- 1Department of Cardiovascular and Metabolic Sciences, Lerner Research Institute, Cleveland Clinic, Cleveland, OH, United States
- 2Department of Genetics and Genome Sciences, Case Western Reserve University, Cleveland, OH, United States
- 3Center for Molecular Medicine and Genetics, Wayne State University, Detroit, MI, United States
- 4Robert M. Berne Cardiovascular Research Center, University of Virginia, Charlottesville, VA, United States
Objectives: We previously found that the pluripotency factor OCT4 is reactivated in smooth muscle cells (SMC) in human and mouse atherosclerotic plaques and plays an atheroprotective role. Loss of OCT4 in SMC in vitro was associated with decreases in SMC migration. However, molecular mechanisms responsible for atheroprotective SMC-OCT4-dependent effects remain unknown.
Methods: Since studies in embryonic stem cells demonstrated that OCT4 regulates long non-coding RNAs (lncRNAs) and microRNAs (miRNAs), making them candidates for OCT4 effect mediators, we applied an in vitro approach to investigate the interactions between OCT4-regulated lncRNAs, mRNAs, and miRNAs in SMC. We used OCT4 deficient mouse aortic SMC (MASMC) treated with the pro-atherogenic oxidized phospholipid POVPC, which, as we previously demonstrated, suppresses SMC contractile markers and induces SMC migration. Differential expression of lncRNAs, mRNAs, and miRNAs was obtained by lncRNA/mRNA expression array and small-RNA microarray. Long non-coding RNA to mRNA associations were predicted based on their genomic proximity and association with vascular diseases. Given a recently discovered crosstalk between miRNA and lncRNA, we also investigated the association of miRNAs with upregulated/downregulated lncRNA-mRNA pairs.
Results: POVPC treatment in SMC resulted in upregulating genes related to the axon guidance and focal adhesion pathways. Knockdown of Oct4 resulted in differential regulation of pathways associated with phagocytosis. Importantly, these results were consistent with our data showing that OCT4 deficiency attenuated POVPC-induced SMC migration and led to increased phagocytosis. Next, we identified several up- or downregulated lncRNA associated with upregulation of the specific mRNA unique for the OCT4 deficient SMC, including upregulation of ENSMUST00000140952-Hoxb5/6 and ENSMUST00000155531-Zfp652 along with downregulation of ENSMUST00000173605-Parp9 and, ENSMUST00000137236-Zmym1. Finally, we found that many of the downregulated miRNAs were associated with cell migration, including miR-196a-1 and miR-10a, targets of upregulated ENSMUST00000140952, and miR-155 and miR-122, targets of upregulated ENSMUST00000155531. Oppositely, the upregulated miRNAs were anti-migratory and pro-phagocytic, such as miR-10a/b and miR-15a/b, targets of downregulated ENSMUST00000173605, and miR-146a/b and miR-15b targets of ENSMUST00000137236.
Conclusion: Our integrative analyses of the lncRNA-miRNA-mRNA interactions in SMC indicated novel potential OCT4-dependent mechanisms that may play a role in SMC phenotypic transitions.
Introduction
Cardiovascular diseases (CVD) are the leading cause of death, with more than 17.3 million fatalities per year around the globe (McClellan et al., 2019). Atherosclerosis is a major root cause of CVD and accounts for a large proportion of these deaths. This is an inflammatory disease of the arterial wall, resulting in the formation of complex atherosclerotic plaques and leading to multiple thrombotic events, including myocardial infarction and stroke (Libby, 2021). Vascular cells, such as smooth muscle cells (SMC), endothelial cells (EC), and macrophages (MФ), contribute to atherosclerosis plaque development.
There is growing evidence that SMC phenotypic transitions have a critical role in the pathogenesis of cardiovascular diseases. Importantly, recent SMC-lineage tracing and single cell (sc)RNAseq studies revealed extensive plasticity of SMC in atherosclerosis (Dobnikar et al., 2018; Wirka et al., 2019; Alencar et al., 2020; Pan et al., 2020). These include the transition of SMC from differentiated cells expressing high levels of contractile SMC-lineage markers (e.g., MYH11) to several phenotypically modulated states, including inflammatory, МФ-like (Shankman et al., 2015), osteogenic-like (Alencar et al., 2020), or myofibroblast-like phenotypes (Wirka et al., 2019). However, the molecular mechanisms regulating these transitions are not fully understood.
Long non-coding RNAs (lncRNA) have been identified and characterized as a sub-class of non-coding RNAs with lengths longer than 200 nucleotides (nts) playing critical regulatory roles in many human diseases, including atherosclerosis (Li et al., 2016; Chen X. et al., 2017). MicroRNAs (miRNAs) are short RNAs with lengths 21–23 nts that bind to mRNAs and post-transcriptionally regulate gene expression. Another important class of non-coding RNA are circular RNAs (circRNAs), single-stranded and covalently closed RNA molecules, which act as transcriptional regulators and have been found in many different species, including mammals (Zhou et al., 2020). Due to their unique structure, circRNA are more stable than other non-coding RNAs and are found in circulation and urine, making them potential good targets for therapeutics and diagnostics (Zhou et al., 2020). Non-coding RNAs account for more than 98% of the human genome (Zhou et al., 2020) and have significant roles in regulating many cellular functions in normal conditions (e.g., during development or normal tissue homeostasis) and pathological conditions (Xue et al., 2017; Salviano-Silva et al., 2018). A growing number of reports describe the role of miRNA and lncRNA in SMC dysfunction in pathological conditions, including cancer (Poniewierska-Baran et al., 2022; Mazziotta et al., 2023a) and atherosclerosis (Kumar et al., 2019; Zhou et al., 2021). However, these mechanisms have not been fully explored.
Long non-coding RNAs regulate gene expression by multiple mechanisms, including serving as “decoy”, “guide”, or “scaffold” to the critical transcriptional factors of other important regulatory genes in a location-dependent manner (Wilusz et al., 2009). It is also known that lncRNAs are associated with chromatin-modifying complexes and are transcribed from enhancers involved in the phase separation of nuclear condensates and domains. These findings suggest a close relationship between lncRNA expression and the spatial regulation of gene expression during development (Mattick et al., 2023). Most of the lncRNAs are localized in the nuclei, and their nucleus-specific lncRNA functions (i.e., chromosome scaffolding, chromatin remodeling, alternative splicing, epigenetic control of transcription, etc.) have been reported (Derrien et al., 2012; Kugel and Goodrich, 2012; Tripathi et al., 2013). However, lncRNAs are also localized in the cytoplasm, and the functions of these cytoplasmic lncRNAs remain poorly understood. These lncRNAs are transcribed from nuclear DNA or expressed locally in the cytoplasm (e.g., mitochondrial DNA-encoded lncRNAs) (Mercer et al., 2011) and are reported to be involved in maintaining cellular structure and functions (Rashid et al., 2016), mRNA translation and stability, availability of cytoplasmic factors, and scaffolding of proteins (reviewed in Noh et al.) (Noh et al., 2018).
One of the regulatory functions of lncRNAs is their ability to act as competitive endogenous RNA (ceRNA) by working as a miRNA “sponge” or as precursor and encoding miRNAs, in turn regulating the effects of miRNA by competing for the miRNA binding to mRNA (McMullen and Drew, 2016). Similar to lncRNAs, circRNAs also exert biological functions by acting as transcriptional regulators, miRNA sponges, and protein templates (Zhou et al., 2020). Due to the complex and complicated nature of interactions between lncRNA, miRNA, and mRNA, multiple groups started to employ integrative analysis between these molecules in different cells and different in vitro and in vivo conditions (Cabili et al., 2011; Du et al., 2016; Qian et al., 2018; Wang et al., 2019; Zou et al., 2019; He et al., 2022; Liu et al., 2023). In particular relevance to SMC, using integrative analysis of altered lncRNA, miRNA, and mRNA expression, Chinnappan et al. (Chinnappan et al., 2019) demonstrated that lncRNA/miRNA/mRNA interactions play a vital role in human pulmonary artery SMC treated with cocaine and HIV-Tat protein. However, similar interactions in the phenotypic transitions of vascular SMC are still unknown.
We recently found that the embryonic stem cell/induced pluripotent stem cell (iPSC) factor OCT4, which was believed to be silenced in somatic cells, plays an atheroprotective role in SMC, in that knockout of Oct4 in SMC led to marked increases in lesion size and multiple indices of plaque instability in Apoe−/− mice (Cherepanova et al., 2016). It has also been shown that SMC-specific knockout of Oct4 protects SMC from hyperproliferation after acute vascular injury (Shin et al., 2023). Moreover, we found that knockout of Oct4 in cultured SMC resulted in decreased cell migration and increased cell proliferation. Mechanistically, we demonstrated that OCT4 directly regulates SMC contractile genes, including ACTA2 and TAGLN. Bulk RNAseq analyses on cultured aortic Oct4 wild-type and knockout SMC demonstrated significant changes in gene expression profiles (Shin et al., 2023), indicating a broad effect of OCT4 in SMC. However, it is still unknown if lncRNA and/or miRNA contribute to the OCT4-dependent effects in SMC.
Since it has been reported that OCT4 transcriptionally regulates miRNA and lncRNA in embryonic (Shi and Jin, 2010) and cancer cells (Zhang et al., 2020), we hypothesized that similar mechanisms may be activated in SMC. This study aims to identify novel potential OCT4-dependent interactions among lncRNAs, mRNAs, and miRNAs that could be involved in vascular SMC phenotypic changes. Herein, we adopted an integrative analysis approach similar to Chinnappan et al (Chinnappan et al., 2019) and others (Cabili et al., 2011; Du et al., 2016; Qian et al., 2018; Wang et al., 2019; Zou et al., 2019; He et al., 2022; Liu et al., 2023) to construct an interactive network of altered lncRNA, miRNA, and mRNA expression in OCT4 deficient mouse aortic SMC (MASMCs) treated with the pro-atherogenic oxidized phospholipid POVPC (1-palmitoyl-2-(5-oxovaleroyl)-sn-glycero phosphatidylcholine) that we previously found induces SMC phenotypic switching, including suppression of SMC contractile markers and mediation of SMC proliferation and migration (Pidkovka et al., 2007; Cherepanova et al., 2009). Our studies identified new interactions between OCT4, lncRNA, and miRNA that advance our understanding not only of OCT4-dependent effects but also general causes of SMC phenotypic transitions associated with atherosclerosis.
Materials and methods
Cell culture and RNA isolation
Primary mouse aortic smooth muscle cells (MASMC) were isolated and grown as previously described (Cherepanova et al., 2016). Red blood cells (RBC) were isolated from the blood of the young (10 weeks of age) C57B6 mice as previously described (Hanson et al., 2008) and immediately used for phagocytosis experiments. The animal study were approved by the University of Virginia (protocol 2500) and Lerner Research Institute Animal Care and Use Committees (protocol 2433). The study was conducted in accordance with the local legislation and institutional requirements. For experiments, cells at the 70% confluency were transfected with blocking siOct4 or non-target siRNA (siNT) (Dharmacon) for 48 h in serum-free transfection media (Dharmacon), followed by the treatment with 10 μg/mL POVPC (Cayman Chem, #10031) or vehicle (DMSO) for 24 h based on our previous findings (Pidkovka et al., 2007). At the end of the experiment, cells were harvested with the Trizol reagent (Invitrogen), which preserves most of the small RNAs, including lncRNAs and miRNAs, and the total RNA was isolated according to the manufacturer’s instructions.
Quantitative real time (qRT)-PCR
The cDNA was prepared using an iScript gDNA Clear cDNA Synthesis Kit (BioRad). Quantitative RT-PCR was performed using RT2 SYBR Green ROX qPCR Master Mix kit (Cat#330522, Qiagen) for Oct4 exon 1 primers or SensiFAST™ SYBR NO-ROX Mix (Bioline) for 18s RNA and lncRNAs. The qRT-PCR primers for Oct4 exon1 and 18s RNA were previously described (Cherepanova et al., 2016). For lncRNAs, we used primers as ENSMUST00000140952 (F: CACCCCAGCCCGGTAAAC R: CATGGGCGATCCACATGAA) and ENSMUST00000173605 (F: TTCCTTCTGCGTCAGTATCATCTT R: CCAAGCTGCTTTGCTCAAATT).
Mouse lncRNA/mRNA and small RNA expression arrays
Long non-coding RNA/mRNA and small RNA microarrays were performed by Arraystar Inc. on the same total RNA samples isolated from MASMC transfected with either siRNA to Oct4 or NT pooled siRNA (Dharmacon) and treated with either DMSO or POVPC (10 μg/mL) for 24 h.
Long non-coding RNA/mRNA
After sample quality controls using Nanodrop ND 1000 and determining RNA integrity using denaturing gel electrophoresis, each sample was amplified and transcribed into fluorescent cRNA along the entire length of the transcripts without 3′bias utilizing a random priming method (Array Star Flash RNA Labeling Kit, Arraystar Inc.). The labeled cRNAs were hybridized onto the mouse LncRNA Array v4.0 (8 × 60 K, Arraystar). These microarrays are highly sensitive and accurate even for low abundance lncRNAs (the quantitative efficiency is as low as 1 transcript per cell). These oligo-based probes hybridize the target RNA at high affinity, independent of other abundant RNAs. Also, the design of the transcript-specific array probe is based on well-established transcript models for every lncRNA isoform, ensuring clear and precise isoform detection and quantification.
Small RNA array
For each sample, 100 ng total RNA was firstly dephosphorylated to form the 3-OH end. The 3-OH-ended RNA was then denatured by DMSO and enzymatically labeled with Cy3. This ligation of small RNAs with Cy3 dye directly at their 3′-OH ends, completely avoids RNA pretreatments to remove internal RNA modifications, abortive reverse transcription due to the modifications and RNA fold hindrance, and skewed PCR amplification steps (Yuan et al., 2022). All these help to preserve the fidelity of native small RNA levels and achieve the unbiased high quantification accuracy. The labeled RNA was hybridized onto Arraystar Mouse small RNA Microarray (8 × 15K, Arraystar).
The Arrays were scanned by the Agilent Scanner G2505C. Agilent Feature Extraction software (version 11.0.1.1) was used to analyze the acquired array images. Quantile normalization and subsequent data processing were performed using the GeneSpring GX v12.1 software package (Agilent Technologies). Arraystar Small RNA Array uses high-affinity probe hybridization to achieve very high sensitivity even for small RNAs at low abundance. The smart probe design incorporates a 5′-hairpin structure and normalized sequence targeting region to specifically distinguish small RNAs with only 1–2 nucleotide differences.
Differential expression analyses
Differential expression analyses were performed after quality control analyses
Long non-coding RNA/mRNA
Differentially expressed lncRNAs and mRNAs with statistical significance were identified through Fold Change filtering between two samples. Differentially expressed LncRNAs and mRNAs with statistical significance between siNT DMSO vs. siNT POVPC (effect of POVPC in wild type SMC), siOCT4-DMSO vs. siNT-DMSO (effect of OCT4 inactivation), and siOCT4 POVPC vs. siOCT4 DMSO (effect of POVPC in OCT4 deficient SMC) groups were identified through Volcano filtering between two groups. Finally, hierarchical clustering was performed to show the distinguishable lncRNAs’ and mRNAs’ expression patterns among samples. The Ingenuity Pathway Analysis (IPA; QIAGEN Inc.) was used to analyze the associated pathways. The statistical significance of a gene association with biological function or pathway was determined using the right-tailed Fisher’s Exact Test.
Small RNAs
Differentially expressed small RNAs between two comparison groups were identified by fold change (FC) of ≥1.5 and statistical significance (p-value) of ≤0.05 thresholds. Hierarchical clustering heatmaps, scatter plots, and volcano plots were plotted to display small RNAs expression patterns among samples by R software. Pathway analysis (KEGG; Kyoto Encyclopedia of Genes and Genomes) and GO analysis (Gene Ontology) (Mazziotta et al., 2023b) were applied to determine these differentially expressed mRNAs’ roles in the biological pathways or GO terms.
Integrative analysis of dysregulated non-coding RNAs and mRNAs
The integrative analysis was adopted from Chinnappan et al. (reference 29) and modified for our experimental design. For analysis, mRNA, miRNA, and lncRNA that were differentially expressed with >1.5-fold up- or downregulated between treatment groups (NT DMSO, NT POVPC, siOCT4 DMSO, siOCT4 POVPC) and p-values less than 0.05 were used. The IPA software was used to investigate the relationship between differentially expressed miRNAs and mRNAs. First—The initial analysis was performed by only taking experimentally validated interactions of miRNA/mRNA, and later, the mRNA expression data were included to take into account the interactions of highly predictive and experimentally validated miRNA/mRNA associations. For the experimentally validated miRNA/mRNA interactions, we utilized the integrated information from TarBase and miRecords (IPA), and for predicted miRNA/mRNA interactions, we used information from TargetScan (IPA). Second—The regulatory relationships between differentially expressed miRNA and lncRNA were extracted from LncBase v2.0. using DIANA Tools (Paraskevopoulou et al., 2016). Only interactions that were experimentally validated were taken into account. Third—Pathway enrichment analysis was performed using IPA software and DAVID (Database for Annotation, Visualization, and Integrated Discovery) software (Huang da et al., 2009). Long non-coding RNAs, which were differentially expressed, were annotated for diseases and biological functions using the LncRNADisease database (Wang et al., 2016).
LncRNA conservation analysis
To assess the potential conservation of selected lncRNAs across species, phyloP analysis (PHAST (cshl.edu) was employed to quantify evolutionary conservation by comparing the sequence of interest to placental mammals and vertebrates, in conjunction with the multiz alignment function from the UCSC genome browser (Kent et al., 2002). Blastn (Basic Local Alignment Search Tool for nucleotides) analysis was conducted to determine human orthologs by querying the lncRNA sequences against the Homo Sapiens genomic and transcript database. Subsequently, the identified lncRNA sequences and their putative orthologs were subjected to alignment using SnapGene software for validation.
SMC migration assay
For experiments, cells at the 70% confluency were transfected with blocking siOct4 or non-target siRNA (NT siRNA) for 48 h in serum-free media, followed by the Boyden chamber transmigration assay in response to different concentrations of POVCP, as previously described (Cherepanova et al., 2016).
Phagocytosis assay
Phagocytosis assay was performed as previously described in Kolb et al. (Kolb et al., 2007). Briefly, Oct4 wild type and knockout SMC(34) were plated in 24 well plates. Cells at 70% confluency were growth arrested with serum-free media with supplements (DMEM/F12 [Gibco], 100 U/mL penicillin/streptomycin [Gibco], 1.6 mM/L L-glutamine [Gibco], L-ascorbic acid 0.2 mM [Sigma], transferrin 5 μg/mL [Sigma], insulin 2.8 μg/mL [Sigma], Na-selenate 6.25 ng/mL [Sigma]) for 24 h and then treated with 0 or 40 μg/mL of oxidized low-density lipoprotein particles (oxLDL) (Biomedical Thechnologies) for 3 days. At day 4, mouse RBSs were plated (∼1 × 105 cells/mL) on the top of SMC for 24 h. Media was aspirated, and cells were washed with PBS. The membrane-bound RBCs were lysed by hypotonic shock (1 min in distilled sterile H2O on ice). SMCs containing phagocytosed RBCs were lysed by 1% Triton-X100 in PBS. Hemoglobin was then separately analyzed in hypotonic (H2O)- and Triton-lysate fractions using 2-7-diaminofluorene reagent (Sigma).
Statistical analysis
For the functional in vitro experiments, the normality of the data was checked using Kolmogorov-Smirnov test. One-way analysis of variance (ANOVA) was used to compare two groups of continuous variables with normal distribution, and two-way ANOVA, followed by post hoc tests, was used to compare multiple group comparisons as previously described (Shin et al., 2023). p < 0.05 was considered significant. GraphPad software 9.4.0 was used for all statistical analyses.
Results
Differential expression of lncRNA in OCT4-deficient MASMC
To investigate the effects of OCT4, we chose to use an siRNA knockdown cell culture model to avoid any long-term effects of OCT4 knockout during cell culture. Mouse aortic SMC were transfected with blocking siOct4 or control non-target siRNA (siNT) followed by treatment with the oxidized phospholipid POVPC. The knockdown efficiency was confirmed by using qRT-PCR (Figure 1A). We recently demonstrated that transient siRNA knockdown and genetic knockout have similar effects on SMC proliferation and expression of SMC contractile genes (Shin et al., 2023). We also found that similarly to the genetic knockout, inhibiting Oct4 by blocking siRNA resulted in decreases in SMC trans-migration in response to POVPC (Figure 1B), indicating that siOct4 knockdown is a good cell culture model to study immediate OCT4-dependent mechanisms in SMC.
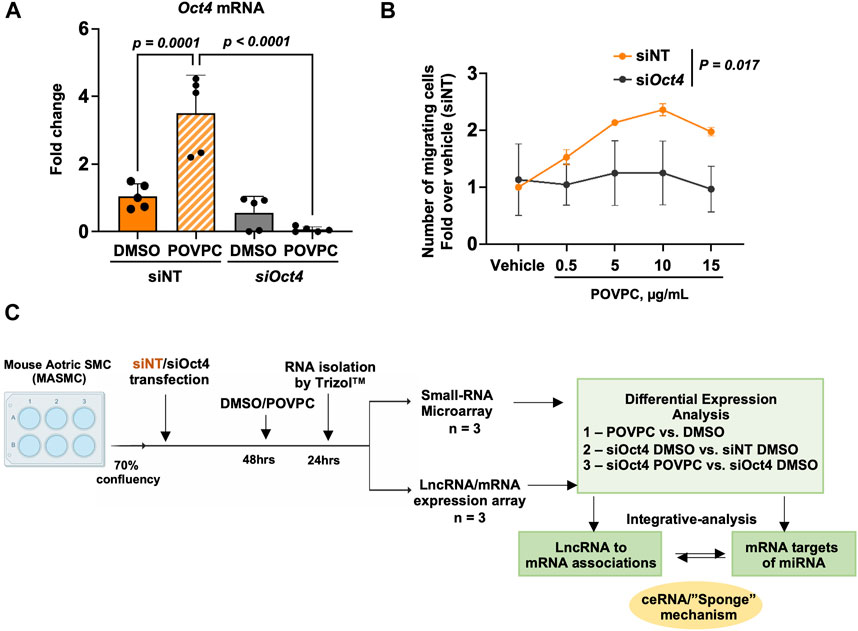
Figure 1. Experimental design. (A) Quantitative RT-PCR analysis to validate the knockdown of Oct4 in mouse aortic SMC (MASMC) transfected with blocking siOct4 or non-target siRNA (siNT), followed by the treatment with the pro-atherogenic oxidized phospholipid POVPC. Values = means ± s. e.m. p values were quantified by two-way ANOVA, followed by Tukey post hoc test; n = 5 biological replicates from 3 independent experiments. (B) Oct4 knockdown (siOct4) attenuated POVPC-induced SMC migration. p values for siNT vs. siOct4 across all concentrations of POVPC were quantified by two-way ANOVA; n = 3 independent experiments. (C) The schematic shows the overall workflow of the analysis. DAVID—The Database for Annotation, Visualization, and Integrated Discovery (DAVID) v6.8.
Next, as shown in the schematic diagram in Figure 1C, expression arrays were used to capture lncRNA/mRNA and small-RNA expression profiles in treated MASMC. Analyses identified multiple differentially expressed lncRNAs (Figure 2; Supplementary Table S1). A total of 632 lncRNA were significantly upregulated, and a total of 819 were downregulated in POVPC vs. DMSO treatment groups (effect of POVPC in wild type SMC) (Figure 2Ai), 1980 lncRNA were significantly upregulated, and 1,358 were downregulated in siOCT4-DMSO vs. siNT-DMSO groups (effect of OCT4 inactivation) (Figure 2Bi), and 752 lncRNAs were significantly upregulated, and 868 were downregulated in siOCT4-POVPC vs. siOCT4-DMSO groups (effect of POVPC in OCT4 deficient SMC) (Figure 2Ci). According to their location in genomic sequences and association with the neighboring gene regions, lncRNAs have been categorized into various classes to identify potential mechanisms of action for these molecules in regulating other genes (Herrera-Solorio et al., 2017), (Figure 2A–Cii). Using this categorization, differentially expressed lncRNAs were found to be 72% intergenic, 12% natural antisense, and 9% intronic antisense regions in POVPC vs. DMSO treatment groups, 70% intergenic, 10% natural antisense, and 12% intronic antisense regions in siOCT4-DMSO vs. siNT-DMSO groups, and 65% intergenic, 16% natural antisense, and 9% intronic antisense regions in siOCT4-POVPC vs. siOCT4-DMSO groups (Figure 2A–Cii) in the genome. Also, the analysis revealed that these lncRNAs were widely dispersed over all chromosomes (Figure 2A–Ciii).
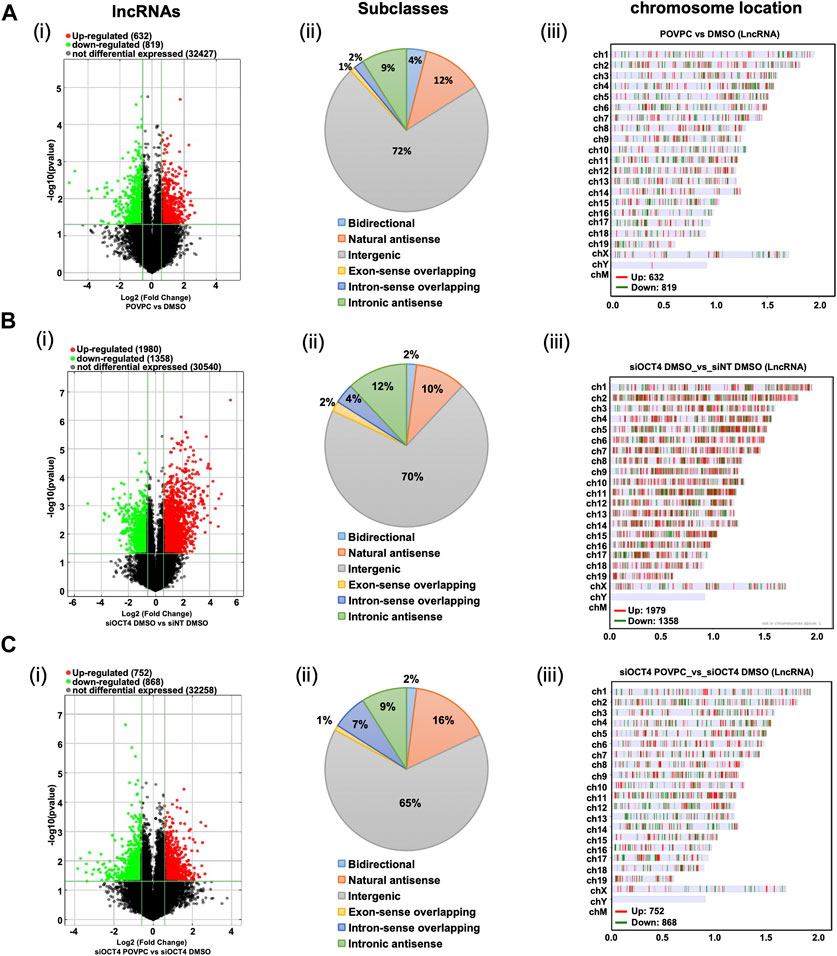
Figure 2. Differential expression and characteristics of lncRNAs. (A) POVPC vs. DMSO treatment groups (effect of POVPC in wild type SMC), (B) siOCT4-DMSO vs. siNT-DMSO groups (effect of OCT4 inactivation), (C) siOCT4-POVPC vs. siOCT4-DMSO groups (effect of POVPC in OCT4 deficient SMC). Volcano plot for significantly dysregulated lncRNAs (i), chromosome location (ii), and Subclasses (iii).
These data indicate that transient knockdown of Oct4 in SMC results in robust dysregulation of lncRNAs, and these OCT4-dependent changes in lncRNA expression are higher than changes in lncRNA expression in response to the oxidized phospholipid POVPC treatment.
Differential expression of mRNA in MASMC
Analysis of the mRNA expression levels (Supplementary Table S2) in the POVPC vs. DMSO treatment groups showed that 449 mRNAs were upregulated and 514 mRNAs were downregulated (Figure 3Ai), 991 up and 730 down in the siOCT4-DMSO vs. siNT-DMSO groups (Figure 3Bi), and 625 up and 720 down in the siOCT4-POVPC vs. siOCT4-DMSO groups (Figure 3Ci). To estimate the potential of the significantly differently expressed mRNAs to contribute to the phenotypic alterations in MASMC following OCT4 knockdown and POVPC administration, biological functional and pathway analysis was performed based on the GO terms and KEGG pathways.
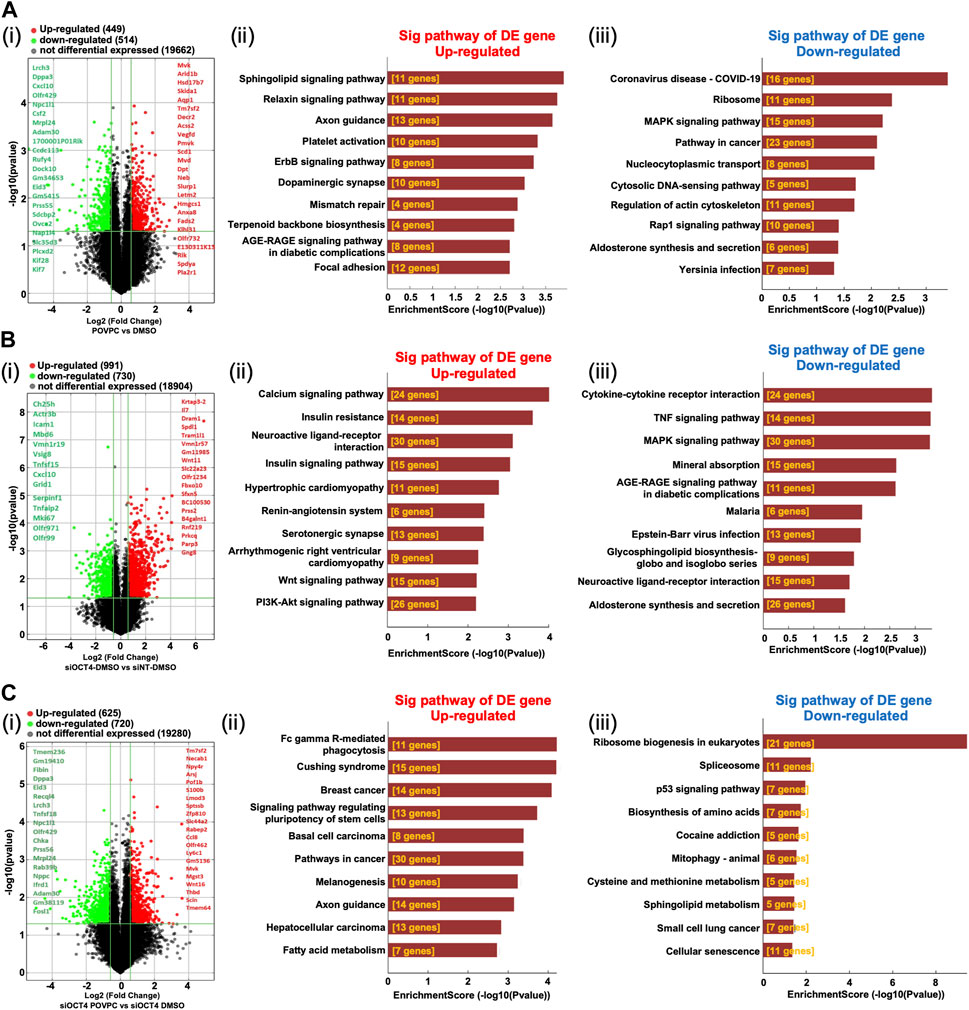
Figure 3. Differential expression and pathway analysis of mRNAs (A) POVPC vs. DMSO treatment groups (effect of POVPC in wild type SMC), (B) siOCT4-DMSO vs. siNT-DMSO groups (effect of OCT4 inactivation), (C) siOCT4-POVPC vs. siOCT4-DMSO groups (effect of POVPC in OCT4 deficient SMC). Volcano plot (i) and KEGG analysis for upregulated (ii) and downregulated (iii) mRNAs.
For POVPC vs. DMSO groups, the axon guidance and focal adhesion pathways appeared among the top upregulated KEGG pathways (Figure 3Aii). Moreover, the regulation of cell adhesion (GO:0030155) and cell migration (GO:0030335) GO terms were linked to both up- and downregulated genes. The COVID-19 and cancer pathways were significantly enriched among the downregulated genes in the POVPC-treated cells compared to the control group (Figure 3iii). For the siOCT4-DMSO vs. siNT-DMSO groups, the insulin and calcium signaling pathways appeared among the top upregulated KEGG pathways (Figure 3Bii), whereas the TNF signaling and cytokine-cytokine receptor pathways were enriched among the top downregulated pathways (Figure 3BIii). For siOCT4-POVPC vs. siOCT4-DMSO groups, the cancer pathways and phagocytosis KEGG pathways were upregulated (Figure 3Cii), whereas the ribosome biogenesis in eukaryotes, spliceosome, and cellular senescence pathways were downregulated (Figure 3Ciii). The ribosome biogenesis (GO:0042254) and mesenchymal cell differentiation (GO:0030154) GO terms were linked to both up- and downregulated genes.
Importantly, these results were consistent with our data showing that OCT4 deficiency attenuated POVPC-induced SMC migration (Figure 1B) and led to increased phagocytosis (Figure 4A).
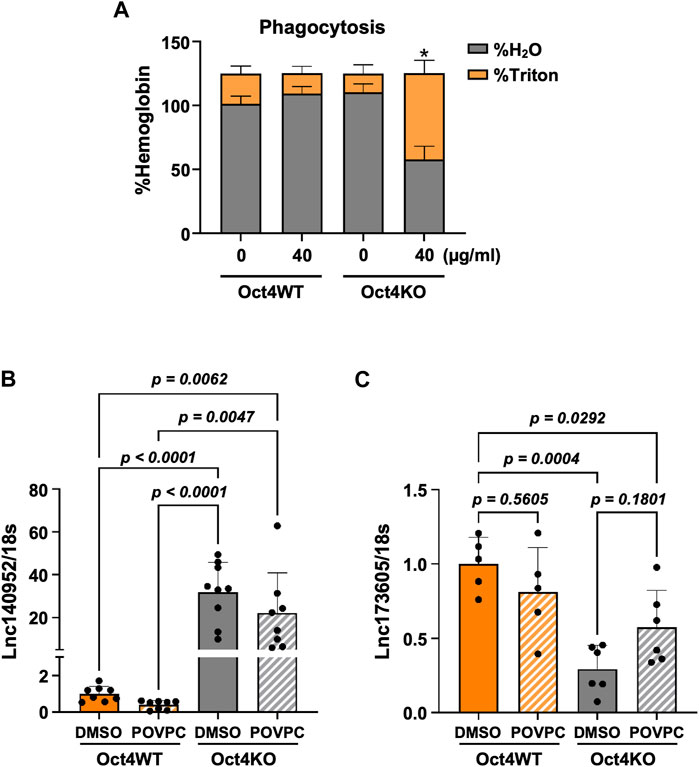
Figure 4. Loss of OCT4 in MASMC resulted in increased phagocytosis and differential expression of lncRNAs ENSMUST00000140952 (Hoxb5os) and ENSMUST00000173605. (A) Oct4 wild-type (WT) and knockout (KO) MASMC were treated with oxLDL for 3 days. At day four, mouse red blood cells (RBSs) were plated on the top of SMC for 24 h. The membrane-bound RBCs were lysed by hypotonic shock (1 min in water on ice). MASMCs containing phagocytosed RBCs were lysed by 1% Triton-X100 in PBS. Hemoglobin was then separately assayed in hypotonic (H2O) and Triton lysates using 2-7-diaminofluorene reagent. *p < 0.05 by two-way ANOVA, followed by Tukey post hoc test (for differences in hemoglobin in the Triton fractions); n = 3 independent experiments. (B,C) Quantitative RT-PCR showing relative levels of ENSMUST00000140952 (B) and ENSMUST00000173605 (C) in Oct4 WT and KO MASMSc treated with POVPC. p values were quantified by two-way ANOVA, followed by Tukey post hoc test; n = 5 biological replicates from 3 independent experiments.
Analysis of the functional relationships between lncRNAs and mRNAs in Oct4-deficient MASMCs
To determine the reported associations between the significantly differentially expressed mRNAs and lncRNAs and their established functional importance, we searched the LncDisease database. We examined the relationships between all mRNAs and lncRNAs that significantly altered in the treated group compared to the controls. Our analysis revealed 37 highly dysregulated lncRNAs connected to several differently expressed CVD-related mRNAs. The top candidates in the POVPC vs. DMSO groups were the upregulated lncRNA ENSMUST00000140952 linked to the elevated Hoxb6 mRNA and downregulated lncRNA ENSMUST00000151998 associated with downregulation of the nearby gene Notum (Figure 5Ai). In siOCT4-DMSO vs. si-NT-DMSO groups, the upregulated lncRNA ENSMUST00000131663 was associated with upregulated Aff3, upregulated ENSMUST00000155531 was associated with upregulated Zfp652, whereas downregulated ENSMUST00000137236 was associated with upregulation of Zmym1 (Figure 5Bi). In siOCT4-POVPC vs. siOCT4-DMSO groups (Figure 5Ci), upregulated ENSMUST00000140952 was associated with upregulated Hoxb5, whereas downregulated ENSMUST00000173605 was associated with upregulated Parp9.
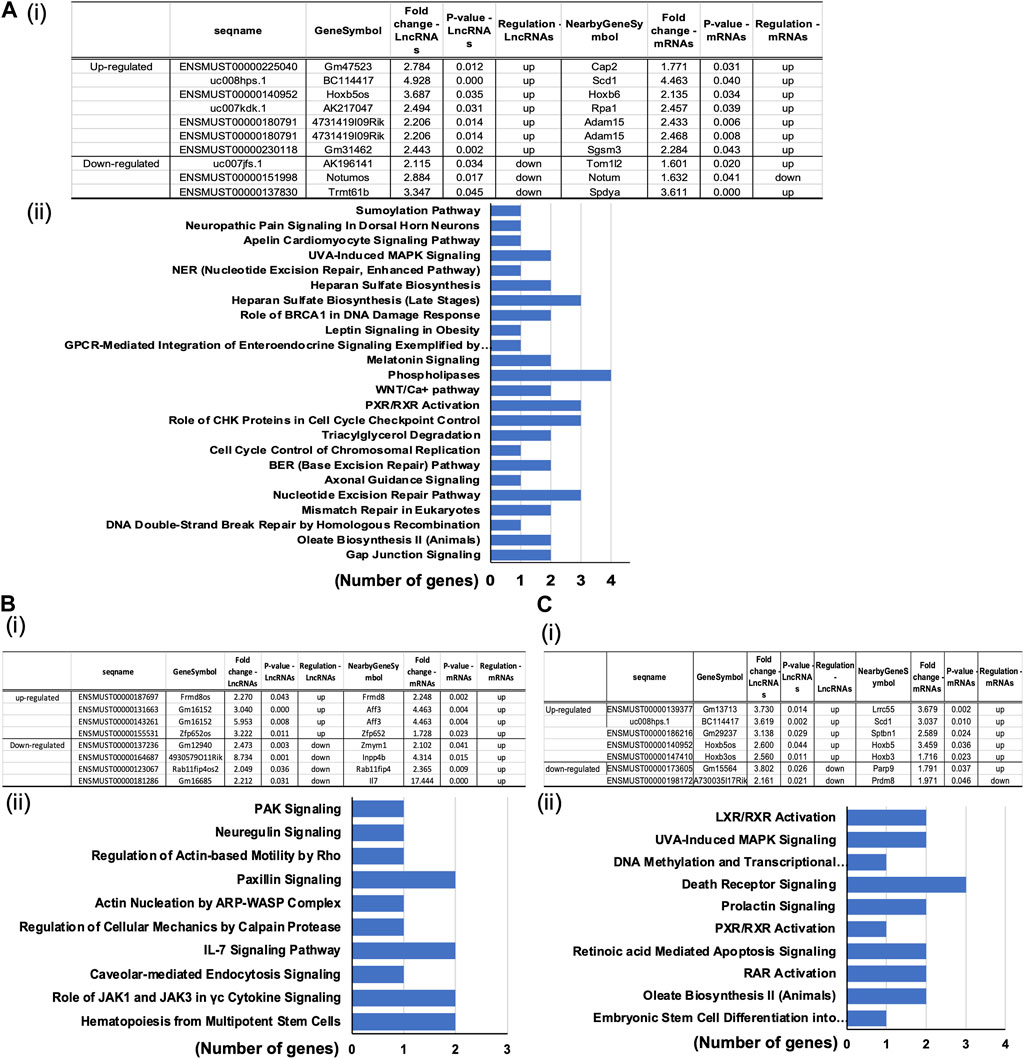
Figure 5. Predicted association of dysregulated lncRNAs to altered mRNA expression. (A) POVPC vs. DMSO treatment groups (effect of POVPC in wild type SMC), (B) siOCT4-DMSO vs. siNT-DMSO groups (effect of OCT4 inactivation), (C) siOCT4-POVPC vs. siOCT4-DMSO groups (effect of POVPC in OCT4 deficient SMC). Table lists microarray details of selected top lncRNA/mRNA predicted associations along with (i); KEGG pathway enrichment for mRNAs associated with lncRNAs based on the genomic proximity (<300 kbp) from IPA analysis (ii).
Furthermore, we analyzed the evolutionary conservation of these lncRNAs to assess whether these associations and potential mechanisms are conserved among vertebrates. The lncRNAs ENSMUST00000140952, ENSMUST00000155531, and ENSMUST00000137236 exhibit significant conservation across mammals, as calculated by phyloP (PHAST, cshl.edu), and UCSC browser multiz alignment, indicating the presence of potential orthologs with similar functions in other species (Kent et al., 2002). In contrast, ENSMUST00000173605 did not demonstrate any conservation (Supplementary Figure S1). Blast alignment of the three conserved lncRNAs identified three potential human orthologs: HOXB3-AS3, ZNF652-AS1, and ZMYM1, respectively. These orthologs not only display partial sequence conservation but are also positionally conserved within their genomic regions (Supplementary Figures S2–S4). Notably, the ZMYM1 gene is a protein-coding gene, but it has six non-protein processed transcripts that partially align (47%) with ENSMUST00000137236, suggesting they could function as antisense regulators for ZMYM1. While the conservation of ENSMUST00000140952.2 associated gene Hoxb5os with the human gene HOXB3-AS3 has been previously described (Papaioannou et al., 2019; Degani et al., 2021), further experimental validation of these bioinformatic predictions will be necessary to confirm the roles of ZNF652-AS1 and ZMYM1.
To validate our results further, we used our previously published bulk RNA sequencing data from OCT4 wild-type and knockout MASMC treated with POVPC (GSE75044) (Cherepanova et al., 2016). Importantly, despite the different OCT4 deficiency conditions (permanent long-term Oct4 knockout vs. transient siRNA-induced knockdown), we found that genes that were identified as targets of the lncRNA in our current study, were also significantly upregulated in the OCT4 knockout cells, including upregulation of Hoxb5/6, Aff3, and Zfp652. In contrast, Notum was upregulated in wild-type cells in response to POVPC treatment (Supplementary Figure S5). Moreover, quantitative RT-PCR showed marked increases in ENSMUST00000140952 (Figure 4B) and decreases in ENSMUST00000173605 (Figure 4C) in Oct4 knockout cells compared to wild-type SMC.
We examined the lncRNA and mRNA array data to find lncRNAs that may act as cis (promoter-associated) regulators of mRNAs since lncRNAs are known to regulate the expression of proximal protein-coding genes through cis-regulatory mechanisms (Marchese et al., 2017). Based on their genetic proximity (less than 300 kbp), 135 pairs of lncRNA-mRNA interactions were identified for the statistically differentially expressed lncRNAs, including antisense lncRNAs in all three experimental groups (Supplementary Table S3). Interestingly, the highest number of lncRNA-mRNA pairs was observed in the siOCT4-DMSO vs. siNT-DMSO groups (89 pairs vs. 23 pairs in other studied groups), indicating that the effect of OCT4 is a primary driver of the observed lncRNA/mRNA interactions.
Functional analysis of these mRNAs by the DAVID tool showed their connection to several functional pathways, including Phospholipases, Cell cycle control, and Nucleotide excision repair pathways (Figure 5Aii) in POVPC vs. DMSO groups. Pathways related to the Paxillin signaling, IL-7 signaling, and The role of JAK1 and JAK3 in cytokine signaling were some of the enriched pathways in the siOCT4-DMSO vs. siNT-DMSO groups (Figure 5Bii). Further, in siOCT4-POVPC vs. siOCT4-DMSO groups, enrichment of pathways related to the LXR/RAR receptor signaling and Apoptosis were observed Figure 5Cii).
Dysregulation of miRNAs in Oct4 deficient MASMCs
A previous study performed a co-analysis of miRNA/mRNA in SMC dedifferentiation and found that a third of transcripts are regulated by miRNA (Du et al., 2022). To identify OCT4-dependent miRNAs, we performed a small RNA microarray on RNA isolated from MASMC transfected with a non-targeted pool and siRNA-OCT4 and treated with DMSO/POVPC for 24 h (Supplementary Table S4). Differential expression analysis identified 21 miRNAs in the POVPC vs. DMSO groups (Figure 6Ai, ii), 72 miRNAs in the siOCT4-DMSO vs. siNT-DMSO (Figure 6Bi, ii) groups, and 36 miRNAs in siOCT4-POVPC vs. siOCT4-DMSO (Figure 6Ci, ii. Supplementary Figure S6 shows the volcano plots for all the significantly dysregulated miRNAs.
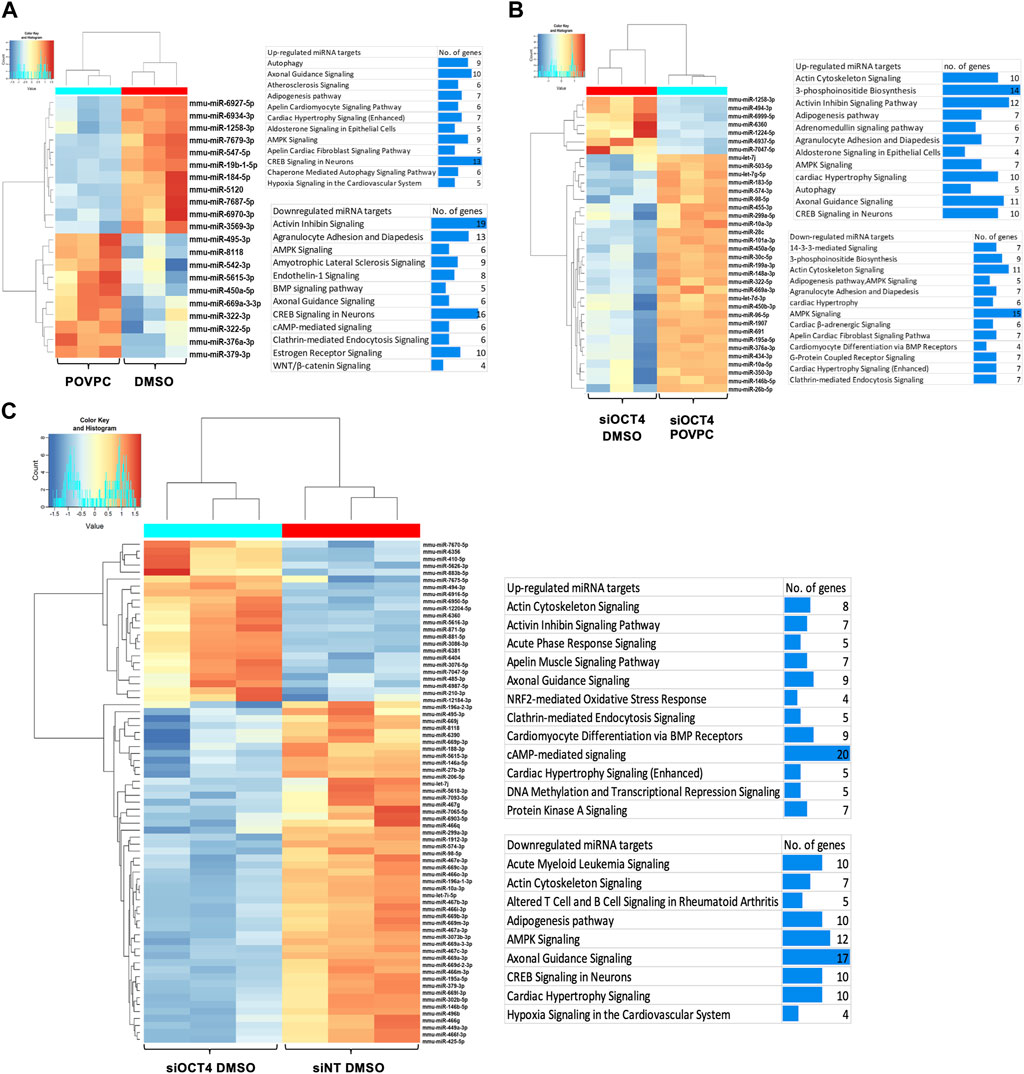
Figure 6. Differential expression of miRNAs and their predicted and experimentally validated mRNA targets in MASMCs (A) POVPC vs. DMSO treatment groups (effect of POVPC in wild type SMC), (B) siOCT4-DMSO vs. siNT-DMSO groups (effect of OCT4 inactivation), (C) siOCT4-POVPC vs. siOCT4-DMSO groups (effect of POVPC in OCT4 deficient SMC). Hierarchical clustering for differentially expressed miRNAs (i); biological functional analysis of significantly dysregulated miRNAs using IPA tool on all the known downstream mRNA targets of these miRNAs (i).
Differentially expressed miRNAs and mRNAs in different experimental groups were subjected to miRNA/mRNA cross-analyses. First, we identified these miRNAs’ known downstream target genes using the IPA miRNA target filtering tool. Then, we used DAVID to perform a biological functional analysis on these genes to determine which genes are regulated by these miRNAs and decide on their functional relevance (Figure 6Aiii, Biii, Ciii). This functional analysis utilizing differentially expressed mRNA targets of miRNAs indicated that differentially expressed miRNAs may upregulate mRNAs linked to the Atherosclerosis signaling, Axon guidance, and Autophagy in POVPC vs. DMSO groups and downregulate the Activin inhibin signaling, AMPK signaling, and Endothelin-1 signaling (Figure 6Aiii). Also, in the siOCT4-DMSO vs. si-NT-DMSO groups, mRNA targets of miRNAs, which were found to be upregulated, included Axon guidance, DNA methylation and Transcriptional repression signaling. In contrast, downregulated mRNA targets included AMPK signaling, Adipogenesis pathway, and Acute myeloid leukemia signaling (Figure 6Biii). In the siOCT4-POVPC vs. siOCT4-DMSO groups, the upregulated miRNA targets included Cardiac hypertrophy signaling, Axon guidance signaling, and CREB signaling in neurons, whereas the downregulated miRNA targets included AMPK signaling, Actin cytoskeleton signaling, and 3-phosphoinositide biosynthesis (Figure 6Ciii).
Integrative analysis of differentially expressed lncRNA, miRNA, and mRNA in OCT4-deficient MASMC
To explore the ability of lncRNA to act as ceRNAs (to affect mRNA levels by sequestering common miRNAs that target both lncRNAs and mRNAs), we integrated upregulated lncRNAs/mRNAs with the downregulated miRNAs. For this analysis, we chose lncRNAs that were highly upregulated with a raw signal intensity of more than 100 (corresponding to the highly expressed genes in microarrays). Only lncRNAs listed in GENCODE and Ensemble or characterized by Cabili et al. (Yuan et al., 2022) were chosen for annotation purposes. We obtained data on miRNA binding to particular lncRNAs using LncBase v.2, included in DIANA Tools (Paraskevopoulou et al., 2016).
Three of the picked lncRNAs that were upregulated had more than one binding site for six downregulated miRNAs. Thirteen of the upregulated mRNAs were potential targets of these six miRNAs. Next, we connected these lncRNA/miRNAs pairs to the expression of mRNAs by functioning via potential ceRNA-mechanism (Table 1). In the POVPC vs. DMSO and siOCT4-POVPC vs. siOCT4-DMSO groups, ENSMUST00000140952 was shown to have seven and four potential binding sites for the downregulated mmu-miR-10a and mmu-miR-196a-1, correspondingly. Both miRNAs were previously linked to cell migration (Cui et al., 2021; Zeng and Li, 2014). These relationships may help to understand how ENSMUST00000140952 contributes to the upregulation of mRNAs such as Hoxb6, Hoxb7, Hoxb4, Hoxb9, Hoxb5, and Hoxb3, which are controlled by these miRNAs (Yang et al., 2018) (Table 1). In the siOCT4-DMSO vs. siNT-DMSO groups, the ENSMUST00000131663 lncRNA contained four binding sites for downregulated mmu-miR-486-5p and mmu-miR-96 recognized for controlling a number of the elevated mRNAs involved in proliferation such as Aff3 (Lefevre et al., 2015), Rev1 (Chen et al., 2022), and Eif5b (Chukka et al., 2021), whereas the ENSMUST00000155531 lncRNA had four binding sites for downregulated mmu-miR-155 and mmu-miR-122 known to regulate mRNAs that were upregulated, such as Zfp652, Gngt2, Phospho1, and Phb (Table 1). Overall, these data indicate that upregulated lncRNAs may be able to sequester miRNAs in MASMCs, controlling the expression of their downstream target genes.
Our analysis also found an inter-regulatory link between downregulated mRNA/lncRNA and upregulated miRNAs. In this instance, three of the selected downregulated lncRNAs, including ENSMUST00000151998, ENSMUST00000137236, and ENSMUST00000173605, had more than one binding site for nine of the upregulated miRNAs (Table 2). Eighty-eigh of the downregulated mRNAs were the targets of these nine miRNAs. In the POVPC vs. DMSO groups, ENSMUST00000151998 had 22 and 6 anticipated binding sites for the upregulated mmu-miR-15a-5p and mmu-miR-322-5p, respectively. These lncRNA/miRNA relationships provide a potential mechanism on how this lncRNA contributes to the downregulation of mRNAs that are regulated by these miRNAs (Table 2). The ENSMUST00000137236 lncRNA, in siOCT4-DMSO vs. siNT-DMSO, had 15 and 13 predicted binding sites for the upregulated mmu-miR-146a-5p/mmu-miR-146b-5p and mmu-miR-15b-5p, respectively. These relationships provide a potential mechanism on how this lncRNA contributes to the downregulation of mRNAs (Table 2). In the siOCT4-POVPC vs. siOCT4-DMSO groups, downregulated ENSMUST00000173605 had three predicted binding sites for mmu-miR-10a-5p/mmu-miR-10b-5p, and two predicted sites for mmu-miR-15a-5p/mmu-miR-15b-5p and mmu-miR-30a-5p (all upregulated miRNAs), which were reported to regulate cell migration and apoptosis (Shen et al., 2021; Li et al., 2022; Chen et al., 2020), and phagocytosis (Cho et al., 2019; Murinello et al., 2019) and were associated with downregulated mRNAs (Table 2).
Our integrative analysis indicates that OCT4 is a critical regulator of lncRNAs and miRNAs and their cross-interactions in mouse aortic SMC. Together with our published and new functional studies about the role of OCT4 in SMC phenotypic transitions, we can conclude that OCT4-dependent lncRNA/miRNA/mRNA interactions might significantly contribute to SMC-dependent vascular pathologies, including atherosclerosis (Figure 7).
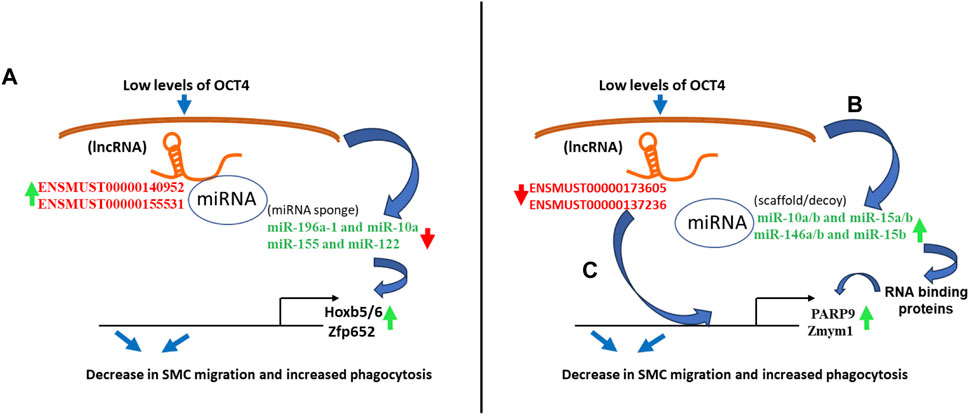
Figure 7. Schematic showing regulatory mechanisms obtained from the integrative analysis of the lncRNA-miRNA-mRNA interactions in OCT4 deficient SMCs. (A) Long non-coding RNAs (ENSMUST00000140952, ENSMUST00000155531) act as miRNA sponges (miR-196a-1 and miR-10a, miR-155 and miR-122) that regulate target gene expression (Hoxb5/6, Zfp652) (B) Decreases in lncRNAs (ENSMUST00000173605, ENSMUST00000137236) result in increased miRNA expression (miR-10a/b and miR-15a/b, miR-146a/b and miR-15b), which serve as scaffolding factors that “chaperone” RNA-binding proteins (RBPs) to form functional RNPs. In addition, or associated with this role, some small ncRNAs may act as molecular decoys, impairing the RBP-guided control of RNA fate by competing with other RNA substrates and, in turn, regulating the mRNA expression (Parp9, Zmym1) (C) Long non-coding RNAs (ENSMUST00000173605, ENSMUST00000137236) directly bind to mRNAs (Parp9, Zmym1) and regulate mRNA stability or competitively bind to mRNA to improve mRNA stability.
Discussion
We previously demonstrated that the pluripotency factor OCT4 plays a critical regulatory role in vascular SMC phenotypic transitions in vitro and in vivo. Bulk RNAseq performed on Oct4 knockout and wild-type MASMC revealed a robust dysregulation of gene expression in OCT4 deficient cells compared to control. However, nothing was known about the effects of OCT4 on non-coding RNAs or their potential contribution to OCT4-dependent responses in SMC. In this study, we performed an integrative bioinformatics analysis of the lncRNAs, miRNA, and mRNAs cross-interactions and predicted the potential role of these interactions in regulating OCT4-dependent phenotypic transitions of SMC. We utilized lncRNA/mRNA expression array and small RNA microarray to evaluate lncRNAs, miRNAs, and mRNA expression levels in mouse aortic SMC transfected with siRNA blocking Oct4 (OCT4 deficient SMC). Our integrative analysis demonstrated an important role of OCT4 in regulating lncRNAs and miRNAs in vascular SMC and revealed several OCT4-dependent lncRNA/miRNA/mRNA associations in the differential regulation of pathways associated with cell migration, proliferation, and phagocytosis.
Our integrative analysis of the lncRNA/miRNA/mRNA interactions demonstrated another level of complexity in the molecular mechanisms responsible for vascular SMC phenotypic transitions. We previously showed that pluripotency factors OCT4 and Krϋppel-like factor 4 (KLF4) are the key transcriptional factors regulating SMC transitions in vitro and in vivo. Using SMC-lineage tracing and SMC-specific knockout mouse models, we found that OCT4 played an atheroprotective role in SMC, while KLF4 had the opposite atheropromoting role (Shankman et al., 2015; Cherepanova et al., 2016). Intriguingly, we found that KLF4 directly positively regulates OCT4 activation (Cherepanova et al., 2016), an observation that does not fit the opposite roles of these two transcriptional factors. Interestingly, previous studies in human embryonic stem cells showed that miR-145 is involved in a double-negative feedback loop along with OCT4 and KLF4 regulating balancing in cell pluripotency and differentiation (Xu et al., 2009). Our new data make it interesting to speculate that lncRNA/miRNA interactions might be involved in the OCT4/KLF4 crosstalk in SMC.
One of the top upregulated lncRNAs in MASMCs treated with the oxidized phospholipid POVPC and under OCT4 deficient (siOCT4-POVPC vs. siOCT4-DMSO) conditions was ENSMUST00000140952 localized in the nucleus, in the sense strand from the Hoxb5/6 gene. HOXB5/6 has been associated with an increase in proliferation in breast cancer tissues and cell lines (Lee et al., 2015) and has been reported to promote the proliferation and migration of pancreatic cancer cells (Gao et al., 2020). Further studies are needed to determine the molecular relationship between Hoxb5/6 and the adjacent lncRNA ENSMUST00000140952 in MASMC and atherosclerosis.
Long non-coding RNAs exhibit a faster evolutionary rate and are not as conserved across vertebrates as other RNA sequences. Among the limited number of lncRNAs conserved across species, their sequences are only partially preserved (Johnsson et al., 2014). While the field of non-coding RNA research is progressing towards revising potential models for lncRNA functional conservation, with a focus on short motif and structural domain analysis (Ross et al., 2021; Ross and Ulitsky, 2022), the notable degree of conservation observed in certain lncRNAs, such as the case of Hoxb5os/HOXB3-AS3 (Papaioannou et al., 2019; Degani et al., 2021), suggests their potential significance during development and physiological processes. Additionally, their dysregulation in various disease conditions may further underscore their functional relevance.
In addition, our analyses predicted associations between the gene/lncRNA pairs: Zmym1/ENSMUST00000137236 and Parp9/ENSMUST00000173605. The expression of the lncRNA, ENSMUST00000137236, was downregulated, and its associated mRNA, Zmym1, was upregulated in OCT4 deficient (siOCT4-DMSO vs. siNT-DMSO) MASMCs. The Zmym1 gene has been reported to promote epithelial-to-mesenchymal transition and metastasis, cell growth and migration by inhibiting E-cadherin expression in gastric cancer (Yue et al., 2019). Downregulated ENSMUST00000173605 associated with upregulated Parp9 (siOCT4-POVPC vs. siOCT4-DMSO). Parp9 is reported to be involved in promoting the proliferation, survival, and chemotherapy resistance in lymphoma and prostate cancer, and its over-expression in human breast cancer is associated with cancer cell migration (Tang et al., 2018). In addition, PARP9 has been reported to regulate pro-inflammatory responses in macrophages (Iwata et al., 2016).
Analysis of differentially expressed miRNAs and their mRNA targets in OCT4 deficient cells revealed several upregulated miRNAs, including miR-10a/b, miR-15a/b, and miR-146a/b that target mRNAs involved in anti-migratory and pro-phagocytic signaling. Micro RNA-10a/b is implicated in the suppression of cardiac hypertrophy and cell survival (Stadthagen et al., 2013), while miR-15a/b has been implicated in other processes, such as suppression of cell survival and induction of apoptosis in chronic myeloid leukemia and endometrial cancer cells (Chen D. et al., 2017; Wang et al., 2017). Further, miR-146a is known to inhibit cancer migration, invasion, and metastasis by downregulating vascular endothelial growth factor (VEGF) through dual pathways in hepatocellular carcinoma cells (Sun et al., 2015; Yin et al., 2019).
It is known that lncRNAs control gene expression via sponging miRNAs, which in turn control mRNAs (Zhang et al., 2018). Several lncRNAs with binding sites for these downregulated anti-proliferative miRNAs were identified by our integrative bioinformatics analysis of the elevated lncRNA and mRNAs and the downregulated miRNAs in OCT4 deficient cells. We observed that ENSMUST00000140952 and ENSMUST00000155531 possessed binding sites for the miRNAs miR-196a-1 and miR-10a and miR-155 and miR-122, suggesting that they may be potential regulators of these miRNAs. Micro RNA-10a and miR-196a are reported to be oncogenic factors and are upregulated in various malignant tumor tissues (Bryant et al., 2012; Sun et al., 2012). Also, in non-small cell lung cancer (NSCLC) tissues, miR-196a was significantly upregulated, which in turn resulted in NSCLC cell migration and invasion, partially via downregulation of HOXA5 (Liu et al., 2012), and miR-155 has been implicated in with inflammation and cardiovascular diseases (Schroen and Heymans, 2012). In addition, previous reports characterized miR-155 and miR-122 as suppressors of cell migration and oxidative stress, including vascular SMC (Tong et al., 2023), whereas knockdown of miR-155-5p results in increased proliferation and migration of human brain micro-vessel ECs (Liu et al., 2015). Another significantly upregulated lncRNA, ENSMUST00000131663, had potential binding sites for miR-486-5p, which is known to function as a diagnostic marker for carotid artery stenosis and preventing endothelial dysfunction by inhibiting inflammation and oxidative stress (Zhu et al., 2022).
Similar to Chinnappan et al. (Chinnappan et al., 2019), who performed their integrative analysis on human pulmonary artery SMC, we found an association between the downregulated histone deacetylase 4 (Hdac4) and upregulated miR-10a/b (Table 2). This finding is very intriguing in that it suggests a critical role of this OCT4-dependent association in different types of SMC and adds another layer of complexity (chromatin modifiers) to the predicted OCT4-lncRNA-miRNA-regulatory network.
In summary, this study is the first attempt to elucidate novel potential OCT4-dependent interplay between lncRNAs, mRNAs, and miRNAs, which may play a role in phenotypic transitions of vascular SMC. We show that knockout of OCT4 results in robust dysregulation of lncRNA, miRNA, and their interactions, indicating that these interactions might be responsible for the observed OCT4-dependent functional effects (e.g., SMC migration, proliferation, and phagocytosis).
Limitations of the study and future directions
However, our study has a few limitations. First, the lncRNA functions were characterized based on their primary sequence. However, recent reports suggest the additional role of tertiary structure in deciding lncRNA function (Mattick et al., 2023). Therefore, further functional and mechanistic studies are needed to investigate the role of the predicted lncRNA/miRNA/mRNA associations in SMC phenotypic transitions in vitro and in vivo. Second, although most of the non-coding RNAs were conserved in our study, it is possible that the OCT4-dependent molecular mechanisms associated with the murine phenotypic transition of vascular SMC may not be directly applicable to humans. Hence, additional experiments are required to validate the significance of the identified genes. Third, all experiments and analyses were performed in vitro using primary culture SMC. Therefore, further in vivo studies are needed to validate these results.
Data availability statement
The datasets presented in this study can be found in online repositories. Array data generated in this study were deposited into the Gene Expression Omnibus database [accession numbers GSE249982 (lnc/mRNA) and GSE249983 (small RNA/miRNA)]. We also used our previously published bulk RNAseq data (GSE75044).
Ethics statement
The animal study was approved by Animal protocols were approved by the University of Virginia and Lerner Research Institute Animal Care and Use Committees. The study was conducted in accordance with the local legislation and institutional requirements.
Author contributions
AM: Conceptualization, Data curation, Formal Analysis, Investigation, Methodology, Validation, Writing–original draft, Writing–review and editing. JH: Data curation, Formal Analysis, Investigation, Validation, Writing–review and editing. IK: Conceptualization, Data curation, Investigation, Methodology, Writing–review and editing. JS: Data curation, Formal Analysis, Investigation, Visualization, Writing–review and editing. ST: Conceptualization, Data curation, Formal Analysis, Investigation, Writing–review and editing. CE-D: Conceptualization, Data curation, Formal Analysis, Investigation, Writing–review and editing. GO: Funding acquisition, Resources, Writing–review and editing. OC: Conceptualization, Data curation, Formal Analysis, Funding acquisition, Investigation, Methodology, Project administration, Resources, Supervision, Validation, Visualization, Writing–original draft, Writing–review and editing.
Funding
The author(s) declare that financial support was received for the research, authorship, and/or publication of this article. This work was supported by American Heart Association (AHA) grant 23CDA1044815 (to CE-D) and US National Institute of Health (NIH) grants R01 HL150193 (to OC) and R01 HL156849 and R01 HL136314 (to GO).
Acknowledgments
We thank Rupande Tripathi (University of Virginia), and Sarin Gole and Valentina Verbovetskaya (Lerner Research Institute, Cleveland Clinic) for technical assistance.
Conflict of interest
The authors declare that the research was conducted in the absence of any commercial or financial relationships that could be construed as a potential conflict of interest.
Publisher’s note
All claims expressed in this article are solely those of the authors and do not necessarily represent those of their affiliated organizations, or those of the publisher, the editors and the reviewers. Any product that may be evaluated in this article, or claim that may be made by its manufacturer, is not guaranteed or endorsed by the publisher.
Supplementary material
The Supplementary Material for this article can be found online at: https://www.frontiersin.org/articles/10.3389/fgene.2024.1356558/full#supplementary-material
References
Alencar, G. F., Owsiany, K. M., Karnewar, S., Sukhavasi, K., Mocci, G., Nguyen, A. T., et al. (2020). Stem cell pluripotency genes Klf4 and Oct4 regulate complex SMC phenotypic changes critical in late-stage atherosclerotic lesion pathogenesis. Circulation 142 (21), 2045–2059. doi:10.1161/CIRCULATIONAHA.120.046672
Bryant, A., Palma, C. A., Jayaswal, V., Yang, Y. W., Lutherborrow, M., and Ma, D. D. (2012). miR-10a is aberrantly overexpressed in Nucleophosmin1 mutated acute myeloid leukaemia and its suppression induces cell death. Mol. Cancer 11, 8. doi:10.1186/1476-4598-11-8
Cabili, M. N., Trapnell, C., Goff, L., Koziol, M., Tazon-Vega, B., Regev, A., et al. (2011). Integrative annotation of human large intergenic noncoding RNAs reveals global properties and specific subclasses. Genes Dev. 25 (18), 1915–1927. doi:10.1101/gad.17446611
Chen, C., Tang, J., Xu, S., Zhang, W., and Jiang, H. (2020). miR-30a-5p inhibits proliferation and migration of lung squamous cell carcinoma cells by targeting FOXD1. Biomed. Res. Int. 2020, 2547902. doi:10.1155/2020/2547902
Chen, D., Wu, D., Shao, K., Ye, B., Huang, J., and Gao, Y. (2017b). MiR-15a-5p negatively regulates cell survival and metastasis by targeting CXCL10 in chronic myeloid leukemia. Am. J. Transl. Res. 9 (9), 4308–4316.
Chen, X., Yan, C. C., Zhang, X., and You, Z. H. (2017a). Long non-coding RNAs and complex diseases: from experimental results to computational models. Brief. Bioinform 18 (4), 558–576. doi:10.1093/bib/bbw060
Chen, Y., Jie, X., Xing, B., Wu, Z., Yang, X., Rao, X., et al. (2022). REV1 promotes lung tumorigenesis by activating the Rad18/SERTAD2 axis. Cell Death Dis. 13 (2), 110. doi:10.1038/s41419-022-04567-5
Cherepanova, O. A., Gomez, D., Shankman, L. S., Swiatlowska, P., Williams, J., Sarmento, O. F., et al. (2016). Activation of the pluripotency factor OCT4 in smooth muscle cells is atheroprotective. Nat. Med. 22 (6), 657–665. doi:10.1038/nm.4109
Cherepanova, O. A., Pidkovka, N. A., Sarmento, O. F., Yoshida, T., Gan, Q., Adiguzel, E., et al. (2009). Oxidized phospholipids induce type VIII collagen expression and vascular smooth muscle cell migration. Circ. Res. 104 (5), 609–618. doi:10.1161/CIRCRESAHA.108.186064
Chinnappan, M., Gunewardena, S., Chalise, P., and Dhillon, N. K. (2019). Analysis of lncRNA-miRNA-mRNA interactions in hyper-proliferative human pulmonary arterial smooth muscle cells. Sci. Rep. 9 (1), 10533. doi:10.1038/s41598-019-46981-4
Cho, Y. K., Son, Y., Kim, S. N., Song, H. D., Kim, M., Park, J. H., et al. (2019). MicroRNA-10a-5p regulates macrophage polarization and promotes therapeutic adipose tissue remodeling. Mol. Metab. 29, 86–98. doi:10.1016/j.molmet.2019.08.015
Chukka, P. A. R., Wetmore, S. D., and Thakor, N. (2021). Established and emerging regulatory roles of eukaryotic translation initiation factor 5B (eIF5B). Front. Genet. 12, 737433. doi:10.3389/fgene.2021.737433
Cui, J., Yuan, Y., Shanmugam, M. K., Anbalagan, D., Tan, T. Z., Sethi, G., et al. (2021). MicroRNA-196a promotes renal cancer cell migration and invasion by targeting BRAM1 to regulate SMAD and MAPK signaling pathways. Int. J. Biol. Sci. 17 (15), 4254–4270. doi:10.7150/ijbs.60805
Degani, N., Lubelsky, Y., Perry, R. B., Ainbinder, E., and Ulitsky, I. (2021). Highly conserved and cis-acting lncRNAs produced from paralogous regions in the center of HOXA and HOXB clusters in the endoderm lineage. PLoS Genet. 17 (7), e1009681. doi:10.1371/journal.pgen.1009681
Derrien, T., Johnson, R., Bussotti, G., Tanzer, A., Djebali, S., Tilgner, H., et al. (2012). The GENCODE v7 catalog of human long noncoding RNAs: analysis of their gene structure, evolution, and expression. Genome Res. 22 (9), 1775–1789. doi:10.1101/gr.132159.111
Dobnikar, L., Taylor, A. L., Chappell, J., Oldach, P., Harman, J. L., Oerton, E., et al. (2018). Disease-relevant transcriptional signatures identified in individual smooth muscle cells from healthy mouse vessels. Nat. Commun. 9 (1), 4567. doi:10.1038/s41467-018-06891-x
Du, M., Espinosa-Diez, C., Liu, M., Ahmed, I. A., Mahan, S., Wei, J., et al. (2022). miRNA/mRNA co-profiling identifies the miR-200 family as a central regulator of SMC quiescence. iScience 25 (5), 104169. doi:10.1016/j.isci.2022.104169
Du, Z., Sun, T., Hacisuleyman, E., Fei, T., Wang, X., Brown, M., et al. (2016). Integrative analyses reveal a long noncoding RNA-mediated sponge regulatory network in prostate cancer. Nat. Commun. 7, 10982. doi:10.1038/ncomms10982
Gao, Y., Fei, X., Kong, L., and Tan, X. (2020). HOXB5 promotes proliferation, migration, and invasion of pancreatic cancer cell through the activation of the GSK3β/β-catenin pathway. Anticancer Drugs 31 (8), 828–835. doi:10.1097/CAD.0000000000000948
Hanson, M. S., Stephenson, A. H., Bowles, E. A., Sridharan, M., Adderley, S., and Sprague, R. S. (2008). Phosphodiesterase 3 is present in rabbit and human erythrocytes and its inhibition potentiates iloprost-induced increases in cAMP. Am. J. Physiol. Heart Circ. Physiol. 295 (2), H786–H793. doi:10.1152/ajpheart.00349.2008
He, C., Wang, Y., Zhu, J., Li, Y., Chen, J., and Lin, Y. (2022). Integrative analysis of lncRNA-miRNA-mRNA regulatory network reveals the key lncRNAs implicated potentially in the differentiation of adipocyte in goats. Front. Physiol. 13, 900179. doi:10.3389/fphys.2022.900179
Herrera-Solorio, A. M., Armas-Lopez, L., Arrieta, O., Zuniga, J., Pina-Sanchez, P., and Avila-Moreno, F. (2017). Histone code and long non-coding RNAs (lncRNAs) aberrations in lung cancer: implications in the therapy response. Clin. Epigenetics 9, 98. doi:10.1186/s13148-017-0398-3
Huang da, W., Sherman, B. T., and Lempicki, R. A. (2009). Systematic and integrative analysis of large gene lists using DAVID bioinformatics resources. Nat. Protoc. 4 (1), 44–57. doi:10.1038/nprot.2008.211
Iwata, H., Goettsch, C., Sharma, A., Ricchiuto, P., Goh, W. W., Halu, A., et al. (2016). PARP9 and PARP14 cross-regulate macrophage activation via STAT1 ADP-ribosylation. Nat. Commun. 7, 12849. doi:10.1038/ncomms12849
Johnsson, P., Lipovich, L., Grander, D., and Morris, K. V. (2014). Evolutionary conservation of long non-coding RNAs; sequence, structure, function. Biochim. Biophys. Acta 1840 (3), 1063–1071. doi:10.1016/j.bbagen.2013.10.035
Kent, W. J., Sugnet, C. W., Furey, T. S., Roskin, K. M., Pringle, T. H., Zahler, A. M., et al. (2002). The human genome browser at UCSC. Genome Res. 12 (6), 996–1006. doi:10.1101/gr.229102
Kolb, S., Vranckx, R., Huisse, M. G., Michel, J. B., and Meilhac, O. (2007). The phosphatidylserine receptor mediates phagocytosis by vascular smooth muscle cells. J. Pathol. 212 (3), 249–259. doi:10.1002/path.2190
Kugel, J. F., and Goodrich, J. A. (2012). Non-coding RNAs: key regulators of mammalian transcription. Trends Biochem. Sci. 37 (4), 144–151. doi:10.1016/j.tibs.2011.12.003
Kumar, S., Williams, D., Sur, S., Wang, J. Y., and Jo, H. (2019). Role of flow-sensitive microRNAs and long noncoding RNAs in vascular dysfunction and atherosclerosis. Vasc. Pharmacol. 114, 76–92. doi:10.1016/j.vph.2018.10.001
Lee, J. Y., Hur, H., Yun, H. J., Kim, Y., Yang, S., Kim, S. I., et al. (2015). HOXB5 promotes the proliferation and invasion of breast cancer cells. Int. J. Biol. Sci. 11 (6), 701–711. doi:10.7150/ijbs.11431
Lefevre, L., Omeiri, H., Drougat, L., Hantel, C., Giraud, M., Val, P., et al. (2015). Combined transcriptome studies identify AFF3 as a mediator of the oncogenic effects of β-catenin in adrenocortical carcinoma. Oncogenesis 4 (7), e161. doi:10.1038/oncsis.2015.20
Li, H., Zhu, H., and Ge, J. (2016). Long noncoding RNA: recent updates in atherosclerosis. Int. J. Biol. Sci. 12 (7), 898–910. doi:10.7150/ijbs.14430
Li, Y., Li, D., Yang, Y., and Wang, J. (2022). miR-15a-5p regulates liver cancer cell migration, apoptosis and cell cycle progression by targeting transcription factor E2F3. Crit. Rev. Eukaryot. Gene Expr. 32 (6), 1–10. doi:10.1615/CritRevEukaryotGeneExpr.2022042503
Libby, P. (2021). The changing landscape of atherosclerosis. Nature 592 (7855), 524–533. doi:10.1038/s41586-021-03392-8
Liu, X. H., Lu, K. H., Wang, K. M., Sun, M., Zhang, E. B., Yang, J. S., et al. (2012). MicroRNA-196a promotes non-small cell lung cancer cell proliferation and invasion through targeting HOXA5. BMC Cancer 12, 348. doi:10.1186/1471-2407-12-348
Liu, Y., Pan, Q., Zhao, Y., He, C., Bi, K., Chen, Y., et al. (2015). MicroRNA-155 regulates ROS production, NO generation, apoptosis and multiple functions of human brain microvessel endothelial cells under physiological and pathological conditions. J. Cell Biochem. 116 (12), 2870–2881. doi:10.1002/jcb.25234
Liu, Z., Yang, J., Wang, N., Liu, J., Geng, J., Zhu, J., et al. (2023). Integrative lncRNA, circRNA, and mRNA analysis reveals expression profiles of six forensic body fluids/tissue. Int. J. Leg. Med. doi:10.1007/s00414-023-03131-w
Marchese, F. P., Raimondi, I., and Huarte, M. (2017). The multidimensional mechanisms of long noncoding RNA function. Genome Biol. 18 (1), 206. doi:10.1186/s13059-017-1348-2
Mattick, J. S., Amaral, P. P., Carninci, P., Carpenter, S., Chang, H. Y., Chen, L. L., et al. (2023). Long non-coding RNAs: definitions, functions, challenges and recommendations. Nat. Rev. Mol. Cell Biol. 24 (6), 430–447. doi:10.1038/s41580-022-00566-8
Mazziotta, C., Cervellera, C. F., Badiale, G., Vitali, I., Touze, A., Tognon, M., et al. (2023b). Distinct retinoic gene signatures discriminate Merkel cell polyomavirus-positive from -negative Merkel cell carcinoma cells. J. Med. Virol. 95 (7), e28949. doi:10.1002/jmv.28949
Mazziotta, C., Cervellera, C. F., Lanzillotti, C., Touze, A., Gaboriaud, P., Tognon, M., et al. (2023a). MicroRNA dysregulations in Merkel cell carcinoma: molecular mechanisms and clinical applications. J. Med. Virol. 95 (1), e28375. doi:10.1002/jmv.28375
McClellan, M., Brown, N., Califf, R. M., and Warner, J. J. (2019). Call to action: urgent challenges in cardiovascular disease: a presidential advisory from the American Heart association. Circulation 139 (9), e44–e54. doi:10.1161/CIR.0000000000000652
McMullen, J. R., and Drew, B. G. (2016). Long non-coding RNAs (lncRNAs) in skeletal and cardiac muscle: potential therapeutic and diagnostic targets? Clin. Sci. (Lond). 130 (24), 2245–2256. doi:10.1042/CS20160244
Mercer, T. R., Neph, S., Dinger, M. E., Crawford, J., Smith, M. A., Shearwood, A. M., et al. (2011). The human mitochondrial transcriptome. Cell 146 (4), 645–658. doi:10.1016/j.cell.2011.06.051
Murinello, S., Usui, Y., Sakimoto, S., Kitano, M., Aguilar, E., Friedlander, H. M., et al. (2019). miR-30a-5p inhibition promotes interaction of Fas(+) endothelial cells and FasL(+) microglia to decrease pathological neovascularization and promote physiological angiogenesis. Glia 67 (2), 332–344. doi:10.1002/glia.23543
Noh, J. H., Kim, K. M., McClusky, W. G., Abdelmohsen, K., and Gorospe, M. (2018). Cytoplasmic functions of long noncoding RNAs. Wiley Interdiscip. Rev. RNA 9 (3), e1471. doi:10.1002/wrna.1471
Pan, H., Xue, C., Auerbach, B. J., Fan, J., Bashore, A. C., Cui, J., et al. (2020). Single-cell genomics reveals a novel cell state during smooth muscle cell phenotypic switching and potential therapeutic targets for atherosclerosis in mouse and human. Circulation 142 (21), 2060–2075. doi:10.1161/CIRCULATIONAHA.120.048378
Papaioannou, D., Petri, A., Dovey, O. M., Terreri, S., Wang, E., Collins, F. A., et al. (2019). The long non-coding RNA HOXB-AS3 regulates ribosomal RNA transcription in NPM1-mutated acute myeloid leukemia. Nat. Commun. 10 (1), 5351. doi:10.1038/s41467-019-13259-2
Paraskevopoulou, M. D., Vlachos, I. S., Karagkouni, D., Georgakilas, G., Kanellos, I., Vergoulis, T., et al. (2016). DIANA-LncBase v2: indexing microRNA targets on non-coding transcripts. Nucleic Acids Res. 44 (D1), D231–D238. doi:10.1093/nar/gkv1270
Pidkovka, N. A., Cherepanova, O. A., Yoshida, T., Alexander, M. R., Deaton, R. A., Thomas, J. A., et al. (2007). Oxidized phospholipids induce phenotypic switching of vascular smooth muscle cells in vivo and in vitro. Circ. Res. 101 (8), 792–801. doi:10.1161/CIRCRESAHA.107.152736
Poniewierska-Baran, A., Sluczanowska-Glabowska, S., Malkowska, P., Sierawska, O., Zadroga, L., Pawlik, A., et al. (2022). Role of miRNA in melanoma development and progression. Int. J. Mol. Sci. 24 (1), 201. doi:10.3390/ijms24010201
Qian, Y., Mao, Z. D., Shi, Y. J., Liu, Z. G., Cao, Q., and Zhang, Q. (2018). Comprehensive analysis of miRNA-mRNA-lncRNA networks in non-smoking and smoking patients with chronic obstructive pulmonary disease. Cell Physiol. Biochem. 50 (3), 1140–1153. doi:10.1159/000494541
Rashid, F., Shah, A., and Shan, G. (2016). Long non-coding RNAs in the cytoplasm. Genomics Proteomics Bioinforma. 14 (2), 73–80. doi:10.1016/j.gpb.2016.03.005
Ross, C. J., Rom, A., Spinrad, A., Gelbard-Solodkin, D., Degani, N., and Ulitsky, I. (2021). Uncovering deeply conserved motif combinations in rapidly evolving noncoding sequences. Genome Biol. 22 (1), 29. doi:10.1186/s13059-020-02247-1
Ross, C. J., and Ulitsky, I. (2022). Discovering functional motifs in long noncoding RNAs. Wiley Interdiscip. Rev. RNA 13 (4), e1708. doi:10.1002/wrna.1708
Salviano-Silva, A., Lobo-Alves, S. C., Almeida, R. C., Malheiros, D., and Petzl-Erler, M. L. (2018). Besides pathology: long non-coding RNA in cell and tissue homeostasis. Noncoding RNA 4 (1), 3. doi:10.3390/ncrna4010003
Schroen, B., and Heymans, S. (2012). Small but smart--microRNAs in the centre of inflammatory processes during cardiovascular diseases, the metabolic syndrome, and ageing. Cardiovasc Res. 93 (4), 605–613. doi:10.1093/cvr/cvr268
Shankman, L. S., Gomez, D., Cherepanova, O. A., Salmon, M., Alencar, G. F., Haskins, R. M., et al. (2015). KLF4-dependent phenotypic modulation of smooth muscle cells has a key role in atherosclerotic plaque pathogenesis. Nat. Med. 21 (6), 628–637. doi:10.1038/nm.3866
Shen, D., Zhao, H. Y., Gu, A. D., Wu, Y. W., Weng, Y. H., Li, S. J., et al. (2021). miRNA-10a-5p inhibits cell metastasis in hepatocellular carcinoma via targeting SKA1. Kaohsiung J. Med. Sci. 37 (9), 784–794. doi:10.1002/kjm2.12392
Shi, G., and Jin, Y. (2010). Role of Oct4 in maintaining and regaining stem cell pluripotency. Stem Cell Res. Ther. 1 (5), 39. doi:10.1186/scrt39
Shin, J., Tkachenko, S., Gomez, D., Tripathi, R., Owens, G. K., and Cherepanova, O. A. (2023). Smooth muscle cells-specific loss of OCT4 accelerates neointima formation after acute vascular injury. Front. Cardiovasc Med. 10, 1276945. doi:10.3389/fcvm.2023.1276945
Stadthagen, G., Tehler, D., Hoyland-Kroghsbo, N. M., Wen, J., Krogh, A., Jensen, K. T., et al. (2013). Loss of miR-10a activates lpo and collaborates with activated Wnt signaling in inducing intestinal neoplasia in female mice. PLoS Genet. 9 (10), e1003913. doi:10.1371/journal.pgen.1003913
Sun, M., Liu, X. H., Li, J. H., Yang, J. S., Zhang, E. B., Yin, D. D., et al. (2012). MiR-196a is upregulated in gastric cancer and promotes cell proliferation by downregulating p27(kip1). Mol. Cancer Ther. 11 (4), 842–852. doi:10.1158/1535-7163.MCT-11-1015
Sun, X., Zhang, J., Hou, Z., Han, Q., Zhang, C., and Tian, Z. (2015). miR-146a is directly regulated by STAT3 in human hepatocellular carcinoma cells and involved in anti-tumor immune suppression. Cell Cycle 14 (2), 243–252. doi:10.4161/15384101.2014.977112
Tang, X., Zhang, H., Long, Y., Hua, H., Jiang, Y., and Jing, J. (2018). PARP9 is overexpressed in human breast cancer and promotes cancer cell migration. Oncol. Lett. 16 (3), 4073–4077. doi:10.3892/ol.2018.9124
Tong, Y., Zhou, M. H., Li, S. P., Zhao, H. M., Zhang, Y. R., Chen, D., et al. (2023). MiR-155-5p attenuates vascular smooth muscle cell oxidative stress and migration via inhibiting BACH1 expression. Biomedicines 11 (6), 1679. doi:10.3390/biomedicines11061679
Tripathi, V., Shen, Z., Chakraborty, A., Giri, S., Freier, S. M., Wu, X., et al. (2013). Long noncoding RNA MALAT1 controls cell cycle progression by regulating the expression of oncogenic transcription factor B-MYB. PLoS Genet. 9 (3), e1003368. doi:10.1371/journal.pgen.1003368
Wang, J., Ma, R., Ma, W., Chen, J., Yang, J., Xi, Y., et al. (2016). LncDisease: a sequence based bioinformatics tool for predicting lncRNA-disease associations. Nucleic Acids Res. 44 (9), e90. doi:10.1093/nar/gkw093
Wang, L. X., Wan, C., Dong, Z. B., Wang, B. H., Liu, H. Y., and Li, Y. (2019). Integrative analysis of long noncoding RNA (lncRNA), microRNA (miRNA) and mRNA expression and construction of a competing endogenous RNA (ceRNA) network in metastatic melanoma. Med. Sci. Monit. 25, 2896–2907. doi:10.12659/MSM.913881
Wang, Z. M., Wan, X. H., Sang, G. Y., Zhao, J. D., Zhu, Q. Y., and Wang, D. M. (2017). miR-15a-5p suppresses endometrial cancer cell growth via Wnt/β-catenin signaling pathway by inhibiting WNT3A. Eur. Rev. Med. Pharmacol. Sci. 21 (21), 4810–4818.
Wilusz, J. E., Sunwoo, H., and Spector, D. L. (2009). Long noncoding RNAs: functional surprises from the RNA world. Genes Dev. 23 (13), 1494–1504. doi:10.1101/gad.1800909
Wirka, R. C., Wagh, D., Paik, D. T., Pjanic, M., Nguyen, T., Miller, C. L., et al. (2019). Atheroprotective roles of smooth muscle cell phenotypic modulation and the TCF21 disease gene as revealed by single-cell analysis. Nat. Med. 25 (8), 1280–1289. doi:10.1038/s41591-019-0512-5
Xu, N., Papagiannakopoulos, T., Pan, G., Thomson, J. A., and Kosik, K. S. (2009). MicroRNA-145 regulates OCT4, SOX2, and KLF4 and represses pluripotency in human embryonic stem cells. Cell 137 (4), 647–658. doi:10.1016/j.cell.2009.02.038
Xue, M., Zhuo, Y., and Shan, B. (2017). MicroRNAs, long noncoding RNAs, and their functions in human disease. Methods Mol. Biol. 1617, 1–25. doi:10.1007/978-1-4939-7046-9_1
Yang, S., Lee, J. Y., Hur, H., Oh, J. H., and Kim, M. H. (2018). Up-regulation of HOXB cluster genes are epigenetically regulated in tamoxifen-resistant MCF7 breast cancer cells. BMB Rep. 51 (9), 450–455. doi:10.5483/bmbrep.2018.51.9.020
Yin, C., Han, Q., Xu, D., Zheng, B., Zhao, X., and Zhang, J. (2019). SALL4-mediated upregulation of exosomal miR-146a-5p drives T-cell exhaustion by M2 tumor-associated macrophages in HCC. Oncoimmunology 8 (7), 1601479. doi:10.1080/2162402X.2019.1601479
Yuan, L., Tang, Y., Yin, L., Lin, X., Luo, Z., Wang, S., et al. (2022). Microarray analysis reveals changes in tRNA-derived small RNAs (tsRNAs) expression in mice with septic cardiomyopathy. Genes (Basel) 13 (12), 2258. doi:10.3390/genes13122258
Yue, B., Song, C., Yang, L., Cui, R., Cheng, X., Zhang, Z., et al. (2019). METTL3-mediated N6-methyladenosine modification is critical for epithelial-mesenchymal transition and metastasis of gastric cancer. Mol. Cancer 18 (1), 142. doi:10.1186/s12943-019-1065-4
Zeng, T., and Li, G. (2014). MicroRNA-10a enhances the metastatic potential of cervical cancer cells by targeting phosphatase and tensin homologue. Mol. Med. Rep. 10 (3), 1377–1382. doi:10.3892/mmr.2014.2370
Zhang, G., Li, S., Lu, J., Ge, Y., Wang, Q., Ma, G., et al. (2018). LncRNA MT1JP functions as a ceRNA in regulating FBXW7 through competitively binding to miR-92a-3p in gastric cancer. Mol. Cancer 17 (1), 87. doi:10.1186/s12943-018-0829-6
Zhang, Q., Han, Z., Zhu, Y., Chen, J., and Li, W. (2020). The role and specific mechanism of OCT4 in cancer stem cells: a review. Int. J. Stem Cells 13 (3), 312–325. doi:10.15283/ijsc20097
Zhou, W. Y., Cai, Z. R., Liu, J., Wang, D. S., Ju, H. Q., and Xu, R. H. (2020). Circular RNA: metabolism, functions and interactions with proteins. Mol. Cancer 19 (1), 172. doi:10.1186/s12943-020-01286-3
Zhou, Y., Zhang, S., Ji, W., Gan, X., Hua, L., Hou, C., et al. (2021). LncRNA landscape of coronary atherosclerosis reveals differentially expressed LncRNAs in proliferation and migration of coronary artery smooth muscle cells. Front. Cell Dev. Biol. 9, 656636. doi:10.3389/fcell.2021.656636
Zhu, B., Liu, W., Xu, Q., and Liu, H. L. (2022). MicroRNA-486-5p functions as a diagnostic marker for carotid artery stenosis and prevents endothelial dysfunction through inhibiting inflammation and oxidative stress. Bioengineered 13 (4), 8667–8675. doi:10.1080/21655979.2022.2054500
Keywords: smooth muscle cells, long non-coding RNAs, micro RNAs, integrative analysis, OCT4 activation
Citation: Mahajan A, Hong J, Krukovets I, Shin J, Tkachenko S, Espinosa-Diez C, Owens GK and Cherepanova OA (2024) Integrative analysis of the lncRNA-miRNA-mRNA interactions in smooth muscle cell phenotypic transitions. Front. Genet. 15:1356558. doi: 10.3389/fgene.2024.1356558
Received: 15 December 2023; Accepted: 25 March 2024;
Published: 10 April 2024.
Edited by:
Fang Fang, RTI International, United StatesReviewed by:
John Charles Rotondo, University of Ferrara, ItalyMontserrat Climent, Humanitas Research Hospital, Italy
Copyright © 2024 Mahajan, Hong, Krukovets, Shin, Tkachenko, Espinosa-Diez, Owens and Cherepanova. This is an open-access article distributed under the terms of the Creative Commons Attribution License (CC BY). The use, distribution or reproduction in other forums is permitted, provided the original author(s) and the copyright owner(s) are credited and that the original publication in this journal is cited, in accordance with accepted academic practice. No use, distribution or reproduction is permitted which does not comply with these terms.
*Correspondence: Olga A. Cherepanova, cherepol@ccf.org