashvili, M. N., Kozak, K. N., Gebremedhin, D., Allen, L. A., Gifford, A. L., Allen, K. P., et al. (2020). Effect of nearby construction activity on endothelial function, sensitivity to nitric oxide, and potassium channel activity in the middle cerebral arteries of rats. J. Am. Assoc. Lab. Anim. Sci. 59 (4), 411–422. doi:10.30802/AALAS-JAALAS-19-000116
Torres, S. J., and Nowson, C. A. (2007). Relationship between stress, eating behavior, and obesity. Nutrition 23 (11-12), 887–894. doi:10.1016/j.nut.2007.08.008
Tran, I., and Gellner, A. K. (2023). Long-term effects of chronic stress models in adult mice. J. Neural Transm. (Vienna) 130, 1133–1151. doi:10.1007/s00702-023-02598-6
van den Bos, R., Harteveld, M., and Stoop, H. (2009). Stress and decision-making in humans: performance is related to cortisol reactivity, albeit differently in men and women. Psychoneuroendocrinology 34 (10), 1449–1458. doi:10.1016/j.psyneuen.2009.04.016
van der Knaap, L. J., Oldehinkel, A. J., Verhulst, F. C., van Oort, F. V., and Riese, H. (2015). Glucocorticoid receptor gene methylation and HPA-axis regulation in adolescents. The TRAILS study. Psychoneuroendocrinology 58, 46–50. doi:10.1016/j.psyneuen.2015.04.012
van der Valk, E. S., Savas, M., and van Rossum, E. F. C. (2018). Stress and obesity: are there more susceptible individuals? Curr. Obes. Rep. 7 (2), 193–203. doi:10.1007/s13679-018-0306-y
Wang, Z., Cheng, Y., Li, Y., Han, J., Yuan, Z., Li, Q., et al. (2022). The relationship between obesity and depression is partly dependent on metabolic health status: a nationwide inpatient sample database study. Front. Endocrinol. (Lausanne) 13, 880230. doi:10.3389/fendo.2022.880230
Wiley, J. W., Higgins, G. A., and Athey, B. D. (2016). Stress and glucocorticoid receptor transcriptional programming in time and space: implications for the brain-gut axis. Neurogastroenterol. Motil. 28 (1), 12–25. doi:10.1111/nmo.12706
Williams, G. R., Franklyn, J. A., and Sheppard, M. C. (1991). Thyroid hormone and glucocorticoid regulation of receptor and target gene mRNAs in pituitary GH3 cells. Mol. Cell Endocrinol. 80 (1-3), 127–138. doi:10.1016/0303-7207(91)90149-m
Willner, P. (2017). The chronic mild stress (CMS) model of depression: history, evaluation and usage. Neurobiol. Stress 6, 78–93. doi:10.1016/j.ynstr.2016.08.002
Willner, P. (2017). Reliability of the chronic mild stress model of depression: a user survey. Neurobiol. Stress 6, 68–77. doi:10.1016/j.ynstr.2016.08.001
Xiao, Y., Liu, D., Cline, M. A., and Gilbert, E. R. (2020). Chronic stress, epigenetics, and adipose tissue metabolism in the obese state. Nutr. Metab. (Lond) 17, 88. doi:10.1186/s12986-020-00513-4
Xu, Z., Zhang, Y., Hou, B., Gao, Y., Wu, Y., and Zhang, C. (2011). Chronic corticosterone administration from adolescence through early adulthood attenuates depression-like behaviors in mice. J. Affect Disord. 131 (1-3), 128–135. doi:10.1016/j.jad.2010.11.005
Yengo, L., Sidorenko, J., Kemper, K. E., Zheng, Z., Wood, A. R., Weedon, M. N., et al. (2018). Meta-analysis of genome-wide association studies for height and body mass index in ∼700000 individuals of European ancestry. Hum. Mol. Genet. 27 (20), 3641–3649. doi:10.1093/hmg/ddy271
Zheng, G., Victor Fon, G., Meixner, W., Creekmore, A., Zong, Y., Colacino, J., et al. (2017). Chronic stress and intestinal barrier dysfunction: glucocorticoid receptor and transcription repressor HES1 regulate tight junction protein Claudin-1 promoter. Sci. Rep. 7 (1), 4502. doi:10.1038/s41598-017-04755-w
Keywords: obesity, stress, HPA axis, genetics, behavior, anxiety, Krtcap3
Citation: Szalanczy AM, Fitzpatrick M, Beeson A, Bui T, Dyson C, Eller S, Landry J, Scott C, Grzybowski M, Klotz J, Geurts AM, Weiner JL, Redei EE and Solberg Woods LC (2024) Chronic stress from adolescence to adulthood increases adiposity and anxiety in rats with decreased expression of Krtcap3. Front. Genet. 14:1247232. doi: 10.3389/fgene.2023.1247232
Received: 25 June 2023; Accepted: 21 December 2023;
Published: 23 January 2024.
Edited by:
Daiva Nielsen, McGill University, CanadaReviewed by:
Andre Portella, McGill University, CanadaRyan W. Logan, University of Massachusetts Medical School, United States
Copyright © 2024 Szalanczy, Fitzpatrick, Beeson, Bui, Dyson, Eller, Landry, Scott, Grzybowski, Klotz, Geurts, Weiner, Redei and Solberg Woods. This is an open-access article distributed under the terms of the Creative Commons Attribution License (CC BY). The use, distribution or reproduction in other forums is permitted, provided the original author(s) and the copyright owner(s) are credited and that the original publication in this journal is cited, in accordance with accepted academic practice. No use, distribution or reproduction is permitted which does not comply with these terms.
*Correspondence: Leah C. Solberg Woods, lsolberg@wakehealth.edu