- Department of Molecular and Systems Biology, Geisel School of Medicine at Dartmouth College, Hanover, NH, United States
Serotonin (5-HT) selectively excites subpopulations of pyramidal neurons in the neocortex via activation of 5-HT2A (2A) receptors coupled to Gq subtype G-protein alpha subunits. Gq-mediated excitatory responses have been attributed primarily to suppression of potassium conductances, including those mediated by KV7 potassium channels (i.e., the M-current), or activation of non-specific cation conductances that underlie calcium-dependent afterdepolarizations (ADPs). However, 2A-dependent excitation of cortical neurons has not been extensively studied, and no consensus exists regarding the underlying ionic effector(s) involved. In layer 5 of the mouse medial prefrontal cortex, we tested potential mechanisms of serotonergic excitation in commissural/callosal (COM) projection neurons, a subpopulation of pyramidal neurons that exhibits 2A-dependent excitation in response to 5-HT. In baseline conditions, 5-HT enhanced the rate of action potential generation in COM neurons experiencing suprathreshold somatic current injection. This serotonergic excitation was occluded by activation of muscarinic acetylcholine (ACh) receptors, confirming that 5-HT acts via the same Gq-signaling cascades engaged by ACh. Like ACh, 5-HT promoted the generation of calcium-dependent ADPs following spike trains. However, calcium was not necessary for serotonergic excitation, as responses to 5-HT were enhanced (by >100%), rather than reduced, by chelation of intracellular calcium with 10 mM BAPTA. This suggests intracellular calcium negatively regulates additional ionic conductances gated by 2A receptors. Removal of extracellular calcium had no effect when intracellular calcium signaling was intact, but suppressed 5-HT response amplitudes, by about 50%, when BAPTA was included in patch pipettes. This suggests that 2A excitation involves activation of a non-specific cation conductance that is both calcium-sensitive and calcium-permeable. M-current suppression was found to be a third ionic effector, as blockade of KV7 channels with XE991 (10 μM) reduced serotonergic excitation by ∼50% in control conditions, and by ∼30% with intracellular BAPTA present. Together, these findings demonstrate a role for at least three distinct ionic effectors, including KV7 channels, a calcium-sensitive and calcium-permeable non-specific cation conductance, and the calcium-dependent ADP conductance, in mediating serotonergic excitation of COM neurons.
Introduction
In the prefrontal cortex (PFC), serotonin (5-HT) acts as a neurotransmitter to regulate diverse cognitive processes, including working memory (Williams et al., 2002; Liy-Salmeron and Meneses, 2008), cognitive flexibility (Clarke et al., 2004, 2005), impulsivity (Harrison et al., 1997; Winstanley et al., 2003), attention (Winstanley et al., 2003), and fear (Almada et al., 2015; Marinho et al., 2015; Leon et al., 2017). 5-HT regulates prefrontal circuits by acting on a variety of receptor subtypes differentially expressed in subpopulations of cortical neurons. In pyramidal cells, which comprise heterogeneous subpopulations of glutamatergic neurons, 5-HT acts primarily via two G-protein-coupled receptors that have opposing influences on neuronal excitability. 5-HT1A (1A) receptors are expressed in perisomatic or axonal compartments (Azmitia et al., 1996; DeFelipe et al., 2001; Czyrak et al., 2003; Cruz et al., 2004) and inhibit neurons by enhancing potassium conductances downstream of Gi/o-associated G-protein βγ subunits (Andrade and Nicoll, 1987; Colino and Halliwell, 1987; Davies et al., 1987; Tanaka and North, 1993; Spain, 1994; Luscher et al., 1997; Goodfellow et al., 2009). On the other hand, 5-HT2A (2A) receptors coupled to Gq subtype G-protein α subunits are expressed in dendritic compartments (Willins et al., 1997; Jakab and Goldman-Rakic, 1998; Xu and Pandey, 2000; Miner et al., 2003), and act generally to enhance pyramidal neuron excitability. However, the ionic mechanisms responsible for 2A-dependent excitation of pyramidal neurons have been less well studied (Araneda and Andrade, 1991; Spain, 1994; Villalobos et al., 2005, 2011), largely due to the fact that the 2A receptor is not universally expressed in adult neurons. As a result, much of our understanding of 2A signaling is inferred from studies of cholinergic excitation, which is mediated by the more ubiquitous Gq-coupled M1-subtype muscarinic acetylcholine (ACh) receptor. Classically, Gq-coupled excitation in pyramidal neurons is associated with suppression of potassium conductances, including those mediating the “M” (muscarine-suppressed) current and those responsible for afterhyperpolarizations (AHPs) in pyramidal neurons following bouts of action potential generation (Krnjevic et al., 1971; Schwindt et al., 1988; McCormick and Williamson, 1989; Araneda and Andrade, 1991; Wang and McCormick, 1993; Villalobos et al., 2005, 2011). However, reduction of potassium conductances does not fully account for Gq-mediated excitation in neocortical pyramidal neurons (Andrade, 1991; Haj-Dahmane and Andrade, 1996), as Gq-coupled muscarinic ACh and 5-HT receptors also engage inward currents generated by calcium-dependent (Haj-Dahmane and Andrade, 1998; Yan et al., 2009; Lei et al., 2014) and independent (Haj-Dahmane and Andrade, 1996; Shalinsky et al., 2002; Egorov et al., 2003; Magistretti et al., 2004; Baker et al., 2018) non-specific cation channels.
Here, to better understand the mechanisms underlying 2A-mediated excitation in the cerebral cortex, we take advantage of the selective and robust 2A excitation that occurs in a subpopulation of pyramidal neurons that project to the contralateral cerebral hemisphere (Avesar and Gulledge, 2012). Using fluorescent retrograde tracers injected unilaterally in the mouse medial PFC (mPFC), we challenged 2A excitation in retrograde-labeled commissural/callosal (COM) projection neurons with a combination of pharmacological and ionic substitution approaches. Our results demonstrate that serotonin 2A receptors engage multiple ionic effectors, including the M-current, a calcium-sensitive but calcium-permeable non-specific cation conductance, and the calcium-dependent non-specific cation conductance underlying the ADP, to promote action potential generation in COM neurons.
Materials and Methods
Animals
Experiments involved adult (6-to-10-week-old) C57BL/6J male and female mice housed in 12:12 h light:dark cycle and provided food and water ad libitum. All experiments were carried out according to methods approved by the Institutional Animal Care and Use Committee of Dartmouth College. No significant sex-dependent differences in cellular physiology or 5-HT responses were observed (Table 1).
Retrograde Labeling
Red or green fluorescent microbeads (Retrobeads, Lumafluor Inc.) were injected unilaterally into the left prelimbic cortex using age-appropriate coordinates (Paxinos and Franklin, 2004) to label COM neurons in the contralateral mPFC. Animals were anesthetized throughout surgeries with vaporized isoflurane (∼2%). Following craniotomy, a microsyringe was lowered into the mPFC and 300 nL of undiluted Retrobead solution was injected over a 10 min period. Animals were allowed to recover from surgery for at least 72 h before use in electrophysiological experiments. Locations of microbead injections were confirmed post hoc in coronal sections of the mPFC.
Slice Preparation
Following isoflurane anesthesia and decapitation, brains were removed and submerged in artificial cerebral spinal fluid (aCSF) containing, in mM: 125 NaCl, 25 NaHCO3, 3 KCl, and 1.25 NaH2PO4, 0.5 CaCl2, 5 MgCl2, 25 glucose, and saturated with 95% O2 / 5% CO2. Coronal brain slices (250 μm thick) of the mPFC were cut using a Leica VT 1200 slicer and stored in a chamber filled with aCSF containing 2 mM CaCl2 and 1 mM MgCl2 at 35°C for ∼45 min, then kept at room temperature (∼26°C) until use in experiments.
Electrophysiology
Slices were transferred to a recording chamber continuously perfused with oxygenated aCSF at 35–36°C and visualized with an Olympus BX51WI microscope. Unless otherwise noted, whole-cell current-clamp recordings of Retrobead-labeled COM neurons in layer 5 were made with patch pipettes (∼5 MΩ) filled with our standard intracellular solution containing, in mM: 135 K-gluconate, 2 NaCl, 2 MgCl2, 10 HEPES, 3 Na2ATP, and 0.3 NaGTP (pH 7.2 with KOH). Epifluorescence illumination using 470 nm or 530 nm LEDs was used to target Retrobead-labeled layer 5 COM neurons in the prelimbic cortex for whole-cell recording. Data were acquired with Axograph software (Axograph Scientific) using BVC-700 amplifiers (Dagan Corporation) and ITC-18 digitizers (HEKA Instruments). Membrane potentials were sampled at 25 kHz, filtered at 10 kHz, and corrected for a junction potential of +12 mV. As 2A-mediated excitation is activity-dependent (Stephens et al., 2014), responses to 5-HT were measured during periods of action potential generation (∼5 Hz) produced by somatic DC current injection. Mean currents injected, and the resulting baseline firing frequencies, for different experimental conditions are listed in Table 2.
5-HT (100 μM) was dissolved in aCSF, loaded into a patch pipette placed ∼50 μm from the targeted soma, and pneumatically applied for 1 s at ∼15 psi. COM neurons were classified as “COM-excited” or “COM-biphasic” based on their initial response to 5-HT (see Avesar and Gulledge, 2012). At 100 μM, all excitatory effects of 5-HT on COM neurons can be attributed to 2A receptors, as excitation is eliminated in the presence of 500 nM MDL 11939 (Avesar and Gulledge, 2012; Stephens et al., 2014). To preserve focus on 2A-driven signaling, only COM-excited neurons were used for experiments. Serotonergic excitation was quantified as the average instantaneous spike frequency (ISF) over the first 500 ms following the peak post-5-HT increase in ISF, as measured relative to the mean baseline ISF (i.e., as the percent increase above baseline firing rates), and as the integral of the increased ISF over time.
Pharmacological Manipulations
Carbamylcholine chloride (carbachol, 50–100 μM; Sigma–Aldrich) was used to activate Gq-coupled M1 muscarinic acetylcholine receptors, which mediate cholinergic excitation of pyramidal neurons in the mPFC and hippocampus (Gulledge et al., 2009; Dasari and Gulledge, 2011). XE991 (10 μM; Tocris Bioscience or Cayman Chemicals) was used to block KV7 channels. 1,2-Bis(2-Aminophenoxy)ethane-N,N,N′,N′-tetraacetic acid (BAPTA, 10 mM; Sigma–Aldrich) was included in the patch-pipette solution in some experiments to chelate intracellular calcium. For experiments using nominally calcium-free aCSF, CaCl2 was replaced with either equimolar or 5 mM MgCl2 (no differences were observed between these two substitution conditions). In some experiments, extracellular KCl was reduced to 0.5 mM, with NaCl being raised to 127.5 mM.
Statistical Analyses
Unless otherwise noted, data are presented as mean ± SEM, and were assessed with either Student’s t-test (two-tailed, paired or unpaired) or a one-way ANOVA (two-tailed, repeated measures where appropriate) using Wizard for Mac version 1.9 (Evan Miller). Significance was defined as p < 0.05.
Results
To characterize the ionic mechanisms underlying 2A-receptor-mediated serotonergic excitation in the cerebral cortex, we made electrical recordings from labeled callosal/commissural (COM) projection neurons in layer 5 of the mouse mPFC. Because 2A-mediated excitation of COM neurons requires coincident exogenous excitatory drive (Stephens et al., 2014), 5-HT was focally applied during periods of sustained action potential generation (∼5 Hz) produced by somatic DC-current injection (Figure 1A). Under these conditions, 5-HT (1 s at ∼15 psi) generated robust increases in instantaneous spike frequency (ISF) that persisted for many seconds. In these same neurons, 5-HT delivered at resting membrane potentials had little, if any, effect (Figure 1A). Across all COM neurons recorded with our normal whole-cell pipette solution (n = 54), the magnitude of serotonergic excitation, as measured by the peak increase in ISF following 5-HT application, was negatively correlated with baseline firing rate (p = 0.004, R = -0.39, Figure 1B), suggesting a ceiling effect of serotonergic excitation at high baseline firing rates. Thus, for each experimental manipulation, we adjusted current intensities to maintain similar baseline firing frequencies across experimental conditions (Table 2).
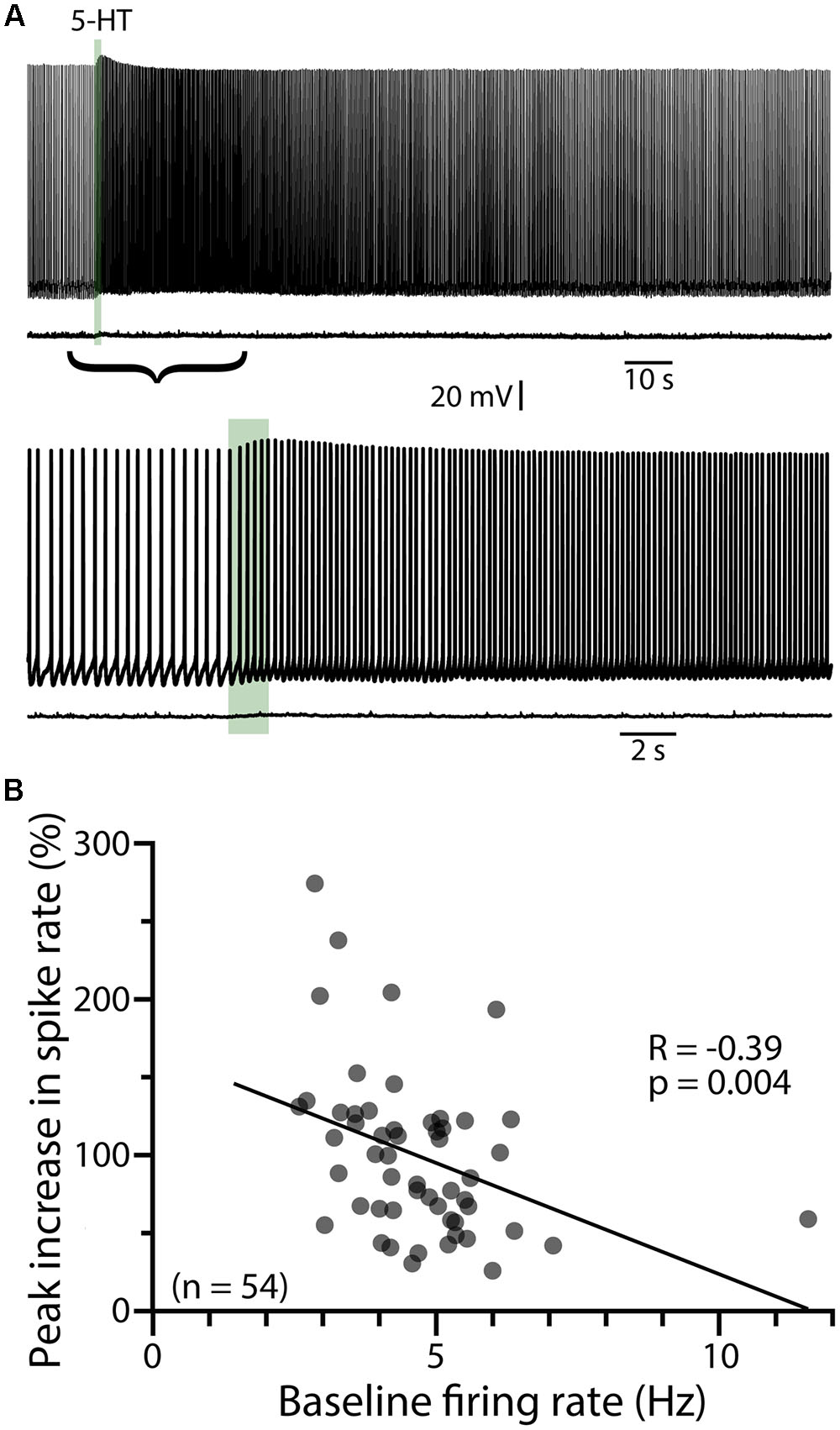
FIGURE 1. Serotonergic excitation of COM neurons. (A) Voltage traces showing responses to focal application of 5-HT (100 μM, 1 s, ∼15 psi; green bar) during current-evoked action potential generation (above) or at the resting membrane potential of -74 mV (below). Lower set of traces shows the 5-HT responses at a faster timescale. (B) Plot of peak changes in instantaneous spike frequencies following 5-HT application vs. baseline firing rates for all neurons recorded with standard (i.e., non-BAPTA) pipette solution (see also, Tables 1, 2). Regression analysis revealed a negative correlation between baseline firing rate and the magnitude of serotonergic excitation.
Cholinergic Activation of L5PNs Occludes Serotonergic Excitation
Since serotonergic responses are qualitatively similar to cholinergic responses mediated by M1 muscarinic ACh receptors (Araneda and Andrade, 1991; Haj-Dahmane and Andrade, 1999; Gulledge et al., 2009), we first tested whether 5-HT and ACh share common intracellular signaling cascades by measuring responses to 5-HT before and after tonically activating muscarinic ACh receptors with carbachol (50–100 μM, bath applied). In baseline conditions, focal application of 5-HT produced mean increases in peak ISF of 117 ± 23% (n = 9; p < 0.001, paired Student’s t-test), with response integrals being 168 ± 34 Hz•s (Figure 2A). Bath application of carbachol depolarized COM neurons (by 6 ± 2 mV) and lowered the current necessary to evoke sustained action potential generation by 54 ± 16%, from 138 ± 24 pA to 71 ± 26 pA (n = 6 of 9 neurons). Carbachol induced spontaneous firing in 3 of 9 neurons. Across all 9 neurons, bath-applied carbachol fully occluded serotonergic excitation, as 5-HT failed to enhance action potential frequency during cholinergic stimulation (n = 9; Figure 2). Instead, 5-HT application in the presence of carbachol reduced the firing frequency of COM neurons (peak change in ISF = -40 ± 10%; n = 9, p = 0.003, paired Student’s t-test; Figures 2B,C), resulting in negative response integrals (-103 ± 39 Hz•s). These results confirm significant overlap of Gq-signaling cascades downstream of M1 and 2A receptors in COM neurons.
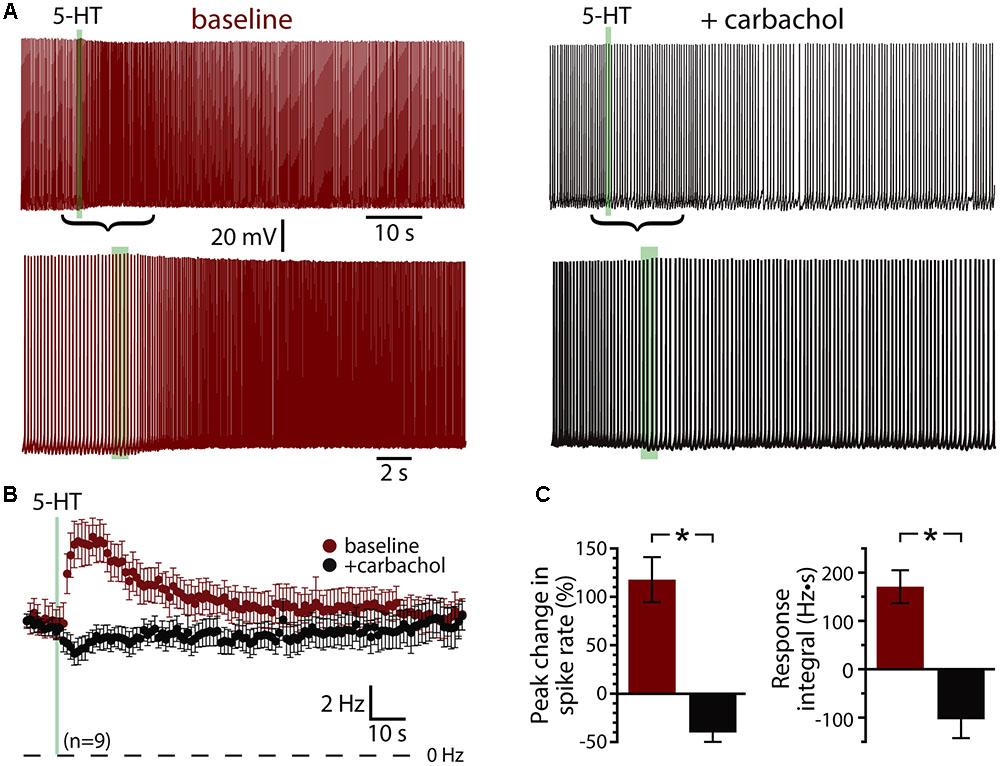
FIGURE 2. Serotonergic and cholinergic excitation share signaling mechanisms. (A) Voltage traces showing responses to focal application of 5-HT (100 μM, 1 s, ∼15 psi; green bar) during periods of action potential generation in control conditions (left; +66 pA DC current injection) and after bath-application of the cholinergic agonist carbachol (right; no current injection in 50 μM carbachol). Lower traces show 5-HT responses in the two conditions at a faster timescale. (B) Plots of mean (±SEM) instantaneous spike frequencies in baseline conditions (red) and in the presence of 50–100 μM carbachol (black) for 9 neurons. (C) Comparisons of the percent change in firing (left) and response integrals (right) for serotonergic responses in control and carbachol conditions.
5-HT Generates Afterdepolarizations in COM Neurons
A prominent feature of muscarinic excitation of pyramidal neurons is the generation of afterdepolarizations (ADPs) that occur after pairing M1 receptor activation with brief suprathreshold current injections (Andrade, 1991; Haj-Dahmane and Andrade, 1998; Gulledge et al., 2009; Dasari et al., 2013). Similarly, 5-HT acting via 2A receptors can initiate ADPs in neocortical pyramidal neurons (Araneda and Andrade, 1991; Spain, 1994; Zhang and Arsenault, 2005). We confirmed that 5-HT promotes ADPs in COM neurons by evoking trains of ten action potentials using brief (2 ms) high-amplitude (3 nA) current steps presented at 25 Hz (Figure 3A). On some trials, 5-HT was focally applied 1 s before current pulses, and peak depolarization relative to the resting membrane potential (RMP) was measured in the first 1 s following the spike train. Pairing 5-HT with current pulses generated small ADPs with mean amplitudes of 1.6 ± 0.2 mV (n = 16; p < 0.001, paired Student’s t-test; Figure 3B). Serotonergic ADPs were absent when the calcium chelating agent BAPTA (10 mM) was included in the patch-pipette solution (n = 6; Figure 3B), confirming that they are gated by intracellular calcium signaling (Spain, 1994; Haj-Dahmane and Andrade, 1998; Zhang and Arsenault, 2005; Dasari et al., 2013). These results suggest that the calcium-dependent and Gq-triggered ADP may represent a common ionic mechanism contributing to serotonergic and cholinergic excitation in COM neurons.
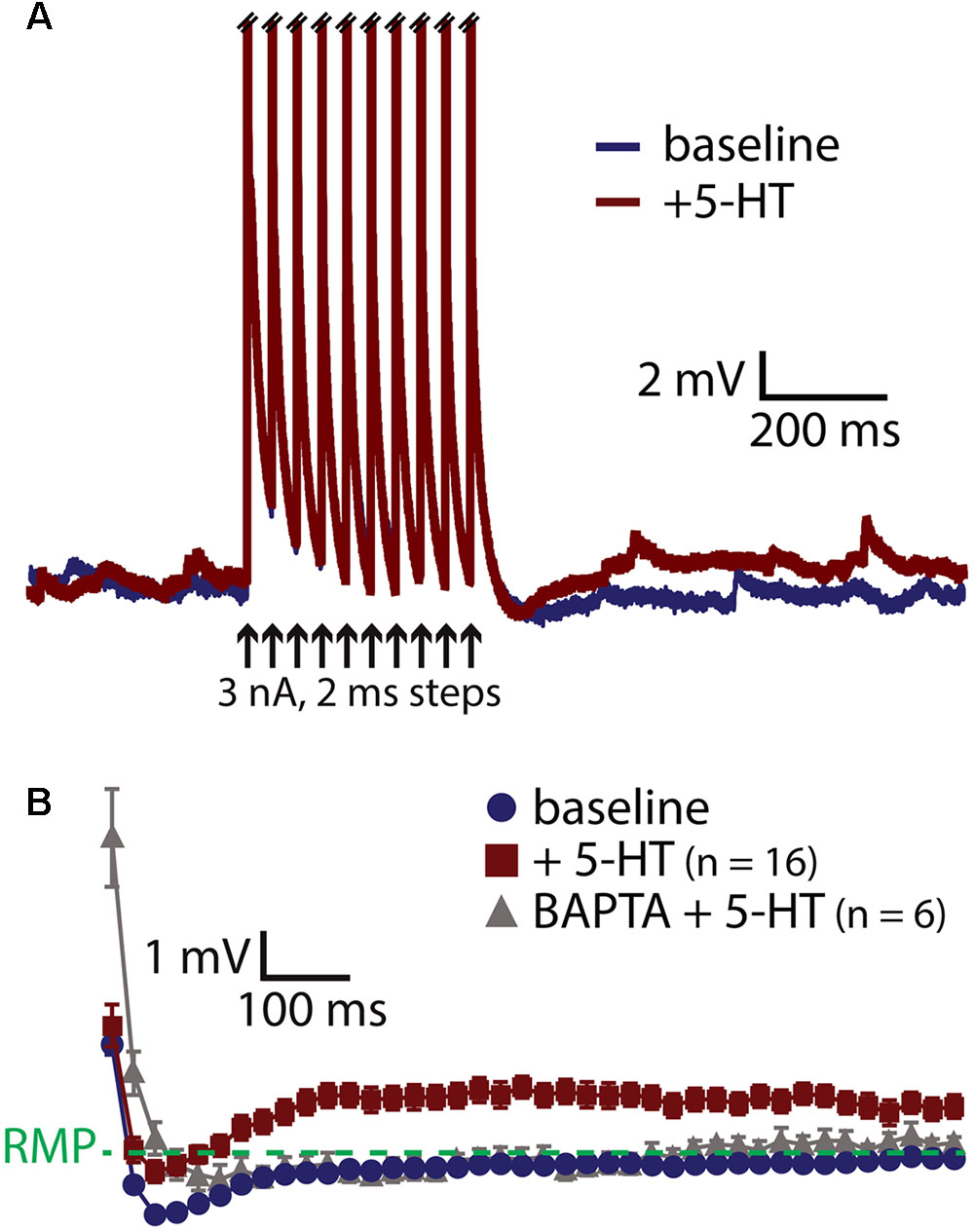
FIGURE 3. 5-HT induces a calcium-dependent afterdepolarization (ADP) in COM neurons. (A) Voltage traces from a COM neuron experiencing a train of ten high-amplitude (3 nA, 2 ms, 25 Hz) current injections in baseline conditions (blue) and following focal application of 5-HT (red). Action potentials are truncated. Black arrows indicate current step applications. (B) Average voltage responses following spike trains paired with 5-HT, resampled to 50 Hz and plotted as mean ± SEM, for 16 neurons in baseline conditions (blue) and after 5-HT (red). Gray symbols show responses following 5-HT application in a different group of COM neurons filled with 10 mM BAPTA (n = 6).
Role of Calcium in Serotonergic Excitation of COM Neurons
If the ADP current is the major contributor to serotonergic excitation in COM neurons, chelation of intracellular calcium with BAPTA should reduce or eliminate excitatory responses to 5-HT. Instead, addition of BAPTA (10 mM) to the pipette solution (n = 13) enhanced serotonergic excitation, with mean 5-HT response amplitudes being twice as large as in COM neurons patched with our standard intracellular solution (Figure 4). In control neurons (n = 16), peak increases in ISF were 113 ± 13% above baseline values, while in BAPTA-filled neurons (n = 13) peak ISF increases were 226 ± 23% above baseline values (p < 0.001 vs. control; Student’s t-test). While response integrals in these BAPTA-filled COM neurons (249 ± 56 Hz•s) were not significantly larger than those in control neurons (176 ± 26 Hz•s; p = 0.22; Figure 4C), in three additional independent experimental groups (see below) we observed significant enhancement of both amplitudes and integrals of 5-HT responses in BAPTA-filled neurons relative to controls. These findings demonstrate that serotonergic excitation, as a whole, is not calcium-dependent, and suggest that the calcium-dependent ADP may not represent the major ionic effector mediating 5-HT responses in COM neurons. Instead, the enhancement of responses in the presence of intracellular BAPTA suggests that intracellular calcium acts as a negative regulator for additional ionic effectors contributing to 2A-mediated excitation.
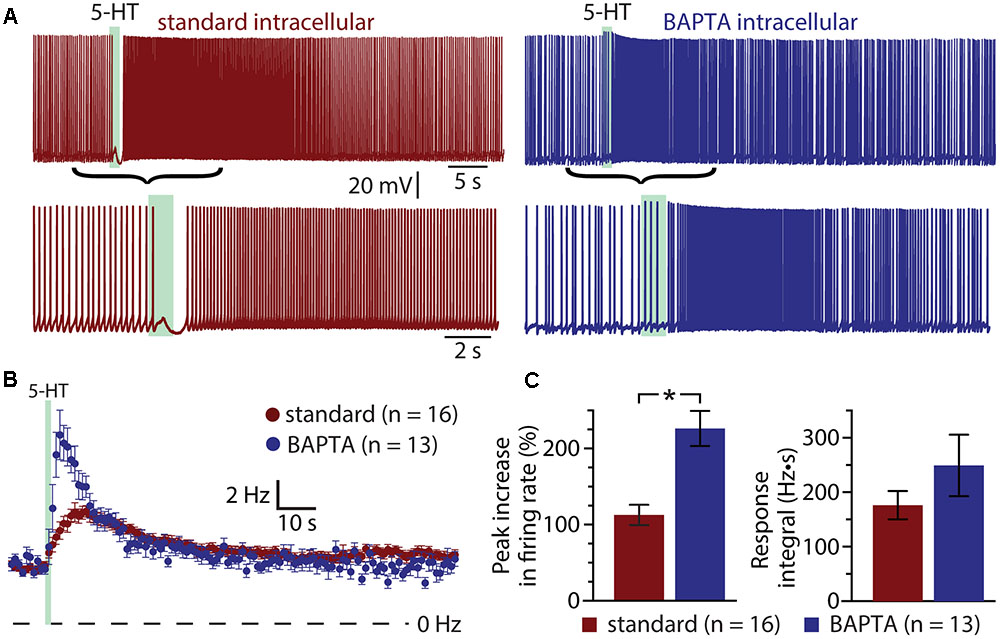
FIGURE 4. Serotonergic excitation of COM neurons is not calcium-dependent. (A) Serotonergic responses in COM neurons recorded with standard intracellular solution (red) or with a solution containing 10 mM BAPTA (blue). Lower traces show the responses at a faster timescale. (B) Plots of mean (±SEM) instantaneous spike frequencies in control (red; n = 16) and BAPTA-containing (blue; n = 13) COM neurons. (C) Comparisons of the peak increase in instantaneous spike frequencies (left) and response integrals (right) for COM neurons recorded in control (red) or BAPTA (blue) conditions.
If activity- and/or 5-HT-driven calcium entry negatively regulates serotonergic excitation of COM neurons, removal of extracellular calcium should enhance 5-HT responses in a manner similar to inclusion of intracellular BAPTA. To test this possibility, we measured responses to 5-HT in COM neurons in baseline conditions and after replacing extracellular calcium with magnesium (see Materials and Methods; Figure 5). Successful elimination of extracellular calcium was verified in a subset of neurons (n = 4) by measuring electrically evoked EPSPs before, during, and after calcium replacement (Figure 5A). While removal of extracellular calcium eliminated synaptic responses, it did not affect the magnitude or integrals of serotonergic responses (n = 9; Figures 5B–D). Peak increases in ISFs were 78 ± 11% and 75 ± 12% above initial firing frequencies in baseline and calcium-free conditions, respectively (p = 0.83, paired Student’s t-test), while response integrals for the two conditions were 141 ± 29 Hz•s and 164 ± 44 Hz•s, respectively (p = 0.58). This consistency in 5-HT responses across experimental conditions occurred in spite of a small (1.5 ± 0.6 Hz; p = 0.043, paired Student’s t-test) increase in baseline firing frequency that would otherwise predict a 22 ± 9% decrease in peak excitation (see Figure 1 and Table 2). Thus, with normal intracellular calcium signaling intact, removal of extracellular calcium had little, if any, effect on 5-HT response amplitudes or integrals.
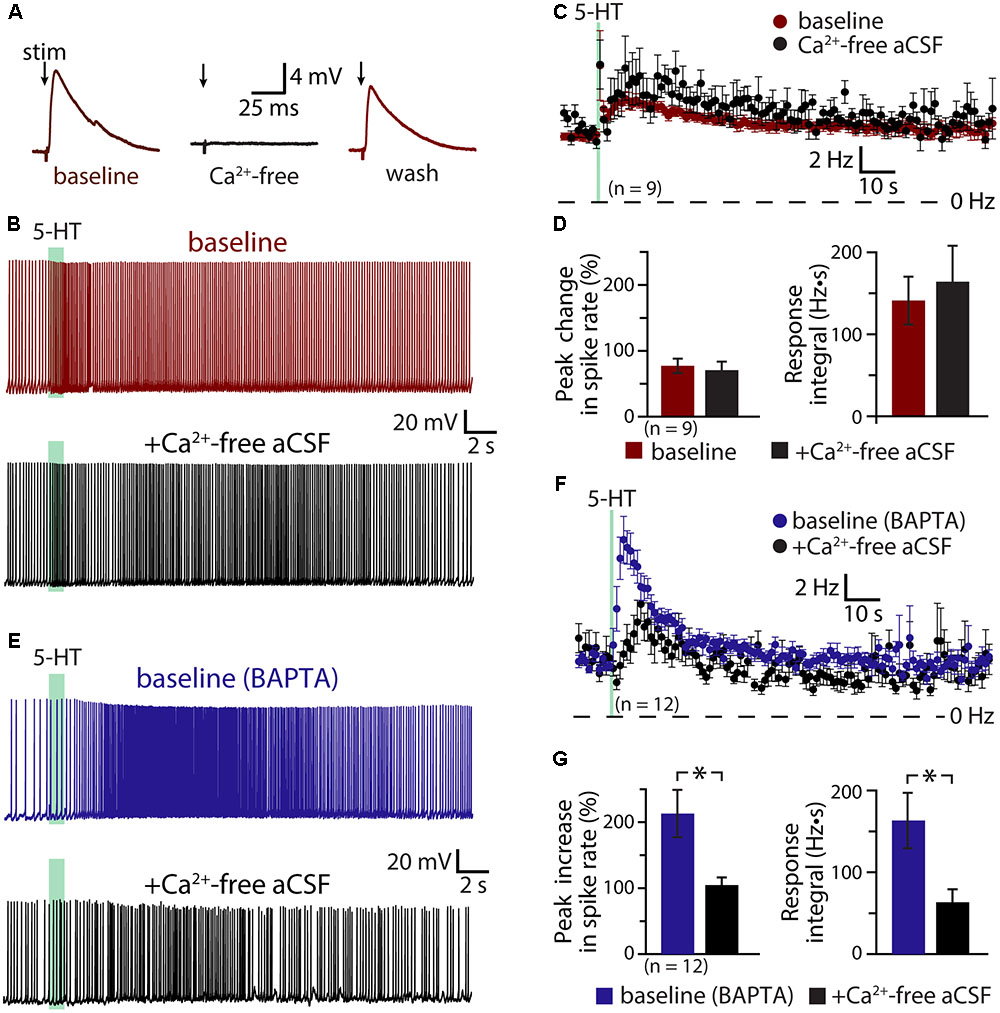
FIGURE 5. Serotonergic excitation involves a calcium conductance. (A) Electrically evoked EPSPs (indicated by arrows) in a COM neuron in baseline conditions (red), after replacement of extracellular calcium with magnesium (black), and after return to normal calcium conditions (red). (B) Serotonergic responses in a COM neuron in control conditions (red trace) and after replacement of extracellular calcium with magnesium (black trace). Green bars indicate duration of 5-HT application. (C) Plots of mean instantaneous spike frequencies in baseline (red) and zero-calcium (black) conditions for 9 COM neurons. (D) Comparisons of peak increases in instantaneous spike frequencies (left) and response integrals (right) for COM neurons before (red) and after (black) removal of extracellular calcium. (E) Responses to 5-HT (green bars) in COM neurons recorded with 10 mM BAPTA in the whole-cell pipette, before (blue) and after (black) removal of extracellular calcium. (F) Plots of mean (±SEM) instantaneous spike frequencies for 12 BAPTA-filled COM neuron in baseline (blue) and calcium-free (black) conditions. (G) Comparisons of 5-HT response amplitudes (left) and integrals (right) in BAPTA-filled COM neurons in baseline (blue) and calcium-free (black) conditions.
The lack of effect of extracellular calcium removal on 5-HT responses likely reflects competing cellular processes. For instance, even as removal of calcium may enhance some 5-HT-gated conductances, it will also eliminate the calcium-dependent ADP conductance and the calcium component of any calcium-permeable cation conductances contributing to excitation. To further explore the impact of extracellular calcium on serotonergic signaling in COM neurons, we filled additional neurons with BAPTA (10 mM) and measured serotonergic responses before and after replacement of extracellular calcium with magnesium (Figures 5E,F). As in our earlier experiments, the presence of intracellular BAPTA led to robust serotonergic excitation in baseline (i.e., normal extracellular calcium) conditions, with peak increases in ISF being 213 ± 36% above initial firing rates, and response integrals being 163 ± 34 Hz•s (n = 12). Subsequent removal of extracellular calcium greatly diminished both peak excitation (to 105 ± 11%; p = 0.007, paired Student’s t-test) and response integrals (to 63 ± 16 Hz•s; p = 0.004, Figure 5G). Because intracellular calcium signaling was blocked with BAPTA throughout these experiments, the reduction in response amplitudes and integrals observed after removal of extracellular calcium cannot be explained by effects on the ADP or other calcium-dependent conductances. Instead, these results point to involvement of a calcium-permeable cation conductance that, under normal conditions, is suppressed by intracellular calcium, but which is enhanced after chelation of intracellular calcium. Because responses in the presence of both intracellular BAPTA (which itself doubles 5-HT response amplitudes) and calcium-free aCSF (which in the absence of BAPTA has no effect on 5-HT responses amplitudes) results in responses comparable in size to those in control conditions (i.e., with normal intracellular and extracellular calcium levels), this calcium-sensitive calcium conductance appears to be balanced with the ADP and other calcium-dependent conductances gated by 2A receptors.
Role of M-current in Serotonergic Excitation
A classic ionic effector associated with Gq-coupled receptors that may exhibit calcium-sensitivity is the M- (“muscarinic”) current mediated by voltage-dependent KV7 (KCNQ) potassium channels (Delmas and Brown, 2005). Does M-current contribute to serotonergic excitation of COM neurons? If so, does intracellular calcium reduce the contribution of M-current to excitatory responses? To test the role of M-current suppression in generating serotonergic excitation, we measured 5-HT responses in COM neurons before and after bath applying the selective M-current blocker XE991 (10 μM; Figures 6A,B). XE991 depolarized RMPs, from -80 ± 2 to -76 ± 1 mV (n = 10; p = 0.014, paired Student’s t-test), and increased input resistances (RN), from 155 ± 14 to 185 ± 16 MΩ (p = 0.004, paired Student’s t-test), indicating that the M-current contributes to the resting membrane conductance of layer 5 COM neurons in the mouse mPFC. XE991 also reduced both the magnitude (by 49 ± 5%) and integral (by 56 ± 15%) of serotonergic excitation. Peak increases in ISF in response to 5-HT dropped from 94 ± 15% in baseline conditions to 50 ± 11% after application of XE991 (p = 0.001, paired Student’s t-test), while response integrals dropped from 132 ± 23 Hz•s to 48 ± 16 Hz•s (p = 0.009, Figures 6E,F). The substantial reduction in response amplitudes and integrals in the presence of XE991 suggests that suppression of the M-current is a key mediator of serotonergic excitation in COM neurons.
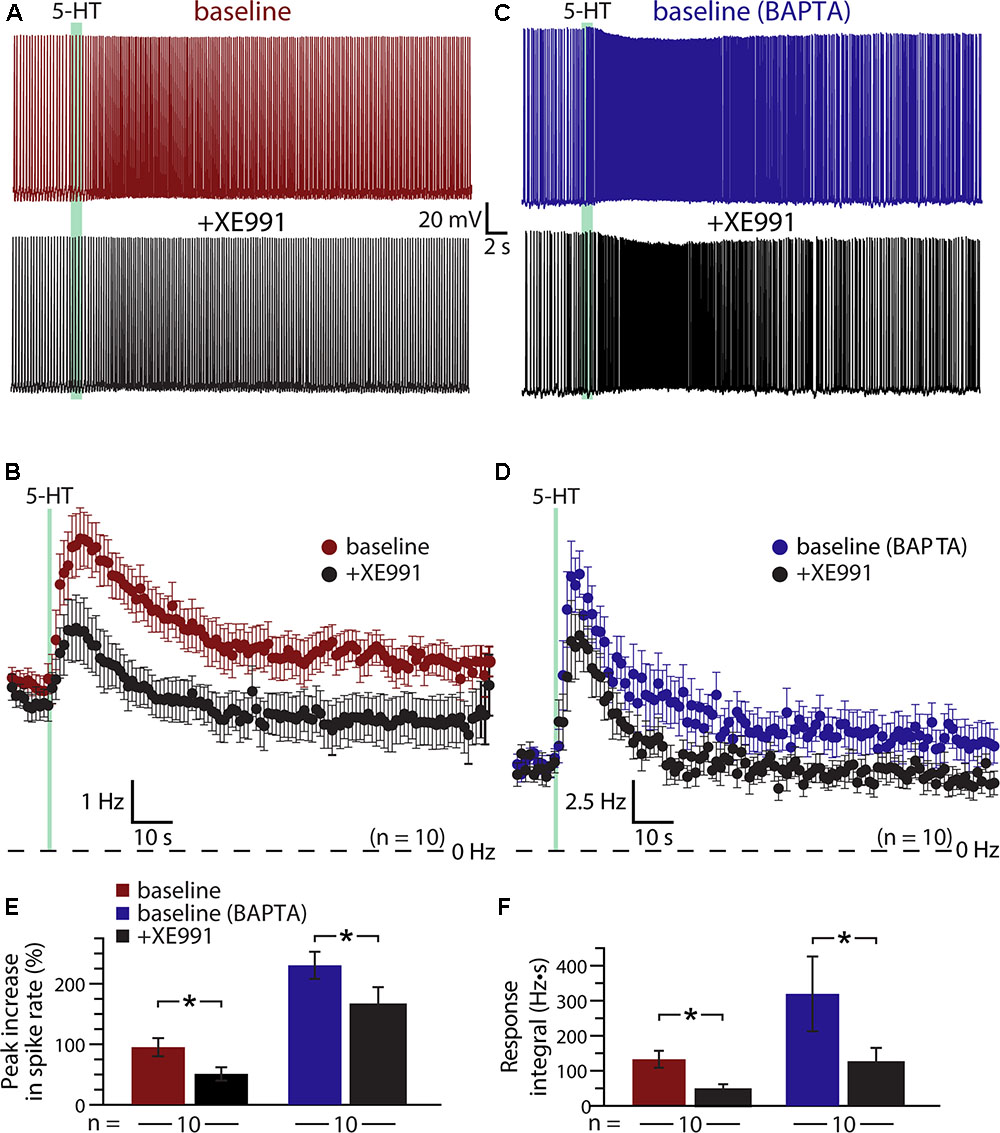
FIGURE 6. Suppression of KV7 channels contributes to serotonergic excitation of COM neurons. (A,C) Responses to 5-HT (green bars) in a control COM neuron (red, A) and a COM neuron patched with 10 mM BAPTA (blue, C) in baseline conditions and after blockade of KV7 channels with 10 μM XE991 (black). (B,D) Plots of mean instantaneous spike frequencies for 10 control (B) and 10 BAPTA-filled (D) COM neurons in baseline (red or blue) and XE991 (black) conditions. (E,F) Comparisons of peak increases in instantaneous spike frequencies (E) and response integrals (F) in baseline conditions (red or blue) and after addition of XE991 (black) for control and BAPTA-filled COM neurons, respectively.
If calcium sensitivity of M-current (see, for instance, Selyanko and Brown, 1996) accounts for the enhanced serotonergic excitation observed after intracellular calcium chelation, 5-HT responses after blockade of KV7 channels should be similar in both control and BAPTA-filled neurons. We tested this prediction by recording from a second group of neurons (n = 10) filled with 10 mM BAPTA (Figures 6C,D). Consistent with our earlier experiments using intracellular BAPTA, baseline responses to 5-HT were much larger in amplitude (mean increase in ISF was 230 ± 22%; p < 0.001 vs. neurons lacking BAPTA; Student’s t-test, n = 10 per group), and integral (319 ± 107 Hz•s; p = 0.10) in BAPTA-filled neurons relative to responses in neurons with intracellular calcium signaling intact. However, intracellular BAPTA did not enhance the impact of KV7 blockade with 10 μM XE991. With BAPTA present, serotonergic excitation in XE991 (+164 ± 30% peak increase in ISF) remained three times larger than in control neurons treated with XE991 (p = 0.002, Student’s t-test; Figures 6E,F). However, mean integrals (122 ± 43 Hz•s), while generally larger than in control neurons treated with XE991, were not statistically different in magnitude (p = 0.13). Overall, peak response amplitudes were reduced by 29 ± 9% (p = 0.022 vs. baseline responses; paired Student’s t-test) and integrals by 57 ± 11% (p = 0.024 vs. baseline responses) in BAPTA-filled neurons. Thus, the XE991-sensitive portions of serotonin responses in control and BAPTA recording conditions were similar, suggesting that M-current alone does not account for the calcium-sensitivity of 5-HT excitation in COM neurons.
The results above suggest that 5-HT acts via at least three distinct mechanisms (KV7 suppression, the ADP conductance, and a calcium-sensitive calcium conductance) to enhance the excitability of COM neurons. To test whether M-current is the dominant potassium conductance contributing to serotonergic excitation, we enhanced the driving force for potassium by lowering the external potassium concentration ([K+]o) six-fold to 0.5 mM (replaced with equimolar sodium; Figure 7). By increasing the driving force for potassium, this manipulation will enhance the impact of M-current suppression by 5-HT, but will also act to reduce the net current through potassium-permeable non-specific cation conductances. In neurons recorded with control intracellular solution, lowering [K+]o revealed a brief inhibition occurring immediately after 5-HT application that was absent in control conditions (Figures 7A,C); these inhibitory responses are likely Gq-driven hyperpolarizations (mediated by SK-type potassium channels) that occur regularly in pyramidal neurons following M1 muscarinic receptor activation (Gulledge et al., 2009), but which are only rarely observed in response to 5-HT in control conditions. Lowering [K+]o enhanced this early potassium conductance, and reduced the magnitude of serotonergic excitation by 31 ± 9% (n = 10, paired). In control conditions (e.g., 3 mM [K+]o), 5-HT generated peak responses of 82 ± 14% with integrals of 157 ± 44 Hz•s. After reducing extracellular potassium to 0.5 mM, peak excitation was 61 ± 15% (p = 0.003 relative to control conditions) with integrals of 117 ± 47 Hz•s (p = 0.057, Figure 7D). Because the larger driving force for potassium is expected to increase 5-HT excitation by enhancing the contribution of M-current suppression, the observed reductions in response magnitudes and integrals suggest the participation of potassium-permeable non-specific cation conductances, such as the ADP conductance (Haj-Dahmane and Andrade, 1998).
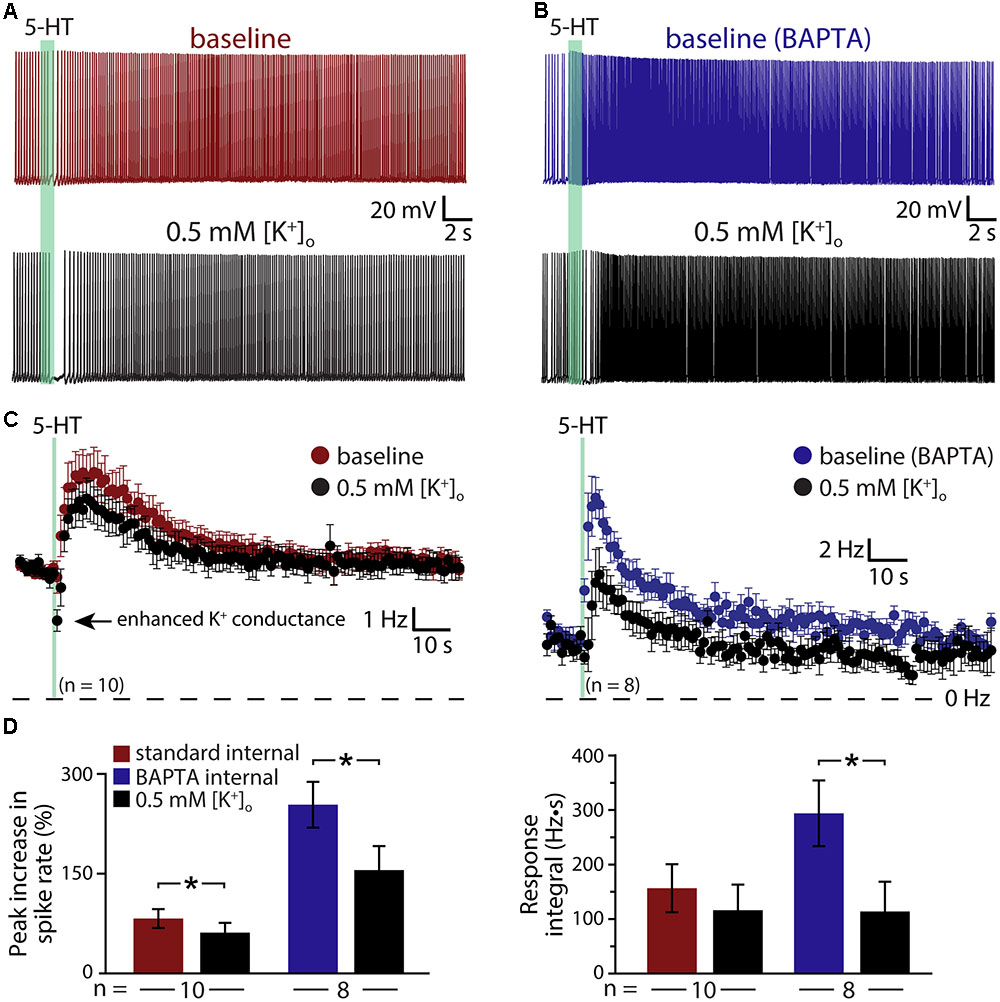
FIGURE 7. Serotonergic excitation of COM neurons involves activation of non-selective cation conductances. (A,B) Responses to 5-HT (green bars) in baseline conditions and after reducing extracellular potassium to 0.5 mM (black traces) in control (red, A) or BAPTA-filled (10 mM, blue, B) COM neurons. (C) Plots of mean instantaneous spike frequencies for COM neurons in baseline (red or blue) and low [K+]o (black) conditions for neurons recorded with standard intracellular solution or with BAPTA included, respectively. (D) Comparisons of peak increases in instantaneous spike frequencies (left) and response integrals (right) in baseline conditions (red or blue) and after lowering [K+]o to 0.5 mM (black).
To further test the impact of potassium manipulations on 5-HT responses in the absence of the non-specific cation conductance underlying the ADP, and calcium-activated potassium conductances, we repeated experiments in COM neurons filled with 10 mM BAPTA (Figures 7B,C). With intracellular calcium chelated, baseline responses were again large, with 254 ± 34% increases in ISF and 295 ± 61 Hz•s response integrals (n = 8). When [K+]o was lowered to 0.5 mM, response amplitudes were reduced by 42 ± 7% (to 156 ± 36% above baseline firing rates; p < 0.001; paired Student’s t-test), while response integrals were decreased by 63 ± 12% (to 114 ± 55 Hz•s; p = 0.029; Figure 7D). The percent reductions in both amplitudes (p = 0.342) and integrals (p = 0.559) after lowering [K+]o in BAPTA filled neurons were similar to those observed in control neurons, suggesting that the calcium-sensitive conductance enhanced by intracellular BAPTA must also be a potassium-permeable non-specific cation conductance.
Discussion
Activation of Gq-coupled receptors, including serotonergic 2A and muscarinic M1 receptors, enhances the intrinsic excitability of many neurons throughout the nervous system. As common and robust as these responses are, it is surprising that there is not yet consensus on their underlying mechanisms. Data regarding cortical 2A signaling are particularly limited, in part due to the selective expression of these receptors in subpopulations of cortical neurons in adult animals (Weber and Andrade, 2010; Avesar and Gulledge, 2012). Therefore, much of our understanding of 2A receptor signaling has been inferred from studies of the more ubiquitous Gq-coupled muscarinic ACh receptors. Here, we selectively targeted 2A-responsive COM neurons to probe the mechanisms underlying serotonergic excitation. Our results confirm that 2A and M1 receptors share downstream signaling pathways, and point to at least three distinct ionic effectors contributing to serotonergic excitation: suppression of KV7 conductances, activation of a non-specific cation channel that is both calcium-sensitive and calcium-permeable, and activation of the calcium-dependent non-specific cation conductance responsible for the ADP (Figure 8).
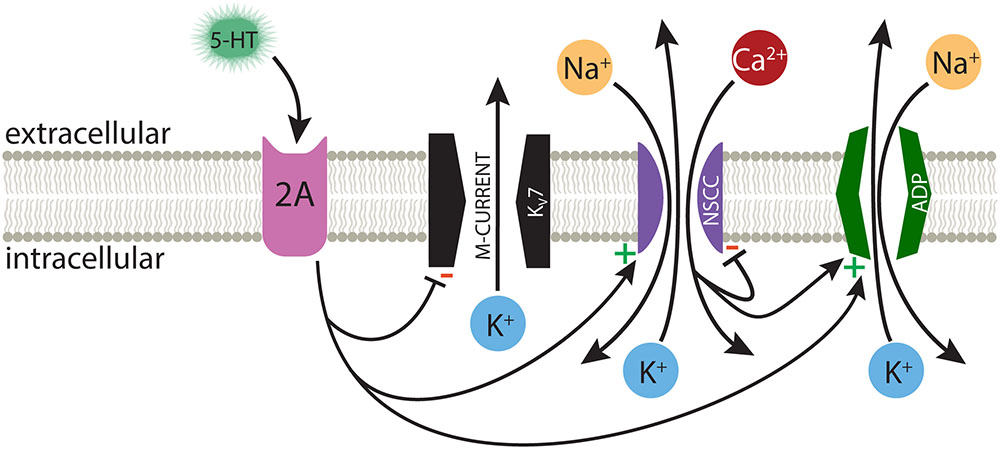
FIGURE 8. Ionic effectors contributing to serotonergic excitation of COM neurons. Activation of Gq-coupled 5-HT2A receptors in COM neurons targets a range of ionic effectors, including suppression of M-current, calcium-dependent activation of the ADP current, and activation of a calcium-sensitive, but calcium-permeable, non-specific cation conductance (NSCC).
Ionic Conductances Contributing to Serotonergic Excitation of COM Neurons
Both M-current suppression (Caulfield et al., 1994) and activation of non-specific cation conductances (Haj-Dahmane and Andrade, 1996) have been proposed as mechanisms for Gq-mediated excitation. We found that under control conditions, with intracellular calcium signaling intact, suppression of M-current accounted for about half of the total serotonergic response. While KV7 channels are reported to be calcium-sensitive (Selyanko and Brown, 1996), the impact of M-current suppression was similar in control neurons and those filled with BAPTA, with responses to 5-HT in the presence of both BAPTA and XE991 remaining much larger than responses measured in control conditions with intracellular calcium signaling and M-current intact. This suggests that the calcium-sensitivity of KV7 channels cannot account for the larger 5-HT responses observed after chelation of intracellular calcium.
We also confirmed that 5-HT generates calcium-dependent ADPs in COM neurons. However, quantifying the relative contribution of the ADP conductance to enhanced action potential generation is problematic, as the ion channel mediating the ADP remains a matter of debate (Kuzmiski and MacVicar, 2001; Yan et al., 2009; Dasari et al., 2013; Lei et al., 2014), and attempts to block the conductance with intracellular BAPTA, or by removal of extracellular calcium, paradoxically enhanced the magnitude of serotonergic excitation. Indeed, in four independent experimental groups, baseline responses to 5-HT in the presence of intracellular BAPTA were twice as large as responses in control neurons with normal intracellular calcium signaling. These findings demonstrate that the ADP conductance is not required for serotonergic excitation, and that the net effect of normal levels of intracellular calcium is moderation, rather than amplification, of serotonergic excitation.
Two observations suggest that the calcium-sensitivity of 5-HT responses reflects a non-specific cation conductance that itself is calcium permeable. First, the involvement of non-specific cation conductances is strongly suggested by the effect of reducing extracellular potassium. While this manipulation enhances selective potassium currents (e.g., the M-current), it also lowers the equilibrium potential for potassium-permeable non-specific cation conductances. Thus, the reduction in serotonergic excitation observed after lowering extracellular potassium suggests the involvement of non-specific cation conductances, such as the ADP conductance (Haj-Dahmane and Andrade, 1998). Since we continued to observe a reduction in response amplitudes after ADP currents were blocked by BAPTA, the remaining calcium-sensitive calcium conductance, which was amplified by chelation of intracellular calcium, is likely also a potassium-permeable non-specific cation conductance. Second, we found that the effect of removing extracellular calcium was dependent on the baseline state of intracellular calcium signaling within neurons. In control conditions, with calcium- signaling intact, removal of extracellular calcium had no impact on 5-HT response amplitudes or integrals. Yet, when intracellular calcium signaling was blocked with BAPTA, and baseline responses to 5-HT were twice as large as in control neurons, removal of extracellular calcium greatly reduced the amplitudes and integrals of 5-HT responses, such that they became comparable in size to control 5-HT responses observed in the absence of BAPTA. These findings suggest the involvement of a calcium-permeable non-specific cation conductance that is itself suppressed by intracellular calcium (Figure 8). Under normal conditions, with intracellular calcium signaling intact, removal of extracellular calcium is expected to have two opposing effects: it will eliminate a charge carrier for the calcium-permeable non-specific cation conductance, thereby hyperpolarizing the effective equilibrium potential for the conductance, but will also enhance the maximum conductance by relieving the channel from negative regulation by intracellular calcium. Our results suggest these two competing influences are fairly balanced with the calcium-dependent ADP conductance, such that calcium-removal in control conditions has little impact on serotonergic responses. However, when the conductance is maximized by chelating intracellular calcium with BAPTA, subsequent removal of extracellular calcium acts only to reduce net current, such that 5-HT responses become smaller and equivalent to responses observed in control conditions.
These findings regarding calcium regulation of serotonergic excitation are most comparable to those of Magistretti et al. (2004), who found Gq-mediated cholinergic responses in layer 2 pyramidal neurons in the entorhinal cortex to be bidirectionally influenced by calcium influx. They found that modest increases in intracellular calcium enhanced net inward current (attributed to a calcium-dependent ADP-like conductance), while larger increases in intracellular calcium, including normal levels of calcium accumulation during periods of action potential generation, acted to suppress cholinergic excitation (Magistretti et al., 2004). While Magistretti et al. (2004) concluded that their results reflected bidirectional calcium regulation of the ADP conductance, results from our experiments using BAPTA and calcium-free aCSF dissociate the calcium-activated ADP conductance from the additional calcium-sensitive non-specific cation conductance. Co-activation of two non-specific cation conductances with opposing calcium regulation may contribute to the robustness of Gq-mediated excitation by maintaining a consistent net current amplitude independent of intracellular calcium levels, while also promoting calcium-influx when intracellular calcium levels are low. This negative feedback of intracellular calcium on the calcium-permeable conductance should act to stabilize intracellular calcium levels, and could provide a mechanism for calcium store refilling after Gq receptor signaling (Dasari et al., 2017).
Gq-Signaling in Cortical Projection Neurons
The ionic mechanisms contributing to serotonergic excitation of COM neurons appear to be conserved across cortical neuron subtypes and transmitter signaling systems. We have recently found that phasic activation of the Gq-coupled M1 muscarinic ACh receptor enhances the excitability of pyramidal neurons in layer 5 of the mouse mPFC, an enhancement more robust and longer lasting in corticopontine (CPn) neurons relative to COM neurons (Baker et al., 2018; see also Dembrow et al., 2010). Further investigation of the mechanisms underlying this robust excitation in CPn neurons revealed that the M1 mAChR pathway involves the same combination of ionic effectors (M-current suppression, the ADP conductance, and a calcium-sensitive and calcium-permeable non-specific cation conductance) as described here in relation to serotonergic 2A signaling in COM neurons.
Some notable differences do exist, however, in Gq-mediated excitation of COM and CPn neurons by 5-HT and ACh, respectively. For instance, although stimulation of muscarinic ACh receptors robustly generated transient SK-channel mediated inhibition in both COM and CPn neurons (Baker et al., 2018), in normal conditions (3 mM [K+]o) we rarely observed 5-HT-triggered SK-dependent inhibition in mouse COM neurons, even as ACh and 5-HT generate similar increases in ISF (∼100% above baseline levels) in these same neurons. Even after enhancing the driving force for potassium channels with lowered extracellular potassium, SK-like responses to 5-HT were observed in only 7 of 10 neurons, and had shorter durations (0.7 ± 0.2 s) than SK responses generated by ACh in either COM (∼1.5 s) or CPn (∼1 s) neurons (Baker et al., 2018). This may reflect differential subcellular localization of M1 muscarinic receptors relative to serotonergic 2A receptors in COM neurons, or subtle differences in Gq-coupling to second messenger systems. Another difference is the relative contribution of ionic effectors in generating excitatory responses. In CPn neurons, suppression of M-current accounted for only ∼20% of total cholinergic excitation, while chelation of intracellular calcium enhanced responses by only ∼25% (Baker et al., 2018). This suggests that the ADP conductance may play a larger role in mediating cholinergic excitation in CPn neurons relative to COM neurons. Thus, M-current suppression and the calcium-sensitive non-specific cation conductance appear more important for serotonergic excitation of COM neurons, relative to cholinergic excitation of CPn neurons.
In addition to 2A and M1 receptors, neocortical pyramidal neurons express a wide variety of aminergic, glutamatergic, purinergic, and peptidergic receptors that couple to Gq alpha subunits. Our results reveal multiple ionic effectors contributing to serotonergic 2A signal transduction in COM neurons, and point to significant overlap of 2A signaling with M1 muscarinic receptors in these same neurons (see also Baker et al., 2018), suggesting that a common set of ionic effectors is targeted by a wide variety of transmitter systems. While parallel Gq signaling cascades enhance the fidelity and robustness of excitatory responses, targeted expression of Gq-coupled receptors and/or downstream ionic effectors in specific cellular compartments may allow fine-tuning of local responses to neuromodulatory input. Expression of additional, non-Gq-coupled receptors (e.g., 5-HT1A receptors), and the potential formation of heteromeric receptor complexes (McCormick and Botta, 2017), further adds to the diversity of Gq signaling. A detailed understanding of Gq signaling in the cerebral cortex will clarify our understanding of how neuromodulators facilitate cognition, and, especially in regard to 2A receptors, may facilitate development of pharmacological interventions for psychiatric disorders that are more efficacious and have fewer side effects than approaches that target G-protein-coupled receptors directly.
Ethics Statement
This study was carried out in accordance with the recommendations of the Association for Assessment and Accreditation of Laboratory Animal Care International (AAALAC). The protocol was approved by the Institutional Animal Care and Use Committee of Dartmouth College.
Author Contributions
AG and ES designed the research. AG, AB, and ES conducted the research and analyzed the data. AG, ES, and AB wrote the manuscript.
Funding
This work was supported by a grant from the National Institute for Mental Health (R01 MH099054; AG) and a Frank and Myra Weiser Scholar Award, AG.
Conflict of Interest Statement
The authors declare that the research was conducted in the absence of any commercial or financial relationships that could be construed as a potential conflict of interest.
Acknowledgments
The authors thank Saiko Ikeda for technical assistance.
References
Almada, R. C., Coimbra, N. C., and Brandao, M. L. (2015). Medial prefrontal cortex serotonergic and GABAergic mechanisms modulate the expression of contextual fear: intratelencephalic pathways and differential involvement of cortical subregions. Neuroscience 284, 988–997. doi: 10.1016/j.neuroscience.2014.11.001
Andrade, R. (1991). Cell excitation enhances muscarinic cholinergic responses in rat association cortex. Brain Res. 548, 81–93. doi: 10.1016/0006-8993(91)91109-E
Andrade, R., and Nicoll, R. A. (1987). Pharmacologically distinct actions of serotonin on single pyramidal neurones of the rat hippocampus recorded in vitro. J. Physiol. 394, 99–124. doi: 10.1113/jphysiol.1987.sp016862
Araneda, R., and Andrade, R. (1991). 5-Hydroxytryptamine2 and 5-hydroxytryptamine 1A receptors mediate opposing responses on membrane excitability in rat association cortex. Neuroscience 40, 399–412. doi: 10.1016/0306-4522(91)90128-B
Avesar, D., and Gulledge, A. T. (2012). Selective serotonergic excitation of callosal projection neurons. Front. Neural Circuits 6:12. doi: 10.3389/fncir.2012.00012
Azmitia, E. C., Gannon, P. J., Kheck, N. M., and Whitaker-Azmitia, P. M. (1996). Cellular localization of the 5-HT1A receptor in primate brain neurons and glial cells. Neuropsychopharmacology 14, 35–46. doi: 10.1016/S0893-133X(96)80057-1
Baker, A. L., O’Toole, R. J., and Gulledge, A. T. (2018). Preferential cholinergic excitation of corticopontine neurons. J. Physiol. (in Press). doi: 10.1113/JP275194
Caulfield, M. P., Jones, S., Vallis, Y., Buckley, N. J., Kim, G. D., Milligan, G., et al. (1994). Muscarinic M-current inhibition via G alpha q/11 and alpha-adrenoceptor inhibition of Ca2+ current via G alpha o in rat sympathetic neurones. J. Physiol. 477, 415–422. doi: 10.1113/jphysiol.1994.sp020203
Clarke, H. F., Dalley, J. W., Crofts, H. S., Robbins, T. W., and Roberts, A. C. (2004). Cognitive inflexibility after prefrontal serotonin depletion. Science 304, 878–880. doi: 10.1126/science.1094987
Clarke, H. F., Walker, S. C., Crofts, H. S., Dalley, J. W., Robbins, T. W., and Roberts, A. C. (2005). Prefrontal serotonin depletion affects reversal learning but not attentional set shifting. J. Neurosci. 25, 532–538. doi: 10.1523/JNEUROSCI.3690-04.2005
Colino, A., and Halliwell, J. V. (1987). Differential modulation of three separate K-conductances in hippocampal CA1 neurons by serotonin. Nature 328, 73–77. doi: 10.1038/328073a0
Cruz, D. A., Eggan, S. M., Azmitia, E. C., and Lewis, D. A. (2004). Serotonin1A receptors at the axon initial segment of prefrontal pyramidal neurons in schizophrenia. Am. J. Psychiatry 161, 739–742. doi: 10.1176/appi.ajp.161.4.739
Czyrak, A., Czepiel, K., Mackowiak, M., Chocyk, A., and Wedzony, K. (2003). Serotonin 5-HT1A receptors might control the output of cortical glutamatergic neurons in rat cingulate cortex. Brain Res. 989, 42–51. doi: 10.1016/S0006-8993(03)03352-3
Dasari, S., Abramowitz, J., Birnbaumer, L., and Gulledge, A. T. (2013). Do canonical transient receptor potential channels mediate cholinergic excitation of cortical pyramidal neurons? Neuroreport 24, 550–554. doi: 10.1097/WNR.0b013e3283621344
Dasari, S., and Gulledge, A. T. (2011). M1 and M4 receptors modulate hippocampal pyramidal neurons. J. Neurophysiol. 105, 779–792. doi: 10.1152/jn.00686.2010
Dasari, S., Hill, C., and Gulledge, A. T. (2017). A unifying hypothesis for M1 muscarinic receptor signalling in pyramidal neurons. J. Physiol. 595, 1711–1723. doi: 10.1113/JP273627
Davies, M. F., Deisz, R. A., Prince, D. A., and Peroutka, S. J. (1987). Two distinct effects of 5-hydroxytryptamine on single cortical neurons. Brain Res. 423, 347–352. doi: 10.1016/0006-8993(87)90861-4
DeFelipe, J., Arellano, J. I., Gomez, A., Azmitia, E. C., and Munoz, A. (2001). Pyramidal cell axons show a local specialization for GABA and 5-HT inputs in monkey and human cerebral cortex. J. Comp. Neurol. 433, 148–155. doi: 10.1002/cne.1132
Delmas, P., and Brown, D. A. (2005). Pathways modulating neural KCNQ/M (Kv7) potassium channels. Nat. Rev. Neurosci. 6, 850–862. doi: 10.1038/nrn1785
Dembrow, N. C., Chitwood, R. A., and Johnston, D. (2010). Projection-specific neuromodulation of medial prefrontal cortex neurons. J. Neurosci. 30, 16922–16937. doi: 10.1523/JNEUROSCI.3644-10.2010
Egorov, A. V., Angelova, P. R., Heinemann, U., and Müller, W. (2003). Ca2+-independent muscarinic excitation of rat medial entorhinal cortex layer V neurons. Eur. J. Neurosci. 18, 3343–3351. doi: 10.1111/j.1460-9568.2003.03050.x
Goodfellow, N. M., Benekareddy, M., Vaidya, V. A., and Lambe, E. K. (2009). Layer II/III of the prefrontal cortex: Inhibition by the serotonin 5-HT1A receptor in development and stress. J. Neurosci. 29, 10094–10103. doi: 10.1523/JNEUROSCI.1960-09.2009
Gulledge, A. T., Bucci, D. J., Zhang, S. S., Matsui, M., and Yeh, H. H. (2009). M1 receptors mediate cholinergic modulation of excitability in neocortical pyramidal neurons. J. Neurosci. 29, 9888–9902. doi: 10.1523/JNEUROSCI.1366-09.2009
Haj-Dahmane, S., and Andrade, R. (1996). Muscarinic activation of a voltage-dependent cation nonselective current in rat association cortex. J. Neurosci. 16, 3848–3861.
Haj-Dahmane, S., and Andrade, R. (1998). Ionic mechanism of the slow afterdepolarization induced by muscarinic receptor activation in rat prefrontal cortex. J. Neurophysiol. 80, 1197–1210. doi: 10.1152/jn.1998.80.3.1197
Haj-Dahmane, S., and Andrade, R. (1999). Muscarinic receptors regulate two different calcium-dependent non-selective cation currents in rat prefrontal cortex. Eur. J. Neurosci. 11, 1973–1980. doi: 10.1046/j.1460-9568.1999.00612.x
Harrison, A. A., Everitt, B. J., and Robbins, T. W. (1997). Central 5-HT depletion enhances impulsive responding without affecting the accuracy of attentional performance: interactions with dopaminergic mechanisms. Psychopharmacology (Berl.) 133, 329–342. doi: 10.1007/s002130050410
Jakab, R. L., and Goldman-Rakic, P. S. (1998). 5-Hydroxytryptamine2A serotonin receptors in the primate cerebral cortex: possible site of action of hallucinogenic and antipsychotic drugs in pyramidal cell apical dendrites. Proc. Natl. Acad. Sci. U.S.A. 95, 735–740. doi: 10.1073/pnas.95.2.735
Krnjevic, K., Pumain, R., and Renaud, L. (1971). The mechanism of excitation by acetylcholine in the cerebral cortex. J. Physiol. 215, 247–268. doi: 10.1113/jphysiol.1971.sp009467
Kuzmiski, J. B., and MacVicar, B. A. (2001). Cyclic nucleotide-gated channels contribute to the cholinergic plateau potential in hippocampal CA1 pyramidal neurons. J. Neurosci. 21, 8707–8714.
Lei, Y. T., Thuault, S. J., Launay, P., Margolskee, R. F., Kandel, E. R., and Siegelbaum, S. A. (2014). Differential contribution of TRPM4 and TRPM5 nonselective cation channels to the slow afterdepolarization in mouse prefrontal cortex neurons. Front. Cell. Neurosci. 8:267. doi: 10.3389/fncel.2014.00267
Leon, L. A., Castro-Gomes, V., Zarate-Guerrero, S., Corredor, K., Mello Cruz, A. P., Brandao, M. L., et al. (2017). Behavioral effects of systemic, infralimbic and prelimbic injections of a serotonin 5-HT2A antagonist in carioca high- and low-conditioned freezing rats. Front. Behav. Neurosci. 11:117. doi: 10.3389/fnbeh.2017.00117
Liy-Salmeron, G., and Meneses, A. (2008). Effects of 5-HT drugs in prefrontal cortex during memory formation and the ketamine amnesia-model. Hippocampus 18, 965–974. doi: 10.1002/hipo.20459
Luscher, C., Jan, L. Y., Stoffel, M., Malenka, R. C., and Nicoll, R. A. (1997). G protein-coupled inwardly rectifying K+ channels (GIRKs) mediate postsynaptic but not presynaptic transmitter actions in hippocampal neurons. Neuron 19, 687–695. doi: 10.1016/S0896-6273(00)80381-5
Magistretti, J., Ma, L., Shalinsky, M. H., Lin, W., Klink, R., and Alonso, A. (2004). Spike patterning by Ca2+-dependent regulation of a muscarinic cation current in entorhinal cortex layer II neurons. J. Neurophysiol. 92, 1644–1657. doi: 10.1152/jn.00036.2004
Marinho, A. L., Vila-Verde, C., Fogaca, M. V., and Guimaraes, F. S. (2015). Effects of intra-infralimbic prefrontal cortex injections of cannabidiol in the modulation of emotional behaviors in rats: contribution of 5HT(1)A receptors and stressful experiences. Behav. Brain Res. 286, 49–56. doi: 10.1016/j.bbr.2015.02.023
McCormick, D. A., and Williamson, A. (1989). Convergence and divergence of neurotransmitter action in human cerebral cortex. Proc. Natl. Acad. Sci. U.S.A. 86, 8098–8102. doi: 10.1073/pnas.86.20.8098
McCormick, P. J., and Botta, J. (2017). “Heteromers form novel signaling complexes,” in G-Protein-Coupled Receptor Dimers, eds K. Herrick-Davis, G. Milligan, and G. Di Giovanni (Cham: Springer), 467–475. doi: 10.1007/978-3-319-60174-8_19
Miner, L. A., Backstrom, J. R., Sanders-Bush, E., and Sesack, S. R. (2003). Ultrastructural localization of serotonin2A receptors in the middle layers of the rat prelimbic prefrontal cortex. Neuroscience 116, 107–117. doi: 10.1016/S0306-4522(02)00580-8
Paxinos, G., and Franklin, K. B. J. (2004). The Mouse Brain in Stereotaxic Coordinates. San Diego, CA: Elsevier.
Schwindt, P. C., Spain, W. J., Foehring, R. C., Chubb, M. C., and Crill, W. E. (1988). Slow conductances in neurons from cat sensorimotor cortex in vitro and their role in slow excitability changes. J. Neurophysiol. 59, 450–467. doi: 10.1152/jn.1988.59.2.450
Selyanko, A. A., and Brown, D. A. (1996). Intracellular calcium directly inhibits potassium M channels in excised membrane patches from rat sympathetic neurons. Neuron 16, 151–162. doi: 10.1016/S0896-6273(00)80032-X
Shalinsky, M. H., Magistretti, J., Ma, L., and Alonso, A. A. (2002). Muscarinic activation of a cation current and associated current noise in entorhinal-cortex layer-II neurons. J. Neurophysiol. 88, 1197–1211. doi: 10.1152/jn.2002.88.3.1197
Spain, W. J. (1994). Serotonin has different effects on two classes of Betz cells from the cat. J. Neurophysiol. 72, 1925–1937. doi: 10.1152/jn.1994.72.4.1925
Stephens, E. K., Avesar, D., and Gulledge, A. T. (2014). Activity-dependent serotonergic excitation of callosal projection neurons in the mouse prefrontal cortex. Front. Neural Circuits 8:97. doi: 10.3389/fncir.2014.00097
Tanaka, E., and North, R. A. (1993). Actions of 5-hydroxytryptamine on neurons of the rat cingulate cortex. J. Neurophysiol. 69, 1749–1757. doi: 10.1152/jn.1993.69.5.1749
Villalobos, C., Beique, J. C., Gingrich, J. A., and Andrade, R. (2005). Serotonergic regulation of calcium-activated potassium currents in rodent prefrontal cortex. Eur. J. Neurosci. 22, 1120–1126. doi: 10.1111/j.1460-9568.2005.04307.x
Villalobos, C., Foehring, R. C., Lee, J. C., and Andrade, R. (2011). Essential role for phosphatidylinositol 4,5-bisphosphate in the expression, regulation, and gating of the slow afterhyperpolarization current in the cerebral cortex. J. Neurosci. 31, 18303–18312. doi: 10.1523/JNEUROSCI.3203-11.2011
Wang, Z., and McCormick, D. A. (1993). Control of firing mode of corticotectal and corticopontine layer V burst-generating neurons by norepinephrine, acetylcholine, and 1S,3R-ACPD. J. Neurosci. 13, 2199–2216.
Weber, E. T., and Andrade, R. (2010). Htr2a gene and 5-HT2A receptor expression in the cerebral cortex studied using genetically modified mice. Front. Neurosci. 4:36. doi: 10.3389/fnins.2010.00036
Williams, G. V., Rao, S. G., and Goldman-Rakic, P. S. (2002). The physiological role of 5-HT2A receptors in working memory. J. Neurosci. 22, 2843–2854.
Willins, D. L., Deutch, A. Y., and Roth, B. L. (1997). Serotonin 5-HT2A receptors are expressed on pyramidal cells and interneurons in the rat cortex. Synapse 27, 79–82. doi: 10.1002/(SICI)1098-2396(199709)27:1<79::AID-SYN8<3.0.CO;2-A
Winstanley, C. A., Chudasama, Y., Dalley, J. W., Theobald, D. E., Glennon, J. C., and Robbins, T. W. (2003). Intra-prefrontal 8-OH-DPAT and M100907 improve visuospatial attention and decrease impulsivity on the five-choice serial reaction time task in rats. Psychopharmacology 167, 304–314. doi: 10.1007/s00213-003-1398-x
Xu, T., and Pandey, S. C. (2000). Cellular localization of serotonin2A (5HT2A) receptors in the rat brain. Brain Res. Bull. 51, 499–505. doi: 10.1016/S0361-9230(99)00278-6
Yan, H. D., Villalobos, C., and Andrade, R. (2009). TRPC channels mediate a muscarinic receptor-induced afterdepolarization in cerebral cortex. J. Neurosci. 29, 10038–10046. doi: 10.1523/JNEUROSCI.1042-09.2009
Keywords: serotonin, cortex, pyramidal neuron, 5-HT2A receptor, calcium, M-current, Kv7 channels, afterdepolarization
Citation: Stephens EK, Baker AL and Gulledge AT (2018) Mechanisms Underlying Serotonergic Excitation of Callosal Projection Neurons in the Mouse Medial Prefrontal Cortex. Front. Neural Circuits 12:2. doi: 10.3389/fncir.2018.00002
Received: 03 October 2017; Accepted: 04 January 2018;
Published: 18 January 2018.
Edited by:
Michael E. Hasselmo, Boston University, United StatesReviewed by:
John J. Woodward, Medical University of South Carolina, United StatesLaura Schrader, Tulane University, United States
Copyright © 2018 Stephens, Baker and Gulledge. This is an open-access article distributed under the terms of the Creative Commons Attribution License (CC BY). The use, distribution or reproduction in other forums is permitted, provided the original author(s) or licensor are credited and that the original publication in this journal is cited, in accordance with accepted academic practice. No use, distribution or reproduction is permitted which does not comply with these terms.
*Correspondence: Allan T. Gulledge, YWxsYW4uZ3VsbGVkZ2VAZGFydG1vdXRoLmVkdQ==
†These authors have contributed equally to this work.