Establishment of topographic circuit zones in the cerebellum of scrambler mutant mice
- Department of Pathology and Immunology, Department of Neuroscience, Baylor College of Medicine, Jan and Dan Duncan Neurological Research Institute of Texas Children's Hospital, Houston, TX, USA
The cerebellum is organized into zonal circuits that are thought to regulate ongoing motor behavior. Recent studies suggest that neuronal birthdates, gene expression patterning, and apoptosis control zone formation. Importantly, developing Purkinje cell zones are thought to provide the framework upon which afferent circuitry is organized. Yet, it is not clear whether altering the final placement of Purkinje cells affects the assembly of circuits into topographic zones. To gain insight into this problem, we examined zonal connectivity in scrambler mice; spontaneous mutants that have severe Purkinje cell ectopia due to the loss of reelin-disabled1 signaling. We used immunohistochemistry and neural tracing to determine whether displacement of Purkinje cell zones into ectopic positions triggers defects in zonal connectivity within sensory-motor circuits. Despite the abnormal placement of more than 95% of Purkinje cells in scrambler mice, the complementary relationship between molecularly distinct Purkinje cell zones is maintained, and consequently, afferents are targeted into topographic circuits. These data suggest that although loss of disabled1 distorts the Purkinje cell map, its absence does not obstruct the formation of zonal circuits. These findings support the hypothesis that Purkinje cell zones play an essential role in establishing afferent topography.
Introduction
The cerebellum is organized into an array of sagittal zones that control sensory-motor behavior (Seoane et al., 2005; Pijpers et al., 2008; Apps and Hawkes, 2009; Horn et al., 2010; Cerminara and Apps, 2011). Zones are best revealed by the expression patterns of genes and proteins in Purkinje cells (Apps and Hawkes, 2009; White et al., 2012; White and Sillitoe, 2013). For example, the Purkinje cell antigen zebrinII/aldolase C (Brochu et al., 1990; Ahn et al., 1994) is expressed in a striking array of well-defined zones (Sillitoe and Hawkes, 2002). ZebrinII zones are integrated into a broader map where they have an intricate relationship to the expression of several other Purkinje cell proteins. In some cases, zebrinII zones are complementary to the expression pattern of proteins such as phospholipase C β4 (PLCβ4; Armstrong and Hawkes, 2000; Sarna et al., 2006), while in other cases they are co-expressed with proteins such as phospholipase C β3 (PLCβ3; Armstrong and Hawkes, 2000; Sarna et al., 2006). Zones are defined not only by Purkinje cell expression but also by the organization of their axonal inputs. Each zone is innervated by a specific subset of climbing fiber afferents that directly contact Purkinje cells (Apps and Hawkes, 2009; White and Sillitoe, 2013).
Although we are beginning to understand how Purkinje cell zones form, our understanding of how the cerebellar cortical circuit is assembled into zonal connectivity patterns remains unclear. The leading hypothesis is that Purkinje cell patterning may restrict cerebellar interneurons and afferent projections to topographic zones (Sillitoe and Joyner, 2007; Apps and Hawkes, 2009). If Purkinje cell zones do provide the platform for zone assembly, then incoming afferents should be sensitive to the dispersal of Purkinje cells into their final locations. Here, we test the hypothesis that altering Purkinje cell placement will disrupt the assembly of circuits into distinct sagittal zones. We test this hypothesis using mice with defective reelin signaling because this molecular pathway has an established role in controlling the dispersal of embryonic Purkinje cells into a perfect monolayer in mature mice (Howell et al., 1997; Larouche and Hawkes, 2006; Miyata et al., 2010).
The spontaneous mutant scrambler contains an autosomal recessive mutation in the gene that encodes disabled1 (dab1), an adaptor protein that is essential for reelin signaling (Goldowitz et al., 1997; Howell et al., 1997; Sheldon et al., 1997; Rice et al., 1998). In the cerebellum, Purkinje cells selectively express disabled1 (Gallagher et al., 1998; Rice et al., 1998). Loss of disabled1 in mice disrupts cerebellar morphogenesis and causes severe ataxia (Sweet et al., 1996). The scrambler cerebellum is small and the lobules never develop because the size of the granule cell population is severely diminished by ~80% and more than 95% of Purkinje cells fail to complete their migration into a monolayer (Goldowitz et al., 1997). As a result, most Purkinje cells are located in ectopic masses within the central core of the cerebellum (Goldowitz et al., 1997). Although climbing and mossy fiber afferents terminate within ectopic Purkinje cell masses in reeler mutant mice (Blatt and Eisenman, 1988; Vig et al., 2005), it is not clear whether zonally organized afferents are targeted into molecularly distinct Purkinje cell zones. In this study, we exploit the scrambler mouse as a model for disrupting cerebellar patterning to ask whether zonal circuits are established despite the dramatic displacement of Purkinje cells into ectopic zones that are located within the central core of the cerebellum.
Materials and Methods
Mice
All animal studies were carried out under an approved IACUC animal protocol according to the institutional guidelines at Albert Einstein College of Medicine and Baylor College of Medicine. Female and male neuropeptide Y (Npy-Gfp) transgenic mice (Pinto et al., 2004; Nishiyama et al., 2007) and female scrambler mice, which lack the disabled1 gene (Dab1scm/scm; Sweet et al., 1996), were obtained from The Jackson Laboratory, (Bar Harbor, ME) and maintained in our colony on a C57BL/6J background. Here, we refer to the disabled1 mutants as scrambler. The scrambler and Npy-Gfp strains were intercrossed to generate scrambler:Npy-Gfp double transgenic mice in order to genetically mark climbing fibers in scrambler mutants (n = 12 homozygous scrambler:Npy-Gfp mutants). Mice carrying the Npy-Gfp allele were identified by genotyping using a standard polymerase chain reaction with primers designed to detect Gfp (GFP 5′ sense: CTGGTCGAGCTGGACGGCGACG, GFP 3′antisense: CACGAACTCCAGCAGGACCATG and the expected band size is ~ 600 bp). scrambler:Npy-Gfp mice were genotyped for Gfp, phenotyped based on the severe postnatal ataxia observed in reelin pathway mutants (Goldowitz et al., 1997), and analyzed at 1-2 months of age. For anterograde tracing in developing animals (see below for the details of the procedure), whole litters were collected at postnatal day (P) 4/5 from scrambler heterozygote X heterozygote crosses, and each pup in the litter injected with tracer. Homozygous mutant pups were identified upon dissection, based on their small cerebella and well-understood lobule dysmorphology compared to littermate controls (Gallagher et al., 1998). Noon on the day a vaginal plug was detected was considered embryonic day (E) 0.5. The day of birth was designated as postnatal day 0 (P0). Adults were designated as P28 or older.
Immunohistochemistry
Mice were anesthetized with avertin. Once all reflexes were abolished (e.g., lack of blink and corneal reflexes), the blood was flushed through the heart by perfusing with 0.1 M phosphate buffered saline (PBS; pH7.2). The tissue was then fixed by perfusing with 4% paraformaldehyde (4% PFA) diluted in PBS. The brains were then postfixed for 24–48 h in 4% PFA and then cryoprotected in buffered sucrose solutions (15 and 30% diluted in PBS). Serial 40 μm thick coronal sections were cut on a cryostat and collected as free-floating sections in PBS. Immunohistochemistry was carried out as described previously (Reeber et al., 2011). Briefly, tissue sections were washed thoroughly, blocked with 10% normal goat serum (NGS; Sigma, St. Louis MO, USA) for 1 h at room temperature and then incubated in 0.1 M PBS containing 10% NGS, 0.1% Tween-20 and the primary antibodies (see below) for 16–18 h at room temperature. The tissue sections were then washed three times in PBS and incubated in secondary antibodies (see below) for 2 h at room temperature. The tissue was rinsed again and immunoreactivity revealed as described below.
Monoclonal anti-zebrinII (Brochu et al., 1990) was used directly from spent hybridoma culture medium at a concentration of 1:250 (gift from Dr. Richard Hawkes, University of Calgary). Rabbit polyclonal anti-PLCβ4 (1:250) was purchased from Chemicon (Temecula, CA, USA), and revealed an identical pattern of Purkinje cell zones to what has previously been described (Sarna et al., 2006). Mouse monoclonal anti-Vesicular Glutamate Transporter 2 (VGLUT2; 1:500; Cat. # MAB5504) was purchased from Chemicon (Millipore; Billerica, MA) and was used to visualize climbing and mossy fiber terminals (Hisano et al., 2002; Gebre et al., 2012; Reeber and Sillitoe, 2011). Rabbit anti-GFP (1:1000) was purchased from Molecular Probes (Invitrogen; Carlsbad, CA). Chicken anti-GFP (1:2000) was purchased from Abcam (Cambridge, MA).
We tested three different anti-Npy antibodies on mouse sections at various concentrations (1:100 1:250 1:500, 1:1000, and 1:2000). Anti-Npy (Cat. # NAY-8060-V) was purchased from Peptides International (Louisville, KY) and has been shown to label zones in the rat cerebellum (Ueyama et al., 1994). The second Npy antibody tested was a rabbit polyclonal (Cat. # sc-28943) purchased from Santa Cruz (Dallas, TX). The third antibody tested was a rabbit polyclonal (NB600-1094) that was purchased from Novus Biologicals (Littleton, CO).
We visualized immunoreactive complexes either using diaminobenzidine (DAB; 0.5 mg/ml; Sigma, St. Louis, MO, USA) or fluorescence. For the DAB reaction we used horseradish peroxidase (HRP) conjugated goat anti-rabbit secondary antibodies (diluted 1:200 in PBS; DAKO, Carpinteria, CA, USA) to bind the primary antibodies. Staining for fluorescent immunohistochemistry was carried out using Alexa 488- and 555-conjugated immunoglobulins (Molecular Probes Inc., Eugene, OR, USA), both diluted to 1:1500. Tissues sections were coverslipped using either Entellan mounting media (DAB; Electron Microscopy Sciences, Hatfield, PA) or FLUORO-GEL with Tris buffer (Electron Microscopy Sciences, Hatfield, PA). We tested the specificity of the secondary antibodies by processing the tissue sections in the absence of primary antibodies. No signal was detected in such control experiments indicating that the staining we observed was not due to non-specific signals from the Alexa or HRP conjugated antibodies (data not shown).
Anterograde Tracing
Anterograde tracing was performed according to previous protocols (Sillitoe et al., 2010; Reeber et al., 2011). Approximately 250 nl of a 2% solution of WGA (wheat germ agglutinin) conjugated to Alexa Fluor 555 or 488 (Cat. #W32464 and Cat. #W11261, Invitrogen, Carlsbad, CA) was pressure injected into the lower thoracic-upper lumbar spinal cord of P4/P5 (n = 5 for each genotype) or adult mice (n = 5 for each genotype). After a 24 h (pups) or a 48 h (adults) survival period the mice were anesthetized as described above and then perfused with 4% PFA (described above). WGA-Alexa traced neurons are visible immediately upon cellular uptake and thus no additional tissue staining is required for labeling. Therefore, after the perfusion, the WGA-Alexa traced tissue was either cut and mounted for imaging or further processed for immunohistochemistry in order to examine the relationship between afferent projections and Purkinje cell zones. After tracing, the spinal cord was also cut in order to examine the size of the injection spot (local injections that span only one vertebral segment are ideal for pattern analysis) and to ensure that only limited tissue damage was caused by the injection (Reeber et al., 2011).
Statistical Analysis
In wild type mice, WGA-Alexa Fluor 555 accumulates as punctate deposits in mossy fiber terminals, which highlights the structure of the large terminal rosettes (arrowheads in Figures 3A,B; Reeber and Sillitoe, 2011; Reeber et al., 2011). The number of WGA-Alexa Fluor 555 labeled mossy fiber terminals were computed based on an arbitrarily determined boundary that fits within the limits of a single Purkinje cell zone on 40 μm cut sections (all counts were restricted to within 200 μm in the anterior-posterior axis of the cerebellum). At least 3 wild type and 3 scrambler tissue sections were used for the analysis (n = 5 animals/genotype). The number of WGA-Alexa labeled mossy fiber terminals, which were identified as large terminal rosettes, were counted on each tissue section. The sum of the number of mossy fiber terminals for each region was computed and the mean for each region was used to calculate the standard error of the mean, SEM. The p value was acquired using an unpaired t-test (GraphPad Prism) to compare the differences in the number of terminals between wild type and scrambler mutants.
For climbing fiber analysis, we acquired images from 4 to 5 sections through the anterior cerebellum of wild type and scrambler mutants (3 animals each) that were doubled stained for Gfp and PLCβ4, and then measured the distance of a medial-lateral region of cortex that was occupied by zones that were stained using each antibody. The regions that were heavily stained for Gfp revealed the most obvious climbing fiber zones. We computed a region of overlap between the two markers—the mean percentage overlap in a given region was used to calculate the SEM. The p value was acquired using an unpaired t-test (GraphPad Prism) to compare the difference in percent overlap between afferent zones and Purkinje cell zones in wild type versus scrambler mutants. P < 0.05 was considered to be significant.
Microscopy and Data Analysis
Photomicrographs of tissue sections were captured using Leica DFC360 FX (fluorescence) and DFC 490 (DAB reacted tissue sections) cameras mounted on a Leica DM5500 microscope. Images of tissue sections were acquired and analyzed using Leica Application Suite and Leica Application Suite FX software packages supplemented with a deconvolution module. For deconvolution, stacks of 10–20 sections in the z-axis were collected every 0.2–0.5 μm. The z stack images were deconvolved (~10 iterations) and then analyzed as compressed projections or as individual slices. All raw data was imported into Adobe Photoshop CS4 and corrected for brightness and contrast only. Schematics were created in Adobe Illustrator CS4.
Results
Ectopic Purkinje Cells Organize into Molecularly Distinct Zones in Scrambler Mutants
In reeler mice, zebrinII and p-path are expressed within alternating zones of ectopic Purkinje cells (Edwards et al., 1994). Similarly, removal of the reelin receptors, Vldlr and Apoer2, results in a large population of ectopic Purkinje cells that organize into zebrinII and PLCβ4 zones (Larouche et al., 2008). Removal of disabled1, which functions downstream of the Vldlr and Apoer2 receptors, also induces ectopic Purkinje cell clusters that express zebrinII. In addition, loss of diabled1 results in ectopic clusters of HSP25 expressing Purkinje cells (Armstrong et al., 2009). In this study we found that in scrambler mutants, zebrinII and PLCβ4 form a parasagittal zonal pattern, similar to, but distorted compared to the wild type map (Figures 1A–D; Gallagher et al., 1998). The boundaries between zebrinII and PLCβ4 zones are poorly delineated, and in some cases we observed domains with overlapping expression where Purkinje cells expressing zebrinII were extensively intermingled with those expressing PLCβ4 (arrowhead Figure 1D). The relationship between ectopic zones was replicated on either side of the cerebellar midline and the major zones were symmetrically distributed across the medial-lateral extent of the cerebellum (Figures 1C,D). Importantly, the overall zonal pattern in scrambler mutants was consistent between individuals (n = 8; Figures 1C,D). These data support the idea that the fundamental map of molecular zones is present in reelin signaling mutants, and despite the Purkinje cell ectopia the individual relationships between zones are maintained.
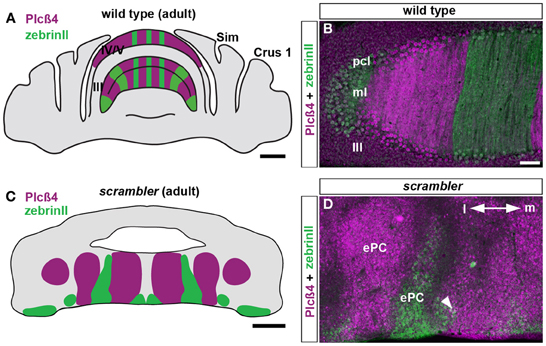
Figure 1. ZebrinII and PLCβ4 expressing Purkinje cells are organized into discrete domains in adult scrambler mutants. (A,B) The expression pattern of PLCβ4 is complementary to the expression pattern of zebrinII as observed on adult coronal tissue sections cut through the wild type mouse cerebellum. (C,D) In adult scrambler mutants, PLCβ4 and zebrinII zones are present in relatively normal configurations (i.e. complementarity between zones is maintained) but are organized into ectopic Purkinje clusters. The boundaries between zebrinII and PLCβ4 are poorly delineated and in some regions, we observed overlapping expression (arrowhead in D). Lobule numbers are indicated by Roman numerals. Abbreviations: pcl, Purkinje cell layer; ml, molecular layer; ePC, ectopic Purkinje cells; m, cerebellar medial; l, lateral. Scale bar in A = 1 mm; B = 100 μm (applies to D); C = 500 μm.
Mossy Fibers are Targeted into Zones of Ectopic Purkinje Cells in Scrambler Mutants
During development, Purkinje cells are thought to establish zonal circuits by guiding the targeting of sensory projections (Apps and Hawkes, 2009). Accordingly, afferents in adults form a precise topographic map that respects the Purkinje cell zone boundaries (Gravel et al., 1987; Gravel and Hawkes, 1990; Akintunde and Eisenman, 1994; Zagrebelsky et al., 1996, 1997; Sawada et al., 2008; Armstrong et al., 2009; Pakan et al., 2010; Reeber and Sillitoe, 2011). We reasoned that if the Purkinje cell zonal map is fully represented in scrambler mutants, albeit in an almost entirely ectopic location, afferent fibers should be precisely targeted in ectopic zones if they depend on Purkinje cell organization for topographic targeting. To test the hypothesis that afferent connectivity respects Purkinje cell zonal boundaries despite the abnormal cytoarchitecture in scrambler mice, we analyzed the neuroanatomy of the spinocerebellar tract, which is a major sensory afferent pathway that projects to the cerebellum from all levels of the spinal cord (Arsenio Nunes and Sotelo, 1985; Sillitoe et al., 2010; Reeber and Sillitoe, 2011; Reeber et al., 2011).
The compartmental organization of spinocerebellar mossy fibers is a classic model for studying mossy fiber topography because its termination pattern clearly illustrates the degree to which cerebellar afferents are organized at the structural (Voogd et al., 1969; Arsenio Nunes and Sotelo, 1985; Yaginuma and Matsushita, 1989; Gravel and Hawkes, 1990; Vogel and Prittie, 1994; Reeber et al., 2011), developmental (Vig et al., 2005; Sillitoe et al., 2010; Reeber et al., 2011) and functional (Perciavalle et al., 1998; Valle et al., 2012) levels. Based upon these well-known characteristics of the pathway, we injected WGA-Alexa conjugated tracers into upper lumber-lower thoracic spinocerebellar neurons to examine the terminal field distribution of afferent fibers within ectopic Purkinje cells in scrambler mutants. Two days after injecting WGA-Alexa tracer into the lower thoracic-upper lumbar region of the spinal cord, mossy fiber axons and terminals were clearly revealed within the cerebellum (Figures 2B,C; n = 5 of each genotype; Reeber and Sillitoe, 2011; Reeber et al., 2011). We confirmed that WGA-Alexa 555 is expressed in mossy fiber terminals by co-labeling with VGLUT2, which is a pre-synaptic marker for mossy fiber terminals (Figure 2A; Hisano et al., 2002). In accordance with previous studies, spinocerebellar mossy fiber terminals in wild type mice project almost exclusively to the vermis and terminate selectively within lobules I–V and VIII/IX (Vogel and Prittie, 1994; Vig et al., 2005; Sillitoe et al., 2010; Reeber et al., 2011). Double labeling with PLCβ4 and zebrinII in WGA-Alexa 555 traced wild type mice revealed that spinocerebellar mossy fiber terminals align mainly with the PLCβ4 subset of Purkinje cells, and only minimally with zebrinII Purkinje cells (Figures 2D,F).
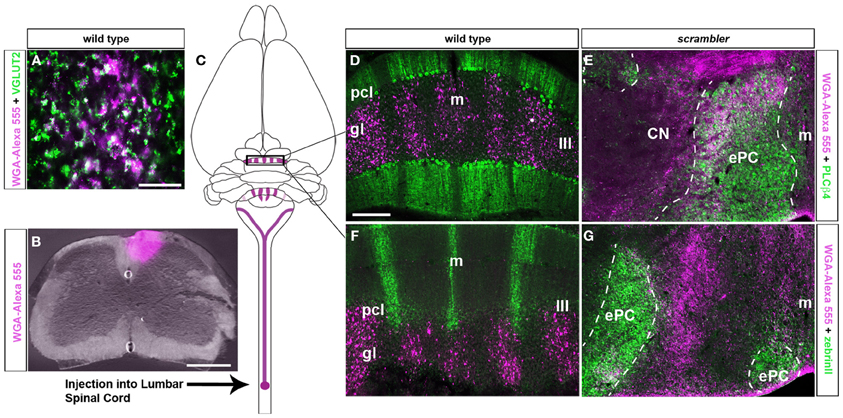
Figure 2. Spinocerebellar mossy fiber targeting into longitudinal zones is conserved in scrambler mutant mice. (A) VGLUT2 is a well-established pre-synaptic marker for mossy fiber terminals. Deconvolution microscopy demonstrates that VGLUT2 and WGA-Alexa 555 co-label a sub-population of spinocerebellar mossy fibers. (B) Image of an injection site after delivering WGA-Alexa 555 into the lower thoracic - upper lumbar region of the adult spinal cord. (C) The schematic illustrates the origin and termination of WGA-Alexa 555 labeled spinocerebellar neurons. (D) In wild type mice, WGA-Alexa 555 labeled mossy fibers terminals align with PLCβ4 immunoreactive Purkinje cells. (E) Similar to wild type mice, in scrambler mutant mice spinocerebellar fibers align with PLCβ4 immunoreactive Purkinje cell clusters. The dotted lines in panels E and G indicate the boundaries of PLCβ4 or zebrinII immunoreactive Purkinje cells. (F) In wild type mice, spinocerebellar fibers do not align with zebrinII immuoreactive Purkinje cells. (G) Ectopic spinocerebellar fibers in scrambler mutant mice do not innervate zebrinII immunoreactive Purkinje cell clusters. Abbreviations: ePC, ectopic Purkinje cells; m, midline; CN, cerebellar nuclei. Scale bar in A = 50 μm; in B = 500 μm; in D = 200 μm (applies to D–G).
Similar to wild type mice (Figures 2D,F), spinocerebellar mossy fiber terminals in scrambler mice heavily innervate zones of PLCβ4 immunoreactive Purkinje cells, although within ectopic positions (Figures 2E,G). Despite targeting appropriate Purkinje cell zones in scrambler mice, mossy fiber zone boundaries were not sharply delineated (Figures 2E,G) and the terminals within the ectopic clusters were morphologically abnormal. As we have previously reported in wild type mice, WGA-Alexa Fluor 555 accumulates as punctate deposits in mossy fiber terminals, which highlights the structure of the large terminal rosettes (arrowheads in Figures 3A,B; Reeber and Sillitoe, 2011; Reeber et al., 2011). In contrast, in adult scrambler mice only ~20% of the traced mossy fibers had the complex “grape-like” glomeruli structure that is typical of cerebellar mossy fibers (Figures 3C,D). Profile counts of large mossy fibers terminals revealed an average of 45 (±2.1) labeled rosettes within a specified region of cerebellar cortex in wild type mice, whereas scrambler mutants contained 9 (±1.0) labeled terminal rosettes (Figures 3C,D; p < 0.0001) within an equivalent sized region. We conclude that despite the lack of clear zonal boundaries and properly structured terminals, the topography of spinocerebellar afferent zones is not affected by the displacement of Purkinje cells that occurs after loss of disabled1 function in scrambler mutant mice.
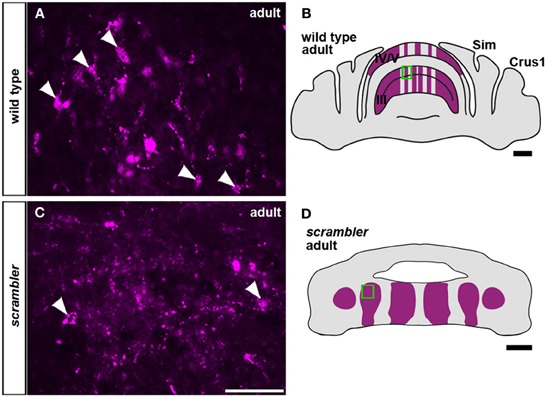
Figure 3. Mossy fiber terminals within ectopic Purkinje cell clusters fail to form large grape-like glomeruli (rosettes). (A,C) Anterogradely transported WGA-Alexa 555 accumulates as punctate deposits in mossy fiber axons and terminals. (A,B) High magnification image of WGA-Alexa 555 traced mossy fiber glomeruli in the granule cell layer of wild type adult mice (boxed area in B). The arrowheads are highlighting typical large terminal rosettes. (C,D) In scrambler adult mice, mossy fibers fail to differentiate into the complex “grape-like” glomerular structure. Arrowheads are pointing to relatively normal mossy fiber terminals within the ectopic cluster from the boxed region in (D). Scale bar in B = 1 mm; C = 50 μm (applies to A,C); D = 500 μm.
Early Postnatal Targeting of Mossy Fibers is not Altered in Scrambler Mutants
Because mossy fiber zonal boundaries are poorly resolved in adult scrambler mutants, we wondered whether an altered developmental timetable could contribute to the formation of a distorted mossy fiber afferent map. To determine whether the initial patterning of mossy fibers into zones is altered in scrambler mutant mice, we used WGA-Alexa 555 to examine spinocerebellar tract development during the first week after birth. We previously determined that spinocerebellar mossy fibers resolve into a zonal pattern by ~P5 in mice (Sillitoe et al., 2010). Therefore, we injected mice with tracer at P4/P5 and then one day later we compared mossy fiber topography in wild type and scrambler mice (Figure 4; n = 5 of each genotype; Reeber and Sillitoe, 2011; Reeber et al., 2011). Consistent with our previous report, by P5 we observed rudimentary zones of spinocerebellar mossy fiber terminals within lobules I-V and VIII/IX in wild type mice. We also found that similar to the adult, P5 mossy fiber terminals align with PLCβ4 immunoreactive Purkinje cells (Figures 4A,B). Spinocerebellar mossy fiber terminals in scrambler mice also terminated in zones at P5. Strikingly, afferent terminals in scrambler were mainly targeted into ectopic PLCβ4 Purkinje cell clusters (Figures 4C,D). Note that in both wild type and scrambler mice scattered WGA-Alexa traced terminals can be seen in the PLCβ4 negative domains because afferent pruning is not complete at this stage of development. Unlike adult scrambler mice that have abnormal mossy fiber terminal structure, we observed that mossy fiber terminals in P4/5 scrambler mice have a comparable immature structure to wild type pups of the same age (Figures 5A–D). These data suggest that in scrambler, mossy fiber afferents resolve into zones at the right time, and they target the correct subsets of Purkinje cells. In addition, although mossy fibers in scrambler mutants may not differentiate properly, the gross anatomical features typical of granular layer presynaptic terminals do form. In summary, the spatial and temporal properties of spinocerebellar afferent zonation are not dependent on reelin-disabled1 signaling, although the structural features of clear-cut sharp zones with large terminal rosettes are altered when disabled1 is deleted. Our data support the hypothesis that Purkinje cell patterning may be the major determinant of spinocerebellar afferent zone formation (Sotelo, 2004).
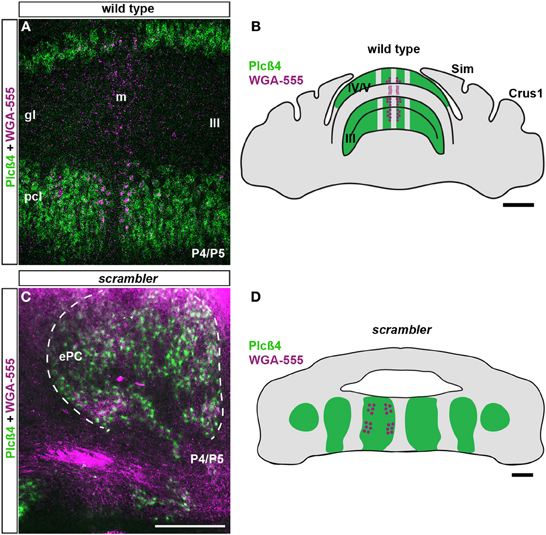
Figure 4. Postnatal spinocerebellar afferents are topographically targeted in scrambler mutants. (A). In P4/5 wild type mice, WGA-Alexa 555 labeled mossy fiber terminals align with PLCβ4 immunoreactive Purkinje cells. (C) Similar to wild type mice, in P4/5 scrambler mutant mice spinocerebellar fibers align with PLCβ4 immunoreactive Purkinje cell clusters. The dotted line in panel C indicates the boundary of an ectopic PLCβ4 immunoreactive Purkinje cell cluster. (B,D) The schematic illustrates the termination of WGA-Alexa 555 labeled spinocerebellar mossy fibers in wild type (B) and scrambler (D) mice. Abbreviations: ePC, ectopic Purkinje cells; pcl, Purkinje cell layer; gl, granule cell layer. Scale bar in B = 750 μm; C = 200 μm (applies to A, C); D = 200 μm.
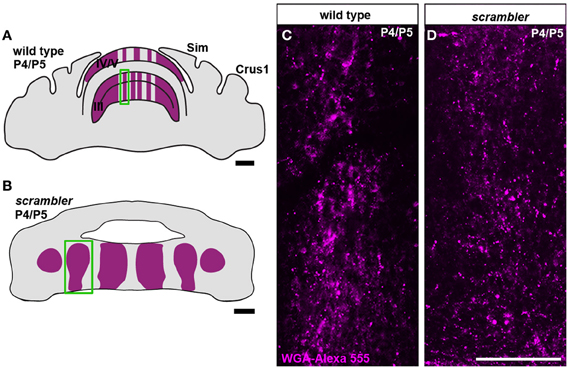
Figure 5. Mossy fiber terminal structure is established in postnatal scrambler mice. (A,C) Anterogradely transported WGA- Alexa 555 accumulates as punctate deposits in spinocerebellar mossy fiber axons and terminals in wild type P4/5 mice. (B,D) Punctate deposits were also observed in the terminals of P4/5 scrambler mutant mice. The images in panels (C,D) were taken from the boxed regions in panels (A,B), respectively. Scale bar in A = 750 μm; B = 200 μm; D = 100 μm (applies to C,D).
Climbing Fiber Zones are Revealed Using Npy-Gfp Transgene Expression
Climbing fiber projections arise from cells in the inferior olivary complex of the brainstem and monoinnervate Purkinje cells. Although neuroanatomic approaches have been indispensible for studying olivo-cerebellar circuit architecture (Fujita and Sugihara, 2013), more precise methods are required for routinely examining climbing fiber circuit topography in normal and mutant mice. The surgical procedures for injecting tracers into the olive require skilled surgeries (Blatt and Eisenman, 1988) and routinely injecting the same sub-nuclei to reveal olivo-cerebellar topography is challenging (Sotelo et al., 1984). These challenges in studying olivo-cerebellar connectivity arise mainly because the ventral location of the inferior olive makes it hard to access during surgery. We thought that these problems could be overcome if reporter gene expression in an Npy-Gfp transgenic line (Nishiyama et al., 2007) labeled only a subset of climbing fibers (Figures 6A,C,C′). Npy protein is a known marker for climbing fibers zones in the rat cerebellum (Ueyama et al., 1994; Morara et al., 1997). Because Npy antibodies are unreliable in mouse, and the three we tested did not reveal positive staining (data not shown; see methods), we analyzed the Npy-Gfp transgenic line to test whether this transgene would label zones of climbing fibers (n = 6). We first confirmed the identity of genetically labeled afferents as climbing fibers by double labeling transgenic tissue sections with GFP and VGLUT2, an established marker for climbing fiber terminals (Figure 6A; Hisano et al., 2002). Climbing fiber axons were observed in the white matter and could be traced through the granular layer, and into the molecular layer where they terminated upon the proximal two thirds of Purkinje cell dendrites (Figure 6A).
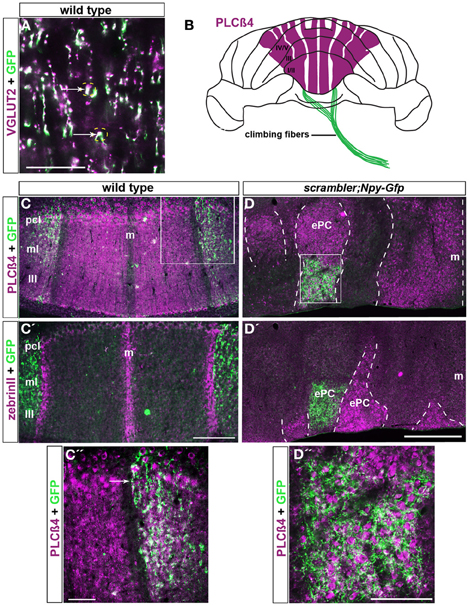
Figure 6. Npy-Gfp labeled climbing fiber topography is maintained in scrambler mutants. (A). VGLUT2 is a molecular marker known to label climbing fiber terminals. VGLUT2 and Npy-Gfp co-label a sub-population of climbing fibers (arrows). (B) Whole mount schematic illustrating the normal pattern of Npy-Gfp labeled climbing fibers innervating PLCβ4 immunoreactive Purkinje cell zones. (C,C') Npy-Gfp is expressed in two broad parasagittal zones that span ~500 μm on either side of the midline in adult wild type mice. (C) Npy-Gfp labeled climbing fiber zones overlap with PLCβ4 immunoreactive Purkinje cell zones. (C') Npy-Gfp labeled climbing fiber zones respect Purkinje cell stripe boundaries and do not invade the zebrinII immunoreactive Purkinje cell zones. (D,D′) Npy-Gfp is expressed in climbing fibers in scrambler:Npy-Gfp mutant mice. (D) Similar to wild type mice, in scrambler mice climbing fibers selectively innervate PLCβ4 immunoreactive Purkinje cell clusters. The dotted lines in panels (D,D′) indicate the boundaries of PLCβ4 and zebrinII immunoreactive Purkinje cells. (D') Npy-Gfp expressing climbing fibers do not target zebrinII labeled Purkinje cells in scrambler mutant mice. (C″,D″) High magnification images of boxed regions in (C,D) show that Npy-Gfp is expressed in climbing fiber zones that terminate upon PLCβ4 immunopositive Purkinje cells in both wild type and scrambler mutant mice. Scale bar in A = 60 μm; in C' = 250 μm (applies to C–C); in C″= 50 μm; in D = 500 μm (applies to D–D′); in D″ = 100 μm.
Not all climbing fibers expressed the Npy-Gfp transgene. Labeled climbing fibers were most obvious in the anterior lobules of the cerebellum. In lobules I–V, the transgene is expressed in at least two zones of climbing fibers that are located on either side of the midline (Figures 6B–C″). The climbing fibers in both zones terminate selectively upon PLCβ4 immunopositive Purkinje cells and do not invade the adjacent zebrinII Purkinje cell zones (arrows in Figures 6C–C″). Thus, the afferent termination pattern of Npy-Gfp labeled climbing fibers follows the topographical relationship known to exist between climbing fibers and Purkinje cells (Apps and Hawkes, 2009). We propose that the Npy-Gfp transgenic mouse is a useful new tool for genetically labeling climbing fiber zones in vivo.
Climbing Fibers Terminate within Zones of Ectopic Purkinje Cells in Scrambler Mutants
To further test the hypothesis that afferent fiber zones are correctly matched to Purkinje cell subtypes despite the abnormal cytoarchitecture in scrambler mice, we crossed the Npy-Gfp transgene onto the background of the scrambler allele to examine climbing fiber-Purkinje cell topography. We examined scrambler: Npy-Gfp mice, which are homozygous for the scrambler allele and hemizygous for the Npy-Gfp allele, for patterning defects (n =12). Despite the severe ectopia of Purkinje cells in scrambler, we found that Npy-Gfp labeled climbing fibers were nevertheless topographically aligned with specific Purkinje cell clusters (Figures 6D–D″). Double staining showed that in scrambler mutants, similar to wild type mice, the Npy-Gfp expressing climbing fibers selectively innervated PLCβ4 immunoreactive Purkinje cell clusters (Figures 6D,D″). Moreover, quantification of the overlap between a heavily expressing Gfp region located within a PLCβ4 zone revealed a 49% (±8%) overlap in an anterior zone of wild type mice and a 37% (±4%) overlap in a scrambler mutant zone (the difference between the genotypes was not significant at p = 0.2079). In adjacent regions, we observed close to 100% overlap between afferent and Purkinje cell zones (for both wild type and mutant; Figures 6C′,D″). Thus, although the percent overlap may be different depending on the region of cerebellum analyzed, the relationship is consistent between animals and equivalent in wild type versus mutants. Overall, these results reveal that the loss of reelin-disabled1 signaling, and the ectopic placement of Purkinje cells do not interfere with climbing fiber recognition of molecularly distinct Purkinje cells.
Discussion
The cerebellum is organized into a complex array of topographic sagittal zones; zones are best defined by the molecular expression patterns in Purkinje cells (Apps and Hawkes, 2009). In this investigation, we sought to determine whether altering Purkinje cell patterning by genetically inducing severe ectopia affects the targeting of afferent fibers into specific zones. We used transgenic mice that express Gfp driven by a neuropeptide Y gene promoter and a novel WGA-Alexa tracing approach to label climbing fibers and mossy fibers in the developing and adult cerebellum. Using the spontaneous mutant scrambler as a model for Purkinje cell mis-patterning, we demonstrate that despite the severe ectopia of Purkinje cell zones, the topography of afferent zones is established and afferent-target specificity is maintained.
Complex Purkinje Cell Zonation is Established in Reelin Signaling Mutants
Cerebellar zones are clearly delineated by the patterned expression of several genes and proteins (Apps and Hawkes, 2009; White and Sillitoe, 2013). For example, zebrinII (adolase C; Ahn et al., 1994; Hawkes and Herrup, 1995) and PLCβ4 (Armstrong and Hawkes, 2000; Sarna et al., 2006) are expressed in complementary subsets of Purkinje cells. Mutations in the components of the reelin pathway cause severe morphogenetic abnormalities in mice, but the Purkinje cell zones that express 5'-nucleotidase, zebrinII, HSP25, and p-path are clearly represented within ectopic clusters (Caviness and Rakic, 1978; Goffinet, 1984; Eisenman, 1988; Edwards et al., 1994; Gallagher et al., 1998; Larouche et al., 2008; Armstrong et al., 2009). Thus, despite the severe Purkinje cell ectopia in reeler, disabled1, Apoer2 and Vldlr mutants, Purkinje cell zonation is still established (Blatt and Eisenman, 1988; Vig et al., 2005; Larouche et al., 2008). Combined with our data, these studies suggest that the major molecular divisions of the cerebellar zonal map are represented in reelin signaling mutants, although within ectopic cellular clusters.
Cerebellar Afferents are Topographically Organized in Scrambler Mutant Mice
The organization of parasagittal Purkinje cell zones is mirrored by the topography of mossy fiber (Gravel and Hawkes, 1990; Akintunde and Eisenman, 1994; Armstrong et al., 2009; Pakan et al., 2010) and climbing fiber (Gravel et al., 1987; Zagrebelsky et al., 1996, 1997; Sawada et al., 2008) terminal fields. The timing and precision of this relationship led to the hypothesis that Purkinje cell zones, which emerge in the embryo, determine the patterned organization of afferent projections during late embryogenesis and early postnatal development (Sillitoe and Joyner, 2007; Apps and Hawkes, 2009). In this study, we tested the role of Purkinje cell placement during zonal circuit formation by examining scrambler mice (Goldowitz et al., 1997; Howell et al., 1997; Sheldon et al., 1997; Rice et al., 1998). Despite the severe ectopia of Purkinje cells in scrambler mice, Npy-Gfp labeled climbing fibers and WGA-Alexa traced spinocerebellar mossy fibers terminate within severely ectopic positions although still in alignment with their normal targets, the PLCβ4 immunoreactive Purkinje cell clusters (Figures 2, 6). Accordingly, somatostatin immunoreactive mossy fibers correctly innervate HSP25-immunoreactive zones in scrambler and weaver mutant mice, which is another mouse strain with ectopic Purkinje cells (Armstrong et al., 2009). These data are consistent with observations from reeler mutants, in which climbing fiber, spinocerebellar, and vestibulocerebellar afferents were shown to terminate into relatively normal anterior-posterior locations (Vig et al., 2005).
We also observed that within the ectopic Purkinje cell clusters, mossy fiber terminals failed to differentiate into their typical complex “grape-like” structure (Figure 3). Surprisingly, despite this lack of apparent structural complexity at the synapse, in vivo recordings in reeler mutants demonstrated that afferents do make connections in the ectopic clusters and afferent information is transferred to their ectopic Purkinje cells with some level of fidelity (Mariani et al., 1977). Taken together, these data indicate that the major functional circuits of the cerebellum are established in reelin signaling mutants, and that Purkinje cell topography may influence several stages of cerebellar circuit formation.
Afferent Targeting is not Altered in Early Postnatal Scrambler Mutant Mice
Spinocerebellar mossy fibers enter the cerebellar anlage at approximately E13/14 in mouse (Grishkat and Eisenman, 1995) and at ~E12 in rat (Ashwell and Zhang, 1992, 1998). Mossy fiber topography is set up before most granule cells differentiate and migrate into the developing internal granular layer (Arsenio Nunes and Sotelo, 1985); during this time, mossy fibers directly contact clusters of immature Purkinje cells (Mason and Gregory, 1984; Grishkat and Eisenman, 1995; Sillitoe et al., 2010). It is postulated that mossy fibers disperse along with Purkinje cells as clusters transform into mature zones. During postnatal development, granule cells migrate past the Purkinje cells to form the internal granular layer, and then mossy fiber terminals translocate from Purkinje cells to granule cells (Sillitoe and Hawkes, 2013). This model is consistent with our observation that in scrambler, which lack ~80% of their granule cells, and in other agranular mutants, spinocerebellar mossy fiber topography is established despite the absence of a normal mossy fiber-granule cell-Purkinje cell circuit (Arsenio Nunes and Sotelo, 1985; Arsenio Nunes et al., 1988; Eisenman and Arlinghaus, 1991). We found that at P5, in scrambler mutants, mossy fibers are targeted into their “correct” circuits, although within ectopic Purkinje cell zones. Thus, as long as Purkinje cells can resolve into different chemical phenotypes, zonal circuit connectivity proceeds with limited disruption.
Author Contributions
Stacey L. Reeber, Courtney A. Loeschel, and Roy V. Sillitoe designed the experiments. Stacey L. Reeber, Amanda Franklin, and Roy V. Sillitoe performed the experiments. Stacey L. Reeber, Courtney A. Loeschel and Roy V. Sillitoe wrote the paper.
Conflict of Interest Statement
The authors declare that the research was conducted in the absence of any commercial or financial relationships that could be construed as a potential conflict of interest.
Acknowledgments
We thank Samrawit A. Gebre for technical assistance. This work was supported by funds from Albert Einstein College of Medicine of Yeshiva University (New York, NY) and Baylor College of Medicine and Texas Children's Hospital (Houston, TX). Roy V. Sillitoe is supported by the Caroline Wiess Law Fund for Research in Molecular Medicine, a BCM IDDRC Project Development Award, and by BCM IDDRC Grant Number 5P30HD024064 from the Eunice Kennedy Shriver National Institute Of Child Health & Human Development and by Grant Number C06RR029965 from the National Center For Research Resources. The content is solely the responsibility of the authors and does not necessarily represent the official views of the National Center For Research Resources or the National Institutes of Health.
References
Ahn, A. H., Dziennis, S., Hawkes, R., and Herrup, K. (1994). The cloning of zebrin II reveals its identity with aldolase C. Development 120, 2081–2090.
Akintunde, A., and Eisenman, L. M. (1994). External cuneocerebellar projection and Purkinje cell zebrin II bands: a direct comparison of parasagittal banding in the mouse cerebellum. J. Chem. Neuroanat. 7, 75–86. doi: 10.1016/0891-0618(94)90009-4
Apps, R., and Hawkes, R. (2009). Cerebellar cortical organization: a one-map hypothesis. Nat. Rev. Neurosci. 10, 670–681. doi: 10.1038/nrn2698
Armstrong, C. L., Chung, S. H., Armstrong, J. N., Hochgeschwender, U., Jeong, Y. G., and Hawkes, R. (2009). A novel somatostatin-immunoreactive mossy fiber pathway associated with HSP25-immunoreactive purkinje cell stripes in the mouse cerebellum. J. Comp. Neurol. 517, 524–538. doi: 10.1002/cne.22167
Armstrong, C. L., and Hawkes, R. (2000). Pattern formation in the cerebellar cortex. Biochem. Cell Biol. 78, 551–562. doi: 10.1139/o00-071
Arsenio Nunes, M. L., and Sotelo, C. (1985). Development of the spinocerebellar system in the postnatal rat. J. Comp. Neurol. 237, 291–306. doi: 10.1002/cne.902370302
Arsenio Nunes, M. L., Sotelo, C., and Wehrle, R. (1988). Organization of spinocerebellar projection map in three types of agranular cerebellum: purkinje cells vs. granule cells as organizer element. J. Comp. Neurol. 273, 120–136. doi: 10.1002/cne.902730110
Ashwell, K. W., and Zhang, L. L. (1992). Ontogeny of afferents to the fetal rat cerebellum. Acta Anat. (Basel) 145, 17–23. doi: 10.1159/000147336
Ashwell, K. W., and Zhang, L. I. (1998). Prenatal development of the vestibular ganglion and vestibulocerebellar fibres in the rat. Anat. Embryol. 198, 149–161. doi: 10.1007/s004290050173
Blatt, G. J., and Eisenman, L. M. (1988). Topographic and zonal organization of the olivocerebellar projection in the reeler mutant mouse. J. Comp. Neurol. 267, 603–615. doi: 10.1002/cne.902670412
Brochu, G., Maler, L., and Hawkes, R. (1990). Zebrin II: a polypeptide antigen expressed selectively by Purkinje cells reveals compartments in rat and fish cerebellum. The Journal of comparative Neurology 291, 538–552. doi: 10.1002/cne.902910405
Caviness, V. S. Jr., and Rakic, P. (1978). Mechanisms of cortical development: a view from mutations in mice. Annu. Rev. Neurosci. 1, 297–326. doi: 10.1146/annurev.ne.01.030178.001501
Cerminara, N. L., and Apps, R. (2011). Behavioural significance of cerebellar modules. Cerebellum 10, 484–494. doi: 10.1007/s12311-010-0209-2
Edwards, M. A., Leclerc, N., Crandall, J. E., and Yamamoto, M. (1994). Purkinje cell compartments in the reeler mutant mouse as revealed by Zebrin II and 90-acetylated glycolipid antigen expression. Anat. Embryol. 190, 417–428. doi: 10.1007/BF00235488
Eisenman, L. M. (1988). Histochemical localization of 5'-nucleotidase in the reeler mutant mouse. Neurosci. Lett. 94, 70–75. doi: 10.1016/0304-3940(88)90272-8
Eisenman, L. M., and Arlinghaus, L. E. (1991). Spinocerebellar projection in the meander tail mutant mouse: organization in the granular posterior lobe and the agranular anterior lobe. Brain Res. 558, 149–152. doi: 10.1016/0006-8993(91)90733-C
Fujita, H., and Sugihara, I. (2013). Branching patterns of olivocerebellar axons in relation to the compartmental organization of the cerebellum. Front. Neural Circuits 7:3. doi: 10.3389/fncir.2013.00003
Gallagher, E., Howell, B. W., Soriano, P., Cooper, J. A., and Hawkes, R. (1998). Cerebellar abnormalities in the disabled (mdab1-1) mouse. J. Comp. Neurol. 402, 238–251.
Gebre, S. A., Reeber, S. L., and Sillitoe, R. V. (2012). Parasagittal compartmentation of cerebellar mossy fibers as revealed by the patterned expression of vesicular glutamate transporters VGLUT1 and VGLUT2. Brain Struct. Funct. 217, 165–180. doi: 10.1007/s00429-011-0339-4
Goffinet, A. M. (1984). Events governing organization of postmigratory neurons: studies on brain development in normal and reeler mice. Brain Res. 319, 261–296.
Goldowitz, D., Cushing, R. C., Laywell, E., D'Arcangelo, G., Sheldon, M., Sweet, H. O., et al. (1997). Cerebellar disorganization characteristic of reeler in scrambler mutant mice despite presence of reelin. J. Neurosci. 17, 8767–8777.
Gravel, C., and Hawkes, R. (1990). Parasagittal organization of the rat cerebellar cortex: direct comparison of Purkinje cell compartments and the organization of the spinocerebellar projection. J. Comp. Neurol. 291, 79–102. doi: 10.1002/cne.902910107
Gravel, C., Leclerc, N., Rafrafi, J., Sasseville, R., Thivierge, L., and Hawkes, R. (1987). Monoclonal antibodies reveal the global organization of the cerebellar cortex. J. Neurosci. Methods 21, 145–157. doi: 10.1016/0165-0270(87)90112-9
Grishkat, H. L., and Eisenman, L. M. (1995). Development of the spinocerebellar projection in the prenatal mouse. J. Comp. Neurol. 363, 93–108. doi: 10.1002/cne.903630109
Hawkes, R., and Herrup, K. (1995). Aldolase C/zebrin II and the regionalization of the cerebellum. J. Mol. Neurosci. 6, 147–158. doi: 10.1007/BF02736761
Hisano, S., Sawada, K., Kawano, M., Kanemoto, M., Xiong, G., Mogi, K., et al. (2002). Expression of inorganic phosphate/vesicular glutamate transporters (BNPI/VGLUT1 and DNPI/VGLUT2) in the cerebellum and precerebellar nuclei of the rat. Brain Res. Mol. Brain Res. 107, 23–31. doi: 10.1016/S0169-328X(02)00442-4
Horn, K. M., Pong, M., and Gibson, A. R. (2010). Functional relations of cerebellar modules of the cat. J. Neurosci. 30, 9411–9423.
Howell, B. W., Hawkes, R., Soriano, P., and Cooper, J. A. (1997). Neuronal position in the developing brain is regulated by mouse disabled-1. Nature 389, 733–737. doi: 10.1038/39607
Larouche, M., Beffert, U., Herz, J., and Hawkes, R. (2008). The Reelin receptors Apoer2 and Vldlr coordinate the patterning of Purkinje cell topography in the developing mouse cerebellum. PLoS ONE 3:e1653. doi: 10.1371/journal.pone.0001653
Larouche, M., and Hawkes, R. (2006). From clusters to stripes: the developmental origins of adult cerebellar compartmentation. Cerebellum 5, 77–88. doi: 10.1080/14734220600804668
Mariani, J., Crepel, F., Mikoshiba, K., Changeux, J. P., and Sotelo, C. (1977). Anatomical, physiological and biochemical studies of the cerebellum from Reeler mutant mouse. Philos. Trans. R. Soc. Lond. B Biol. Sci. 281, 1–28. doi: 10.1098/rstb.1977.0121
Mason, C. A., and Gregory, E. (1984). Postnatal maturation of cerebellar mossy and climbing fibers: transient expression of dual features on single axons. J. Neurosci. 4, 1715–1735.
Miyata, T., Ono, Y., Okamoto, M., Masaoka, M., Sakakibara, A., Kawaguchi, A., et al. (2010). Migration, early axonogenesis, and Reelin-dependent layer-forming behavior of early/posterior-born Purkinje cells in the developing mouse lateral cerebellum. Neural Dev. 5:23. doi: 10.1186/1749-8104-5-23
Morara, S., Marcotti, W., Provini, L., and Rosina, A. (1997). Neuropeptide Y (NPY) expression is up-regulated in the rat inferior olive during development. Neuroreport 8, 3743–3747. doi: 10.1097/00001756-199712010-00017
Nishiyama, H., Fukaya, M., Watanabe, M., and Linden, D. J. (2007). Axonal motility and its modulation by activity are branch-type specific in the intact adult cerebellum. Neuron 56, 472–487. doi: 10.1016/j.neuron.2007.09.010
Pakan, J. M., Graham, D. J., and Wylie, D. R. (2010). Organization of visual mossy fiber projections and zebrin expression in the pigeon vestibulocerebellum. J. Comp. Neurol. 518, 175–198. doi: 10.1002/cne.22192
Perciavalle, V., Bosco, G., and Poppele, R. E. (1998). Spatial organization of proprioception in the cat spinocerebellum. Purkinje cell responses to passive foot rotation. Eur. J. Neurosci. 10, 1975–1985. doi: 10.1046/j.1460-9568.1998.00212.x
Pijpers, A., Winkelman, B. H., Bronsing, R., and Ruigrok, T. J. (2008). Selective impairment of the cerebellar C1 module involved in rat hind limb control reduces step-dependent modulation of cutaneous reflexes. J. Neurosci. 28, 2179–2189. doi: 10.1523/JNEUROSCI.4668-07.2008
Pinto, S., Roseberry, A. G., Liu, H., Diano, S., Shanabrough, M., Cai, X., et al. (2004). Rapid rewiring of arcuate nucleus feeding circuits by leptin. Science 304, 110–115. doi: 10.1126/science.1089459
Reeber, S. L., Gebre, S. A., and Sillitoe, R. V. (2011). Fluorescence mapping of afferent topography in three dimensions. Brain Struct. Funct. doi: 10.1007/s00429-011-0304-2
Reeber, S. L., and Sillitoe, R. V. (2011). Patterned expression of a cocaine- and amphetamine-regulated transcript (CART) peptide reveals complex circuit topography in the rodent cerebellar cortex. J. Comp. Neurol. 519, 1781–1796. doi: 10.1002/cne.22601
Rice, D. S., Sheldon, M., D'Arcangelo, G., Nakajima, K., Goldowitz, D., and Curran, T. (1998). Disabled-1 acts downstream of Reelin in a signaling pathway that controls laminar organization in the mammalian brain. Development 125, 3719–3729.
Sarna, J. R., Marzban, H., Watanabe, M., and Hawkes, R. (2006). Complementary stripes of phospholipase Cbeta3 and Cbeta4 expression by Purkinje cell subsets in the mouse cerebellum. J. Comp. Neurol. 496, 303–313. doi: 10.1002/cne.20912
Sawada, K., Fukui, Y., and Hawkes, R. (2008). Spatial distribution of corticotropin-releasing factor immunopositive climbing fibers in the mouse cerebellum: analysis by whole mount immunohistochemistry. Brain Res. 1222, 106–117. doi: 10.1016/j.brainres.2008.05.029
Seoane, A., Apps, R., Balbuena, E., Herrero, L., and Llorens, J. (2005). Differential effects of trans-crotononitrile and 3-acetylpyridine on inferior olive integrity and behavioural performance in the rat. Eur. J. Neurosci. 22, 880–894. doi: 10.1111/j.1460-9568.2005.04230.x
Sheldon, M., Rice, D. S., D'Arcangelo, G., Yoneshima, H., Nakajima, K., Mikoshiba, K., et al. (1997). Scrambler and yotari disrupt the disabled gene and produce a reeler-like phenotype in mice. Nature 389, 730–733. doi: 10.1038/39601
Sillitoe, R. V., and Hawkes, R. (2002). Whole-mount immunohistochemistry: a high-throughput screen for patterning defects in the mouse cerebellum. J. Histochem. Cytochem. 50, 235–244. doi: 10.1177/002215540205000211
Sillitoe, R. V., and Hawkes, R. (2013). “Zones and stripes: development of cerebellar topography,” in Handbook of the Cerebellum and Cerebellar Disorders, eds M. Manto, D. L. Gruol, J. D. Schmahmann, N. Koibuchi, and F. Rossi (New York, NY: Springer), 43–59.
Sillitoe, R. V., and Joyner, A. L. (2007). Morphology, molecular codes, and circuitry produce the three-dimensional complexity of the cerebellum. Annu. Rev. Cell Dev. Biol. 23, 549–577. doi: 10.1146/annurev.cellbio.23.090506.123237
Sillitoe, R. V., Vogel, M. W., and Joyner, A. L. (2010). Engrailed homeobox genes regulate establishment of the cerebellar afferent circuit map. J. Neurosci. 30, 10015–10024. doi: 10.1523/JNEUROSCI.0653-10.2010
Sotelo, C. (2004). Cellular and genetic regulation of the development of the cerebellar system. Prog. Neurobiol. 72, 295–339. doi: 10.1016/j.pneurobio.2004.03.004
Sotelo, C., Bourrat, F., and Triller, A. (1984). Postnatal development of the inferior olivary complex in the rat. II. Topographic organization of the immature olivocerebellar projection. J. Comp. Neurol. 222, 177–199. doi: 10.1002/cne.902220204
Sweet, H. O., Bronson, R. T., Johnson, K. R., Cook, S. A., and Davisson, M. T. (1996). Scrambler, a new neurological mutation of the mouse with abnormalities of neuronal migration. Mamm. Genome 7, 798–802. doi: 10.1007/s003359900240
Ueyama, T., Houtani, T., Nakagawa, H., Baba, K., Ikeda, M., Yamashita, T., et al. (1994). A subpopulation of olivocerebellar projection neurons express neuropeptide Y. Brain Res. 634, 353–357. doi: 10.1016/0006-8993(94)91943-7
Valle, M. S., Eian, J., Bosco, G., and Poppele, R. E. (2012). The organization of cortical activity in the anterior lobe of the cat cerebellum during hindlimb stepping. Experimental brain research. Experimentelle Hirnforschung. Exp. Cereb. 216, 349–365. doi: 10.1007/s00221-011-2938-y
Vig, J., Goldowitz, D., Steindler, D. A., and Eisenman, L. M. (2005). Compartmentation of the reeler cerebellum: segregation and overlap of spinocerebellar and secondary vestibulocerebellar fibers and their target cells. Neuroscience 130, 735–744. doi: 10.1016/j.neuroscience.2004.09.051
Vogel, M. W., and Prittie, J. (1994). Topographic spinocerebellar mossy fiber projections are maintained in the lurcher mutant. J. Comp. Neurol. 343, 341–351. doi: 10.1002/cne.903430212
Voogd, J., Broere, G., and van Rossum, J. (1969). The medio-lateral distribution of the spinocerebellar projection in the anterior lobe and the simple lobule in the cat and a comparison with some other afferent fibre systems. Psychiatr. Neurol. Neurochir. 72, 137–151.
White, J. J., Reeber, S. L., Hawkes, R., and Sillitoe, R. V. (2012). Wholemount immunohistochemistry for revealing complex brain topography. J. Vis. Exp. 62:e4042. doi: 10.3791/4042
White, J. J., and Sillitoe, R. V. (2013). Development of the cerebellum: from gene expression patterns to circuit maps. Wiley Interdiscip Rev. Dev. Biol. 2, 149–164. doi: 10.1002/wdev.65
Yaginuma, H., and Matsushita, M. (1989). Spinocerebellar projections from the upper lumbar segments in the cat, as studied by anterograde transport of wheat germ agglutinin-horseradish peroxidase. J. Comp. Neurol. 281, 298–319. doi: 10.1002/cne.902810211
Zagrebelsky, M., Rossi, F., Hawkes, R., and Strata, P. (1996). Topographically organized climbing fibre sprouting in the adult rat cerebellum. Eur. J. Neurosci. 8, 1051–1054. doi: 10.1111/j.1460-9568.1996.tb01591.x
Keywords: positional map, circuitry, topography, disabled1, connectivity, cerebellum
Citation: Reeber SL, Loeschel CA, Franklin A and Sillitoe RV (2013) Establishment of topographic circuit zones in the cerebellum of scrambler mutant mice. Front. Neural Circuits 7:122. doi: 10.3389/fncir.2013.00122
Received: 22 March 2013; Accepted: 01 July 2013;
Published online: 22 July 2013.
Edited by:
Charles F. Stevens, The Salk Institute for Biological Studies, USAReviewed by:
Henrik Jörntell, Lund University, SwedenBassem Hassan, Vlaams Instituut voor Biotechnologie, Belgium
Copyright © 2013 Reeber, Loeschel, Franklin and Sillitoe. This is an open-access article distributed under the terms of the Creative Commons Attribution License, which permits use, distribution and reproduction in other forums, provided the original authors and source are credited and subject to any copyright notices concerning any third-party graphics etc.
*Correspondence: Roy V. Sillitoe, Department of Pathology and Immunology, Department of Neuroscience, Baylor College of Medicine, Jan and Dan Duncan Neurological Research Institute of Texas Children's Hospital, 1250 Moursund Street, Suite 1325, Houston, TX 77030, USA e-mail: sillitoe@bcm.edu