Anatomy and Function of Ventral Tegmental Area Glutamate Neurons
- 1Brown Foundation Institute of Molecular Medicine for the Prevention of Human Diseases, UTHealth McGovern Medical School, Houston, TX, United States
- 2Neuroscience Program, The University of Texas MD Anderson Cancer Center UTHealth Graduate School of Biomedical Sciences, Houston, TX, United States
The ventral tegmental area (VTA) is well known for regulating reward consumption, learning, memory, and addiction behaviors through mediating dopamine (DA) release in downstream regions. Other than DA neurons, the VTA is known to be heterogeneous and contains other types of neurons, including glutamate neurons. In contrast to the well-studied and established functions of DA neurons, the role of VTA glutamate neurons is understudied, presumably due to their relatively small quantity and a lack of effective means to study them. Yet, emerging studies have begun to reveal the importance of glutamate release from VTA neurons in regulating diverse behavioral repertoire through a complex intra-VTA and long-range neuronal network. In this review, we summarize the features of VTA glutamate neurons from three perspectives, namely, cellular properties, neural connections, and behavioral functions. Delineation of VTA glutamatergic pathways and their interactions with VTA DA neurons in regulating behaviors may reveal previously unappreciated functions of the VTA in other physiological processes.
Introduction
The ventral tegmental area (VTA) located in the midbrain controls diverse behavioral repertoire, including reward processing, aversion, stress modulation, drug addiction, learning, and memory (Haber and Fudge, 1997; Ikemoto, 2007; Arias-Carrion et al., 2010; Polter and Kauer, 2014). The VTA functional diversity is partly reflected by its cellular and circuit heterogeneities. The VTA is composed of ∼60% dopaminergic neurons (DA neurons), ∼35% GABAergic neurons (GABA neurons), and ∼5% glutamate neurons (glutamate neurons) (Nair-Roberts et al., 2008; Yamaguchi et al., 2015). Other than these three classical neurotransmitters, VTA neurons also release peptides, including cholecystokinin, neurotensin, corticotropin-releasing factor, brain-derived neurotrophic factor, and calbindin (Seroogy et al., 1988; Jayaraman et al., 1990; Hyman et al., 1994; Liang et al., 1996; Grieder et al., 2014). Co-release of classical neurotransmitters and neuropeptides increases the cellular heterogeneity of VTA neurons. At the circuit level, these diverse VTA neurons make direct synaptic contacts with multiple brain regions, including the prefrontal cortex (PFC), the nucleus accumbens (NACc), the pedunculopontine tegmentum (PPTg), the laterodorsal tegmentum nucleus (LDTg), the lateral habenula (LHb), the periaqueductal gray (PAG), the bed nucleus of the stria terminalis (BNST), the lateral hypothalamus (LH), the ventral pallidum (VP), and the dorsal raphe nucleus (DRN) (Morales and Margolis, 2017). Intricate interactions among different VTA neuron populations and their diverse input and output projections mediate the behavioral repertoire of VTA function.
The DA neurons in the VTA have been a hot topic and are considered the major therapeutic target for treating reward-related disorders, such as drug addictions and mood disorders due to their key roles in directing reward-related responses (Price and Drevets, 2010; Polter and Kauer, 2014). Other than VTA DA neurons, increasing studies suggest that VTA GABA neurons are also important for behavioral regulation by forming local synapses onto DA neurons or sending projections to remote brain sites. VTA GABA neurons have been found to modulate reward consumption, depression, stress, and sleep by altering DA release from neighboring DA neurons (Van Zessen et al., 2012; Bouarab et al., 2019; Chowdhury et al., 2019; Yu et al., 2019, 2021; Galaj et al., 2020). Long-range connections of GABA neurons also intertwine with VTA dopaminergic circuits (Beier et al., 2019; Bouarab et al., 2019), suggesting that the function of VTA GABA neurons is at least partially dependent on DA release. Relatively less has been focused on VTA glutamate signaling due to their rare existence in the VTA. However, recent studies suggest that VTA glutamate neurons regulate reward reinforcement, aversive behaviors, wakefulness, and defensive behaviors (Mangieri et al., 2019; Yu et al., 2019; Barbano et al., 2020; Zell et al., 2020), emphasizing the importance of VTA glutamate release. This review aims to give a general overview of VTA glutamate neurons in the aspects of cellular features, neural connections, and behavioral functions that can be DA dependent or independent.
Molecular and Electrophysiological Features of Ventral Tegmental Area Glutamate Neurons
In the brain, glutamate is synthesized from glutamine by glutaminase and then packaged into vesicles by vesicular glutamate transporters (Vgluts) for its synaptic release (Takamori et al., 2000). Glutamate neurons in the VTA mainly express Vglut2 but not Vglut1 or Vglut3 (Yamaguchi et al., 2007, 2011). Vglut2-expressing glutamate neurons are mostly located in the anterior and middle line of the VTA, where these neurons outnumber DA neurons (Yamaguchi et al., 2007). In addition, Vglut2-expressing neurons form asymmetry synapses and release glutamate to downstream targets once activated, confirming that VTA Vglut2-expressing neurons release glutamate and form excitatory synapse (Chuhma et al., 2004; Hnasko et al., 2012). In addition, about 35% of NACc-projecting VTA neurons and 66% of PFC-projecting VTA neurons contain Vglut2 (Stuber et al., 2010; Tecuapetla et al., 2010; Yamaguchi et al., 2011; Gorelova et al., 2012), implicating a role for glutamate release from the VTA to both NACc and PFC in mediating the VTA function.
The VTA glutamate neurons are heterogeneous. A subset of these neurons only releases glutamate, while others also express tyrosine hydrolase (TH) and may co-release DA (Hnasko et al., 2010, 2012; Stuber et al., 2010; Tecuapetla et al., 2010; Morales and Root, 2014; Root et al., 2016). Surprisingly, about 50% of Vglut2+ TH+ expressing neurons do not express vesicular monoamine transporter 2 (Vmat2), DA transporter (DAT), or DA receptor 2 (D2) (Li et al., 2013), which are either required for DA release or normally expressed in VTA DA neurons, indicating that this subset of Vglut2+ TH+ expressing neurons may solely release glutamate despite expressing TH. The release of glutamate from VTA DA neurons is speculated to be responsible for fast responses (Chuhma et al., 2004; Lavin et al., 2005). Interestingly, there appears to be a development requirement for Vglut2 expression in the VTA. The knockout of Vglut2 from midbrain DA neurons in the neonatal stage results in morphological abnormalities of these neurons (Fortin et al., 2012; Papathanou et al., 2018) and alterations in risk-taking behaviors as well as DA responses to amphetamine (Birgner et al., 2010; Alsio et al., 2011; Berube-Carriere et al., 2012). However, the knockout of Vglut2 from DA neurons in the adult stage had minimal effects on either morphology or behaviors (Papathanou et al., 2018). These contrasting observations indicate an important function of developmentally transient Vglut2 expression in VTA DA neurons. The transient expression of Vglut2 during development in a subset of VTA-DA neurons is supported by a reduced proportion of VTA Vglut2+ TH+ neurons in adulthood compared to neonates (Berube-Carriere et al., 2009, 2012; Moss et al., 2011). In line with this, neonatal knockout of Vmat2 from Vglut2 expressing neurons also leads to almost complete deletion of Vmat2 expression in the VTA and results in developmental abnormalities and death (Cai et al., 2021), reminiscent phenotypes of knockout of Vmat2 at the whole body (Wang et al., 1997), suggesting extensive transient co-localization between Vmat2 and Vglut2 in the VTA during development. It is interesting to note that the off-expression of Vglut2 in adulthood is an integral part of normal VTA DA neuron development and function as over-expression of Vglut2 in these neurons in adulthood causes neuronal death (Steinkellner et al., 2018). These observations suggest an importance of developmental Vglut2 expression, potentially via glutamate release, in the normal development of VTA DA neurons. Thus, it appears that VTA Vglut2+ TH+ neurons in adulthood may represent only a small subset of Vglut2+ TH+ neurons in neonates, in which residual Vglut2 expression remains.
A small subset of VTA glutamate neurons co-expresses vesicular GABA transporter (Vgat) and co-releases GABA (Root et al., 2014; Yoo et al., 2016). Most VTA Vglut2+Vgat+ neurons are located in the anterior portion of the interfascicular nucleus (IF) (Root et al., 2020). These neurons can establish adjacent asymmetric and symmetric synapses on downstream neurons (Root et al., 2014), suggesting an intriguing co-existence of both excitatory glutamatergic and inhibitory GABAergic synapses formed by these individual presynaptic neurons. Consistently, activation of these neurons is able to induce both fast excitation and inhibition in downstream neurons, the net responses of which seem to be projection-specific (Root et al., 2014; Yoo et al., 2016). Another small subset of Vglut2+ neurons expresses both TH and glutamic acid decarboxylases (GADs) (Root et al., 2014), another marker for GABAergic neurons. However, whether these neurons co-release all three neurotransmitters remains to be demonstrated. Although VTA neurons are known to co-express abundant neuropeptides (Seroogy et al., 1988; Jayaraman et al., 1990; Hyman et al., 1994; Liang et al., 1996; Grieder et al., 2014), relatively less is known about whether VTA glutamate neurons also co-release peptides (Wise and Morales, 2010).
The VTA glutamate neurons exhibit a unique electric property. The hyperpolarization-activated cation current (Ih) mediated by hyperpolarization-activated cyclic nucleotide-gated channels is an important contributor to both resting membrane potential and dendritic integration (Pape, 1996; Robinson and Siegelbaum, 2003). Similar to medial, but contrary to lateral VTA DA neurons, VTA glutamate neurons have a small or no Ih, which leads to a lower resting membrane potential (Hnasko et al., 2012). In addition, compared to lateral DA neurons, VTA glutamate neurons also present a shallower afterhyperpolarization (AHP) and consequent higher firing rate (Hnasko et al., 2012). In addition, ex vivo electrophysiological recordings reveal that VTA glutamate neurons present a relatively more hyperpolarized resting membrane potential, greater rheobase, and lower spontaneous firing frequency compared to GABA neurons (Miranda-Barrientos et al., 2021). These studies suggest that VTA glutamate neurons are more excitable than other VTA neurons.
Neural Connections of Ventral Tegmental Area Glutamate Neurons
Intrinsic Connections
Accumulating data support an existence of strong intrinsic connections among VTA neurons. Anatomical and electrophysiological data reveal that VTA GABA neurons form local synapses onto other VTA neurons (Omelchenko and Sesack, 2009; Matsui and Williams, 2011). For DA neurons, the inhibitory input from local GABA neurons appears to be weaker than long-range inhibitory ones (Omelchenko and Sesack, 2009). However, disrupting local VTA GABA release causes major malfunctions in stress and anxiety modulation, which can be rescued by restoring GABAergic control on DA neurons (Van Zessen et al., 2012; Bouarab et al., 2019; Chowdhury et al., 2019; Yu et al., 2021), suggesting the importance of intrinsic GABAergic action in controlling VTA DA neurons and maintaining normal behavioral responses.
Ultrastructural studies suggest that ∼50% of VTA fibers forming local synaptic contacts contain Vglut2, and these glutamatergic fibers establish asymmetric synapses on both DA and non-DA neurons (Dobi et al., 2010). Optical stimulation of VTA glutamate neurons elicits AMPA/NMDA receptor-dependent firing in NACc-projecting DA neurons via monosynaptic connections, suggesting a local excitatory synapse connection between glutamate and DA neurons (Wang et al., 2015). This local excitatory connection is important as VTA glutamate neuron activation leads to changes in reward-related responses (Wang et al., 2015; Yoo et al., 2016). Interestingly, the D2 receptor has been detected in VTA glutamate neurons, and the application of D2 agonists is able to hyperpolarize these neurons (Hnasko et al., 2012), suggesting a reciprocal connection between VTA DA and glutamate neurons. VTA glutamate neurons also receive local inhibitory input from GABA neurons, thereby allowing GABA neurons to restrain neuronal activities of VTA glutamate neurons (Yu et al., 2019). Although none has been reported, it is conceivable that VTA glutamate neurons send local excitatory inputs to VTA GABA neurons.
Long-Range Connections
Given the known heterogeneity of VTA neurons, it is important to investigate the differential remote connectivity of these neurons. VTA DA neurons mainly form synaptic contacts with limbic and cortical regions, including the NACc, amygdala, and PFC (Hnasko et al., 2012; Taylor et al., 2014; Morales and Margolis, 2017). Ventral tegmental area glutamate neurons establish long-range connections either in parallel with or distinct from those of VTA DA neurons. An input–output map has been drawn using a monosynaptic viral tracing approach. In general, VTA glutamate neurons have a grossly similar input–output pattern to VTA DA neurons but with some distinct features (Beier et al., 2015, 2019). For example, similar to VTA DA neurons, VTA glutamate neurons that project to the NACc receive more inputs from the striatal site and fewer projections from the DRN. Yet, distinct from VTA DA neurons, glutamate neurons that project to the PFC and the amygdala receive more inputs from the VP and the LH (Beier et al., 2019).
A) Afferent inputs
Monosynaptic rabies virus tracing results show that VTA glutamate neurons receive strong upstream projections from the LH, DRN, cortical regions, VP, LDTg, and PAG (Faget et al., 2016). Similar to VTA DA neurons, VTA glutamate neurons receive heavy inputs from the LH and the DRN. However, VTA glutamate neurons receive proportionally more projections from cortical regions, while DA neurons receive more projections from striatal regions (Faget et al., 2016). Cortical inputs to VTA glutamate neurons are from a variety of subregions, mainly the somatosensory motor, insular, and cingulate cortices (Faget et al., 2016). Although the general afferent projections to VTA glutamate neurons are clear, specific upstream neuronal subtypes and their detailed mechanisms in the regulation of VTA glutamate neuron activities are largely underexplored.
B) Efferent projections
The VTA glutamate neurons send projections that are parallel with VTA DA neurons, such as NACc and PFC, as well as to regions with few VTA dopaminergic inputs, such as LHb and VP (Hnasko et al., 2012; Morales and Root, 2014). As mentioned above, VTA glutamate neurons send abundant projections to the ventral striatum, especially the medial shell of the NACc (Figure 1) (Yamaguchi et al., 2011; Hnasko et al., 2012). TH is present in the majority of VTA glutamate neurons that project to the NACc, and more than half of TH expressing neurons projecting to the NACc co-release glutamate (Stuber et al., 2010; Tecuapetla et al., 2010; Yamaguchi et al., 2011; Hnasko et al., 2012; Mongia et al., 2019), VTA glutamate neurons can drive positive reinforcement by releasing glutamate in the NACc even in the absence of DA release (Zell et al., 2020). VTA glutamatergic projections in the NACc are also sufficient to promote wakefulness and rapid eye movement (REM) independent of DA release (Yu et al., 2019).
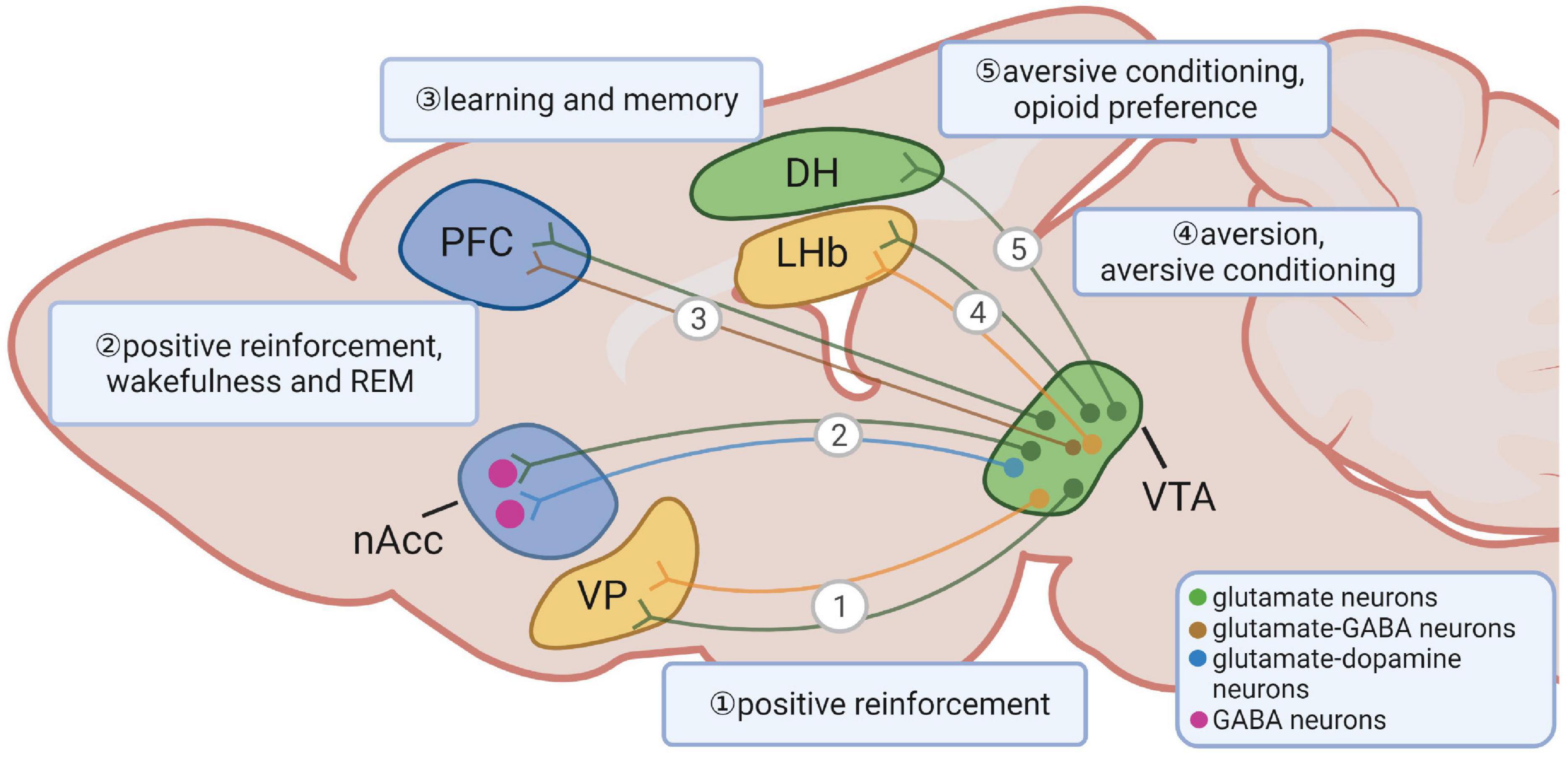
Figure 1. Long-range projections of ventral tegmental area (VTA) glutamate neurons and their functions. VTA glutamate neurons project to the ventral pallidum, the nucleus accumbens, the prefrontal cortex, the lateral habenula and the dorsal hippocampus. The shapes and locations can only represent the general structure and location. The segregated lines with arrows represent a simplified diagram of general projection patterns rather than indicating an existence of parallel and non-overlapping projection patterns from the indicated neurons.
Electrophysiological data suggest that glutamate release from the VTA depolarizes PFC neurons and evokes monosynaptic excitatory postsynaptic potentials (EPSPs) (Lavin et al., 2005; Yamaguchi et al., 2011; Gorelova et al., 2012; Hnasko et al., 2012). In addition, VTA glutamate neuron-induced EPSPs in the PFC can be eliminated by glutamate but not DA antagonists (Hnasko et al., 2012). Ultrastructure studies show that ∼60% of PFC projecting VTA neurons contain Vglut2, half of which are TH positive (Yamaguchi et al., 2011; Gorelova et al., 2012), suggesting that Vglut2 positive neurons compose a large portion of mesocortical neurons.
As previously mentioned, VTA glutamate neurons also send abundant projections to structures that receive less DA inputs, such as the LHb and the VP (Figure 1) (Hnasko et al., 2012; Root et al., 2014; Taylor et al., 2014; Yoo et al., 2016). The majority of Vglut2 positive fibers in the LHb lack TH, and DA release is not detected after optical activation of TH+ fibers from the VTA (Hnasko et al., 2012; Taylor et al., 2014). Surprisingly, axons from most mesohabenular neurons are found to co-express Vglut2 and markers of GABAergic neurons, and form both symmetric and asymmetric synapses onto LHb neurons (Root et al., 2014, 2018b; Yoo et al., 2016). Selective ex vivo activation of mesohabenular axons evokes GABAA-mediated outward and AMPA-mediated inward currents on individual neurons (Root et al., 2014). While these neurons co-release two neurotransmitters, glutamate and GABA are packaged and released from distinct pools of vesicles and synapses, suggesting an independent mechanism in accumulation, release, and recycling of each neurotransmitter (Root et al., 2018b). Interestingly, ex vivo train activation of glutamatergic projections to the LHb produces a net inhibitory effect, leading to a persistent decrease in the firing rate of postsynaptic cells (Yoo et al., 2016), suggesting a dominant role of GABA over glutamate release.
The VTA Vglut2-expressing fibers are present at the rostra-caudal “finger-like” extent of the VP. Similar to VTA Vglut2-expressing fibers in the LHb, the majority of these fibers lack TH expression (Hnasko et al., 2012). Optogenetic stimulation of these fibers elicits both AMPAR- and NMDAR-mediated currents, suggesting the existence of functional excitatory glutamatergic terminals (Hnasko et al., 2012; Yoo et al., 2016). Interestingly, optical activation induces gabazine-sensitive inhibitory postsynaptic currents (IPSCs) in the VP (Yoo et al., 2016), suggesting that VTA Vglut2 neurons projecting to the VP also co-release GABA. Unlike the net inhibitory effect from activating VTA glutamatergic fibers in the LHb, activation of VTA Vglut2 expressing terminals in the VP elicits a consistent increase of firing in postsynaptic neurons, suggesting that, contrary to what has been found in the LHb, the effect by glutamate release from VTA glutamatergic fibers in the VP dominates over the effect by GABA release (Yoo et al., 2016).
The VTA dopaminergic inputs to the dorsal hippocampus (DH) have long been proposed to be involved in memory modulation (Lisman and Grace, 2005; Mcnamara et al., 2014; Rosen et al., 2015). However, this idea has been challenged due to the scarcity of dopaminergic projections found in the DH (Kempadoo et al., 2016; Takeuchi et al., 2016). A recent study shows that VTA glutamate neurons send one-way projections to the DH (Ntamati and Luscher, 2016; Adeniyi et al., 2020; Han et al., 2020). In addition, these DH-projecting glutamate neurons are TH negative and are mostly located in rostral VTA (Han et al., 2020). Interestingly, postsynaptic currents in the granule cell layer of the dentate gyrus elicited by monosynaptic VTA projections are sensitive to both GABA and AMPA receptor antagonists, suggesting that the upstream VTA neurons co-release GABA and glutamate (Ntamati and Luscher, 2016). Thus, VTA glutamate neurons share parallel projections to those of VTA DA neurons but also exhibit distinct projections that may function independently of DA action.
Behavioral Regulation by Ventral Tegmental Area Glutamate Neurons
Behaviors Associated With Changes in Valence
Extensive research has been focused on the functional roles of VTA DA neurons, and these neurons are known to play distinct roles in both positive and negative reinforcement, resulting in preference and avoidance behaviors, respectively (Adcock et al., 2006; Brischoux et al., 2009; Bromberg-Martin et al., 2010). VTA DA neurons respond with increased activities to both rewarding and aversive stimuli (Salamone and Correa, 2012), suggesting physiological implications of these neurons in response to diverse and even conflicting environmental settings. Despite complicated DA neuron responses to seemingly conflicting cues, acute activation of these neurons leads to positive reinforcement and behavioral preference (Tsai et al., 2009; Adamantidis et al., 2011; Witten et al., 2011).
Compared to VTA DA neurons, the role of VTA glutamate neurons seems to be more complicated with inconsistent responses toward rewarding and aversive cues and may induce both preference and avoidance behaviors (Root et al., 2018a). In vivo Ca2 + recording data reveal that VTA glutamate neurons exhibit increased overall firing to both aversive and rewarding stimuli (Montardy et al., 2019; Mcgovern et al., 2021). Interestingly, a small population of VTA glutamate neurons increases firing to both aversive and rewarding stimulus (Root et al., 2018a), which might be explained by the functional heterogeneity of different VTA glutamate neuron populations. For instance, an in vivo Ca2 + recording study reveals that Vglut2+ Vgat– neurons signal cues predicting reward and Vglut2+ Vgat+ neurons signal unconditioned rewarding and aversive outcomes (Root et al., 2020). However, in vivo electrophysiology recording shows that the majority of VTA glutamate neurons decrease their firing, while a small subset shows no change in firing, in response to reward stimulus (Root et al., 2018a,2020). This inconsistency might be due to the insensitivity of Ca2 + indicator toward the decrease of neuronal activity (Chen et al., 2013). Nevertheless, these observations implicate VTA glutamate neurons functioning in physiological responses to various environmental cues.
Despite responses of VTA glutamate neurons to both rewarding and aversive cues, direct optical activation of VTA glutamate neurons induces conditioned place preference and appetitive instrumental conditioning (Wang et al., 2015; Yoo et al., 2016). The rewarding effects of VTA glutamate neurons are suggested to be mediated through the activation of VTA DA neurons that project to the NACc (Wang et al., 2015). However, the anatomical connection between VTA glutamate and DA neurons in mediating the rewarding effects remains to be verified. Interestingly, VTA glutamate neurons could induce reinforcement in the absence of DA release (Zell et al., 2020), suggesting a DA-independent effect on rewarding behaviors. However, sustained stimulation of VTA glutamate neurons is less preferred and could even manifest as apparent behavioral avoidance (Yoo et al., 2016). Similarly, in contrast to the previous discussion on inducing rewarding effects, optical stimulation of VTA glutamate neurons may induce aversive escape behaviors (Barbano et al., 2020). The reasons underlying these contrasting observations are unknown but may involve different subsets of VTA glutamate neurons or different stimulation protocols, which may cause different and sometimes opposite effects on neuron activity (Wang et al., 2015; Yoo et al., 2016; Root et al., 2020; Zell et al., 2020). Therefore, VTA glutamate neurons signal both rewarding and aversive stimuli and may induce rewarding or aversive effects.
Reminiscent of conflicting behavioral outcomes by activating VTA glutamate neurons in remote projecting sites, acute activation of VTA long-range glutamatergic terminals also produces diverse and conflicting behaviors. The mesolimbic pathway is important for aversive conditioning (Pezze and Feldon, 2004; Zweifel et al., 2011). Optogenetic activation of VTA Vglut2→NACc projections induces aversion through glutamate-mediated action on local GABAergic interneurons (Qi et al., 2016). The LHb is a brain region known for its function in conditioning aversion and reward (Lammel et al., 2012; Stopper and Floresco, 2014). Acute optogenetic activation of VTA glutamatergic fibers in the LHb elicits aversion and produces aversive conditioning (Root et al., 2014). Acute activation of VTA glutamatergic terminals in the LHb, VP, and NACc induces self-stimulation (Yoo et al., 2016; Zell et al., 2020). VTA glutamatergic projections to the DH are significant for the formation and retrieval of context memories with aversive stimulus, suggesting that VTA Vglut2→DH projections represent negative valence (Han et al., 2020). The reason underlying these apparent discrepancies within VTA glutamatergic projections to different downstream regions is unknown due to the distinct roles of different subsets of VTA glutamate neurons.
Drug Addiction
The μ-opioid receptors (MORs) are well-known to mediate the rewarding analgesic effects of commonly prescribed and abused opioids (Kieffer and Gaveriaux-Ruff, 2002). It is well established that MORs modulate opioid reward through inhibition of GABA transmission and subsequent DA neuron disinhibition (Steffensen et al., 2006; Hjelmstad et al., 2013; Matsui et al., 2014; Fields and Margolis, 2015). Recent studies suggest that MORs also inhibit glutamatergic transmission onto general Vglut2 expressing synapses, suggesting a role of glutamate neurons in opioid effects (Reeves et al., 2021). In addition, opioid administration brings an immediate effect of increasing cerebral blood flow in the anterior cingulate cortex, thalamus, and amygdala (Schlaepfer et al., 1998; Kosel et al., 2008), followed by long-lasting euphoric effects (Denier et al., 2013), further indicating that glutamate transmission plays a potential role in the “rush” after opioid administration. Histological data reveal the presence of functional MORs in VTA glutamate neurons (Miranda-Barrientos et al., 2021). Opioids, such as morphine, have been shown to activate VTA DA neurons by inhibiting GABAergic inputs and strengthening glutamatergic inputs (Jalabert et al., 2011; Chen et al., 2015). VTA glutamate transmission also plays an important role in reinstatement of heroin seeking (Bossert et al., 2004). Moreover, optically activating the VTA Vglut2→DH projections promotes opioid preference (Han et al., 2020). Thus, increasing evidence suggests that VTA glutamate neurons mediate opioid effects.
While its use in the United States gains more popularity due to the ongoing legalization process (Schulden et al., 2009), cannabis can also be aversive to certain groups of people (D’souza et al., 2004; Parsons and Hurd, 2015). Similar paradoxical effects have also been observed in experimental animals. Δ9-Tetrahydrocannabinol (Δ9-THC), the primary psychoactive ingredient of cannabis, is rewarding to squirrel monkeys but not to rhesus monkeys (Mansbach et al., 1994; John et al., 2018). In addition, low doses of Δ9-THC facilitate intracranial self-stimulation (ISS), while high doses inhibit ISS (Vlachou et al., 2007; Wiebelhaus et al., 2015). The Δ9-THC cannabis reward action is believed to be mediated by activation of the CB1 receptor on VTA GABA neurons and disinhibition of VTA DA neurons (Lupica and Riegel, 2005). Yet, the mechanisms underlying the aversive effects of cannabis are unclear. Mounting evidence demonstrates abundant expression of the CB1 receptor in VTA glutamate neurons (Melis et al., 2000, 2004; Han et al., 2017). It is proposed that the aversive effects of cannabis are due to its dose-dependent inhibition of VTA glutamate neurons. Given its rewarding effects through VTA GABA neurons, the overall effects of cannabis depend on the ratio of CB1 expression levels in the VTA GABA over VTA glutamate neurons (Han et al., 2017).
The VTA is also an important site mediating the nicotine effects. Nicotine addiction is mediated through the nicotinic acetylcholine receptor (nAChR). The expression of β2-containing nAChRs has been detected in VTA DA neurons (Klink et al., 2001; Changeux, 2010), and the nicotine treatment promotes VTA DA release (Mansvelder and Mcgehee, 2002). In addition, blocking nicotine signaling in the VTA prevents the induction of nicotine preference (Tolu et al., 2013; Durand-De Cuttoli et al., 2018). Recently, nicotinic receptors have been found in VTA glutamate neurons (Yan et al., 2018), and application of nicotine induces glutamatergic excitatory potentiation on VTA DA neurons (Gao et al., 2010; Mao et al., 2011; Yan et al., 2018). In addition, VTA glutamatergic signaling has also been implicated in regulating the reinstatement of cocaine seeking (You et al., 2007; Wise and Morales, 2010; Williams et al., 2014) and benzodiazepine effects (Heikkinen et al., 2009) by regulating VTA DA signaling. These data suggest that VTA glutamatergic inputs to DA neurons might play a role in nicotine addiction.
Other Behaviors
A) Learning and memory
The VTA glutamate neurons are known to form asymmetrical synapses and evoke EPSCs on the PFC and the DH, both of which are known to regulate learning and memory (Miller and Cohen, 2001; Curtis and D’esposito, 2003; Matus-Amat et al., 2004). Within the PFC, VTA glutamatergic projections function as a signal for rapid behavioral responses, such as prediction error signaling (Gorelova et al., 2012). Within the DH, VTA glutamatergic projections signal negative valence to memory circuits, leading to the formation of fear-inducing context memories and to context-specific reinstatement of fear (Han et al., 2020). Thus, VTA glutamate neurons play a role in memory consolidation, at least through projecting to PFC and DH.
B) Sleep and wakefulness
The VTA DA neurons are known to promote wakefulness through DA D2-like receptors (Eban-Rothschild et al., 2016; Oishi et al., 2017). VTA glutamate neurons increase activity during wakefulness and REM sleep while decreasing firing during non-REM sleep (Yu et al., 2019). Chemogenetic activation of VTA glutamate neurons increases the duration of wakefulness during the inactive phase, and ablation of VTA glutamate neurons reduces wakefulness and increases NREM sleep during the active phase (Yu et al., 2019). It will be interesting to understand the functional anatomy of VTA glutamate neurons with the known brain circuits regulating the sleep-wake cycle.
C) Defensive behaviors
The VTA glutamate neurons encode danger signals and are required for the innate escape behavior in response to threatening stimuli (Barbano et al., 2020). Lateral hypothalamus glutamate neurons are known to increase the activity to predator odors, and optogenetic activation of LH glutamate neurons induces innate defensive behaviors (Lecca et al., 2017; Mangieri et al., 2018; Chen et al., 2020). Ultrastructure and electrophysiology data show that LH glutamate neurons send projections to VTA glutamate neurons (Kempadoo et al., 2013; Barbano et al., 2020). Interestingly, LH glutamate neurons send projections to the paraventricular nucleus (PVH) glutamate neurons, which elicit strong escape jumping behaviors upon activation (Mangieri et al., 2018). PVH glutamate neurons can in turn activate putative VTA glutamate neurons for defensive behaviors (Mangieri et al., 2019). Thus, LH glutamate neurons promote defensive behaviors through both direct projections and indirect projections via PVH glutamate neurons to VTA glutamate neurons.
D) Eating behaviors
The VTA neuronal signaling has been found to be negatively correlated with obesity development in human (Baik, 2013; Guo et al., 2014), and specifically, suggesting that patients with obesity have a tendency to consume more calories for compensating the low level of DA signaling. In rodents, VTA neurons have been demonstrated to mediate the function of energy balance-related hormones in feeding and body weight regulation (Fulton et al., 2006; Hommel et al., 2006; Narayanan et al., 2010; Perello and Zigman, 2012; Cone et al., 2014). Although most studies focus on VTA DA neurons, the functional roles of VTA glutamate neurons have been emerging. Especially, activation of glucagon-like peptide-1 (GLP-1) neurons in the VTA inhibits feeding by increasing AMPA/Kainate signaling in VTA glutamate neuron projections to the NACc (Mietlicki-Baase et al., 2014), suggesting the role of VTA glutamate neurons in regulating the mediation of GLP-1 from the VTA in feeding behaviors. In addition, the LH and PVH are two important regions for feeding behaviors and body weight homeostasis (Gutierrez et al., 2011; Bonnavion et al., 2016; Krashes et al., 2016; Sutton et al., 2016). Since these two regions send glutamatergic projections or GABAergic projections to VTA glutamate neurons or GABA neurons that regulate VTA glutamate release (Nieh et al., 2016; Tyree and De Lecea, 2017; Mangieri et al., 2019; Barbano et al., 2020), VTA glutamate neurons are well-positioned to modulate homeostatic feeding and body weight balance. Given the known role of VTA in mediating hedonic feeding and VTA glutamate neurons in regulating positive reinforcement (Rossi and Stuber, 2018), these neurons might also be involved in hedonic feeding and contribute to diet-induced obesity. Future studies are warranted to address the function of these neurons in homeostatic and hedonic feeding as well as body weight regulation.
Author Contributions
JC wrote the manuscript with significant inputs from QT. Both authors contributed to the article and approved the submitted version.
Conflict of Interest
The authors declare that the research was conducted in the absence of any commercial or financial relationships that could be construed as a potential conflict of interest.
Publisher’s Note
All claims expressed in this article are solely those of the authors and do not necessarily represent those of their affiliated organizations, or those of the publisher, the editors and the reviewers. Any product that may be evaluated in this article, or claim that may be made by its manufacturer, is not guaranteed or endorsed by the publisher.
Acknowledgments
We acknowledge the support from NIH R01 DK114279, R21NS108091, R01 DK109934, R01 DK120858, DOD W81XWH-19-1-0429 to QT, and Russell and Diana Hawkins Family Foundation Discovery Fellowship to JC. QT is the holder of the Cullen Chair in Molecular Medicine at McGovern Medical School of UTHealth. Figure 1 was created with BioRender.com.
References
Adamantidis, A. R., Tsai, H. C., Boutrel, B., Zhang, F., Stuber, G. D., Budygin, E. A., et al. (2011). Optogenetic interrogation of dopaminergic modulation of the multiple phases of reward-seeking behavior. J. Neurosci. 31, 10829–10835. doi: 10.1523/JNEUROSCI.2246-11.2011
Adcock, R. A., Thangavel, A., Whitfield-Gabrieli, S., Knutson, B., and Gabrieli, J. D. (2006). Reward-motivated learning: mesolimbic activation precedes memory formation. Neuron 50, 507–517. doi: 10.1016/j.neuron.2006.03.036
Adeniyi, P. A., Shrestha, A., and Ogundele, O. M. (2020). Distribution of VTA glutamate and dopamine terminals, and their significance in CA1 neural network activity. Neuroscience 446, 171–198. doi: 10.1016/j.neuroscience.2020.06.045
Alsio, J., Nordenankar, K., Arvidsson, E., Birgner, C., Mahmoudi, S., Halbout, B., et al. (2011). Enhanced sucrose and cocaine self-administration and cue-induced drug seeking after loss of VGLUT2 in midbrain dopamine neurons in mice. J. Neurosci. 31, 12593–12603. doi: 10.1523/JNEUROSCI.2397-11.2011
Arias-Carrion, O., Stamelou, M., Murillo-Rodriguez, E., Menendez-Gonzalez, M., and Poppel, E. (2010). Dopaminergic reward system: a short integrative review. Int. Arch. Med. 3:24. doi: 10.1186/1755-7682-3-24
Baik, J. H. (2013). Dopamine signaling in food addiction: role of dopamine D2 receptors. BMB Rep. 46, 519–526. doi: 10.5483/bmbrep.2013.46.11.207
Barbano, M. F., Wang, H. L., Zhang, S., Miranda-Barrientos, J., Estrin, D. J., Figueroa-Gonzalez, A., et al. (2020). VTA Glutamatergic neurons mediate innate defensive behaviors. Neuron 107, 368–382.e8. doi: 10.1016/j.neuron.2020.04.024
Beier, K. T., Gao, X. J., Xie, S., Deloach, K. E., Malenka, R. C., and Luo, L. (2019). Topological organization of ventral tegmental area connectivity revealed by viral-genetic dissection of input-output relations. Cell Rep. 26, 159–167.e6. doi: 10.1016/j.celrep.2018.12.040
Beier, K. T., Steinberg, E. E., Deloach, K. E., Xie, S., Miyamichi, K., Schwarz, L., et al. (2015). Circuit architecture of VTA dopamine neurons revealed by systematic input-output mapping. Cell 162, 622–634. doi: 10.1016/j.cell.2015.07.015
Berube-Carriere, N., Guay, G., Fortin, G. M., Kullander, K., Olson, L., Wallen-Mackenzie, A., et al. (2012). Ultrastructural characterization of the mesostriatal dopamine innervation in mice, including two mouse lines of conditional VGLUT2 knockout in dopamine neurons. Eur. J. Neurosci. 35, 527–538. doi: 10.1111/j.1460-9568.2012.07992.x
Berube-Carriere, N., Riad, M., Dal Bo, G., Levesque, D., Trudeau, L. E., and Descarries, L. (2009). The dual dopamine-glutamate phenotype of growing mesencephalic neurons regresses in mature rat brain. J. Comp. Neurol. 517, 873–891. doi: 10.1002/cne.22194
Birgner, C., Nordenankar, K., Lundblad, M., Mendez, J. A., Smith, C., Le Greves, M., et al. (2010). VGLUT2 in dopamine neurons is required for psychostimulant-induced behavioral activation. Proc. Natl. Acad. Sci. U.S.A. 107, 389–394. doi: 10.1073/pnas.0910986107
Bonnavion, P., Mickelsen, L. E., Fujita, A., De Lecea, L., and Jackson, A. C. (2016). Hubs and spokes of the lateral hypothalamus: cell types, circuits and behaviour. J. Physiol. 594, 6443–6462. doi: 10.1113/JP271946
Bossert, J. M., Liu, S. Y., Lu, L., and Shaham, Y. (2004). A role of ventral tegmental area glutamate in contextual cue-induced relapse to heroin seeking. J. Neurosci. 24, 10726–10730. doi: 10.1523/JNEUROSCI.3207-04.2004
Bouarab, C., Thompson, B., and Polter, A. M. (2019). VTA GABA neurons at the interface of stress and reward. Front. Neural. Circuits 13:78. doi: 10.3389/fncir.2019.00078
Brischoux, F., Chakraborty, S., Brierley, D. I., and Ungless, M. A. (2009). Phasic excitation of dopamine neurons in ventral VTA by noxious stimuli. Proc. Natl. Acad. Sci. U.S.A. 106, 4894–4899. doi: 10.1073/pnas.0811507106
Bromberg-Martin, E. S., Matsumoto, M., and Hikosaka, O. (2010). Dopamine in motivational control: rewarding, aversive, and alerting. Neuron 68, 815–834. doi: 10.1016/j.neuron.2010.11.022
Cai, J., Cassidy, R. M., Morrill, J., and Tong, Q. (2021). “Neurotransmitter co-transmission in brain control of feeding and body weight,” in Neuronal Signaling in Metabolic Regulation, 1st Edn, ed. Q. Tong (Boca Raton, FL: CRC Press).
Changeux, J. P. (2010). Nicotine addiction and nicotinic receptors: lessons from genetically modified mice. Nat. Rev. Neurosci. 11, 389–401. doi: 10.1038/nrn2849
Chen, L., Cai, P., Wang, R. F., Lu, Y. P., Chen, H. Y., Guo, Y. R., et al. (2020). Glutamatergic lateral hypothalamus promotes defensive behaviors. Neuropharmacology 178:108239. doi: 10.1016/j.neuropharm.2020.108239
Chen, M., Zhao, Y., Yang, H., Luan, W., Song, J., Cui, D., et al. (2015). Morphine disinhibits glutamatergic input to VTA dopamine neurons and promotes dopamine neuron excitation. Elife 4:e09275. doi: 10.7554/eLife.09275
Chen, T. W., Wardill, T. J., Sun, Y., Pulver, S. R., Renninger, S. L., Baohan, A., et al. (2013). Ultrasensitive fluorescent proteins for imaging neuronal activity. Nature 499, 295–300. doi: 10.1038/nature12354
Chowdhury, S., Matsubara, T., Miyazaki, T., Ono, D., Fukatsu, N., Abe, M., et al. (2019). GABA neurons in the ventral tegmental area regulate non-rapid eye movement sleep in mice. Elife 8:e44928. doi: 10.7554/eLife.44928
Chuhma, N., Zhang, H., Masson, J., Zhuang, X., Sulzer, D., Hen, R., et al. (2004). Dopamine neurons mediate a fast excitatory signal via their glutamatergic synapses. J. Neurosci. 24, 972–981. doi: 10.1523/JNEUROSCI.4317-03.2004
Cone, J. J., Mccutcheon, J. E., and Roitman, M. F. (2014). Ghrelin acts as an interface between physiological state and phasic dopamine signaling. J. Neurosci. 34, 4905–4913. doi: 10.1523/JNEUROSCI.4404-13.2014
Curtis, C. E., and D’esposito, M. (2003). Persistent activity in the prefrontal cortex during working memory. Trends Cogn. Sci. 7, 415–423. doi: 10.1016/s1364-6613(03)00197-9
D’souza, D. C., Perry, E., Macdougall, L., Ammerman, Y., Cooper, T., Wu, Y. T., et al. (2004). The psychotomimetic effects of intravenous delta-9-tetrahydrocannabinol in healthy individuals: implications for psychosis. Neuropsychopharmacology 29, 1558–1572. doi: 10.1038/sj.npp.1300496
Denier, N., Schmidt, A., Gerber, H., Schmid, O., Riecher-Rossler, A., Wiesbeck, G. A., et al. (2013). Association of frontal gray matter volume and cerebral perfusion in heroin addiction: a multimodal neuroimaging study. Front. Psychiatry 4:135. doi: 10.3389/fpsyt.2013.00135
Dobi, A., Margolis, E. B., Wang, H. L., Harvey, B. K., and Morales, M. (2010). Glutamatergic and nonglutamatergic neurons of the ventral tegmental area establish local synaptic contacts with dopaminergic and nondopaminergic neurons. J. Neurosci. 30, 218–229. doi: 10.1523/JNEUROSCI.3884-09.2010
Durand-De Cuttoli, R., Mondoloni, S., Marti, F., Lemoine, D., Nguyen, C., Naude, J., et al. (2018). Manipulating midbrain dopamine neurons and reward-related behaviors with light-controllable nicotinic acetylcholine receptors. Elife 7:e37487. doi: 10.7554/eLife.37487
Eban-Rothschild, A., Rothschild, G., Giardino, W. J., Jones, J. R., and De Lecea, L. (2016). VTA dopaminergic neurons regulate ethologically relevant sleep-wake behaviors. Nat. Neurosci. 19, 1356–1366. doi: 10.1038/nn.4377
Faget, L., Osakada, F., Duan, J., Ressler, R., Johnson, A. B., Proudfoot, J. A., et al. (2016). Afferent inputs to neurotransmitter-defined cell types in the ventral tegmental area. Cell Rep. 15, 2796–2808. doi: 10.1016/j.celrep.2016.05.057
Fields, H. L., and Margolis, E. B. (2015). Understanding opioid reward. Trends Neurosci. 38, 217–225. doi: 10.1016/j.tins.2015.01.002
Fortin, G. M., Bourque, M. J., Mendez, J. A., Leo, D., Nordenankar, K., Birgner, C., et al. (2012). Glutamate corelease promotes growth and survival of midbrain dopamine neurons. J. Neurosci. 32, 17477–17491. doi: 10.1523/JNEUROSCI.1939-12.2012
Fulton, S., Pissios, P., Manchon, R. P., Stiles, L., Frank, L., Pothos, E. N., et al. (2006). Leptin regulation of the mesoaccumbens dopamine pathway. Neuron 51, 811–822. doi: 10.1016/j.neuron.2006.09.006
Galaj, E., Han, X., Shen, H., Jordan, C. J., He, Y., Humburg, B., et al. (2020). Dissecting the role of GABA neurons in the VTA versus SNr in opioid reward. J. Neurosci. 40, 8853–8869. doi: 10.1523/JNEUROSCI.0988-20.2020
Gao, M., Jin, Y., Yang, K., Zhang, D., Lukas, R. J., and Wu, J. (2010). Mechanisms involved in systemic nicotine-induced glutamatergic synaptic plasticity on dopamine neurons in the ventral tegmental area. J. Neurosci. 30, 13814–13825. doi: 10.1523/JNEUROSCI.1943-10.2010
Gorelova, N., Mulholland, P. J., Chandler, L. J., and Seamans, J. K. (2012). The glutamatergic component of the mesocortical pathway emanating from different subregions of the ventral midbrain. Cereb. Cortex 22, 327–336. doi: 10.1093/cercor/bhr107
Grieder, T. E., Herman, M. A., Contet, C., Tan, L. A., Vargas-Perez, H., Cohen, A., et al. (2014). VTA CRF neurons mediate the aversive effects of nicotine withdrawal and promote intake escalation. Nat. Neurosci. 17, 1751–1758. doi: 10.1038/nn.3872
Guo, J., Simmons, W. K., Herscovitch, P., Martin, A., and Hall, K. D. (2014). Striatal dopamine D2-like receptor correlation patterns with human obesity and opportunistic eating behavior. Mol. Psychiatry 19, 1078–1084. doi: 10.1038/mp.2014.102
Gutierrez, R., Lobo, M. K., Zhang, F., and De Lecea, L. (2011). Neural integration of reward, arousal, and feeding: recruitment of VTA, lateral hypothalamus, and ventral striatal neurons. IUBMB Life 63, 824–830. doi: 10.1002/iub.539
Haber, S. N., and Fudge, J. L. (1997). The primate substantia nigra and VTA: integrative circuitry and function. Crit. Rev. Neurobiol. 11, 323–342. doi: 10.1615/critrevneurobiol.v11.i4.40
Han, X., He, Y., Bi, G. H., Zhang, H. Y., Song, R., Liu, Q. R., et al. (2017). CB1 receptor activation on VGLUT2-expressing glutamatergic neurons underlies delta(9)-tetrahydrocannabinol (Delta(9)-THC)-induced aversive effects in mice. Sci. Rep. 7:12315. doi: 10.1038/s41598-017-12399-z
Han, Y., Zhang, Y., Kim, H., Grayson, V. S., Jovasevic, V., Ren, W., et al. (2020). Excitatory VTA to DH projections provide a valence signal to memory circuits. Nat. Commun. 11:1466. doi: 10.1038/s41467-020-15035-z
Heikkinen, A. E., Moykkynen, T. P., and Korpi, E. R. (2009). Long-lasting modulation of glutamatergic transmission in VTA dopamine neurons after a single dose of benzodiazepine agonists. Neuropsychopharmacology 34, 290–298. doi: 10.1038/npp.2008.89
Hjelmstad, G. O., Xia, Y., Margolis, E. B., and Fields, H. L. (2013). Opioid modulation of ventral pallidal afferents to ventral tegmental area neurons. J. Neurosci. 33, 6454–6459. doi: 10.1523/JNEUROSCI.0178-13.2013
Hnasko, T. S., Chuhma, N., Zhang, H., Goh, G. Y., Sulzer, D., Palmiter, R. D., et al. (2010). Vesicular glutamate transport promotes dopamine storage and glutamate corelease in vivo. Neuron 65, 643–656. doi: 10.1016/j.neuron.2010.02.012
Hnasko, T. S., Hjelmstad, G. O., Fields, H. L., and Edwards, R. H. (2012). Ventral tegmental area glutamate neurons: electrophysiological properties and projections. J. Neurosci. 32, 15076–15085. doi: 10.1523/JNEUROSCI.3128-12.2012
Hommel, J. D., Trinko, R., Sears, R. M., Georgescu, D., Liu, Z. W., Gao, X. B., et al. (2006). Leptin receptor signaling in midbrain dopamine neurons regulates feeding. Neuron 51, 801–810. doi: 10.1016/j.neuron.2006.08.023
Hyman, C., Juhasz, M., Jackson, C., Wright, P., Ip, N. Y., and Lindsay, R. M. (1994). Overlapping and distinct actions of the neurotrophins BDNF, NT-3, and NT-4/5 on cultured dopaminergic and GABAergic neurons of the ventral mesencephalon. J. Neurosci. 14, 335–347. doi: 10.1523/JNEUROSCI.14-01-00335.1994
Ikemoto, S. (2007). Dopamine reward circuitry: two projection systems from the ventral midbrain to the nucleus accumbens-olfactory tubercle complex. Brain Res. Rev. 56, 27–78. doi: 10.1016/j.brainresrev.2007.05.004
Jalabert, M., Bourdy, R., Courtin, J., Veinante, P., Manzoni, O. J., Barrot, M., et al. (2011). Neuronal circuits underlying acute morphine action on dopamine neurons. Proc. Natl. Acad. Sci. U.S.A. 108, 16446–16450. doi: 10.1073/pnas.1105418108
Jayaraman, A., Nishimori, T., Dobner, P., and Uhl, G. R. (1990). Cholecystokinin and neurotensin mRNAs are differentially expressed in subnuclei of the ventral tegmental area. J. Comp. Neurol. 296, 291–302. doi: 10.1002/cne.902960209
John, W. S., Martin, T. J., Solingapuram Sai, K. K., Nader, S. H., Gage, H. D., Mintz, A., et al. (2018). Chronic delta(9)-THC in rhesus monkeys: effects on cognitive performance and dopamine D2/D3 receptor availability. J. Pharmacol. Exp. Ther. 364, 300–310. doi: 10.1124/jpet.117.244194
Kempadoo, K. A., Mosharov, E. V., Choi, S. J., Sulzer, D., and Kandel, E. R. (2016). Dopamine release from the locus coeruleus to the dorsal hippocampus promotes spatial learning and memory. Proc. Natl. Acad. Sci. U.S.A. 113, 14835–14840. doi: 10.1073/pnas.1616515114
Kempadoo, K. A., Tourino, C., Cho, S. L., Magnani, F., Leinninger, G. M., Stuber, G. D., et al. (2013). Hypothalamic neurotensin projections promote reward by enhancing glutamate transmission in the VTA. J. Neurosci. 33, 7618–7626. doi: 10.1523/JNEUROSCI.2588-12.2013
Kieffer, B. L., and Gaveriaux-Ruff, C. (2002). Exploring the opioid system by gene knockout. Prog. Neurobiol. 66, 285–306. doi: 10.1016/s0301-0082(02)00008-4
Klink, R., De Kerchove D’exaerde, A., Zoli, M., and Changeux, J.-P. (2001). Molecular and physiological diversity of nicotinic acetylcholine receptors in the midbrain dopaminergic nuclei. J. Neurosci. 21, 1452–1463. doi: 10.1523/JNEUROSCI.21-05-01452.2001
Kosel, M., Noss, R. S., Hammig, R., Wielepp, P., Bundeli, P., Heidbreder, R., et al. (2008). Cerebral blood flow effects of acute intravenous heroin administration. Eur. Neuropsychopharmacol. 18, 278–285. doi: 10.1016/j.euroneuro.2007.11.007
Krashes, M. J., Lowell, B. B., and Garfield, A. S. (2016). Melanocortin-4 receptor-regulated energy homeostasis. Nat. Neurosci. 19, 206–219. doi: 10.1038/nn.4202
Lammel, S., Lim, B. K., Ran, C., Huang, K. W., Betley, M. J., Tye, K. M., et al. (2012). Input-specific control of reward and aversion in the ventral tegmental area. Nature 491, 212–217. doi: 10.1038/nature11527
Lavin, A., Nogueira, L., Lapish, C. C., Wightman, R. M., Phillips, P. E., and Seamans, J. K. (2005). Mesocortical dopamine neurons operate in distinct temporal domains using multimodal signaling. J. Neurosci. 25, 5013–5023. doi: 10.1523/JNEUROSCI.0557-05.2005
Lecca, S., Meye, F. J., Trusel, M., Tchenio, A., Harris, J., Schwarz, M. K., et al. (2017). Aversive stimuli drive hypothalamus-to-habenula excitation to promote escape behavior. Elife 6:e30697. doi: 10.7554/eLife.30697
Li, X., Qi, J., Yamaguchi, T., Wang, H. L., and Morales, M. (2013). Heterogeneous composition of dopamine neurons of the rat A10 region: molecular evidence for diverse signaling properties. Brain Struct. Funct. 218, 1159–1176. doi: 10.1007/s00429-012-0452-z
Liang, C. L., Sinton, C. M., Sonsalla, P. K., and German, D. C. (1996). Midbrain dopaminergic neurons in the mouse that contain calbindin-D28k exhibit reduced vulnerability to MPTP-induced neurodegeneration. Neurodegeneration 5, 313–318. doi: 10.1006/neur.1996.0042
Lisman, J. E., and Grace, A. A. (2005). The hippocampal-VTA loop: controlling the entry of information into long-term memory. Neuron 46, 703–713. doi: 10.1016/j.neuron.2005.05.002
Lupica, C. R., and Riegel, A. C. (2005). Endocannabinoid release from midbrain dopamine neurons: a potential substrate for cannabinoid receptor antagonist treatment of addiction. Neuropharmacology 48, 1105–1116. doi: 10.1016/j.neuropharm.2005.03.016
Mangieri, L. R., Jiang, Z., Lu, Y., Xu, Y., Cassidy, R. M., Justice, N., et al. (2019). Defensive behaviors driven by a hypothalamic-ventral midbrain circuit. eNeuro 6, 1–19. doi: 10.1523/ENEURO.0156-19.2019
Mangieri, L. R., Lu, Y., Xu, Y., Cassidy, R. M., Xu, Y., Arenkiel, B. R., et al. (2018). A neural basis for antagonistic control of feeding and compulsive behaviors. Nat. Commun. 9:52. doi: 10.1038/s41467-017-02534-9
Mansbach, R. S., Nicholson, K. L., Martin, B. R., and Balster, R. L. (1994). Failure of Delta(9)-tetrahydrocannabinol and CP 55,940 to maintain intravenous self-administration under a fixed-interval schedule in rhesus monkeys. Behav. Pharmacol. 5, 219–225. doi: 10.1097/00008877-199404000-00014
Mansvelder, H. D., and Mcgehee, D. S. (2002). Cellular and synaptic mechanisms of nicotine addiction. J. Neurobiol. 53, 606–617. doi: 10.1002/neu.10148
Mao, D., Gallagher, K., and Mcgehee, D. S. (2011). Nicotine potentiation of excitatory inputs to ventral tegmental area dopamine neurons. J. Neurosci. 31, 6710–6720. doi: 10.1523/JNEUROSCI.5671-10.2011
Matsui, A., Jarvie, B. C., Robinson, B. G., Hentges, S. T., and Williams, J. T. (2014). Separate GABA afferents to dopamine neurons mediate acute action of opioids, development of tolerance, and expression of withdrawal. Neuron 82, 1346–1356. doi: 10.1016/j.neuron.2014.04.030
Matsui, A., and Williams, J. T. (2011). Opioid-sensitive GABA inputs from rostromedial tegmental nucleus synapse onto midbrain dopamine neurons. J. Neurosci. 31, 17729–17735. doi: 10.1523/JNEUROSCI.4570-11.2011
Matus-Amat, P., Higgins, E. A., Barrientos, R. M., and Rudy, J. W. (2004). The role of the dorsal hippocampus in the acquisition and retrieval of context memory representations. J. Neurosci. 24, 2431–2439. doi: 10.1523/JNEUROSCI.1598-03.2004
Mcgovern, D. J., Polter, A. M., and Root, D. H. (2021). Neurochemical signaling of reward and aversion to ventral tegmental area glutamate neurons. J. Neurosci. 41, 5471–5486. doi: 10.1523/JNEUROSCI.1419-20.2021
Mcnamara, C. G., Tejero-Cantero, A., Trouche, S., Campo-Urriza, N., and Dupret, D. (2014). Dopaminergic neurons promote hippocampal reactivation and spatial memory persistence. Nat. Neurosci. 17, 1658–1660. doi: 10.1038/nn.3843
Melis, M., Gessa, G. L., and Diana, M. (2000). Different mechanisms for dopaminergic excitation induced by opiates and cannabinoids in the rat midbrain. Prog. Neuropsychopharmacol. Biol. Psychiatry 24, 993–1006. doi: 10.1016/s0278-5846(00)00119-6
Melis, M., Perra, S., Muntoni, A. L., Pillolla, G., Lutz, B., Marsicano, G., et al. (2004). Prefrontal cortex stimulation induces 2-arachidonoyl-glycerol-mediated suppression of excitation in dopamine neurons. J. Neurosci. 24, 10707–10715. doi: 10.1523/JNEUROSCI.3502-04.2004
Mietlicki-Baase, E. G., Ortinski, P. I., Reiner, D. J., Sinon, C. G., Mccutcheon, J. E., Pierce, R. C., et al. (2014). Glucagon-like peptide-1 receptor activation in the nucleus accumbens core suppresses feeding by increasing glutamatergic AMPA/kainate signaling. J. Neurosci. 34, 6985–6992. doi: 10.1523/JNEUROSCI.0115-14.2014
Miller, E. K., and Cohen, J. D. (2001). An integrative theory of prefrontal cortex function. Annu. Rev. Neurosci. 24, 167–202. doi: 10.1146/annurev.neuro.24.1.167
Miranda-Barrientos, J., Chambers, I., Mongia, S., Liu, B., Wang, H. L., Mateo-Semidey, G. E., et al. (2021). Ventral tegmental area GABA, glutamate, and glutamate-GABA neurons are heterogeneous in their electrophysiological and pharmacological properties. Eur. J. Neurosci. 54, 4061–4084. doi: 10.1111/ejn.15156
Mongia, S., Yamaguchi, T., Liu, B., Zhang, S., Wang, H., and Morales, M. (2019). The Ventral tegmental Area has calbindin neurons with the capability to co-release glutamate and dopamine into the nucleus accumbens. Eur. J. Neurosci. 50, 3968–3984. doi: 10.1111/ejn.14493
Montardy, Q., Zhou, Z., Lei, Z., Liu, X., Zeng, P., Chen, C., et al. (2019). Characterization of glutamatergic VTA neural population responses to aversive and rewarding conditioning in freely-moving mice. Sci. Bull. 64, 1167–1178. doi: 10.1016/j.scib.2019.05.005
Morales, M., and Margolis, E. B. (2017). Ventral tegmental area: cellular heterogeneity, connectivity and behaviour. Nat. Rev. Neurosci. 18, 73–85. doi: 10.1038/nrn.2016.165
Morales, M., and Root, D. H. (2014). Glutamate neurons within the midbrain dopamine regions. Neuroscience 282, 60–68. doi: 10.1016/j.neuroscience.2014.05.032
Moss, J., Ungless, M. A., and Bolam, J. P. (2011). Dopaminergic axons in different divisions of the adult rat striatal complex do not express vesicular glutamate transporters. Eur. J. Neurosci. 33, 1205–1211. doi: 10.1111/j.1460-9568.2011.07594.x
Nair-Roberts, R. G., Chatelain-Badie, S. D., Benson, E., White-Cooper, H., Bolam, J. P., and Ungless, M. A. (2008). Stereological estimates of dopaminergic, GABAergic and glutamatergic neurons in the ventral tegmental area, substantia nigra and retrorubral field in the rat. Neuroscience 152, 1024–1031. doi: 10.1016/j.neuroscience.2008.01.046
Narayanan, N. S., Guarnieri, D. J., and Dileone, R. J. (2010). Metabolic hormones, dopamine circuits, and feeding. Front. Neuroendocrinol. 31, 104–112. doi: 10.1016/j.yfrne.2009.10.004
Nieh, E. H., Vander Weele, C. M., Matthews, G. A., Presbrey, K. N., Wichmann, R., Leppla, C. A., et al. (2016). Inhibitory input from the lateral hypothalamus to the ventral tegmental area disinhibits dopamine neurons and promotes behavioral activation. Neuron 90, 1286–1298. doi: 10.1016/j.neuron.2016.04.035
Ntamati, N. R., and Luscher, C. (2016). VTA projection neurons releasing GABA and glutamate in the dentate gyrus. eNeuro 3, 1–12. doi: 10.1523/ENEURO.0137-16.2016
Oishi, Y., Suzuki, Y., Takahashi, K., Yonezawa, T., Kanda, T., Takata, Y., et al. (2017). Activation of ventral tegmental area dopamine neurons produces wakefulness through dopamine D2-like receptors in mice. Brain Struct. Funct. 222, 2907–2915. doi: 10.1007/s00429-017-1365-7
Omelchenko, N., and Sesack, S. R. (2009). Ultrastructural analysis of local collaterals of rat ventral tegmental area neurons: GABA phenotype and synapses onto dopamine and GABA cells. Synapse 63, 895–906. doi: 10.1002/syn.20668
Papathanou, M., Creed, M., Dorst, M. C., Bimpisidis, Z., Dumas, S., Pettersson, H., et al. (2018). Targeting vglut2 in mature dopamine neurons decreases mesoaccumbal glutamatergic transmission and identifies a role for glutamate co-release in synaptic plasticity by increasing baseline AMPA/NMDA ratio. Front. Neural. Circuits 12:64. doi: 10.3389/fncir.2018.00064
Pape, H. C. (1996). Queer current and pacemaker: the hyperpolarization-activated cation current in neurons. Annu. Rev. Physiol. 58, 299–327. doi: 10.1146/annurev.ph.58.030196.001503
Parsons, L. H., and Hurd, Y. L. (2015). Endocannabinoid signalling in reward and addiction. Nat. Rev. Neurosci. 16, 579–594. doi: 10.1038/nrn4004
Perello, M., and Zigman, J. M. (2012). The role of ghrelin in reward-based eating. Biol. Psychiatry 72, 347–353. doi: 10.1016/j.biopsych.2012.02.016
Pezze, M. A., and Feldon, J. (2004). Mesolimbic dopaminergic pathways in fear conditioning. Prog. Neurobiol. 74, 301–320. doi: 10.1016/j.pneurobio.2004.09.004
Polter, A. M., and Kauer, J. A. (2014). Stress and VTA synapses: implications for addiction and depression. Eur. J. Neurosci. 39, 1179–1188. doi: 10.1111/ejn.12490
Price, J. L., and Drevets, W. C. (2010). Neurocircuitry of mood disorders. Neuropsychopharmacology 35, 192–216.
Qi, J., Zhang, S., Wang, H. L., Barker, D. J., Miranda-Barrientos, J., and Morales, M. (2016). VTA glutamatergic inputs to nucleus accumbens drive aversion by acting on GABAergic interneurons. Nat. Neurosci. 19, 725–733. doi: 10.1038/nn.4281
Reeves, K. C., Kube, M. J., Grecco, G. G., Fritz, B. M., Munoz, B., Yin, F., et al. (2021). Mu opioid receptors on vGluT2-expressing glutamatergic neurons modulate opioid reward. Addict. Biol. 26:e12942. doi: 10.1111/adb.12942
Robinson, R. B., and Siegelbaum, S. A. (2003). Hyperpolarization-activated cation currents: from molecules to physiological function. Annu. Rev. Physiol. 65, 453–480. doi: 10.1146/annurev.physiol.65.092101.142734
Root, D. H., Barker, D. J., Estrin, D. J., Miranda-Barrientos, J. A., Liu, B., Zhang, S., et al. (2020). Distinct signaling by ventral tegmental area glutamate, GABA, and combinatorial glutamate-GABA neurons in motivated behavior. Cell Rep. 32:108094. doi: 10.1016/j.celrep.2020.108094
Root, D. H., Estrin, D. J., and Morales, M. (2018a). Aversion or salience signaling by ventral tegmental area glutamate neurons. iScience 2, 51–62. doi: 10.1016/j.isci.2018.03.008
Root, D. H., Mejias-Aponte, C. A., Zhang, S., Wang, H. L., Hoffman, A. F., Lupica, C. R., et al. (2014). Single rodent mesohabenular axons release glutamate and GABA. Nat. Neurosci. 17, 1543–1551. doi: 10.1038/nn.3823
Root, D. H., Wang, H. L., Liu, B., Barker, D. J., Mod, L., Szocsics, P., et al. (2016). Glutamate neurons are intermixed with midbrain dopamine neurons in nonhuman primates and humans. Sci. Rep. 6:30615. doi: 10.1038/srep30615
Root, D. H., Zhang, S., Barker, D. J., Miranda-Barrientos, J., Liu, B., Wang, H. L., et al. (2018b). Selective brain distribution and distinctive synaptic architecture of dual glutamatergic-GABAERGIC neurons. Cell Rep. 23, 3465–3479. doi: 10.1016/j.celrep.2018.05.063
Rosen, Z. B., Cheung, S., and Siegelbaum, S. A. (2015). Midbrain dopamine neurons bidirectionally regulate CA3-CA1 synaptic drive. Nat. Neurosci. 18, 1763–1771. doi: 10.1038/nn.4152
Rossi, M. A., and Stuber, G. D. (2018). Overlapping brain circuits for homeostatic and hedonic feeding. Cell Metab. 27, 42–56. doi: 10.1016/j.cmet.2017.09.021
Salamone, J. D., and Correa, M. (2012). The mysterious motivational functions of mesolimbic dopamine. Neuron 76, 470–485. doi: 10.1016/j.neuron.2012.10.021
Schlaepfer, T. E., Strain, E. C., Greenberg, B. D., Preston, K. L., Lancaster, E., Bigelow, G. E., et al. (1998). Site of opioid action in the human brain: mu and kappa agonists’ subjective and cerebral blood flow effects. Am. J. Psychiatry 155, 470–473. doi: 10.1176/ajp.155.4.470
Schulden, J. D., Thomas, Y. F., and Compton, W. M. (2009). Substance abuse in the United States: findings from recent epidemiologic studies. Curr. Psychiatry Rep. 11, 353–359. doi: 10.1007/s11920-009-0053-6
Seroogy, K., Ceccatelli, S., Schalling, M., Hokfelt, T., Frey, P., Walsh, J., et al. (1988). A subpopulation of dopaminergic neurons in rat ventral mesencephalon contains both neurotensin and cholecystokinin. Brain Res. 455, 88–98. doi: 10.1016/0006-8993(88)90117-5
Steffensen, S. C., Stobbs, S. H., Colago, E. E., Lee, R. S., Koob, G. F., Gallegos, R. A., et al. (2006). Contingent and non-contingent effects of heroin on mu-opioid receptor-containing ventral tegmental area GABA neurons. Exp. Neurol. 202, 139–151. doi: 10.1016/j.expneurol.2006.05.023
Steinkellner, T., Zell, V., Farino, Z. J., Sonders, M. S., Villeneuve, M., Freyberg, R. J., et al. (2018). Role for VGLUT2 in selective vulnerability of midbrain dopamine neurons. J. Clin. Invest. 128, 774–788. doi: 10.1172/JCI95795
Stopper, C. M., and Floresco, S. B. (2014). What’s better for me? Fundamental role for lateral habenula in promoting subjective decision biases. Nat. Neurosci. 17, 33–35. doi: 10.1038/nn.3587
Stuber, G. D., Hnasko, T. S., Britt, J. P., Edwards, R. H., and Bonci, A. (2010). Dopaminergic terminals in the nucleus accumbens but not the dorsal striatum corelease glutamate. J. Neurosci. 30, 8229–8233. doi: 10.1523/JNEUROSCI.1754-10.2010
Sutton, A. K., Myers, M. G. Jr., and Olson, D. P. (2016). The role of PVH circuits in leptin action and energy balance. Annu. Rev. Physiol. 78, 207–221. doi: 10.1146/annurev-physiol-021115-105347
Takamori, S., Rhee, J. S., Rosenmund, C., and Jahn, R. (2000). Identification of a vesicular glutamate transporter that defines a glutamatergic phenotype in neurons. Nature 407, 189–194. doi: 10.1038/35025070
Takeuchi, T., Duszkiewicz, A. J., Sonneborn, A., Spooner, P. A., Yamasaki, M., Watanabe, M., et al. (2016). Locus coeruleus and dopaminergic consolidation of everyday memory. Nature 537, 357–362. doi: 10.1038/nature19325
Taylor, S. R., Badurek, S., Dileone, R. J., Nashmi, R., Minichiello, L., and Picciotto, M. R. (2014). GABAERGIC and glutamatergic efferents of the mouse ventral tegmental area. J. Comp. Neurol. 522, 3308–3334. doi: 10.1002/cne.23603
Tecuapetla, F., Patel, J. C., Xenias, H., English, D., Tadros, I., Shah, F., et al. (2010). Glutamatergic signaling by mesolimbic dopamine neurons in the nucleus accumbens. J. Neurosci. 30, 7105–7110. doi: 10.1523/JNEUROSCI.0265-10.2010
Tolu, S., Eddine, R., Marti, F., David, V., Graupner, M., Pons, S., et al. (2013). Co-activation of VTA DA and GABA neurons mediates nicotine reinforcement. Mol. Psychiatry 18, 382–393. doi: 10.1038/mp.2012.83
Tsai, H. C., Zhang, F., Adamantidis, A., Stuber, G. D., Bonci, A., De Lecea, L., et al. (2009). Phasic firing in dopaminergic neurons is sufficient for behavioral conditioning. Science 324, 1080–1084. doi: 10.1126/science.1168878
Tyree, S. M., and De Lecea, L. (2017). Lateral hypothalamic control of the ventral tegmental area: reward evaluation and the driving of motivated behavior. Front. Syst. Neurosci. 11:50. doi: 10.3389/fnsys.2017.00050
Van Zessen, R., Phillips, J. L., Budygin, E. A., and Stuber, G. D. (2012). Activation of VTA GABA neurons disrupts reward consumption. Neuron 73, 1184–1194. doi: 10.1016/j.neuron.2012.02.016
Vlachou, S., Nomikos, G. G., Stephens, D. N., and Panagis, G. (2007). Lack of evidence for appetitive effects of delta 9-tetrahydrocannabinol in the intracranial self-stimulation and conditioned place preference procedures in rodents. Behav. Pharmacol. 18, 311–319. doi: 10.1097/FBP.0b013e3282186cf2
Wang, H. L., Qi, J., Zhang, S., Wang, H., and Morales, M. (2015). Rewarding effects of optical stimulation of ventral tegmental area glutamatergic neurons. J. Neurosci. 35, 15948–15954. doi: 10.1523/JNEUROSCI.3428-15.2015
Wang, Y.-M., Gainetdinov, R. R., Fumagalli, F., Xu, F., Jones, S. R., Bock, C. B., et al. (1997). Knockout of the vesicular monoamine transporter 2 gene results in neonatal death and supersensitivity to cocaine and amphetamine. Neuron 19, 1285–1296. doi: 10.1016/s0896-6273(00)80419-5
Wiebelhaus, J. M., Grim, T. W., Owens, R. A., Lazenka, M. F., Sim-Selley, L. J., Abdullah, R. A., et al. (2015). Delta9-tetrahydrocannabinol and endocannabinoid degradative enzyme inhibitors attenuate intracranial self-stimulation in mice. J. Pharmacol. Exp. Ther. 352, 195–207. doi: 10.1124/jpet.114.218677
Williams, C. L., Buchta, W. C., and Riegel, A. C. (2014). CRF-R2 and the heterosynaptic regulation of VTA glutamate during reinstatement of cocaine seeking. J. Neurosci. 34, 10402–10414. doi: 10.1523/JNEUROSCI.0911-13.2014
Wise, R. A., and Morales, M. (2010). A ventral tegmental CRF-glutamate-dopamine interaction in addiction. Brain Res. 1314, 38–43. doi: 10.1016/j.brainres.2009.09.101
Witten, I. B., Steinberg, E. E., Lee, S. Y., Davidson, T. J., Zalocusky, K. A., Brodsky, M., et al. (2011). Recombinase-driver rat lines: tools, techniques, and optogenetic application to dopamine-mediated reinforcement. Neuron 72, 721–733. doi: 10.1016/j.neuron.2011.10.028
Yamaguchi, T., Qi, J., Wang, H. L., Zhang, S., and Morales, M. (2015). Glutamatergic and dopaminergic neurons in the mouse ventral tegmental area. Eur. J. Neurosci. 41, 760–772. doi: 10.1111/ejn.12818
Yamaguchi, T., Sheen, W., and Morales, M. (2007). Glutamatergic neurons are present in the rat ventral tegmental area. Eur. J. Neurosci. 25, 106–118. doi: 10.1111/j.1460-9568.2006.05263.x
Yamaguchi, T., Wang, H. L., Li, X., Ng, T. H., and Morales, M. (2011). Mesocorticolimbic glutamatergic pathway. J. Neurosci. 31, 8476–8490. doi: 10.1523/jneurosci.1598-11.2011
Yan, Y., Peng, C., Arvin, M. C., Jin, X. T., Kim, V. J., Ramsey, M. D., et al. (2018). Nicotinic cholinergic receptors in VTA glutamate neurons modulate excitatory transmission. Cell Rep. 23, 2236–2244. doi: 10.1016/j.celrep.2018.04.062
Yoo, J. H., Zell, V., Gutierrez-Reed, N., Wu, J., Ressler, R., Shenasa, M. A., et al. (2016). Ventral tegmental area glutamate neurons co-release GABA and promote positive reinforcement. Nat. Commun. 7:13697. doi: 10.1038/ncomms13697
You, Z. B., Wang, B., Zitzman, D., Azari, S., and Wise, R. A. (2007). A role for conditioned ventral tegmental glutamate release in cocaine seeking. J. Neurosci. 27, 10546–10555. doi: 10.1523/JNEUROSCI.2967-07.2007
Yu, X., Ba, W., Zhao, G., Ma, Y., Harding, E. C., Yin, L., et al. (2021). Dysfunction of ventral tegmental area GABA neurons causes mania-like behavior. Mol. Psychiatry 26, 5213–5228. doi: 10.1038/s41380-020-0810-9
Yu, X., Li, W., Ma, Y., Tossell, K., Harris, J. J., Harding, E. C., et al. (2019). GABA and glutamate neurons in the VTA regulate sleep and wakefulness. Nat. Neurosci. 22, 106–119. doi: 10.1038/s41593-018-0288-9
Zell, V., Steinkellner, T., Hollon, N. G., Warlow, S. M., Souter, E., Faget, L., et al. (2020). VTA glutamate neuron activity drives positive reinforcement absent dopamine co-release. Neuron 107, 864–873e4. doi: 10.1016/j.neuron.2020.06.011
Keywords: VTA, VGLUT2, dopamine, neural circuits, reward, addiction
Citation: Cai J and Tong Q (2022) Anatomy and Function of Ventral Tegmental Area Glutamate Neurons. Front. Neural Circuits 16:867053. doi: 10.3389/fncir.2022.867053
Received: 31 January 2022; Accepted: 30 March 2022;
Published: 20 May 2022.
Edited by:
Elizabeth Hanson Moss, Baylor College of Medicine, United StatesReviewed by:
Giulia Chiacchierini, Italian Institute of Technology (IIT), ItalyRobert James Wickham, Elizabethtown College, United States
Copyright © 2022 Cai and Tong. This is an open-access article distributed under the terms of the Creative Commons Attribution License (CC BY). The use, distribution or reproduction in other forums is permitted, provided the original author(s) and the copyright owner(s) are credited and that the original publication in this journal is cited, in accordance with accepted academic practice. No use, distribution or reproduction is permitted which does not comply with these terms.
*Correspondence: Qingchun Tong, qingchun.tong@uth.tmc.edu