- 1Centre for Ophthalmology, Institute for Ophthalmic Research, University of Tübingen, Tübingen, Germany
- 2Werner Reichardt Centre for Integrative Neuroscience (CIN), University of Tübingen, Tübingen, Germany
- 3Bernstein Center for Computational Neuroscience Tübingen, University of Tübingen, Tübingen, Germany
During neuronal degenerative diseases, neuronal microcircuits undergo severe structural alterations, leading to remodeling of synaptic connectivity. The functional consequences of such remodeling are mostly unknown. For instance, in mutant rd1 mouse retina, a common model for Retinitis Pigmentosa, rod bipolar cells (RBCs) establish contacts with remnant cone photoreceptors (cones) as a consequence of rod photoreceptor cell death and the resulting lack of presynaptic input. To assess the functional connectivity in the remodeled, light-insensitive outer rd1 retina, we recorded spontaneous population activity in retinal wholemounts using Ca2+ imaging and identified the participating cell types. Focusing on cones, RBCs and horizontal cells (HCs), we found that these cell types display spontaneous oscillatory activity and form synchronously active clusters. Overall activity was modulated by GABAergic inhibition from interneurons such as HCs and/or possibly interplexiform cells. Many of the activity clusters comprised both cones and RBCs. Opposite to what is expected from the intact (wild-type) cone-ON bipolar cell pathway, cone and RBC activity was positively correlated and, at least partially, mediated by glutamate transporters expressed on RBCs. Deletion of gap junctional coupling between cones reduced the number of clusters, indicating that electrical cone coupling plays a crucial role for generating the observed synchronized oscillations. In conclusion, degeneration-induced synaptic remodeling of the rd1 retina results in a complex self-sustained outer retinal oscillatory network, that complements (and potentially modulates) the recently described inner retinal oscillatory network consisting of amacrine, bipolar and ganglion cells.
Introduction
The mutant rd1 mouse (Bowes et al., 1990) is an intensively studied animal model for human Retinitis Pigmentosa-related retinal degeneration. In the rd1 retina, rod photoreceptors (rods) start degenerating around postnatal day 10 (P10) and are virtually absent by P21 (Carter-Dawson et al., 1978; Jimenez et al., 1996). During this progressive rod degeneration, cones, although not directly affected by the rd1 mutation, undergo secondary degeneration. Some atrophied cones remain in the outer retina for over 1 year (Garcia-Fernandez et al., 1995). However, with the loss of cone outer segments after P24 (Lin et al., 2009), light-evoked retinal activity is absent in the rd1 retina (Stasheff, 2008), thus, the disease leads to complete blindness within the first postnatal month.
Loss of light-driven activity is accompanied by a dramatic increase in spontaneous activity of the inner retina: such activity has been described in bipolar cells (Borowska et al., 2011) and ganglion cells (Margolis et al., 2008). It was suggested that AII amacrine cells and ON-cone bipolar cells form an intrinsic oscillator that serves as a potential source of this spontaneous activity (Borowska et al., 2011; Menzler and Zeck, 2011; Trenholm et al., 2012). In the healthy retina, AIIs receive glutamatergic input exclusively from rod bipolar cells (RBCs) (for review, see Bloomfield and Dacheux, 2001). However, if spontaneous activity in the AII/ON-cone bipolar cell network of rd1 retina is intrinsic or modulated by RBCs has remained unclear.
In the outer rd1 retina, cones and RBCs undergo structural synaptic remodeling: cones establish ectopic synapses with RBC somata (Peng et al., 2000). Rod bipolar cells lose their dendrites and down-regulate expression of metabotropic glutamate receptor 6 (mGluR6) (Strettoi and Pignatelli, 2000) as well as the respective effector cation channel TRPM1 (Krizaj et al., 2010) but remain active (Borowska et al., 2011). It is conceivable that the substantial remodeling of the rd1 retina following photoreceptor loss leads to generation of spontaneous activity in the outer retina, which may contribute to or modulate the oscillatory activity observed in the inner retina. Investigating activity in the remodeled outer rd1 retina is important as it improves our understanding of the general synaptic mechanisms underlying spontaneous activity in degenerated nervous tissue. Moreover, it is crucial to identify potential means for spontaneous activity suppression (Toychiev et al., 2013), which may greatly improve e.g., the responsiveness of the degenerated retina to optogenetic approaches (Lagali et al., 2008; Busskamp et al., 2010) and electronic implants (Zrenner et al., 2011; Zrenner, 2013) as treatments for vision loss.
Here, we studied neuronal activity in the outer retina of adult rd1 mice using Ca2+ imaging and consecutive immunohistochemistry. We investigated how synaptic remodeling alters network function and found spontaneous, synchronized Ca2+ oscillations in cell clusters consisting of cones, RBCs and/or HCs. Our data suggest that gap junctionally-coupled cones, modulated by GABAergic inhibition from HCs, are responsible for generating synchronized, outer retinal activity. We further show that correlated activity between cones and RBCs depends on glutamate transporters expressed by RBCs, suggesting the appearance of atypical, sign-conserving cone-RBC synapses in the remodeled outer rd1 retina.
Methods and Materials
Animals
We used adult mice (both genders) at postnatal days (P) 30–60 crossbred from the transgenic HR2.1:TN-XL (Wei et al., 2012) and Cx36−/− (Güldenagel et al., 2001) lines with the C3H/rd1 (Bowes et al., 1990) strain. The resulting rd1 x HR2.1:TN-XL and rd1 x Cx36−/− mice were homozygous for the rd1 allele. We used n = 32 rd1 x HR2.1:TN-XL mice and n = 12 C3H/rd1 mice; both lines are referred to as “rd1.” In addition, we used n = 4 rd1 x Cx36−/− mice. Animals were anesthetized with Isoflurane (Baxter, Germany) and killed by cervical dislocation. All procedures were performed in accordance with the law on animal protection (Tierschutzgesetz) issued by the German Federal Government.
Tissue Preparation
Eyes were enucleated and the retina was isolated in extracellular solution containing (in mM): 125 NaCl, 2.5 KCl, 2 CaCl2, 1 MgCl2, 1.25 NaH2PO4, 26 NaHCO3, and 20 glucose, and was maintained at pH 7.4 using carboxygen (95% CO2/5% O2). All chemicals were purchased from Sigma-Aldrich (Germany) and Merck (Germany). Since rd1 degeneration progresses with age and retinal eccentricity, recorded fields (146 × 110 μm) were consistently taken within a ~800 μm radius from the optic disk. Retinas were incubated in extracellular solution containing 0.27 μM Fura-2-AM and 0.1% Pluronic acid (Invitrogen, Eugene, USA) for 35′ at room temperature (RT), washed, mounted on filter membranes (Millipore, 0.8 μm pores) outer retina side up, and transferred to the recording chamber, where the tissue was perfused with carboxygenated medium at 32°C.
Notably, we were not able to evoke light-driven activity in rd1 mouse retina at P30 (as measured by Ca2+ imaging in the outer retina and by electrical recordings from ganglion cells; own unpublished data), which is consistent with earlier reports (Stasheff, 2008).
Calcium Imaging and Data Analysis
To record Ca2+ signals simultaneously in different cell types, including cones, RBCs and HCs, we decided to use a synthetic fluorescent Ca2+ indicator that can be loaded non-selectively into the retinal tissue (see above). Because ratiometric measurements are much less sensitive to experimental artifacts (e.g., tissue motion during drug application), we selected the Ca2+ indicator Fura-2 (Grynkiewicz et al., 1985). The genetically encoded Ca2+ sensor TN-XL, which is selectively expressed in cones in the rd1 x HR2.1:TN-XL strain, was here only used for the alignment of recorded with immunostained retinal regions (see Methods, Immunohistochemistry). No differences in activity were detected between cones that contained only Fura-2 (in C3H/rd1 animals) and those containing both Fura-2 and TN-XL (in rd1 x HR2.1:TN-XL animals), therefore we consider it unlikely that the presence of two Ca2+ buffers critically affected our results.
For Fura-2 Ca2+ imaging, we used an upright fluorescence microscope (BX50WI, Olympus, Germany) equipped with a 40x water immersion objective (LUMPLFLN, 40x/0.80W, ∞/0, Olympus), a polychromator (POLYCHROME II, Till Photonics, Germany) and a CCD camera (TILL Imago X.Y, Till Photonics) with a resolution of 640 × 480 pixels (9.9 × 9.9 μm pixels, with a bit depth of 12), corresponding to a retinal area of 0.25 × 0.25 μm per pixel when using the 40x objective. For all experiments a 2 × 2 pixel binning was used. Single-plane two-channel image stacks of the Fura-2 fluorescence in the outer retina were acquired at 10 Hz (λexc = 340 and 380 nm; Olympus U-MNU filter set, 30 ms exposure time) using the TillVision software (v4.0, Till Photonics).
For offline analysis, ratio image stacks were generated by dividing the fluorescence images recorded at the two excitation wavelengths (F340/380). Cells that generated transient Ca2+ events (“active cells”) were identified by calculating the standard deviation (SD) image of each stack using ImageJ (http://rsbweb.nih.gov/ij). The SD images also confirmed that the observed activity was almost exclusively restricted to clearly delineated areas of 5–15 μm in diameter (cf. Figure 2B, left column), pointing at active cells and arguing against substantial contributions from the surrounding neuropil.
For the subsequent analysis we used custom scripts (MATLAB, The MathWorks, Germany). Active cells were manually encircled by regions of interest (ROIs) to retrieve their F340/380 (ratio) response trace. We used F340/380 as a proxy for changes in Ca2+ concentration. Because we were mainly interested in Ca2+ spiking activity (see below) and not in absolute Ca2+ levels, we refrained from calibrating the system. The baseline was determined by averaging F340/380 trace sections between events across the cells of each recorded field. Only cells with signal-to-noise ratios ≥ 10 (with the transient amplitude as signal and 1 SD of the baseline fluctuations as “noise”) were included in the analysis. From the ratio traces we then determined the mean Ca2+ event frequency (Fmean) for each cell using a peak detection routine (based on MATLAB's findpeaks routine). In addition, the pair-wise Pearson's correlation coefficient index (Ci) was calculated for all cell pairs (using the corrcoef MATLAB routine). Higher event frequencies, for instance as a result of pharmacologically blocking inhibitory feedback (e.g., Figures 6B,E), could, in principle, result in Ci values purely by chance. Therefore, we tested for dependence between Fmean and Ci (using linear regression) but did not find any substantial correlations (control: R2 = 0.011; with TPMPA/Gabazine, e.g., Figure 6B: R2 = 0.009; with NBQX, Figure 6E: R2 = 0.001). This indicates that Ci can indeed be used as a measure for changes in correlated activity between cells. Cell position and type, as well as Fmean and Ci were then compiled to activity maps of each recorded region. The Wilcoxon signed-rank test and the student's t-test were used to evaluate drug effects; statistical significance is indicated as *p ≤ 0.05, **p ≤ 0.01, ***p ≤ 0.001, and all parameters are given as mean ± s.e.m.
Note that we did not detect comparable spontaneous events in cones in retinal whole mounts of HR2.1:TN-XL “wild-type” mice (our unpublished data). This is consistent with the observation of Borowska et al. (2011) that bipolar cells recorded in wild-type mouse whole mounts do not display spontaneous (oscillatory) activity. In contrast, in retinal slices of HR2.1:TN-XL “wild-type” mice we did observe spontaneous events, in particular, when HC feedback inhibition was pharmacologically blocked (see Discussion).
Pharmacology
Drugs were bath applied for 10′ and washed out for 20′. We used (in μM): 50 TPMPA (GABAC receptor antagonist; 1,2,5,6-Tetrahydropyridin-4-yl)met hylphosphinic acid), 10 Gabazine (Gz, GABAA receptor antagonist; 6-Imino-3-(4-methoxyphenyl)-1(6H)-pyridazine-butanoic acid hydrobromide), 75 DL-TBOA (glutamate transporter antagonist; DL-threo-β-Benzyloxyaspartic acid) and 100 CPPG (mGluR6 antagonist; (RS)-α-Cyclopropyl-4-phosphonophenylglycine) were purchased from Tocris Bioscience; 1 strychnine (glycine receptor antagonist), 100 Verapamil (L-type voltage-gated calcium channel blocker) and 100 carbenoxolone (CBX, gap junction blocker; (3β,20β)-3-(3-Carboxy-1-oxopropoxy)-11-oxoolean-12-en-29-oic acid disodium) were purchased from Sigma-Aldrich; 100 L-AP4 (mGluR6 agonist; L-2-amino-4-phosphonobutyric acid) and 20 NBQX (AMPA/kainate-type GluR antagonist; 2,3-Dioxo-6-nitro-1,2,3,4-tetrahydro-benzo[f]quinoxaline-7-sulfonamide) were purchased from Bio Trend. For some experiments, glutamate (250 μM) was directly puffed with a glass pipette for 750 ms onto recorded outer retina neurons using a VC3−8 perfusion system (ALA Scientific Instruments, USA).
Immunohistochemistry
After Ca2+ imaging, retinas were fixated with 4% paraformaldehyde (PFA) in extracellular solution for 15′ at RT and washed twice for 20′ in 0.1 M phosphate buffered saline (PBS, in mM: 20 NaH2PO4, 80 Na2HPO4, 154 NaCl; pH 7.4) at 4°C. Retinas were then incubated overnight at 4°C in blocking solution containing 0.3% Triton X-100, 1% BSA and 10% corresponding normal serum from the host animals used for generating the respective secondary antibodies. Subsequently, retinas were incubated for 1–3 days in primary antibodies (Table 1), washed 6 times for 5′ in PBS and then incubated overnight at 4°C in secondary antibodies (1:750, Alexa Fluor conjugates; Invitrogen). After mounting on slides using Vectashield (Vector, Burlingame, CA, USA) the retinas were imaged (stacks with 0.3–0.95 μm z-axis steps) using a Zeiss Imager Z1 Apotome (Oberkochen, Germany; Plan-Apochromat 5x/0.16, 20x/0.8 and EC Plan-Neofluar 40x/1.3 oil; filter set #38 for Alexa Fluor 488, #10 for Alexa Fluor 568, #50 for Alexa Fluor 633, #49 for DAPI). Fixated vertical retina sections (22 μm) were incubated overnight in primary antibodies, for 1 h in secondary antibodies and subsequently imaged as described above (stacks w/ 0.32 μm z-axis steps).
In the rd1 x HR2.1:TN-XL mouse line, in which cones are fluorescently labeled by TN-XL, identification of cell types and alignment of Ca2+ imaged regions with the tissue after immunostaining was greatly facilitated. Directly after Ca2+ imaging, a picture of the recorded region was taken to visualize TN-XL-expressing cones (using the U-MSWG Olympus filter set). The pattern of TN-XL-positive cones was then used as landmark to find the recorded region on the immunostained retina and to determine active cell types by overlaying Fura-2 image, TN-XL image, and the images of the immunostained retina. We observed little shrinkage of the retinal tissue (5–7% compared to the living retina); in any case, because that shrinkage was homogenous we were able to register pictures from live-imaging and immunostained material reliably (in 51 of 58 cases; for examples, see Figures 1C, 7A). In the rd1 x HR2.1:TN-XL line, TN-XL labeling in cones was intensified using antibodies against GFP (which also works for labeling TN-XL). In experiments using the C3H/rd1 and rd1 x Cx36−/− lines we identified cones using recoverin staining (Lambrecht and Koch, 1992). To identify Fura-2 labeled non-cone cell types, we stained the fixed tissue for PKCα to label RBCs (Berrebi et al., 1991) and for calbindin to label HCs (Pasteels et al., 1990). The Fura-2 labeled cells that were not stained by these two antibodies likely represented cone bipolar cells.
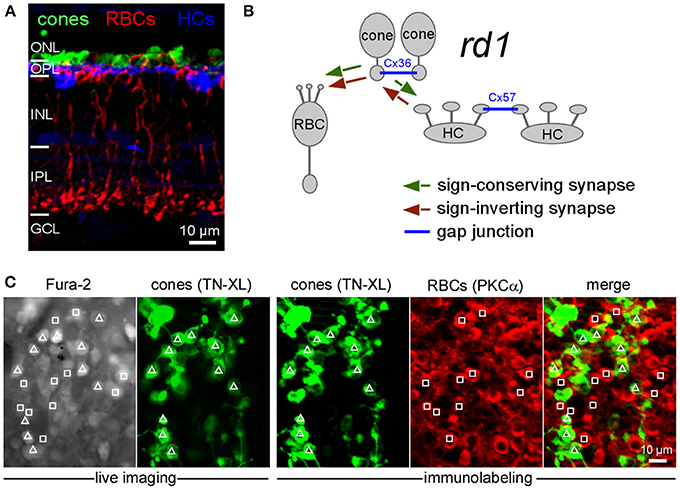
Figure 1. Cell type identification following Ca2+ imaging in the outer retina of rd1 mice. (A) Vertical section of a P35 rd1 retina immunostained for TN-XL (cones), PKCα (RBCs) and calbindin (HCs). (B) Drawing illustrating sign-conserving (green), sign-inverting (red), and electrical (blue) synaptic interactions in the outer rd1 retina. For simplicity, interactions between HCs and RBCs are not shown. (C) From left to right: Fura-2-labeled neurons in outer retina in living P35 rd1 wholemount retina (F340 channel), cones expressing TN-XL (live image taken at the same retinal location as Fura-2 image; triangles indicate cones), immunolabeling of same wholemount retina subsequent to the Ca2+ imaging experiment showing cones stained for TN-XL and PKCα-stained RBCs (red, squares). (ONL, outer nuclear layer; OPL, outer plexiform layer; INL, inner nuclear layer; IPL, inner plexiform layer; GCL, ganglion cell layer; RBC, rod bipolar cell; HC, horizontal cell).
Results
Neurons in the Outer rd1 Retina Display Spontaneous Activity
At the adult age of mice when Ca2+ recordings were performed, cone somata form a single irregular cell layer in the outer rd1 mouse retina (Figures 1A,C) (Carter-Dawson et al., 1978; Jimenez et al., 1996). Therefore, all neuron types of the rd1 outer retina (Figure 1B, cones, HCs and bipolar cells), are accessible from the distal retinal surface and their activity can be recorded in the Fura-2 loaded rd1 retinal wholemount using Ca2+ imaging (Figure 1C). Subsequent to the Ca2+ imaging, we performed immunocytochemistry to identify cones, RBCs and HCs (Figures 1C, 7A). In adult rd1 mice, up to 35% of all Fura-2 loaded cells in the outer retina were spontaneously active and displayed transient Ca2+ events (Figures 2A,B; cones: τ = 112 ± 182 ms, n = 30; RBCs: τ = 177 ± 173 ms, n = 22; unidentified: τ = 135 ± 152 ms, n = 15). While the mean frequency (Fmean) for individual cells reached up to 3 Hz, the studied types/groups of cells were typically dominated by frequencies below 1 Hz (cones: Fmean = 0.65 ± 0.35 Hz, n = 902; RBCs: Fmean = 0.38 ± 0.42 Hz, n = 766; HCs: Fmean = 0.26 ± 0.15 Hz, n = 43; unidentified: Fmean = 0.57 ± 0.61 Hz, n = 94; see also Figure 2E).
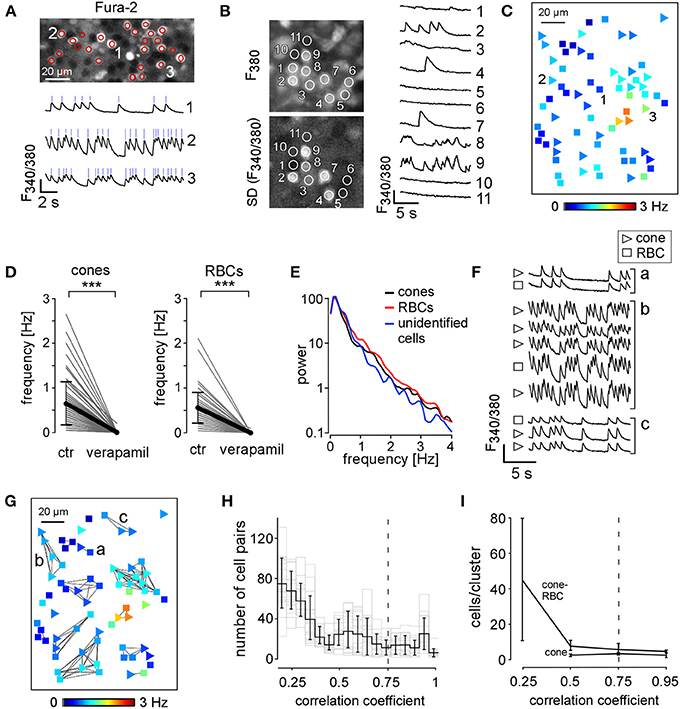
Figure 2. Spontaneous activity in the outer retina of rd1 mice. (A) Fura-2-labeled rd1 retina wholemount (SD image of F340/380 ratio) with active cells encircled (regions of interest, ROIs) and exemplary Ca2+ traces (ROIs 1–3; blue ticks mark peaks of Ca2+ transients used to determine Fmean). (B) left: wholemount with ROIs placed on different regions: Fura-2-labeled cells, ROIs 1–9; active cells, ROIs 2–4, 7–9; unlabeled cells/space, ROIs 10,11 (top: averaged time series, F380 channel; bottom: SD image of F340/380 ratio, same time series). Right: corresponding Ca2+ traces. (C) Activity map showing distribution of mean frequency (Fmean, color-coded) of spontaneous Ca2+ transients in cones (triangles) and RBCs (squares). (D) Verapamil abolishes spontaneous activityin cones (left) and RBCs (right). (E) Power spectrum of the activity in cones (black, n = 81), RBCs (red, n = 108), and unidentified cells (blue, n = 4); data from 3 experiments. (F) Exemplary Ca2+ traces of cells exhibiting synchronous activity (clusters a-c in G). (G) Activity map showing clusters with synchronous Ca2+ transients (cross-correlation coefficient ≥ 0.75 indicated by lines). (H) Histogram showing the distribution of cross correlations between measured cell pairs (n = 19 retinas) for different correlation coefficients. Gray dashed line indicates correlation coefficient ≥ 0.75 used for our analysis. (I) Plot showing cluster size (cells/cluster) of cone-only and cone-RBC clusters for different correlation coefficients. Cluster size did not vary for correlation coefficients ≥ 0.5. All error bars indicate s.e.m.
Of each recorded field we generated an “activity map” that provided information about cell type, position, and Fmean of every active, identified cell (Figure 2C). Cells analyzed in n = 25 rd1 retinas included 50.1% cones, 42.6% RBCs, 2.3% HCs, as well as 5% unidentified cells. The latter consisted of cells that could not be clearly assigned to stained counterparts and presumably also contained one or more types of cone bipolar cell. Because they were infrequent and likely a heterogeneous group, we did not study them further.
Spontaneous Ca2+ Transients in the Outer rd1 Retina Rely on Voltage-Gated Ca2+ Channels
To assess whether spontaneous Ca2+ transients in rd1 cones depend on activation of L-type voltage-gated Ca2+ channels (VGCCs), which are present in cones (Nachman-Clewner et al., 1999), we applied the specific antagonist verapamil. Blocking L-type VGCCs eliminated spontaneous cone activity (Figure 2D, left), suggesting that fluctuations of the cone membrane potential that activate VGGCs are required for the observed cone Ca2+ transients. Verapamil also eliminated spontaneous RBC activity (Figure 2D, right), likely as a result of both the blockade of L-type VGCCs in RBCs (Protti and Llano, 1998) and the reduction of glutamatergic drive from cones. Together these results suggest that the measured Ca2+ transients reflect electrical neuronal activity in the outer rd1 retina. It is possible that the frequency of spontaneous Ca2+ events represents an underestimate of the cells' electrical activity, as we can detect only membrane potential fluctuations that are large enough to trigger VGGCs. Furthermore, it is possible that Ca2+ release from internal stores contributed to the measured signals (Wei et al., 2012). In principle, spontaneous activity in the inner rd1 retina (Borowska et al., 2011) may also contribute to outer retinal activity i.e., via glycinergic and/or GABAergic input from amacrine cells (ACs) to BC terminals or possibly via GABAergic interplexiform cells to the outer plexiform layer (OPL). However, it is unlikely that the observed outer retinal activity relied on such input, because blocking glycine receptors had no effect while GABA receptor antagonists even enhanced that activity (Table 2, cf. also Figure 6B). Additionally, multi-electrode recordings in the rd1 retina (Ye and Goo, 2007) showed that while spiking activity is blocked by TTX, a slow (~3.2 Hz) component persists. Because TTX was demonstrated to block inner retinal activity (Trenholm et al., 2012), the finding by Ye and Goo (2007) points at the presence of oscillatory network in the rd1 retina that does not depend on inner retinal activity.
Clusters of Synchronous Activity in the rd1 Outer Retina
Calcium transients were often synchronized in neighboring cells, forming clusters of correlated oscillatory activity (Figure 2F). Such correlated activity between cells is indicated in activity maps by connecting lines visualizing synchronized cell clusters (Figure 2G). While already correlation coefficient thresholds ≥ 0.5 were sufficient to reliably identify clusters (Figures 2H,I, cf. also respective histograms in Figures 5E, 6H,I for different pharmacological conditions), we used a more “conservative” threshold of 0.75 to focus on the strong interactions between cells.
Most activity clusters comprised both cones and RBCs. Under control conditions, we detected in 22 rd1 retinas a total of 144 clusters, including 92 cone-RBC clusters (5.5 ± 3.4 cells/cluster), 31 cone-only clusters (3.0 ± 1.1 cells/cluster, see Table 3) and 21 RBC-only clusters (2.2 ± 0.4 cells/cluster). It is likely that the relatively rare RBC-only clusters actually were cone-RBC clusters for which the contributing cones could not be identified—for instance because they were located outside the recorded area (see, for example, lower left cluster in Figure 2G). Therefore, we did not include RBC-only clusters in our analysis. We also found synchronous activity between cones, RBCs and unidentified cells (data not shown). Because unidentified cells were rather infrequent and represent a heterogeneous group (see above), we excluded this group from the cluster analysis. In addition, in some experiments we found synchronous oscillatory activity among HCs and between HCs and cones (Figure 7).
Cone and Rod Bipolar Cells Activity is Synchronized by EAAT5 Receptors
In the wild-type mammalian retina, ON bipolar cells, including RBCs, integrate glutamatergic photoreceptor output using the sign-inverting mGluR6 (Nakajima et al., 1993; Pang et al., 2010). Thus, activity in photoreceptors and ON bipolar cells is expected to be negatively correlated. Our finding of positively correlated activity between cones and RBCs in the rd1 retina was therefore surprising. It suggests that previously described ectopic synapses between the bulb-like neurites sprouting from cones and RBC somata (Figure 3; see also Peng et al., 2000; Cuenca et al., 2004) are functional and sign-conserving. A potential alternative explanation is that fluorescence signals from neighboring labeled cells combined into one ROI caused false-positive correlations. However, we think that the contribution of such “bleeding-in” was marginal (see Figure 2B and Methods): in the x-y plane Ca2+ transients were localized to defined cell-sized areas and, thus, mostly well-separated from their active neighbors, and along the z-axis, we typically saw little “stacking” of active cells.
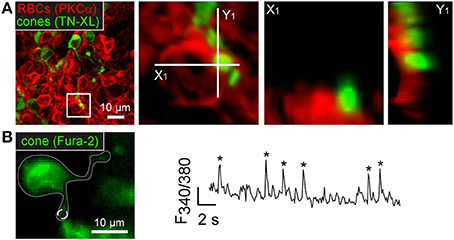
Figure 3. Degenerating cones sprout and contact rod bipolar cells in rd1 mice. (A) Immunolabeled cones (TN-XL, green) and RBCs (PKCα, red) in P35 rd1 retina. Higher magnification view of boxed area showing a RBC soma contacted by a cone neurite. X-images show horizontal and Y-images vertical single-plane sections of higher magnification view. (B) Left: Fura-2-and TN-XL-labeled cone (gray line outlines cone; dashed white circle indicates ROI). Right: Ca2+ trace (F340/380 ratio) with transients (indicated by asterisks) recorded in ROI in cone process (white dashed circle).
A possible biological explanation for sign-conserving synaptic transmission between rd1 cones and RBCs could be degeneration-induced expression of AMPA/kainate-type GluRs on RBCs (Chua et al., 2009). However, this seems unlikely, because application of NBQX, an AMPA/kainate-type GluR antagonist, increased the activity of RBCs and the number of cone-RBC clusters (see Tables 2, 3; cf. also Figures 6E,F), likely due to a reduction of inhibition by HCs (for discussion, see Thoreson and Mangel, 2012), which possess AMPA/kainate-type GluRs (Schubert et al., 2006; Ströh et al., 2013).
An alternative explanation involves glutamate transporters and a transmission pathway that has been described in fish bipolar cells (Grant and Dowling, 1995). In wild-type mice, the glutamate transporter EAAT5 is present on both RBC axon terminal and soma (Wersinger et al., 2006). EAAT5 exhibits a Cl− conductance that is activated by glutamate binding but independent from the actual glutamate transport across the membrane (Fairman et al., 1995; Arriza et al., 1997). Because the Cl− reversal potential in the RBC dendro-somatic compartment is more positive than the resting potential (Billups and Attwell, 2002; Varela et al., 2005), activation of the EAAT5 Cl− conductance by glutamate released from the cones is expected to trigger Cl− efflux from the RBCs. The bipolar cells would depolarize, leading to activation of VGCCs (Protti and Llano, 1998) and, consequently synchronize Ca2+ transients in cones and RBCs.
To test this hypothesis, we first confirmed the presence of EAAT5 on rd1 cones and RBCs using immunocytochemistry (Figures 4A–C). Then we measured the effect of the general glutamate transporter antagonist TBOA on the synchronized Ca2+ activity between cones and RBCs (Figure 4D). As predicted by our hypothesis, TBOA significantly reduced the numbers of cone-RBC clusters (Figure 4E; for this and further statistics, see Table 3), likely by blocking EAAT5. Because of the comparably low number of clusters that we typically found under control conditions, we also evaluated the effect of TBOA on tissue in which we first enhanced the activity (and thereby the cluster number). Blocking GABAA/C receptors with the antagonists Gabazine/TPMPA increased spontaneous activity and enhanced synchronous activity between cones and RBCs (Figures 4F,G; see Tables 2, 3). This observation is consistent with inhibitory GABAergic feedback (see Horizontal cells attenuate activity in the rd1 outer retina), as rd1 cones (Pattnaik et al., 2000) express GABA receptors—notably, in contrast to wild-type cones (Kemmler et al., 2014). To study the TBOA effect on enhanced rd1 activity, we first applied Gabazine/TPMPA and then added TBOA. Co-application of TBOA reduced RBC activity (Figure 4F) but at the same time increased activity in cones (Table 2). The opposite effect on the two cell types is not surprising, because—other than in RBCs (see above)—cone Cl− concentration is low and, thus, the Cl− reversal potential is more negative than their resting potential (Picaud et al., 1995). Hence, blockage of a transporter-mediated Cl− inward current should lead to cone disinhibition. In line with the previous experiments (Figure 4E), TBOA strongly reduced the number of cone-RBC clusters also in the presence of GABA receptor antagonists (Figure 4G, Table 3). At the same time, the number of cone-only clusters increased but not significantly, which likely reflects “decoupling” of cone-RBC connections but may also partially explained by the increased number of active cones, that is, more cone-only clusters reached our correlation threshold.
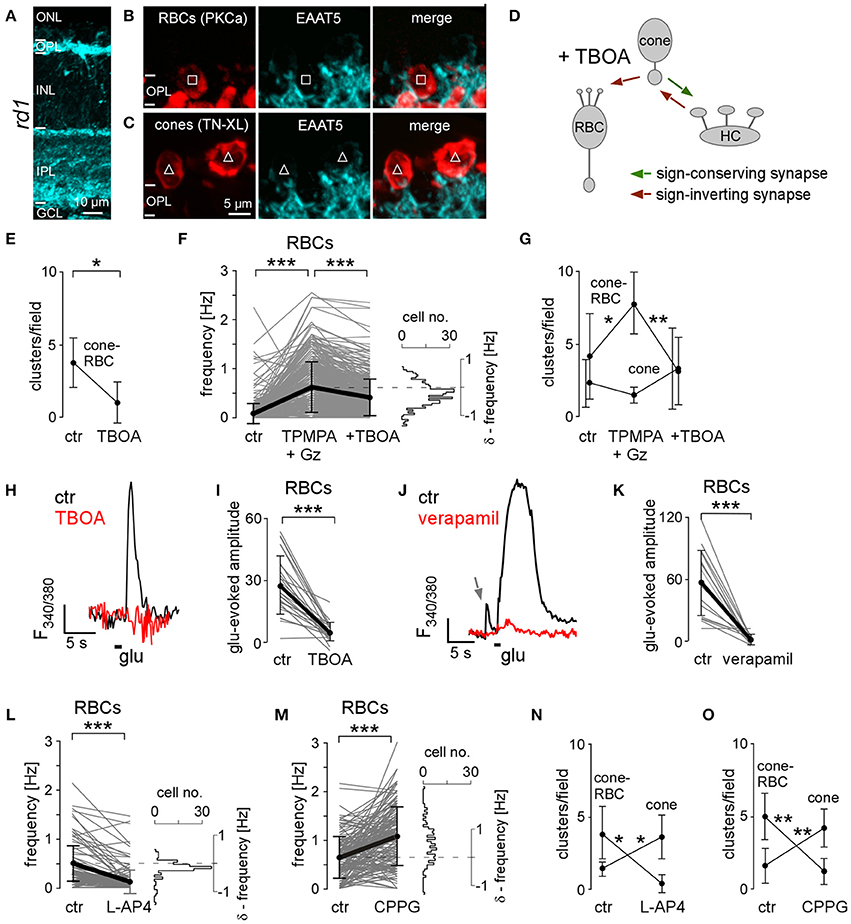
Figure 4. Glutamate transporters mediate input from rd1 cones to rod bipolar cells. (A–C) Vertical sections of P35 rd1 retina immunostained for EAAT5 (A–C, cyan) and co-labeled for RBCs (B, PKCα in red, square) or cones (C, TN-XL in red, triangles). (D) Drawing shows synaptic interactions with glutamate transporter antagonist TBOA blocking sign-conserved cone input to RBCs. (E) Number of cone-RBC clusters before (ctr) and with TBOA. (F) Mean frequency (Fmean) of Ca2+ transients in RBCs before (ctr), with GABA receptor blockers (TPMPA+Gz), and with TBOA applied in addition (+TBOA). (G) Effect of the drugs (from F) on the number of clusters. (H) Example recording showing glutamate puff-evoked Ca2+ signal in a RBC before (ctr, black) and with TBOA (red). (I) Effect of TBOA on the glutamate puff-evoked Ca2+ signal in RBCs (n = 26). (J) Example recording showing glutamate puff-evoked Ca2+ signal in a RBC before (ctr, black) and with verapamil (red); gray arrow indicates spontaneous Ca2+ transient. (K) Effect of verapamil on the glutamate puff-evoked Ca2+ signal in RBCs (n = 17). (L,M) Fmean in RBCs before, with the mGluR6 agonist L-AP4 (L), and with the mGluR6 antagonist CPPG (M). (N,O) Effect of L-AP4 and CPPG on cluster number. All error bars indicate s.e.m.
The results so far predict that activation of glutamate transporters on rd1 RBCs should cause depolarization due to their high intracellular Cl− concentration and Ca2+ responses due to VGCC activation. To test this prediction, we puffed glutamate directly onto RBCs and found that glutamate evoked large Ca2+ transients (Figures 4H,J). Puffing glutamate in the presence of TBOA (Figures 4H,I) or verapamil (Figures 4J,K) failed to evoke responses, suggesting that indeed glutamate transporter-mediated depolarization and downstream activation of VGCCs underlie Ca2+ transients in RBCs. The possibility that glutamate acted on HCs which, in turn, synaptically excited RBCs, can be excluded: both the application of NBQX (blocking HC input) and of TPMPA+Gz (blocking potential RBC input from HCs) increased spontaneous activity in RBCs (see also below), indicating that there is no direct (GABAergic) excitatory drive from HCs to RBCs. Thus, our data indicate that correlated cone-RBC activity is based on sign-conserving ectopic synapses between cones and RBCs and mediated, at least partially, by glutamate transporters (likely EAAT5).
mGluR Modulates Synaptic Activity Between Cones and RBCs
Is there a role for mGluR6, which mediates transmission from rods to RBCs in wild-type mammalian retina (Nakajima et al., 1993), also in rd1? To test this, we bath-applied the group III mGluR agonist L-AP4 and antagonist CPPG and measured spontaneous activity. We found that L-AP4 decreased RBC activity, whereas CPPG led to an increase in RBC activity (Figures 4L,M, Table 2). This result is consistent with the “normal,” sign-inverting mGluR6 signaling: L-AP4 mimics glutamate binding to mGluR6, leading to the closure of downstream TRPM1 cation channels, whereas CPPG mimics glutamate unbinding from mGluR6, leading to the opening of TRPM1 channels (Morgans et al., 2009). During L-AP4 or CPPG application, cone-RBC clusters were almost absent and the number of cone-only clusters increased significantly (Figures 4N,O). This suggests that: (i) rd1 RBCs still express functional mGluR6, although the transmission from the cones appears to be dominantly mediated by glutamate transporters, as we find cone-RBC activity to be positively and not negatively correlated (see previous section). (ii) Hyperpolarizing or depolarizing the rd1 RBCs by modulation of the TRPM1 channels via the activation or blockade of mGluR6, respectively, appears to “clamp” the cells' membrane potential and thereby effectively “decouples” RBCs from cone input—restricting synchronous activity largely to cone-only clusters.
In addition to mGluR6 on RBCs, there is also at least one other group III mGluR in the OPL that is sensitive to L-AP4 and CPPG: the mGluR8 auto-receptor on photoreceptors (Koulen et al., 1999). Other than for RBCs, not only the antagonist CPPG significantly increased the frequency of cone Ca2+ transients, but also the agonist L-AP4 (see Table 2). However, the relative cone Ca2+ level (as reflected by the mean baseline ratio) increased with CPPG (control: 1.3 ± 0.4, CPPG: 1.7 ± 0.6, n = 197, p ≤ 0.001) but decreased with L-AP4 (control: 1.5 ± 0.6, L-AP4: 1.2 ± 0.6, n = 122, p ≤ 0.001), consistent with mGluR8 auto-receptors modulating the cone's basal Ca2+ level (Koulen et al., 1999). Why cones became more “spiky” despite a reduction in basal Ca2+ level during L-AP4 application remains to be investigated.
Taken together, glutamate released from rd1 cones modulates both the activity of cones (auto-reception via mGluR8, EAATs) and RBCs (via mGluR6, EAATs), nevertheless, transmission from cones to RBCs appears to be dominated by sign-conserving glutamate transporter activation.
Disrupting Electrical Cone Coupling Eliminates Synchronous Activity in the Outer rd1 Retina
The great majority of the clusters included cones, indicating that spontaneous activity may be initially generated in the “island-like” small groups of cones and is then synaptically spread to RBCs and HCs. But what synchronizes spontaneous activity in cones in the first place? Cones are electrically coupled via gap junctions formed by connexin36 (Cx36) (Feigenspan et al., 2004). In the rd1 retina, neuritic protrusions of remnant cones express Cx36 (Figure 5A), suggesting that clustered cones remain electrically coupled during degeneration. To uncouple the cone network, we used the gap junction blocker carbenoxolone (CBX). Since CBX is non-selective and therefore also uncouples the gap junctions of the HC network, we first reduced HC feedback using GABA receptor blockers and then co-applied CBX (Figures 5B,C). Note that CBX may also contribute to the reduction of HC-cone feedback by eliminating the alternative hemichannel-mediated ephaptic HC feedback pathway (Kamermans and Fahrenfort, 2004). We found the number of cone-only and cone-RBC clusters was significantly decreased by adding CBX (Figures 5D,E, Table 3), suggesting that electrical cone coupling is important for synchronizing rd1 activity.
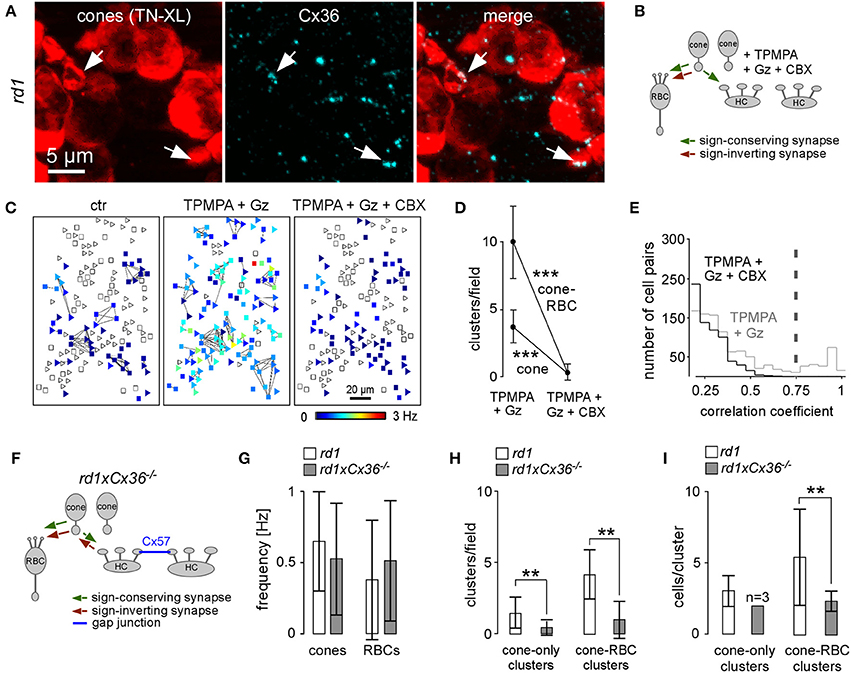
Figure 5. Uncoupling of gap junctions between cones reduces correlated activity but not spontaneous activity. (A) Left: immunolabeling of a P30 rd1 wholemount retina showing cone somata (TN-XL, red) and smaller neurite protrusions. Middle: Cx36 immunolabeling (cyan) in same region. Right: overlay showing that cone neurite protrusions express Cx36 (arrows). (B) Drawing shows synaptic interactions with CBX blocking electrical coupling among cones and among HCs as well as possible ephaptic HC feedback. (C) Representative activity map before (ctr), with GABA receptor blockers (TPMPA+Gz), and with additional gap junction blocker CBX (cones, triangles; RBCs, squares). (D) Effect of the drugs on cluster number. (E) Distribution of correlation coefficients for control (gray line, TPMPA+Gz) and drug condition (black line, TPMPA+Gz+CBX). Gray dashed line indicates correlation coefficient ≥ 0.75 used for our analysis. (F) Drawing illustrating the situation in the rd1 x Cx36−/− retina with cone-cone coupling selectively abolished. (G) Genetic deletion of Cx36 did not affect Fmean. (H) Comparison of cluster number in rd1 and Cx36 knockout mice (rd1 x Cx36−/−). (I) Bar graphs showing that the cone-RBC cluster size was reduced in rd1 x Cx36−/−. All error bars indicate s.e.m.
Because CBX acts non-selectively on gap junctions and hemichannels, and has potential side-effects on VGCCs (Vessey et al., 2004), we also used rd1 mice crossbred with a Cx36 knockout line (rd1 x Cx36−/−) (Figure 5F), in which photoreceptor coupling is eliminated. While the overall activity in cones and RBCs was not altered in the rd1 x Cx36−/− retina (Figure 5G, Table 2), the number of cone-only and cone-RBC clusters (Figure 5H) and the number of cells per cone-RBC clusters (Figure 5I, Table 3) were significantly lower. This supports our interpretation of the CBX effect that electrical coupling between cones is crucial for synchronizing activity clusters in the outer rd1 retina. That the results in the rd1 x Cx36−/− retina are very similar to those with CBX also argues against a substantial suppressive effect on VGCCs at the CBX concentrations we used.
Horizontal Cells Attenuate Activity in the rd1 Outer Retina
Mouse horizontal cells receive glutamatergic input from cones via AMPA/kainate-type GluRs (Schubert et al., 2006; Ströh et al., 2013). In turn, they provide feedback and feedforward input to photoreceptors and bipolar cells, respectively, via diverse synaptic mechanisms. These mechanisms include GABAergic, ephaptic and pH-mediated feedback (reviewed in Thoreson and Mangel, 2012). In the rd1 retina, HC neurites sprout vertically toward the outer and inner retina (Rossi et al., 2003) and, thus, it is likely that glutamatergic transmission from cones to HCs but also feedback (and feedforward) input from HCs is altered. For example, in the wild-type mouse retina, ionotropic GABA receptors are expressed on HCs (Feigenspan and Weiler, 2004) acting as auto-receptors (Liu et al., 2013) but are not expressed on cones (Kemmler et al., 2014), whereas rd1 cones do express GABAA/C receptors (Pattnaik et al., 2000). Notably, also GABAergic interplexiform cells form synapses in the outer retina (Dedek et al., 2009) and therefore may participate in controlling the activity of neurons in the outer rd1 retina.
As shown above, GABAergic HC feedback (and potentially GABAergic inhibition provided by interplexiform cells) play a role in rd1 outer retina in dampening cone and RBC spontaneous activity. GABA receptor blockers were particularly effective in disinhibiting cones, and likely as a consequence of increased cone input, also RBCs: in both cell types Fmean (Figures 6A,B, Table 2, cf. Figure 5C) and the number of all cluster types increased significantly (Figures 6C,H, Table 3). While the mean number of cells per cluster remained constant (Table 3), the cone/RBC ratio in cone-RBC clusters increased from 1 to 1.4 (Figure 6D), suggesting that HCs primarily modulate cone activity in rd1. The disinhibitory effect of GABA receptor blockers may be mediated either directly by acting on the GABA receptors expressed by cone axon terminals, or additionally, indirectly by inhibiting HCs and reducing ephaptic HC feedback (Liu et al., 2013). Either way, GABAergic transmission results in modulation of cone activity. Additionally, the increase of cone-RBC cluster number in the presence of GABA receptor antagonists argues against a prominent GABAergic input from the inner retina on cluster activity, but of course, cannot exclude a general modulatory effect on RBC activity.
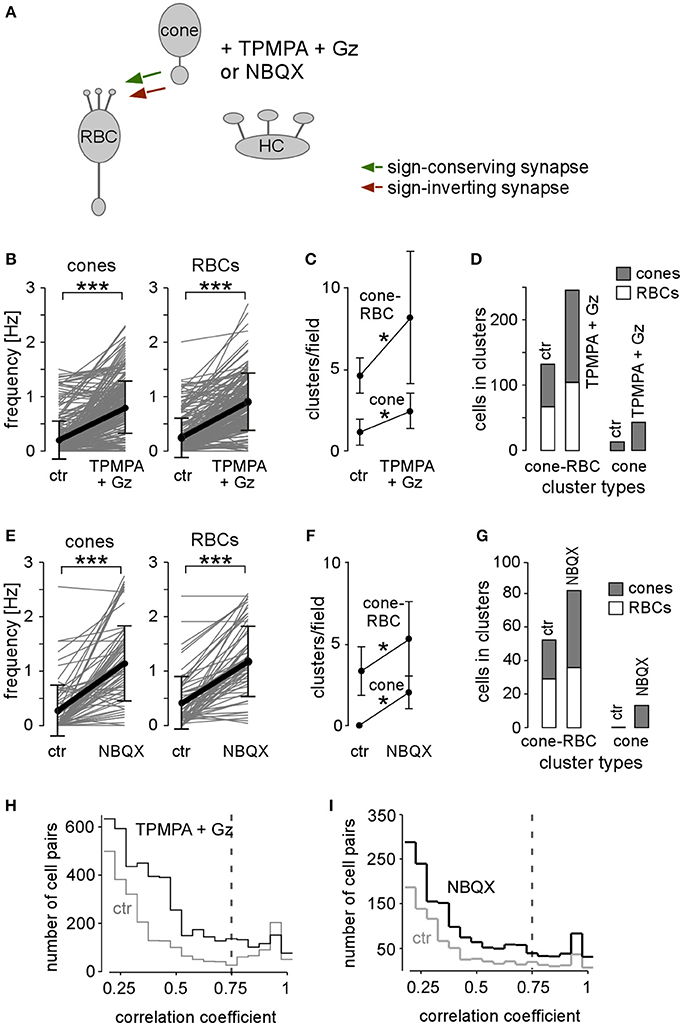
Figure 6. Blocking HCs increases synchronous activity in cones and rod bipolar cells. (A) Drawing shows synaptic interactions of outer retinal neurons with GABA receptor antagonists (TPMPA+Gz) or AMPA/kainate-type GluR antagonist (NBQX). (B–D) Effect of TPMPA+Gz on Fmean (B), cluster number (C) and cluster composition (D). (E–G) Effect of NBQX on Fmean (E), cluster number (F) and cluster composition (G). (H,I) Distribution of correlation coefficients for control (gray lines, ctr) and drug conditions [black lines: TPMPA+Gz (H), NBQX (I)]. Gray dashed line indicates correlation coefficient ≥ 0.75 used for our analysis. All error bars indicate s.e.m.
To “silence” HCs without having to block the different possible feedback pathways to cones, we suppressed their glutamatergic input using AMPA/kainate-type GluR antagonist NBQX. Application of NBQX had similar effects as Gabazine/TPMPA: (i) Some cones and RBCs that were not spontaneously active under control condition started to generate Ca2+ transients and (ii) activity increased in already active cones and RBCs (Figure 6E; Table 2). (iii) New activity clusters formed (Figures 6F,I; Table 3), and (iv) the cluster composition changed, with the cone/RBC ratio in cone-RBC clusters increasing from 0.8 to 1.3 (Figure 6G) while the average number of cells per cone-RBC cluster remained unaltered (Table 3). Thus, depriving HCs from their glutamatergic input resulted in a higher number of active cones and RBCs as well as more clusters. This indicates that spontaneous activity at least in rd1 cones is directly attenuated by HCs, in line with our GABA receptor blocker results.
It is noteworthy that NBQX does not only affect HCs, but also inner retinal activity and, thus, potentially also feedback to the OPL; i.e., via dopamine (Witkovsky, 2004). Nevertheless, we consider it unlikely that such feedback is responsible for the observed increase in cone and RBC activity, because dopamine effects are typically much slower (Witkovsky, 2004). Furthermore, the results of our glutamate puffing experiments (see Figures 4H–K) argue against the presence of ionotropic GluRs on rd1 RBCs—in contrast to what was reported for rd1 ON cone bipolar cells (Chua et al., 2009)—and, thus, against a direct NBQX effect on RBCs.
Horizontal Cells are Functionally Coupled in the rd1 Mouse Retina
In addition to the cone network, also HCs form an electrical network. Fura-2 loaded HCs were easily identified because of their regularly spaced large somata, as confirmed by subsequent immunostaining with antibodies against calbindin (Figures 7A,B). In strong contrast to the fragmented cluster activity of cones and RBCs, all HCs in a recorded field participated in a single activity cluster (Figure 7C). In wild-type mice, HCs form a large electrically coupled “syncytium” using Cx57 (Hombach et al., 2004). To test if the observed activity in the rd1 HC network was synchronized by electrical coupling, we applied CBX and found that this eliminated HC Ca2+ activity almost completely (Figures 7C–E, Tables 2, 3). It is unlikely that the cone input to HCs was abolished by CBX, because selective deletion of coupling between cones (rd1 x Cx36−/− mouse) reduced the number and size of cone-only and cone-RBC clusters but did not significantly affect Fmean in cones and RBCs (see above and Figures 5F–H). We therefore think that loss of HC activity was mainly due to CBX uncoupling the HC network, supporting the notion that HCs in rd1 mice form a functional network that may spread oscillatory activity laterally in the outer retina. However, because such concerted HC activity was infrequently observed (3 out of 51 recorded fields), we did not investigate this aspect of rd1 remodeling in detail.
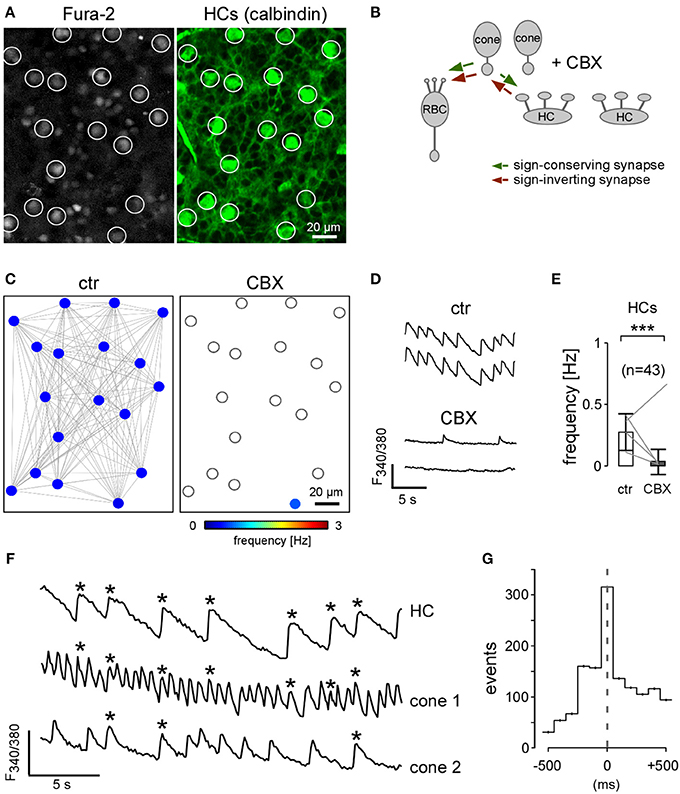
Figure 7. Horizontal cells form a large electrically coupled network in the rd1 retina. (A) Left: Fura-2-labeled outer retinal neurons in P35 rd1 retina (open circles mark HC cell bodies). Right: consecutive immunolabeling of the same region for calbindin confirmed that the cells are HCs. (B) Drawing shows that the gap junction blocker carbenoxolone (CBX) blocks coupling between HCs (and cones). (C) Activity map before (ctr) and during the application of CBX. (D) Example Ca2+ traces of two HCs before and with CBX. (E) CBX reduced Fmean in HCs. All error bars indicate s.e.m. (F) Exemplary Ca2+ traces of two cones and one HC (asterisks indicate synchronous Ca2+ transients of the HC and two neighboring cones). (G) Histogram showing the time-binned distribution of cone Ca2+ transients (n = 121) relative to HC events (n = 43; aligned to 0 ms; n = 3 retinas).
Horizontal Cells and Cones Show Synchronized Activity in the Outer rd1 Retina
To analyze whether HC activity is driven by cone activity or, alternatively, HCs drive cones, we measured Ca2+ transients and determined Fmean of consecutively identified HCs and cones (Figure 7G, Table 2). Typically, Ca2+ transients in HCs (0.26 ± 0.15 Hz) coincided with cone Ca2+ transients (0.53 ± 0.42 Hz), whereas not every cone transient was accompanied by a HC transient (Figure 7F). Quantification of events in the two cell types (time-binned histogram, Figure 7G) revealed that cone Ca2+ transients tended to precede HC events, indicating–within the limited resolution of our imaging system—that cones drive HC activity and not vice versa.
Discussion
As a consequence of rod photoreceptor degeneration, the rd1 mouse retina undergoes an extensive anatomical remodeling (reviewed in Jones and Marc, 2005), leading to spontaneous, light-independent activity in inner retinal neurons, such as ganglion cells (Stasheff, 2008). In the present study, we describe spontaneous activity in outer retinal neurons of the rd1 mouse, and show that cones and RBCs form clusters of synchronized spontaneous activity. This correlated activity is mediated by synaptic contacts and mechanisms that result from remodeling and are atypical when compared to wild-type retina (for summary, see Figure 8): (i) Cones make functional synapses onto RBCs and (ii) signal transmission from cones to RBCs is predominantly mediated by glutamate transporters (likely EAAT5) rather than by mGluR6. (iii) This activity is attenuated by HC feedback, primarily via GABAA/C receptors on cones rather than via a complex mechanism that involves GABA auto-receptors on HCs (Liu et al., 2013).
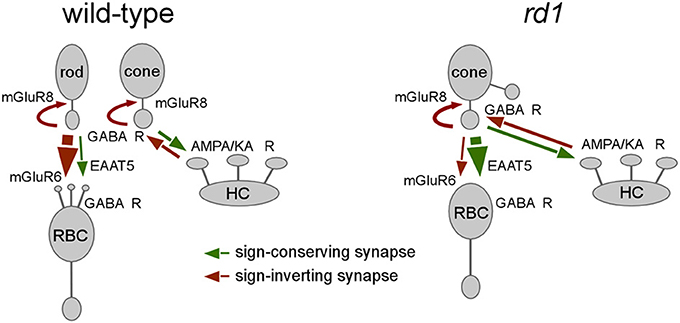
Figure 8. Functional remodeling in the outer rd1 retina. Drawings illustrating the differences in synaptic interactions between wild-type (left) and the synaptically remodeled rd1 (right) outer retina. For simplicity, interactions between HCs and rods/RBCs in wild-type and gap junctions are not shown (sign-conserving synapses, green arrows; sign-inverting synapses, red arrows; EAAT5, excitatory amino acid transporter 5, mGluR; metabotropic glutamate receptors; GABA R, GABA receptors; AMPA/KA R, AMPA/kainate-type glutamate receptors).
Mechanisms Underlying Synchronous Oscillatory Activity in the Outer rd1 Retina
Due to secondary degeneration (Carter-Dawson et al., 1978), the cone mosaic in rd1 retina is severely disturbed, with remnant cones forming small “island-like” aggregations. Despite the grave re-organization of their synapses (e.g., Strettoi and Pignatelli, 2000), rd1 cones express the gap junction-forming Cx36, as reported for wild-type cones (Feigenspan et al., 2004). Instead of a global gap junctionally-coupled network, rd1 cones therefore form multiple local networks. Indeed, with cone coupling genetically ablated, activity was asynchronous, suggesting that cone coupling within clusters plays an important role in synchronizing and possibly enhancing oscillatory activity in the rd1 outer retina. We therefore propose that spontaneous activity in the outer rd1 retina originates in electrically coupled remnant cones—complementary to the inner retina where spontaneous activity originates in the AII/ON-cone bipolar cell network (Borowska et al., 2011; Trenholm et al., 2012).
What is the mechanism underlying intrinsic spontaneous activity in rd1 cones? Our data show that cone activity was abolished by blocking VGCCs, indicating that fluctuations in cone membrane potential are involved. Indeed, wild-type cones were shown to possess regenerative membrane properties that can lead to spiking (reviewed in Baden et al., 2013), as demonstrated for primate cones (Schnapf et al., 1990). In mouse cones, spike-like Ca2+ events were also observed in recordings of vertical retina slices (Wei et al., 2012). Interestingly, while many cones showed signs of active currents (i.e., rebound Ca2+ transients at light-offset; cf. Figure 4 in Wei et al., 2012), only few mouse cones actually displayed spiking activity. It is tempting to compare the situation at the surface of a retinal wild-type slice with the rd1 outer retina, since in both cases stringent control of cone output by HC feedback might be disturbed: in the slice by mechanical damage, and in rd1 as a consequence of remodeling. In the intact retina, ON bipolar cells and HCs contact cone pedicles with invaginating contacts (Boycott and Wässle, 1999; Haverkamp et al., 2000) forming an enclosed, complex multi-synaptic structure. This well-defined structure serves to precisely control glutamate release from cones, and provides the microenvironment necessary for hemichannel-mediated ephaptic HC-cone feedback (reviewed in Kamermans and Fahrenfort, 2004), likely modulated by GABA auto-receptors on HCs (Liu et al., 2013). In rd1, the cone synapse is likely less “encapsulated” due to synaptic re-wiring of cone pedicles with RBCs (Peng et al., 2000), and therefore the feedback is likely less effective, leading to cone depolarization and the observed spontaneous activity, which then propagates within the local cone network. As indicated by Pattnaik et al. (2000), rd1 cones possess GABA receptors—in contrast to the situation in wild-type (Kemmler et al., 2014)—and therefore a GABAergic HC-cone feedback is likely functional but by itself may not be sufficient to prevent spontaneous cone activity. In addition, rd1 cones lose their outer segments and thus their source of light-evoked hyperpolarization. Consequently, the rd1 cone membrane potential may be more depolarized than in intact cones, making it more likely that potential fluctuations reach the VGCCs' activation threshold.
Synchronous Activity in rd1 Cones and Rod Bipolar Cells
One important finding of this study is that rd1 RBCs receive glutamatergic cone input primarily via sign-conserving synapses that involve glutamate transporters (likely EAAT5). Sign-inverting mGluR6, which mediate sign-inverting input from rods to RBCs in the wild-type retina, appears to play a minor role, an observation that is in agreement with a previous study showing that rd1 RBCs are much less sensitive for mGluR6 agonists (Chua et al., 2009). The decrease in mGluR6 on rd1 RBCs may be related to the fact that the mGluR6 complex comprises a plethora of different membrane and cytoplasmic proteins, including the receptor itself, a G protein, nyctalopin and TRPM1 channels (Cao et al., 2011). It is therefore conceivable that mGluR6-dependent transmission is more vulnerable to degeneration-induced imbalances in protein expression level (Hauck et al., 2006)—the down-regulation of proteins such mGluR6 and TRPM1 (Strettoi and Pignatelli, 2000; Krizaj et al., 2010) may be sufficient to inactivate the mGluR6 signaling cascade. In contrast, the here described EAAT-mediated transmission pathway in rd1 retina relies on the expression of a single trans-membrane protein, which is already present on wild-type RBCs (EAAT5, see Wersinger et al., 2006), making it a suitable candidate to dominate transmission in the newly formed, ectopic cone-RBC synapses. It is possible that the remodeled, possibly “leaky” synapse between cones and RBCs promotes an EAAT-mediated pathway, as glutamate released from the cone may diffuse further (“spill-over”) than in the wild-type situation. However, in view of the dendritic/somatic EAAT expression we found in rd1 RBCs, action of glutamate at the distal bipolar cell end is most probable. Also, if glutamate released from cones actually were diffusing to RBC axon terminals, a hyperpolarizing response would be expected, as Cl− concentration is low in the RBC terminals (Billups and Attwell, 2002; Varela et al., 2005), resulting in an EAAT-mediated Cl− influx.
Functional Relevance for Inner Retinal Networks
How does the spontaneous activity in cones and RBCs relate to the oscillations described earlier in the inner rd1 retina? In wild-type retina, RBCs synapse onto AIIs (Bloomfield and Dacheux, 2001) and, thus, RBCs could relay the spontaneous cone activity to inner retinal neurons. While RBC axon terminals are structurally intact and AIIs are functional in rd1 (Borowska et al., 2011), it is still unclear whether the RBC-AII synapse is functionally intact (Barhoum et al., 2008). Because blocking glutamatergic input from bipolar cells (including RBCs) failed to abolish oscillatory activity in the degenerating inner retina, it was proposed that the electrically-coupled AII/ON-cone bipolar cell network by itself is sufficient for initiating the oscillations (Borowska et al., 2011; Yee et al., 2012; Margolis et al., 2014). Additionally, the frequency of oscillatory events in rd1 inner retinal neurons is higher (>10 Hz) than that of the Ca2+ signals in outer retina neurons (<3 Hz) observed in this study. This argues against the outer retina as the sole source of inner retina activity, but it does not exclude a contribution of outer retinal activity, i.e., via RBCs and possibly other bipolar cell types. Taken together, oscillatory activity in the rd1 retina is likely generated independently by electrically coupled networks consisting of cones in the outer and AIIs/ON-cone bipolar cells in the inner retina. An important difference, however, may be that the activity in the outer rd1 retina results from synaptic remodeling, whereas for the inner retina a correlation between aberrant activity and structural changes has not (yet) been described.
Implication of Spontaneous Activity in rd1 Retina on Therapeutic Approaches for Vision Rescue
Spontaneous activity in the retina of blind human patients has not yet been directly recorded. Nevertheless, patients with retinal degenerative disorders, such as Retinitis Pigmentosa or age-related macular degeneration, often report a discomforting “misperception” of bright, light-independent flashes, a phenomenon described as photopsia (Bittner et al., 2009). It is conceivable that the spontaneous activity described in the rd1 mouse also exists in retinal degeneration patients, potentially manifesting as photopsia. Moreover, photopsia poses a severe problem for the development of therapeutic strategies, such as electrical retinal implants (Klauke et al., 2011; Zrenner, 2013) and optogenetic approaches (Lagali et al., 2008). Recently, the implantation of electrical prostheses demonstrated partial restoration of limited vision at advanced stages of photoreceptor degeneration in humans: treated patients were able to distinguish basic geometric forms, read large letters and even discriminate facial expressions. However, some patients reported “blurring” of visual stimuli that exceeded what was to be expected from the spatial and temporal resolution of the implant chip (Zrenner et al., 2011). A possible explanation may be that signal transmission efficiency from the implant to second-order retinal neurons is reduced and degraded by spontaneous activity in the retinal network. For the same reason, optogenetic approaches that aim at rendering remnant photoreceptors or second-order neurons light-sensitive (Lagali et al., 2008), potentially suffer from spontaneous activity in the degenerating retinal network. As reported here, disrupting the electrically coupled retinal networks genetically or pharmacologically largely abolished synchronous oscillations of rd1 outer retinal neurons. Similar effects of gap junction blockers were reported for the spontaneous activity in the inner retina (Borowska et al., 2011; Menzler and Zeck, 2011; Toychiev et al., 2013). Therefore, uncoupling electrical networks in the retina, e.g., by (more selective and, importantly, non-toxic) gap junction blockers, may possess therapeutic potential for treating photopsia.
Conflict of Interest Statement
The authors declare that the research was conducted in the absence of any commercial or financial relationships that could be construed as a potential conflict of interest.
Acknowledgments
We thank G. Eske and S. Bolz for excellent technical assistance, T. Baden, B. Antkowiak, R. Kemmler and F. Paquet-Durand for critical reading of the manuscript, and K. Kranz and K. Dedek for providing transgenic mice. This work was supported by the Deutsche Forschungsgemeinschaft (EXC 307, CIN to Thomas Euler, Timm Schubert) and the German Federal Ministry of Education and Research (ANI—FKZ 16SV3891 to Wadood Haq, Eberhart Zrenner, HOPE2—FKZ 01GM1108A to Blanca Arango-Gonzalez, BCCN—FKZ 01GQ1002 to Wadood Haq, Eberhart Zrenner, Thomas Euler).
References
Arriza, J. L., Eliasof, S., Kavanaugh, M. P., and Amara, S. G. (1997). Excitatory amino acid transporter 5, a retinal glutamate transporter coupled to a chloride conductance. Proc. Natl. Acad. Sci. U.S.A 94, 4155–4160. doi: 10.1073/pnas.94.8.4155
Baden, T., Euler, T., Weckström, M., and Lagnado, L. (2013). Spikes and ribbon synapses in early vision. Trends Neurosci. 36, 480–488. doi: 10.1016/j.tins.2013.04.006
Barhoum, R., Martinez-Navarrete, G., Corrochano, S., Germain, F., Fernandez-Sanchez, L., De La Rosa, E. J., et al. (2008). Functional and structural modifications during retinal degeneration in the rd10 mouse. Neuroscience 155, 698–713. doi: 10.1016/j.neuroscience.2008.06.042
Berrebi, A. S., Oberdick, J., Sangameswaran, L., Christakos, S., Morgan, J. I., and Mugnaini, E. (1991). Cerebellar Purkinje cell markers are expressed in retinal bipolar neurons. J. Comp. Neurol. 308, 630–649. doi: 10.1002/cne.903080409
Billups, D., and Attwell, D. (2002). Control of intracellular chloride concentration and GABA response polarity in rat retinal ON bipolar cells. J. Physiol. 545, 183–198. doi: 10.1113/jphysiol.2002.024877
Bittner, A. K., Diener-West, M., and Dagnelie, G. (2009). A survey of photopsias in self-reported retinitis pigmentosa: location of photopsias is related to disease severity. Retina 29, 1513–1521. doi: 10.1097/IAE.0b013e3181af0d57
Bloomfield, S. A., and Dacheux, R. F. (2001). Rod vision: pathways and processing in the mammalian retina. Prog. Retin. Eye Res. 20, 351–384. doi: 10.1016/S1350-9462(00)00031-8
Borowska, J., Trenholm, S., and Awatramani, G. B. (2011). An intrinsic neural oscillator in the degenerating mouse retina. J. Neurosci. 31, 5000–5012. doi: 10.1523/JNEUROSCI.5800-10.2011
Bowes, C., Li, T., Danciger, M., Baxter, L. C., Applebury, M. L., and Farber, D. B. (1990). Retinal degeneration in the rd mouse is caused by a defect in the beta subunit of rod cGMP-phosphodiesterase. Nature 347, 677–680. doi: 10.1038/347677a0
Boycott, B., and Wässle, H. (1999). Parallel processing in the mammalian retina: the Proctor Lecture. Invest. Ophthalmol. Vis. Sci. 40, 1313–1327.
Busskamp, V., Duebel, J., Balya, D., Fradot, M., Viney, T. J., Siegert, S., et al. (2010). Genetic reactivation of cone photoreceptors restores visual responses in retinitis pigmentosa. Science 329, 413–417. doi: 10.1126/science.1190897
Cao, Y., Posokhova, E., and Martemyanov, K. A. (2011). TRPM1 forms complexes with nyctalopin in vivo and accumulates in postsynaptic compartment of ON-bipolar neurons in mGluR6-dependent manner. J. Neurosci. 31, 11521–11526. doi: 10.1523/JNEUROSCI.1682-11.2011
Carter-Dawson, L. D., Lavail, M. M., and Sidman, R. L. (1978). Differential effect of the rd mutation on rods and cones in the mouse retina. Invest. Ophthalmol. Vis. Sci. 17, 489–498.
Chua, J., Fletcher, E. L., and Kalloniatis, M. (2009). Functional remodeling of glutamate receptors by inner retinal neurons occurs from an early stage of retinal degeneration. J. Comp. Neurol. 514, 473–491. doi: 10.1002/cne.22029
Cuenca, N., Pinilla, I., Sauve, Y., Lu, B., Wang, S., and Lund, R. D. (2004). Regressive and reactive changes in the connectivity patterns of rod and cone pathways of P23H transgenic rat retina. Neuroscience 127, 301–317. doi: 10.1016/j.neuroscience.2004.04.042
Dedek, K., Breuninger, T., de Sevilla Müller, L. P., Maxeiner, S., Schultz, K., Janssen-Bienhold, U., et al. (2009). A novel type of interplexiform amacrine cell in the mouse retina. Eur. J. Neurosci. 30, 217–228. doi: 10.1111/j.1460-9568.2009.06808.x
Fairman, W. A., Vandenberg, R. J., Arriza, J. L., Kavanaugh, M. P., and Amara, S. G. (1995). An excitatory amino-acid transporter with properties of a ligand-gated chloride channel. Nature 375, 599–603. doi: 10.1038/375599a0
Feigenspan, A., Janssen-Bienhold, U., Hormuzdi, S., Monyer, H., Degen, J., Sohl, G., et al. (2004). Expression of connexin36 in cone pedicles and OFF-cone bipolar cells of the mouse retina. J. Neurosci. 24, 3325–3334. doi: 10.1523/JNEUROSCI.5598-03.2004
Feigenspan, A., and Weiler, R. (2004). Electrophysiological properties of mouse horizontal cell GABAA receptors. J. Neurophys. 92, 2789–2801. doi: 10.1152/jn.00284.2004
Garcia-Fernandez, J. M., Jimenez, A. J., and Foster, R. G. (1995). The persistence of cone photoreceptors within the dorsal retina of aged retinally degenerate mice (rd/rd): implications for circadian organization. Neurosci. Lett. 187, 33–36. doi: 10.1016/0304-3940(95)11330-Y
Grant, G. B., and Dowling, J. E. (1995). A glutamate-activated chloride current in cone-driven ON bipolar cells of the white perch retina. J. Neurosci. 15, 3852–3862.
Grynkiewicz, G., Poenie, M., and Tsien, R. Y. (1985). A new generation of Ca2+ indicators with greatly improved fluorescence properties. J. Biol. Chem. 260, 3440–3450.
Güldenagel, M., Ammermüller, J., Feigenspan, A., Teubner, B., Degen, J., Sohl, G., et al. (2001). Visual transmission deficits in mice with targeted disruption of the gap junction gene connexin36. J. Neurosci. 21, 6036–6044.
Hauck, S. M., Ekström, P. A., Ahuja-Jensen, P., Suppmann, S., Paquet-Durand, F., Van Veen, T., et al. (2006). Differential modification of phosducin protein in degenerating rd1 retina is associated with constitutively active Ca2+/calmodulin kinase II in rod outer segments. Mol. Cell. Proteomics 5, 324–336. doi: 10.1074/mcp.M500217-MCP200
Haverkamp, S., Grünert, U., and Wässle, H. (2000). The cone pedicle, a complex synapse in the retina. Neuron 27, 85–95. doi: 10.1016/S0896-6273(00)00011-8
Hombach, S., Janssen-Bienhold, U., Sohl, G., Schubert, T., Bussow, H., Ott, T., et al. (2004). Functional expression of connexin57 in horizontal cells of the mouse retina. Eur. J. Neurosci. 19, 2633–2640. doi: 10.1111/j.0953-816X.2004.03360.x
Jimenez, A. J., Garcia-Fernandez, J. M., Gonzalez, B., and Foster, R. G. (1996). The spatio-temporal pattern of photoreceptor degeneration in the aged rd/rd mouse retina. Cell Tissue Res. 284, 193–202. doi: 10.1007/s004410050579
Jones, B. W., and Marc, R. E. (2005). Retinal remodeling during retinal degeneration. Exp. Eye Res. 81, 123–137. doi: 10.1016/j.exer.2005.03.006
Kamermans, M., and Fahrenfort, I. (2004). Ephaptic interactions within a chemical synapse: hemichannel-mediated ephaptic inhibition in the retina. Curr. Opin. Neurobiol. 14, 531–541. doi: 10.1016/j.conb.2004.08.016
Kemmler, R., Schultz, K., Dedek, K., Euler, T., and Schubert, T. (2014). Differential regulation of cone calcium signals by different horizontal cell feedback mechanisms in the mouse retina. J. Neurosci. 34, 11826–11843. doi: 10.1523/JNEUROSCI.0272-14.2014
Klauke, S., Goertz, M., Rein, S., Hoehl, D., Thomas, U., Eckhorn, R., et al. (2011). Stimulation with a wireless intraocular epiretinal implant elicits visual percepts in blind humans. Invest. Ophthalmol. Vis. Sci. 52, 449–455. doi: 10.1167/iovs.09-4410
Koulen, P., Kuhn, R., Wässle, H., and Brandstätter, J. H. (1999). Modulation of the intracellular calcium concentration in photoreceptor terminals by a presynaptic metabotropic glutamate receptor. Proc. Natl. Acad. Sci. U.S.A. 96, 9909–9914. doi: 10.1073/pnas.96.17.9909
Krizaj, D., Huang, W., Furukawa, T., Punzo, C., and Xing, W. (2010). Plasticity of TRPM1 expression and localization in the wild type and degenerating mouse retina. Vision Res. 50, 2460–2465. doi: 10.1016/j.visres.2010.08.034
Lagali, P. S., Balya, D., Awatramani, G. B., Münch, T. A., Kim, D. S., Busskamp, V., et al. (2008). Light-activated channels targeted to ON bipolar cells restore visual function in retinal degeneration. Nat. Neurosci. 11, 667–675. doi: 10.1038/nn.2117
Lambrecht, H. G., and Koch, K. W. (1992). Recoverin, a novel calcium-binding protein from vertebrate photoreceptors. Biochim. Biophys. Acta 1160, 63–66. doi: 10.1016/0167-4838(92)90038-F
Lin, B., Masland, R. H., and Strettoi, E. (2009). Remodeling of cone photoreceptor cells after rod degeneration in rd mice. Exp. Eye Res. 88, 589–599. doi: 10.1016/j.exer.2008.11.022
Liu, X., Hirano, A. A., Sun, X., Brecha, N. C., and Barnes, S. (2013). Calcium channels in rat horizontal cells regulate feedback inhibition of photoreceptors through an unconventional GABA- and pH-sensitive mechanism. J. Physiol. 591, 3309–3324. doi: 10.1113/jphysiol.2012.248179
Margolis, D. J., Gartland, A. J., Singer, J. H., and Detwiler, P. B. (2014). Network Oscillations Drive correlated spiking of on and off ganglion cells in the rd1 mouse model of retinal degeneration. PLoS ONE 9:e86253. doi: 10.1371/journal.pone.0086253
Margolis, D. J., Newkirk, G., Euler, T., and Detwiler, P. B. (2008). Functional stability of retinal ganglion cells after degeneration-induced changes in synaptic input. J. Neurosci. 28, 6526–6536. doi: 10.1523/JNEUROSCI.1533-08.2008
Menzler, J., and Zeck, G. (2011). Network oscillations in rod-degenerated mouse retinas. J. Neurosci. 31, 2280–2291. doi: 10.1523/JNEUROSCI.4238-10.2011
Morgans, C. W., Zhang, J., Jeffrey, B. G., Nelson, S. M., Burke, N. S., Duvoisin, R. M., et al. (2009). TRPM1 is required for the depolarizing light response in retinal ON-bipolar cells. Proc. Natl. Acad. Sci. U.S.A. 106, 19174–19178. doi: 10.1073/pnas.0908711106
Nachman-Clewner, M., St. Jules, R., and Townes-Anderson, E. (1999). L-type calcium channels in the photoreceptor ribbon synapse: localization and role in plasticity. J. Comp. Neurol. 415, 1–16.
Nakajima, Y., Iwakabe, H., Akazawa, C., Nawa, H., Shigemoto, R., Mizuno, N., et al. (1993). Molecular characterization of a novel retinal metabotropic glutamate receptor mGluR6 with a high agonist selectivity for L-2-amino-4-phosphonobutyrate. J. Biol. Chem. 268, 11868–11873.
Pang, J. J., Gao, F., Lem, J., Bramblett, D. E., Paul, D. L., and Wu, S. M. (2010). Direct rod input to cone BCs and direct cone input to rod BCs challenge the traditional view of mammalian BC circuitry. Proc. Natl. Acad. Sci. U.S.A. 107, 395–400. doi: 10.1073/pnas.0907178107
Pasteels, B., Rogers, J., Blachier, F., and Pochet, R. (1990). Calbindin and calretinin localization in retina from different species. Vis. Neurosci. 5, 1–16. doi: 10.1017/S0952523800000031
Pattnaik, B., Jellali, A., Sahel, J., Dreyfus, H., and Picaud, S. (2000). GABAC receptors are localized with microtubule-associated protein 1B in mammalian cone photoreceptors. J. Neurosci. 20, 6789–6796.
Peng, Y. W., Hao, Y., Petters, R. M., and Wong, F. (2000). Ectopic synaptogenesis in the mammalian retina caused by rod photoreceptor-specific mutations. Nat. Neurosci. 3, 1121–1127. doi: 10.1038/80639
Picaud, S., Larsson, H. P., Wellis, D. P., Lecar, H., and Werblin, F. (1995). Cone photoreceptors respond to their own glutamate release in the tiger salamander. Proc. Natl. Acad. Sci. U.S.A. 92, 9417–9421. doi: 10.1073/pnas.92.20.9417
Protti, D. A., and Llano, I. (1998). Calcium currents and calcium signaling in rod bipolar cells of rat retinal slices. J. Neurosci. 18, 3715–3724.
Rossi, C., Strettoi, E., and Galli-Resta, L. (2003). The spatial order of horizontal cells is not affected by massive alterations in the organization of other retinal cells. J. Neurosci. 23, 9924–9928.
Schnapf, J. L., Nunn, B. J., Meister, M., and Baylor, D. A. (1990). Visual transduction in cones of the monkey Macaca fascicularis. J. Physiol. 427, 681–713.
Schubert, T., Weiler, R., and Feigenspan, A. (2006). Intracellular calcium is regulated by different pathways in horizontal cells of the mouse retina. J. Neurophysiol. 96, 1278–1292. doi: 10.1152/jn.00191.2006
Stasheff, S. F. (2008). Emergence of sustained spontaneous hyperactivity and temporary preservation of OFF responses in ganglion cells of the retinal degeneration (rd1) mouse. J. Neurophysiol. 99, 1408–1421. doi: 10.1152/jn.00144.2007
Strettoi, E., and Pignatelli, V. (2000). Modifications of retinal neurons in a mouse model of retinitis pigmentosa. Proc. Natl. Acad. Sci. U.S.A. 97, 11020–11025. doi: 10.1073/pnas.190291097
Ströh, S., Sonntag, S., Janssen-Bienhold, U., Schultz, K., Cimiotti, K., Weiler, R., et al. (2013). Cell-specific cre recombinase expression allows selective ablation of glutamate receptors from mouse horizontal cells. PLoS ONE 8:e83076. doi: 10.1371/journal.pone.0083076
Thoreson, W. B., and Mangel, S. C. (2012). Lateral interactions in the outer retina. Prog. Retin. Eye Res. 31, 407–441. doi: 10.1016/j.preteyeres.2012.04.003
Toychiev, A. H., Ivanova, E., Yee, C. W., and Sagdullaev, B. T. (2013). Block of gap junctions eliminates aberrant activity and restores light responses during retinal degeneration. J. Neurosci. 33, 13972–13977. doi: 10.1523/JNEUROSCI.2399-13.2013
Trenholm, S., Borowska, J., Zhang, J., Hoggarth, A., Johnson, K., Barnes, S., et al. (2012). Intrinsic oscillatory activity arising within the electrically coupled AII amacrine-ON cone bipolar cell network is driven by voltage-gated Na+ channels. J. Physiol. 590, 2501–2517. doi: 10.1113/jphysiol.2011.225060
Varela, C., Blanco, R., and De La Villa, P. (2005). Depolarizing effect of GABA in rod bipolar cells of the mouse retina. Vision Res. 45, 2659–2667. doi: 10.1016/j.visres.2005.03.020
Vessey, J. P., Lalonde, M. R., Mizan, H. A., Welch, N. C., Kelly, M. E., and Barnes, S. (2004). Carbenoxolone inhibition of voltage-gated Ca channels and synaptic transmission in the retina. J. Neurophysiol. 92, 1252–1256. doi: 10.1152/jn.00148.2004
Wei, T., Schubert, T., Paquet-Durand, F., Tanimoto, N., Chang, L., Koeppen, K., et al. (2012). Light-driven calcium signals in mouse cone photoreceptors. J. Neurosci. 32, 6981–6994. doi: 10.1523/JNEUROSCI.6432-11.2012
Wersinger, E., Schwab, Y., Sahel, J. A., Rendon, A., Pow, D. V., Picaud, S., et al. (2006). The glutamate transporter EAAT5 works as a presynaptic receptor in mouse rod bipolar cells. J. Physiol. 577, 221–234. doi: 10.1113/jphysiol.2006.118281
Witkovsky, P. (2004). Dopamine and retinal function. Doc. Ophthalmol. 108, 17–40. doi: 10.1023/B:DOOP.0000019487.88486.0a
Ye, J. H., and Goo, Y. S. (2007). The slow wave component of retinal activity in rd/rd mice recorded with a multi-electrode array. Physiol. Meas. 28, 1079–1088. doi: 10.1088/0967-3334/28/9/009
Yee, C. W., Toychiev, A. H., and Sagdullaev, B. T. (2012). Network deficiency exacerbates impairment in a mouse model of retinal degeneration. Front. Syst. Neurosci. 6:8. doi: 10.3389/fnsys.2012.00008
Zrenner, E. (2013). Fighting blindness with microelectronics. Sci. Transl. Med. 5, 210ps216. doi: 10.1126/scitranslmed.3007399
Keywords: degeneration, synapse remodeling, photopsia, retina, glutamate transporter
Citation: Haq W, Arango-Gonzalez B, Zrenner E, Euler T and Schubert T (2014) Synaptic remodeling generates synchronous oscillations in the degenerated outer mouse retina. Front. Neural Circuits 8:108. doi: 10.3389/fncir.2014.00108
Received: 15 July 2014; Accepted: 19 August 2014;
Published online: 05 September 2014.
Edited by:
Rachel O. Wong, University of Washington, USAReviewed by:
David J. Margolis, Rutgers University, USADaniel Kerschensteiner, Washington University in St. Louis, USA
Copyright © 2014 Haq, Arango-Gonzalez, Zrenner, Euler and Schubert. This is an open-access article distributed under the terms of the Creative Commons Attribution License (CC BY). The use, distribution or reproduction in other forums is permitted, provided the original author(s) or licensor are credited and that the original publication in this journal is cited, in accordance with accepted academic practice. No use, distribution or reproduction is permitted which does not comply with these terms.
*Correspondence: Thomas Euler and Timm Schubert, Werner Reichardt Centre for Integrative Neuroscience (CIN), Institute for Ophthalmic Research, University of Tübingen, Otfried-Müller-Str. 25, 72076 Tübingen, Germany e-mail:dGltbS5zY2h1YmVydEBjaW4udW5pLXR1ZWJpbmdlbi5kZQ==;dGhvbWFzLmV1bGVyQGNpbi51bmktdHVlYmluZ2VuLmRl