- 1Institute of Physiology and Pathophysiology, University of Heidelberg, Heidelberg, Germany
- 2Mediterranean Institute of Neurobiology (INMED), INSERM, Marseille, France
Memory formation is associated with the generation of transiently stable neuronal assemblies. In hippocampal networks, such groups of functionally coupled neurons express highly ordered spatiotemporal activity patterns which are coordinated by local network oscillations. One of these patterns, sharp wave-ripple complexes (SPW-R), repetitively activates previously established groups of memory-encoding neurons, thereby supporting memory consolidation. This function implies that repetition of specific SPW-R induces plastic changes which render the underlying neuronal assemblies more stable. We modeled this repetitive activation in an in vitro model of SPW-R in mouse hippocampal slices. Weak electrical stimulation upstream of the CA3-CA1 networks reliably induced SPW-R of stereotypic waveform, thus representing re-activation of similar neuronal activity patterns. Frequent repetition of these patterns (100 times) reduced the variance of both, evoked and spontaneous SPW-R waveforms, indicating stabilization of pre-existing assemblies. These effects were most pronounced in the CA1 subfield and depended on the timing of stimulation relative to spontaneous SPW-R. Additionally, plasticity of SPW-R was blocked by application of a NMDA receptor antagonist, suggesting a role for associative synaptic plasticity in this process. Thus, repetitive activation of specific patterns of SPW-R causes stabilization of memory-related networks.
Introduction
The mammalian hippocampus supports the formation and consolidation of spatial, episodic and declarative memories. At the network level, neuronal activity is organized in coherent spatio-temporal patterns which correlate with distinct processes in memory formation (Sirota and Buzsáki, 2005). Recording of multiple single units in freely moving rodents has revealed that hippocampal network oscillations go along with precisely timed action potentials of specific neurons which fire in a place-specific manner. Sequential activation of such place cells during spatial exploration forms a representation of the animal’s trajectory (O’Keefe and Recce, 1993). In subsequent phases of slow-wave sleep, the same sequences are repetitively activated, probably mediating memory consolidation (Wilson and McNaughton, 1994; Lee and Wilson, 2002). In this state, sequential activation of place cells occurs on top of sharp wave-ripple complexes (SPW-R; Ylinen et al., 1995; Csicsvari et al., 1999). It has been suggested that this re-activation of neuronal activity sequences mediates memory consolidation by transferring transiently stable activity into the neocortex for long-term storage (Buzsáki, 1989). In line with this concept, memory consolidation is associated with irregular oscillatory activity containing SPW-R (Girardeau et al., 2009; Mölle and Born, 2011; Sadowski et al., 2011).
Work during the past decades has revealed much insight into the cellular mechanisms underlying memory formation, including Hebbian plasticity of synapses in major hippocampal projection pathways (Bliss and Lomo, 1973; Köhr et al., 2003; Buschler et al., 2012; Goh and Manahan-Vaughan, 2013). Oscillation-associated assembly formation constitutes a potential link between synaptic plasticity and network-level information processing. Indeed, theta-gamma states support synaptic plasticity (Palmer et al., 2004; Lisman et al., 2005) and may thus allow for transient assembly formation. In accordance, in vitro data show that high frequency stimulation can induce spontaneous SPW-R oscillations in rat hippocampal slices (Behrens et al., 2005). Additionally, this induction is NMDA-R activation dependent and indicates an essential role of synaptic strengthening in hippocampal assembly formation. Interestingly, SPW-Rs also favor associative strengthening of synapses (King et al., 1999), and their selective disruption impairs spatial memory formation (Girardeau et al., 2009). Together, these processes may account for the rapid formation and subsequent consolidation of space-encoding assemblies in hippocampal networks (Frank et al., 2003; Carr et al., 2012). It is, thus, likely that repeated activation of assemblies during SPW-R strengthens connectivity between participating neurons, resulting in transiently stable, highly specific activity patterns (Buzsáki and Draguhn, 2004). While we know much about plasticity of synapses, the activity-dependent formation of neuronal assemblies within the different hippocampal subfields remains, however, poorly understood.
In previous work we showed that different neuronal assemblies are reflected in different waveforms of spontaneously occurring SPW-R (Reichinnek et al., 2010). Therefore, extracellular recordings of SPW-R (Maier et al., 2003) and subsequent waveform sorting can be taken as a proxy of monitoring distinct neuronal assemblies. Here, we tested the effect of repetitive activation of such neuronal groups on the diversity and stability of spontaneous network activity patterns.
Materials and Methods
All animal procedures were performed in accordance with the guidelines of the European Community Council and approved by the state government of Baden-Württemberg.
Experimental Outline
Slice Preparation
Experiments were performed on slices of four to 8 weeks old male mice (C57Bl6). Animals were decapitated after being deeply anesthetized with CO2. Subsequently, the brain was separated and transferred into cooled (0–4°C) artificial cerebral spinal fluid (ACSF: NaCl 124 mM, KCl 3.0 mM, MgSO4 1.8 mM, CaCl2 1.6 mM, Glucose 10 mM, NaH2PO4 1.25 mM, NaHCO3 26 mM), saturated with 95% O2 and 5% CO2 (corresponding to pH 7.4 at 37°C). Before slicing, frontal lobe and cerebellum were removed, and the remaining tissue block was glued to the holding chamber of a Leica Vibratome (VT1000S). Horizontal slices of 450 μm thickness were cut and subsequently transferred to a Haas-type interface recording chamber where they recovered for at least 2 h at 32–34°C at the border between ACSF (flow: 1.5–2.0 ml/min) and humidified gas (95% O2 and 5% CO2).
Recording and Stimulation
Extracellular field potentials were recorded in stratum pyramidale of CA3a, CA3b, and CA1 by use of three tetrodes, each consisting out of four twisted wires (Tungsten California Fine Wire, 12.5 μm) connected to an EXT-T2 amplifier (npi electronics, Tamm, Germany). Signals were amplified (×200), low pass filtered at 8 kHz and digitized at 20 kHz for offline analysis (CED Power 1401 mkII expanded by a CED 2805SA-8 Analog BCN box, recording program Spike 2.0, CED, Cambridge, UK).
Two stimulation electrodes (“Micro Probes” Platinum/Iridium, 100 kΩ at 1 kHz, 75 μm tip separation) were placed into the granule cell layer of the supra-pyramidal blade of area dentata. Electrode positions and stimulation strengths were optimized such that unipolar stimulation pulses evoked field events in CA3 and CA1 which resembled waveforms of spontaneously occurring SPW-R (Figures 1A,B). In order to avoid direct interference with ongoing network activity, pulses were triggered at a delay of 150 ms after a preceding spontaneous SPW-R waveform. Alternating stimulation of both electrodes (at intervals of 60 s) caused two different waveform sets of evoked SPW-R at recording sites in CA3a, CA3b and CA1. After 20 stimulations performed at each site, we applied a series of 100 repetitive stimulations at 10 s intervals to one of the two stimulation electrodes, followed again by 20 alternating stimulations at both sites. The first group of control slices underwent the baseline stimulation protocol (alternating stimulation at both sites at intervals of 60 s) but not the repetitive stimulation at 0.1 Hz (1000 s pause at the respective time). Since there was no repetitively stimulated and not repetitively stimulated pattern in this condition we subsequently recorded two evoked waveform data sets in each slice. In a second control condition the 100 repetitive stimulations were triggered without delay on a spontaneously occurring SPW-R while baseline stimulations were still performed with a delay of 150 ms. In a third control the original experiment was repeated in the presence of the NMDA-R antagonist APV (30 μM).
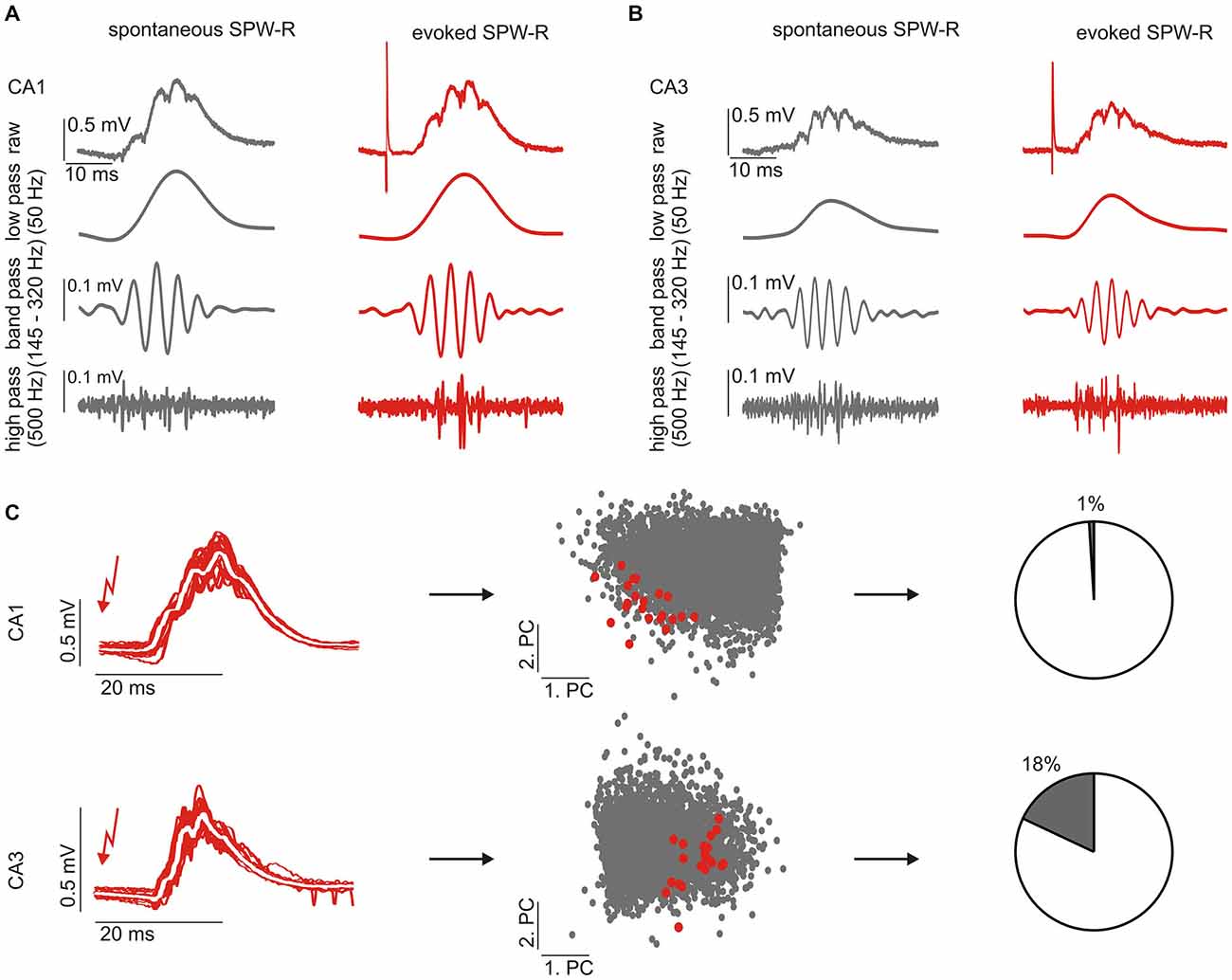
Figure 1. Electrically evoked sharp wave-ripple complexes (SPW-R) closely resemble spontaneous events. (A,B) Example traces of spontaneous (left) and evoked (right) SPW-R in CA1 (A) and CA3 (B), respectively. Evoked SPW-R show the same features like spontaneous SPW-R in different frequency bands corresponding to the sharp wave (low pass), ripple oscillations (band pass) and multi-unit activity (high pass). (C) Individual SPW-R waveforms (left) were analyzed by principal component analysis (PCA) and their similarity was quantified in the first 10 dimensions of the principal component (PC) space (middle, only two PCs are shown). Red dots indicate individual evoked SPW-R shown on the left panel, gray dots indicate spontaneous SPW-R. A smaller inter-group pair-wise average distance indicates more similar waveforms. To compare evoked and spontaneous events we quantified the overlap of events (right, see Methods).
Data Analysis
Analysis of Field Potentials
Raw data were analyzed with custom-made routines written in Matlab. To extract SPW-R events, data were low-pass filtered (50 Hz) and local maxima with amplitudes above 0.15 mV were detected within 30 ms time windows. This threshold corresponds to ~4 standard deviations of event-free baseline noise (Both et al., 2008) and allows for reliable detection of SPW-R. Identified SPW-Rs were analyzed within a time interval starting 33 ms before and ending 67 ms after the peak of the sharp wave. Waveforms of evoked SPW-R-like events were processed similarly to spontaneous SPW-R after removal of the stimulus artifact from raw data traces. Extracellular multi-unit activity was detected by high-pass filtering at 500 Hz and setting a negative threshold at 4.5 SDs from background noise.
The stability of the extracellular signal was controlled by monitoring amplitude and frequency of spontaneous SPW-R during baseline recording. These parameters were regarded stable when rejecting the null hypothesis of finding a change at 5% significance level according to the Wald-Wolfowitz test. In some slices stimulation failed to evoke a downstream response pattern at one site during the course of the experiment. We suspected this to be a local effect since stimulation at the other site and spontaneous SPW-Rs were not affected. Subsequently we excluded experiments with less than ten evoked waveforms to ensure sufficient numbers of evoked events.
Waveform Analysis
For analysis of SPW-R waveforms, signals were down-sampled to 5 kHz, resulting in 500 sample points within a 100 ms window. This data set was further reduced by principal component analysis (PCA; Nicolelis et al., 1995; Lopes-dos-Santos et al., 2013). In accordance with our previous work (Reichinnek et al., 2010) we used the first 10 principal components to describe events within a 10 dimensional feature space. The first PC mainly reflected waveform amplitude and was divided by two in order to reduce the relative contribution of this parameter.
Similarity or difference of waveforms was analyzed by calculating the pairwise Euclidian distance between waveforms within the 10-dimensional principal component space. Subsequently, we calculated the pair-wise Euclidian distance between all spontaneous as well as evoked events before and after repetitive stimulation. This resulted in distance distributions for evoked and spontaneous events, respectively. To compare changes in the variety of waveforms, we divided the median distance after the repetitive stimulation by the median distance before the repetitive stimulation.
In a different approach, we measured the overlap between the repetitive evoked waveform distribution and the evoked SPW-Rs from the site without repetitive stimulation. To quantify this overlap we calculated the distances between the group of evoked but not repetitively stimulated events and the distribution of the group of evoked and repetitively stimulated events. In this case, we used the Mahalanobis distance which is a multi-dimensional way of measuring how many standard deviations a specific event is distant from the center of a distribution. Events from the group of evoked but not repetitively stimulated events were considered similar to the repetitive stimulated SPW-R waveform distribution if the Mahalanobis distance was smaller than the first percentile of the Mahalanobis distances within the distribution of the group of repetitively stimulated events itself. Subsequently, the overlap between both groups of evoked events was defined as the percentage of events from the group of evoked events that were not repetitively stimulated but were within the distribution of evoked events that underwent repetitive stimulation. In analogy the overlap between spontaneous SPW-R and the repetitive stimulated waveform pattern was calculated.
Statistics
Quantitative results are given as median, the 25th and the 75th percentiles. Whiskers in the box plots correspond to the 2.5th and 97.5th percentiles. For statistical analysis we tested whether data were normally distributed by Kolmogorov-Smirnoff-Test (significance level > 0.05). Paired normally distributed data were analyzed by paired t-test, whereas non-paired data were tested by two sided t-test. Non-normally distributed data were compared by Wilcoxon signed-rank test and Wilcoxon rank sum test, respectively. In all cases, p < 0.05 was regarded as significant.
Results
Weak Stimulation of the Dentate Gyrus Elicits Physiological SPW-R Waveforms
Compound potentials similar to spontaneous SPW-R can be evoked by weak electrical stimulation in CA3 (Reichinnek et al., 2010). In order to evoke such events in both hippocampal subfields, CA3 and CA1, we stimulated the upstream network of the DG which is powerfully connected to CA3 pyramidal cells and inhibitory neurons (Acsády et al., 1998). To avoid interference with the ongoing network activity stimuli were applied at 150 ms after the occurrence of a spontaneous SPW-R event. Under these conditions, weak electrical stimulation within the supra-pyramidal blade of the granule cell layer (100 μs, 0.5–5 V) reliably evoked field potential transients in the pyramidal cell layers of CA3 (n = 12 slices) and CA1 (n = 10 slices), respectively. These transient potentials resembled spontaneous sharp wave-ripple events and had characteristic, rather stereotypic waveforms (Figures 1A,B). Although we observed a lower number of unit-events on evoked waveforms overall quantitative analysis further confirmed the similarity between evoked field events and spontaneous SPW-R (Table 1). Moreover, it should be noted that spontaneously occurring SPW-R show heterogeneous levels of multi-unit activity not reflected by the median value across slices.
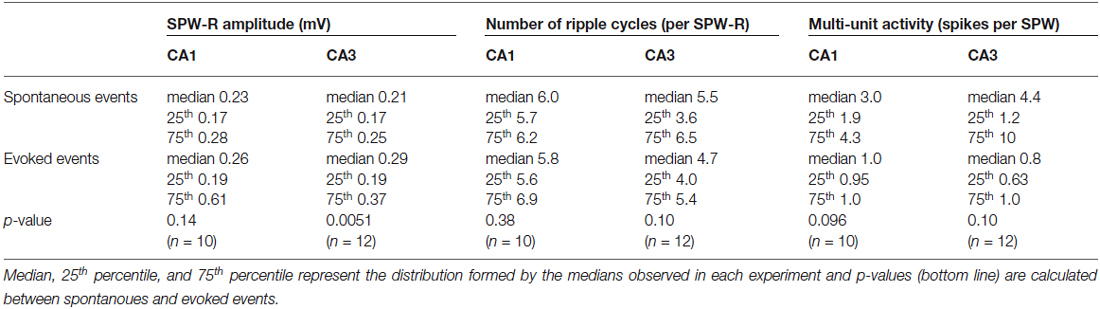
Table 1. Spontanoues (top) and evoked (bottom) waveforms are compared regarding amplitude (left), ripple occurrence (middle), and unit occurrence (right) in CA1 and CA3.
For further comparison of spontaneous and evoked waveform patterns we described the events by a reduced set of parameters following principal component analysis (PCA, see Methods). The principal components describing evoked events in both hippocampal subfields (CA1 and CA3) were reliably located within the parameter space covered by spontaneous events (Figure 1C), which underlines the similarity of both patterns. In summary, we conclude that stimulation-evoked network events in CA3 and CA1 are very similar to spontaneous ongoing spontaneous SPW-R activity and are therefore referred to as “evoked SPW-R”.
Evoked SPW-R Waveforms are Stabilized by Repetitive Stimulation
Memory consolidation has been suggested to depend on the repetitive re-play of hippocampal activity patterns during SPW-R (Wilson and McNaughton, 1994; Lee and Wilson, 2002; Girardeau et al., 2009). We therefore asked for the effect of repetitive activation of similar sets of neurons through electrical stimulation. Twenty highly stereotypic SPW-R waveforms were evoked every 60 s alternating at two stimulation sites within the DG. Subsequently, one site was selected and 100 repetitive pulses were performed at 10 s intervals followed by 20 more stimulations every 60 s at both sites (Figure 2A). Stability of evoked waveform sets prior and after the 100 repetitive stimulation pulses was analyzed within the 10-dimensional PCA parameter space by calculating the pair-wise Euclidian distance between individual SPW-R.
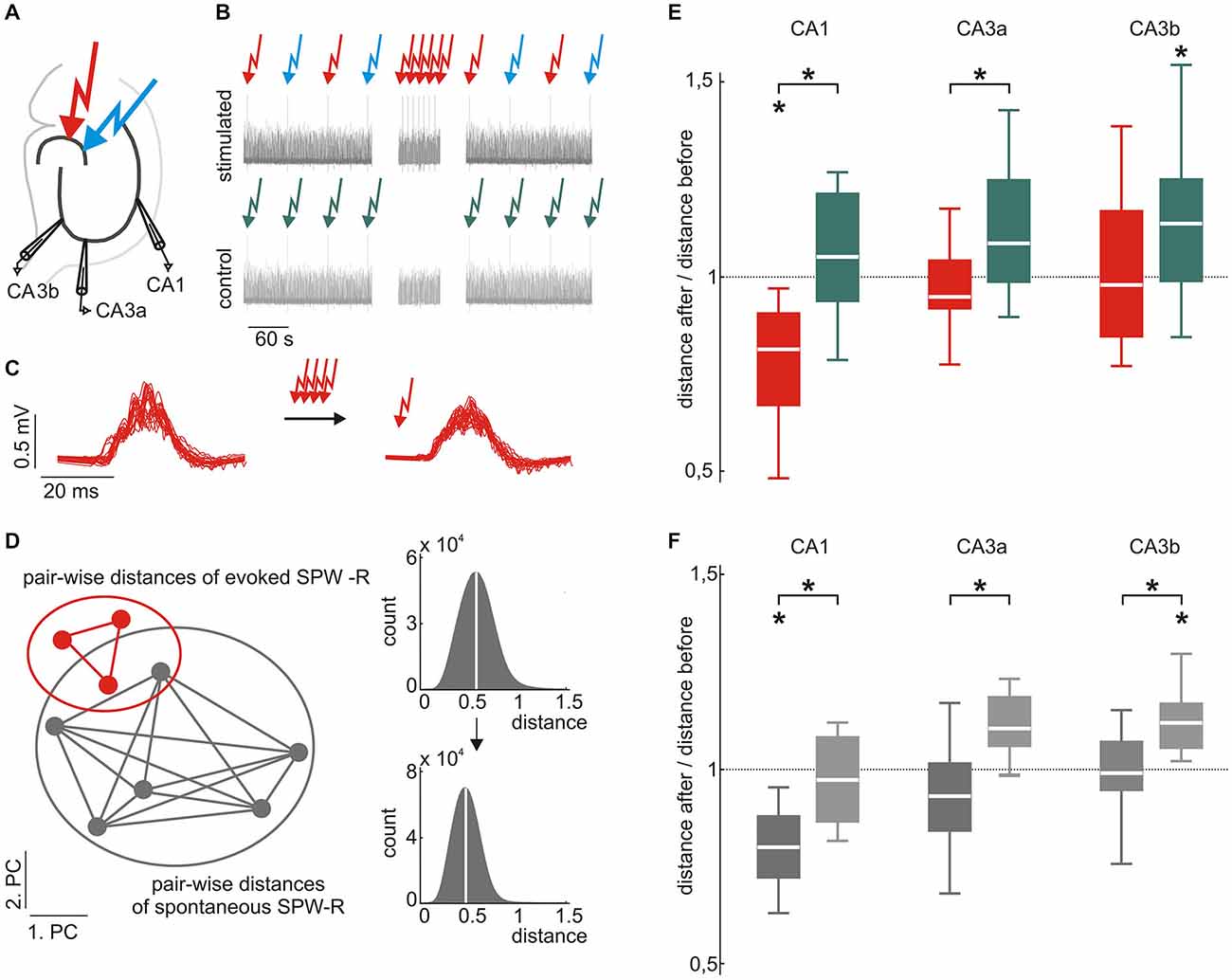
Figure 2. Evoked and spontaneous SPW-R waveforms are stabilized by low frequency stimulation. (A) Scheme of the electrode positions. Recording electrodes were located in CA3a, CA3b, and CA1. Two different locations in the dentate gyrus (DG) were electrically stimulated to evoke SPW-R complexes. (B) Scheme of the stimulation paradigm (top) and the control (bottom). Red and blue arrows indicate electrical stimulation at two different locations in the DG. Low-frequency repetitive stimulation (100 stimuli every 10 s, middle part) was only performed at one of the locations. Under control conditions, stimulation was paused instead for 1000 s. (C) Example of evoked SPW-R before (left) and after (right) repetitive stimulation. (D) Homogeneity of evoked and spontaneous SPW-R was quantified by the median intra-group pair-wise Euclidian distance in the 10-dimensional PC space. Smaller median distances indicate more similar waveforms. (E) Evoked SPW-R waveforms in CA1 became more similar after repetitive stimulation (red; median 0.81, 25th 0.66, 75th 0.90, p < 0.05, n = 8), whereas events under control conditions did not (green; median 1.05, 25th 0.93, 75th 1.21, n = 11 data sets from 7 slices), which resulted in a significant group difference (p < 0.01). Similarly, in CA3a we found a significant difference between both conditions (p < 0.05, repetitive stimulation: median 0.95, 25th 0.91, 75th 1.04, n = 8; control: median 1.08, 25th 0.98, 75th 1.25, n = 11 data sets from 6 slices). In CA3b repetitive stimulation (median 0.97, 25th 0.84, 75th 1.17, n = 12) prevented an increase of variability that was observed under control conditions (median 1.14, 25th 0.98, 75th 1.25, p < 0.05, n = 17 data sets from 9 slices). (F) Spontaneous events also showed a reduced variability after repetitive stimulation of evoked events as compared to control conditions (p < 0.05 for all subfields). Additionally, spontaneous events in CA1 became significantly less variable after repetitive stimulation (repetitive stimulation: median 0.80, 25th 0.72, 75th 0.88, p < 0.01, n = 10; control: median 0.97, 25th 0.86, 75th 1.08). Moreover, spontaneous events in CA3 became more diverse under control conditions (CA3a: median 1.10, 25th 1.06, 75th 1.18, p = 0.075, n = 6; CA3b: median 1.12, 25th 1.06, 75th 1.17, p < 0.01, n = 9), which was inhibited by 100 repetitive stimulations (CA3a: median 0.93, 25th 0.84, 75th 1.02; CA3b: median 0.99, 25th 0.95, 75th 1.07). “*” means that the finding is significant (p < 0.05).
In CA1, median pair-wise distances of evoked SPW-R were consistently reduced following the 100 repetitive stimulations. This was quantified by the ratio of median distances after/before stimulation which was 0.81 (p = 0.029, n = 8; Figure 2D). In contrast, slices that did not undergo the 100 repetitive stimulations (1000 s stimulation pause respectively) showed no reduction in waveform variability (ratio of median distances late/early recording period 1.05, p = 0.25, n = 11 data sets from 7 slices). The difference between both conditions was significant (p = 0.0023) indicating that repetitive activation mediates stabilization of evoked SPW-R waveform patterns in CA1. Within CA3, SPW-Rs were recorded at two different sites (CA3a and CA3b). Under control conditions (1000 s stimulation pause), waveforms in CA3a showed a trend towards increased diversity over time (median distances late/early recording period 1.08, p = 0.051, n = 11 data sets from 6 slices), which reached significance in CA3b (median 1.14, p = 0.016, n = 17 data sets from 9 slices). The increase in variance in both CA3 regions was prevented by 100 repetitive stimulations within the DG, which is similar to the results obtained in CA1. In stimulated slices, median distance after/before repetitive stimulations was 0.95 (p = 0.72, n = 8) in CA3a and in CA3b, respective value was 0.97 (p = 0.55, n = 12). When compared to un-stimulated slices, the reduction of variability was significant for CA3a (p = 0.045) while the ratio of waveform distances in CA3b did not reach significance (p = 0.10).
As stated above, baseline stimulation was alternating between two different sites within the supra-pyramidal blade of the DG. Repetitive stimulation at 0.1 Hz (100 pulses) was, however, restricted to one of the two locations. Interestingly, SPW-R waveforms in CA1 elicited from the other site (which was not repetitively stimulated) did also show reduced variance following the 100 repetitive stimulations at the other site. This was reflected by the ratio of the median distances after/before stimulation of 0.66 (25th 0.64, 75th 0.75, p = 0.0039, n = 7, p = 0.00037 in comparison to the “un-stimulated” control condition). In CA3, similar to the site which was repetitively stimulated, evoked events at the site which was not repetitively stimulated did not show any change in variability (CA3a ratio after/before median 1.01, 25th 0.92, 75th 1.31, p = 0.67, n = 8, p = 0.71 in comparison to “un-stimulated” control condition; CA3b, ratio after/before median 1.01, 25th 0.84, 75th 1.09, p = 0.76, n = 8, p = 0.051 in comparison to the “un-stimulated” control condition).
Spontaneous Events Mimic Evoked Events and Reduce Their Variability
Surprisingly, reduction of waveform variability after 100 repetitive stimulations was also observed for spontaneous SPW-R in CA1 and CA3. Median Euclidian distances of spontaneously occurring events in CA1 were significantly smaller in slices that had undergone 100 repetitive stimulation pulses as compared to “un-stimulated” slices (p = 0.0044). Accordingly, the ratio of distances after/before stimulation was 0.80 (p = 0.0035, n = 10) in the repetitively stimulated slices. In “un-stimulated” control condition this value was 0.97 (p = 0.64, n = 7). In CA3, variability of spontaneous SPW-R increased under control conditions, similar to the evoked events (CA3a: median 1.10, p = 0.075, n = 6; CA3b: median 1.12, p = 0.0054, n = 9). Again, this progressive increase in variability was reduced by 100 intermittent repetitive stimulations (CA3a: median 0.93, p = 0.24, n = 10; CA3b: median 0.99, p = 0.82, n = 12). The differences in variability ratios between the repetitively stimulated condition and the “un-stimulated” control were significant in CA3a (p = 0.015) and CA3b (p = 0.0069), respectively.
Overall, these results indicate that repetitive activation of limited neuronal subsets in the hippocampal formation reduces variance of subsequent network patterns, indicating activity-dependent stabilization of hippocampal assemblies.
Stimulation-Evoked Waveform Sets Increase Similarity
Since evoked waveform sets from both stimulation sites became more homogeneous despite the fact that only one location was repetitively stimulated 100 times (see Figure 2), we analyzed the distinctiveness of both patterns. To do this, we calculated the Mahalanobis distance between both groups of evoked events within the principal component space (see Methods). In CA1 we found an initial overlap of 60%, which significantly increased to 94% (p = 0.000095, n = 5) by the 100 repetitive stimulations (see Figure 3). In contrast, in CA3 the overlap between both evoked patterns did not change significantly. In CA3a the initial overlap was 51% before, and 59% after the 100 stimulations (p = 0.71, n = 6). In CA3b we observed an overlap of 81% prior to and 80% after the repetitive stimulation (p = 0.80, n = 8, Figure 3C).
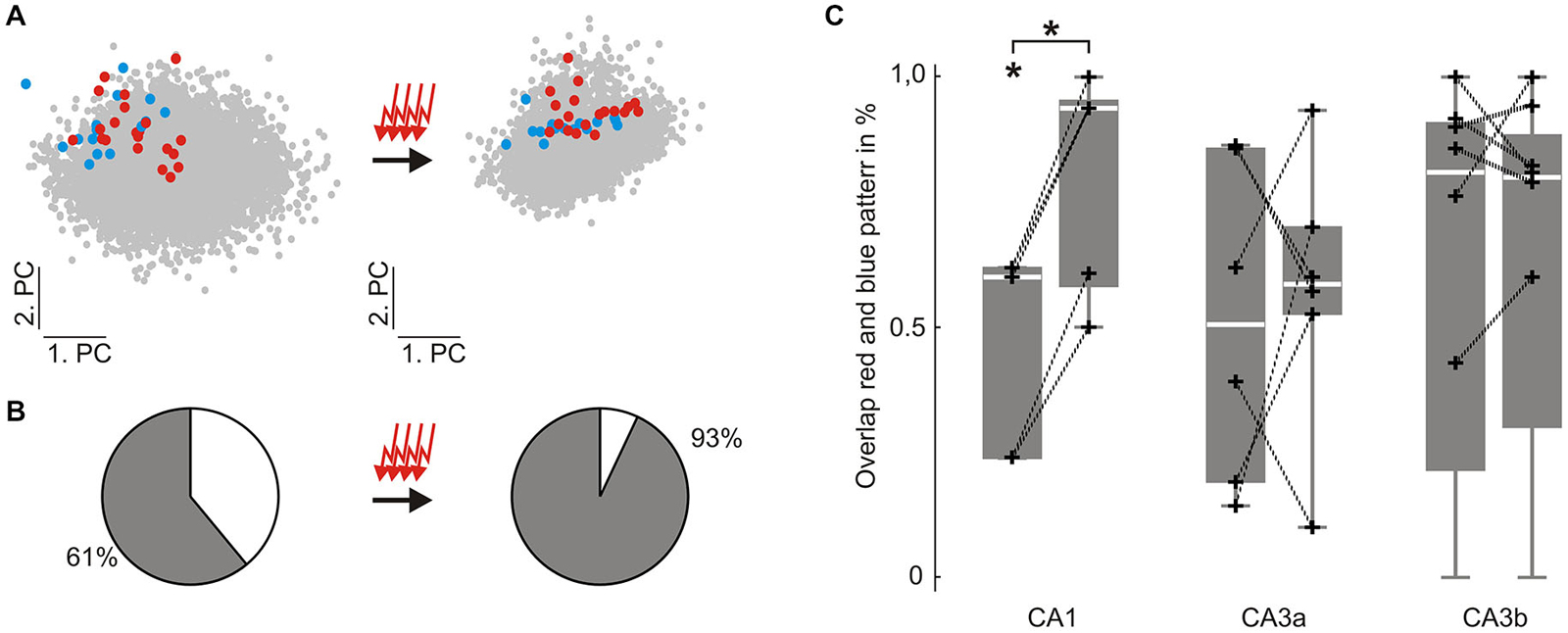
Figure 3. Similarity between two different groups of evoked SPW-R increases in CA1 but not in CA3 following repetitive stimulation. (A) colored dots indicate individual evoked SPW-R waveforms depicted in two dimensions of the principal component (PC) space before (left) and after (right) 100 repetitive stimulations. Red and blue dots are evoked by electrical stimulation at two different locations within the DG. Only the location corresponding to the red dots is repetitively stimulated. Gray dots indicate spontaneous SPW-R. (B) Similarity between the two different groups of evoked events was quantified by their overlap in the 10-dimensional PC space. (C) Overlap of the two groups of evoked events was significantly increased in CA1 (initial overlap: median 60%, 25th 24%, 75th 62%; past stimulation: median 94%, 25th 58%, 75th 95%, p < 0.0001, n = 5). However, in CA3 overlap between both stimulation-induced patterns did not change (CA3a: initial overlap: median 51% 25th 19%, 75th 86%; past stimulation: median 59%, 25th 53%, 75th 70%; CA3b: initial overlap: median 81% 25th 21%, 75th 91%; past stimulation: median 80%, 25th 30%, 75th 88%). “*” means that the finding is significant (p < 0.05). “+” represent the original data underlying the distribution.
In slices that had not undergone 100 repetitive stimulations, the overlap between events was calculated in both directions (data not shown). In contrast to the stimulated condition, overlap remained stable in CA1 (p = 0.25, n = 10 data sets within 5 slices) and percentages of overlap were lower than in the above described repetitive stimulation paradigm (initial overlap: median 17%, 25th 5%, 75th 48%; after stimulation pause: median 5%, 25th 5%, 75th 32%). Likewise, CA3 overlap did not change significantly (CA3a: p = 0.19, n = 10 data sets within 5 slices; CA3b: p = 0.38, n = 16 data sets within 8 slices; CA3a initial overlap 38%, 25th 31%, 75th 88%; past stimulation pause 28%, 25th 13%, 75th 60%; CA3b initial overlap 33%, 25th 6%, 75th 78%; past stimulation pause 22%, 25th 2%, 75th 50%).
In summary, both evoked SPW-R waveform sets in CA1 became more similar to each other when one of the stimulation locations was repetitively stimulated 100 times. No significant change in similarity could be observed in CA3 for the repetitive stimulation paradigm. Moreover, similarity of SPW-R waveforms remained the same in the whole hippocampus, when no repetitive stimulation was performed.
SPW-R Stabilization is Affected by the Timing of Stimulation
Electrical stimulation during an ongoing SPW-R did not elicit a typical SPW-R waveform. This situation can be used to distinguish between the effects of the electrical stimulus itself and the effects of pattern repetition. We therefore tested the effects of 100 repetitive stimulations (~0.1 Hz) timed on a spontaneously occurring SPW (Figure 4A) and compared it to the above described stimulation paradigm (SPW-R evoked by stimulating 150 ms after a spontaneous event). In CA1, stimulating during spontaneous SPW-R did not induce any change in evoked or spontaneous SPW-R waveforms (evoked: median 0.96, p = 0.75, n = 7; spontaneous: median 1.01, p = 0.99, n = 8). Subsequently, when compared to the above described original repetitive stimulation paradigm (100 times, 150 ms after spontaneous SPW-R at 0.1 Hz) we found significant difference for evoked (p = 0.024) and spontaneous (p = 0.00035) waveform stability (Figures 4B,C).
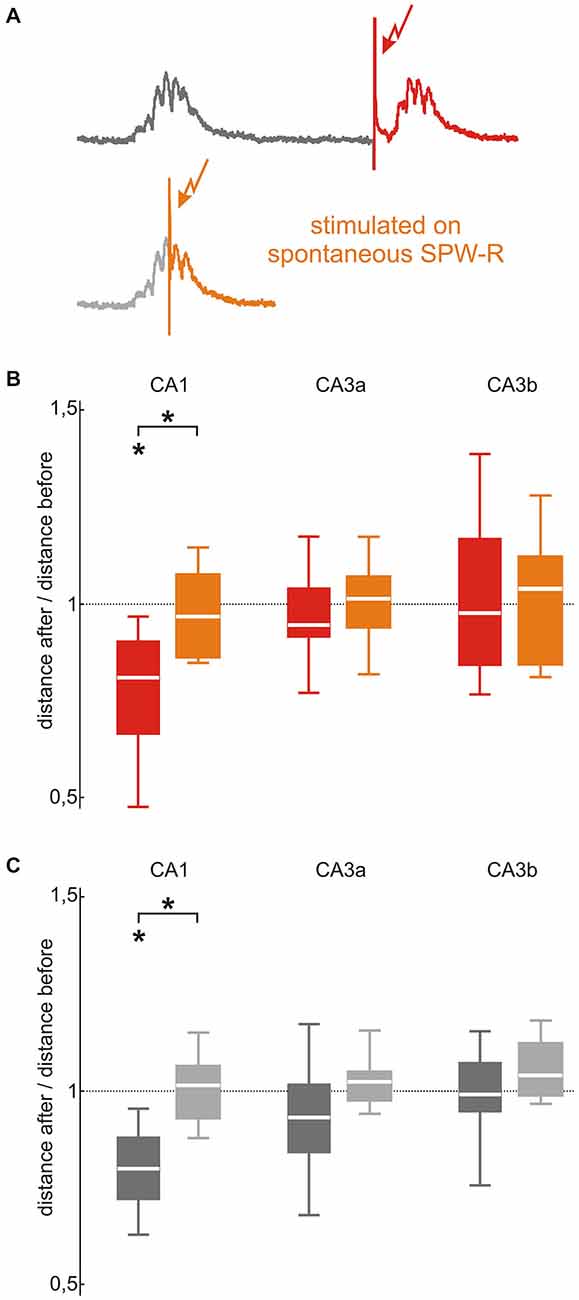
Figure 4. SPW-R stabilization depends on the timing of the stimulation in CA1 but not in CA3. (A) In the original stimulation paradigm (upper trace, red) stimulations were triggered 150 ms after spontaneous SPW-R. To investigate the effect of stimulation timing, repetitive stimulations were performed during spontaneous SPW-R in a second control paradigm (lower trace, orange). (B) The stabilizing effect of the repetitive stimulation performed 150 ms after a spontaneous event (red; p < 0.05, n = 8) abolished by stimulating during a spontaneous SPW-R in CA1 (orange; median 0.96, 25th 0.85, 75th 1.07, n = 7, p < 0.05 between both conditions). This difference was absent in the CA3 (CA3a: median 1.01, 25th 0.93, 75th 1.07; CA3b: median 1.03, 25th 0.84, 75th 1.12) region. (C) Spontaneously occurring SPW-R showed the same effect as evoked events: In CA1 the stabilizing effect of repetitive stimulation 150 ms after spontaneous events (dark gray; p < 0.01, n = 10) was abolished by stimulating during spontaneous SPW-R (light gray; median 1.01, 25th 0.93, 75th 1.07, n = 8, p < 0.001 between both conditions) but not in CA3 (CA3a: median 1.02, 25th 0.97, 75th 1.05; CA3b: median 1.04, 25th 0.99, 75th 1.12). “*” means that the finding is significant (p < 0.05).
In CA3, 100 repetitive stimulations during an ongoing spontaneous SPW-R did not induce changes in SPW-R waveforms of spontaneous or evoked events (Figures 4B,C). Thus, findings were similar to the ones from the above-described 100 repetitive stimulation 150 ms after a spontaneous SPW-R (CA3a p = 0.66 for evoked and p = 0.12 for spontaneous events; CA3b p = 0.90 for evoked events and p = 0.19 for spontaneous events). In CA3a, the after/before distance ratios of evoked (median 1.01, p = 0.80, n = 6) and spontaneous SPW-R (median 1.02, p = 0.30, n = 8) were stable. Neither in CA3b SPW-R variability alterations were observed for evoked (median 1.03, p = 0.79, n = 7) nor spontaneous SPW-R (median 1.04, p = 0.11, n = 8).
Modulation of SPW-R Waveform Stability Depends on NMDA Receptor Activation
Finally, we asked whether NMDA receptor-dependent synaptic plasticity plays a role for stimulation-induced changes of hippocampal assemblies. We therefore repeated the original repetitive stimulation paradigm under bath application of the NMDA receptor antagonist APV (30 μM) over the entire course of the experiment (Figure 5). Under these conditions, median pair-wise distances of evoked events were not affected by 100 repetitive stimulations (CA1 median 0.96, p = 0.41, n = 6; CA3a median 0.95, p = 0.28, n = 7; CA3b median 1.03, p = 0.54, n = 7). Likewise, spontaneously occurring SPW-R remained stable (CA1 median 0.84, p = 0.051, n = 8; CA3a median 1.0, p = 0.56, n = 7; CA3b median 1.08, p = 0.16, n = 8). Compared to the original stimulation paradigm in absence of APV evoked SPW-R stability was significantly lower in CA1 (p = 0.047) but not in CA3 (CA3a p = 0.89; CA3b p = 0.68). Interestingly, we found no significant difference in spontaneous SPW-R variability between slices recorded with to slices recorded without APV bath application (CA1 p = 0.33; CA3a p = 0.44; CA3b p = 0.14).
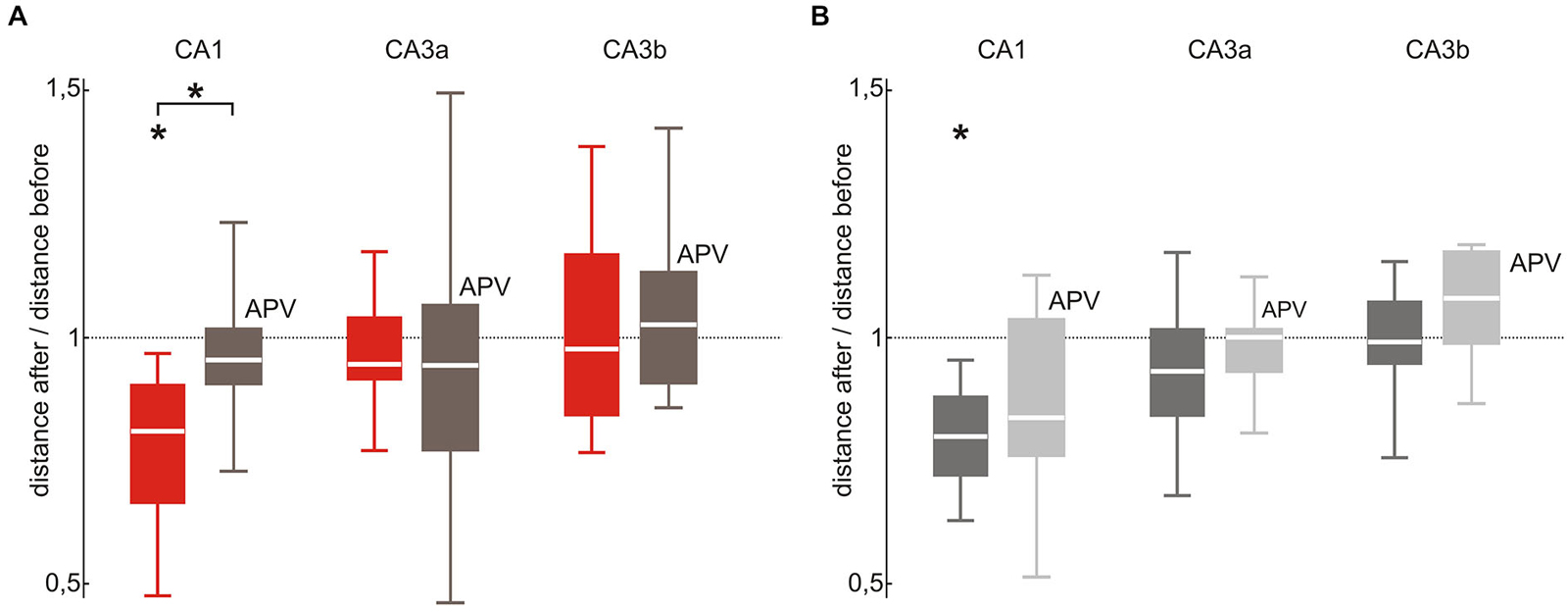
Figure 5. SPW-R stabilization depends on NMDA receptor activation in CA1 but not in CA3. (A) In CA1 the stabilizing effect of repetitive stimulation (red; p < 0.05 n = 8) was abolished by bath application of the NMDA receptor blocker APV (brown; median 0.96, 25th 0.91, 75th 1.0, n = 6, p < 0.05 between both conditions). This difference was absent in the CA3 (CA3a: median 0.95, 25th 0.78, 75th 1.07; CA3b: median 1.03 25th 0.91, 75th 1.14) region. (B) The variability change of spontaneous SPW-R observed after 100 repetitive stimulations in CA1 (dark gray; p < 0.01 n = 10) did not occur under bath application of APV (light gray; CA1: median 0.84, 25th 0.77, 75th 1.05, p = 0.51, n = 8). However, there was no significant different across paradigms. Neither in CA3 repetitive stimulation under bath application of APV altered spontaneous SPW-R variability (CA3a: median 1.0, 25th 0.94, 75th 1.02; CA3b: median 1.08, 25th 0.99, 75th 1.18). “*” means that the finding is significant (p < 0.05).
Discussion
The formation, stabilization and retrieval of precisely timed activity of specific neuronal assemblies may represent a network-level correlate of memory. Conserved temporal relationships between discharges of selected neurons have been observed in various brain regions (Wilson and McNaughton, 1994; Pizzagalli et al., 2003; Pitzalis et al., 2013). In the present study, we made use of an in vitro model of hippocampal sharp wave-ripple (SPW-R) complexes. We evoked field events in mouse hippocampal slices by a sparse and distributed activation of CA3 neurons through electrical stimulation of presynaptic neurons in the upstream DG. On evoked downstream response pattern we observed lower multi-unit activity than on average spontaneous SPW-R which possibly originate from not completely resolved peri-somatic inhibition. Since different levels of multi-unit activity are also found in spontaneously occurring SPW-R and stimulation induced waveform were highly similar to spontaneous SPW-R patterns, we hoped to activate physiological neuronal assemblies in the hippocampal subfields of CA1 and CA3.
We observed that specific and repetitive activation of evoked SPW-R complexes induced a stabilization of the stimulated patterns in a region specific manner. In CA1, repetitive stimulation (0.1 Hz, 100 times) of defined patterns led to an increased stability of the evoked assembly activity. Surprisingly, we also observed a stabilization of the evoked pattern that was not repetitively stimulated. This may be explained by the large overlap between parameters of both patterns which did even increase following stimulation. Interestingly, spontaneously occurring SPW-R also appeared to be stabilized by the repetitive activation of a specific assembly, as reflected by a reduced variability of spontaneously occurring waveforms. In CA3, repetitive stimulation prevented the increased distance between evoked and spontaneous SPW-R which was observed under control conditions without repetitive stimulation.
Local field potentials are the excitatory and inhibitory postsynaptic potentials as well as action potentials of individual cells (Schomburg et al., 2012). Features of well-defined field potential waveforms can be used to investigate the underlying assembly composition in vitro (Reichinnek et al., 2010) and in vivo (Agarwal et al., 2014). Specifically, we have shown previously that the stability and plasticity of neuronal assemblies can reliably be investigated by analyzing the distinct waveforms of SPW-R complexes (Reichinnek et al., 2010; Zylla et al., 2013). While neuronal assemblies cannot be precisely defined with this method, the assembly composition of the slice as a whole can be appropriately analyzed and compared (Reichinnek et al., 2010). We strived to activate a sparse and distributed population of neurons in the CA3 region by stimulation within the supra-pyramidal blade of the dentate gyrus (DG). At this location we expect to stimulate both, DG granule cells and fibers of the perforant path which both innervate CA3 pyramidal cells. As horizontal slices only maintain about 10% of the fibers between the DG and the hippocampus proper, this method of stimulation will generate a sparse and distributed activation of postsynaptic neurons in the CA3 region (Bischofberger et al., 2006; Geiger J, personal communication).
The stabilizing effect of repetitive activation on SPW-R complexes in CA1 was dependent on NMDA receptors (suggesting a contribution of synaptic plasticity, e.g., at the Schaffer collateral synapse) and on the timing of stimulation with respect to spontaneous SPW-R. Plasticity was induced when stimuli were set at ~150 ms after a spontaneous SPW-R while stimulation during an ongoing SPW-R event was without any effect. In this case, the evoked events were clearly different from spontaneous SPW-R and showed high variability. These data suggest that stimulation during spontaneous SPWR leads to aberrant, incomplete or overlapping activation of assemblies, thereby preventing stabilization of pre-existing neuronal connections. Interestingly, neither NMDA receptor blockade nor incomplete and unspecific assembly activation altered the stabilizing effect of repetitive stimulation in the CA3 region. This finding is compatible with a role of mossy fiber synapses for changes in assemblies (Nicoll and Schmitz, 2005; Evstratova and Tóth, 2014). Thus, our results indicate different mechanisms of activity-depended assembly plasticity within the CA3 and the CA1 sub-networks.
Classical protocols for long term potentiation (LTP) at the Schaffer collateral synapse use stimulation frequencies at around 100 Hz (Larson et al., 1986; Barnes et al., 1994; Durand et al., 1996; Abraham and Huggett, 1997; Colgin et al., 2004). The same paradigm is also able to induce spontaneous SPW-R oscillations in “silent” hippocampal slices (Behrens et al., 2005). Additionally, it has been shown that stimulation frequencies below 10 Hz induce hardly any potentiation and that a 1 Hz stimulation incudes long term depression (LTD) rather than LTP (Dunwiddie and Lynch, 1978). Interestingly, memory consolidation by SPW-R complexes in vivo is characterized by a low frequency occurrence of SPW at 2–4 Hz (Steriade et al., 1993; Ylinen et al., 1995; Csicsvari et al., 1999; Wolansky et al., 2006; Schall et al., 2008; Mölle and Born, 2011; Sadowski et al., 2011) while each of the complexes generates several cycles of high frequency oscillations (Ylinen et al., 1995). One consequence of superimposed ripple oscillations is the highly synchronized and near coincident spiking of neurons (Wilson and McNaughton, 1994) that might provide a privileged condition for plasticity processes during SPW-R (Csicsvari et al., 1999; King et al., 1999; Girardeau et al., 2009; Sadowski et al., 2011).
Why do CA3 and CA1 behave differently? The CA3 region has been suggested to form an auto-associative network for transient storage of memories (Kesner, 2013; Rolls, 2013). Moreover, it supports pattern completion upon partial cue activation (Nakazawa et al., 2002; Kesner and Rolls, 2015). Additionally, CA3 serves as the initiator of SPW-R oscillations during sleep and might play an important role in guaranteeing the replay of stored network patterns (Buzsáki, 1989; Wilson and McNaughton, 1994). The underlying pronounced plasticity might explain the tendency of CA3 to form new assemblies following repetitive consolidation of specific sub-sets of neurons. CA1, on the other hand, forms a critical interface between the direct input of environmental information by the entorhinal cortex and the pre-processed information from the CA3 region. This region has been proposed to act as a comparator between previously stored input patterns from CA3 and the actual input coming directly from the entorhinal cortex (Vinogradova, 2001; Kumaran and Maguire, 2007). In addition, CA1 serves as a major source of outputs from hippocampal to neocortical areas and thus plays a crucial role in the transfer process of memory from the hippocampus to the neocortex (Chrobak and Buzsáki, 1994, 1996). These properties might require a higher stability of information which is reflected by both, a stable re-activation of neuronal assemblies under control condition and the tendency to stabilize pre-existing assemblies by repetitive activation.
In summary, low-frequency repetitive activation of specific fast oscillating neuronal activity patterns causes a stabilization and consolidation of the underlying neuronal assemblies. This process of network-level plasticity expresses characteristic regional differences, in line with the proposed differential functions of CA3 (pattern completion and auto-associative memory) and CA1 (novelty detection and readout of existing assemblies).
Conflict of Interest Statement
The authors declare that the research was conducted in the absence of any commercial or financial relationships that could be construed as a potential conflict of interest.
Acknowledgments
Support was provided by the SFB636 in which Martin Keller attended the graduate program “Translational Neuroscience”. Susanne Reichinnek held a postdoctoral fellowship of the Medical Faculty of Heidelberg University. Special thanks go to Martina Müller for her assistance concerning the data analysis and to Fabian Roth and Claus Bruehl for their helpful support during the experiments.
References
Abraham, W. C., and Huggett, A. (1997). Induction and reversal of long-term potentiation by repeated high-frequency stimulation in rat hippocampal slices. Hippocampus 7, 137–145. doi: 10.1002/(sici)1098-1063(1997)7:2<137::aid-hipo3>3.0.co;2-k
Acsády, L., Kamondi, A., Sík, A., Freund, T., and Buzsáki, G. (1998). GABAergic cells are the major postsynaptic targets of mossy fibers in the rat hippocampus. J. Neurosci. 18, 3386–3403.
Agarwal, G., Stevenson, I. H., Berényi, A., Mizuseki, K., Buzsáki, G., and Sommer, F. T. (2014). Spatially distributed local fields in the hippocampus encode rat position. Science 344, 626–630. doi: 10.1126/science.1250444
Barnes, C. A., Jung, M. W., McNaughton, B. L., Korol, D. L., Andreasson, K., and Worley, P. F. (1994). LTP saturation and spatial learning disruption: effects of task variables and saturation levels. J. Neurosci. 14, 5793–5806.
Behrens, C. J., van den Boom, L. P., de Hoz, L., Friedman, A., and Heinemann, U. (2005). Induction of sharp wave-ripple complexes in vitro and reorganization of hippocampal networks. Nat. Neurosci. 8, 1560–1567. doi: 10.1038/nn1571
Bischofberger, J., Engel, D., Li, L., Geiger, J. R., and Jonas, P. (2006). Patch-clamp recording from mossy fiber terminals in hippocampal slices. Nat. Protoc. 1, 2075–2081. doi: 10.1038/nprot.2006.312
Bliss, T. V., and Lomo, T. (1973). Long-lasting potentiation of synaptic transmission in the dentate area of the anaesthetized rabbit following stimulation of the perforant path. J. Physiol. 232, 331–356. doi: 10.1113/jphysiol.1973.sp010273
Both, M., Bähner, F., Von Bohlen Und Halbach, O., and Draguhn, A. (2008). Propagation of specific network patterns through the mouse hippocampus. Hippocampus 18, 899–908. doi: 10.1002/hipo.20446
Buschler, A., Goh, J. J., and Manahan-Vaughan, D. (2012). Frequency dependency of NMDA receptor-dependent synaptic plasticity in the hippocampal CA1 region of freely behaving mice. Hippocampus 22, 2238–2248. doi: 10.1002/hipo.22041
Buzsáki, G. (1989). Two-stage model of memory trace formation: a role for “noisy” brain states. Neuroscience 31, 551–570. doi: 10.1016/0306-4522(89)90423-5
Buzsáki, G., and Draguhn, A. (2004). Neuronal oscillations in cortical networks. Science 304, 1926–1929. doi: 10.1126/science.1099745
Carr, M. F., Karlsson, M. P., and Frank, L. M. (2012). Transient slow gamma synchrony underlies hippocampal memory replay. Neuron 75, 700–713. doi: 10.1016/j.neuron.2012.06.014
Chrobak, J. J., and Buzsáki, G. (1994). Selective activation of deep layer (V-VI) retrohippocampal cortical neurons during hippocampal sharp waves in the behaving rat. J. Neurosci. 14, 6160–6170.
Chrobak, J. J., and Buzsáki, G. (1996). High-frequency oscillations in the output networks of the hippocampal-entorhinal axis of the freely behaving rat. J. Neurosci. 16, 3056–3066.
Colgin, L. L., Kubota, D., Jia, Y., Rex, C. S., and Lynch, G. (2004). Long-term potentiation is impaired in rat hippocampal slices that produce spontaneous sharp waves. J. Physiol. 558, 953–961. doi: 10.1113/jphysiol.2004.068080
Csicsvari, J., Hirase, H., Czurkó, A., Mamiya, A., and Buzsáki, G. (1999). Oscillatory coupling of hippocampal pyramidal cells and interneurons in the behaving rat. J. Neurosci. 19, 274–287.
Dunwiddie, T., and Lynch, G. (1978). Long-term potentiation and depression of synaptic responses in the rat hippocampus: localization and frequency dependency. J. Physiol. 276, 353–367. doi: 10.1113/jphysiol.1978.sp012239
Durand, G. M., Kovalchuk, Y., and Konnerth, A. (1996). Long-term potentiation and functional synapse induction in developing hippocampus. Nature 381, 71–75. doi: 10.1038/381071a0
Evstratova, A., and Tóth, K. (2014). Information processing and synaptic plasticity at hippocampal mossy fiber terminals. Front. Cell. Neurosci. 8:28. doi: 10.3389/fncel.2014.00028
Frank, M. J., Rudy, J. W., and O’reilly, R. C. (2003). Transitivity, flexibility, conjunctive representations and the hippocampus. II. A computational analysis. Hippocampus 13, 341–354. doi: 10.1002/hipo.10084
Girardeau, G., Benchenane, K., Wiener, S. I., Buzsáki, G., and Zugaro, M. B. (2009). Selective suppression of hippocampal ripples impairs spatial memory. Nat. Neurosci. 12, 1222–1223. doi: 10.1038/nn.2384
Goh, J. J., and Manahan-Vaughan, D. (2013). Synaptic depression in the CA1 region of freely behaving mice is highly dependent on afferent stimulation parameters. Front. Integr. Neurosci. 7:1. doi: 10.3389/fnint.2013.00001
Kesner, R. P. (2013). A process analysis of the CA3 subregion of the hippocampus. Front. Cell. Neurosci. 7:78. doi: 10.3389/fncel.2013.00078
Kesner, R. P., and Rolls, E. T. (2015). A computational theory of hippocampal function and tests of the theory: new developments. Neurosci. Biobehav. Rev. 48c, 92–147. doi: 10.1016/j.neubiorev.2014.11.009
King, C., Henze, D. A., Leinekugel, X., and Buzsáki, G. (1999). Hebbian modification of a hippocampal population pattern in the rat. J. Physiol. 521(Pt. 1), 159–167. doi: 10.1111/j.1469-7793.1999.00159.x
Köhr, G., Jensen, V., Koester, H. J., Mihaljevic, A. L., Utvik, J. K., Kvello, A., et al. (2003). Intracellular domains of NMDA receptor subtypes are determinants for long-term potentiation induction. J. Neurosci. 23, 10791–10799.
Kumaran, D., and Maguire, E. A. (2007). Which computational mechanisms operate in the hippocampus during novelty detection? Hippocampus 17, 735–748. doi: 10.1002/hipo.20326
Larson, J., Wong, D., and Lynch, G. (1986). Patterned stimulation at the theta frequency is optimal for the induction of hippocampal long-term potentiation. Brain Res. 368, 347–350. doi: 10.1016/0006-8993(86)90579-2
Lee, A. K., and Wilson, M. A. (2002). Memory of sequential experience in the hippocampus during slow wave sleep. Neuron 36, 1183–1194. doi: 10.1016/s0896-6273(02)01096-6
Lisman, J. E., Talamini, L. M., and Raffone, A. (2005). Recall of memory sequences by interaction of the dentate and CA3: a revised model of the phase precession. Neural Netw. 18, 1191–1201. doi: 10.1016/j.neunet.2005.08.008
Lopes-dos-Santos, V., Ribeiro, S., and Tort, A. B. (2013). Detecting cell assemblies in large neuronal populations. J. Neurosci. Methods 220, 149–166. doi: 10.1016/j.jneumeth.2013.04.010
Maier, N., Nimmrich, V., and Draguhn, A. (2003). Cellular and network mechanisms underlying spontaneous sharp wave-ripple complexes in mouse hippocampal slices. J. Physiol. 550, 873–887. doi: 10.1113/jphysiol.2003.044602
Mölle, M., and Born, J. (2011). Slow oscillations orchestrating fast oscillations and memory consolidation. Prog. Brain Res. 193, 93–110. doi: 10.1016/b978-0-444-53839-0.00007-7
Nakazawa, K., Quirk, M. C., Chitwood, R. A., Watanabe, M., Yeckel, M. F., Sun, L. D., et al. (2002). Requirement for hippocampal CA3 NMDA receptors in associative memory recall. Science 297, 211–218. doi: 10.1126/science.1071795
Nicolelis, M. A., Baccala, L. A., Lin, R. C., and Chapin, J. K. (1995). Sensorimotor encoding by synchronous neural ensemble activity at multiple levels of the somatosensory system. Science 268, 1353–1358. doi: 10.1126/science.7761855
Nicoll, R. A., and Schmitz, D. (2005). Synaptic plasticity at hippocampal mossy fibre synapses. Nat. Rev. Neurosci. 6, 863–876. doi: 10.1038/nrn1786
O’Keefe, J., and Recce, M. L. (1993). Phase relationship between hippocampal place units and the EEG theta rhythm. Hippocampus 3, 317–330. doi: 10.1002/hipo.450030307
Palmer, M. J., Isaac, J. T., and Collingridge, G. L. (2004). Multiple, developmentally regulated expression mechanisms of long-term potentiation at CA1 synapses. J. Neurosci. 24, 4903–4911. doi: 10.1523/jneurosci.0170–04.2004
Pitzalis, S., Bozzacchi, C., Bultrini, A., Fattori, P., Galletti, C., and Di Russo, F. (2013). Parallel motion signals to the medial and lateral motion areas V6 and MT+. Neuroimage 67, 89–100. doi: 10.1016/j.neuroimage.2012.11.022
Pizzagalli, D. A., Greischar, L. L., and Davidson, R. J. (2003). Spatio-temporal dynamics of brain mechanisms in aversive classical conditioning: high-density event-related potential and brain electrical tomography analyses. Neuropsychologia 41, 184–194. doi: 10.1016/s0028-3932(02)00148-3
Reichinnek, S., Künsting, T., Draguhn, A., and Both, M. (2010). Field potential signature of distinct multicellular activity patterns in the mouse hippocampus. J. Neurosci. 30, 15441–15449. doi: 10.1523/jneurosci.2535-10.2010
Rolls, E. T. (2013). A quantitative theory of the functions of the hippocampal CA3 network in memory. Front. Cell. Neurosci. 7:98. doi: 10.3389/fncel.2013.00098
Sadowski, J. H., Jones, M. W., and Mellor, J. R. (2011). Ripples make waves: binding structured activity and plasticity in hippocampal networks. Neural Plast. 2011:960389. doi: 10.1155/2011/960389
Schall, K. P., Kerber, J., and Dickson, C. T. (2008). Rhythmic constraints on hippocampal processing: state and phase-related fluctuations of synaptic excitability during theta and the slow oscillation. J. Neurophysiol. 99, 888–899. doi: 10.1152/jn.00915.2007
Schomburg, E. W., Anastassiou, C. A., Buzsáki, G., and Koch, C. (2012). The spiking component of oscillatory extracellular potentials in the rat hippocampus. J. Neurosci. 32, 11798–11811. doi: 10.1523/jneurosci.0656-12.2012
Sirota, A., and Buzsáki, G. (2005). Interaction between neocortical and hippocampal networks via slow oscillations. Thalamus Relat. Syst. 3, 245–259. doi: 10.1017/s1472928807000258
Steriade, M., McCormick, D. A., and Sejnowski, T. J. (1993). Thalamocortical oscillations in the sleeping and aroused brain. Science 262, 679–685. doi: 10.1126/science.8235588
Vinogradova, O. S. (2001). Hippocampus as comparator: role of the two input and two output systems of the hippocampus in selection and registration of information. Hippocampus 11, 578–598. doi: 10.1002/hipo.1073.abs
Wilson, M. A., and McNaughton, B. L. (1994). Reactivation of hippocampal ensemble memories during sleep. Science 265, 676–679. doi: 10.1126/science.8036517
Wolansky, T., Clement, E. A., Peters, S. R., Palczak, M. A., and Dickson, C. T. (2006). Hippocampal slow oscillation: a novel EEG state and its coordination with ongoing neocortical activity. J. Neurosci. 26, 6213–6229. doi: 10.1523/jneurosci.5594-05.2006
Ylinen, A., Bragin, A., Nádasdy, Z., Jandó, G., Szabó, I., Sik, A., et al. (1995). Sharp wave-associated high-frequency oscillation (200 Hz) in the intact hippocampus: network and intracellular mechanisms. J. Neurosci. 15, 30–46.
Keywords: plasticity, sharp wave-ripple, SPW-R, oscillation, hippocampus, assembly, neuronal assemblies, neuronal plasticity
Citation: Keller MK, Draguhn A, Both M and Reichinnek S (2015) Activity-dependent plasticity of mouse hippocampal assemblies in vitro. Front. Neural Circuits 9:21. doi: 10.3389/fncir.2015.00021
Received: 15 February 2015; Accepted: 27 April 2015;
Published online: 18 May 2015.
Edited by:
Alexey Semyanov, University of Nizhny Novgorod, RussiaReviewed by:
Mark Cunningham, University of Newcastle, UKJochen Roeper, Goethe University Frankfurt, Germany
Copyright © 2015 Keller, Draguhn, Both and Reichinnek. This is an open-access article distributed under the terms of the Creative Commons Attribution License (CC BY). The use, distribution and reproduction in other forums is permitted, provided the original author(s) or licensor are credited and that the original publication in this journal is cited, in accordance with accepted academic practice. No use, distribution or reproduction is permitted which does not comply with these terms.
*Correspondence: Martin K. Keller, Institute of Physiology and Pathophysiology, University of Heidelberg, INF 326, 69117 Heidelberg, Germany,bWFydGluLmsua2VsbGVyQGdtYWlsLmNvbQ==