Cholinergic and non-cholinergic projections from the pedunculopontine and laterodorsal tegmental nuclei to the medial geniculate body in guinea pigs
- 1 Department of Anatomy and Neurobiology, Northeastern Ohio Universities Colleges of Medicine and Pharmacy, Rootstown, OH, USA
- 2 School of Biomedical Sciences, Kent State University, Kent, OH, USA
The midbrain tegmentum is the source of cholinergic innervation of the thalamus and has been associated with arousal and control of the sleep/wake cycle. In general, the innervation arises bilaterally from the pedunculopontine tegmental nucleus (PPT) and the laterodorsal tegmental nucleus (LDT). While this pattern has been observed for many thalamic nuclei, a projection from the LDT to the medial geniculate body (MG) has been questioned in some species. We combined retrograde tracing with immunohistochemistry for choline acetyltransferase (ChAT) to identify cholinergic projections from the brainstem to the MG in guinea pigs. Double-labeled cells (retrograde and immunoreactive for ChAT) were found in both the PPT (74%) and the LDT (26%). In both nuclei, double-labeled cells were more numerous on the ipsilateral side. About half of the retrogradely labeled cells were immunonegative, suggesting they are non-cholinergic. The distribution of these immunonegative cells was similar to that of the immunopositive ones: more were in the PPT than the LDT and more were on the ipsilateral than the contralateral side. The results indicate that both the PPT and the LDT project to the MG, and suggest that both cholinergic and non-cholinergic cells contribute substantially to these projections.
Introduction
The medial geniculate body (MG) is part of the auditory thalamus and serves as the primary source of ascending input to the auditory cortex. The MG, like other thalamic nuclei, is under the influence of projections from the brainstem cholinergic nuclei (Mesulam et al., 1983; Steriade et al., 1988; Oakman et al., 1999). These projections arise from the pedunculopontine tegmental nucleus (PPT) and the laterodorsal tegmental nucleus (LDT), collectively referred to as the pontomesencephalic tegmentum (PMT). The cholinergic projections form part of the ascending arousal system and, as such, control the firing mode of thalamic cells through both nicotinic and muscarinic effects (Tebecis, 1972; McCormick and Prince, 1987; Steriade et al., 1988; Jones, 2005). These effects control the flow of information to the cortex and underlie changes in thalamic responsiveness during the sleep-wake cycle and at varying levels of arousal during wakefulness (Edeline, 2003; Steriade, 2004; Hennevin et al., 2007).
There have been numerous studies of cholinergic innervation of the thalamus and a general pattern of projections has emerged (Shute and Lewis, 1967; Hallanger et al., 1987; Steriade et al., 1988). The PPT contributes more cells than the LDT to the thalamic projections. Both nuclei project bilaterally with an ipsilateral dominance (Steriade et al., 1988). It has been reported that up to 85% of the PMT projection to thalamic relay nuclei is cholinergic (Steriade et al., 1988). None of the studies have focused exclusively on the MG, but Steriade et al. (1988) reported that the projection to the MG in cats follows the general pattern described above. In contrast, results from both retrograde and anterograde tracing experiments have reported that there is no projection to the MG from the LDT in rats (Satoh and Fibiger, 1986; Hallanger et al., 1987; Cornwall et al., 1990). The extent to which projections from the LDT and the PPT have distinct functions is unclear; nonetheless, the similarities in their projection patterns to most thalamic nuclei suggest that the contributions are similar across the many systems represented by those thalamic nuclei. The possible lack of a projection from the LDT to the MG suggests that the auditory thalamus may be exceptional in receiving a unique pattern of cholinergic inputs from the brainstem.
Another question concerns the types of cells in the PMT that project to the thalamus. The PPT and the LDT are known as cholinergic nuclei, but contain a variety of cell types, including distinct populations of cholinergic, glutamatergic, and GABAergic neurons (Boucetta and Jones, 2009; Wang and Morales, 2009). Recently there has been increasing interest in the contribution of non-cholinergic cells to the functions ascribed to the PMT. For example, Boucetta and Jones (2009) have recorded the physiological activity of cholinergic, glutamatergic, and GABAergic cells in the PMT and have suggested that all three cell types act in concert to control behavioral states across the sleep-wake cycle. Previous studies have acknowledged a non-cholinergic PMT projection to the thalamus (Steriade et al., 1988), but the relative number of cholinergic and non-cholinergic cells contributing to the projection is unknown for the MG and for most thalamic targets.
We used a combination of retrograde tracers and immunohistochemistry to examine the projection from the PMT to the MG. We describe the relative contributions of the PPT and the LDT to the projection, the proportions of ipsilateral and contralateral projections, and the proportions of cholinergic and non-cholinergic components.
Materials and Methods
During all experiments, efforts were made to minimize suffering and the number of animals used. All procedures were performed in accordance with the Institutional Animal Care and Use Committee and the National Institutes of Health guidelines on the ethical use of animals. Twelve pigmented guinea pigs (Elm Hill; Chelmsford, MA, USA) of either gender weighing 250–500 g were used.
Surgery
Sterile instruments and aseptic technique were used for all surgical procedures. Each animal was anesthetized with isoflurane (4% for induction, 1.5–2.5% for maintenance) in oxygen. The animal’s head was shaved and cleansed with Betadine (Purdue Products L.P., Stamford, CT, Rochester, NY, USA). Ophthalmic ointment (Moisture Eyes PM, Bausch & Lomb, Rochester, NY, USA) was applied to each eye. Atropine sulfate (0.05 mg/kg, i.m.) was administered to decrease respiratory secretions during surgery, and Ketofen (ketoprofen 3 mg/kg, i.m.; Henry Schein, Melville, NY, USA) was given for post-operative pain control. The animal was positioned with its head in a stereotaxic frame. A feedback-controlled heating pad was used for maintaining the animal’s body temperature.
An incision was made in the scalp and the wound edges were injected with Marcaine (0.25% bupivacaine with epinephrine 1:200,000; Hospira, Inc., Lake Forest, IL, USA). A dental drill was used to make a small hole in the skull. Details about the tracers used and the injection parameters for each case are provided in Table 1. After the tracer injection, the opening in the skull was covered with Gelfoam (Harvard Apparatus, Holliston, MA, USA). The scalp was sutured. Anesthesia was then discontinued and the animal was removed from the stereotaxic frame and placed in a clean cage. The animal was monitored in its cage until it was ambulatory and able to eat and drink.
Perfusion and Sectioning
After surgery, the animal remained in the animal care facility for 4–25 days to allow for transport of the tracers. The animal was given an overdose of either sodium pentobarbital (440 mg/kg, i.p.) or isoflurane (5% in oxygen, inhaled). After cessation of breathing and loss of the withdrawal reflex, the animal was perfused through the vascular system with Tyrode’s solution, then 250 ml of 4% paraformaldehyde in 0.1 M phosphate buffer, pH 7.4, followed by 250 ml of the same fixative with 10% sucrose added. The brain was removed and placed in fixative with 25 or 30% sucrose and stored at 4°C. The following day the cerebellum was removed. In some cases the cortex was also removed. The brainstem or brainstem and cortex were frozen and cut in the transverse or sagittal plane on a sliding microtome into 40–50 μm thick sections. The sections were collected serially into six sets.
Histology
For most cases, in one tissue set (containing every sixth section), the tissue sections containing the MG were reacted with cytochrome oxidase (CO) for the identification of MG subdivisions (Anderson et al., 2007). In some cases, one set was stained with thionin for identification of cytoarchitectural borders and landmarks. The remaining sets of tissue were used for immunohistochemistry.
Choline aceyltransferase (ChAT) immunohistochemistry was used to identify putative cholinergic cells as previously reported (Motts et al., 2008) except that primary antibody concentrations were increased two- to eight-fold for fluorescence imaging. Briefly, sections were incubated (1 day at 4°C) with goat anti-ChAT antibody (Chemicon AB 144P, [Millipore, Billerica, MA, USA]) diluted 1:25–1:100. The sections were then treated with 1% biotinylated rabbit anti-goat antibody (BA-5000, Vector Laboratories, Burlingame, CA, USA) and labeled with streptavidin conjugated to a fluorescent marker (AlexaFluor 488 [green] or AlexaFluor 647 [near-infrared]; Invitrogen, Carlsbad, CA, USA) that could be distinguished from the tracers used in the case. The sections were mounted on gelatin-coated slides, air dried, and coverslipped with DPX (Sigma).
Data Analysis
A Neurolucida reconstruction system (version 8; MBF Bioscience, Williston, VT, USA) attached to a Zeiss Axioplan II microscope (Carl Zeiss, Inc., Thornwood, NY, USA) was used to plot labeled cells, nuclear borders, and injection sites. Plots were exported to Adobe Illustrator CS2 (Adobe Systems, Inc., San Jose, CA, USA) for final figure construction.
The brainstem cholinergic nuclei (PPT and LDT) in guinea pigs have been described in detail previously (Leonard et al., 1995; Motts et al., 2008). Some of the borders of the PPT and the LDT are indistinct in a thionin stain, so the distribution of ChAT-immunopositive cells was used to define the extent of the nuclei.
A Zeiss AxioImager Z1 fluorescence microscope with AxioCam HRm (monochrome) and HRc (color) cameras (Zeiss) was used to take photomicrographs. Monochrome images, including all images of AlexaFluor 647, which fluoresces at near infrared wavelengths, were pseudocolored either with the camera software (AxioVision 4.6, Zeiss) or with Photoshop CS2. Adobe Photoshop CS2 was used to add scale bars and labels, scale, and crop images, erase background around tissue sections, and adjust intensity levels in photomicrographs.
Three criteria were used to select cases for quantitative summary. First, sections had to be free from tissue damage that would prevent assessment of injection sites and areas of retrogradely labeled cells. Second, the ChAT immunostain had to be bright and even across sections. Third, there had to be at least 50 retrogradely labeled PMT cells in a bin (every sixth section). Of the 12 animals used for this study, results from 8 animals met the criteria to be included in the quantitative summary. All of the cases used for quantitative analysis and illustration were cut in the transverse plane. Table 1 shows the experimental parameters for each case.
Results
We combined retrograde tracing and immunohistochemistry to identify the cholinergic and non-cholinergic PMT inputs to the MG. The patterns of labeled cells were similar qualitatively for the different tracers and different survival times used in this study. Our experiments included both large injections that involved several MG subdivisions, and smaller injections, which were largely confined to one or two subdivisions (Figure 1).
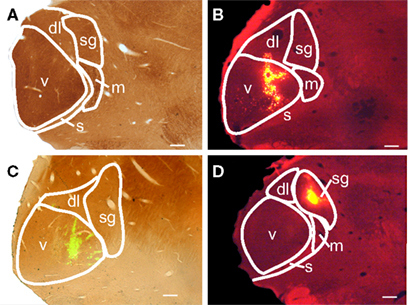
Figure 1. Photomicrographs of medial geniculate body (MG) subdivisions and representative tracer injection sites in transverse sections. (A) Section stained with cytochrome oxidase (CO) showing the subdivisions of MG: dl, dorsolateral; m, medial; s, shell; sg, suprageniculate; v, ventral. (B) Injection of red beads that is centered in the ventral MG (v) and extends into the dorsolateral subdivision (dl). This photomicrograph is of the right MG, but was flipped to make comparisons between the cases easier. GP 595R. (C) Injection of green beads confined to the ventral subdivision. The image is an overlay of a fluorescence image to visualize the GB and a brightfield image to show the CO counterstain. GP 587L. (D) An injection of red beads within the suprageniculate subdivision (sg). This photomicrograph is of the right MG, but was flipped to make comparisons between the cases easier. GP 586R. Scale bars = 0.5 mm for A–D.
A large injection labeled many cells in the PMT and in numerous areas associated with the brainstem auditory pathways. The latter areas included the cochlear nucleus, superior olivary complex, ventral nucleus of the lateral lemniscus and adjacent paralemniscal area, nucleus sagulum, and a region dorsal to the dorsal nucleus of the lateral lemniscus (where a collection of cholinergic cells stretches from the PPT to the laterally placed nucleus sagulum). Among all these regions, only the nuclei of the PMT contained retrogradely labeled cells that were also immunopositive for ChAT (Figure 2). The remainder of this paper focuses on the retrogradely labeled cells within the PMT.
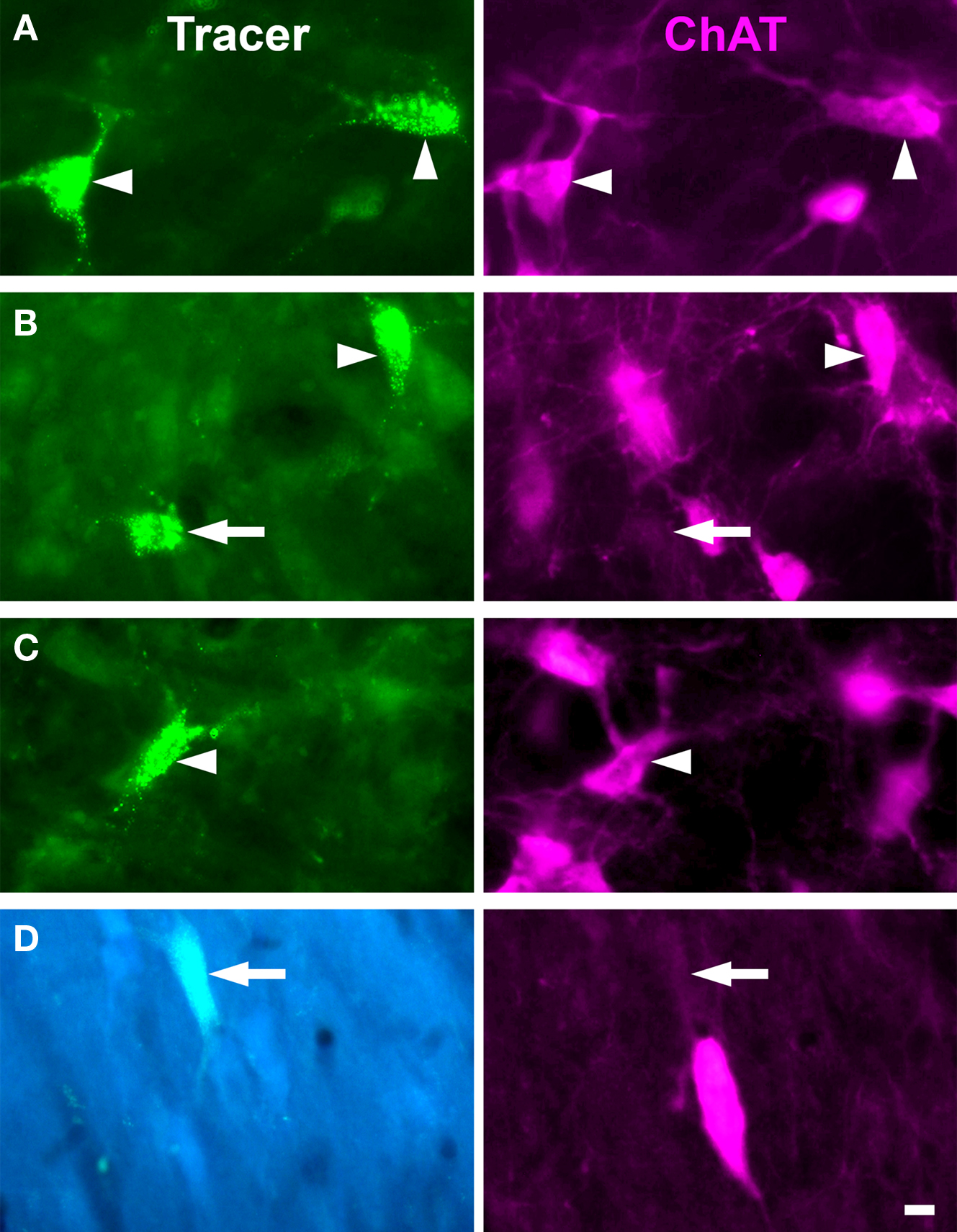
Figure 2. Photomicrographs of labeled cells in the PMT after injections in MG. In each panel the left image shows cells labeled with retrograde tracer [green beads for A–C (GP 585L), FluoroGold for D (GP 484L)]. The right image shows the same field of view filtered for ChAT immunolabel. Arrowheads indicate cells labeled with both tracer and immunolabel; arrows indicate cells labeled with tracer only. (A,B) PPT cells projecting to the ipsilateral MG. (C) PPT cell projecting to the contralateral MG. (D) LDT cell projecting to contralateral MG. All photomicrographs are taken from transverse sections. Scale bar = 10 μm.
The distribution of labeled cells in the PMT after a large FG injection is illustrated in Figure 3A. This case (GP 604L) had a large injection in the left MG that almost completely filled the dorsolateral subdivision and included significant portions of the medial, suprageniculate, and ventral subdivisions. A small part of the shell region was included in the injection as well. The injection spread dorsally and rostrally beyond MG to include the dorsal lateral geniculate and lateral posterior nuclei. We found retrogradely labeled cells in the PPT and the LDT on both sides. More labeled cells were present on the ipsilateral side and, on each side, more were present in the PPT than the LDT. In each of the nuclei, some of the retrogradely labeled cells were ChAT immunopositive; overall, these constituted 53% of the retrogradely labeled cells in PMT (Table 2). In each nucleus, immunonegative cells were located near immunopositive cells, suggesting that the immunostain was effective in the area and that the tracer-labeled, immunonegative cells were non-cholinergic (Figure 2). Together, these data indicate that both cholinergic and non-cholinergic PMT cells contribute to the projection to the MG.
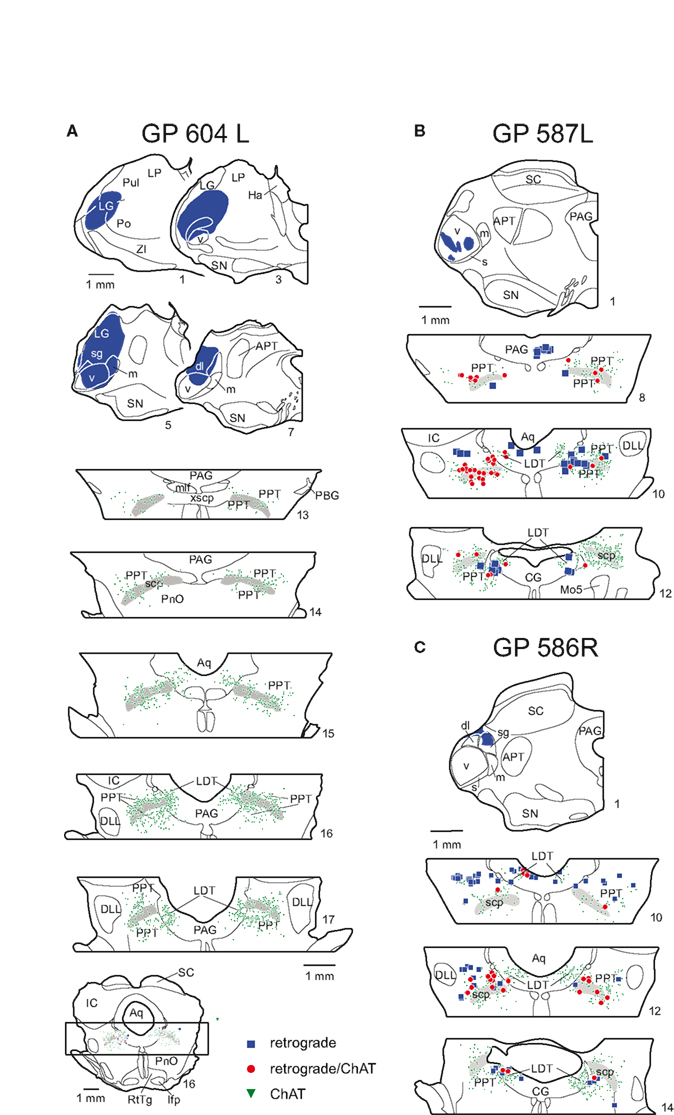
Figure 3. Plots of labeled cells in the PMT after large (A) or small (B,C) injections in the thalamus. (A) Drawings of transverse sections showing a large FluoroGold injection (blue shading, sections 1–7 [left side of section only]) in the left thalamus and labeled cells in the PMT nuclei (sections 13–17). An entire midbrain section is shown (bottom of column) for orientation of brainstem sections. Only the portion of tegmentum that included the PPT and the LDT (indicated by box) are shown in the remaining sections. The legend indicates the type of label in all panels; each symbol represents at least one labeled cell. GP 604L. (B) Drawing through the center of a green bead injection site confined to the ventral subdivision of the MG. Labeled cells are shown in the PMT at 3 rostro-caudal levels. GP 587L. (C) Drawing through the center of a red bead injection in the MG suprageniculate subdivision, and resulting label in the PMT. The drawings were flipped to make comparisons between the cases easier. GP 586R. In all panels, the sections are numbered from rostral to caudal with each whole number representing a 300-μm spacing. The extent of the PPT and LDT nuclei are indicated by the distribution of the ChAT immunopositive cells (green triangles). The ChAT immunopositive cells within the periaqueductal gray (PAG) are in the LDT; the ChAT immunopositive cells outside the PAG are in the PPT. The superior cerebellar peduncle is shown in gray as it is a helpful landmark for the PPT. APT, anterior pretectal nucleus; Aq, aqueduct; CG, central gray; dl, dorsolateral subdivision of MG; DLL, dorsal nucleus of lateral lemniscus; Ha, habenula; IC, inferior colliculus; LDT, laterodorsal tegmental nucleus; lfp; longitudinal fasciculus of the pons; LG, lateral geniculate body; LP, lateral posterior nucleus; m, medial subdivision of MG; mlf, medial longitudinal fasciculus; Mo5, motor nucleus of 5th nerve; PAG, periaqueductal gray; PBG, parabigeminal nucleus; PnO, pontine reticular nucleus, oral part; Po, posterior nucleus of the thalamus; PPT, pedunculopontine tegmental nucleus; Pul, pulvinar nucleus; RtTg, reticulotegmental nucleus of the pons; s, shell subdivision of MG; SC, superior colliculus; scp, superior cerebellar peduncle; sg, suprageniculate subdivision of MG; SN, substantia nigra; v, ventral subdivision of MG; xscp, decussation of scp; ZI, zona incerta.
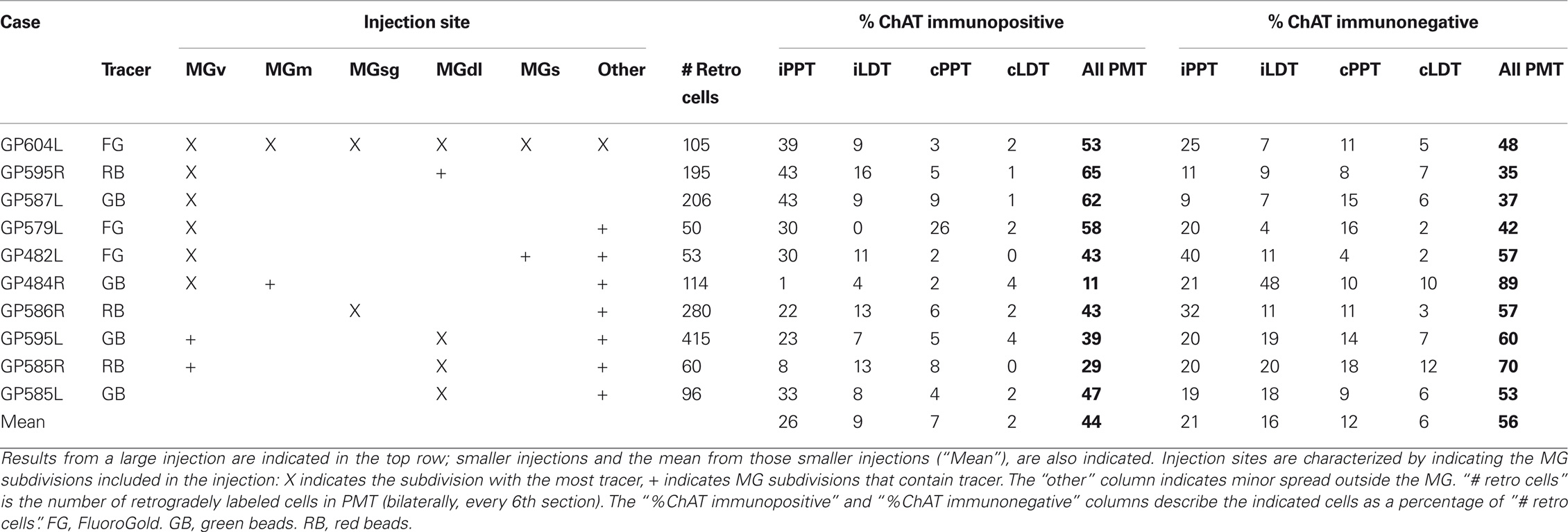
Table 2. Description of the injection sites and resultant PMT label in cases used for quantitative analysis.
Interpretation of the results just described is complicated by spread of the injection into areas surrounding the MG, which also receive inputs from the PMT nuclei (Steriade et al., 1988). We now describe results from smaller injections; two of these were confined entirely to the MG and the remainder had only slight spread outside the MG (Table 2). Figure 3B shows an injection in the MGv and resulting label in PMT. Figure 3C shows an injection in the suprageniculate subdivision of the MG and resulting label. In both cases, the results were qualitatively similar to large injections. Quantitative analysis revealed some additional points (Table 2; Figure 4). The LDT contained double-labeled cells in every case, although they were relatively few and not always present bilaterally. While the percentage of ChAT-immunopositive cells varied (11–65%), it was not possible to relate the variation to the location of the injection; e.g., the 3 highest percentages for ChAT+ cells were associated with injections in the MGv, but so was the lowest value. The reasons for the variation are not clear. Across the nine experiments with small injections, 44% of the retrogradely labeled cells in the PMT were ChAT immunopositive (compared to 53% after the large injection). In fact, the ipsilateral PPT was the only nucleus in which the retrogradely labeled ChAT-immunopositive cells outnumbered the retrogradely labeled ChAT-immunonegative cells on average. This suggests that non-cholinergic cells may be more prominent in the PMT projections to the MG than to other thalamic nuclei.
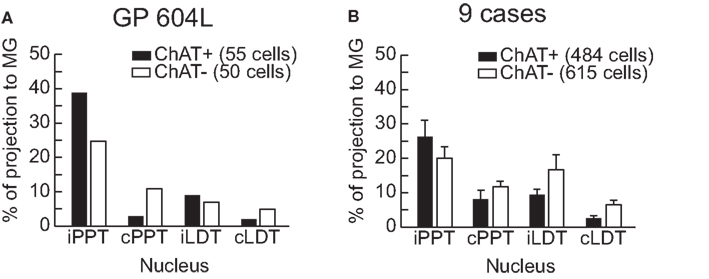
Figure 4. Graphs to illustrate the contribution of ipsilateral and contralateral PPT and LDT to the overall projection to MG. Tracer-labeled cells were counted in every sixth section and tallied according to whether they were ChAT+ or ChAT−. The results are expressed as a percentage of the total number of tracer-labeled cells. (A) Results from a very large injection (GP604L). (B) Aggregate results from nine cases with small injections located almost entirely within the MG. Error bars indicate standard error of the mean. cLDT, contralateral laterodorsal tegmental nucleus; cPPT, contralateral pedunculopontine tegmental nucleus; iLDT, ipsilateral laterodorsal tegmental nucleus; iPPT, ipsilateral pedunculopontine tegmental nucleus.
Discussion
Our results show that both the PPT and the LDT are sources of cholinergic input to the MG in guinea pigs. In addition, a sizable non-cholinergic projection to the MG also arises in the PPT and the LDT. We first address the technical issues of our experiments. We then discuss our data in the context of previous studies and the functional significance of the projections.
Technical Considerations
We used an antibody against ChAT as a marker for cholinergic cells. ChAT is considered a specific marker for cholinergic cells (Levey and Wainer, 1982; Armstrong et al., 1983; German et al., 1985; Maley et al., 1988). The antibody has been validated previously in guinea pigs (Motts et al., 2008), and we used the same fixation and tissue processing parameters as in the previous study. We see clearly labeled cells in the expected areas, such as the cranial nerve motor nuclei. We conclude that the immunolabeled cells are cholinergic.
It is always possible that an immunohistochemical assay will fail to label every cell that contains the antigen. However, we obtained immunopositive and immunonegative retrogradely labeled cells within the same focal plane (Figure 2), suggesting that the immunolabeling reagents penetrated the tissue and that the immunonegative cells are likely to be non-cholinergic. In addition to cholinergic cells, the PPT and the LDT contain GABAergic and glutamatergic cells (Vincent et al., 1986; Lavoie and Parent, 1994; Ford et al., 1995; Leonard et al., 1995; Jia et al., 2003; Wang and Morales, 2009). It is likely that cells of either or both of these types were among our immunonegative cells. Future studies are necessary to identify the non-cholinergic cell types and determine their contributions to the PMT projections. This information will help in understanding the effects of the PMT projection to the MG across the sleep-wake cycle.
Two final issues relate to the injections. First, some injections spread beyond the MG. Other thalamic nuclei are innervated by the PMT, and we would expect that some of the cells labeled in these cases terminate outside the MG. However, small injections confined to the MG produce a distribution of retrogradely labeled cells that was similar to that after the larger injections. We conclude that the quantitative differences between our cases with small injections versus the large injection reflect specifics of the PMT projection to the MG. More meaningful comparison will require future experiments with small injections confined to other thalamic nuclei.
A related issue concerns the possibility of labeling axons of passage whereby injections in the caudal thalamus, where the MG is located, could label PMT cells that project to more rostral thalamic (or extrathalamic) targets. We have used red beads and green beads in many of our experiments. These tracers are less likely than other tracers to be taken up by axons of passage (Katz and Iarovici, 1990). In addition, we have used micropipettes for some of our injections. Micropipettes cause less damage than syringes, thus decreasing the likelihood of tracer uptake by axons of passage. While we cannot rule out the possibility of labeling PMT cells that project outside the MG, we believe that such cells were in the minority and are unlikely to alter the main conclusions.
Functional Implications
Our results are generally consistent with previous studies that the MG is a target of brainstem cholinergic cells (Hallanger et al., 1987; Steriade et al., 1988). These results are very similar to those in cats (Steriade et al., 1988) but differ from those in rats, where both anterograde and retrograde experiments failed to label a projection from the LDT to the MG (Satoh and Fibiger, 1986; Hallanger et al., 1987; Cornwall et al., 1990). Our results demonstrate both cholinergic and non-cholinergic projections from the LDT to the MG in guinea pigs. The LDT projections are bilateral with an ipsilateral dominance, and involve fewer cells than the projections from the PPT. Additional experiments will be needed to determine whether the apparent differences in rats are due to the species or to differences in methods.
Another quantitative difference relates to the percentage of the PMT projection that is cholinergic. Previous reports have described the PMT projection to MG as up to 85% cholinergic in cats (Steriade et al., 1988), and PMT projections to the thalamus in general as 91% cholinergic (Sofroniew et al., 1985). However, our data in guinea pigs show only 44% of the projection is cholinergic (Table 2). It is possible that our immunostaining did not label every cholinergic cell. Another issue here is how one defines the borders of the PMT nuclei. Many of these borders, especially for the PPT, are difficult to identify. We use an operational definition based on the distribution of the presumptive cholinergic cells (Leonard et al., 1995). Small differences between species in the distribution of different cell types could lead to inclusion of more or fewer non-cholinergic cells within the PPT border. As mentioned above, there is growing evidence for important roles of the non-cholinergic projections from these areas (e.g., Boucetta and Jones, 2009). Future experiments designed to identify the neurotransmitters used by presumptive non-cholinergic cells will be necessary both to provide insight into functions and to clarify the relative contributions of different transmitters to the PMT projection.
It is likely that all MG subdivisions receive both cholinergic and non-cholinergic inputs from PMT. All subdivisions of the MG contain nicotinic and muscarinic receptors (Schwartz, 1986). However, there are reports that principle sensory nuclei can have a non-uniform distribution of ChAT immunopositive axons (e.g., Fitzpatrick et al., 1989). All of our injections, regardless of subdivisions involved, labeled cholinergic and non-cholinergic cells. We do not have sufficient numbers of cases with injections restricted to single subdivisions to make conclusions about the types of PMT cells (cholinergic or non-cholinergic) that contribute to the projection to each MG subdivision. Even with such data, this issue will benefit from examination of cholinergic axons in the MG.
The PMT projections to the thalamus have long been recognized as important for the generation of the REM component of sleep (Rye, 1997). While the cholinergic component has received the most attention, recently the non-cholinergic PPT and LDT contributions to the sleep-wake cycle have been considered (Boucetta and Jones, 2009). The authors suggested that the cholinergic, GABAergic, and glutamatergic cells act in concert to modulate sleep-wake cycles. These projections presumably control the flow of information through the thalamus in relation to the various stages of the sleep wake cycle as well as different levels of vigilance during waking. Our data show that cholinergic and non-cholinergic cells project to the MG, suggesting that multiple transmitters could modulate the MG during the sleep-wake cycle. This suggestion is consistent with physiological studies of MG activity during different phases of the sleep-wake cycle (Edeline, 2003; Hennevin et al., 2007).
It is possible that the PMT projections serve additional functions as well. Approximately half of the cells in both the PPT and LDT respond to sound (Reese et al., 1995a,b), suggesting a substantial auditory role for these nuclei. In addition to their projections to the MG, the PPT and LDT also project to the inferior colliculus (Motts and Schofield, 2009) and the cochlear nucleus (Motts and Schofield, 2005). The PPT and LDT are also the targets of descending projections from the auditory cortex (Schofield and Motts, 2009). The cortical projections appear to contact cholinergic PMT cells that project to the IC (Schofield, 2010), suggesting that the cortex can modify the level of cholinergic input to some subcortical auditory centers. Such projections might allow the cortex to modulate activity in subcortical centers according to the salience of a recent acoustic stimulus. In this regard, it would be interesting to determine whether the cortical projections also contact PMT cells (cholinergic or otherwise) that project to the MG.
Pontomesencephalic tegmentum cells are also involved in prepulse inhibition of startle via cholinergic projections to the caudal pontine nuclei (Koch et al., 1993). Prepulse inhibition has been viewed as a form of gating that allows the auditory system to continue processing acoustic information without the interruption that would be induced by a startle response. It will be of particular interest to know if the same PMT cells project to the caudal pontine nuclei and to the MG or another auditory nucleus. Such projections may serve to enhance processing (increase gain?) in the auditory nuclei while suppressing the motor response to an otherwise startling stimulus.
Conflict of Interest Statement
The authors declare that the research was conducted in the absence of any commercial or financial relationships that could be construed as a potential conflict of interest.
Acknowledgments
We gratefully acknowledge Colleen Sowick and Megan Storey-Workley for their expert technical assistance. We thank Dr. Jeffrey G. Mellott and Dr. Kyle Nakamoto for comments on an earlier draft. Supported by National Institutes of Health DC04391 and DC08463.
References
Anderson, L. A, Wallace, M. N, and Palmer, A. R. (2007). Identification of subdivisions in the medial geniculate body of the guinea pig. Hear. Res. 228, 156–167.
Armstrong, D. M., Saper, C. B., Levey, A. I., Wainer, B. H., and Terry, R. D. (1983). Distribution of cholinergic neurons in rat brain: demonstrated by the immunocytochemical localization of choline acetyltransferase. J. Comp. Neurol. 216, 53–68.
Boucetta, S., and Jones, B. E. (2009). Activity profiles of cholinergic and intermingled GABAergic and putative glutamatergic neurons in the pontomesencephalic tegmentum of urethane-anesthetized rats. J. Neurosci. 29, 4664–4674.
Cornwall, J., Cooper, J. D., and Phillipson, O. T. (1990). Afferent and efferent connections of the laterodorsal tegmental nucleus in the rat. Brain Res. Bull. 25, 271–284.
Edeline, J. M. (2003). The thalamo-cortical auditory receptive fields: regulation by the states of vigilance, learning and the neuromodulatory systems. Exp. Brain Res. 153, 554–572.
Fitzpatrick, D., Diamond, I. T., and Raczkowski, D. (1989). Cholinergic and monoaminergic innervation of the cat’s thalamus: comparison of the lateral geniculate nucleus with other principal sensory nuclei. J. Comp. Neurol. 288, 647–675.
Ford, B., Holmes, C. J., Mainville, L., and Jones, B. E. (1995). GABAergic neurons in the rat pontomesencephalic tegmentum: codistribution with cholinergic and other tegmental neurons projecting to the posterior lateral hypothalamus. J. Comp. Neurol. 363, 177–196.
German, D. C., Bruce, G., and Hersh, L. B. (1985). Immunohistochemical staining of cholinergic neurons in the human brain using a polyclonal antibody to human choline acetyltransferase. Neurosci. Lett. 61, 1–5.
Hallanger, A. E., Levey, A. I., Lee, H. J., Rye, D. B., and Wainer, B. H. (1987). The origins of cholinergic and other subcortical afferents to the thalamus in the rat. J. Comp. Neurol. 262, 105–124.
Hennevin, E., Huetz, C., and Edeline, J. M. (2007). Neural representations during sleep: from sensory processing to memory traces. Neurobiol. Learn. Mem. 87, 416–440.
Jia, H. G., Yamuy, J., Sampogna, S., Morales, F. R., and Chase, M. H. (2003). Colocalization of gamma-aminobutyric acid and acetylcholine in neurons in the laterodorsal and pedunculopontine tegmental nuclei in the cat: a light and electron microscopic study. Brain Res. 992, 205–219.
Jones, B. E. (2005). From waking to sleeping: neuronal and chemical substrates. Trends Pharmacol. Sci. 26, 578–586.
Katz, L. C., and Iarovici, D. M. (1990). Green fluorescent latex microspheres: a new retrograde tracer. Neuroscience 34, 511–520.
Koch, M., Kungel, M., and Herbert, H. (1993). Cholinergic neurons in the pedunculopontine tegmental nucleus are involved in the mediation of prepulse inhibition of the acoustic startle response in the rat. Exp. Brain Res. 97, 71–82.
Lavoie, B., and Parent, A. (1994). Pedunculopontine nucleus in the squirrel monkey: distribution of cholinergic and monoaminergic neurons in the mesopontine tegmentum with evidence for the presence of glutamate in cholinergic neurons. J. Comp. Neurol. 344, 190–209.
Leonard, C. S., Kerman, I., Blaha, G., Taveras, E., and Taylor, B. (1995). Interdigitation of nitric oxide synthase-, tyrosine hydroxylase-, and serotonin-containing neurons in and around the laterodorsal and pedunculopontine tegmental nuclei of the guinea pig. J. Comp. Neurol. 362, 411–432.
Levey, A. I., and Wainer, B. H. (1982). Cross-species and intraspecies reactivities of monoclonal antibodies against choline acetyltransferase. Brain Res. 234, 469–473.
Maley, B. E., Frick, M. L., Levey, A. I., Wainer, B. H., and Elde, R. P. (1988). Immunohistochemistry of choline acetyltransferase in the guinea pig brain. Neurosci. Lett. 84, 137–142.
McCormick, D. A., and Prince, D. A. (1987). Actions of acetylcholine in the guinea-pig and cat medial and lateral geniculate nuclei, in vitro. J. Physiol. 392, 147–165.
Mesulam, M. M., Mufson, E. J, Wainer, B. H., and Levey, A. I. (1983). Central cholinergic pathways in the rat: an overview based on an alternative nomenclature (Ch1-Ch6). Neuroscience 10, 1185–1201.
Motts, S. D., and Schofield, B. R. (2005). Olivary and extra-olivary sources of cholinergic input to the cochlear nucleus. Assoc. Res. Otolaryngol. Abs. 27, 242.
Motts, S. D., and Schofield, B. R. (2009). Sources of cholinergic input to the inferior colliculus. Neuroscience 160, 103–114.
Motts, S. D., Slusarczyk, A. S., Sowick, C. S., and Schofield, B. R. (2008). Distribution of cholinergic cells in guinea pig brainstem. Neuroscience 154, 186–195.
Oakman, S. A., Faris, P. L., Cozzari, C., and Hartman, B. K. (1999). Characterization of the extent of pontomesencephalic cholinergic neurons’ projections to the thalamus: comparison with projections to midbrain dopaminergic groups. Neuroscience 94, 529–547.
Reese, N. B., Garcia-Rill, E., and Skinner, R. D. (1995a). Auditory input to the pedunculopontine nucleus: I. Evoked potentials. Brain Res. Bull. 37, 257–264.
Reese, N. B., Garcia-Rill, E., and Skinner, R. D. (1995b). Auditory input to the pedunculopontine nucleus: II. Unit responses. Brain Res. Bull. 37, 265–273.
Rye, D. B. (1997). Contributions of the pedunculopontine region to normal and altered REM sleep. Sleep 20, 757–788.
Satoh, K., and Fibiger, H. C. (1986). Cholinergic neurons of the laterodorsal tegmental nucleus: efferent and afferent connections. J. Comp. Neurol. 253, 277–302.
Schofield, B. R. (2010). Projections from auditory cortex to midbrain cholinergic neurons that project to the inferior colliculus. Neuroscience 166, 231–240.
Schofield, B. R., and Motts, S. D. (2009). Projections from auditory cortex to cholinergic cells in the midbrain tegmentum of guinea pigs. Brain Res. Bull. 80, 163–170.
Schwartz, R. D. (1986). Autoradiographic distribution of high affinity muscarinic and nicotinic cholinergic receptors labeled with [3H]acetylcholine in rat brain. Life Sci. 38, 2111–2119.
Shute, C. C., and Lewis, P. R. (1967). The ascending cholinergic reticular system: neocortical, olfactory and subcortical projections. Brain 90, 497–520.
Sofroniew, M. V., Priestley, J. V., Consolazione, A., Eckenstein, F., and Cuello, A. C. (1985). Cholinergic projections from the midbrain and pons to the thalamus in the rat, identified by combined retrograde tracing and choline acetyltransferase immunohistochemistry. Brain Res. 329, 213–223.
Steriade, M. (2004). Acetylcholine systems and rhythmic activities during the waking-sleep cycle. Prog. Brain Res. 145, 179–196.
Steriade, M., Paré, D., Parent, A., and Smith, Y. (1988). Projections of cholinergic and non-cholinergic neurons of the brainstem core to relay and associational thalamic nuclei in the cat and macaque monkey. Neuroscience 25, 47–67.
Tebecis, A. K. (1972). Cholinergic and non-cholinergic transmission in the medial geniculate nucleus of the cat. J. Physiol. 226, 153–172.
Vincent, S. R., Satoh, K., and Fibiger, H. C. (1986). The localization of central cholinergic neurons. Prog. Neuropsychopharmacol. Biol. Psychiatry 10, 637–656.
Keywords: acetylcholine, arousal, auditory, GABA, glutamate, thalamus, sleep
Citation: Motts SD and Schofield BR (2010) Cholinergic and non-cholinergic projections from the pedunculopontine and laterodorsal tegmental nuclei to the medial geniculate body in guinea pigs. Front. Neuroanat. 4:137. doi: 10.3389/fnana.2010.00137
Received: 12 March 2010;
Accepted: 19 September 2010;
Published online: 19 October 2010
Edited by:
Enrique Saldaña, Universidad de Salamanca, SpainReviewed by:
Dolores E. López García, Universidad de Salamanca, SpainMarisela Morales, National Institutes of Health, USA
Copyright: © 2010 Motts and Schofield. This is an open-access article subject to an exclusive license agreement between the authors and the Frontiers Research Foundation, which permits unrestricted use, distribution, and reproduction in any medium, provided the original authors and source are credited.
*Correspondence: Brett R. Schofield, Department of Anatomy and Neurobiology, Northeastern Ohio Universities Colleges of Medicine and Pharmacy, PO Box 95, Rootstown, OH 44272, USA. e-mail: bschofie@neoucom.edu