- Institut de Génétique et Développement de Rennes, Faculté de Médecine, CNRS UMR6290, Université de Rennes 1, Rennes, France
The vertebrate embryonic prosencephalon gives rise to the hypothalamus, which plays essential roles in sensory information processing as well as control of physiological homeostasis and behavior. While patterning of the hypothalamus has received much attention, initial neurogenesis in the developing hypothalamus has mostly been neglected. The first differentiating progenitor cells of the hypothalamus will give rise to neurons that form the nucleus of the tract of the postoptic commissure (nTPOC) and the nucleus of the mammillotegmental tract (nMTT). The formation of these neuronal populations has to be highly controlled both spatially and temporally as these tracts will form part of the ventral longitudinal tract (VLT) and act as a scaffold for later, follower axons. This review will cumulate and summarize the existing data available describing initial neurogenesis in the vertebrate hypothalamus. It is well-known that the Notch signaling pathway through the inhibition of proneural genes is a key regulator of neurogenesis in the vertebrate central nervous system. It has only recently been proposed that loss of Notch signaling in the developing chick embryo causes an increase in the number of neurons in the hypothalamus, highlighting an early function of the Notch pathway during hypothalamus formation. Further analysis in the chick and mouse hypothalamus confirms the expression of Notch components and Ascl1 before the appearance of the first differentiated neurons. Many newly identified proneural target genes were also found to be expressed during neuronal differentiation in the hypothalamus. Given the critical role that hypothalamic neural circuitry plays in maintaining homeostasis, it is particularly important to establish the targets downstream of this Notch/proneural network.
Introduction
The hypothalamus is an evolutionary ancient structure in the rostral brain that plays a central role in the regulation of physiological processes such as hunger, thermoregulation, reproduction and behavior in adult vertebrates. The adult hypothalamus is subdivided into regions, each containing well documented clusters of neurons with defined functions (Simerly, 2004). Countless work involving physiological and genetic studies has focused on signaling molecules and transcription factors that control hypothalamus morphogenesis and the emergence of different neuronal subtypes (Shimogori et al., 2010). However, relatively little attention has been paid to the process through which the initial neurons are induced and specified in the primordium of the vertebrate hypothalamus, despite their key roles in pioneering the major axon pathways in the forebrain (Wilson et al., 1990; Mastick and Easter, 1996; Ware and Schubert, 2011). The first differentiating cells of the hypothalamus will give rise to neurons that form the nucleus of the tract of the postoptic commissure (nTPOC) and the nucleus of the mammillotegmental tract (nMTT). Recent advances in the chick model has established that a Notch/proneural regulatory loop is implicated very early during the differentiation of these neurons (Ratié et al., 2013). The aim of this review is to highlight a role for Notch signaling during nTPOC and nMTT differentiation; including key findings from zebrafish, chick and mouse models, which has contributed to our understanding of this field. A potential cascade involving Ascl1 and target genes will be discussed to determine the possible regulation of these initial hypothalamic neurons.
Patterning of the Vertebrate Hypothalamic Primordium
During early embryogenesis the hypothalamus develops within the secondary prosencephalon (Puelles and Rubenstein, 2003; Martinez-Ferre and Martinez, 2012; Puelles et al., 2012). Developmental studies performed in zebrafish, chick and mouse indicate Sonic Hedgehog (SHH), secreted by the underlying prechordal plate mesendoderm, induces the formation of the hypothalamus (Dale et al., 1997; Mathieu et al., 2002; Aoto et al., 2009). Loss of Shh leads to missing ventral structures including the hypothalamus in zebrafish (Varga et al., 2001) and mouse (Chiang et al., 1996). In humans, mutations in the Shh gene results in holoprosencephaly, the most frequent human brain malformation that includes hypothalamic defects (Mercier et al., 2011). However, SHH alone is not sufficient to induce specific hypothalamus identity. The prechordal plate expresses numerous other secreted proteins that are involved in the development of the overlying hypothalamus primordium including Wnt antagonists, NODAL and Bone Morophogenic Proteins (BMP; Pera and Kessel, 1997; Kiecker and Niehrs, 2001; Mathieu et al., 2002; Manning et al., 2006; Cavodeassi and Houart, 2012).
Specific patterning of the hypothalamus begins when the hypothalamic primordium expresses the transcription factor Nkx2.1 from Hamburger and Hamilton stage (HH)8 in chick and embryonic day (E)8 in mouse (Shimamura et al., 1995; Pera and Kessel, 1998; Sussel et al., 1999; Crossley et al., 2001). This expression of Nkx2.1, along with Nkx2.2 is dependent on the presence of Shh in the prechordal plate (Barth and Wilson, 1995; Pera and Kessel, 1997; Rohr et al., 2001; Mathieu et al., 2002). SHH is then required to coordinate tissue growth and acquisition of anteroposterior (AP), dorsoventral (DV) and mediolateral patterning of the hypothalamus (Manning et al., 2006; Szabó et al., 2009).
At HH10, Shh, Nkx2.1 and Nkx2.2 expression expands in the basal plate of the chick prosencephalon, with the same rostral expression at the level of the presumptive anterior hypothalamus (AH) that corresponds to the prospective chiasmatic area (also called suboptical domain) (Crossley et al., 2001). A new Nkx2.1 expression domain develops at HH12, just rostral to the hypothalamus in the basal telencephalon called the postoptic area (POA). In zebrafish and mouse, the same dynamic expression patterns of Shh, Nkx2.1 and Nkx2.2 is present within the hypothalamus (Figure 1). By HH13 in chick and E9.5 in the mouse, Shh and Nkx2.1 expression has expanded further and the hypothalamic primordium is morphologically evident. Studies in chick show that once the hypothalamic primordium is established, SHH down-regulation mediated by local production of BMPs is necessary for establishing region-specific transcriptional profiles (Patten and Placzek, 2002; Manning et al., 2006; Ohyama et al., 2008). This leads to the subdivisions of the primordial hypothalamus into three regions, the AH, the tuberal hypothalamus (TH) and the mammillary hypothalamus (MH), with each region expressing specific markers (Figure 1; Alvarez-Bolado et al., 2012; Wolf and Ryu, 2013).
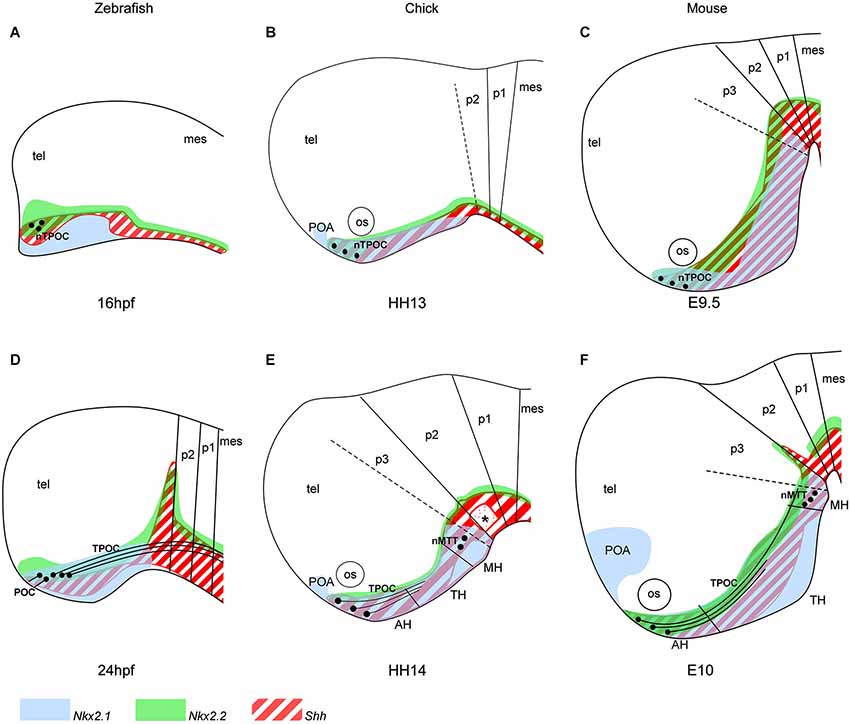
Figure 1. Organization of the hypothalamic primordium in the rostral vertebrate brain. (A–C) The first nTPOC neurons arise in the hypothalamus in zebrafish (A) at 16 hpf, chick (B) at HH13 and mouse (C) at E9.5. (D) Axons project caudally from the nTPOC forming the TPOC and rostrally from the nTPOC to form the POC in zebrafish at 24 hpf. (E, F) The nTPOC neurons begin projecting axons and the first nMTT neurons arise in chick at HH14 (E) and in mouse (F) at E10. The hypothalamus is specifically marked by three genes, Nkx2.1 (light blue), Nkx2.2 (green) and Shh (red stripes). (A, D) Gene expression in zebrafish is based on the following studies: Shh, Nkx2.2 (Barth and Wilson, 1995; Hjorth and Key, 2001) and Nkx2.1a (Rohr and Concha, 2000; Rohr et al., 2001). (B, E) Gene expression in chick is based on the following studies: Shh (Bardet et al., 2010), Nkx2.1 (Ratié et al., 2013) and Nkx2.2 (Gimeno and Martinez, 2007). (C, F) Gene expression in mouse is based on the following studies: Shh (Shimamura et al., 1995; Alvarez-Bolado et al., 2012), Nkx2.1 and Nkx2.2 (Shimamura et al., 1995). (E, F) The three subdivisions of the hypothalamus (AH, TH and MH) in chick and mouse is based on Shh expression (Alvarez-Bolado et al., 2012; Ratié et al., 2013). (E) Asterisk, Shh negative region, overlapping where the ventral MLF neurons differentiate. For all schematics, subdivisions of the brain is based on the prosomeric model (Mastick and Easter, 1996; Hauptmann et al., 2002; Puelles and Rubenstein, 2003; Ware and Schubert, 2011). (D–F) Zona limitans intrathalamica (ZLI) marks the p2/p3 boundary. Although other neuronal populations are present in the brain at these stages, they are not added to focus on the hypothalamic neurons. AH, anterior hypothalamus; mes, mesencephalon; MH, mammillary hypothalamus; nMTT, nucleus of the tract of the mammillotegmental tract; nTPOC, nucleus of the tract of the postoptic commissure; os, optic stalk; POA, postoptic area; POC, postoptic commissure; p1–p3, prosomeres 1–3; TPOC, tract of the postoptic commissure; TH, tuberal hypothalamus; tel, telencephalon.
Initial Neurogenesis in the Vertebrate Hypothalamus
The first neurons that differentiate in the vertebrate brain give rise to the highly conserved early axon scaffold (Chitnis and Kuwada, 1990; Wilson et al., 1990; Easter et al., 1993; Mastick and Easter, 1996; Barreiro-Iglesias et al., 2008; Ware and Schubert, 2011; Ware et al., 2014). This is an important structure for the guidance of later, follower axons allowing more complex connections to form. Predating the mature hypothalamic neuronal clusters, two small GABAergic positive populations differentiate within the hypothalamus (Figure 1; Patel et al., 1994). The first neurons in the hypothalamic primordium differentiate to give rise to the nTPOC (also termed the ventro-rostral cluster (vrc) in anamniotes) at 16 hpf in zebrafish (Figure 1A; Chitnis and Kuwada, 1990; Ross et al., 1992) and HH13 in chick (Figure 1B; Ware and Schubert, 2011). An early birth-dating study has shown that hypothalamic neurogenesis in the mouse begins at E10 (Shimada and Nakamura, 1973). However, it is well-known that the initial nTPOC neurons arise at E9.5, suggesting neurogenesis begins earlier than previously thought (Figure 1C; Easter et al., 1993; Mastick and Easter, 1996; Ricaño-Cornejo et al., 2011). From the nTPOC neurons, axons extend and project caudally within the basal plate. The tract of the postoptic commissure (TPOC) axons project into the mesencephalon where these axons form part of the ventral longitudinal tract (VLT) along with the medial longitudinal fascicle (MLF) and later the mammillotegmental tract (MTT; Ware and Schubert, 2011). The MTT forms from a second set of neurons (nMTT) that differentiate later in the caudal hypothalamus of amniotes from HH14 in chick and E10 in mouse (Figures 1E,F; Puelles et al., 1987; Easter et al., 1993; Mastick and Easter, 1996). While the presence and location of the nTPOC is conserved in all vertebrates studied, the nMTT is not present in zebrafish, at least during early development (Barreiro-Iglesias et al., 2008). Neurons do however form later in the zebrafish MH, but it is not possible to comment on the homology with the nMTT (Wolf and Ryu, 2013). The postoptic commissure (POC) forms by 24 hpf, projecting axons from the nTPOC rostrally to form a commissure across the rostral midline connecting the left and right sides of the neural tube (Figure 1D; Ross et al., 1992; Bak and Fraser, 2003). The POC is likely to form in chick and mouse at later stages but has not been studied exhaustively (Croizier et al., 2011; Ware and Schubert, 2011).
The prosomeric model and hypothalamic markers such as Shh, Nkx2.1 and Nkx2.2 confirms the nTPOC and nMTT neurons form within the hypothalamus (Figure 1; Hjorth and Key, 2001; Puelles and Rubenstein, 2003). The nTPOC arises just below the optic stalk at the midline of the AH area and the nMTT in the lateral edge of the caudal hypothalamus in the MH (Figure 1; Easter et al., 1993). The nTPOC neurons differentiate along the boundaries of many gene expression areas in zebrafish (Macdonald et al., 1994), however the mechanism by which these neurons differentiate has been overlooked.
Some zebrafish and mouse mutants are available where the formation of these hypothalamic axon tracts is affected. Some genes are implicated in the differentiation of the neurons such as Six3 (Ando et al., 2005), but many studies focus on the effect of gene inactivation on axon guidance, including Fgf8 (Shanmugalingam et al., 2000), Pax6 (Mastick et al., 1997; Nural and Mastick, 2004), Slits and Robos (Ricaño-Cornejo et al., 2011) and Sim1/Sim2 (Marion et al., 2005). Functionally, the TPOC is important for the guidance of other axon tracts. Ablation of the TPOC axons in the zebrafish embryo affects the patterning of the early axon scaffold (Chitnis and Kuwada, 1991). In zebrafish Cyclops mutants, the TPOC does not form, leading to the misguidance of the tract of the posterior commissure (TPC) axons (Patel et al., 1994). More recently, a study in the embryonic mouse has shown later hypothalamic axons from the melanin-concentrating hormone (MCH) neurons use the TPOC for guidance (Croizier et al., 2011). While the potential function of the TPOC neurons is not known, lypophilic tracing shows that the TPOC axons project into the hindbrain, although the target of these axons remains a mystery (Ware and Schubert, 2011). It is also unclear whether these neurons are still present postnatally, it could be that their sole purpose is to provide axons for guidance and then simply die after connections are made in the adult brain (Easter et al., 1993). The MTT may also function in the guidance of other tracts but this has not been studied exhaustively. In mouse, the MTT is likely to guide the mammillothalamic tract (MTH) that forms later in mouse contributing to the principle mammillary tract (Marion et al., 2005). The MTH is not known to form in zebrafish or chick. The MTT axons project to the tegmentum and are described as having a role in visceral function and processing special information in the adult human brain (Alpeeva and Makarenko, 2007; Kwon et al., 2011).
No attention has been brought to the mechanism by which the nTPOC and nMTT neurons differentiate, until 2013, when Notch components were first described as being present very early in the hypothalamus of the developing chick embryo (Ratié et al., 2013). A basic PubMed search of the key words Notch and hypothalamus generated very few publications and many of which are based in adult models or describe differentiation of late forming embryonic neurons (Chapouton et al., 2011; Aujla et al., 2013). This indicates a surprising lack of investigations surrounding neurogenesis of the initial hypothalamus neurons, when considering these early neurons have been described through-out the 1990s in different vertebrate species. As these neurons contribute to the early axon scaffold and are essential for the set-up of more complex connections, it is surely essential to understand how they differentiate and how they are specified. Finally, considering Notch along with the proneural network is a well-known signaling pathway, little is known about the implication of Notch signaling or neurogenic factors involved in the formation of the nTPOC and nMTT neurons.
Neurogenesis and the Notch/Proneural Network
Notch signaling is an evolutionary conserved signaling pathway involved in cell-cell communication regulating multiple processes throughout development. The Notch signaling pathway has previously been reviewed in detail, here a brief outline is described (Pierfelice et al., 2011). First identified in Drosophila, the Notch pathway has been confirmed to have similar roles in vertebrates (Coffman et al., 1990; Artavanis-Tsakonas and Simpson, 1991; Artavanis-Tsakonas et al., 1999). The core pathway consists of the interaction between a transmembrane Notch receptor anchored in one cell, with a transmembrane Notch ligand (Delta or Serrate/Jagged) in a neighboring cell. Upon receptor-ligand binding a series of proteolytic cleavages are triggered that releases the intracellular domain of Notch (NICD), which forms a nuclear complex with recombination signal binding protein for immunoglobulin kappa J region (RBPJ). This complex activates the transcription of target genes (Tamura et al., 1995; Fortini, 2009). The best characterized direct targets of the NICD/RBPJ complex are the Hes (Hairy-Enhancer of Split) and Hey (Hes related type) genes (Jarriault et al., 1995; Maier and Gessler, 2000). They are class-C basic helix-loop-helix (bHLH) proteins that function as transcriptional repressors and can function together as homodimers or heterodimers (Iso et al., 2003).
One function of Notch relies on lateral induction, which is defined as the process by which a ligand-expressing cell stimulates those cells nearby to upregulate ligand expression, promoting ligand propagation and coordinated cell behavior (Eddison et al., 2000). The other function of Notch is lateral inhibition, whereby a ligand-expressing cell inhibits the expression of the ligand in the neighboring cells, therefore preventing those cells from adopting the same fate and generating a patched cellular pattern (Bray, 2006). It is associated with salt-and-pepper like patterns of gene expression (Fior and Henrique, 2009). For example, these two modes of Notch pathway operation coexist during inner ear development. Each mode relies on an associated gene regulatory network (Kiernan, 2013; Neves et al., 2013). Expression and functional studies suggest that lateral induction and lateral inhibition are associated with different Notch ligands that initiate signaling (Brooker et al., 2006; Saravanamuthu et al., 2009; Petrovic et al., 2014). The association of DLL1 with lateral inhibition is a general theme during neural development (Henrique et al., 1995; Adam et al., 1998; Kageyama et al., 2010).
Notch signaling has a very well-known role in neurogenesis, controlling the balance between proliferation of neural progenitor cells (NPCs) and differentiation of NPCs into neuronal and glial cells (Campos-Ortega, 1993; Chitnis et al., 1995; de La Pompa et al., 1997; reviewed by Paridaen and Huttner, 2014). In the neuroepithelium, neuron production is mostly controlled by lateral inhibition, where a regulatory loop is formed, with proneural genes controlling the expression of Notch ligands (Bertrand et al., 2002). The ligand, DLL1, can bind and active NOTCH in neighboring cells. When the Notch signaling pathway is activated, transcriptional repressors (such as Hes or Hey genes) are expressed that prevent expression of proneural genes, inhibiting differentiation and therefore cells remain as progenitors. Cells expressing the ligand and therefore lacking Notch signaling can no longer express transcriptional repressors, leading to the upregulation of bHLH proneural transcription factors such as Ascl1 or Neurog1/2. Under this Notch/proneural network the cell can exit the cell cycle and undergoes neural differentiation (Bertrand et al., 2002). This differentiation step is controlled by several classes of transcription factors that determine the identity of the neuron produced. Among them, a number of bHLH differentiation genes are switched on, such as Nhlh1 or NeuroD4, followed by specific neuronal genes.
Notch Signaling in the Vertebrate Hypothalamus Primordium
There are numerous studies investigating the expression and function of Notch components, proneural genes and downstream targets. However, as mentioned previously there is very little data describing the role of Notch signaling during the differentiation of the nTPOC and nMTT neurons. When Notch signaling is inhibited in the developing chick embryo, the number of nTPOC neurons increases, along with ectopic expression of many genes within the hypothalamus, confirming Notch has a role during hypothalamic neurogenesis at this early stage (Ratié et al., 2013). This study describes a typical neurogenic phenotype expected for the loss of Notch function, working by lateral inhibition.
For the first time, the Notch components Dll1, Hes5 and Hey1 are shown to be expressed just before HH11 in the presumptive AH of the chick embryonic brain where the first nTPOC neurons will differentiate at HH13 (Ware and Schubert, 2011; Ratié et al., 2013). Expression of Notch components during initial neurogenesis in the zebrafish and mouse has been extensively studied, however for much of the data, it is difficult to interpret the expression in the hypothalamic primordium as no special attention was given to this area at early stages. The expression of Notch receptors in zebrafish are first described at 16 hpf in the prosencephalon and appear to overlap in the area where the nTPOC forms (Bierkamp and Campos-Ortega, 1993; Dyer et al., 2014). In mouse, while Notch3 is ubiquitously expressed in the neuroectoderm from E8.0, Notch2 and Hes1 are expressed in the ventral prosencephalon from E8.5 and Notch1 is expressed from E9.5 (Reaume et al., 1992; Williams et al., 1995; Koop et al., 1996). Remarkably, little information is present in the literature about when these genes are first expressed in the developing hypothalamus (Bettenhausen et al., 1995; de La Pompa et al., 1997; Leimeister et al., 1999; Barsi et al., 2005). Therefore, in this review, expression of Notch components are analyzed using in situ hybridization data to deal with this deficiency (Figure 2). Dll1, Hes5 and Hey1 mRNA probes are used to show the presence of Notch activity, focusing more specifically in the hypothalamus. At E8.0, Dll1, Hes5 and Hey1 are not expressed in the mouse presumptive hypothalamus (Figures 2A–C), it is only from E8.5, before the initial neurons differentiate that Dll1 and Hes5 expression is first observed (Figures 2E,E’,F,F’, arrowheads). Flat-mounted preparations of the ventral midline reveal a salt-and-pepper like pattern for these genes in the rostral hypothalamus (Figures 2E’,F’). Expression continues in the AH at E9 for Dll1 and Hes5 (Figures 2I,J, arrowhead), while Hey1 expression first starts to be expressed in the same region (Figure 2K, arrowhead). At E9.5, Dll1, Hes5 and Hey1 are first expressed in the MH where the nMTT neurons will differentiate at E10 (Figures 2M–O, unfilled arrowhead). It is important to note that the genes analyzed here are not specific for either the nTPOC or nMTT, but are also expressed by other early developing neurons such as those in the nucleus of the mesencephalic tract of the trigeminal nerve (nmesV; Figure 2). This is not surprising as Notch is a very general pathway involved in neuron progenitor expansion (Kageyama et al., 2009). Flat-mounted preparations performed at E9.5 confirm the localized expression of Hes5 (Figure 2N) and Hey1 (Figure 2O) in the AH.
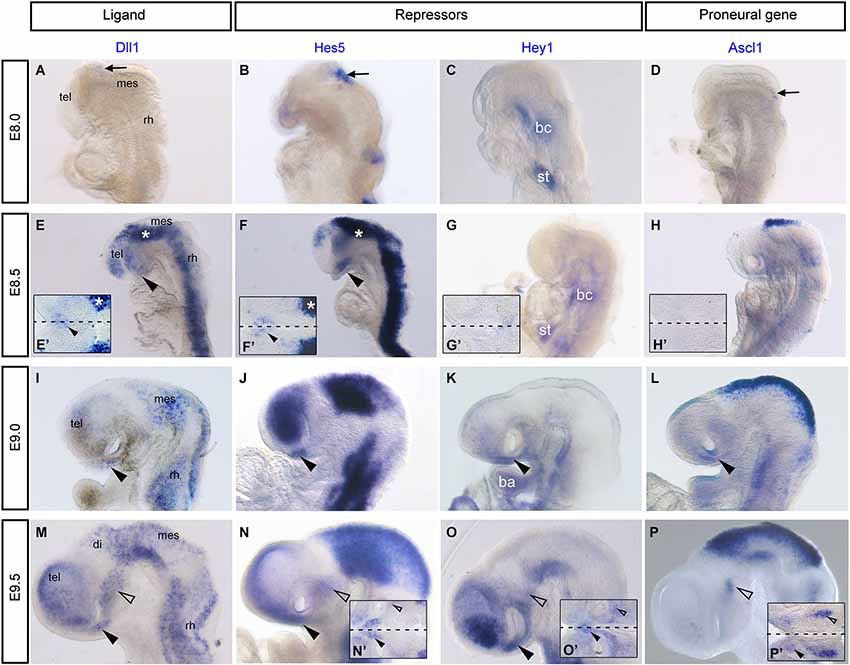
Figure 2. Notch components Dll1, Hes5, Hey1 and the proneural gene, Ascl1 are expressed in the developing mouse hypothalamus. Whole mount in situ hybridization was performed on mouse embryos as previously described (Chapman et al., 2002). Digoxigenin labeled mRNA probes were made from the following plasmids Dll1, Hes5, Hey1 and Ascl1 (Guillemot and Joyner, 1993). (A–D) E8.0, no expression of Dll1 (A), Hes5 (B), Hey1 (C) and Ascl1 (D) in the ventral prosencephalon. Arrows indicate dorsal expression that corresponds to NPCs and early differentiating descending tract of the mesencephalic nucleus of the trigeminal nerve (DTmesV) neurons. (E–H) E8.5, expression of Dll1 (E) and Hes5 (F) throughout the neural tube and in the AH (arrowhead). Asterisks in (E) and (F) correspond to dorsal staining in flat-mounted preparations in (E’) and (F’). Expression of Hey1 (G) and Ascl1 (H) is not yet present in the AH. (E’–H’) Flat-mounted preparation of the hypothalamus in the same embryo at E8.5. Expression of Dll1 (E’) and Hes5 (F’) in the AH (arrowhead). Dashed line represents ventral midline. (I–L) E9.0, expression of Dll1 (I), Hes5 (J) in the AH (arrowhead). Expression of Hey1 (K) and Ascl1 (L) begin in the AH (arrowhead). (C, G, K) Expression of Hey1 in the branchial cleft (bc), septum transversum (st) and branchial arch (ba) (Leimeister et al., 1999). (M-P) E9.5, expression of Dll1 (M), Hes5 (N), Hey1 (O) and Ascl1 (P) in the AH (arrowhead) and in the MH (unfilled arrowhead). (M’–P’) Flat-mounted preparations of the hypothalamus at E9.5, arrowheads indicate expression in the AH and unfilled arrowheads indicate expression in the MH. Dashed lines represent ventral midline. mes, mesencephalon; tel, telencephalon; rh, rhombencephalon.
Bringing together the data from the literature and in situ hybridization of mouse embryos presented here, this highlights that Notch signaling is active very early in the AH and MH where the nTPOC and nMTT neurons will develop respectively (Mastick and Easter, 1996; Ratié et al., 2013). The data also suggests that redundancy could be strong between the direct Notch target genes as multiple transcriptional repressors such as Hes1, Hes5 and Hey1 are expressed in the developing hypothalamus.
Like with the expression studies described in this section, no functional data about neurogenesis in the early hypothalamus is available in zebrafish and mouse. There are several models lacking Notch signaling, which exhibit an increase in neurons throughout the embryo (de La Pompa et al., 1997; Itoh et al., 2003). For example, in the zebrafish and mouse mindbomb/Mib1 mutants, Dll1 ubiquitination is affected and aberrant neurogenesis due to lower expression of Hes1 and Hes5 is observed throughout the embryo (Itoh et al., 2003; Barsi et al., 2005; Koo et al., 2005). A similar phenotype is also present in RBPj mutant mice, where Notch activity is absent (Oka et al., 1995; de La Pompa et al., 1997). As all these mutant mice display early lethality, no description is available to indicate whether neurogenesis is disturbed in the hypothalamus. Conditional loss-of-function mice lacking RBPJ, using Nkx2.1-Cre to specifically knock-out Notch signaling in the hypothalamus, shows that Notch signaling is essential for the differentiation of late arcuate hypothalamic neurons in the mouse from E13.5 (Aujla et al., 2013). This study did not identify a role in the initial neurons, but we would assume there would be an increase in the number of nTPOC and nMTT neurons in these mutant mice.
Many other knock-out or ectopic expression studies of Notch components describe an effect on neurogenesis throughout the embryo. The Dll1 mutant mouse has not been well studied for a neurogenesis phenotype (Hrabe de Angelis et al., 1997; Przemeck et al., 2003). However, Dll1 does regulate primary neurogenesis in the Xenopus embryo (Chitnis et al., 1995).
There appears to be much redundancy between genes of the Notch pathway, which could explain why a function for Notch during nTPOC neuronal differentiation has not been described before in the mouse. For example, Hes5 does not show any phenotype in single mutants (Cau et al., 2000). Double or triple knock-out mice produce more obvious phenotypes and prove redundancy occurs between these genes (Hatakeyama et al., 2004; Kageyama et al., 2008a). The absence of both Hes1 and Hes5 leads to aberrant neuronal localization. Interestingly, expression of Dll1 and Ascl1 is highly upregulated in the ventral diencephalon of E9.5 Hes1/Hes5 double mutants as are the number of βIII-tubulin (Tuj1) positive cells (Hatakeyama et al., 2004). The capacity of these bHLH proteins to do the same job, may also explain why there is discrepancy between their expressions in chick compared with mouse. For example, flat-mounted preparations of chick embryos at HH15 (Figure 3A, arrowhead) and HH14 (Ratié et al., 2013) confirm specific expression of Hey1 in the AH, whereas expression is throughout the developing hypothalamus in the mouse (Figures 2O,O’).
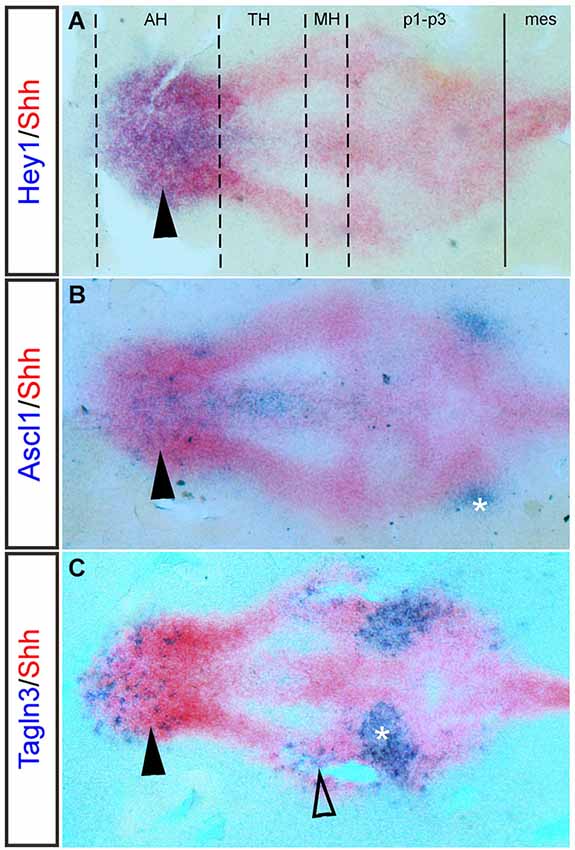
Figure 3. Hey1, Ascl1 and Tagln3 expression overlaps with Shh in the chick hypothalamus primordium. Double labeling of Hey1, Ascl1 and Tagln3 (Purple, Digoxigenin labeled probes) at HH15 with the dynamic hypothalamic marker, Shh (Red, Fluorescein labeled mRNA probe) in chick confirms expression of these genes in the hypothalamic domains. (A) Hey1 expression is specifically expressed in the anterior hypothalamus (AH) (arrowhead), overlapping the area where the nTPOC neurons will differentiate. Dashed lines indicate the boundaries of the hypothalamic domains, while the solid line marks the diencephalic-mesencephalic boundary (DMB). (B) Ascl1 expression is located in the AH (arrowhead). Asterisk labels the nTPOC located in p1 (Ware and Schubert, 2011). (C) Tagln3 expression is located in the AH (arrowhead) and in the mammillary hypothalamus (MH), overlapping where the nMTT neurons differentiate (unfilled arrowhead). Asterisk labels the ventral medial longitudinal fascicle (nMLF) located in p2 (Ware and Schubert, 2011). mes, mesencephalon; p1-p3, prosomeres 1–3; TH; tuberal hypothalamus.
Proneural Gene Expression in the Vertebrate Hypothalamus Primordium
Induction of the Notch/proneural loop is essential in the developing hypothalamus as this will eventually lead to the correct number of cells differentiating into nTPOC and nMTT neurons as well as maintaining the progenitor population.
Ascl1 is a well-studied proneural bHLH transcription factor, its expression and function during early embryogenesis has been well described in many vertebrates (Johnson et al., 1990; Ferreiro et al., 1993; Guillemot and Joyner, 1993; Jasoni et al., 1994; Mcnay et al., 2006). In zebrafish, Ascl1 expression appears early at 12 hpf in cells prior to the appearance of markers indicative of overt differentiation, by 16 hpf, Ascl1 expression overlaps with the nTPOC (Allende and Weinberg, 1994; Ando et al., 2005). In the Notch inhibited chick model, embryos display an upregulation of Ascl1 expression in the AH, overlapping the nTPOC (Ratié et al., 2013). Flat-mounted preparations of HH15 chick hypothalamus show that Ascl1 expression overlaps with Shh expression in the AH (Figure 3B, arrowhead). The expression of Ascl1 in the hypothalamus is examined further by in situ hybridization between E8 and E9.5 in the mouse embryo, like with the Notch components, expression of Ascl1 has been badly interpreted in this region (Figures 2D,H,L,P). Ascl1 starts to be expressed in the developing hypothalamus at E9.0 (Figure 2L, arrowhead). At E9.5, flat-mounted preparations indicate that Ascl1 is specifically expressed in a salt-and-pepper like pattern in the AH (Figures 2P,P’, arrowhead) and in the MH (Figures 2P,P’, unfilled arrowhead). Ascl1 is important for the differentiation of late hypothalamic neurons because in Ascl1 knock-out mice differentiation of neuroendocrine neurons is disturbed (Mcnay et al., 2006). Although the authors did not specifically look at the nTPOC or nMTT neurons it can be assumed these neurons will be affected.
During initiation of neuronal differentiation various proneural genes are recruited, but the specific proneural genes involved could be different between species and neuronal populations. Here, the expression of other proneural genes has been researched in the developing hypothalamus. Remarkably, as Neurog1/2 are not expressed in the hypothalamus (Ratié et al., 2013), Ascl1 appears to be the only proneural gene expressed in the ventral chick AH, at least during early development. Lateral inhibition is the process controlling differentiation of these neurons but the precise mechanisms is different between chick and mouse. There are several lines of evidence to suggest this including restriction of Ascl1 expression to the AH in chick (Figure 2B), where in mouse Ascl1 is expressed in both the AH and MH (Figure 2P). To date, no other proneural gene has been described in the developing chick MH. Neurog1 and Neurog2 are not found in the ventral hypothalamus of zebrafish and mouse (Ando et al., 2005; Mcnay et al., 2006; Osório et al., 2010), but a third member of the neurogenin family, Neurog3 has been identified, specifically expressed in the AH (Wang et al., 2001; Villasenor et al., 2008; Pelling et al., 2011). Neurog3 expression is regulated by Ascl1 (Mcnay et al., 2006), but in Neurog3 mutant mice there is no effect on early neurogenesis in the hypothalamus (Pelling et al., 2011; Anthwal et al., 2013). This lack of phenotype could be due to redundancy between the two proneural genes. It would be interesting to analyses Ascl1/Neurog3 mutant mice to determine whether there is an additional defect in the formation of the nTPOC.
Additionally, in zebrafish and mouse, Ascl1 and Neurog3 may act together to control the processes of lateral inhibition leading to the differentiation of the nTPOC, whereas in chick differentiation is specifically regulated by Ascl1.
As the capacity to regulate differentiation steps during neurogenesis is shared by all the proneural genes (Guillemot, 2007), it may explain why neuronal differentiation in the vertebrate hypothalamus is not conserved.
Description of Proneural Target Genes within the Hypothalamic Primordium
In the absence of Notch activity during nTPOC differentiation in the chick hypothalamus, Ascl1 is upregulated and induces expression of a wide spectrum of neuron specific genes (Castro et al., 2011; Ratié et al., 2013). While upregulation of some neuronal genes like Nhlh1 or Stmn2 is expected in tissue lacking Notch signaling, other genes identified are not associated with a role in hypothalamic development, such as Transgelin 3 (Tagln3) and Chromogranin A (Chga). Nhlh1 and Chga mutant mice are available, but there is no phenotype or effect on neurogenesis, suggesting redundancy with other genes (Krüger and Braun, 2002; Hendy et al., 2006; Schmid et al., 2007). Tagln3 appears to be a good marker because it is strongly expressed in the areas where both the nTPOC and nMTT form. In a flat-mounted preparation of HH15 chick hypothalamus, double labeling with Shh and Tagln3 reveals expression of Tagln3 in the AH and MH where the nTPOC and nMTT neurons are respectively located (Figure 3C, arrowhead and unfilled arrowhead). Tagln3 is also expressed in the ventral MLF population, which are the first neurons to develop in the brain (Figure 3C, asterisk). While these target genes are all expressed in post-mitotic neurons (Theodorakis et al., 2002; Pape et al., 2008; Xie et al., 2008; Burzynski et al., 2009; Ratié et al., under review) no specific function can be attributed to these genes during nTPOC and nMTT development.
Another set of genes are specifically upregulated in the chick AH when Notch signaling is inhibited, Slit1 and Robo2, which are well-known components involved in axon guidance (Chisholm and Tessier-Lavigne, 1999). They guide the TPOC axons through the hypothalamus (Devine and Key, 2008; Ricaño-Cornejo et al., 2011) and the regulation of these genes is Notch dependent (Ratié et al., 2013).
Analysis of the promoter regions in Slit1, Robo2, Tagln3 and Chga reveal binding sites of Hes5, Hey1, Ascl1 and Nhlh1 providing further evidence these target genes are part of the Notch/proneural regulatory network involved in neuronal differentiation in the hypothalamus (Ratié et al., 2013).
Molecular Cascade of Neurogenesis Onset in the Chick Hypothalamus Primordium
In order to corroborate this network of genes, the expression of Notch components and target genes is analyzed by in situ hybridization in the chick hypothalamus to provide further evidence for the existence of a molecular cascade that is Notch/proneural dependent.
The molecular cascade begins with the expression of Notch components and proneural genes followed by other bHLH transcription factors, target genes and well-known neuronal markers (Figure 4). Notch1, Hes5, Dll1, Ascl1, Nhlh1, NeuroD4, Stmn2, HuC/D and Chga are examples chosen to evaluate the stage of their first expression in the AH (Figure 4). At HH10, the first components to be expressed in the developing hypothalamus are Notch1, Dll1 and Ascl1, followed by Hes5 that form a regulatory loop (Figure 4A; Ratié et al., 2013). This mechanism has been well described in the literature for the induction of neurogenesis by lateral inhibition (Bertrand et al., 2002; Kageyama et al., 2008b).
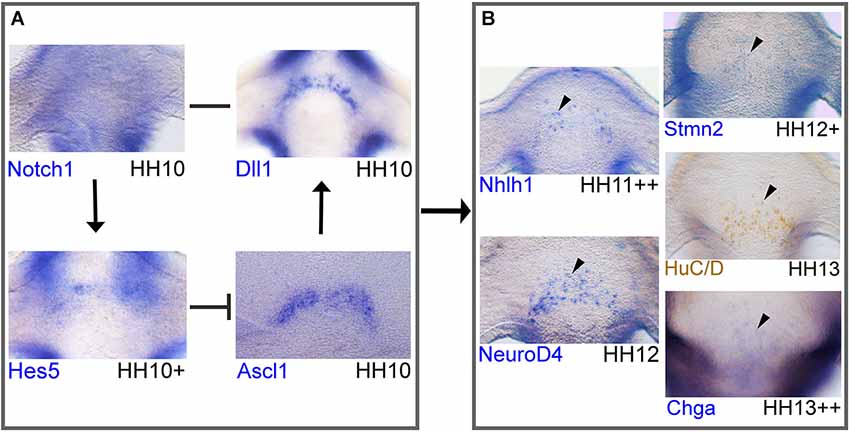
Figure 4. Network of Notch/proneural genes and initial expression of downstream targets in the developing chick hypothalamus. Whole-mount in situ hybridization or immunohistochemistry of markers in chick. Immunohistochemistry protocol has been described elsewhere (Lumsden and Keynes, 1989). Anti-HuC/D mouse (1:500; molecular probes; A21271) primary antibody was detected with a peroxidase-conjugated rabbit-anti-mouse secondary antibody (1:2000; Jackson ImmunoResearch; 315-035-045). Probes were obtained from cDNA and subcloned into pCRII-TOPO (Invitrogen) to make RNA probes or plasmids were obtained from other sources: Dll1 and Notch1 (kind gifts from Dr Frank Schubert). (A, B) Frontal view of the AH in the developing hypothalamus where the nTPOC neurons will differentiate. This network of genes is based on in silico results and data from Ratié et al., 2013. Expression of all markers, except Notch1 have a horseshoe shape. (A) Notch network loop in NPCs. Notch1 expression is ubiquitous throughout the hypothalamus at HH10. Dll1 and Ascl1 expression in the hypothalamus at HH10. Hes5 expression in the hypothalamus at HH10+. (B) Genes are upregulated in post-mitotic differentiating neurons. Expression of Nhlh1 at HH11+ and NeuroD4 at HH12. Stmn2 expression at HH12+, HuC/D expression at HH13 and Chga expression, first appears at HH13++ in very few cells in the developing hypothalamus. Genes are expressed in a salt-and-pepper like pattern (arrowhead). Expression confirms Notch components and Ascl1 are expressed first, followed by the expression of downstream targets. Arrows represent activation of downstream targets. Barred lines represent repression of downstream targets. A single line represents direct binding between ligand and receptor.
Notch1 is present in the AH, ubiquitously expressed (Figure 4A) compared with Dll1, Ascl1 and Hes5 that are expressed in a salt-and-pepper like pattern with a horseshoe shape (Figure 4). This is in agreement with a lateral inhibition model taking place in the AH. In this model, when Ascl1 is active in a NPC, this can upregulate other bHLH genes such as Nhlh1 (Ratié et al., 2013). Nhlh1 and NeuroD4 are analyzed as they are known markers of differentiation and expressed in the hypothalamus (Murdoch et al., 1999; Abu-Elmagd et al., 2001; Ratié et al., 2013). These genes are expressed from HH12, with Nhlh1 expression appearing slightly earlier at HH11++ (Figure 4B). Other genes are upregulated from around HH13 such as, the well-known neuronal markers Stmn2 and HuC/D but also new markers such as Chga (Figure 4B; Ratié et al., 2013).
BrdU labeling suggests Dll1 expressing cells have exited the cell cycle (Henrique et al., 1995; Myat et al., 1996) therefore NPCs destined to become nTPOC neurons exit the cell cycle around HH10 as seen with Dll1 expression (Figure 4A). It suggests that as early as HH10, the Dll1 positive cells of the hypothalamus are destined to become neurons several stages before they become mature neurons expressing markers such as Stmn2 or HuC/D at HH13. These results provide further evidence that the Notch/proneural loop is active in the hypothalamus from a very early stage before the first neurons appear.
Concluding Remarks
In this review, data has been discussed implicating the Notch/proneural network with a role during the differentiation of the first two groups of neurons that develop in the hypothalamus, the nTPOC and nMTT. There is still specific functional data lacking in the hypothalamus to conclude the specific mechanisms in which these neurons differentiate, but a general picture using expression data and interpretation of other functional models has been achieved. The same Notch/proneural network is likely to regulate differentiation of nTPOC neurons in zebrafish, chick and mouse. Considering the conservation of the TPOC axon tract and the Notch signaling pathway, this is not surprising. Data regarding proneural gene expression in the chick MH is still too scarce to conclude, but some of the components of the Notch/proneural network are expressed in the mouse MH before the nMTT neurons differentiate. This expression suggests the same mechanisms occur between the nTPOC and nMTT, only the players for nMTT differentiation are yet to be found in chick.
It is still not known what triggers this Notch/proneural loop in these hypothalamic NPCs. Neuronal specification during spinal cord development is initially generated by activities of two competing signaling pathways: SHH and BMP/Wnt (Ericson et al., 1997; Jessell, 2000; Liem et al., 2000). Evidence is emerging to suggest that SHH and BMP may play a similar role in the differentiation of the early hypothalamic neurons (Manning et al., 2006; Ahsan et al., 2007; Szabó et al., 2009; Alvarez-Bolado et al., 2012). However, how these signaling pathways integrate the Notch/proneural network has to be investigated in the developing hypothalamus. Future work will require a study to identify transcription factors that are necessary for the patterning of the AH and MH very early during vertebrate development.
One thing is clear, this review highlights lots of open questions regarding initial neuronal differentiation in the hypothalamus as well as general patterning of the hypothalamic regions. We hope that this review will encourage the scientific communities to investigate the phenotype of their mutants during earlier stages when the nTPOC and nMTT neurons develop.
A final thought, distinct late hypothalamic cell types dysfunction can lead to metabolic or homeostatic disorders and there is evidence that this is the case in congenital obesity (Gibson et al., 2004; Bingham et al., 2008). Therefore, could a defect in the induction and specification of the initial neurons lead to such disorders as these neurons are essential to the axon tract formation of the late hypothalamic neurons (such as the MCH neurons) (Croizier et al., 2011).
Author Contributions
Michelle Ware and Valérie Dupé set up and designed the experiments. Michelle Ware and Houda Hamdi-Rozé performed the experiments. Michelle Ware and Valérie Dupé wrote the manuscript. All authors read, discussed and edited the manuscript.
Conflict of Interest Statement
The authors declare that the research was conducted in the absence of any commercial or financial relationships that could be construed as a potential conflict of interest.
Acknowledgments
We would like to thank the members of the David laboratory for suggestions and comments. This work was supported by the Agence Nationale de la Recherche (grant no. ANR-12-BSV1-0007-01, Valérie Dupé). We also thank the animal house platform ARCHE (SFR Biosit, Rennes, France). We are grateful to receive the following plasmids: mouse Ascl1 (Dr Francois Guillemot, London, UK), chick Dll1 and Notch1 (Dr Frank Schubert, Portsmouth, UK).
Abbreviations
AH, anterior hypothalamus; bHLH, basic helix-loop-helix; MH, mammillary hypothalamus; MTT, mammillotegmental tract; TH, tuberal hypothalamus; NPC, neural progenitor cell; nTPOC, nucleus of the tract of the postoptic commissure; nMTT, nucleus of mamillo-tegmental tract; TPOC, tract of the postoptic commissure.
References
Abu-Elmagd, M., Ishii, Y., Cheung, M., Rex, M., Le Rouëdec, D., and Scotting, P. J. (2001). cSox3 expression and neurogenesis in the epibranchial placodes. Dev. Biol. 237, 258–269. doi: 10.1006/dbio.2001.0378
Pubmed Abstract | Pubmed Full Text | CrossRef Full Text | Google Scholar
Adam, J., Myat, A., Le Roux, I., Eddison, M., Henrique, D., Ish-Horowicz, D., et al. (1998). Cell fate choices and the expression of Notch, Delta and Serrate homologues in the chick inner ear: parallels with Drosophila sense-organ development. Development 125, 4645–4654.
Ahsan, M., Riley, K. L., and Schubert, F. R. (2007). Molecular mechanisms in the formation of the medial longitudinal fascicle. J. Anat. 211, 177–187. doi: 10.1111/j.1469-7580.2007.00774.x
Pubmed Abstract | Pubmed Full Text | CrossRef Full Text | Google Scholar
Allende, M. L., and Weinberg, E. S. (1994). The expression pattern of two zebrafish achaete-scute homolog (ash) genes is altered in the embryonic brain of the cyclops mutant. Dev. Biol. 166, 509–530. doi: 10.1006/dbio.1994.1334
Pubmed Abstract | Pubmed Full Text | CrossRef Full Text | Google Scholar
Alpeeva, E. V., and Makarenko, I. G. (2007). [Perinatal development of mammillotegmental connections in rats]. Ontogenez 38, 86–93.
Alvarez-Bolado, G., Paul, F. A., and Blaess, S. (2012). Sonic hedgehog lineage in the mouse hypothalamus: from progenitor domains to hypothalamic regions. Neural Dev. 7:4. doi: 10.1186/1749-8104-7-4
Pubmed Abstract | Pubmed Full Text | CrossRef Full Text | Google Scholar
Ando, H., Kobayashi, M., Tsubokawa, T., Uyemura, K., Furuta, T., and Okamoto, H. (2005). Lhx2 mediates the activity of Six3 in zebrafish forebrain growth. Dev. Biol. 287, 456–468. doi: 10.1016/j.ydbio.2005.09.023
Pubmed Abstract | Pubmed Full Text | CrossRef Full Text | Google Scholar
Anthwal, N., Pelling, M., Claxton, S., Mellitzer, G., Collin, C., Kessaris, N., et al. (2013). Conditional deletion of neurogenin-3 using Nkx2.1iCre results in a mouse model for the central control of feeding, activity and obesity. Dis. Model. Mech. 6, 1133–1145. doi: 10.1242/dmm.011916
Pubmed Abstract | Pubmed Full Text | CrossRef Full Text | Google Scholar
Aoto, K., Shikata, Y., Imai, H., Matsumaru, D., Tokunaga, T., Shioda, S., et al. (2009). Mouse Shh is required for prechordal plate maintenance during brain and craniofacial morphogenesis. Dev. Biol. 327, 106–120. doi: 10.1016/j.ydbio.2008.11.022
Pubmed Abstract | Pubmed Full Text | CrossRef Full Text | Google Scholar
Artavanis-Tsakonas, S., Rand, M. D., and Lake, R. J. (1999). Notch signaling: cell fate control and signal integration in development. Science 284, 770–776. doi: 10.1126/science.284.5415.770
Pubmed Abstract | Pubmed Full Text | CrossRef Full Text | Google Scholar
Artavanis-Tsakonas, S., and Simpson, P. (1991). Choosing a cell fate: a view from the Notch locus. Trends Genet. 7, 403–408. doi: 10.1016/0168-9525(91)90264-Q
Pubmed Abstract | Pubmed Full Text | CrossRef Full Text | Google Scholar
Aujla, P. K., Naratadam, G. T., Xu, L., and Raetzman, L. T. (2013). Notch/Rbpjkappa signaling regulates progenitor maintenance and differentiation of hypothalamic arcuate neurons. Development 140, 3511–3521. doi: 10.1242/dev.098681
Pubmed Abstract | Pubmed Full Text | CrossRef Full Text | Google Scholar
Bak, M., and Fraser, S. E. (2003). Axon fasciculation and differences in midline kinetics between pioneer and follower axons within commissural fascicles. Development 130, 4999–5008. doi: 10.1242/dev.00713
Pubmed Abstract | Pubmed Full Text | CrossRef Full Text | Google Scholar
Bardet, S. M., Ferran, J. L., Sanchez-Arrones, L., and Puelles, L. (2010). Ontogenetic expression of sonic hedgehog in the chicken subpallium. Front. Neuroanat. 4:28. doi: 10.3389/fnana.2010.00028
Pubmed Abstract | Pubmed Full Text | CrossRef Full Text | Google Scholar
Barreiro-Iglesias, A., Villar-Cheda, B., Abalo, X. M., Anadón, R., and Rodicio, M. C. (2008). The early scaffold of axon tracts in the brain of a primitive vertebrate, the sea lamprey. Brain Res. Bull. 75, 42–52. doi: 10.1016/j.brainresbull.2007.07.020
Pubmed Abstract | Pubmed Full Text | CrossRef Full Text | Google Scholar
Barsi, J. C., Rajendra, R., Wu, J. I., and Artzt, K. (2005). Mind bomb1 is a ubiquitin ligase essential for mouse embryonic development and Notch signaling. Mech. Dev. 122, 1106–1117. doi: 10.1016/j.mod.2005.06.005
Pubmed Abstract | Pubmed Full Text | CrossRef Full Text | Google Scholar
Barth, K. A., and Wilson, S. W. (1995). Expression of zebrafish nk2.2 is influenced by sonic hedgehog/vertebrate hedgehog-1 and demarcates a zone of neuronal differentiation in the embryonic forebrain. Development 121, 1755–1768.
Bertrand, N., Castro, D. S., and Guillemot, F. (2002). Proneural genes and the specification of neural cell types. Nat. Rev. Neurosci. 3, 517–530. doi: 10.1038/nrn874
Pubmed Abstract | Pubmed Full Text | CrossRef Full Text | Google Scholar
Bettenhausen, B., Hrabe De Angelis, M., Simon, D., Guénet, J. L., and Gossler, A. (1995). Transient and restricted expression during mouse embryogenesis of Dll1, a murine gene closely related to Drosophila Delta. Development 121, 2407–2418.
Bierkamp, C., and Campos-Ortega, J. (1993). A zebrafish homologue of the Drosophila neurogenic gene Notch and its pattern of transcription during early embryogenesis. Mech. Dev. 43, 87–100. doi: 10.1016/0925-4773(93)90027-U
Pubmed Abstract | Pubmed Full Text | CrossRef Full Text | Google Scholar
Bingham, N. C., Anderson, K. K., Reuter, A. L., Stallings, N. R., and Parker, K. L. (2008). Selective loss of leptin receptors in the ventromedial hypothalamic nucleus results in increased adiposity and a metabolic syndrome. Endocrinology 149, 2138–2148. doi: 10.1210/en.2007-1200
Pubmed Abstract | Pubmed Full Text | CrossRef Full Text | Google Scholar
Bray, S. J. (2006). Notch signalling: a simple pathway becomes complex. Nat. Rev. Mol. Cell Biol. 7, 678–689. doi: 10.1038/nrm2009
Pubmed Abstract | Pubmed Full Text | CrossRef Full Text | Google Scholar
Brooker, R., Hozumi, K., and Lewis, J. (2006). Notch ligands with contrasting functions: Jagged1 and Delta1 in the mouse inner ear. Development 133, 1277–1286. doi: 10.1242/dev.02284
Pubmed Abstract | Pubmed Full Text | CrossRef Full Text | Google Scholar
Burzynski, G. M., Delalande, J. M., and Shepherd, I. (2009). Characterization of spatial and temporal expression pattern of SCG10 during zebrafish development. Gene Expr. Patterns 9, 231–237. doi: 10.1016/j.gep.2008.12.010
Pubmed Abstract | Pubmed Full Text | CrossRef Full Text | Google Scholar
Campos-Ortega, J. A. (1993). Mechanisms of early neurogenesis in Drosophila melanogaster. J. Neurobiol. 24, 1305–1327. doi: 10.1002/neu.480241005
Pubmed Abstract | Pubmed Full Text | CrossRef Full Text | Google Scholar
Castro, D. S., Martynoga, B., Parras, C., Ramesh, V., Pacary, E., Johnston, C., et al. (2011). A novel function of the proneural factor Ascl1 in progenitor proliferation identified by genome-wide characterization of its targets. Genes Dev. 25, 930–945. doi: 10.1101/gad.627811
Pubmed Abstract | Pubmed Full Text | CrossRef Full Text | Google Scholar
Cau, E., Gradwohl, G., Casarosa, S., Kageyama, R., and Guillemot, F. (2000). Hes genes regulate sequential stages of neurogenesis in the olfactory epithelium. Development 127, 2323–2332.
Cavodeassi, F., and Houart, C. (2012). Brain regionalization: of signaling centers and boundaries. Dev. Neurobiol. 72, 218–233. doi: 10.1002/dneu.20938
Pubmed Abstract | Pubmed Full Text | CrossRef Full Text | Google Scholar
Chapman, S. C., Schubert, F. R., Schoenwolf, G. C., and Lumsden, A. (2002). Analysis of spatial and temporal gene expression patterns in blastula and gastrula stage chick embryos. Dev. Biol. 245, 187–199. doi: 10.1006/dbio.2002.0641
Pubmed Abstract | Pubmed Full Text | CrossRef Full Text | Google Scholar
Chapouton, P., Webb, K. J., Stigloher, C., Alunni, A., Adolf, B., Hesl, B., et al. (2011). Expression of hairy/enhancer of split genes in neural progenitors and neurogenesis domains of the adult zebrafish brain. J. Comp. Neurol. 519, 1748–1769. doi: 10.1002/cne.22599
Pubmed Abstract | Pubmed Full Text | CrossRef Full Text | Google Scholar
Chiang, C., Litingtung, Y., Lee, E., Young, K. E., Corden, J. L., Westphal, H., et al. (1996). Cyclopia and defective axial patterning in mice lacking sonic hedgehog gene function. Nature 383, 407–413. doi: 10.1038/383407a0
Pubmed Abstract | Pubmed Full Text | CrossRef Full Text | Google Scholar
Chisholm, A., and Tessier-Lavigne, M. (1999). Conservation and divergence of axon guidance mechanisms. Curr. Opin. Neurobiol. 9, 603–615. doi: 10.1016/S0959-4388(99)00021-5
Pubmed Abstract | Pubmed Full Text | CrossRef Full Text | Google Scholar
Chitnis, A., Henrique, D., Lewis, J., Ish-Horowicz, D., and Kintner, C. (1995). Primary neurogenesis in Xenopus embryos regulated by a homologue of the Drosophila neurogenic gene Delta. Nature 375, 761–766. doi: 10.1038/375761a0
Pubmed Abstract | Pubmed Full Text | CrossRef Full Text | Google Scholar
Chitnis, A. B., and Kuwada, J. Y. (1990). Axonogenesis in the brain of zebrafish embryos. J. Neurosci. 10, 1892–1905.
Chitnis, A. B., and Kuwada, J. Y. (1991). Elimination of a brain tract increases errors in pathfinding by follower growth cones in the zebrafish embryo. Neuron 7, 277–285. doi: 10.1016/0896-6273(91)90266-3
Pubmed Abstract | Pubmed Full Text | CrossRef Full Text | Google Scholar
Coffman, C., Harris, W., and Kintner, C. (1990). Xotch, the xenopus homolog of Drosophila notch. Science 249, 1438–1441. doi: 10.1126/science.2402639
Pubmed Abstract | Pubmed Full Text | CrossRef Full Text | Google Scholar
Croizier, S., Amiot, C., Chen, X., Presse, F., Nahon, J.-L., Wu, J. Y., et al. (2011). Development of posterior hypothalamic neurons enlightens a switch in the prosencephalic basic plan. PLoS One 6:e28574. doi: 10.1371/journal.pone.0028574
Pubmed Abstract | Pubmed Full Text | CrossRef Full Text | Google Scholar
Crossley, P. H., Martinez, S., Ohkubo, Y., and Rubenstein, J. L. (2001). Coordinate expression of Fgf8, Otx2, Bmp4 and Shh in the rostral prosencephalon during development of the telencephalic and optic vesicles. Neuroscience 108, 183–206. doi: 10.1016/S0306-4522(01)00411-0
Pubmed Abstract | Pubmed Full Text | CrossRef Full Text | Google Scholar
Dale, J. K., Vesque, C., Lints, T. J., Sampath, T. K., Furley, A., Dodd, J., et al. (1997). Cooperation of BMP7 and SHH in the induction of forebrain ventral midline cells by prechordal mesoderm. Cell 90, 257–269. doi: 10.1016/S0092-8674(00)80334-7
Pubmed Abstract | Pubmed Full Text | CrossRef Full Text | Google Scholar
de La Pompa, J. L., Wakeham, A., Correia, K. M., Samper, E., Brown, S., Aguilera, R. J., et al. (1997). Conservation of the Notch signalling pathway in mammalian neurogenesis. Development 124, 1139–1148.
Devine, C. A., and Key, B. (2008). Robo-slit interactions regulate longitudinal axon pathfinding in the embryonic vertebrate brain. Dev. Biol. 313, 371–383. doi: 10.1016/j.ydbio.2007.10.040
Pubmed Abstract | Pubmed Full Text | CrossRef Full Text | Google Scholar
Dyer, C., Linker, C., Graham, A., and Knight, R. (2014). Specification of sensory neurons occurs through diverse developmental programs functioning in the brain and spinal cord. Dev. Dyn. 243, 1429–1439. doi: 10.1002/dvdy.24184
Pubmed Abstract | Pubmed Full Text | CrossRef Full Text | Google Scholar
Easter, S. S. Jr., Ross, L. S., and Frankfurter, A. (1993). Initial tract formation in the mouse brain. J. Neurosci. 13, 285–299.
Eddison, M., Le Roux, I., and Lewis, J. (2000). Notch signaling in the development of the inner ear: lessons from Drosophila. Proc. Natl. Acad. Sci. U S A 97, 11692–11699. doi: 10.1073/pnas.97.22.11692
Pubmed Abstract | Pubmed Full Text | CrossRef Full Text | Google Scholar
Ericson, J., Briscoe, J., Rashbass, P., Van Heyningen, V., and Jessell, T. M. (1997). Graded sonic hedgehog signaling and the specification of cell fate in the ventral neural tube. Cold Spring Harb. Symp. Quant. Biol. 62, 451–466. doi: 10.1101/SQ7.1997.062.01.053
Pubmed Abstract | Pubmed Full Text | CrossRef Full Text | Google Scholar
Ferreiro, B., Skoglund, P., Bailey, A., Dorsky, R., and Harris, W. A. (1993). XASH1, a xenopus homolog of achaete-scute: a proneural gene in anterior regions of the vertebrate CNS. Mech. Dev. 40, 25–36. doi: 10.1016/0925-4773(93)90085-C
Pubmed Abstract | Pubmed Full Text | CrossRef Full Text | Google Scholar
Fior, R., and Henrique, D. (2009). “Notch-Off”: a perspective on the termination of Notch signalling. Int. J. Dev. Biol. 53, 1379–1384. doi: 10.1387/ijdb.072309rf
Pubmed Abstract | Pubmed Full Text | CrossRef Full Text | Google Scholar
Fortini, M. E. (2009). Notch signaling: the core pathway and its posttranslational regulation. Dev. Cell 16, 633–647. doi: 10.1016/j.devcel.2009.03.010
Pubmed Abstract | Pubmed Full Text | CrossRef Full Text | Google Scholar
Gibson, W. T., Pissios, P., Trombly, D. J., Luan, J., Keogh, J., Wareham, N. J., et al. (2004). Melanin-concentrating hormone receptor mutations and human obesity: functional analysis. Obes. Res. 12, 743–749. doi: 10.1038/oby.2004.89
Pubmed Abstract | Pubmed Full Text | CrossRef Full Text | Google Scholar
Gimeno, L., and Martinez, S. (2007). Expression of chick Fgf19 and mouse Fgf15 orthologs is regulated in the developing brain by Fgf8 and Shh. Dev. Dyn. 236, 2285–2297. doi: 10.1002/dvdy.21237
Pubmed Abstract | Pubmed Full Text | CrossRef Full Text | Google Scholar
Guillemot, F. (2007). Spatial and temporal specification of neural fates by transcription factor codes. Development 134, 3771–3780. doi: 10.1242/dev.006379
Pubmed Abstract | Pubmed Full Text | CrossRef Full Text | Google Scholar
Guillemot, F., and Joyner, A. L. (1993). Dynamic expression of the murine Achaete-Scute homologue Mash-1 in the developing nervous system. Mech. Dev. 42, 171–185. doi: 10.1016/0925-4773(93)90006-J
Pubmed Abstract | Pubmed Full Text | CrossRef Full Text | Google Scholar
Hatakeyama, J., Bessho, Y., Katoh, K., Ookawara, S., Fujioka, M., Guillemot, F., et al. (2004). Hes genes regulate size, shape and histogenesis of the nervous system by control of the timing of neural stem cell differentiation. Development 131, 5539–5550. doi: 10.1242/dev.01436
Pubmed Abstract | Pubmed Full Text | CrossRef Full Text | Google Scholar
Hauptmann, G., Söll, I., and Gerster, T. (2002). The early embryonic zebrafish forebrain is subdivided into molecularly distinct transverse and longitudinal domains. Brain Res. Bull. 57, 371–375. doi: 10.1016/S0361-9230(01)00691-8
Pubmed Abstract | Pubmed Full Text | CrossRef Full Text | Google Scholar
Hendy, G. N., Li, T., Girard, M., Feldstein, R. C., Mulay, S., Desjardins, R., et al. (2006). Targeted ablation of the chromogranin a (Chga) gene: normal neuroendocrine dense-core secretory granules and increased expression of other granins. Mol. Endocrinol. 20, 1935–1947. doi: 10.1210/me.2005-0398
Pubmed Abstract | Pubmed Full Text | CrossRef Full Text | Google Scholar
Henrique, D., Adam, J., Myat, A., Chitnis, A., Lewis, J., and Ish-Horowicz, D. (1995). Expression of a Delta homologue in prospective neurons in the chick. Nature 375, 787–790. doi: 10.1038/375787a0
Pubmed Abstract | Pubmed Full Text | CrossRef Full Text | Google Scholar
Hjorth, J. T., and Key, B. (2001). Are pioneer axons guided by regulatory gene expression domains in the zebrafish forebrain? High-resolution analysis of the patterning of the zebrafish brain during axon tract formation. Dev. Biol. 229, 271–286. doi: 10.1006/dbio.2000.9980
Pubmed Abstract | Pubmed Full Text | CrossRef Full Text | Google Scholar
Hrabe de Angelis, M., Mcintyre, J. 2nd, and Gossler, A. (1997). Maintenance of somite borders in mice requires the Delta homologue DII1. Nature 386, 717–721. doi: 10.1038/386717a0
Pubmed Abstract | Pubmed Full Text | CrossRef Full Text | Google Scholar
Iso, T., Kedes, L., and Hamamori, Y. (2003). HES and HERP families: multiple effectors of the notch signaling pathway. J. Cell. Physiol. 194, 237–255. doi: 10.1002/jcp.10208
Pubmed Abstract | Pubmed Full Text | CrossRef Full Text | Google Scholar
Itoh, M., Kim, C. H., Palardy, G., Oda, T., Jiang, Y. J., Maust, D., et al. (2003). Mind bomb is a ubiquitin ligase that is essential for efficient activation of Notch signaling by Delta. Dev. Cell 4, 67–82. doi: 10.1016/S1534-5807(02)00409-4
Pubmed Abstract | Pubmed Full Text | CrossRef Full Text | Google Scholar
Jarriault, S., Brou, C., Logeat, F., Schroeter, E. H., Kopan, R., and Israel, A. (1995). Signalling downstream of activated mammalian Notch. Nature 377, 355–358. doi: 10.1038/377355a0
Pubmed Abstract | Pubmed Full Text | CrossRef Full Text | Google Scholar
Jasoni, C. L., Walker, M. B., Morris, M. D., and Reh, T. A. (1994). A chicken achaete-scute homolog (CASH-1) is expressed in a temporally and spatially discrete manner in the developing nervous system. Development 120, 769–783.
Jessell, T. M. (2000). Neuronal specification in the spinal cord: inductive signals and transcriptional codes. Nat. Rev. Genet. 1, 20–29. doi: 10.1038/35049541
Pubmed Abstract | Pubmed Full Text | CrossRef Full Text | Google Scholar
Johnson, J. E., Birren, S. J., and Anderson, D. J. (1990). Two rat homologues of Drosophila achaete-scute specifically expressed in neuronal precursors. Nature 346, 858–861. doi: 10.1038/346858a0
Pubmed Abstract | Pubmed Full Text | CrossRef Full Text | Google Scholar
Kageyama, R., Niwa, Y., Shimojo, H., Kobayashi, T., and Ohtsuka, T. (2010). “Chapter Ten - Ultradian oscillations in Notch signaling regulate dynamic biological events,” in Current Topics in Developmental Biology, ed K. Raphael (San Diego: Academic Press), 311–331.
Kageyama, R., Ohtsuka, T., and Kobayashi, T. (2008a). Roles of Hes genes in neural development. Dev. Growth Differ. 50(Suppl. 1), S97–S103. doi: 10.1111/j.1440-169X.2008.00993.x
Pubmed Abstract | Pubmed Full Text | CrossRef Full Text | Google Scholar
Kageyama, R., Ohtsuka, T., Shimojo, H., and Imayoshi, I. (2008b). Dynamic Notch signaling in neural progenitor cells and a revised view of lateral inhibition. Nat. Neurosci. 11, 1247–1251. doi: 10.1038/nn.2208
Pubmed Abstract | Pubmed Full Text | CrossRef Full Text | Google Scholar
Kageyama, R., Ohtsuka, T., Shimojo, H., and Imayoshi, I. (2009). Dynamic regulation of Notch signaling in neural progenitor cells. Curr. Opin. Cell Biol. 21, 733–740. doi: 10.1016/j.ceb.2009.08.009
Pubmed Abstract | Pubmed Full Text | CrossRef Full Text | Google Scholar
Kiecker, C., and Niehrs, C. (2001). The role of prechordal mesendoderm in neural patterning. Curr. Opin. Neurobiol. 11, 27–33. doi: 10.1016/S0959-4388(00)00170-7
Pubmed Abstract | Pubmed Full Text | CrossRef Full Text | Google Scholar
Kiernan, A. E. (2013). Notch signaling during cell fate determination in the inner ear. Semin. Cell Dev. Biol. 24, 470–479. doi: 10.1016/j.semcdb.2013.04.002
Pubmed Abstract | Pubmed Full Text | CrossRef Full Text | Google Scholar
Koo, B. K., Lim, H. S., Song, R., Yoon, M. J., Yoon, K. J., Moon, J. S., et al. (2005). Mind bomb 1 is essential for generating functional Notch ligands to activate Notch. Development 132, 3459–3470. doi: 10.1242/dev.01922
Pubmed Abstract | Pubmed Full Text | CrossRef Full Text | Google Scholar
Koop, K. E., Macdonald, L. M., and Lobe, C. G. (1996). Transcripts of Grg4, a murine groucho-related gene, are detected in adjacent tissues to other murine neurogenic gene homologues during embryonic development. Mech. Dev. 59, 73–87. doi: 10.1016/0925-4773(96)00582-5
Pubmed Abstract | Pubmed Full Text | CrossRef Full Text | Google Scholar
Krüger, M., and Braun, T. (2002). The neuronal basic helix-loop-helix transcription factor NSCL-1 is dispensable for normal neuronal development. Mol. Cell. Biol. 22, 792–800. doi: 10.1128/MCB.22.3.792-800.2002
Pubmed Abstract | Pubmed Full Text | CrossRef Full Text | Google Scholar
Kwon, H. G., Hong, J. H., and Jang, S. H. (2011). Mammillotegmental tract in the human brain: diffusion tensor tractography study. Neuroradiology 53, 623–626. doi: 10.1007/s00234-011-0858-y
Pubmed Abstract | Pubmed Full Text | CrossRef Full Text | Google Scholar
Leimeister, C., Externbrink, A., Klamt, B., and Gessler, M. (1999). Hey genes: a novel subfamily of hairy- and Enhancer of split related genes specifically expressed during mouse embryogenesis. Mech. Dev. 85, 173–177. doi: 10.1016/S0925-4773(99)00080-5
Pubmed Abstract | Pubmed Full Text | CrossRef Full Text | Google Scholar
Liem, K. F. Jr., Jessell, T. M., and Briscoe, J. (2000). Regulation of the neural patterning activity of sonic hedgehog by secreted BMP inhibitors expressed by notochord and somites. Development 127, 4855–4866.
Lumsden, A., and Keynes, R. (1989). Segmental patterns of neuronal development in the chick hindbrain. Nature 337, 424–428. doi: 10.1038/337424a0
Pubmed Abstract | Pubmed Full Text | CrossRef Full Text | Google Scholar
Macdonald, R., Xu, Q., Barth, K. A., Mikkola, I., Holder, N., Fjose, A., et al. (1994). Regulatory gene expression boundaries demarcate sites of neuronal differentiation in the embryonic zebrafish forebrain. Neuron 13, 1039–1053. doi: 10.1016/0896-6273(94)90044-2
Pubmed Abstract | Pubmed Full Text | CrossRef Full Text | Google Scholar
Maier, M. M., and Gessler, M. (2000). Comparative analysis of the human and mouse Hey1 promoter: hey genes are new Notch target genes. Biochem. Biophys. Res. Commun. 275, 652–660. doi: 10.1006/bbrc.2000.3354
Pubmed Abstract | Pubmed Full Text | CrossRef Full Text | Google Scholar
Manning, L., Ohyama, K., Saeger, B., Hatano, O., Wilson, S. A., Logan, M., et al. (2006). Regional morphogenesis in the hypothalamus: a BMP-Tbx2 pathway coordinates fate and proliferation through Shh downregulation. Dev. Cell 11, 873–885. doi: 10.1016/j.devcel.2006.09.021
Pubmed Abstract | Pubmed Full Text | CrossRef Full Text | Google Scholar
Marion, J. F., Yang, C., Caqueret, A., Boucher, F., and Michaud, J. L. (2005). Sim1 and Sim2 are required for the correct targeting of mammillary body axons. Development 132, 5527–5537. doi: 10.1242/dev.02142
Pubmed Abstract | Pubmed Full Text | CrossRef Full Text | Google Scholar
Martinez-Ferre, A., and Martinez, S. (2012). Molecular regionalization of the diencephalon. Front. Neurosci. 6:73. doi: 10.3389/fnins.2012.00073
Pubmed Abstract | Pubmed Full Text | CrossRef Full Text | Google Scholar
Mastick, G. S., Davis, N. M., Andrew, G. L., and Easter, S. S. Jr. (1997). Pax-6 functions in boundary formation and axon guidance in the embryonic mouse forebrain. Development 124, 1985–1997.
Mastick, G. S., and Easter, S. S. Jr. (1996). Initial organization of neurons and tracts in the embryonic mouse fore- and midbrain. Dev. Biol. 173, 79–94. doi: 10.1006/dbio.1996.0008
Pubmed Abstract | Pubmed Full Text | CrossRef Full Text | Google Scholar
Mathieu, J., Barth, A., Rosa, F. M., Wilson, S. W., and Peyriéras, N. (2002). Distinct and cooperative roles for Nodal and Hedgehog signals during hypothalamic development. Development 129, 3055–3065.
Mcnay, D. E., Pelling, M., Claxton, S., Guillemot, F., and Ang, S. L. (2006). Mash1 is required for generic and subtype differentiation of hypothalamic neuroendocrine cells. Mol. Endocrinol. 20, 1623–1632. doi: 10.1210/me.2005-0518
Pubmed Abstract | Pubmed Full Text | CrossRef Full Text | Google Scholar
Mercier, S., Dubourg, C., Garcelon, N., Campillo-Gimenez, B., Gicquel, I., Belleguic, M., et al. (2011). New findings for phenotype-genotype correlations in a large European series of holoprosencephaly cases. J. Med. Genet. 48, 752–760. doi: 10.1136/jmedgenet-2011-100339
Pubmed Abstract | Pubmed Full Text | CrossRef Full Text | Google Scholar
Murdoch, J. N., Eddleston, J., Leblond-Bourget, N., Stanier, P., and Copp, A. J. (1999). Sequence and expression analysis of Nhlh1: a basic helix-loop-helix gene implicated in neurogenesis. Dev. Genet. 24, 165–177. doi: 10.1002/(SICI)1520-6408(1999)24:1/2<165::AID-DVG15>3.0.CO;2-V
Pubmed Abstract | Pubmed Full Text | CrossRef Full Text | Google Scholar
Myat, A., Henrique, D., Ish-Horowicz, D., and Lewis, J. (1996). A chick homologue of serrate and its relationship with Notch and Delta homologues during central neurogenesis. Dev. Biol. 174, 233–247. doi: 10.1006/dbio.1996.0069
Pubmed Abstract | Pubmed Full Text | CrossRef Full Text | Google Scholar
Neves, J., Abelló, G., Petrovic, J., and Giraldez, F. (2013). Patterning and cell fate in the inner ear: a case for Notch in the chicken embryo. Dev. Growth Differ. 55, 96–112. doi: 10.1111/dgd.12016
Pubmed Abstract | Pubmed Full Text | CrossRef Full Text | Google Scholar
Nural, H. F., and Mastick, G. S. (2004). Pax6 guides a relay of pioneer longitudinal axons in the embryonic mouse forebrain. J. Comp. Neurol. 479, 399–409. doi: 10.1002/cne.20317
Pubmed Abstract | Pubmed Full Text | CrossRef Full Text | Google Scholar
Ohyama, K., Das, R., and Placzek, M. (2008). Temporal progression of hypothalamic patterning by a dual action of BMP. Development 135, 3325–3331. doi: 10.1242/dev.027078
Pubmed Abstract | Pubmed Full Text | CrossRef Full Text | Google Scholar
Oka, C., Nakano, T., Wakeham, A., de la Pompa, J. L., Mori, C., Sakai, T., et al. (1995). Disruption of the mouse RBP-J kappa gene results in early embryonic death. Development 121, 3291–3301.
Osório, J., Mueller, T., Rétaux, S., Vernier, P., and Wullimann, M. F. (2010). Phylotypic expression of the bHLH genes neurogenin2, neurod and Mash1 in the mouse embryonic forebrain. J. Comp. Neurol. 518, 851–871. doi: 10.1002/cne.22247
Pubmed Abstract | Pubmed Full Text | CrossRef Full Text | Google Scholar
Pape, M., Doxakis, E., Reiff, T., Duong, C. V., Davies, A., Geissen, M., et al. (2008). A function for the calponin family member NP25 in neurite outgrowth. Dev. Biol. 321, 434–443. doi: 10.1016/j.ydbio.2008.07.001
Pubmed Abstract | Pubmed Full Text | CrossRef Full Text | Google Scholar
Paridaen, J. T., and Huttner, W. B. (2014). Neurogenesis during development of the vertebrate central nervous system. EMBO Rep. 15, 351–364. doi: 10.1002/embr.201438447
Pubmed Abstract | Pubmed Full Text | CrossRef Full Text | Google Scholar
Patel, C. K., Rodriguez, L. C., and Kuwada, J. Y. (1994). Axonal outgrowth within the abnormal scaffold of brain tracts in a zebrafish mutant. J. Neurobiol. 25, 345–360. doi: 10.1002/neu.480250402
Pubmed Abstract | Pubmed Full Text | CrossRef Full Text | Google Scholar
Patten, I., and Placzek, M. (2002). Opponent activities of Shh and BMP signaling during floor plate induction in vivo. Curr. Biol. 12, 47–52. doi: 10.1016/S0960-9822(01)00631-5
Pubmed Abstract | Pubmed Full Text | CrossRef Full Text | Google Scholar
Pelling, M., Anthwal, N., Mcnay, D., Gradwohl, G., Leiter, A. B., Guillemot, F., et al. (2011). Differential requirements for neurogenin 3 in the development of POMC and NPY neurons in the hypothalamus. Dev. Biol. 349, 406–416. doi: 10.1016/j.ydbio.2010.11.007
Pubmed Abstract | Pubmed Full Text | CrossRef Full Text | Google Scholar
Pera, E. M., and Kessel, M. (1997). Patterning of the chick forebrain anlage by the prechordal plate. Development 124, 4153–4162.
Pera, E. M., and Kessel, M. (1998). Demarcation of ventral territories by the homeobox gene NKX2.1 during early chick development. Dev. Genes Evol. 208, 168–171. doi: 10.1007/s004270050170
Pubmed Abstract | Pubmed Full Text | CrossRef Full Text | Google Scholar
Petrovic, J., Formosa-Jordan, P., Luna-Escalante, J. C., Abelló, G., Ibañes, M., Neves, J., et al. (2014). Ligand-dependent Notch signaling strength orchestrates lateral induction and lateral inhibition in the developing inner ear. Development 141, 2313–2324. doi: 10.1242/dev.108100
Pubmed Abstract | Pubmed Full Text | CrossRef Full Text | Google Scholar
Pierfelice, T., Alberi, L., and Gaiano, N. (2011). Notch in the vertebrate nervous system: an old dog with new tricks. Neuron 69, 840–855. doi: 10.1016/j.neuron.2011.02.031
Pubmed Abstract | Pubmed Full Text | CrossRef Full Text | Google Scholar
Przemeck, G. K., Heinzmann, U., Beckers, J., and Hrabe De Angelis, M. (2003). Node and midline defects are associated with left-right development in Delta1 mutant embryos. Development 130, 3–13. doi: 10.1242/dev.00176
Pubmed Abstract | Pubmed Full Text | CrossRef Full Text | Google Scholar
Puelles, L., Amat, J. A., and Martinez-de-la-Torre, M. (1987). Segment-related, mosaic neurogenetic pattern in the forebrain and mesencephalon of early chick embryos: I. Topography of AChE-positive neuroblasts up to stage HH18. J. Comp. Neurol. 266, 247–268. doi: 10.1002/cne.902660210
Pubmed Abstract | Pubmed Full Text | CrossRef Full Text | Google Scholar
Puelles, L., Martinez-De-La-Torre, M., Bardet, S., and Rubenstein, J. L. R. (2012). “Chapter 8 - Hypothalamus,” in The Mouse Nervous System, ed C. W. P. Puelles (San Diego: Academic Press), 221–312.
Puelles, L., and Rubenstein, J. L. (2003). Forebrain gene expression domains and the evolving prosomeric model. Trends Neurosci. 26, 469–476. doi: 10.1016/S0166-2236(03)00234-0
Pubmed Abstract | Pubmed Full Text | CrossRef Full Text | Google Scholar
Ratié, L., Ware, M., Barloy-Hubler, F., Romé, H., Gicquel, I., Dubourg, C., et al. (2013). Novel genes upregulated when NOTCH signalling is disrupted during hypothalamic development. Neural Dev. 8:25. doi: 10.1186/1749-8104-8-25
Pubmed Abstract | Pubmed Full Text | CrossRef Full Text | Google Scholar
Reaume, A. G., Conlon, R. A., Zirngibl, R., Yamaguchi, T. P., and Rossant, J. (1992). Expression analysis of a Notch homologue in the mouse embryo. Dev. Biol. 154, 377–387. doi: 10.1016/0012-1606(92)90076-S
Pubmed Abstract | Pubmed Full Text | CrossRef Full Text | Google Scholar
Ricaño-Cornejo, I., Altick, A. L., García-Peña, C. M., Nural, H. F., Echevarría, D., Miquelajáuregui, A., et al. (2011). Slit-Robo signals regulate pioneer axon pathfinding of the tract of the postoptic commissure in the mammalian forebrain. J. Neurosci. Res. 89, 1531–1541. doi: 10.1002/jnr.22684
Pubmed Abstract | Pubmed Full Text | CrossRef Full Text | Google Scholar
Rohr, K. B., Barth, K. A., Varga, Z. M., and Wilson, S. W. (2001). The nodal pathway acts upstream of hedgehog signaling to specify ventral telencephalic identity. Neuron 29, 341–351. doi: 10.1016/S0896-6273(01)00210-0
Pubmed Abstract | Pubmed Full Text | CrossRef Full Text | Google Scholar
Rohr, K. B., and Concha, M. L. (2000). Expression of nk2.1a during early development of the thyroid gland in zebrafish. Mech. Dev. 95, 267–270. doi: 10.1016/S0925-4773(00)00345-2
Pubmed Abstract | Pubmed Full Text | CrossRef Full Text | Google Scholar
Ross, L. S., Parrett, T., and Easter, S. S. Jr. (1992). Axonogenesis and morphogenesis in the embryonic zebrafish brain. J. Neurosci. 12, 467–482.
Saravanamuthu, S. S., Gao, C. Y., and Zelenka, P. S. (2009). Notch signaling is required for lateral induction of Jagged1 during FGF-induced lens fiber differentiation. Dev. Biol. 332, 166–176. doi: 10.1016/j.ydbio.2009.05.566
Pubmed Abstract | Pubmed Full Text | CrossRef Full Text | Google Scholar
Schmid, T., Krüger, M., and Braun, T. (2007). NSCL-1 and -2 control the formation of precerebellar nuclei by orchestrating the migration of neuronal precursor cells. J. Neurochem. 102, 2061–2072. doi: 10.1111/j.1471-4159.2007.04694.x
Pubmed Abstract | Pubmed Full Text | CrossRef Full Text | Google Scholar
Shanmugalingam, S., Houart, C., Picker, A., Reifers, F., Macdonald, R., Barth, A., et al. (2000). Ace/Fgf8 is required for forebrain commissure formation and patterning of the telencephalon. Development 127, 2549–2561.
Shimada, M., and Nakamura, T. (1973). Time of neuron origin in mouse hypothalamic nuclei. Exp. Neurol. 41, 163–173. doi: 10.1016/0014-4886(73)90187-8
Pubmed Abstract | Pubmed Full Text | CrossRef Full Text | Google Scholar
Shimamura, K., Hartigan, D. J., Martinez, S., Puelles, L., and Rubenstein, J. L. (1995). Longitudinal organization of the anterior neural plate and neural tube. Development 121, 3923–3933.
Shimogori, T., Lee, D. A., Miranda-Angulo, A., Yang, Y., Wang, H., Jiang, L., et al. (2010). A genomic atlas of mouse hypothalamic development. Nat. Neurosci. 13, 767–775. doi: 10.1038/nn.2545
Pubmed Abstract | Pubmed Full Text | CrossRef Full Text | Google Scholar
Simerly, R. B. (2004). “Anatomical substrates of hypothalamic integration,” in The Rat Nervous System, ed G. Paxinos (Amsterdam: Elsevier Science), 335–368.
Sussel, L., Marin, O., Kimura, S., and Rubenstein, J. L. (1999). Loss of Nkx2.1 homeobox gene function results in a ventral to dorsal molecular respecification within the basal telencephalon: evidence for a transformation of the pallidum into the striatum. Development 126, 3359–3370.
Szabó, N. E., Zhao, T., Cankaya, M., Theil, T., Zhou, X., and Alvarez-Bolado, G. (2009). Role of neuroepithelial sonic hedgehog in hypothalamic patterning. J. Neurosci. 29, 6989–7002. doi: 10.1523/JNEUROSCI.1089-09.2009
Pubmed Abstract | Pubmed Full Text | CrossRef Full Text | Google Scholar
Tamura, K., Taniguchi, Y., Minoguchi, S., Sakai, T., Tun, T., Furukawa, T., et al. (1995). Physical interaction between a novel domain of the receptor Notch and the transcription factor RBP-Jκ/Su(H). Curr. Biol. 5, 1416–1423. doi: 10.1016/S0960-9822(95)00279-X
Pubmed Abstract | Pubmed Full Text | CrossRef Full Text | Google Scholar
Theodorakis, K., Kyriakopoulou, K., Wassef, M., and Karagogeos, D. (2002). Novel sites of expression of the bHLH gene NSCL1 in the developing nervous system. Mech. Dev. 119(Suppl. 1), S103–S106. doi: 10.1016/S0925-4773(03)00100-X
Pubmed Abstract | Pubmed Full Text | CrossRef Full Text | Google Scholar
Varga, Z. M., Amores, A., Lewis, K. E., Yan, Y. L., Postlethwait, J. H., Eisen, J. S., et al. (2001). Zebrafish smoothened functions in ventral neural tube specification and axon tract formation. Development 128, 3497–3509.
Villasenor, A., Chong, D. C., and Cleaver, O. (2008). Biphasic Ngn3 expression in the developing pancreas. Dev. Dyn. 237, 3270–3279. doi: 10.1002/dvdy.21740
Pubmed Abstract | Pubmed Full Text | CrossRef Full Text | Google Scholar
Wang, X., Chu, L. T., He, J., Emelyanov, A., Korzh, V., and Gong, Z. (2001). A novel zebrafish bHLH gene, neurogenin3, is expressed in the hypothalamus. Gene 275, 47–55. doi: 10.1016/S0378-1119(01)00648-5
Pubmed Abstract | Pubmed Full Text | CrossRef Full Text | Google Scholar
Ware, M., and Schubert, F. R. (2011). Development of the early axon scaffold in the rostral brain of the chick embryo. J. Anat. 219, 203–216. doi: 10.1111/j.1469-7580.2011.01389.x
Pubmed Abstract | Pubmed Full Text | CrossRef Full Text | Google Scholar
Ware, M., Waring, C. P., and Schubert, F. R. (2014). Development of the early axon scaffold in the rostral brain of the small spotted cat shark (Scyliorhinus canicula) embryo. Int. Sch. Res. Not. 2014:8. doi: 10.1155/2014/196594
Williams, R., Lendahl, U., and Lardelli, M. (1995). Complementary and combinatorial patterns of Notch gene family expression during early mouse development. Mech. Dev. 53, 357–368. doi: 10.1016/0925-4773(95)00451-3
Pubmed Abstract | Pubmed Full Text | CrossRef Full Text | Google Scholar
Wilson, S. W., Ross, L. S., Parrett, T., and Easter, S. S. Jr. (1990). The development of a simple scaffold of axon tracts in the brain of the embryonic zebrafish, Brachydanio rerio. Development 108, 121–145.
Wolf, A., and Ryu, S. (2013). Specification of posterior hypothalamic neurons requires coordinated activities of Fezf2, Otp, Sim1a and Foxb1.2. Development 140, 1762–1773. doi: 10.1242/dev.085357
Pubmed Abstract | Pubmed Full Text | CrossRef Full Text | Google Scholar
Xie, J., Wang, W. Q., Liu, T. X., Deng, M., and Ning, G. (2008). Spatio-temporal expression of chromogranin a during zebrafish embryogenesis. J. Endocrinol. 198, 451–458. doi: 10.1677/JOE-08-0221
Pubmed Abstract | Pubmed Full Text | CrossRef Full Text | Google Scholar
Keywords: early axon scaffold, forebrain, differentiation, tract of the postoptic commissure, mammillotegmental tract, hypothalamus patterning, ASCL1
Citation: Ware M, Hamdi-Rozé H and Dupé V (2014) Notch signaling and proneural genes work together to control the neural building blocks for the initial scaffold in the hypothalamus. Front. Neuroanat. 8:140. doi: 10.3389/fnana.2014.00140
Received: 01 October 2014; Paper pending published: 21 October 2014;
Accepted: 10 November 2014; Published online: 02 December 2014.
Edited by:
Gonzalo Alvarez-Bolado, University of Heidelberg, GermanyReviewed by:
Andrea Wizenmann, University of Tuebingen, GermanyPierre-Yves Risold, Université de Franche-Comté, France
Copyright © 2014 Ware, Hamdi-Rozé and Dupé. This is an open-access article distributed under the terms of the Creative Commons Attribution License (CC BY). The use, distribution and reproduction in other forums is permitted, provided the original author(s) or licensor are credited and that the original publication in this journal is cited, in accordance with accepted academic practice. No use, distribution or reproduction is permitted which does not comply with these terms.
*Correspondence: Valérie Dupé, Institut de Génétique et Développement de Rennes, Faculté de Médecine, CNRS UMR6290, Université de Rennes 1, IFR140 GFAS, 2 Avenue du Pr. Léon Bernard, 35043 Rennes Cedex, France e-mail:dmFsZXJpZS5kdXBlQHVuaXYtcmVubmVzMS5mcg==