- 1Department of Neuroscience, Uppsala University, Uppsala, Sweden
- 2Department of Immunology, Genetics and Pathology, Uppsala University, Uppsala, Sweden
Thorough investigation of a neuronal population can help reveal key aspects regarding the nervous system and its development. The retinal horizontal cells have several extraordinary features making them particularly interesting for addressing questions regarding fate assignment and subtype specification. In this review we discuss and summarize data concerning the formation and diversity of horizontal cells, how morphology is correlated to molecular markers, and how fate assignment separates the horizontal lineage from the lineages of other retinal cell types. We discuss the novel and unique features of the final cell cycle of horizontal cell progenitors and how they may relate to retinoblastoma carcinogenesis.
A Brief Introduction to Horizontal Cells
Multipotent retinal progenitor cells (RPCs) in the vertebrate optic cup give rise to the five types of neurons and one type of glia that make up the mature retina (reviewed in Masland, 2001a; Marquardt and Gruss, 2002; Boije et al., 2014). The neuronal cell types: photoreceptors, horizontal, bipolar, amacrine, and ganglion cells, can be further divided into subtypes based on various morphological, functional, and molecular criteria (Masland, 2001b). During the Nineteenth century the Spanish neuroscientist Santiago Ramón y Cajal was the first to classify the retinal neurons and some of their subtypes based on morphological criteria (Ramón y Cajal, 1972). Since then, molecular and functional definitions have often been found to correlate with the morphological classifications thereby validating the oldest taxonomy.
Horizontal cells (HCs) have been identified in all vertebrate retinas from fish to man (Gallego, 1971, 1982, 1986; Peichl et al., 1998). They were found to be the source of the puzzling S-potentials, where hyperpolarization occurs as a response to light stimulus (Svaetichin, 1953; Werblin and Dowling, 1969; Kaneko, 1970). HCs facilitate both long and short range interactions between photoreceptors (PRs) and through inhibitory feed-back they aid in contrast enhancement and color opponency (Twig et al., 2003). There are several reasons why HCs are of particular interest. They have clearly distinguishable subtypes, both by morphological criteria and by molecular markers, allowing the study of subtype formation. Unlike many other cell types in the retina, the final number of HCs is not adjusted by apoptosis (Mayordomo, 2001; Edqvist et al., 2008). HCs undergo a unique bi-directional migration, resulting in a stopover at the basal side of the retina before arriving at their predestined position close to the PRs (Edqvist and Hallböök, 2004). Furthermore, in zebrafish and chicken, committed HCs has been shown to undergo non-apical mitoses in a semi-differentiated state (Godinho et al., 2007; Boije et al., 2009). Recent studies in chicken revealed a novel cell cycle behavior by the HCs that includes endoreplication leading to heteroploidy (Shirazi Fard et al., 2013). Moreover, HCs can divide in the presence of DNA damage and are able to develop into retinoblastoma in a murine model (Ajioka et al., 2007; Shirazi Fard et al., 2014b). In this review we will discuss the progress made regarding these HC features and how these findings relate to general concepts of development, evolution, and tumor formation.
Morphological and Functional Diversity of Horizontal Cells
Horizontal cells can be divided into axon-bearing and axon-less subtypes, a feature that appears to be conserved in the vertebrate lineage with few known exceptions (Gallego, 1986; Peichl et al., 1998; Table 1). The emerging pattern for all vertebrate retinas examined so far is that the axon-bearing HC is universal, and that any additional HC subtype(s) adhere to the axon-less population. In the chicken retina, three different HC subtypes have been described based on morphology; the “brush-shaped” (H1) is axon-bearing, whereas the “stellate” (H2) and the “candelabrum-shaped” (H3) are both axon-less (Figure 1; Genis-Galves et al., 1979; Gallego, 1986). In some species these HC subtypes are referred to as A-, B-, and C-type (Table 1). As will be discussed below, this morphological classification has since been found to correlate with the expression of unique molecular markers.
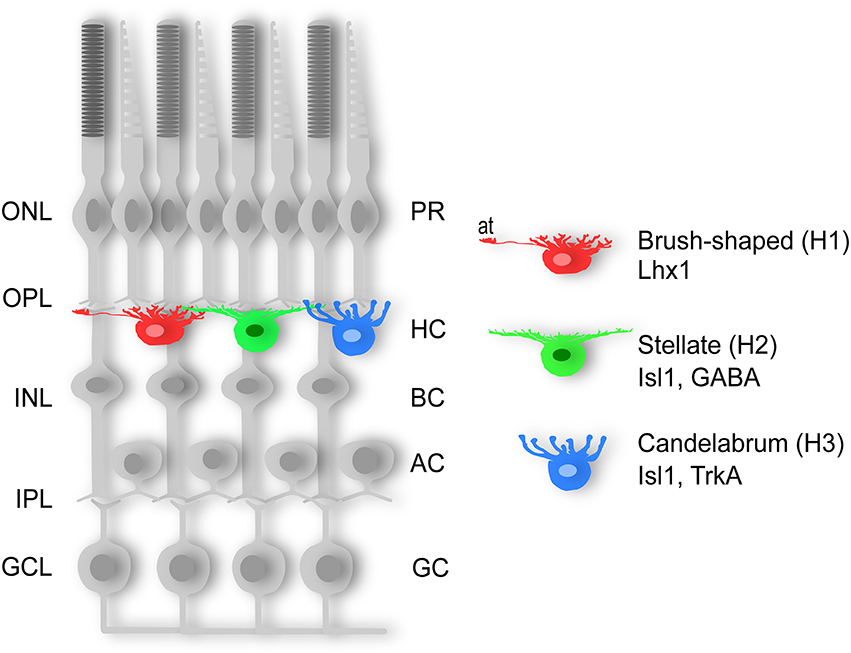
Figure 1. Three horizontal cell types. Schematic diagram of the three subtypes of chicken horizontal cells. The “brush-shaped” (H1) is axon-bearing and expresses Lhx1 (Lim1), whereas the “stellate” (H2) and the “candelabrum-shaped” (H3) are both axon-less and express Isl1. AC; amacrine cell, BC; bipolar cell, GC; ganglion cell, GCL; ganglion cell layer, H1-3; horizontal cell type 1-3, HC; horizontal cell, INL; inner nuclear layer, IPL; inner plexiform layer, ONL; outer nuclear layer, OPL; outer plexiform layer, PR; photoreceptor.
The division into axon-bearing and axon-less HC subtypes has also been suggested to reflect a functional difference where the axon-terminus of H1 HCs mainly connects to rod PRs, whereas the dendritic tree of all subtypes exclusively forms connections with cone PRs (Zhang A. -J. et al., 2006; Zhang J. et al., 2006). Although there is no clear-cut relationship between a low cone/rod ratio and the number of HC subtypes (reviewed in Peichl et al., 1998), the difference in the number of HC subtypes among different species seem to be loosely correlated with the relative numbers of cone and rod PRs. In cone-rich retinas (e.g., the chicken retina), two types of axon-less HCs are present alongside the axon-bearing HCs (Genis-Galves et al., 1979; Gallego, 1986). In contrast, rod-dominated retinas, such as most mammalian retinas (Ahnelt and Kolb, 2000), have only one type of axon-less HC. Extremely rod-dominated retinas, such as the mouse and rat retina, with merely 1–3% cones (Szél et al., 1996; Peichl, 2005), lack the axon-less HC subtypes altogether (Peichl and González-Soriano, 1994). Examples of species with only the axon-bearing HC subtype have been described in such diverse groups as fish, marsupials, and mammals (Peichl and González-Soriano, 1994; Lyser et al., 1999; Hirt and Wagner, 2005; Table 1). Thus, it may be that the loss of axon-less HCs in highly rod-dominated retinas is a result of adaptations to a nocturnal lifestyle, since few cones would make the “cone-specialized” axon-less HCs obsolete.
Molecular Markers for Horizontal Cell Subtypes
Data from our lab and others have shown that all HCs in the chicken retina express the homeodomain transcription factors (TF) Prox1 and Pax6 whereas the LIM/homeodomain TFs Lhx1 (Lim1) and Isl1 are both expressed in half the HC population in a non-overlapping manner (Edqvist et al., 2006, 2008; Fischer et al., 2007). Specifically, the axon-bearing HCs express Lhx1, fibroblast growth factor 19 (Francisco-Morcillo et al., 2005; Okamoto et al., 2009), and calretinin (Edqvist et al., 2008) while both axon-less subtypes lack the expression of these markers, but instead expresses Isl1 (Edqvist et al., 2008; Figure 1). Based on these observations, Lhx1 and Isl1 have emerged as markers for the axon-bearing and axon-less HC subpopulations, respectively.
The axon-less H2 and H3 populations can be distinguished based on their expression of GABA and the nerve growth factor receptor tyrosine kinase TrkA, respectively (Karlsson et al., 1998, 2001; Edqvist and Hallböök, 2004; Boije et al., 2008). Although HCs are often referred to as GABAergic inhibitory interneurons GABA is only present in the H1 and H2 subtypes (Edqvist and Hallböök, 2004; Boije et al., 2008). Certain cadherins and connexins are also expressed in HCs in a subtype-specific pattern (Tanabe et al., 2004, 2006; Puller and Haverkamp, 2011; Pan et al., 2012).
Although Lhx1 was identified as a HC-specific marker in the retina (Liu et al., 2000), the sub-type specific quality of Lhx1 was initially unknown. Isl1 on the other hand is not uniquely expressed in HCs but is also expressed in ganglion cells, certain amacrine cells (ACs), and bipolar cells. However, the combination of Prox1 and Isl1 is exclusive to HCs. When Isl1 was first described as a novel HC subtype-marker in chicken (Edqvist et al., 2006), it was uncertain whether the Lhx1/Isl1 division of chicken HCs into axon-bearing and axon-less subtypes was an evolutionarily conserved feature in other species. Subsequent studies show that Isl1 is expressed in HCs in retinas of various species including fish, frogs, turtles, and pigs (Francisco-Morcillo et al., 2006; Guduric-Fuchs et al., 2009; Álvarez-Hernán et al., 2013; Zhang et al., 2013; Table 1). Taken together, this suggests an evolutionary conserved division of Lhx1 and Isl1 into different HC subtypes that confirms the morphologically based subdivision.
Fate Specification of Horizontal Cells Rely on FoxN4 and Ptf1a
Although several bHLH and homeodomain TFs (e.g., Ascl3, Ngn2, NeuroD1, Atoh3, Pax6, Onecut1 and 2, and Six3) have been associated with HC genesis, all of these required combinatorial over-expression or knock-out in order to affect the HC population (Inoue et al., 2002; Akagi et al., 2004; Wang and Harris, 2005; Wu et al., 2013). Prox1 has been proposed to be crucial for the generation of HCs, though its relatively late onset of expression suggests that it is more likely involved during maturation rather than fate commitment (Dyer et al., 2003; Boije et al., 2008, 2009). However, the complete abolishment of HCs in retinas lacking the winged helix TF FoxN4 indicated that there indeed may be a key fate determinant for HCs (Li et al., 2004). The expression pattern of FoxN4 in the developing retina is conserved in mouse, frog, chicken, and zebrafish (Gouge et al., 2001; Danilova et al., 2004; Schuff et al., 2006; Boije et al., 2008). FoxN4 is expressed throughout the neural epithelium of the optic cup but is upregulated in a subset of cells that are Lhx1 positive suggesting a commitment down the HC path. Knock-out of the bHLH Ptf1a, a down-stream target of FoxN4, turned out to mimic the phenotype of the FoxN4 mutant with a complete loss of HCs and a drastic decrease of ACs (Li et al., 2004; Fujitani et al., 2006). Subsequent studies of FoxN4 and Ptf1a revealed that they are not only required, but also sufficient, for the generation of HCs and ACs (Dullin et al., 2007; Lelièvre et al., 2011; Boije et al., 2013).
Clearly, both FoxN4 and Ptf1a are required for the formation of HCs, but equally so for the generation of ACs. How does this FoxN4/Ptf1a-lineage divide into two closely related, but functionally distinct, cell types? In mice, which only possess the axon-bearing HC subtype, Lhx1 appeared as an obvious candidate for lineage diversification since it uniquely labels all HCs. Conditional Lhx1 mutant mice showed that its ablation caused ectopic HCs with a morphology resembling that of ACs (Poché et al., 2007). Expression of Ptf1a and Prox1 was initiated as in wild type HCs but the retrograde migration from the basal side (see paragraph regarding HC migration) was not performed. Although the ectopic HCs adopted an AC morphology they did not express AC specific markers suggesting proper fate commitment had occurred but that they had an erroneous laminar position (Poché et al., 2007). This indicated that Lhx1 may be a crucial homeodomain protein for the positioning of HCs in the mouse retina. It also indicated that Lhx1 expression is not required for the expression of either Ptf1a or Prox1. The fact that Lhx1 expression is observed during apical mitoses in the chicken retina, prior to the onset of Ptf1a expression, highlights a common mis-perception of the temporal aspect of these genes (Boije et al., 2013). Instead this suggests a process where Ptf1a and Lhx1 are both required but independently regulated in order to specify HCs.
Lineage analysis in the zebrafish retina using transgenic lines driving fluorescent reporter genes under the promoters of Lhx1 and Ptf1a suggests how independent expression of key factors may regulate HC fate assignment. Two things stand out regarding the Lhx1-lineage: firstly, all HC subtypes arise from the Lhx1-lineage, and secondly, most cells of this lineage do not become HCs but adopt the PR fate (Boije et al., 2015). A recent study by the Wong lab further stresses this close relationship between HCs and PRs (Suzuki et al., 2013). Quantification of the different lineages suggested that the generation of the HCs could be explained as the intersectional population of the independent expression of Lhx1 and Ptf1a (Figure 2A; Boije et al., 2015), while cells that expressed only Ptf1a or only Lhx1 become ACs or PRs, respectively. Recalling the conditional Lhx1 mutant mice, there seem to be two pieces to the puzzle; Ptf1a to drive differentiation and Lhx1 to govern positioning.
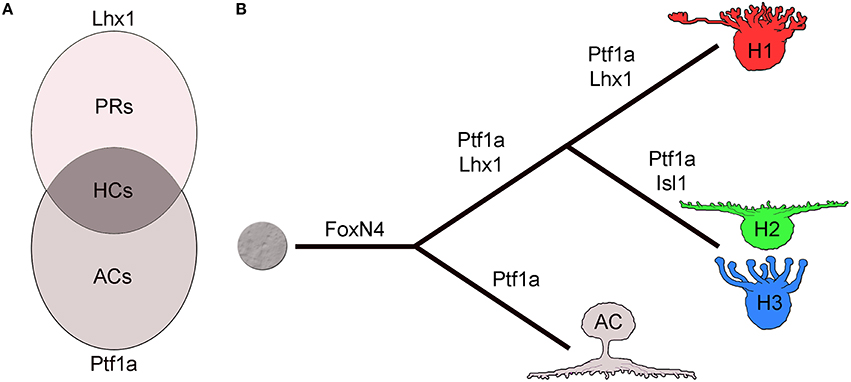
Figure 2. Horizontal cell fate and cell type formation. (A) Horizontal cell fate assignment can be regarded as the intersectional population of the independent expression of Ptf1a and Lhx1. (B) The pathway to horizontal cells is linked to amacrine cells by the expression of FoxN4 and Ptf1a while Lhx1 separates the HC lineage from ACs. Isl1 blocks Lhx1 expression to form the axon-less subtypes. AC; amacrine cell, HC; horizontal cell, H1-3; horizontal cell type 1-3, PR; photoreceptor.
Horizontal Cell Subtype Formation
As the presence of both axon-bearing and axon-less HCs seems to be an evolutionarily conserved feature, it is natural to question how these subtypes arise. While FoxN4 specifies subtype identity in the spinal cord this does not appear to be the case in the retina (Li et al., 2005). Knock-out or over-expression of FoxN4, affected the fate assignment of V2a vs. V2b spinal interneurons via the Notch-Delta pathway (Li et al., 2005; Del Barrio et al., 2007). In the retina however, FoxN4 activates Dll4-Notch signaling suppressing PR fate but it does not seem to play a role in HC subtype formation as both Lhx1 positive (+) and Isl1+ HCs were lost in the mutant and both subtypes were generated by FoxN4 overexpression (Boije et al., 2013). Similarly, Ptf1a has been suggested to determine GABAergic cell fate in the spinal cord and in the cerebellum (Glasgow et al., 2005; Hoshino et al., 2005). In contrast to the mouse retina, which only has the Lhx1 expressing GABAergic HC subtype, the chicken retina also has non GABAergic HCs expressing Isl1. However, Ptf1a overexpression led to an increase in both GABAergic Lhx1 positive HCs and in TrkA, Isl1 double positive HC subtypes (Lelièvre et al., 2011). This suggests that Ptf1a is involved in assigning inhibitory neurons in the retina, rather than specifying GABAergic subtypes.
Since recent lineage analysis implies a common origin of the HC subtypes in zebrafish there may be intrinsic and/or extrinsic factors responsible for the progression from generating one subtype to generating the next. Birth-dating analysis in the chicken retina has revealed that the axon-bearing population is born roughly 1 day prior to the axon-less subtypes (Edqvist et al., 2008). Furthermore, studies in the chicken retina have shown that Isl1, which is the molecular signature of the later born axon-less HC subtypes, is necessary and sufficient to down-regulate the expression of Lhx1 and inducing the phenotypic trait of axon-less HCs (Suga et al., 2009). Overexpression of Isl1 in HCs residing in the HC layer represses endogenous Lhx1 expression and cause a subtype fate switch from the H1 morphology to the H2 but not to H3 (Suga et al., 2009). Expression of a dominant negative Lhx1 variant does not cause subtype fate-switch and overexpression of Lhx1 did not affect the proportion of the HC subtypes. During normal development there are occasionally cells that are double labeled for Lhx1 and Isl1 during the migration to the basal side (Boije et al., 2009). Combined, the lineage data and the dominance of Isl1 over Lhx1, suggests that there is a pool of committed HCs that initiates expression of Isl1 causing a subtype fate-switch (Figure 2B). How this is regulated to produce the 50/50 split in the chicken retina remains unknown. Interestingly, in the mouse retina, which only hosts the Lhx1+ subtype, the number of HCs was found to be inversely dependent on the expression of Isl1 (Whitney et al., 2011). This was shown by the identification of an expression quantitative trait locus in the Isl1-gene from genetic analysis of mouse strains that have inherently different HC numbers. These findings are consistent with a role for Isl1 in regulating the formation or population size of the Lhx1+ subtype and such a role has also been found during the formation of other neuronal subtype-populations (Sun et al., 2008).
Horizontal Cell Migration
As RPCs pass through the cell cycle their nuclei undergo translocation across the neuroepithelium, a process known as interkinetic nuclear migration, while remaining attached to the apical and basal lamina (Baye and Link, 2007). Mitoses typically occur on the apical side of the neuroepithelium and after a neurogenic division the post-mitotic daughter cells detach and migrate toward their final laminar location (Götz and Huttner, 2005). Based on this, one would expect the newborn HCs to simply migrate the short distance from the apical side, to the outer part of the prospective inner nuclear layer, where they reside in the mature retina. However, HCs deviate from this path by migrating from their site of birth across the width of the neuroepithelium and halt near the prospective ganglion cell layer before migrating back again toward their final laminar location (Edqvist and Hallböök, 2004). This phenomenon has also been described in mouse (Liu et al., 2000) and zebrafish (Chow et al., 2015), and the presence of displaced HCs in various species (cat, chicken, macaque, augoti, rabbit, capybara) suggest that this migration pattern may be an evolutionarily conserved HC feature (Prada et al., 1984; Silveira et al., 1989; Wässle et al., 2000). Subsequent studies in the chicken retina showed that H1, H2, and H3 HCs all undertake the same route of migration, but the migration of H1 and H2/H3 is temporally separated by approximately 1 day (Edqvist et al., 2008). The separation in migration correlates with a similar difference regarding birth-dates, indicating that the subtypes are not generated during the maturation process but rather that cells are born into a specific subtype fate. This notion is supported by the fact that Isl1, which specifies HC-subtype, is already expressed during the birth of HCs. A similar phenomenon is known from AC subtype formation in the rat retina where GABAergic ACs are born 2–3 days before acetylcholine and dopamine expressing ACs (Lee et al., 1999).
Why do HCs in the Lhx1 loss-of-function mouse retina adopt AC morphology in the absence of the retrograde migration despite not adopting AC molecular characteristics (Poché et al., 2007)? This may either reflect that the morphological maturation of HCs solely depends on Lhx1 or that there are environmental cues governing this step. Interestingly, recent results show that wild type HCs transplanted into retinas lacking HCs and ACs also fail the retrograde migration (Boije et al., 2015). These HCs are intrinsically wild type with the competence to migrate back but they never the less fail. This phenomenon may be due to the absence of a non-cell autonomous factor, possibly arising from ACs. Interestingly, there seems to exist some regulatory mechanism in which a certain number of ACs has to be present for the retrograde migration of HCs to occur (Boije et al., 2015). Furthermore, these HCs also adopted an AC morphology and extended processes into the inner plexiform layer. This suggests that HC morphological maturation relies, at least partly, on extrinsic cues. One can hypothesize that there may have been an ancient inhibitory neuron specified by Ptf1a expression and that the addition of Lhx1 has split this population in two, the HCs and the ACs. The migration of HCs may therefore be an evolutionary remnant from when these cells were destined to reside together.
Heterogeneity During the Final Cell Cycle of Horizontal Cells
Once a cell undergoes the neurogenic division, the daughter cells become post-mitotic, migrate out to their final laminar position, and initiate differentiation. While this is true for several of the retinal neuronal cell types, chicken HCs can divide once more after having initiated their final migration (Boije et al., 2009). These non-apical mitoses occur on the basal side of the retinal neuroepithelium in a semi differentiated state. However, not all HCs act in this way and three different behaviors have been described during the terminal cell cycle. The first behavior, denoted behavior “one,” resembles that of the RPCs, with an apical mitosis followed by migration and accumulation on the basal side of the retina where they rest for several days before migrating back to the prospective HC layer. HCs with behavior “two” initiate a non-apical neurogenic cell cycle by entering S-phase, however they do not proceed into mitosis and remain with a replicated genome. The presence of such cells was shown by the existence of increased DNA content and double number of chromosomes in post-mitotic HCs (Shirazi Fard et al., 2013). However, the majority of HCs perform a non-apical (basal) mitosis that generates two HCs. Clonal analysis has shown that individually labeled HC progenitors often generated two HCs of the same subtype indicating that the assignment of subtype in many cases occurs prior to the terminal division (Rompani and Cepko, 2008). The non-apical mitoses are denoted behavior “three” and occur later than the behavior “one” and “two”-cells, although there is extensive overlap between the three behaviors (Figure 3). Independent of behavior during the final cell cycle, all HCs undergo retrograde migration and form the prospective HC layer in proximity to the PRs (Shirazi Fard et al., 2013). These behaviors have so far only been carefully studied in the chicken H1 subtype but it is likely that the H2 and H3 HC subtypes have similar behaviors during their neurogenic cell division since they all exhibit basal mitoses in a similar fashion as the H1 HCs do (Boije et al., 2009).
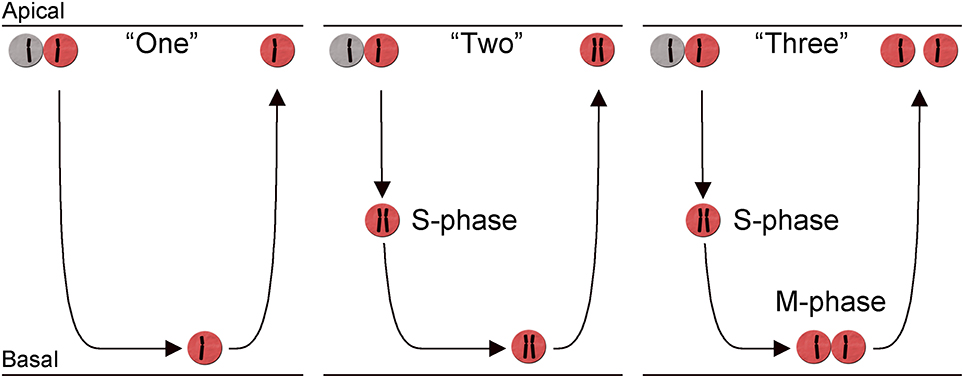
Figure 3. Heterogeneity in the terminal cell division of horizontal cells. Schematic diagram showing three different behaviors during the neurogenic cell division of chicken horizontal H1 (Lhx1+) cells. The diagram illustrates the retina with mitosis (M-phase) and S-phase indicated in relation to the migration across the retina. The migration is indicated by the arrows and the replicated state of each cell, in the three behaviors is indicated by a schematic chromosome. Two chromosomes indicate a cell with replicated DNA. The three different behaviors are denoted: behavior “One,” “Two,” and “Three” in the main text.
A similar heterogeneity during the neurogenic HC division is seen in the zebrafish retina. HCs divide non-apically on the basal side of the zebrafish retina (Weber et al., 2014) or in the HC layer (Godinho et al., 2007). Such mitoses resemble the behavior “three” cells in the chicken retina. In zebrafish HCs are occasionally generated together with a PR as its sister cell and this pattern may represent the behavior “one” with an apical cell division (He et al., 2012). There have been no reports indicating any heterogeneity during the neurogenic cell divisions of either mouse or rat HCs. They migrate and accumulate on the basal side of the retina and stay on the basal side as post-mitotic cells before their retrograde migration to the prospective HC layer. This conclusion finds support in that the rodent retina only has H1 HCs (Peichl and González-Soriano, 1994; Poché and Reese, 2009) and their behavior during the neurogenic cell division corresponds to behavior “one” cells in the chicken retina (Liu et al., 2000).
Cells that fail to enter mitosis after genome replication, similar to that seen for the behavior “two” HCs, are often associated with DNA damage and an active DNA damage response (DDR; Zhou and Elledge, 2000). However, the arrested chicken HCs are not a result of an active DDR since they do not display phosphorylation of histone H2AX nor Rad51 foci, which are hallmarks of an active DDR and repair (Shirazi Fard et al., 2013). Rather, the heteroploid cells are produced by a mechanism referred to as endoreplication (Edgar and Orr-Weaver, 2001). Overall, endoreplication and its regulation is poorly understood, but it has been linked to cells that have initiated differentiation while remaining in the cell cycle (Zanet et al., 2010). It has also been suggested that endoreplication promotes resistance against intrinsic and extrinsic stress (Lee et al., 2009) a feature that may be important to the HCs since their numbers are determined by proliferation rather than apoptosis.
Chromosomal variations in adult tissues are common, and aneuploidy (one form of heteroploidy) has been found in developing and adult cortical interneurons (Rehen et al., 2001; Kingsbury et al., 2005) as well as in chicken retinal ganglion cells (Morillo et al., 2010). However, in contrast to HCs, most aneuploid cells undergo caspase-mediated apoptosis (Voullaire et al., 2000; Rehen et al., 2001; Kingsbury et al., 2005; Rajendran et al., 2008; Zupanc et al., 2009; Peterson et al., 2012). Although the heteroploid HCs appear resistant to apoptosis, they respond to drug-induced DNA damage by phosphorylation of histone H2AX (γH2AX) and formation of γH2AX and RAD51 foci, suggesting a functional DNA damage response (Shirazi Fard et al., 2014a). Still the chicken HCs were able to enter S-phase and complete mitosis (Shirazi Fard et al., 2014b). This ability to enter mitosis in the presence of DNA damage indicates that these cells are able to withstand the effect of the DNA damage response (Shirazi Fard et al., 2014b, 2015). Furthermore, direct activation of p53 by its co-activator Zac1 neither induces cell cycle arrest nor apoptosis in HCs supporting the notion that the HCs are less sensitive to events that activate the p53 system, compared to other retinal cells (Shirazi Fard et al., 2015). These properties may associate with a cell that has the ability to become neoplastic, in fact conditionally inactivating the retinoblastoma Rb1 gene in early murine retinal progenitors leads to degeneration of all retinal cells except HCs, which persist and divide with DNA damage resulting in poly- or aneuploidy (Donovan and Corbo, 2012).
Horizontal Cells and Retinoblastoma
The childhood cancer Retinoblastoma is rare, with a reported incidence of 1 in 15–18,000 births. Since the discovery of RB1 mutations, much effort has been invested into finding the cell-of-origin for retinoblastoma and understanding the carcinogenic mechanisms in those cells. Knock-down of RB1 in human fetal retinal cells was sufficient to induce proliferation of cone PR cells and when grafted, these Rb1-depleted cone precursors were able to form tumors (Xu et al., 2014). This indicates that post-mitotic human cone precursors are sensitive to RB1-depletion and may represent cell-of-origin for retinoblastoma. However, a study performed on mice lacking Rb-family members, reported that HCs are able to re-enter the cell cycle, expand clonally and form metastatic tumors (Ajioka et al., 2007). Horizontal cells may therefore also be considered as a cell-of-origin for retinoblastoma. This poses the question why certain cell types are more prone to becoming malignant following loss-of-function of RB1. As discussed in this review, both the cone PRs and the HCs are among the first retinal cells to be generated during development and they are derived from the same multipotent progenitors (Suzuki et al., 2013; Boije et al., 2014). Whether a photoreceptor or a horizontal cell is the cell-of-origin for retinoblastoma may be less important from a mechanistic perspective. It is more important to understand the molecular pathways that distinguish the properties of these cells from other retinal cells, knowledge that may aid our understanding why these cells have a propensity for neoplastic transformation. In conclusion, HCs seem to have an atypical regulation or execution of their p53-p21 system during a limited period of the development that spans the time during which their neurogenic cell division occurs and that allows for genomic aberrations without triggering apoptosis. Such ability may be a factor that under challenging conditions, allows cells with RB1 loss-of-function mutations to remain in the retina and to develop into cancer initiating cells.
Summary
- There is a clear correlation between the morphologically defined HC subtypes and the classification based on molecular markers, which is evolutionarily conserved; the transcription factor Lhx1 is expressed in axon-bearing subtypes and Isl1 in axon-less subtypes.
- From an evolutionary perspective it seems that while most species have both axon-bearing and axon-less HCs, some animals may have lost the need for axon-less HCs due to adaptation to e.g., nocturnal lifestyles.
- FoxN4 and Ptf1a are crucial in establishing the competence to differentiate into a HC.
- While the Ptf1a-lineage is shared by HCs and ACs, the presence of Lhx1, which is expressed independently of Ptf1a, divides the lineage and thereby aids in the formation of HCs.
- The Lhx1-lineage gives rise to all HC subtypes and onset of Isl1 expression within this lineage drives the phenotypic change to axon-less subtypes.
- During development, committed HC progenitors undertake a bi-directional migration across the width of the retina during which non-apical mitoses occur in some species.
- The ability of HCs to undergo additional rounds of division in a differentiated state may be related to HCs being named the cell-of-origin for retinoblastoma.
Author Contributions
Conceived the review: HB, SS, PE, FH. Wrote the paper: HB, SS, PE, FH.
Funding
Funding was provided by the Swedish Research Council, Medicine and Health.
Conflict of Interest Statement
The authors declare that the research was conducted in the absence of any commercial or financial relationships that could be construed as a potential conflict of interest.
References
Ahnelt, P. K., and Kolb, H. (2000). The mammalian photoreceptor mosaic-adaptive desisgn. Prog. Retin. Eye Res. 19, 711–777. doi: 10.1016/S1350-9462(00)00012-4
Ajioka, I., Martins, R. A. P., Bayazitov, I. T., Donovan, S., Johnson, D. A., Frase, S., et al. (2007). Differentiated horizontal interneurons clonally expand to form metastatic retinoblastoma in mice. Cell 131, 378–390. doi: 10.1016/j.cell.2007.09.036
Akagi, T., Inoue, T., Miyoshi, G., Bessho, Y., Takahashi, M., Lee, J. E., et al. (2004). Requirement of multiple basic helix-loop-helix genes for retinal neuronal subtype specification. J. Biol. Chem. 279, 28492–28498. doi: 10.1074/jbc.M400871200
Álvarez-Hernán, G., Bejarano-Escobar, R., Morona, R., González, A., Martín-Partido, G., and Francisco-Morcillo, J. (2013). Islet-1 immunoreactivity in the developing retina of Xenopus laevis. ScientificWorldJournal 2013:740420. doi: 10.1155/2013/740420
Baye, L. M., and Link, B. A. (2007). Interkinetic nuclear migration and the selection of neurogenic cell divisions during vertebrate retinogenesis. J. Neurosci. 27, 10143–10152. doi: 10.1523/JNEUROSCI.2754-07.2007
Bennis, M., Versaux-Botteri, C., Repérant, J., Armengol, J. A., and Ward, R. (2003). Gamma-aminobutyric acid-synthesizing cells in the retina of the chameleon Chamaeleo chameleon. J. Neurosci. Res. 73, 410–415. doi: 10.1002/jnr.10665
Bloomfield, S. A., and Miller, R. F. (1982). A physiological and morphological study of the horizontal cell types of the rabbit retina. J. Comp. Neurol. 208, 288–303. doi: 10.1002/cne.902080306
Boije, H., Edqvist, P.-H. D., and Hallböök, F. (2008). Temporal and spatial expression of transcription factors FoxN4, Ptf1a, Prox1, Isl1 and Lim1 mRNA in the developing chick retina. Gene Expr. Patterns 8, 117–123. doi: 10.1016/j.modgep.2007.09.004
Boije, H., Edqvist, P.-H. D., and Hallböök, F. (2009). Horizontal cell progenitors arrest in G2-phase and undergo terminal mitosis on the vitreal side of the chick retina. Dev. Biol. 330, 105–113. doi: 10.1016/j.ydbio.2009.03.013
Boije, H., MacDonald, R. B., and Harris, W. A. (2014). Reconciling competence and transcriptional hierarchies with stochasticity in retinal lineages. Curr. Opin. Neurobiol. 27, 68–74. doi: 10.1016/j.conb.2014.02.014
Boije, H., Rulands, S., Dudczig, S., Simons, B. D., and Harris, W. A. (2015). The independent probabilistic firing of transcription factors: a paradigm for clonal variability in the zebrafish retina. Dev. Cell 34, 532–543. doi: 10.1016/j.devcel.2015.08.011
Boije, H., Shirazi Fard, S., Ring, H., and Hallböök, F. (2013). Forkheadbox N4 (FoxN4) triggers context-dependent differentiation in the developing chick retina and neural tube. Differentiation 85, 11–19. doi: 10.1016/j.diff.2012.12.002
Boycott, B. B., Peichl, L., and Wässle, H. (1978). Morphological types of horizontal cell in the retina of the domestic cat. Proc. R. Soc. Lond. B Biol. Sci. 203, 229–245. doi: 10.1098/rspb.1978.0103
Chan, T. L., Goodchild, A. K., and Martin, P. R. (1997). The morphology and distribution of horizontal cells in the retina of a New World monkey, the marmoset Callithrix jacchus: a comparison with macaque monkey. Vis. Neurosci. 14, 125–140. doi: 10.1017/S0952523800008828
Chow, R. W., Almeida, A. D., Randlett, O., Norden, C., and Harris, W. A. (2015). Inhibitory neuron migration and IPL formation in the developing zebrafish retina. Development 142, 2665–2677. doi: 10.1242/dev.122473
Connaughton, V. P., Graham, D., and Nelson, R. (2004). Identification and morphological classification of horizontal, bipolar, and amacrine cells within the zebrafish retina. J. Comp. Neurol. 477, 371–385. doi: 10.1002/cne.20261
Cuenca, N., Deng, P., Linberg, K. A., Lewis, G. P., Fisher, S. K., and Kolb, H. (2002). The neurons of the ground squirrel retina as revealed by immunostains for calcium binding proteins and neurotransmitters. J. Neurocytol. 31, 649–666. doi: 10.1023/A:1025791512555
Dacheux, R. F., and Raviola, E. (1982). Horizontal cells in the retina of the rabbit. J. Neurosci. 2, 1486–1493.
Danilova, N., Visel, A., Willett, C. E., and Steiner, L. A. (2004). Expression of the winged helix/forkhead gene, foxn4, during zebrafish development. Brain Res. Dev. Brain Res. 153, 115–119. doi: 10.1016/j.devbrainres.2004.05.014
Del Barrio, M. G., Taveira-Marques, R., Muroyama, Y., Yuk, D.-I., Li, S., Wines-Samuelson, M., et al. (2007). A regulatory network involving Foxn4, Mash1 and delta-like 4/Notch1 generates V2a and V2b spinal interneurons from a common progenitor pool. Development 134, 3427–3436. doi: 10.1242/dev.005868
de Lima, S. M. A., Ahnelt, P. K., Carvalho, T. O., Silveira, J. S., Rocha, F. A., Saito, C. A., et al. (2005). Horizontal cells in the retina of a diurnal rodent, the agouti (Dasyprocta aguti). Vis. Neurosci. 22, 707–720. doi: 10.1017/S0952523805226032
Donovan, S. L., and Corbo, J. C. (2012). Retinal horizontal cells lacking Rb1 sustain persistent DNA damage and survive as polyploid giant cells. Mol. Biol. Cell 23, 4362–4372. doi: 10.1091/mbc.E12-04-0293
dos Reis, J. W. L., de Carvalho, W. A., Saito, C. A., and Silveira, L. C. L. (2002). Morphology of horizontal cells in the retina of the capuchin monkey, Cebus apella: how many horizontal cell classes are found in dichromatic primates? J. Comp. Neurol. 443, 105–123. doi: 10.1002/cne.10105
Dos Santos, S. N., Dos Reis, J. W. L., Da Silva Filho, M., Kremers, J., and Silveira, L. C. L. (2005). Horizontal cell morphology in nocturnal and diurnal primates: a comparison between owl-monkey (Aotus) and capuchin monkey (Cebus). Vis. Neurosci. 22, 405–415. doi: 10.1017/S0952523805224033
Dowling, J. E., Pak, M. W., and Lasater, E. M. (1985). White perch horizontal cells in culture: methods, morphology and process growth. Brain Res. 360, 331–338. doi: 10.1016/0006-8993(85)91250-8
Dullin, J.-P., Locker, M., Robach, M., Henningfeld, K. A., Parain, K., Afelik, S., et al. (2007). Ptf1a triggers GABAergic neuronal cell fates in the retina. BMC Dev. Biol. 7:110. doi: 10.1186/1471-213X-7-110
Dyer, M. A., Livesey, F. J., Cepko, C. L., and Oliver, G. (2003). Prox1 function controls progenitor cell proliferation and horizontal cell genesis in the mammalian retina. Nat. Genet. 34, 53–58. doi: 10.1038/ng1144
Edgar, B. A., and Orr-Weaver, T. L. (2001). Endoreplication cell cycles: more for less. Cell 105, 297–306. doi: 10.1016/S0092-8674(01)00334-8
Edqvist, P.-H. D., and Hallböök, F. (2004). Newborn horizontal cells migrate bi-directionally across the neuroepithelium during retinal development. Development 131, 1343–1351. doi: 10.1242/dev.01018
Edqvist, P.-H. D., Lek, M., Boije, H., Lindbäck, S. M., and Hallböök, F. (2008). Axon-bearing and axon-less horizontal cell subtypes are generated consecutively during chick retinal development from progenitors that are sensitive to follistatin. BMC Dev. Biol. 8:46. doi: 10.1186/1471-213X-8-46
Edqvist, P. H. D., Myers, S. M., and Hallböök, F. (2006). Early identification of retinal subtypes in the developing, pre-laminated chick retina using the transcription factors Prox1, Lim1, Ap2alpha, Pax6, Isl1, Isl2, Lim3 and Chx10. Eur. J. Histochem. 50, 147–154.
Elshatory, Y., Deng, M., Xie, X., and Gan, L. (2007). Expression of the LIM-homeodomain protein Isl1 in the developing and mature mouse retina. J. Comp. Neurol. 503, 182–197. doi: 10.1002/cne.21390
Famiglietti, E. V. (1990). A new type of wide-field horizontal cell, presumably linked to blue cones, in rabbit retina. Brain Res. 535, 174–179. doi: 10.1016/0006-8993(90)91839-9
Ferreiro-Galve, S., Rodríguez-Moldes, I., and Candal, E. (2010). Calretinin immunoreactivity in the developing retina of sharks: comparison with cell proliferation and GABAergic system markers. Exp. Eye Res. 91, 378–386. doi: 10.1016/j.exer.2010.06.011
Fischer, A. J., Stanke, J. J., Aloisio, G., Hoy, H., and Stell, W. K. (2007). Heterogeneity of horizontal cells in the chicken retina. J. Comp. Neurol. 500, 1154–1171. doi: 10.1002/cne.21236
Francisco-Morcillo, J., Hidalgo-Sánchez, M., and Martín-Partido, G. (2006). Spatial and temporal patterns of proliferation and differentiation in the developing turtle eye. Brain Res. 1103, 32–48. doi: 10.1016/j.brainres.2006.05.052
Francisco-Morcillo, J., Sánchez-Calderón, H., Kawakami, Y., Izpisúa Belmonte, J. C., Hidalgo-Sánchez, M., and Martín-Partido, G. (2005). Expression of Fgf19 in the developing chick eye. Brain Res. Dev. Brain Res. 156, 104–109. doi: 10.1016/j.devbrainres.2004.12.011
Fujitani, Y., Fujitani, S., Luo, H., Qiu, F., Burlison, J., Long, Q., et al. (2006). Ptf1a determines horizontal and amacrine cell fates during mouse retinal development. Development 133, 4439–4450. doi: 10.1242/dev.02598
Gallego, A. (1971). Horizontal and amacrine cells in the mammal's retina. Vision Res. 11(Suppl.3), 33–50. doi: 10.1016/0042-6989(71)90029-0
Gallego, A. (1986). Chapter 7 Comparative studies on horizontal cells and a note on microglial cells. Prog. Retin. Res. 5, 165–206.
Gallego, A., Baron, M., and Gayoso, M. (1975). Horizontal cells of the avian retina. Vision Res. 15, 1029–1030. doi: 10.1016/0042-6989(75)90247-3
Genis-Galves, J., Prada, F., and Armengol, J. (1979). Evidence of three types of horizontal cells in the chick retina. Jpn. J. Ophtamol. 23, 378–387.
Glasgow, S. M., Henke, R. M., MacDonald, R. J., Wright, C. V. E., and Johnson, J. E. (2005). Ptf1a determines GABAergic over glutamatergic neuronal cell fate in the spinal cord dorsal horn. Development 132, 5461–5469. doi: 10.1242/dev.02167
Godinho, L., Williams, P. R., Claassen, Y., Provost, E., Leach, S. D., Kamermans, M., et al. (2007). Nonapical symmetric divisions underlie horizontal cell layer formation in the developing retina in vivo. Neuron 56, 597–603. doi: 10.1016/j.neuron.2007.09.036
Götz, M., and Huttner, W. B. (2005). The cell biology of neurogenesis. Nat. Rev. Mol. Cell Biol. 6, 777–788. doi: 10.1038/nrm1739
Gouge, A., Holt, J., Hardy, A. P., Sowden, J. C., and Smith, H. K. (2001). Foxn4–a new member of the forkhead gene family is expressed in the retina. Mech. Dev. 107, 203–206. doi: 10.1016/S0925-4773(01)00465-8
Guduric-Fuchs, J., Ringland, L. J., Gu, P., Dellett, M., Archer, D. B., and Cogliati, T. (2009). Immunohistochemical study of pig retinal development. Mol. Vis. 15, 1915–1928.
Hack, I., and Peichl, L. (1999). Horizontal cells of the rabbit retina are non-selectively connected to the cones. Eur. J. Neurosci. 11, 2261–2274. doi: 10.1046/j.1460-9568.1999.00647.x
Harman, A. (1994). Horizontal cells in the retina of the brush-tailed possum. Exp. Brain Res. 98, 168–171. doi: 10.1007/BF00229123
Harman, A. M., and Ferguson, J. (1994). Morphology and birth dates of horizontal cells in the retina of a marsupial. J. Comp. Neurol. 340, 392–404. doi: 10.1002/cne.903400309
He, J., Zhang, G., Almeida, A. D., Cayouette, M., Simons, B. D., and Harris, W. A. (2012). How variable clones build an invariant retina. Neuron 75, 786–798. doi: 10.1016/j.neuron.2012.06.033
Hendrickson, A., Yan, Y.-H., Erickson, A., Possin, D., and Pow, D. (2007). Expression patterns of calretinin, calbindin and parvalbumin and their colocalization in neurons during development of Macaca monkey retina. Exp. Eye Res. 85, 587–601. doi: 10.1016/j.exer.2007.07.011
Hirt, B., and Wagner, H.-J. (2005). The organization of the inner retina in a pure-rod deep-sea fish. Brain Behav. Evol. 65, 157–167. doi: 10.1159/000083625
Hokoc, J. N., Oliveira, M. M. M., and Ahnelt, P. (1993). Three types of horizontal cells in a primitive mammal, the opossum (Didelphis marsupialis aurita): a Golgi-LM study. Invest. Ophthalmol. Vis. Sci. 34(Suppl.), 1152.
Hombach, S., Janssen-Bienhold, U., Söhl, G., Schubert, T., Büssow, H., Ott, T., et al. (2004). Functional expression of connexin57 in horizontal cells of the mouse retina. Eur. J. Neurosci. 19, 2633–2640. doi: 10.1111/j.0953-816X.2004.03360.x
Hoshino, M., Nakamura, S., Mori, K., Kawauchi, T., Terao, M., Nishimura, Y. V., et al. (2005). Ptf1a, a bHLH transcriptional gene, defines GABAergic neuronal fates in cerebellum. Neuron 47, 201–213. doi: 10.1016/j.neuron.2005.06.007
Inoue, T., Hojo, M., Bessho, Y., Tano, Y., Lee, J. E., and Kageyama, R. (2002). Math3 and NeuroD regulate amacrine cell fate specification in the retina. Development 129, 831–842.
Kamiji, N. L., Yamamoto, K., Hirasawa, H., Yamada, M., Usui, S., and Kurokawa, M. (2012). Proton feedback mediates the cascade of color-opponent signals onto H3 horizontal cells in goldfish retina. Neurosci. Res. 72, 306–315. doi: 10.1016/j.neures.2012.01.008
Kaneko, A. (1970). Physiological and morphological identification of horizontal, bipolar and amacrine cells in goldfish retina. J. Physiol. 207, 623–633. doi: 10.1113/jphysiol.1970.sp009084
Karlsson, M., Clary, D. O., Lefcort, F. B., Reichardt, L. F., Karten, H. J., and Hallböök, F. (1998). Nerve growth factor receptor TrkA is expressed by horizontal and amacrine cells during chicken retinal development. J. Comp. Neurol. 400, 408–416. doi: 10.1002/(SICI)1096-9861(19981026)400:3<408::AID-CNE9>3.0.CO;2-C
Karlsson, M., Mayordomo, R., Reichardt, L. F., Catsicas, S., Karten, H., and Hallböök, F. (2001). Nerve growth factor is expressed by postmitotic avian retinal horizontal cells and supports their survival during development in an autocrine mode of action. Development 128, 471–479.
Kim, H. G., and Miller, R. F. (1992). Physiological and morphological correlations of horizontal cells in the mudpuppy retina. J. Neurophysiol. 67, 829–840.
Kingsbury, M. A., Friedman, B., McConnell, M. J., Rehen, S. K., Yang, A. H., Kaushal, D., et al. (2005). Aneuploid neurons are functionally active and integrated into brain circuitry. Proc. Natl. Acad. Sci. U.S.A. 102, 6143–6147. doi: 10.1073/pnas.0408171102
Knabe, W., and Kuhn, H. J. (2000). Capillary-contacting horizontal cells in the retina of the tree shrew Tupaia belangeri belong to the mammalian type A. Cell Tissue Res. 299, 307–311.
Kolb, H. (1995). “Outer plexiform layer,” in Webvision: The Organization of the Retina and Visual System, eds H. Kolb, E. Fernandez, and R. Nelson (Salt Lake City, UT: University of Utah Health Sciences Center). (Accessed April 7, 2016).
Kolb, H., Linberg, K. A., and Fisher, S. K. (1992). Neurons of the human retina: a Golgi study. J. Comp. Neurol. 318, 147–187. doi: 10.1002/cne.903180204
Lee, H. O., Davidson, J. M., and Duronio, R. J. (2009). Endoreplication: polyploidy with purpose. Genes Dev. 23, 2461–2477. doi: 10.1101/gad.1829209
Lee, M. Y., Shin, S. L., Han, S. H., and Chun, M. H. (1999). The birthdates of GABA-immunoreactive amacrine cells in the rat retina. Exp. Brain Res. 128, 309–314. doi: 10.1007/s002210050851
Leeper, H. F. (1978). Horizontal cells of the turtle retina. I. Light microscopy of Golgi preparations. J. Comp. Neurol. 182, 777–793. doi: 10.1002/cne.901820503
Leeper, H. F., and Charlton, J. S. (1985). Response properties of horizontal cells and photoreceptor cells in the retina of the tree squirrel, Sciurus carolinensis. J. Neurophysiol. 54, 1157–1166.
Lelièvre, E. C., Lek, M., Boije, H., Houille-Vernes, L., Brajeul, V., Slembrouck, A., et al. (2011). Ptf1a/Rbpj complex inhibits ganglion cell fate and drives the specification of all horizontal cell subtypes in the chick retina. Dev. Biol. 358, 296–308. doi: 10.1016/j.ydbio.2011.07.033
Li, S., Misra, K., Matise, M. P., and Xiang, M. (2005). Foxn4 acts synergistically with Mash1 to specify subtype identity of V2 interneurons in the spinal cord. Proc. Natl. Acad. Sci. U.S.A. 102, 10688–10693. doi: 10.1073/pnas.0504799102
Li, S., Mo, Z., Yang, X., Price, S. M., Shen, M. M., and Xiang, M. (2004). Foxn4 controls the genesis of amacrine and horizontal cells by retinal progenitors. Neuron 43, 795–807. doi: 10.1016/j.neuron.2004.08.041
Linberg, K. A., Suemune, S., and Fisher, S. K. (1996). Retinal neurons of the California ground squirrel, Spermophilus beecheyi: a Golgi study. J. Comp. Neurol. 365, 173–216. doi: 10.1002/(SICI)1096-9861(19960205)365:2<173::AID-CNE1>3.0.CO;2-2
Liu, C. R., Xu, L., Zhong, Y. M., Li, R. X., and Yang, X. L. (2009). Expression of connexin 35/36 in retinal horizontal and bipolar cells of carp. Neuroscience 164, 1161–1169. doi: 10.1016/j.neuroscience.2009.09.035
Liu, W., Wang, J. H., and Xiang, M. (2000). Specific expression of the LIM/homeodomain protein Lim-1 in horizontal cells during retinogenesis. Dev. Dyn. 217, 320–325. doi: 10.1002/(SICI)1097-0177(200003)217:3<320::AID-DVDY10>3.0.CO;2-F
Loeliger, M., and Rees, S. (2005). Immunocytochemical development of the guinea pig retina. Exp. Eye Res. 80, 9–21. doi: 10.1016/j.exer.2004.08.003
Lyser, K. M., Chernomorsky, R., Michalopoulos, C., and Twersky, L. H. (1999). Horizontal cell differentiation in the retina of the Brazilian opossum, Monodelphis domestica. Int. J. Dev. Neurosci. 17, 225–237. doi: 10.1016/S0736-5748(99)00004-0
Lyser, K. M., Li, A., and Nu-ez, M. (1994). Horizontal cells in the rabbit retina: differentiation of subtypes at neonatal and postnatal stages. Int. J. Dev. Neurosci. 12, 673–682. doi: 10.1016/0736-5748(94)90019-1
Mariani, A. P. (1987). Neuronal and synaptic organization of the outer plexiform layer of the pigeon retina. Am. J. Anat. 179, 25–39. doi: 10.1002/aja.1001790105
Marquardt, T., and Gruss, P. (2002). Generating neuronal diversity in the retina: one for nearly all. Trends Neurosci. 25, 32–38. doi: 10.1016/S0166-2236(00)02028-2
Masland, R. H. (2001a). The fundamental plan of the retina. Nat. Neurosci. 4, 877–886. doi: 10.1038/nn0901-877
Masland, R. H. (2001b). Neuronal diversity in the retina. Curr. Opin. Neurobiol. 11, 431–436. doi: 10.1016/S0959-4388(00)00230-0
Mayordomo, R. (2001). Differentiated horizontal cells seem not to be affected by apoptosis during development of the chick retina. Int. J. Dev. Biol. 45, S79–S80.
Mills, S. L., and Catania, K. C. (2004). Identification of retinal neurons in a regressive rodent eye (the naked mole-rat). Vis. Neurosci. 21, 107–117. doi: 10.1017/S0952523804043020
Morillo, S. M., Escoll, P., de la Hera, A., and Frade, J. M. (2010). Somatic tetraploidy in specific chick retinal ganglion cells induced by nerve growth factor. Proc. Natl. Acad. Sci. U.S.A. 107, 109–114. doi: 10.1073/pnas.0906121107
Müller, B., and Peichl, L. (1993). Horizontal cells in the cone-dominated tree shrew retina: morphology, photoreceptor contacts, and topographical distribution. J. Neurosci. 13, 3628–3646.
Nag, T. C., and Wadhwa, S. (2001). Differential expression of syntaxin-1 and synaptophysin in the developing and adult human retina. J. Biosci. 26, 179–191. doi: 10.1007/BF02703642
Ogden, T. E., Mascetti, G. G., and Pierantoni, R. (1984). The internal horizontal cell of the frog. Analysis of receptor input. Invest. Ophthalmol. Vis. Sci. 25, 1382–1394.
Ogden, T. E., Mascetti, G. G., and Pierantoni, R. (1985). The outer horizontal cell of the frog retina: morphology, receptor input, and function. Invest. Ophthalmol. Vis. Sci. 26, 643–656.
Okamoto, M., Bito, T., Noji, S., and Ohuchi, H. (2009). Subtype-specific expression of Fgf19 during horizontal cell development of the chicken retina. Gene Expr. Patterns 9, 306–313. doi: 10.1016/j.gep.2009.02.007
Pan, F., Keung, J., Kim, I.-B., Snuggs, M. B., Mills, S. L., O'Brien, J., et al. (2012). Connexin 57 is expressed by the axon terminal network of B-type horizontal cells in the rabbit retina. J. Comp. Neurol. 520, 2256–2274. doi: 10.1002/cne.23060
Peichl, L. (2005). Diversity of mammalian photoreceptor properties: adaptations to habitat and lifestyle? Anat. Rec. A Discov. Mol. Cell. Evol. Biol. 287, 1001–1012. doi: 10.1002/ar.a.20262
Peichl, L., and González-Soriano, J. (1994). Morphological types of horizontal cell in rodent retinae: a comparison of rat, mouse, gerbil, and guinea pig. Vis. Neurosci. 11, 501–517. doi: 10.1017/S095252380000242X
Peichl, L., Sandmann, D., and Boycott, B. (1998). Comparative anatomy and function of mammalian horizontal cells. Nato Adv. Sci. Life 299, 147–172.
Peterson, S. E., Yang, A. H., Bushman, D. M., Westra, J. W., Yung, Y. C., Barral, S., et al. (2012). Aneuploid cells are differentially susceptible to caspase-mediated death during embryonic cerebral cortical development. J. Neurosci. 32, 16213–16222. doi: 10.1523/JNEUROSCI.3706-12.2012
Poché, R. A., Kwan, K. M., Raven, M. A., Furuta, Y., Reese, B. E., and Behringer, R. R. (2007). Lim1 is essential for the correct laminar positioning of retinal horizontal cells. J. Neurosci. 27, 14099–14107. doi: 10.1523/JNEUROSCI.4046-07.2007
Poché, R. A., and Reese, B. E. (2009). Retinal horizontal cells: challenging paradigms of neural development and cancer biology. Development 136, 2141–2151. doi: 10.1242/dev.033175
Prada, F. A., Armengol, J. A., and Génis-Gálvez, J. M. (1984). Displaced horizontal cells in the chick retina. J. Morphol. 182, 221–225. doi: 10.1002/jmor.1051820208
Puller, C., and Haverkamp, S. (2011). Cell-type-specific localization of protocadherin β16 at AMPA and AMPA/Kainate receptor-containing synapses in the primate retina. J. Comp. Neurol. 519, 467–479. doi: 10.1002/cne.22528
Rajendran, R. S., Wellbrock, U. M., and Zupanc, G. K. H. (2008). Apoptotic cell death, long-term persistence, and neuronal differentiation of aneuploid cells generated in the adult brain of teleost fish. Dev. Neurobiol. 68, 1257–1268. doi: 10.1002/dneu.20656
Rehen, S. K., McConnell, M. J., Kaushal, D., Kingsbury, M. A., Yang, A. H., and Chun, J. (2001). Chromosomal variation in neurons of the developing and adult mammalian nervous system. Proc. Natl. Acad. Sci. U.S.A. 98, 13361–13366. doi: 10.1073/pnas.231487398
Rompani, S. B., and Cepko, C. L. (2008). Retinal progenitor cells can produce restricted subsets of horizontal cells. Proc. Natl. Acad. Sci. U.S.A. 105, 192–197. doi: 10.1073/pnas.0709979104
Sandmann, D., Boycott, B. B., and Peichl, L. (1996a). Blue-cone horizontal cells in the retinae of horses and other equidae. J. Neurosci. 16, 3381–3396.
Sandmann, D., Boycott, B. B., and Peichl, L. (1996b). The horizontal cells of artiodactyl retinae: a comparison with Cajal's descriptions. Vis. Neurosci. 13, 735–746. doi: 10.1017/S0952523800008610
Schieber, N. L., Collin, S. P., and Hart, N. S. (2012). Comparative retinal anatomy in four species of elasmobranch. J. Morphol. 273, 423–440. doi: 10.1002/jmor.11033
Schuff, M., Rössner, A., Donow, C., and Knöchel, W. (2006). Temporal and spatial expression patterns of FoxN genes in Xenopus laevis embryos. Int. J. Dev. Biol. 50, 429–434. doi: 10.1387/ijdb.052126ms
Shirazi Fard, S., All-Ericsson, C., and Hallböök, F. (2014a). The heterogenic final cell cycle of chicken retinal Lim1 horizontal cells is not regulated by the DNA damage response pathway. Cell Cycle 13, 408–417. doi: 10.4161/cc.27200
Shirazi Fard, S., Blixt, M., and Hallböök, F. (2015). The p53 co-activator Zac1 neither induces cell cycle arrest nor apoptosis in chicken Lim1 horizontal progenitor cells. Cell Death Discov. 1:e15023. doi: 10.1038/cddiscovery.2015.23
Shirazi Fard, S., Jarrin, M., Boije, H., Fillon, V., All-Eriksson, C., and Hallböök, F. (2013). Heterogenic final cell cycle by chicken retinal Lim1 horizontal progenitor cells leads to heteroploid cells with a remaining replicated genome. PLoS ONE 8:e59133. doi: 10.1371/journal.pone.0059133
Shirazi Fard, S., Thyselius, M., All-Ericsson, C., and Hallböök, F. (2014b). The terminal basal mitosis of chicken retinal Lim1 horizontal cells is not sensitive to cisplatin-induced cell cycle arrest. Cell Cycle 13, 3698–3706. doi: 10.4161/15384101.2014.964985
Silveira, L. C., Yamada, E. S., and Picanço-Diniz, C. W. (1989). Displaced horizontal cells and biplexiform horizontal cells in the mammalian retina. Vis. Neurosci. 3, 483–488. doi: 10.1017/S0952523800005988
Song, P. I., Matsui, J. I., and Dowling, J. E. (2008). Morphological types and connectivity of horizontal cells found in the adult zebrafish (Danio rerio) retina. J. Comp. Neurol. 506, 328–338. doi: 10.1002/cne.21549
Suga, A., Taira, M., and Nakagawa, S. (2009). LIM family transcription factors regulate the subtype-specific morphogenesis of retinal horizontal cells at post-migratory stages. Dev. Biol. 330, 318–328. doi: 10.1016/j.ydbio.2009.04.002
Sun, Y., Dykes, I. M., Liang, X., Eng, S. R., Evans, S. M., and Turner, E. E. (2008). A central role for Islet1 in sensory neuron development linking sensory and spinal gene regulatory programs. Nat. Neurosci. 11, 1283–1293. doi: 10.1038/nn.2209
Suzuki, S. C., Bleckert, A., Williams, P. R., Takechi, M., Kawamura, S., and Wong, R. O. L. (2013). Cone photoreceptor types in zebrafish are generated by symmetric terminal divisions of dedicated precursors. Proc. Natl. Acad. Sci. U.S.A. 110, 15109–15114. doi: 10.1073/pnas.1303551110
Szél, A., Röhlich, P., Caffé, A. R., and van Veen, T. (1996). Distribution of cone photoreceptors in the mammalian retina. Microsc. Res. Tech. 35, 445–462. doi: 10.1002/(SICI)1097-0029(19961215)35:6<445::AID-JEMT4>3.0.CO;2-H
Tanabe, K., Takahashi, Y., Sato, Y., Kawakami, K., Takeichi, M., and Nakagawa, S. (2006). Cadherin is required for dendritic morphogenesis and synaptic terminal organization of retinal horizontal cells. Development 133, 4085–4096. doi: 10.1242/dev.02566
Tanabe, K., Takeichi, M., and Nakagawa, S. (2004). Identification of a nonchordate-type classic cadherin in vertebrates: chicken Hz-cadherin is expressed in horizontal cells of the neural retina and contains a nonchordate-specific domain complex. Dev. Dyn. 229, 899–906. doi: 10.1002/dvdy.10493
Tarrés, M. A., Baron, M., and Gallego, A. (1986). The horizontal cells in the retina of the owl, Tyto alba, and owlet, Carinae noctua. Exp. Eye Res. 42, 315–321. doi: 10.1016/0014-4835(86)90024-2
Toyoda, J. I., Saito, T., and Kondo, H. (1978). Three types of horizontal cells in the stingray retina: their morphology and physiology. J. Comp. Neurol. 179, 569–579. doi: 10.1002/cne.901790308
Twig, G., Levy, H., and Perlman, I. (2003). Color opponency in horizontal cells of the vertebrate retina. Prog. Retin. Eye Res. 22, 31–68. doi: 10.1016/S1350-9462(02)00045-9
Vardi, N., Kaufman, D. L., and Sterling, P. (1994). Horizontal cells in cat and monkey retina express different isoforms of glutamic acid decarboxylase. Vis. Neurosci. 11, 135–142. doi: 10.1017/S0952523800011172
Villar-Cheda, B., Abalo, X. M., Anadón, R., and Rodicio, M. C. (2006). Calbindin and calretinin immunoreactivity in the retina of adult and larval sea lamprey. Brain Res. 1068, 118–130. doi: 10.1016/j.brainres.2005.11.014
Voullaire, L., Slater, H., Williamson, R., and Wilton, L. (2000). Chromosome analysis of blastomeres from human embryos by using comparative genomic hybridization. Hum. Genet. 106, 210–217. doi: 10.1007/s004390051030
Wang, J. C.-C., and Harris, W. A. (2005). The role of combinational coding by homeodomain and bHLH transcription factors in retinal cell fate specification. Dev. Biol. 285, 101–115. doi: 10.1016/j.ydbio.2005.05.041
Wässle, H., Dacey, D. M., Haun, T., Haverkamp, S., Grünert, U., and Boycott, B. B. (2000). The mosaic of horizontal cells in the macaque monkey retina: with a comment on biplexiform ganglion cells. Vis. Neurosci. 17, 591–608. doi: 10.1017/S0952523800174097
Weber, I. P., Ramos, A. P., Strzyz, P. J., Leung, L. C., Young, S., and Norden, C. (2014). Mitotic position and morphology of committed precursor cells in the zebrafish retina adapt to architectural changes upon tissue maturation. Cell Rep. 7, 386–397. doi: 10.1016/j.celrep.2014.03.014
Werblin, F. S., and Dowling, J. E. (1969). Organization of the retina of the mudpuppy, Necturus maculosus. II. Intracellular recording. J. Neurophysiol. 32, 339–355.
West, R. W. (1978). Bipolar and horizontal cells of the gray squirrel retina: Golgi morphology and receptor connections. Vision Res. 18, 129–136. doi: 10.1016/0042-6989(78)90177-3
Whitney, I. E., Raven, M. A., Ciobanu, D. C., Poché, R. A., Ding, Q., Elshatory, Y., et al. (2011). Genetic modulation of horizontal cell number in the mouse retina. Proc. Natl. Acad. Sci. U.S.A. 108, 9697–9702. doi: 10.1073/pnas.1103253108
Wu, F., Li, R., Umino, Y., Kaczynski, T. J., Sapkota, D., Li, S., et al. (2013). Onecut1 is essential for horizontal cell genesis and retinal integrity. J. Neurosci. 33, 13053–13065. doi: 10.1523/JNEUROSCI.0116-13.2013
Xu, X. L., Singh, H. P., Wang, L., Qi, D.-L., Poulos, B. K., Abramson, D. H., et al. (2014). Rb suppresses human cone-precursor-derived retinoblastoma tumours. Nature 514, 385–388. doi: 10.1038/nature13813
Zanet, J., Freije, A., Ruiz, M., Coulon, V., Sanz, J. R., Chiesa, J., et al. (2010). A mitosis block links active cell cycle with human epidermal differentiation and results in endoreplication. PLoS ONE 5:e15701. doi: 10.1371/journal.pone.0015701
Zhang, A.-J., Zhang, J., and Wu, S. M. (2006). Electrical coupling, receptive fields, and relative rod/cone inputs of horizontal cells in the tiger salamander retina. J. Comp. Neurol. 499, 422–431. doi: 10.1002/cne.21117
Zhang, J., Zhang, A.-J., and Wu, S. M. (2006). Immunocytochemical analysis of GABA-positive and calretinin-positive horizontal cells in the tiger salamander retina. J. Comp. Neurol. 499, 432–441. doi: 10.1002/cne.21116
Zhang, L., Cho, J., Ptak, D., and Leung, Y. F. (2013). The role of egr1 in early zebrafish retinogenesis. PLoS ONE 8:e56108. doi: 10.1371/journal.pone.0056108
Zhou, B.-B. S., and Elledge, S. J. (2000). The DNA damage response: putting checkpoints in perspective. Nature 408, 433–439. doi: 10.1038/35044005
Keywords: cell fate, cell cycle regulation, chicken, development, DNA damage, neuronal subtype, retinoblastoma, zebrafish
Citation: Boije H, Shirazi Fard S, Edqvist PH and Hallböök F (2016) Horizontal Cells, the Odd Ones Out in the Retina, Give Insights into Development and Disease. Front. Neuroanat. 10:77. doi: 10.3389/fnana.2016.00077
Received: 12 April 2016; Accepted: 21 June 2016;
Published: 19 July 2016.
Edited by:
Zoltan F. Kisvarday, University of Debrecen, HungaryReviewed by:
Benjamin E. Reese, University of California, Santa Barbara, USARobert Gabriel, University of Pécs, Hungary
Akos Lukats, Semmelweis University, Hungary
Copyright © 2016 Boije, Shirazi Fard, Edqvist and Hallböök. This is an open-access article distributed under the terms of the Creative Commons Attribution License (CC BY). The use, distribution or reproduction in other forums is permitted, provided the original author(s) or licensor are credited and that the original publication in this journal is cited, in accordance with accepted academic practice. No use, distribution or reproduction is permitted which does not comply with these terms.
*Correspondence: Henrik Boije, aGVucmlrLmJvaWplQG5ldXJvLnV1LnNl