Kcnab1 Is Expressed in Subplate Neurons With Unilateral Long-Range Inter-Areal Projections
- 1Department of Anatomy and Neuroscience, Graduate School of Medicine, Osaka University, Osaka, Japan
- 2Division of Developmental Neuroscience, Department of Child Development, United Graduate School of Child Development, Osaka University, Kanazawa University, Hamamatsu University School of Medicine, Chiba University and University of Fukui, Osaka, Japan
- 3Institute of Biological Sciences, Faculty of Science, University of Malaya, Kuala Lumpur, Malaysia
- 4Division of Cell Biology and Neuroscience, Department of Morphological and Physiological Sciences, Faculty of Medical Sciences, University of Fukui, Fukui, Japan
- 5Research Center for Child Mental Development, University of Fukui, Fukui, Japan
Subplate (SP) neurons are among the earliest-born neurons in the cerebral cortex and heterogeneous in terms of gene expression. SP neurons consist mainly of projection neurons, which begin to extend their axons to specific target areas very early during development. However, the relationships between axon projection and gene expression patterns of the SP neurons, and their remnant layer 6b (L6b) neurons, are largely unknown. In this study, we analyzed the corticocortical projections of L6b/SP neurons in the mouse cortex and searched for a marker gene expressed in L6b/SP neurons that have ipsilateral inter-areal projections. Retrograde tracing experiments demonstrated that L6b/SP neurons in the primary somatosensory cortex (S1) projected to the primary motor cortex (M1) within the same cortical hemisphere at postnatal day (PD) 2 but did not show any callosal projection. This unilateral projection pattern persisted into adulthood. Our microarray analysis identified the gene encoding a β subunit of voltage-gated potassium channel (Kcnab1) as being expressed in L6b/SP. Double labeling with retrograde tracing and in situ hybridization demonstrated that Kcnab1 was expressed in the unilaterally-projecting neurons in L6b/SP. Embryonic expression was specifically detected in the SP as early as embryonic day (E) 14.5, shortly after the emergence of SP. Double immunostaining experiments revealed different degrees of co-expression of the protein product Kvβ1 with L6b/SP markers Ctgf (88%), Cplx3 (79%), and Nurr1 (58%), suggesting molecular subdivision of unilaterally-projecting L6b/SP neurons. In addition to expression in L6b/SP, scattered expression of Kcnab1 was observed during postnatal stages without layer specificity. Among splicing variants with three alternative first exons, the variant 1.1 explained all the cortical expression mentioned in this study. Together, our data suggest that L6b/SP neurons have corticocortical projections and Kcnab1 expression defines a subpopulation of L6b/SP neurons with a unilateral inter-areal projection.
Introduction
The subplate (SP) is known to be a transient layer that contributes substantially to early cortical development, whereas most of its constituent neurons gradually disappear when the cortical layers start to emerge and only a small portion of SP neurons remain in layer 6b (L6b) as development proceeds (Reep and Goodwin, 1988; Chun and Shatz, 1989; Bayer and Altman, 1990; Kostovic and Rakic, 1990; Woo et al., 1991; Allendoerfer and Shatz, 1994; Valverde et al., 1995; Clancy and Cauller, 1999; Reep, 2000; Robertson et al., 2000; Hanganu et al., 2002; Kanold, 2009; Hoerder-Suabedissen and Molnár, 2015). The L6b/SP neurons that remain into adulthood regulate cortical layer formation and modulate information flow into and out of the cortex (Kanold and Shatz, 2006; Friedlander and Torres-Reveron, 2009; Kanold and Luhmann, 2010). In rodents amongst other mammalian species, L6b/SP neurons are morphologically heterogeneous (Kostovic and Rakic, 1990; Valverde et al., 1995; Hanganu et al., 2002; Andjelic et al., 2009; Chen et al., 2009; Hoerder-Suabedissen and Molnár, 2012; Marx and Feldmeyer, 2013; Marx et al., 2017) and diverse in gene expression (Hoerder-Suabedissen et al., 2009; McKellar and Shatz, 2009; Osheroff and Hatten, 2009; Belgard et al., 2011; Oeschger et al., 2012; Hoerder-Suabedissen and Molnár, 2013; Tasic et al., 2016). Electrophysiological studies also revealed distinct intrinsic properties of SP neurons that most likely play an important role in shaping cortical networks during development (Hanganu et al., 2002; Dupont et al., 2006; Luhmann et al., 2009, 2018; Kanold and Luhmann, 2010; Yang et al., 2016; Deng et al., 2017).
SP neurons consist largely of projection neurons, and their axons begin to form projections very early on during development (McConnell et al., 1989; De Carlos and O’Leary, 1992; Clascá et al., 1995; Molnár et al., 1998; Pedraza et al., 2014). SP projection neurons extend their axons to specific cortical and subcortical sites (Usrey and Fitzpatrick, 1996; Zhang and Deschênes, 1997; Kanold and Luhmann, 2010; Hoerder-Suabedissen and Molnár, 2012; Hoerder-Suabedissen et al., 2018). Intracortical axonal projections from the SP target layers 1 and 4 above the SP and also distant cortical regions (Clancy and Cauller, 1999; Piñon et al., 2009; Zhao et al., 2009; Hoerder-Suabedissen and Molnár, 2012; Hoerder-Suabedissen et al., 2018). Axon collaterals of SP neurons project both ipsilaterally and contralaterally within the cortex, although only very few of the latter were found in rodents (De Carlos and O’Leary, 1992; Allendoerfer and Shatz, 1994; Ozaki and Wahlsten, 1998; Del Río et al., 2000; Hanganu et al., 2002; Kanold et al., 2003; Kanold and Shatz, 2006; Hoerder-Suabedissen and Molnár, 2012). Similar intracortical axon projection patterns have been reported in other mammalian species including cats and ferrets (McConnell et al., 1989; Antonini and Shatz, 1990; Friauf et al., 1990; Friauf and Shatz, 1991; Finney et al., 1998).
Molecular characterization of SP neurons at different developmental stages has identified SP-specific genes that are mostly temporal, which indicates the possible changing role of SP neurons underlying functional circuitry development and refinement (Hoerder-Suabedissen et al., 2009, 2013; Oeschger et al., 2012; Hoerder-Suabedissen and Molnár, 2013; Sorensen et al., 2015; Viswanathan et al., 2017). Several studies have shown that deletion of SP resulted in disrupted cortical plate organization and developmental deficits in brain functions (Ghosh et al., 1990; Ghosh and Shatz, 1993; Lein et al., 1999; Kanold et al., 2003; Kanold and Shatz, 2006; Magnani et al., 2012; Tolner et al., 2012). Thus, expression of SP-specific genes at a critical period during development almost certainly underlies the fine orchestration of SP axon projection pattern and regulates cortical maturation. However, the relationship between molecular identity and axon projection target specificity remains largely elusive.
Our study is an effort to characterize axon projection pattern in the mouse L6b/SP, and to correlate it to the specific gene expression pattern of these neurons. This is crucial to understand the diversity of L6b/SP neurons and to enable functional manipulation of the discrete classes to clarify the distinct role of these neurons during cortex maturation. We set out to examine the axonal projection target of L6b/SP neurons and whether this is consistent throughout development. We studied axonal projection from L6b/SP of the primary somatosensory cortex (S1) to the primary motor cortex (M1) since revealing molecular identity and development of this projection would lead to better understanding of a parallel circuit that it forms with the projections from the other layers (Mitchell and Macklis, 2005). We found a proportion of L6b/SP neurons that project unilaterally from S1 to M1 can be molecularly identified by a specific splice variant of the voltage-gated potassium channel Kcnab1. This discrete group of neurons was present at different stages of development, although the expression pattern of Kcnab1 changed as the brain matured. There was also a considerable overlap between the Kvβ1-positive neurons in L6b/SP with known L6b/SP molecular markers. Overall, our results suggested that this molecularly distinctive group of neurons is most likely a L6b/SP subpopulation that survives as the cortex develops and may underlie inter-areal circuit establishment in the cortex.
Materials and Methods
Animals
Wild-type ICR mice were used in this study, and the animals were purchased from SLC Japan and housed under standard conditions with food and water ad libitum and maintained on a 12-h light/dark cycle. Embryonic day (E) 0.5 was defined as 12:00 noon on the day when the vaginal plug was found. During all surgical protocols, animals were deeply anesthetized by intraperitoneal injection of a combination anesthetic (MMB: 0.3 mg/kg of medetomidine, 4.0 mg/kg of midazolam, and 5.0 mg/kg of butorphanol) and intracardially perfused with ice-cold phosphate buffered saline (PBS) followed by 4% paraformaldehyde (PFA). Whole brains were carefully dissected after perfusion and post-fixed in 4% PFA overnight at 4°C, then transferred to 30% sucrose solution (≥24 h or until brain sinks to the bottom of the tube at 4°C). In the case of mouse embryos, the embryos were collected from pregnant dams (wild-type ICR) and the brains were immediately dissected from the embryos and post-fixed in 4% PFA overnight at 4°C, then transferred to 30% sucrose solution. The dams were sacrificed with cervical dislocation after administration of five doses of MMB. All animal experiments were approved by the Animal Research Committee of University of Fukui and the Animal Experimentation Committee of Osaka University, and performed in accordance with the Regulations for Animal Research at the University of Fukui and the Regulations on Animal Experimentation at Osaka University.
Retrograde Labeling and Tissue Processing
Postnatal day (PD) 2 and 3-week-old (PW3) mice were used in the retrograde tracing experiments. PW3 mice were anesthetized and fixed on a stereotaxic frame, and tracer was injected into the cortex according to coordinates determined based on the mouse brain atlas (Paxinos and Franklin, 2008). Tracer used in this study was 2% Fluoro-Gold (Fluorochrome) in distilled water; 0.5% Cholera Toxin Subunit B conjugated with AlexaFluor 488 (Thermo Fisher Scientific) in PBS; or 2% Green RetroBeadsTM IX (Lumaflour) in PBS, and all tracer mixtures were added with 0.1 μg/ml Fast Green. For all injection sites, the anterior/posterior (AP) coordinates were referenced from Bregma, the medial/lateral (ML) coordinates were the distance from the midline at Bregma, and the dorsal/ventral (DV) coordinates were measured from the pial surface of the brain. All measurement units were in mm and are referred to in the following description as [anterior/posterior (AP), medial/lateral (ML), dorsal/ventral (DV)]. Three and six injection sites were selected for both M1 and S1, respectively. For injection into M1, the stereotaxic coordinates were (0.7, 1.0, 0.8), (1.2, 1.2, 0.8), and (1.5, 1.5, 0.8); whereas the stereotaxic coordinates for injection into S1 were (0.0, 3.0/3.5, 0.5), (−0.50, 3.0/2.5, 0.5), and (−1.5, 3.0/2.5, 0.5). Each animal received pressure injection (approximately 40 nl/site) of a tracer delivered via a glass needle (pulled and broken at the tip with forceps to make an opening with a diameter of 40–60 μm) attached to a Hamilton syringe. After 4 days’ survival, animals were transcardially perfused and the brains were processed as mentioned above. Images of the whole FG-injected brains were taken with a fluorescence stereomicroscope (MZ 10F, Leica) with a filter set ET UV LP (Leica), and only the brains that appeared to have sufficient injection were subsequently sectioned. The selected brains were embedded in O.C.T. compound (Sakura) and stored at −80°C, then cut into 14–16 μm sections on a coronal plane using a freezing microtome (Leica model CM3050S). Sections were imaged with BZ-X700 (Keyence) using an appropriate filter cube for FG (ET DAPI/FluoroGold, Chroma Technology Co., Bellows Falls, VT, USA).
As for mice at PD2, the animals were anesthetized by hypothermia and tracer was injected by using a Picospritzer® II microinjector (Parker Hannifin, Cleveland, OH, USA). The injection targets (M1 and S1) were estimated based on parallel experiments whereby the injected mouse was raised to adulthood and injection sites in M1 and S1 were traced to confirm the point of injection. Briefly, injection in M1 and S1 were estimated as referral points from the midline (x) and the most rostral edge of the cortex (y), whereby M1 was x: 1.5 mm, y: 2.0 mm; and S1 was x: 2.5–2.8 mm and y: 3.0–3.5 mm. The mice were let to recover on heated pads and returned to the dams for survival. The mice were perfused 2 days post-injection at PD4 and the brains were processed and imaged as mentioned above.
Microarray Screening
The genes preferentially expressed in association neurons (projecting from S1 to ipsilateral M1) compared to those in callosal neurons [projecting from S1 to contralateral S1 (cS1)] were identified with DNA microarray screening. In brief, cortical layer 2/3 neurons were transfected with pCAGGS-tdTomato by performing in utero electroporation at E15.5, and the mice that had tdTomato labeling of cell bodies in S1 were used in the subsequent procedure. The retrograde tracer Green RetrobeadsTM IX was injected at PD21 into either M1 or cS1 with the help of red fluorescence of tdTomato in these areas (in axon terminals projecting from S1). Mice were transcardially perfused with ice-cold PBS and the brains were dissected out, embedded in O.C.T. compound, and frozen in powdered dry ice. Fresh-frozen sections were cut at 10 μm, thaw-mounted onto glass slides covered with polyphenylene sulfide membrane and air-dried immediately. The labeled association neurons in layers 2/3, 5, and 6b, and labeled callosal neurons in layers 2/3 and 5 were collected from S1 (more than 1,000 cells each) using a laser-captured microdissection system (AS-LMD, Leica). Total RNAs were prepared using NucleoSpin RNA XS kit (Macherey-Nagel). cDNAs were synthesized, amplified, Biotin-labeled and fragmented using OvationTM Pico WTA System, WT-OvationTM Exon Module, and EncoreTM Biotin Module (NuGEN). Labeled cDNAs were hybridized to the GeneChip Mouse Gene 1.0 ST Array (Affymetrix, Santa Clara, CA, USA). Hybridization, washing and scanning were performed with a GeneChip 3,000 7G system (Affymetrix, Santa Clara, CA, USA). Raw signals were subjected to Log-transformation, global normalization, and centering in order to obtain processed signals for cross-sample comparison. The candidate genes preferentially expressed in L6b were selected using Subio Platform (version 1.14, Subio). The microarray data were deposited at Gene Expression Omnibus (GEO) under the accession number GSE123351.
Probe Generation and In situ Hybridization Histochemistry (ISHH)
cDNA fragments of Kcnab1 and Ctgf were PCR-amplified from mouse brain cDNA with the following primer pairs. Kcnab1-fwd.: 5′ GAAATGGGGTGCCAGAAA 3′; rev.: 5′ ATTGTACAGGGCCAGGCA 3′; Ctgf-fwd.: 5′ AGAGTGGAGCGCCTGTTCTA 3′; and rev.: 5′ ACTGGCAGAGTGGTGGTTCT 3′. In order to examine the expression pattern of Kcnab1 splicing variants Kcnab1.1, Kcnab1.2, and Kcnab1.3 in the mouse cortex, in situ probes were generated to specifically recognize each of the subunits. The primer sets used were as follows: Kcnab1.1-fwd.: 5′ CAGCCGAGATCACAGCCTG… 3′; rev.: 5′ CTGCTTTGCGGTGGACTCTT… 3′; Kcnab1.2-fwd.: 5′ ATAAACCTGCCTGTGCAGA… 3′; rev.: 5′ CATGCCTGTCTTTGCCTTG… 3′; Kcnab1.3-fwd.: 5′ AGGCAGATAGGAACTTCCAG… 3′; rev.: 5′ GCTCGCAGAGCTTTAGGT… 3′. Amplified fragments were cloned into pGEM-T vector (Promega). In vitro transcription of cRNA probes was performed with T7 or SP6 RNA polymerase (Roche) using the template plasmids linearized with an appropriate restriction enzyme and RNA DIG labeling mix (Roche) according to the manufacturer’s instructions.
In situ hybridization histochemistry (ISHH) was performed as described before (Yagi et al., 2016) using cryosections (14–16 μm) prepared from mice at E12.5, E14.5, E16.5, and E18.5; and PD3, PD4, PD7, PD21, and PD25 as mentioned above. The cryosections were air dried for 1 h and fixed in 4% PFA in PBS for 10 min at room temperature. The sections were then incubated in 0.2 M HCl for 10 mins, followed by permeabilization with Proteinase K (7.9 μg/ml; Roche) digestion for 10 min at 37°C. Concentration and treatment duration of Proteinase K were halved for the brain samples younger than PD4. Next, the sections were treated with acetic anhydride in 0.1 M triethanolamine for 10 min. The slides were rinsed with PBS in between each step. Finally, the sections were transferred to 5× saline sodium citrate (SSC) for 10 min or longer. Hybridization was carried out with the generated probes in hybridization buffer (50% formamide, 5× SSC, 200 μg/ml yeast tRNA) overnight for at least 16 h at 55°C. High-stringency washes were carried out in the following steps: 5× SSC, 20 min at room temperature; 2× SSC, 20 min at 65°C; two washes with 0.2× SSC, 20 min at 65°C and lastly the slides were transferred to PBS at room temperature. Detection of specific hybridization was performed using anti-Digoxigenin coupled with alkaline phosphatase, and subsequently visualized using nitro blue tetrazolium chloride/5-bromo-4-chloro-3-indolyl-phosphate (NBT/BCIP). Sense probes were used as negative controls and no signals were observed with the sense probes. Bright field images of the stained sections were taken with BZ-X700.
Immunohistochemistry
Brain cryosections were prepared from adult mice (PW8–PW24) and the sections were processed accordingly for double immunofluorescence labeling. After air-drying for an hour at room temperature, the sections were treated with Tris EDTA buffer (pH 8.5) for 1 min at 105°C in the autoclave or for 30 min at 85°C in the water bath. This was followed by short rinses (5 min, three rinses) in PBS before blocking the sections with 5% normal donkey serum, 0.1% TritonX-100 in PBS. The sections were then incubated in primary antibodies (diluted in blocking solution) overnight at 4°C. The primary antibodies used in this study were as follows: CaMKIIα (1:100; rabbit; GeneTex, Irvine, CA, USA), Cplx3 (1:500; rabbit; SYSY), Ctgf (1:200; goat; Santa Cruz, CA, USA), Kvβ1 (1:200; mouse; Santa Cruz), and Nurr1 (1:200; goat; R&D). This was followed by incubation in species-specific fluorescent secondary antibodies (Donkey anti-mouse IgG Alexa Fluor 568 and either Donkey anti-rabbit IgG Alexa Fluor 488 or Donkey anti-goat IgG Alexa Fluor 488, all from Thermo Fisher Scientific, Waltham, MA, USA) for 4–6 h at 4°C. All secondary antibodies were diluted at 1:200 in PBS. Nuclear staining was performed using 4′,6-diamidino-2-phenylindole (DAPI). Fluorescence signals were imaged with a laser scanning confocal microscope (LSM 880 with Airyscan, Zeiss).
Immunohistochemistry against Fluoro-Gold was combined with ISHH. After detection of ISHH signals, sections were incubated with anti-Fluoro-Gold (FG; 1:500; rabbit; Millipore, MA, USA) overnight at 4°C. Immunoperoxidase labeling was performed using a Vectastain Elite ABC Kit (Vector) and a DAB detection kit (Vector) was used for detection, according to the manufacturer’s protocol. Bright field images of the stained sections were taken with BZ-X700.
Splice Variant Identification
Mouse homologues for exons 1.2 and 1.3 of the human Kcnab1 gene were identified by T-BLAST-N search in Ensembl genome database (GRCm38.p61) with the human sequences as queries. The genomic regions encompassing the hit sequences and exon 2 were then subjected to exon/intron prediction with GeneWise2. The identified sequences were deposited at the DNA Data Bank of Japan (DDBJ) under the accession numbers LC437679 for exon 1.2 and LC437680 for exon 1.3.
Cell Quantification
For analysis of retrogradely labeled L6b/SP neurons that expressed Kcnab1, 3 to 7 S1-containing sections per animal × three animals were used for quantification. The bright field images were adjusted for brightness and contrast using Adobe Photoshop CS3 (Adobe). Cell quantification was carried out using the Cell Counter plugin for ImageJ software (National Institutes of Health, Bethesda, MD, USA). Cells within a 4-cell-height from the border between the cortex and the white matter were considered as L6b and included in quantification. While the NBT/BCIP signals were usually devoid of nucleus, DAB tended to stain the entire cell body. Thus, double-positive cells were defined as cells that had DAB signal surrounded by NBT/BCIP signal. Cells without a clear nucleus were not included in quantification. Cells that were too densely stained with NBT/BCIP and/or DAB were excluded. The cell numbers counted are summarized in Supplementary Table S1.
For co-localization analysis for Kvβ1 and L6b markers, three S1-containing sections per animal × three animals were used for quantification. The fluorescence images were adjusted for brightness and contrast using Adobe Photoshop CS3. Cell quantification was carried out using the Cell Counter plugin for ImageJ software. Cells within a 4-cell-height from the border between the cortex and the white matter were considered as L6b and included in quantification. Only the cells with DAPI signals were included in quantification. DAPI-positive cells were also counted to estimate the total cell number in each counted area. Co-localization analysis for Kvβ1 and CaMKIIα was performed in the same manner using two sections per animal × four animals. The cell numbers counted are summarized in Supplementary Table S2.
Analysis of the Public RNAseq Data in the Gene Expression Omnibus (GEO) Database
The TPM (Transcripts per million) data for Kcnab1, Ctgf, Cplx3, and Nurr1 (Nr4a2) genes across 1809 individual cells isolated from the mouse primary visual cortex (V1) were obtained from the GEO3 (accession, GSE 71585; Tasic et al., 2016). Genes were considered as being expressed in a cell when the TPM value in the cell was 10 or larger. The percentage of cells expressing Kcnab1 at two different expression levels (TPM ≥ 10 and TPM ≥ 100) was calculated for each of the eight broad types of cortical cells (astrocytes, endothelial cells, GABAergic neurons, glutamatergic neurons, microglia, oligodendrocytes, oligodendrocyte precursor cells, and unclassified cells). The percentages were also calculated for glutamatergic neurons in different layers and seven classes of GABAergic neurons by combining the numbers of cells for primary cell types in each layer or class. The percentages of cells expressing each of the four genes were calculated for two primary cell types for L6b (Rgs12 and Sepinb11). To specifically examine if Kcnab1 is expressed in GABAergic neurons in L6b, the percentages of cells expressing Kcnab1 among 41 cells (containing 33 glutamatergic neurons and eight GABAergic neurons) dissected from L6b of V1 were also calculated.
Similar analyses were performed using other RNAseq data for the four genes across 3,005 individual cells isolated from mouse S1 and hippocampal CA1 (Zeisel et al., 2015). The annotated expression data (equivalent to the raw data in GEO with the accession GSE60361 except for the cell type annotations) were obtained from the website of Linnarsson lab4. The percentages of Kcnab1-expressing cells (expression score ≥ 1) were calculated for Level-1 classes of cell types and for Level-2 classes of interneurons and pyramidal neurons. Those of cells expressing each of the four genes were calculated for L6b cells.
Results
Layer 6b/SP Contained Corticocortical Projecting Neurons
Discrete neuronal populations occupy each cortical layer and the laminar location of cell bodies commonly corresponds to specific axon projection targets. In order to determine whether neurons in the S1 area project over a long distance to the M1 area or form callosal projections, we injected retrograde tracer FG in M1 and cS1 of mice at age PW3 (Figures 1A,D). This was followed by a survival time of 4 days to allow the tracer to be retrogradely transported. Then we examined the distribution of retrogradely-labeled neurons in S1. We observed FG-labeled neurons in L6b in addition to some in layers 2/3 and 5, when tracer was injected into M1 (Figures 1B,C), consistent with a previous report (Mitchell and Macklis, 2005). When FG was injected in S1 in the other hemisphere under the same condition, there was no labeled neuron in L6b, although FG-labeled neurons were obvious in layers 2/3 to 5 (Figures 1E,F). The retrograde labeling experiments showed the same results when repeated with two other tracers; fluorescence conjugated-cholera toxin B (CTB) and Green RetroBeadsTM (data not shown).
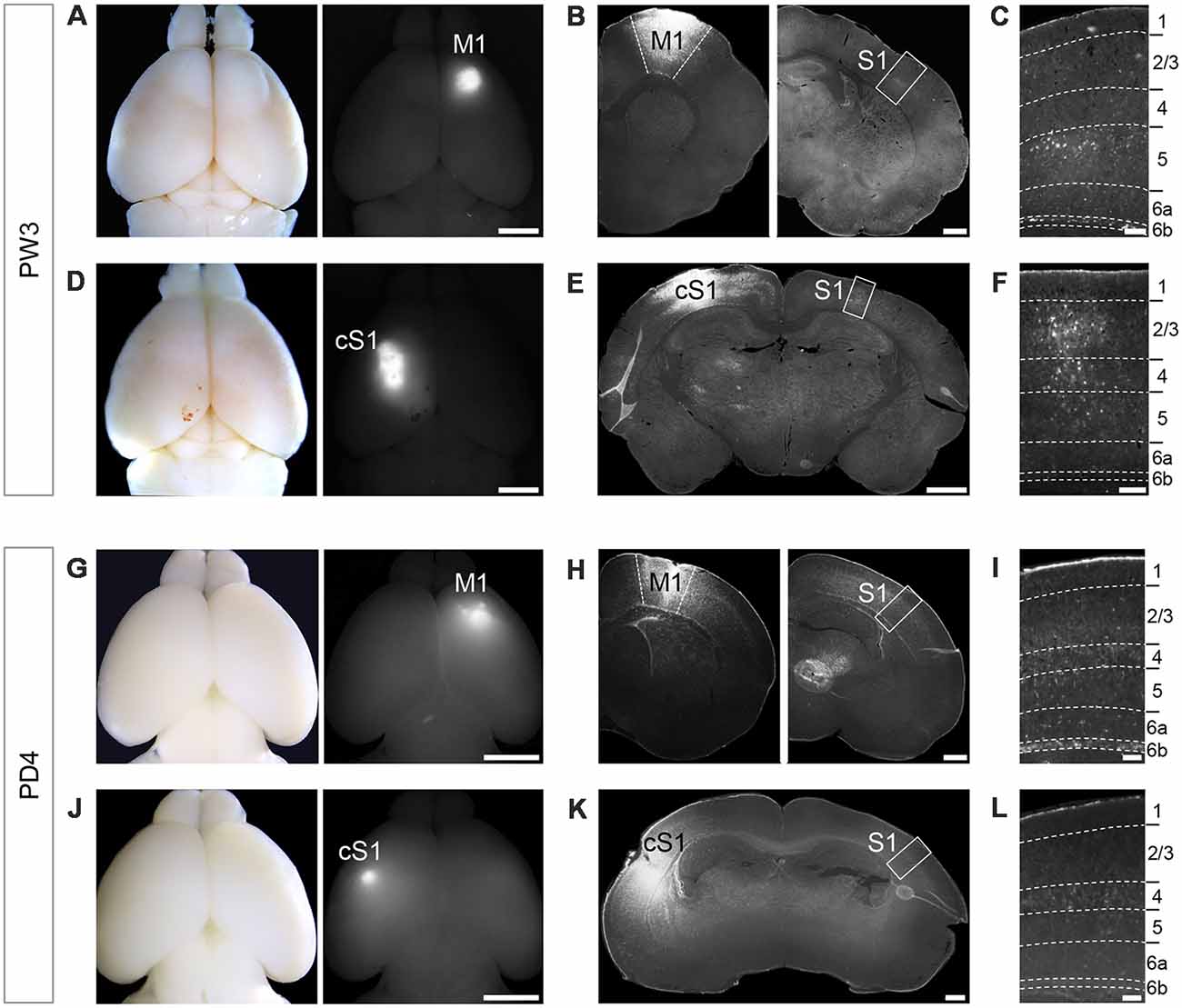
Figure 1. Retrograde tracer injection in M1 of PW3 mouse brain labeled unilaterally-projecting Layer 6b (L6b)/SP neurons in primary somatosensory cortex (S1). (A,D) Whole mount brain images in a bright field (left) and a fluorescent (right) views showing Fluoro-Gold (FG) injections into primary motor cortex (M1; A) and contralateral S1 (cS1; D) at PW3. Scale bars, 2 mm. (B) Coronal sections of the brain shown in (A) at the level of M1 (left) and S1 (right). Scale bar, 500 μm. (C) Higher magnification view of the boxed area in (B). FG-labeled neurons were detected in L6b, as well as other layers. Scale bar, 100 μm. (E) A coronal section of the brain shown in (D) at the level of S1. Scale bar, 500 μm. (F) Higher magnification view of the boxed area in (E). Scale bar, 100 μm. (G,J) Whole mount brain images in a bright field (left) and a fluorescent (right) views showing FG injection into M1 (G) and cS1 (J) at PD2. Scale bars, 2 mm. (H) Coronal sections of the brain shown in (G) at the level of M1 (left) and S1 (right). Scale bar, 500 μm. (I) Higher magnification views of the boxed area in (H). FG-labeled neurons were detected in L6b. Scale bar, 100 μm. (K) A coronal section of the brain shown in (J) at the level of S1. Scale bar, 500 μm. (L) Higher magnification views of the boxed area in (K). Scale bars, 100 μm. Dashed lines in (C), (F), (I), and (L) indicate borders of cortical layers.
We next asked if this projection pattern is consistent throughout postnatal development. When FG was injected at PD2, we found FG-labeled SP neurons at PD4 for tracing from M1 (Figures 1G–I), but not for tracing from cS1 (Figures 1J–L), similar to the results in L6b neurons in PW3 animals. Taken together, L6b/SP neurons in the S1 area projected their axons unilaterally to the M1 area.
Kcnab1 Was Identified as a Candidate Gene Expressed in L6b/SP Association Neurons
In our DNA microarray screening for the genes preferentially expressed in association neurons (projecting from S1 to M1) and those in callosal neurons (projecting from S1 to cS1; Figure 2A), we found many genes preferentially expressed in the association neurons in L6b/SP. We selected 100 candidate genes with the highest enrichment in L6b/SP for shortlisting by ISHH. These included a gene encoding a β subunit of voltage-dependent potassium channel, Kcnab1, as well as known L6b/SP markers including Ctgf (Figure 2B). ISHH analysis with coronal sections of the PD21 cortex confirmed the expression of Kcnab1 in L6b/SP, similar to that of Ctgf. In addition, Kcnab1 was also observed in other layers in a scattered manner (Figures 2C,D). As we found that its expression was specific to SP in the embryonic stages (see below), we focused on the analysis of Kcnab1 in this article.
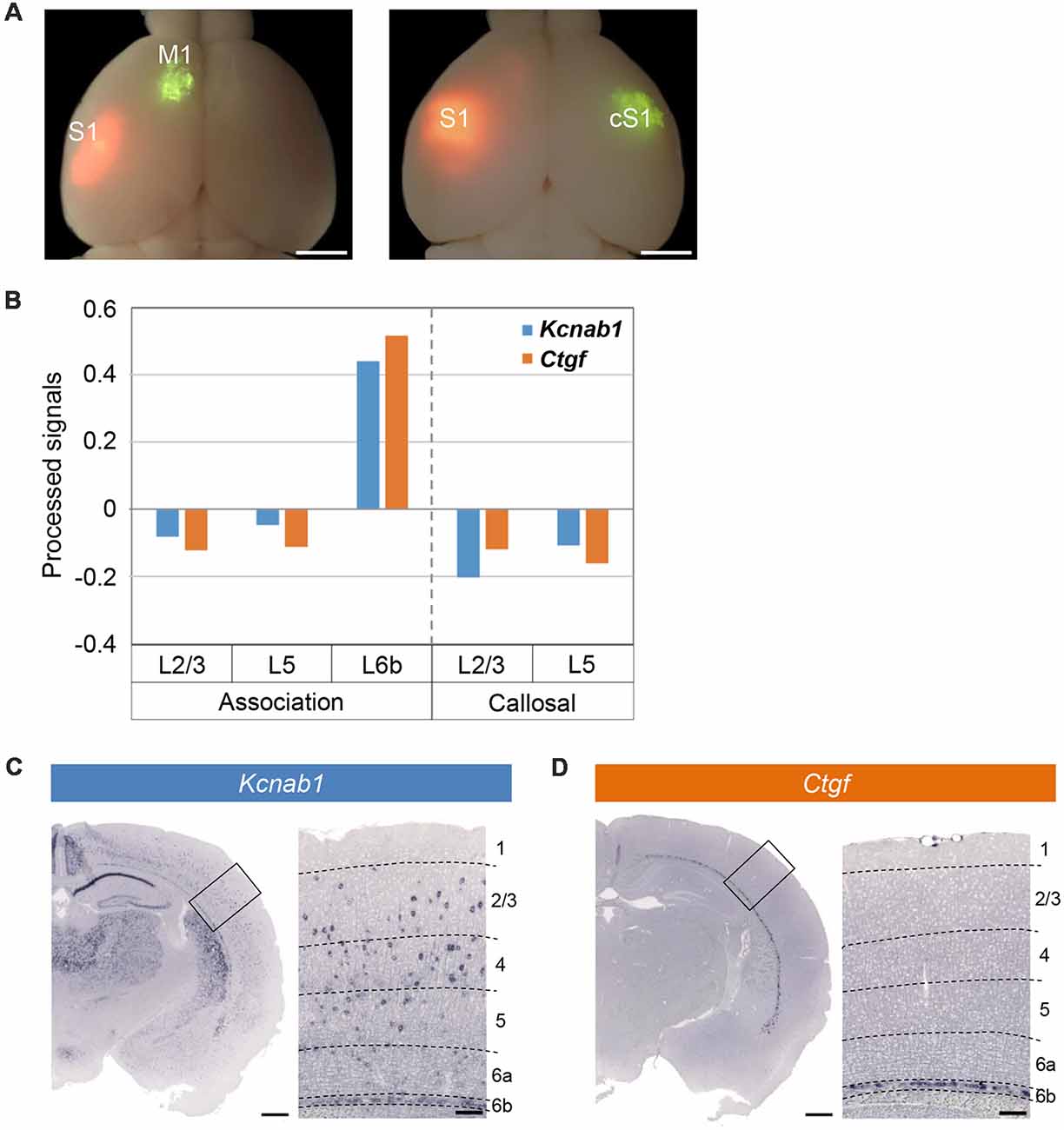
Figure 2. Microarray screening identified Kcnab1 and Ctgf as expressed in L6b. (A) Green RetroBeadsTM was injected into M1 (left panel) or cS1 (right panel) of the brains at PD21 that had been electroporated with pCAGGS-tdTomato at E15.5. Green and red fluorescent images were overlaid onto the bright field image. Scale bars, 2 mm. (B) Relative expression levels of Kcnab1 (blue) and Ctgf (orange) among association neurons in L2/3, L5, and L6b and callosal neurons in L2/3 and L5 compared by microarray analysis. (C,D) In situ hybridization histochemistry (ISHH) for Kcnab1 (C) and Ctgf (D) was carried out on coronal sections of mouse brain at PD21. Higher magnification view of the boxed area is shown on the right of each panel. Dashed lines in these high magnification images indicate borders of cortical layers. Scale bars, 500 μm and 100 μm for low and high magnifications, respectively.
L6b/SP Association Neurons Expressed Kcnab1
From our retrograde tracing experiments and microarray gene analysis, we were inclined to hypothesize that association neurons in L6b/SP were molecularly distinctive. Therefore, brains that were injected with retrograde tracer were processed for ISHH and immunohistochemistry to confirm this hypothesis.
From our retrograde tracing experiment in combination with ISHH, we observed M1-projecting neurons in L6b/SP in S1 of PW3 mice that were double-positive for FG (detected by anti-FG antibody) and Kcnab1 (Figures 3A–C). We examined a total of 366 neurons in S1 L6b/SP (N = 3 mice) that were retrogradely labeled and 83.0 ± 0.02% [mean ± standard error of the mean (SEM)] of them expressed Kcnab1 (Figure 3D, Supplementary Figure S1 and Supplementary Table S1). On the other hand, among 634 Kcnab1-positive neurons in L6b/SP, 50.1 ± 0.10% were of association type. We did not observe any obvious regional biases in cellular distributions of FG+ and Kcnab1+ neurons within S1 (Supplementary Figure S1). Similarly, at the neonatal stage (injection at PD2 and brains fixed at PD4), a proportion of association neurons in L6b/SP expressed Kcnab1 (data not shown).
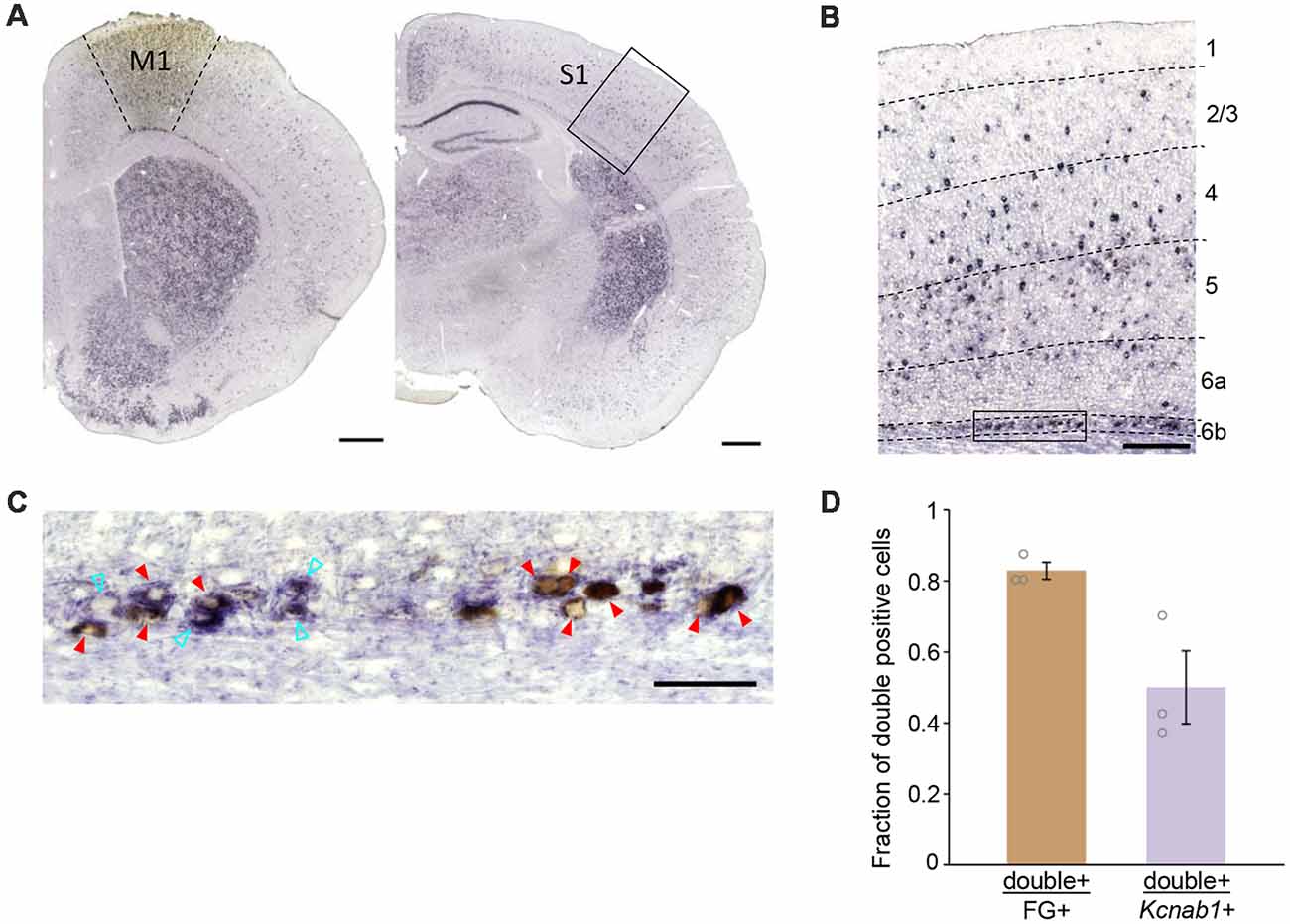
Figure 3. Association neurons in L6b/SP expressed Kcnab1. (A) ISHH was carried out in combination with retrograde tracing detected by anti-FG antibody to determine Kcnab1 expression of the association neurons in the L6b/SP of PW3 mouse. The left panel shows FG injection site in M1, revealed by anti-FG staining (brown patch) and the right panel shows the retrogradely labeled region in S1. Scale bars, 500 μm. (B) Neurons that were double-positive for FG (brown) and Kcnab1 (violet) were observed in L6b as well as the upper layers in S1. Dashed lines indicate borders of cortical layers. Scale bar, 200 μm. (C) Higher magnification view of the boxed area in (B). Red arrowheads indicate double-positive, i.e., association neurons that expressed Kcnab1. Cyan open arrowheads indicate Kcnab1-positive neurons that were not retrogradely labeled. Scale bar, 50 μm. (D) Summary data showing the overlap between neurons retrogradely labeled from M1 (FG+) and Kcnab1+ neurons in L6b/SP at PW3 (n = 366 FG+ neurons and n = 634 Kcnab1+ neurons from N = 3 mice). See also Supplementary Table S1 for the details of quantification results.
Kcnab1 Expression During Embryonic Stage Was Restricted to L6b/SP
Since L6b/SP neurons with a unilateral projection expressed Kcnab1 at both the neonatal and postnatal stages, we set out to examine the onset of Kcnab1 expression in the mouse cortex and whether the expression pattern was maintained throughout development. First, we carried out ISHH for Kcnab1 on coronal sections of the mouse brain at different embryonic stages. Kcnab1 expression was observed in the SP as early as E14.5 (Figure 4), shortly after the SP was formed. Interestingly, Kcnab1 expression was consistently restricted to the SP, the earliest-formed layer, and not detected in other layers throughout embryonic development. Kcnab1 has an earlier onset in comparison to the established L6b/SP marker Ctgf, of which the earliest expression was detected at E16.5 among the stages tested.
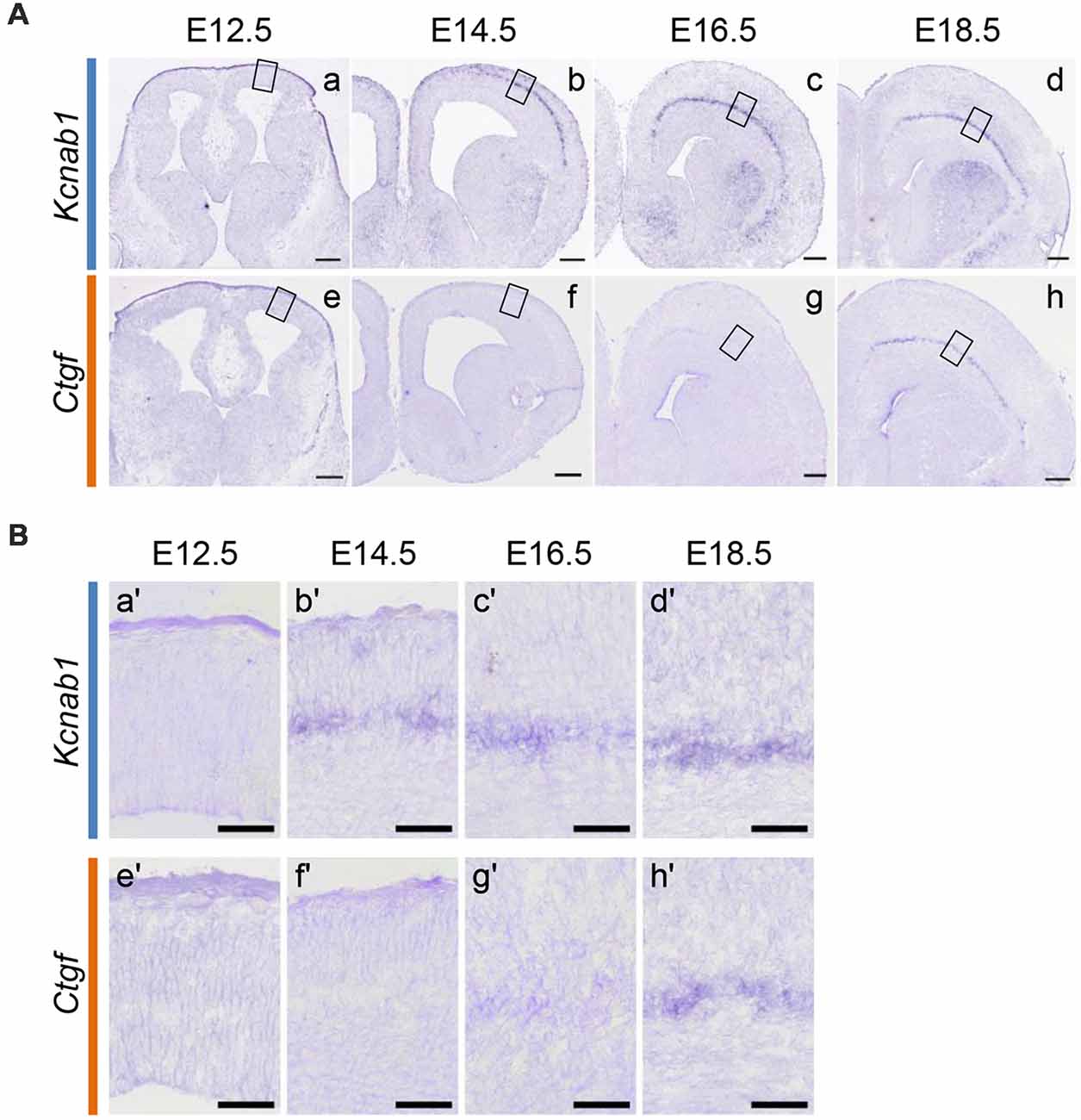
Figure 4. Kcnab1 expression in developing mouse cortex was detected as early as E14.5. (A) ISHH for Kcnab1 (a–d) and Ctgf (e–h) was carried out on coronal sections of the mouse brain at different embryonic stages (E12.5, E14.5, E16.5, and E18.5). Kcnab1 expression was observed starting at E14.5 and it began earlier when compared to Ctgf expression. Scale bar, 200 mm (micrometer). (B) Higher magnification of boxed regions in (A). Kcnab1 expression was restricted to the SP during the embryonic stage (b′–d′). Scale bar 50 μm.
Kcnab1 Expression Spread to Other Layers in the Postnatal Stages
Our results demonstrated that Kcnab1 expression in the mouse cortex changes as the brain matures. Kcnab1-positive neurons were constrained to L6b/SP during embryonic age (Figure 4). However, the expression was less localized in the PD21 brain (Figure 2C). We went on to analyze the expression pattern during early postnatal stages in order to clarify when exactly the expression in other layers started. Our comparison ISHH results showed that Kcnab1 expression was apparent in the upper layers at stage PD3 onwards (Figures 5A,B). As the cortical layers became more prominent, Kcnab1 expression in L6b/SP became weaker whereas numbers of Kcnab1-expressing neurons in other layers increased. Kcnab1-positive neurons in the upper layers were sparse and scattered in layers 2/3, 4, and 5, seemingly with no specific organization pattern (Figure 5B). Together with the expression during the embryonic stage, these data showed that Kcnab1 is expressed in a bipartite manner: a stable expression in the L6b/SP throughout the cortical development and a dynamic expression in other layers that appears postnatally.
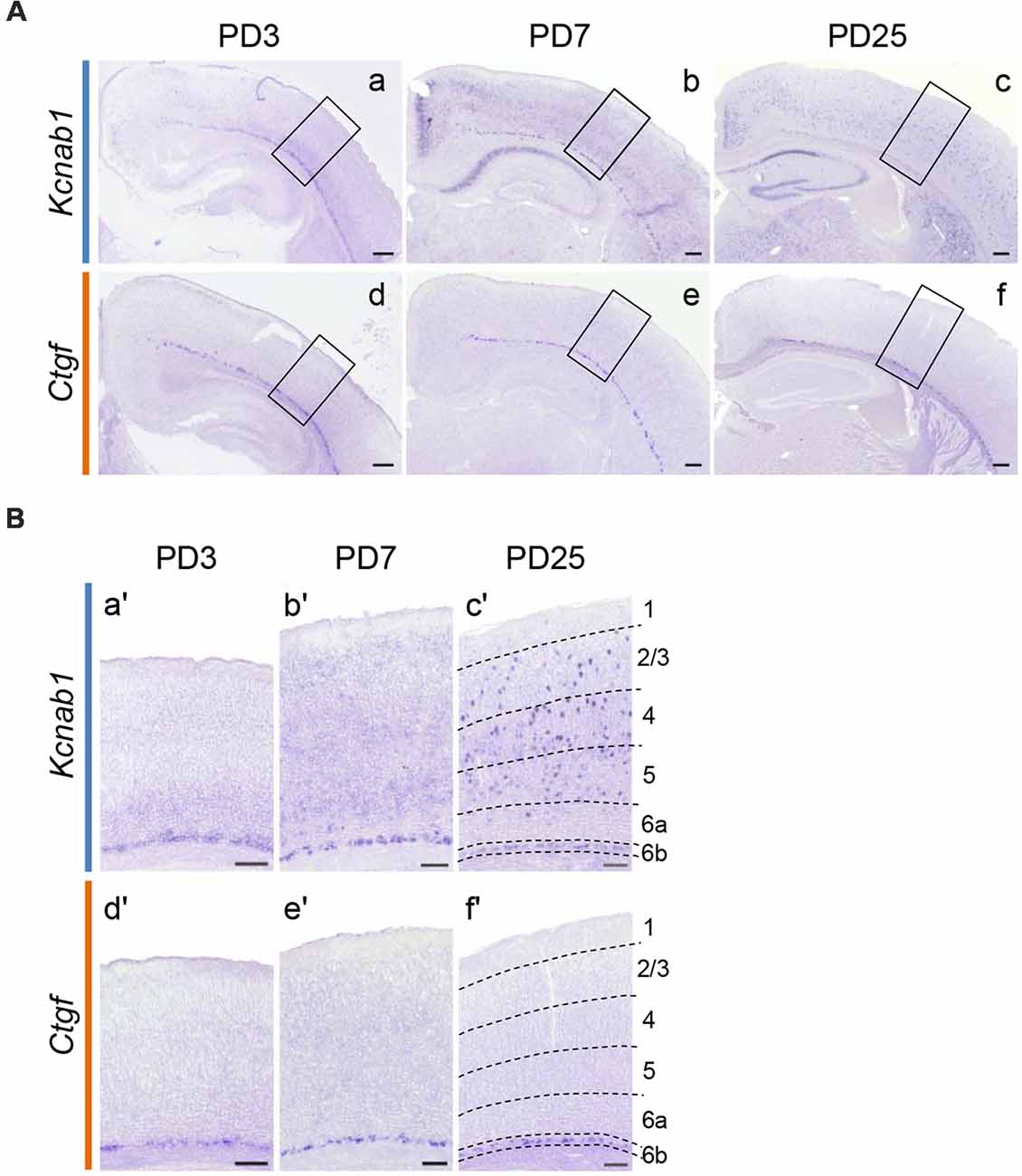
Figure 5. Kcnab1 expression pattern changed during postnatal development. (A) ISHH for Kcnab1 was carried out on coronal sections of the mouse brain at selected postnatal stages (PD3, PD7, and PD25). Scale bars, 200 μm. (B) Higher magnification view of boxed regions in (A). Weak Kcnab1 expression was detected in deep layers above L6b at PD3 (a,a′). Kcnab1 expression gradually spread to the more superficial layers and some cells with higher expression appeared (b,b′), becoming evidently scattered in the upper layers at PD25 (c,c′). In comparison, Ctgf was limited to L6b throughout postnatal development (d–f; d′–f′). Dashed lines in (c′) and (f′) indicate borders of cortical layers. Scale bars, 100 μm.
Kcnab1 Neurons Were a Distinct Subpopulation in L6b/SP
Kcnab1 expression was observed in the mouse cortex shortly after the formation of the SP and it remained throughout development. As a step to further characterize this neuronal subclass in the L6b/SP, we tested the co-localization extent of protein product Kvβ1 and selected L6b/SP markers Ctgf, Cplx3, and Nurr1 (Figures 6A–C) in adult brains. All three markers label neurons in a thin band that comprises 2–3 rows of densely packed cells directly above the white matter, with a certain variation in different cortical areas (Liu and Baker, 1999; Arimatsu et al., 2003; Hoerder-Suabedissen et al., 2009; Wang et al., 2011). L6b/SP neurons identified by these markers are known to overlap to a varying degree (Hoerder-Suabedissen et al., 2009, 2013). We examined a total of 409 Kvβ1-immunoreactive neurons in L6b/SP (in three animals) and a majority (87.8 ± 0.02%) of them co-expressed Ctgf. Among 414 Kvβ1-immunoreactive neurons in L6b/SP (in three animals), more than three quarters (78.7 ± 0.02%) were Cplx3-positive, whereas 57.7 ± 0.04% of a total of 462 Kvβ1-positive neurons examined expressed Nurr1 (Figure 6D and Supplementary Table S2). Among the marker-expressing neurons, the vast majority of them (93.6–95.6%) were Kvβ1-positive (Figure 6D and Supplementary Table S2). Thus, Kcnab1-expressing neurons constitute a distinct subpopulation of L6b/SP neurons.
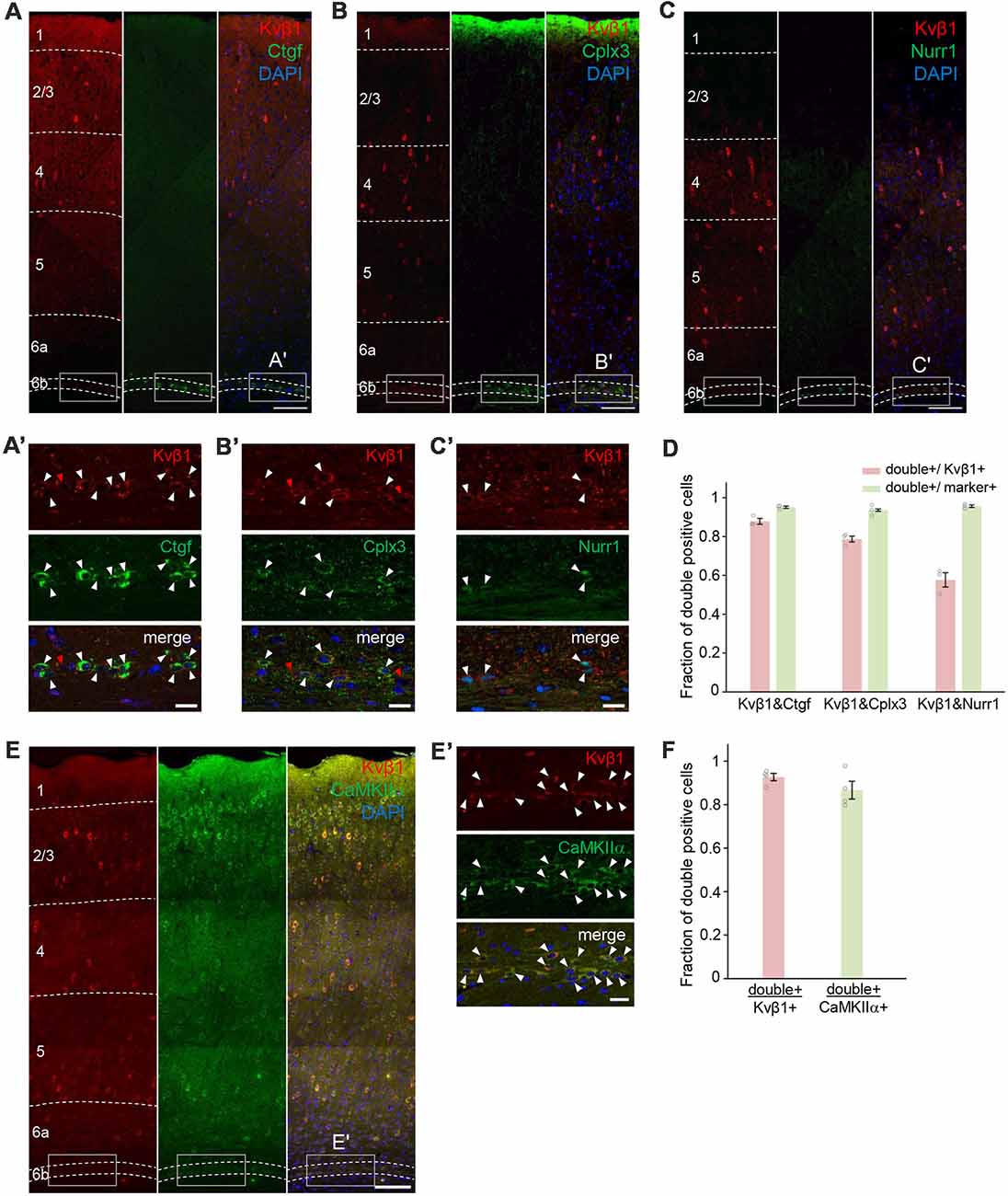
Figure 6. Cellular co-localization of the protein product Kvβ1 and L6b/SP markers in the adult S1. Double immunostaining experiments revealed co-expression of Kvβ1 and L6b/SP markers (A) Ctgf, (B) Cplx3 and (C) Nurr1 in L6b neurons. Dashed lines indicate borders of cortical layers. Scale bars, 100 μm. Boxed areas in (A–C) are shown in higher magnification in (A′–C′). White arrowheads indicate double-positive L6b neurons. Red arrowheads indicate L6b neurons that expressed only Kvβ1. Scale bars, 20 μm. (D) Summary data showing degrees of co-localization. The bar diagrams illustrate the fraction of double-positive cells to Kvβ1- (red) and marker- (green) positive L6b cells. Staining combinations are shown at the bottom of the bars. Kvβ1-positive neurons in L6b expressed all three markers but the proportion of overlap varied. Error bars represent standard error of the mean (SEM; N = 3 animals per marker group). See also Supplementary Table S2 for the details of quantification results. (E) Double immunostaining with excitatory neuronal marker CaMKIIα showed that the majority of Kvβ1-expressing neurons were also CamKIIα-immunoreactive. Dashed lines indicate borders of cortical layers. Scale bar, 100 μm. (E′) Higher magnification images of boxed areas in (E) and arrowheads indicate Kvβ1-CaMKIIα co-localization. Scale bars, 20 μm. (F) Summary data showing high percentages of double-positive neurons both in Kvβ1-expressing neurons and in CaMKIIα-expressing neurons in L6b. Error bars represent SEM (N = 4 animals). See also Supplementary Table S2 for the details of quantification results.
To gain insight into the neurochemical characteristics of Kcnab1-expressing neurons, we also analyzed the co-localization of Kvβ1 and CaMKIIα, a general marker for excitatory neurons in the cortex (Benson et al., 1992; Jones et al., 1994; Zhang et al., 2006; Wang et al., 2013; Pinto and Dan, 2015). Among 837 Kvβ1-positive neurons in L6b/SP, 92.7 ± 0.02% were CaMKIIα-positive (Figures 6E,F and Supplementary Table S2), suggesting that the majority of Kcnab1-expressing neurons were excitatory. A recent study reported that no GABAergic neuron was detected among Ctgf-expressing neurons (Boon et al., 2018). As approximately 12% of Kcnab1-expressing neurons were Ctgf-negative (Figure 6D and Supplementary Table S2), we examined single cell RNAseq data of cortical neurons (Tasic et al., 2016) to see if Kcnab1 is expressed in GABAergic neurons in L6b. Among 41 neurons dissected from L6b, Kcnab1 transcripts were detected in 50.0% of GABAergic neurons as well as in 90.9% of glutamatergic neurons (Supplementary Figure S2). Notably, analysis on the RNAseq data further showed that Kcnab1-expressing neurons in other layers contained both glutamatergic and GABAergic neurons (Supplementary Figure S2). This was also confirmed by the analysis on another RNAseq data set by Zeisel et al. (2015; Supplementary Figure S3).
All Cortical Layers Expressed a Single Kcnab1 Splice Variant
As the presence of three alternative first exons (exon1.1, 1.2 and 1.3) was reported for the human orthologue of Kcnab1 (England et al., 1995; McCormack et al., 1995) and our probe for mouse Kcnab1 spanned from the last quarter of the coding sequence to 3′ UTR shared among three variants, we examined which variant was expressed in the cortex. In the Ensembl genomic database, neither exon 1.2 nor 1.3 was annotated. Thus, we performed the homology search using human orthologous exons as queries and found corresponding mouse exons 1.2 and 1.3 at 132 kb and 169 kb upstream of the exon 1.1, respectively (Figures 7A,B). Amino acid identities between human and mouse proteins within N-terminals encoded by the first exons were 98.6%, 84.0%, and 84.8% for Kvβ1.1, 1.2, and 1.3, respectively. ISHH with the probes specific to each exon revealed that only exon 1.1 among the three variants was expressed in the PD25 cerebral cortex (Figure 7C), recapturing the staining pattern with the probe for 3′ part of the gene (Figure 2C). This held true also for early postnatal stage PD3 (data not shown). Therefore, the expression pattern of Kcnab1 described in this study was explained by that of the variant 1.1 alone.
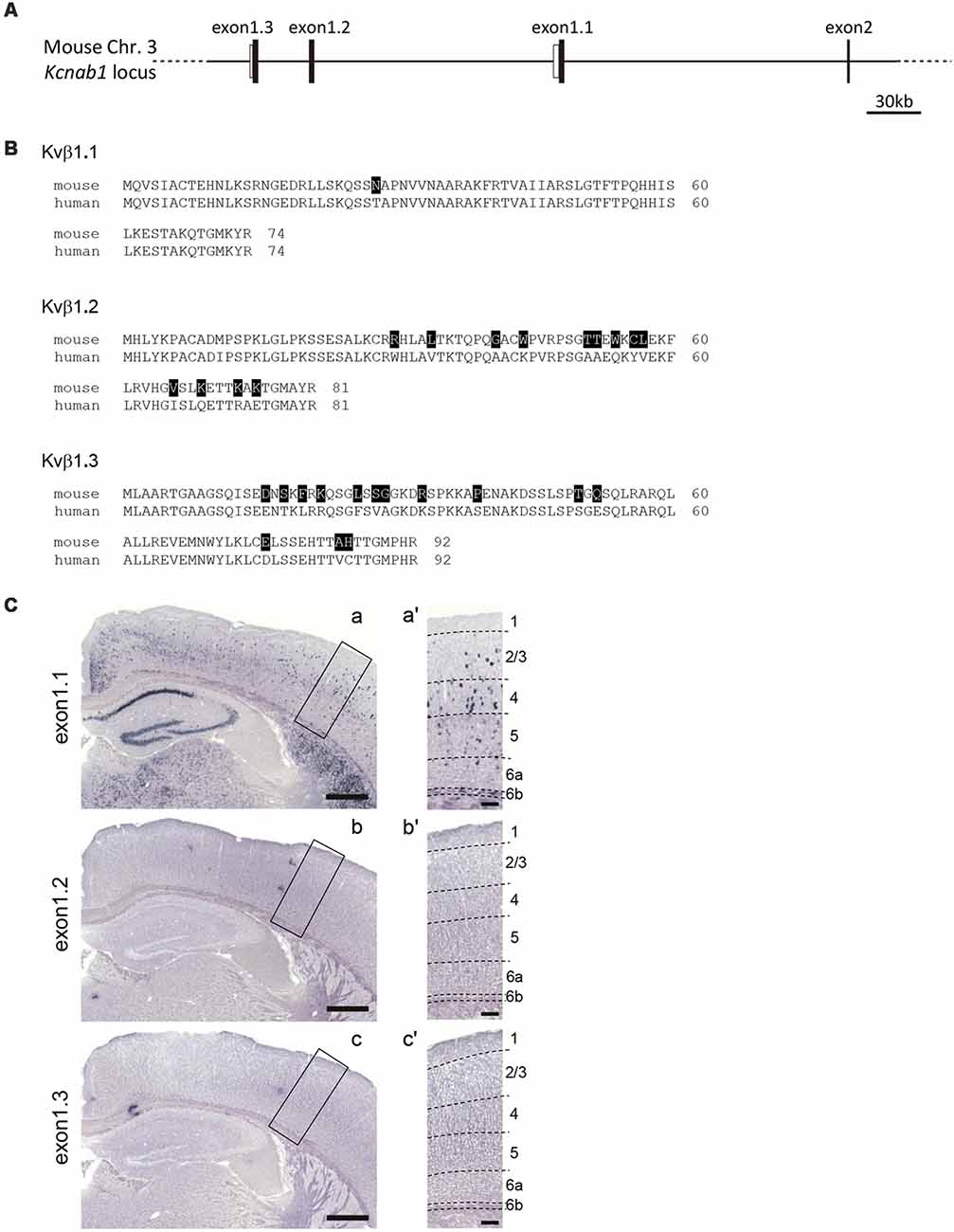
Figure 7. Kcnab1 splice variant Kcnab1.1 was expressed in the mouse cortex. (A) Genomic structure of 5′ part of the Kcnab1 gene locus showing three alternative first exons (exons 1.1–1.3). Filled and open bars indicate coding and non-coding part of the exons. (B) Amino acid sequence alignments of the three alternative N-terminals of the mouse (m) and human (h) Kvβ1 protein encoded by three alternative first exons. The residues in mKvβ1 different from those in hKvβ1 are shown in white letters on black background. (C) ISHH for Kcnab1 splice variants was carried out on PD25 mouse brain sections using the probes specific for exons 1.1–1.3. Only Kcnab1.1 expression was observed in the cortex (a). Neither Kcnab1.2 nor Kcnab1.3 was present in any layers of any cortical areas (b,c). Higher magnification of boxed regions in (a–c) is shown in (a′–c′), an area in S1. Dashed lines indicate borders of cortical layers. Scale bars, 500 μm (a–c); 100 μm (a′–c′).
Discussion
The main findings of this study are: (1) that a discrete population of L6b/SP neurons project unilaterally from S1 to M1, and no callosal projection was observed; (2) that Kcnab1 identifies a proportion of this subclass of L6b/SP neurons with an inter-areal projection; (3) that Kcnab1 is co-expressed with known L6b/SP markers Ctgf, Cplx3, and Nurr1 to different degrees at postnatal stage; and (4) that Kcnab1 expression is restricted to L6b/SP during the early developmental stage but scattered Kcnab1-positive neurons were observed in the upper layers of postnatal cortices.
L6b/SP neurons are strategically located at the interface of the developing cortex and they also occupy a pivotal time window during which cortical connections begin to establish. Thus, axon projections that originate from this region are most likely to influence subsequent functional connectivity organization as cortical lamination proceeds. Projection from L6b/SP as a whole is commonly categorized as corticocortical and corticothalamic, both of which involve morphologically diverse cells (Zhang and Deschênes, 1997; Kumar and Ohana, 2008; Briggs, 2010; Thomson, 2010; Pichon et al., 2012; Marx and Feldmeyer, 2013; Vélez-Fort et al., 2014; Hoerder-Suabedissen et al., 2018). It is of broad opinion that L6b/SP neurons in rodents send few collaterals to the contralateral cortex (De Carlos and O’Leary, 1992; Ozaki and Wahlsten, 1998; Del Río et al., 2000; Hoerder-Suabedissen and Molnár, 2012). A recent report using a transgenic mouse line showed callosal projections from L6b/SP neurons in P7 mice (Hoerder-Suabedissen et al., 2018), although it seems rather hard to exclude the possibility that these projections were from a small population of L6a neurons found in this particular line (Drd1a-Cre (FK164) at GENSAT5). In this study, by retrograde tracing, we observed only ipsilateral inter-areal projection from L6b/SP neurons, and this was consistent at different developmental stages. However, we are yet to confirm if these unilaterally projecting-L6b/SP neurons in S1 solely project to M1 since L6b/SP neurons were reported to have multiple long-range targets (Hoerder-Suabedissen and Molnár, 2012; Hoerder-Suabedissen et al., 2018). Moreover, neonatal SP neurons were shown to project to the opposite hemisphere in other mammalian species (Chun et al., 1987; Antonini and Shatz, 1990). Our finding that Kcnab1 identifies a proportion of the ipsilaterally-projecting neurons in L6b/SP does not exclude the possibility that there are, although most likely minor, SP neurons that have contralateral projections that were not identified in this study due to experimental limitations such as tracer injections into a very restricted area compared with broad applications into the white matter or the corpus callosum in other studies (Ozaki and Wahlsten, 1998; Del Río et al., 2000; Hoerder-Suabedissen and Molnár, 2012; Boon et al., 2018). Hence, it would be most ideal if the tracing experiment is repeated with transgenic reporter line that would allow further characterization of the association neuron subclass, the axon trajectory that originates from this area, and their targets that may include other cortical regions.
Previous studies that combined birth dating of SP neurons and co-expression of SP markers concluded that Ctgf, Cplx3, and Nurr1 indeed labeled early-born SP neurons and, more importantly, a considerable proportion of these early-born neurons survived after the formation of the cortical plate (Liu and Baker, 1999; Arimatsu et al., 2003; Heuer et al., 2003; Hoerder-Suabedissen et al., 2009; Hoerder-Suabedissen and Molnár, 2013). In the adult cortex, we found a prominent overlap between Kcnab1 protein product Kvβ1-immunoreactive neurons and Ctgf, Cplx3, and Nurr1, to varying degrees. This indicates that a considerable number of L6b/SP neurons of distinct identity that were generated during the embryonic stage remain into adulthood. Moreover, our data suggest that there is a molecular subdivision of L6b/SP association neurons. It is possible that one or more of the markers tested are expressed in the Kcnab1-negative population (17%) of the M1-projecting L6b/SP neurons in S1. On the other hand, Kcnab1-expressing neurons without projection to M1 may consist of neurons projecting to other cortical areas including S2, or even to subcortical targets. Furthermore, there is a subdivision of Kcnab1-expressing neurons in terms of neurotransmitters, since Kcnab1 expression in 50% of GABAergic neurons in L6B/SP was evidenced in the analysis on the published single cell RNAseq data sets (Supplementary Figures S2, S3). A similar analysis revealed that some GABAergic neurons expressed the Camk2a gene (Supplementary Figure S4), implying that some of the Kvβ1/CaMKIIα double-positive neurons we detected were possibly GABAergic neurons. It would be an intriguing future work to examine if GABAergic Kcnab1-expressing neurons in L6b/SP have long-range ipsilateral corticocortical projections as shown for a small proportion of GAD65-expressing L6b/SP neurons (Boon et al., 2018).
Kvβ1 is an intracellular regulatory subunit of shaker-type Kv1 channel that rapidly blocks the potassium influx by binding to the channel pore with its N-terminal “ball” domain (Sewing et al., 1996). This fast transient (A-type) current enables neurons to fire repetitively in an input intensity-dependent manner (Connor and Stevens, 1971). The A-type current and input-dependent increase of firing rates are reported for SP neurons, but not for immature pyramidal neurons in the cortical plate of neonatal rat cortex (Luhmann et al., 2000), which is consistent with Kvβ1 expression in L6b/SP. Furthermore, enriched expression in human embryonic SP was reported (Miller et al., 2014), suggesting the conserved regulation and functions of Kvβ1 in prenatal SP between primates and rodents. Previous molecular profiling has reported a variety of genes that are SP-specific at certain developmental stages and only a small number of specific genes—Nurr1, Ctgf, Cplx3, Nxph4, Inpp4b, Htr1d and Tpd52I1 are present in L6b/SP throughout development (Hoerder-Suabedissen et al., 2013). Interestingly, we observed Kvβ1 expression in other cortical layers besides L6b/SP only in the postnatal mouse brain. Thus the unresolved question is whether these Kvβ1 neurons that were scattered in the upper layers were of SP origin (Ozair et al., 2018) or if they were of newly acquired expression-type neurons as development progresses. This would be essential to clarify the function of this L6b/SP subpopulation at different developmental stages since Kvβ1 has been implicated to be involved in certain types of learning and memory (Giese et al., 1998; Murphy et al., 2004).
Heterogeneity of L6b/SP neurons remains a great hurdle to understand the enigmatic role of this likely transient structure. L6b/SP is a robust zone that contains both inhibitory and excitatory neurons (Hoerder-Suabedissen and Molnár, 2015), and it also forms subcortical connections (Killackey and Sherman, 2003; Roth et al., 2016; Chevée et al., 2018; Hoerder-Suabedissen et al., 2018). Although connection between L6b/SP within and outside of the cortex seems to vary across mammalian species (De Carlos and O’Leary, 1992; Ozaki and Wahlsten, 1998; Del Río et al., 2000; Briggs and Usrey, 2007), it is important to note that L6b/SP neurons are essentially involved in cortical information processing. Previous reports have suggested that neurons in L6b/SP go through timely functional changes throughout development (Friedlander and Torres-Reveron, 2009). In this study, however, we have identified a group of L6b/SP association neurons that expressed a specific splice variant of Kcnab1, and the expression in L6b/SP was maintained at all developmental stages. This molecular authenticity that spans development could be a plausible mechanism that modulates L6b/SP neuron specification during cortical maturation, and this would add to the increasing efforts to characterize L6b/SP neurons, thus elucidating the role of L6b/SP neurons in SP development, axon extension, and subsequent brain network establishment.
Ethics Statement
All animal experiments were approved by Animal Research Committee of University of Fukui and Animal Experimentation Committee of Osaka University, and performed in accordance with Regulations for Animal Research at University of Fukui and Regulations on Animal Experimentation at Osaka University.
Author Contributions
ST, YO, and MS designed the research. ST, YO, TS, MT, and MD performed the experiments. ST, YO, TS, and HA analyzed the data. ST, YO, and MS wrote the manuscript.
Funding
This work was supported by grants from Takeda Science Foundation, NOVARTIS Foundation (Japan) for the Promotion of Science, Naito Foundation, and Japan Society for the Promotion of Science (JSPS) KAKENHI [Grant numbers JP23800027, JP24700352, JP25123704, JP26830027 and JP17K07076 (to YO) and JP15K15015, JP25293043, and JP17H04014 (to MS)]. ST was supported by The Ministry of Education, Malaysia (University of Malaya HLCB/PK 2015).
Conflict of Interest Statement
The authors declare that the research was conducted in the absence of any commercial or financial relationships that could be construed as a potential conflict of interest.
Acknowledgments
We thank H. Yoshikawa, S. Kanae, I. Kumano, H. Miyagoshi, C.-C. Wang, and A. Emi for technical assistance, T. Iguchi, S. Yasumura, K. Kuroda, M.-J. Xie, and H. Yagi for helpful discussion, and T. Taniguchi, M. Yamaguchi and Y. Shibuya for secretarial assistance. The microarray screening was supported by the Division of Bioresearch of Life Science Research Laboratory, University of Fukui. Animal experiments were supported by both the Division Laboratory Animal Resources of Life Science Research Laboratory, University of Fukui and The Institute of Experimental Animal Science, Faculty of Medicine, Osaka University.
Footnotes
- ^ http://www.ensembl.org/
- ^ https://www.ebi.ac.uk/Tools/psa/genewise/
- ^ www.ncbi.nlm.nih.gov/geo
- ^ http://linnarssonlab.org/cortex
- ^ http://www.gensat.org/
Supplementary Material
The Supplementary Material for this article can be found online at: https://www.frontiersin.org/articles/10.3389/fnana.2019.00039/full#supplementary-material
References
Allendoerfer, K., and Shatz, C. J. (1994). The subplate, a transient neocortical structure: its role in the development of connections between thalamus and cortex. Annu. Rev. Neurosci. 17, 185–218. doi: 10.1146/annurev.ne.17.030194.001153
Andjelic, S., Gallopin, T., Cauli, B., Hill, E. L., Roux, L., Badr, S., et al. (2009). Glutamatergic nonpyramidal neurons from neocortical layer VI and their comparison with pyramidal and spiny stellate neurons. J. Neurophysiol. 101, 641–654. doi: 10.1152/jn.91094.2008
Antonini, A., and Shatz, C. J. (1990). Relation between putative transmitter phenotypes and connectivity of subplate neurons during cerebral cortical development. Eur. J. Neurosci. 2, 744–761. doi: 10.1111/j.1460-9568.1990.tb00465.x
Arimatsu, Y., Ishida, M., Kaneko, T., Ichinose, S., and Omori, A. (2003). Organization and development of corticocortical associative neurons expressing the orphan nuclear receptor Nurr1. J. Comp. Neurol. 466, 180–196. doi: 10.1002/cne.10875
Bayer, S. A., and Altman, J. (1990). Development of layer I and the subplate in the rat neocortex. Exp. Neurol. 107, 48–62. doi: 10.1016/0014-4886(90)90062-w
Belgard, T. G., Marques, A. C., Oliver, P. L., Abaan, H. O., Sirey, T. M., Hoerder-Suabedissen, A., et al. (2011). A transcriptomic atlas of mouse neocortical layers. Neuron 71, 605–616. doi: 10.1016/j.neuron.2011.06.039
Benson, D. L., Isackson, P. J., Gall, C. M., and Jones, E. G. (1992). Contrasting patterns in the localization of glutamic acid decarboxylase and Ca2+/calmodulin protein kinase gene expression in the rat central nervous system. Neuroscience 46, 825–849. doi: 10.1016/0306-4522(92)90188-8
Boon, J., Clarke, E., Kessaris, N., Goffinet, A., Molnár, Z., and Hoerder-Suabedissen, A. (2018). Long-range projections from sparse populations of GABAergic neurons in murine subplate. J. Comp. Neurol. doi: 10.1002/cne.24592 [Epub ahead of print].
Briggs, F. (2010). Organizing principles of cortical layer 6. Front. Neural Circuits 4:3. doi: 10.3389/neuro.04.003.2010
Briggs, F., and Usrey, W. M. (2007). A fast, reciprocal pathway between the lateral geniculate nucleus and visual cortex in the macaque monkey. J. Neurosci. 27, 5431–5436. doi: 10.1523/JNEUROSCI.1035-07.2007
Chen, C.-C., Abrams, S., Pinhas, A., and Brumberg, J. C. (2009). Morphological heterogeneity of layer VI neurons in mouse barrel cortex. J. Comp. Neurol. 512, 726–746. doi: 10.1002/cne.21926
Chevée, M., Robertson, J. D. J., Cannon, G. H., Brown, S. P., and Goff, L. A. (2018). Variation in activity state, axonal projection and position define the transcriptional identity of individual neocortical projection neurons. Cell Rep. 22, 441–455. doi: 10.1016/j.celrep.2017.12.046
Chun, J. J. M., Nakamura, M. J., and Shatz, C. J. (1987). Transient cells of the developing mammalian telencephalon are peptide-immunoreactive neurons. Nature 325, 617–620. doi: 10.1038/325617a0
Chun, J. J. M., and Shatz, C. J. (1989). Interstitial cells of the adult neocortical white matter are the remnant of the early generated subplate neuron population. J. Comp. Neurol. 282, 555–569. doi: 10.1002/cne.902820407
Clancy, B., and Cauller, L. J. (1999). Widespread projections from subgriseal neurons (layer VII) to layer I in adult rat cortex. J. Comp. Neurol. 407, 275–286. doi: 10.1002/(SICI)1096-9861(19990503)407:2<275::AID-CNE8>3.0.CO;2-0
Clascá, F., Angelucci, A., and Sur, M. (1995). Layer-specific programs of development in neocortical projection neurons. Proc. Natl. Acad. Sci. U S A 92, 11145–11149. doi: 10.1073/pnas.92.24.11145
Connor, J. A., and Stevens, C. F. (1971). Inward and delayed outward membrane currents in isolated neural somata under voltage clamp. J. Physiol. 213, 1–19. doi: 10.1113/jphysiol.1971.sp009364
De Carlos, J. A., and O’Leary, D. D. M. (1992). Growth and targeting of subplate axons and establishment of major cortical pathways. J. Neurosci. 12, 1194–1211. doi: 10.1523/jneurosci.12-04-01194.1992
Del Río, J. A., Martínez, A., Auladell, C., and Soriano, E. (2000). Developmental history of the subplate and developing white matter in the murine neocortex. neuronal organization and relationship with the main afferent systems at embryonic and perinatal stages. Cereb. Cortex 10, 784–801. doi: 10.1093/cercor/10.8.784
Deng, R., Kao, J. P. Y., and Kanold, P. O. (2017). Distinct translaminar glutamatergic circuits to GABAergic interneurons in the neonatal auditory cortex. Cell Rep. 19, 1141–1150. doi: 10.1016/j.celrep.2017.04.044
Dupont, E., Hanganu, I. L., Kilb, W., Hirsch, S., and Luhmann, H. J. (2006). Rapid developmental switch in the mechanisms driving early cortical columnar networks. Nature 439, 79–83. doi: 10.1038/nature04264
England, S. K., Uebele, V. N., Kodali, J., Bennett, P. B., and Tamkunt, M. M. (1995). A novel K+ channel β-subunit (hKvβ1.3) is produced via alternative mRNA splicing. J. Biol. Chem. 270, 28531–28534. doi: 10.1074/jbc.270.48.28531
Finney, E. M., Stone, J. R., and Shatz, C. J. (1998). Major glutamatergic projection from subplate into visual cortex during development. J. Comp. Neurol. 398, 105–118. doi: 10.1002/(SICI)1096-9861(19980817)398:1<105::AID-CNE7>3.0.CO;2-5
Friauf, E., McConnell, S. K., and Shatz, C. J. (1990). Functional synaptic circuits in the subplate during fetal and early postnatal development of cat visual cortex. J. Neurosci. 10, 2601–2613. doi: 10.1523/jneurosci.10-08-02601.1990
Friauf, E., and Shatz, C. (1991). Changing patterns of synaptic input to subplate and cortical plate during development of visual cortex. J. Neurophysiol. 66, 2059–2071. doi: 10.1152/jn.1991.66.6.2059
Friedlander, M. J., and Torres-Reveron, J. (2009). The changing roles of neurons in the cortical subplate. Front. Neuroanat. 3:15. doi: 10.3389/neuro.05.015.2009
Ghosh, A., Antonini, A., McConnell, S. K., and Shatz, C. J. (1990). Requirement for subplate neurons in the formation of thalamocortical connections. Nature 347, 179–181. doi: 10.1038/347179a0
Ghosh, A., and Shatz, C. J. (1993). A role for subplate neurons in the patterning of connections from thalamus to neocortex. Development 117, 1031–1047.
Giese, K. P., Storm, J. F., Reuter, D., Fedorov, N. B., Shao, L. R., Leicher, T., et al. (1998). Reduced K+ channel inactivation, spike broadening and after-hyperpolarization in Kvbeta1.1-deficient mice with impaired learning. Learn. Mem. 5, 257–273.
Hanganu, I. L., Kilb, W., and Luhmann, H. J. (2002). Functional synaptic projections onto subplate neurons in neonatal rat somatosensory cortex. J. Neurosci. 22, 7165–7176. doi: 10.1523/jneurosci.22-16-07165.2002
Heuer, H., Christ, S., Friedrichsen, S., Brauer, D., Winckler, M., Bauer, K., et al. (2003). Connective tissue growth factor: a novel marker of layer vii neurons in the rat cerebral cortex. Neuroscience 119, 43–52. doi: 10.1016/s0306-4522(03)00100-3
Hoerder-Suabedissen, A., Hayashi, S., Upton, L., Nolan, Z., Casas-Torremocha, D., Grant, E., et al. (2018). Subset of cortical layer 6b neurons selectively innervates higher order thalamic nuclei in mice. Cereb. Cortex 28, 1882–1897. doi: 10.1093/cercor/bhy036
Hoerder-Suabedissen, A., and Molnár, Z. (2012). Morphology of mouse subplate cells with identified projection targets changes with age. J. Comp. Neurol. 520, 174–185. doi: 10.1002/cne.22725
Hoerder-Suabedissen, A., and Molnár, Z. (2013). Molecular diversity of early-born subplate neurons. Cereb. Cortex 23, 1473–1483. doi: 10.1093/cercor/bhs137
Hoerder-Suabedissen, A., and Molnár, Z. (2015). Development, evolution and pathology of neocortical subplate neurons. Nat. Rev. Neurosci. 16, 133–146. doi: 10.1038/nrn3915
Hoerder-Suabedissen, A., Oeschger, F. M., Krishnan, M. L., Belgard, T. G., Wang, W. Z., Lee, S., et al. (2013). Expression profiling of mouse subplate reveals a dynamic gene network and disease association with autism and schizophrenia. Proc. Natl. Acad. Sci. U S A 110, 3555–3560. doi: 10.1073/pnas.1218510110
Hoerder-Suabedissen, A., Wang, W. Z., Lee, S., Davies, K. E., Goffinet, A. M., Rakić, S., et al. (2009). Novel markers reveal subpopulations of subplate neurons in the murine cerebral cortex. Cereb. Cortex 19, 1738–1750. doi: 10.1093/cercor/bhn195
Jones, E. G., Huntley, G. W., and Benson, D. L. (1994). Alpha calcium/calmodulin-dependent protein kinase II selectively expressed in a subpopulation of excitatory neurons in monkey sensory-motor cortex: comparison with GAD-67 expression. J. Neurosci. 14, 611–629. doi: 10.1523/jneurosci.14-02-00611.1994
Kanold, P. O. (2009). Subplate neurons: crucial regulators of cortical development and plasticity. Front. Neuroanat. 3:16. doi: 10.3389/neuro.05.016.2009
Kanold, P. O., Kara, P., Reid, R. C., and Shatz, C. J. (2003). Role of subplate neurons in functional maturation of visual cortical columns. Science 301, 521–525. doi: 10.1126/science.1084152
Kanold, P. O., and Luhmann, H. J. (2010). The subplate and early cortical circuits. Annu. Rev. Neurosci. 33, 23–48. doi: 10.1146/annurev-neuro-060909-153244
Kanold, P. O., and Shatz, C. J. (2006). Subplate neurons regulate maturation of cortical inhibition and outcome of ocular dominance plasticity. Neuron 51, 627–638. doi: 10.1016/j.neuron.2006.07.008
Killackey, H. P., and Sherman, S. M. (2003). Corticothalamic projections from the rat primary somatosensory cortex. J. Neurosci. 23, 7381–7384. doi: 10.1523/jneurosci.23-19-07381.2003
Kostovic, I., and Rakic, P. (1990). Developmental history of the transient subplate zone in the visual and somatosensory cortex of the macaque monkey and human brain. J. Comp. Neurol. 297, 441–470. doi: 10.1002/cne.902970309
Kumar, P., and Ohana, O. (2008). Inter- and intralaminar subcircuits of excitatory and inhibitory neurons in layer 6a of the rat barrel cortex. J. Neurophysiol. 100, 1909–1922. doi: 10.1152/jn.90684.2008
Lein, E. S., Finney, E. M., McQuillen, P. S., and Shatz, C. J. (1999). Subplate neuron ablation alters neurotrophin expression and ocular dominance column formation. Proc. Natl. Acad. Sci. U S A 96, 13491–13495. doi: 10.1073/pnas.96.23.13491
Liu, N., and Baker, H. (1999). Activity-dependent Nurr1 and NGFI-B gene expression in adult mouse olfactory bulb. Neuroreport 10, 747–751. doi: 10.1097/00001756-199903170-00016
Luhmann, H. J., Kilb, W., and Hanganu-Opatz, I. L. (2009). Subplate cells: amplifiers of neuronal activity in the developing cerebral cortex. Front. Neuroanat. 3:19. doi: 10.3389/neuro.05.019.2009
Luhmann, H. J., Kirischuk, S., and Kilb, W. (2018). The superior function of the subplate in early neocortical development. Front. Neuroanat. 12:97. doi: 10.3389/fnana.2018.00097
Luhmann, H. J., Reiprich, R. A., Hanganu, I., and Kilb, W. (2000). Cellular physiology of the neonatal rat cerebral cortex: intrinsic membrane properties, sodium and calcium currents. J. Neurosci. Res. 62, 574–584. doi: 10.1002/1097-4547(20001115)62:4<574::aid-jnr12>3.0.co;2-0
Magnani, D., Hasenpusch-Theil, K., and Theil, T. (2012). Gli3 controls subplate formation and growth of cortical axons. Cereb. Cortex 23, 2542–2551. doi: 10.1093/cercor/bhs237
Marx, M., and Feldmeyer, D. (2013). Morphology and physiology of excitatory neurons in layer 6b of the somatosensory rat barrel cortex. Cereb. Cortex 23, 2803–2817. doi: 10.1093/cercor/bhs254
Marx, M., Qi, G., Hanganu-Opatz, I. L., Kilb, W., Luhmann, H. J., and Feldmeyer, D. (2017). Neocortical layer 6B as a remnant of the subplate—a morphological comparison. Cereb. Cortex 27, 1011–1026. doi: 10.1093/cercor/bhv279
McConnell, S. K., Ghosh, A., and Shatz, C. J. (1989). Subplate neurons pioneer the first axon pathway from the cerebral cortex. Science 245, 978–982. doi: 10.1126/science.2475909
McCormack, K., McCormack, T., Tanouye, M., Rudy, B., and Stühmer, W. (1995). Alternative splicing of the human Shaker K+channel β1 gene and functional expression of the β2 gene product. FEBS Lett. 370, 32–36. doi: 10.1016/0014-5793(95)00785-8
McKellar, C. E., and Shatz, C. J. (2009). Synaptogenesis in purified cortical subplate neurons. Cereb. Cortex 19, 1723–1737. doi: 10.1093/cercor/bhn194
Miller, J. A., Ding, S.-L., Sunkin, S. M., Smith, K. A., Ng, L., Szafer, A., et al. (2014). Transcriptional landscape of the prenatal human brain. Nature 508, 199–206. doi: 10.1038/nature13185
Mitchell, B. D., and Macklis, J. D. (2005). Large-scale maintenance of dual projections by callosal and frontal cortical projection neurons in adult mice. J. Comp. Neurol. 482, 17–32. doi: 10.1002/cne.20428
Molnár, Z., Adams, R., and Blakemore, C. (1998). Mechanisms underlying the early establishment of thalamocortical connections in the rat. J. Neurosci. 18, 5723–5745. doi: 10.1523/jneurosci.18-15-05723.1998
Murphy, G. G., Fedorov, N. B., Giese, K. P., Ohno, M., Friedman, E., Chen, R., et al. (2004). Increased neuronal excitability, synaptic plasticity and learning in aged Kvβ1.1 knockout mice. Curr. Biol. 14, 1907–1915. doi: 10.1016/j.cub.2004.10.021
Oeschger, F. M., Wang, W. Z., Lee, S., García-Moreno, F., Goffinet, A. M., Arbonés, M. L., et al. (2012). Gene expression analysis of the embryonic subplate. Cereb. Cortex 22, 1343–1359. doi: 10.1093/cercor/bhr197
Osheroff, H., and Hatten, M. E. (2009). Gene expression profiling of preplate neurons destined for the subplate: genes involved in transcription, axon extension, neurotransmitter regulation, steroid hormone signaling and neuronal survival. Cereb. Cortex 19, i126–i134. doi: 10.1093/cercor/bhp034
Ozair, M. Z., Kirst, C., van den Berg, B. L., Ruzo, A., Rito, T., and Brivanlou, A. H. (2018). hPSC modeling reveals that fate selection of cortical deep projection neurons occurs in the subplate. Cell Stem Cell 23, 60–73.e6. doi: 10.1016/j.stem.2018.05.024
Ozaki, H. S., and Wahlsten, D. (1998). Timing and origin of the first cortical axons to project through the corpus callosum and the subsequent emergence of callosal projection cells in mouse. J. Comp. Neurol. 400, 197–206. doi: 10.1002/(sici)1096-9861(19981019)400:2<197::aid-cne3>3.0.co;2-4
Paxinos, G., and Franklin, K. (2008). The Mouse Brain in Stereotaxic Coordinates, Compact 3rd Edition. 3rd Edn. San Diego, CA: Academic Press.
Pedraza, M., Hoerder-Suabedissen, A., Albert-Maestro, M. A., Molnár, Z., and De Carlos, J. A. (2014). Extracortical origin of some murine subplate cell populations. Proc. Natl. Acad. Sci. U S A 111, 8613–8618. doi: 10.1073/pnas.1323816111
Pichon, F., Nikonenko, I., Kraftsik, R., and Welker, E. (2012). Intracortical connectivity of layer VI pyramidal neurons in the somatosensory cortex of normal and barrelless mice. Eur. J. Neurosci. 35, 855–869. doi: 10.1111/j.1460-9568.2012.08011.x
Piñon, M. C., Jethwa, A., Jacobs, E., Campagnoni, A., and Molnár, Z. (2009). Dynamic integration of subplate neurons into the cortical barrel field circuitry during postnatal development in the Golli-tau-eGFP (GTE) mouse. J. Physiol. 587, 1903–1915. doi: 10.1113/jphysiol.2008.167767
Pinto, L., and Dan, Y. (2015). Cell-type-specific activity in prefrontal cortex during goal-directed behavior. Neuron 87, 437–450. doi: 10.1016/j.neuron.2015.06.021
Reep, R. (2000). Cortical layer VII and persistent subplate cells in mammalian brains. Brain. Behav. Evol. 56, 212–234. doi: 10.1159/000047206
Reep, R. L., and Goodwin, G. S. (1988). Layer VII of rodent cerebral cortex. Neurosci. Lett. 90, 15–20. doi: 10.1016/0304-3940(88)90779-3
Robertson, R. T., Annis, C. M., Baratta, J., Haraldson, S., Ingeman, J., Kageyama, G. H., et al. (2000). Do subplate neurons comprise a transient population of cells in developing neocortex of rats? J. Comp. Neurol. 426, 632–650. doi: 10.1002/1096-9861(20001030)426:4<632::AID-CNE10>3.0.CO;2-4
Roth, M. M., Dahmen, J. C., Muir, D. R., Imhof, F., Martini, F. J., and Hofer, S. B. (2016). Thalamic nuclei convey diverse contextual information to layer 1 of visual cortex. Nat. Neurosci. 19, 299–307. doi: 10.1038/nn.4197
Sewing, S., Roeper, J., and Pongs, O. (1996). Kvβ1 subunit binding specific for Shaker-related potassium channel α subunits. Neuron 16, 455–463. doi: 10.1016/s0896-6273(00)80063-x
Sorensen, S. A., Bernard, A., Menon, V., Royall, J. J., Glattfelder, K. J., Desta, T., et al. (2015). Correlated gene expression and target specificity demonstrate excitatory projection neuron diversity. Cereb. Cortex 25, 433–449. doi: 10.1093/cercor/bht243
Tasic, B., Menon, V., Nguyen, T. N., Kim, T. K., Jarsky, T., Yao, Z., et al. (2016). Adult mouse cortical cell taxonomy revealed by single cell transcriptomics. Nat. Neurosci. 19, 335–346. doi: 10.1038/nn.4216
Thomson, A. M. (2010). Neocortical layer 6, a review. Front. Neuroanat. 4:13. doi: 10.3389/fnana.2010.00013
Tolner, E. A., Sheikh, A., Yukin, A. Y., Kaila, K., and Kanold, P. O. (2012). Subplate neurons promote spindle bursts and thalamocortical patterning in the neonatal rat somatosensory cortex. J. Neurosci. 32, 692–702. doi: 10.1523/jneurosci.1538-11.2012
Usrey, W., and Fitzpatrick, D. (1996). Specificity in the axonal connections of layer VI neurons in tree shrew striate cortex: evidence for distinct granular and supragranular systems. J. Neurosci. 16, 1203–1218. doi: 10.1523/jneurosci.16-03-01203.1996
Valverde, F., López-Mascaraque, L., Santacana, M., and De Carlos, J. A. (1995). Persistence of early-generated neurons in the rodent subplate: assessment of cell death in neocortex during the early postnatal period. J. Neurosci. 15, 5014–5024. doi: 10.1523/jneurosci.15-07-05014.1995
Vélez-Fort, M., Rousseau, C. V., Niedworok, C. J., Wickersham, I. R., Rancz, E. A., Brown, A. P. Y., et al. (2014). The stimulus selectivity and connectivity of layer six principal cells reveals cortical microcircuits underlying visual processing. Neuron 83, 1431–1443. doi: 10.1016/j.neuron.2014.08.001
Viswanathan, S., Sheikh, A., Looger, L. L., and Kanold, P. O. (2017). Molecularly defined subplate neurons project both to thalamocortical recipient layers and thalamus. Cereb. Cortex 27, 4759–4768. doi: 10.1093/cercor/bhw271
Wang, W. Z., Oeschger, F. M., Montiel, J. F., García-Moreno, F., Hoerder-Suabedissen, A., and Krubitzer, L. (2011). Comparative aspects of subplate zone studied with gene expression in sauropsids and mammals. Cereb. Cortex 21, 2187–2203. doi: 10.1093/cercor/bhq278
Wang, X., Zhang, C., Szábo, G., and Sun, Q. Q. (2013). Distribution of CaMKIIα expression in the brain in vivo, studied by CaMKIIα-GFP mice. Brain Res. 1518, 9–25. doi: 10.1016/j.brainres.2013.04.042
Woo, T. U., Beale, J. M., and Finlay, B. L. (1991). Dual fate of subplate neurons in a rodent. Cereb. Cortex 1, 433–443. doi: 10.1093/cercor/1.5.433
Yagi, H., Oka, Y., Komada, M., Xie, M. J., Noguchi, K., and Sato, M. (2016). Filamin A interacting protein plays a role in proper positioning of callosal projection neurons in the cortex. Neurosci. Lett. 612, 18–24. doi: 10.1016/j.neulet.2015.11.049
Yang, J.-W., Reyes-Puerta, V., Kilb, W., and Luhmann, H. J. (2016). Spindle bursts in neonatal rat cerebral cortex. Neural Plast. 2016:3467832. doi: 10.1155/2016/3467832
Zeisel, A., Muñoz-Manchado, A. B., Codeluppi, S., Lönnerberg, P., La Manno, G., Juréus, A., et al. (2015). Cell types in the mouse cortex and hippocampus revealed by single-cell RNA-seq. Science 347, 1138–1142. doi: 10.1126/science.aaa1934
Zhang, Z. W., and Deschênes, M. (1997). Intracortical axonal projections of lamina VI cells of the primary somatosensory cortex in the rat: a single-cell labeling study. J. Neurosci. 17, 6365–6379. doi: 10.1523/jneurosci.17-16-06365.1997
Zhang, C., Szabó, G., Erdélyi, F., Rose, J. D., and Sun, Q. Q. (2006). Novel interneuronal network in the mouse posterior piriform cortex. J. Comp. Neurol. 499, 1000–1015. doi: 10.1002/cne.21166
Keywords: subplate, axon projection, Kcnab1, cerebral cortex, potassium channel, corticocortical projection, neuronal circuit, FluoroGold
Citation: Tiong SYX, Oka Y, Sasaki T, Taniguchi M, Doi M, Akiyama H and Sato M (2019) Kcnab1 Is Expressed in Subplate Neurons With Unilateral Long-Range Inter-Areal Projections. Front. Neuroanat. 13:39. doi: 10.3389/fnana.2019.00039
Received: 10 December 2018; Accepted: 20 March 2019;
Published: 03 May 2019.
Edited by:
Francisco Clasca, Autonomous University of Madrid, SpainReviewed by:
Kouichi C. Nakamura, University of Oxford, United KingdomZoltan Molnar, University of Oxford, United Kingdom
Diana Casas-Torremocha, Autonomous University of Madrid, Spain
Copyright © 2019 Tiong, Oka, Sasaki, Taniguchi, Doi, Akiyama and Sato. This is an open-access article distributed under the terms of the Creative Commons Attribution License (CC BY). The use, distribution or reproduction in other forums is permitted, provided the original author(s) and the copyright owner(s) are credited and that the original publication in this journal is cited, in accordance with accepted academic practice. No use, distribution or reproduction is permitted which does not comply with these terms.
*Correspondence: Makoto Sato, makosato@anat2.med.osaka-u.ac.jp
† These authors have contributed equally to this work