New approaches for the neuroimaging of gene expression
- 1The Russell H. Morgan Department of Radiology and Radiological Science, Johns Hopkins University School of Medicine, Baltimore, MD, USA
- 2Cellular Imaging Section and Vascular Biology Program, Institute for Cell Engineering, The Johns Hopkins University School of Medicine, Baltimore, MD, USA
- 3F. M. Kirby Research Center for Functional Brain Imaging, Kennedy Krieger Institute, Baltimore, MD, USA
With more than 20,000 genes in the human genome now identified and a similar number of genes in the rat and mouse genome known (Gibbs et al., 2004), elucidating their function has become a major challenge. An important contribution to understanding genetic pathways was made by the development of reporter genes. A reporter gene is a gene whose product can be readily detected and either fused to the gene of interest or cloned instead of that particular gene. Optical reporter genes are the most commonly used and widely developed. Throughout the years, multiple genes have been cloned from a variety of organisms that emit light via bioluminescence or fluorescence, in multiple distinguishable wavelengths. An emerging new class of reporter genes encode for proteins with an affinity for radioisotopes or positron emitter probes. These receptors, transporters, or enzymes can provide quantitative images upon administration of suitable radiolabeled probes (Serganova et al., 2007). Magnetic resonance imaging (MRI) reporter genes are unique among all the reporter genes used with the various imaging modalities, since they can provide information about gene expression that can be co-registered with soft tissue anatomical and functional information (Gilad et al., 2007b). A key feature of reporters for MRI (and other non-invasive imaging techniques) is that they enable serial temporal imaging within the same subject. This is particularly useful for studying dynamic processes, for example, the migration of stem cells and progenitors, neuronal plasticity, the mechanisms of development and adaptation, disease progression, and response to trauma or illness, all of which require serial imaging of the same individual over time.
The process of uncovering reporter genes for molecular-genetic imaging usually begins by searching for a gene with desirable imaging properties, such as the green fluorescent protein (GFP) (Shimomura et al., 1962). Advances in recombinant DNA technology have made it possible to clone and express these reporters in both prokaryotic and eukaryotic cells (de Wet et al., 1985; Chalfie et al., 1994). For most candidate reporter genes, a significant improvement in detection efficiency has been achieved after mutations were systematically introduced (Cormack et al., 1996), or by mutations that created variants that could be detected with multiple excitation frequencies (Shaner et al., 2004; Zhao et al., 2005). Directed evolution is a process of generating a library of genes with random mutations and screening for mutants with a superior signal (e.g., enhanced signal), and then repeating the same process using the improved protein as a starting point for mutagenesis until a significant improvement in detection efficiency is achieved. For example, this process was recently applied to the bacterial cytochrome P450-BM3, a putative MRI sensor for dopamine, and improved its sensitivity 100-fold (Shapiro et al., 2010).
Over the past decade, viral vectors have been widely used for gene delivery to the central nervous system (CNS). Viruses of different types (e.g., adeno-associated virus, herpes simplex virus, and lentivirus) have several advantages: they can carry a large amount of genetic material, from 8 to 150 Kb; they can infect a wide range of cells, including non-dividing cells (as most of the cells in the CNS are); and they lead to transient or stable gene expression, depending on the virus type (Davidson and Breakefield, 2003). Lentiviruses are derived from the immunodeficiency virus type 1 (HIV-1). These are retroviruses in which the genetic material is stored as RNA, and the viral particles are coated with a lipid envelope with embedded glycoproteins. The particle size is approximately 100 nm in diameter and can carry 7–8 Kb of genetic material. Lentiviruses induce a minimal inflammatory response and achieve long-term gene expression in the brain (Davidson and Breakefield, 2003; Jakobsson and Lundberg, 2006).
Lentiviral vectors have been used in the CNS to generate models for diseases and for therapy. They are favored because of their ability to infect a vast range of cells and deliver multiple genes simultaneously with long-term expression. Lentivirus has been used to deliver mutant α-Synuclein into the substantia nigra of rats to generate a model of Parkinson's disease (PD) (Lo Bianco et al., 2002). The large transgene capacity of the lentivirus was used to deliver three genes to the biosynthesis pathway of dopamine, which led to physical improvement in a chemically induced rat model of PD (Azzouz et al., 2002). In addition, stable expression of light-activated channels in the mouse's hippocampus was achieved using lentivirus to study neuronal circuits (Zhang et al., 2007). In the majority of these studies, fluorescent genes were used as reporter genes, which usually allow only ex vivo analysis or an extremely invasive imaging procedure.
One of the genes in which the ability to monitor and visualize its activity via reporter genes has been highly desired, is c-fos, a member of the immediate early gene family (IEG). Initially discovered as a proto-oncogene (Sonnenberg et al., 1989; Sheng and Greenberg, 1990), c-fos is well known for being activated in neurons. It is a transcription factor that is transcribed immediately (within minutes) after extracellular activation, which leads to de novo synthesis of the c-fos protein. The c-fos is then dimerized with c-Jun (another IEG) and activates the transcription of late-response genes, which are the target genes that result in neuronal plasticity (Sheng and Greenberg, 1990). In the CNS, c-fos is induced by several neurotransmitters, Ca2+ influx (Sheng and Greenberg, 1990), and growth factors (NGF, PDGF, and EGF) (Smeyne et al., 1993). The half-life of the c-fos mRNA in nature is 15–20 min (Sheng and Greenberg, 1990) and the protein levels peak after two-to-three hours and almost completely decay within 8 h (Sonnenberg et al., 1989). However, once the c-fos promoter drives the expression of the reporter gene, the half-life will be dependent on the reporter's mRNA and protein properties. For example, the in vitro expression in the CHO (Chinese Hamster Ovary) cell line of mutated green florescent protein (GFP) had a much shorter half-life than the wild type GFP, both of which were under the regulation of the c-fos promoter (Bi et al., 2002). It is interesting to note that, in the same study, it was shown that GFP expression was three times higher under the c-fos promoter than the CMV promoter. In vivo imaging of c-fos expression was tested with several imaging modalities (Wada et al., 2010). High expression in the hippocampus was demonstrated using chemical agents [the GABA antagonist pentylenetetrazol (PTZ) and kainic acid (KA)] in transgenic mice that had the reporter c-fos-LacZ fusion gene. The LacZ is a reporter gene that can be detected using histochemical staining in fixed brain slices. The c-fos-LacZ levels were detected as early as 30 min, peaked after 5 h, and returned to basal levels within 24 h (Smeyne et al., 1993). In another transgenic mice model, the LacZ gene was fused to a gene that encodes to the Tau protein in order to target the expressed protein to the neuronal processes. The fusion protein was under c-fos promoter regulation and was used to demonstrate functional neuronal circuits (Murphy et al., 2004).
Recently, a new approach for imaging changes in gene expression in real-time in deep brain areas associated with post-injury plasticity, in a living animal, was developed (Jouroukhin et al., 2014). A lentivirus that encodes to a yellow fluorescent gene (ZsYellow) driven by the c-fos promoter was introduced into rat cortical neurons. Using an advanced, fiber-based, optical imaging setup that enabled high-resolution imaging of deep brain areas, (Pelled et al., 2006) changes in gene expression were monitored in real-time (Figure 1). The results demonstrated that, in addition to functional MRI and electrophysiological changes that were characterized previously (Pelled et al., 2009; Li et al., 2011; Han et al., 2013), sensory deprivation induced changes in gene expression as well (Jouroukhin et al., 2014). This new approach can be expanded to a large array of promoters that can report with minimal invasiveness on changes in gene expression.
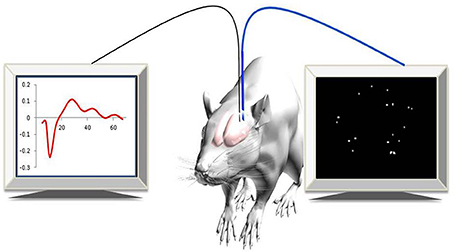
Figure 1. Experimental paradigm. Simultaneous optical and electrophysiology data were recorded in anesthetized rats expressing c-fos::ZsYellow1.
Alternatively, the optical reporter gene can be replaced with reporter genes that were developed for MRI. Rapid improvements in MRI hardware and techniques have led to increased spatial resolution (on the order of 50–100 μm for rodent imaging in vivo), which can be further enhanced by the expression of certain endogenous proteins that increase the MRI contrast (Duyn and Koretsky, 2011). Among a long list of MRI reporter genes that have been developed in the past two decades, there are reporters that are substrate-independent, such as ferritin(Genove et al., 2005; Cohen et al., 2007), the artificial gene called LRP (Lysine-Rich Protein) (Gilad et al., 2007a; Farrar et al., in press) and the human protamine-1 (hPRM-1) (Bar-Shir et al., 2014), which provide contrast based on Chemical Exchange Saturation Transfer (CEST) MRI (van Zijl and Yadav, 2011). These artificial reporters are evolving to become the most suitable for brain imaging, as their contrast is independent of whether the imaging substrate can cross the blood brain barrier.
Conflict of Interest Statement
Assaf A. Gilad is a co-owner of SenCEST LLC. The authors declare that the research was conducted in the absence of any commercial or financial relationships that could be construed as a potential conflict of interest.
Acknowledgments
This study was supported by R01NS072171, R01NS079288, and MSCRFII-0042.
References
Azzouz, M., Martin-Rendon, E., Barber, R. D., Mitrophanous, K. A., Carter, E. E., Rohll, J. B., et al. (2002). Multicistronic lentiviral vector-mediated striatal gene transfer of aromatic L-amino acid decarboxylase, tyrosine hydroxylase, and GTP cyclohydrolase I induces sustained transgene expression, dopamine production, and functional improvement in a rat model of Parkinson's disease. J. Neurosci. 22, 10302–10312.
Bar-Shir, A., Liu, G., Chan, K. W., Oskolkov, N., Song, X., Yadav, N. N., et al. (2014). Human protamine-1 as an MRI reporter gene based on chemical exchange. ACS Chem. Biol. 9, 134–138. doi: 10.1021/cb400617q
Pubmed Abstract | Pubmed Full Text | CrossRef Full Text | Google Scholar
Bi, J. X., Wirth, M., Beer, C., Kim, E. J., Gu, M. B., and Zeng, A. P. (2002). Dynamic characterization of recombinant Chinese hamster ovary cells containing an inducible c-fos promoter GFP expression system as a biomarker. J. Biotechnol. 93, 231–242. doi: 10.1016/S0168-1656(01)00400-X
Pubmed Abstract | Pubmed Full Text | CrossRef Full Text | Google Scholar
Chalfie, M., Tu, Y., Euskirchen, G., Ward, W. W., and Prasher, D. C. (1994). Green fluorescent protein as a marker for gene expression. Science 263, 802–805. doi: 10.1126/science.8303295
Pubmed Abstract | Pubmed Full Text | CrossRef Full Text | Google Scholar
Cohen, B., Ziv, K., Plaks, V., Israely, T., Kalchenko, V., Harmelin, A., et al. (2007). MRI detection of transcriptional regulation of gene expression in transgenic mice. Nat. Med. 13, 498–503. doi: 10.1038/nm1497
Pubmed Abstract | Pubmed Full Text | CrossRef Full Text | Google Scholar
Cormack, B. P., Valdivia, R. H., and Falkow, S. (1996). FACS-optimized mutants of the green fluorescent protein (GFP). Gene 173, 33–38. doi: 10.1016/0378-1119(95)00685-0
Pubmed Abstract | Pubmed Full Text | CrossRef Full Text | Google Scholar
Davidson, B. L., and Breakefield, X. O. (2003). Viral vectors for gene delivery to the nervous system. Nat. Rev. Neurosci. 4, 353–364. doi: 10.1038/nrn1104
Pubmed Abstract | Pubmed Full Text | CrossRef Full Text | Google Scholar
de Wet, J. R., Wood, K. V., Helinski, D. R., and Deluca, M. (1985). Cloning of firefly luciferase cDNA and the expression of active luciferase in Escherichia coli. Proc. Natl. Acad. Sci. U.S.A. 82, 7870–7873. doi: 10.1073/pnas.82.23.7870
Pubmed Abstract | Pubmed Full Text | CrossRef Full Text | Google Scholar
Duyn, J. H., and Koretsky, A. P. (2011). Novel frontiers in ultra-structural and molecular MRI of the brain. Curr. Opin. Neurol. 24, 386–393. doi: 10.1097/WCO.0b013e328348972a
Pubmed Abstract | Pubmed Full Text | CrossRef Full Text | Google Scholar
Farrar, C. T., Buhrman, J. S., Liu, G., Kleijn, A., Lamfers, M. L., McMahon, M. T., et al. (in press). Establishing the lysine-rich protein CEST reporter gene as a CEST-MRI detector for oncolytic virotherapy. Radiology.
Genove, G., Demarco, U., Xu, H., Goins, W. F., and Ahrens, E. T. (2005). A new transgene reporter for in vivo magnetic resonance imaging. Nat. Med. 450–454. doi: 10.1038/nm1208
Pubmed Abstract | Pubmed Full Text | CrossRef Full Text | Google Scholar
Gibbs, R. A., Weinstock, G. M., Metzker, M. L., Muzny, D. M., Sodergren, E. J., Scherer, S., et al. (2004). Genome sequence of the Brown Norway rat yields insights into mammalian evolution. Nature 428, 493–521. doi: 10.1038/nature02426
Pubmed Abstract | Pubmed Full Text | CrossRef Full Text | Google Scholar
Gilad, A. A., Mcmahon, M. T., Walczak, P., Winnard, P. T. Jr., Raman, V., van Laarhoven, H. W., et al. (2007a). Artificial reporter gene providing MRI contrast based on proton exchange. Nat. Biotechnol. 25, 217–219. doi: 10.1038/nbt1277
Pubmed Abstract | Pubmed Full Text | CrossRef Full Text | Google Scholar
Gilad, A. A., Winnard, P. T. Jr., van Zijl, P. C., and Bulte, J. W. (2007b). Developing MR reporter genes: promises and pitfalls. NMR Biomed. 20, 275–290. doi: 10.1002/nbm.1134
Pubmed Abstract | Pubmed Full Text | CrossRef Full Text | Google Scholar
Han, Y., Li, N., Zeiler, S. R., and Pelled, G. (2013). Peripheral nerve injury induces immediate increases in layer v neuronal activity. Neurorehabil. Neural Repair. 27, 664–672. doi: 10.1177/1545968313484811
Pubmed Abstract | Pubmed Full Text | CrossRef Full Text | Google Scholar
Jakobsson, J., and Lundberg, C. (2006). Lentiviral vectors for use in the central nervous system. Mol. Ther. 13, 484–493. doi: 10.1016/j.ymthe.2005.11.012
Pubmed Abstract | Pubmed Full Text | CrossRef Full Text | Google Scholar
Jouroukhin, Y., Nonyane, B. A., Gilad, A. A., and Pelled, G. (2014). Molecular neuroimaging of post-injury plasticity. J. Mol. Neurosci. doi: 10.1007/s12031-014-0347-y
Pubmed Abstract | Pubmed Full Text | CrossRef Full Text | Google Scholar
Li, N., Downey, J. E., Bar-Shir, A., Gilad, A. A., Walczak, P., Kim, H., et al. (2011). Optogenetic-guided cortical plasticity after nerve injury. Proc. Natl. Acad. Sci. U.S.A. 108, 8838–8843. doi: 10.1073/pnas.1100815108
Pubmed Abstract | Pubmed Full Text | CrossRef Full Text | Google Scholar
Lo Bianco, C., Ridet, J. L., Schneider, B. L., Deglon, N., and Aebischer, P. (2002). alpha -Synucleinopathy and selective dopaminergic neuron loss in a rat lentiviral-based model of Parkinson's disease. Proc. Natl. Acad. Sci. U.S.A. 99, 10813–10818. doi: 10.1073/pnas.152339799
Pubmed Abstract | Pubmed Full Text | CrossRef Full Text | Google Scholar
Murphy, M., Greferath, U., Nag, N., Nithianantharajah, J., and Wilson, Y. M. (2004). Tracing functional circuits using c-Fos regulated expression of marker genes targeted to neuronal projections. Front. Biosci. 9:40–47. doi: 10.2741/1203
Pubmed Abstract | Pubmed Full Text | CrossRef Full Text | Google Scholar
Pelled, G., Bergstrom, D. A., Tierney, P. L., Conroy, R. S., Chuang, K. H., Yu, D., et al. (2009). Ipsilateral cortical fMRI responses after peripheral nerve damage in rats reflect increased interneuron activity. Proc. Natl. Acad. Sci. U.S.A. 106, 14114–14119. doi: 10.1073/pnas.0903153106
Pubmed Abstract | Pubmed Full Text | CrossRef Full Text | Google Scholar
Pelled, G., Dodd, S. J., and Koretsky, A. P. (2006). Catheter confocal fluorescence imaging and functional magnetic resonance imaging of local and systems level recovery in the regenerating rodent sciatic nerve. Neuroimage 30, 847–856. doi: 10.1016/j.neuroimage.2005.10.027
Pubmed Abstract | Pubmed Full Text | CrossRef Full Text | Google Scholar
Serganova, I., Ponomarev, V., and Blasberg, R. (2007). Human reporter genes: potential use in clinical studies. Nucl. Med. Biol. 34, 791–807. doi: 10.1016/j.nucmedbio.2007.05.009
Pubmed Abstract | Pubmed Full Text | CrossRef Full Text | Google Scholar
Shaner, N. C., Campbell, R. E., Steinbach, P. A., Giepmans, B. N., Palmer, A. E., and Tsien, R. Y. (2004). Improved monomeric red, orange and yellow fluorescent proteins derived from Discosoma sp. red fluorescent protein. Nat Biotechnol 22, 1567–1572. doi: 10.1038/nbt1037
Pubmed Abstract | Pubmed Full Text | CrossRef Full Text | Google Scholar
Shapiro, M. G., Westmeyer, G. G., Romero, P. A., Szablowski, J. O., Kuster, B., Shah, A., et al. (2010). Directed evolution of a magnetic resonance imaging contrast agent for noninvasive imaging of dopamine. Nat. Biotechnol. 28, 264–270. doi: 10.1038/nbt.1609
Pubmed Abstract | Pubmed Full Text | CrossRef Full Text | Google Scholar
Sheng, M., and Greenberg, M. E. (1990). The regulation and function of c-fos and other immediate early genes in the nervous system. Neuron 4, 477–485. doi: 10.1016/0896-6273(90)90106-P
Pubmed Abstract | Pubmed Full Text | CrossRef Full Text | Google Scholar
Shimomura, O., Johnson, F. H., and Saiga, Y. (1962). Extraction, purification and properties of aequorin, a bioluminescent protein from the luminous hydromedusan, Aequorea. J. Cell. Comp. Physiol. 59, 223–239. doi: 10.1002/jcp.1030590302
Pubmed Abstract | Pubmed Full Text | CrossRef Full Text | Google Scholar
Smeyne, R. J., Schilling, K., Oberdick, J., Robertson, L., Luk, D., Curran, T., et al. (1993). A fos-lac Z transgenic mouse that can be used for neuroanatomic mapping. Adv. Neurol. 59, 285–291.
Sonnenberg, J. L., Macgregor-Leon, P. F., Curran, T., and Morgan, J. I. (1989). Dynamic alterations occur in the levels and composition of transcription factor AP-1 complexes after seizure. Neuron 3, 359–365. doi: 10.1016/0896-6273(89)90260-2
Pubmed Abstract | Pubmed Full Text | CrossRef Full Text | Google Scholar
van Zijl, P. C., and Yadav, N. N. (2011). Chemical exchange saturation transfer (CEST): what is in a name and what isn't? Magn. Reson. Med. 65, 927–948. doi: 10.1002/mrm.22761
Pubmed Abstract | Pubmed Full Text | CrossRef Full Text | Google Scholar
Wada, M., Watanabe, S., Chung, U., Higo, N., Taniguchi, T., and Kitazawa, S. (2010). Noninvasive bioluminescence imaging of c-fos expression in the mouse barrel cortex. Behav. Brain Res. 208, 158–162. doi: 10.1016/j.bbr.2009.11.024
Pubmed Abstract | Pubmed Full Text | CrossRef Full Text | Google Scholar
Zhang, F., Wang, L.-P., Brauner, M., Liewald, J. F., Kay, K., Watzke, N., et al. (2007). Multimodal fast optical interrogation of neural circuitry. Nature 446, 633–639. doi: 10.1038/nature05744
Pubmed Abstract | Pubmed Full Text | CrossRef Full Text | Google Scholar
Zhao, H., Doyle, T. C., Coquoz, O., Kalish, F., Rice, B. W., and Contag, C. H. (2005). Emission spectra of bioluminescent reporters and interaction with mammalian tissue determine the sensitivity of detection in vivo. J. Biomed. Opt. 10, 41210. doi: 10.1117/1.2032388
Pubmed Abstract | Pubmed Full Text | CrossRef Full Text | Google Scholar
Keywords: imaging, brain, c-fos, reporter genes, plasticity
Citation: Gilad AA and Pelled G (2015) New approaches for the neuroimaging of gene expression. Front. Integr. Neurosci. 9:5. doi: 10.3389/fnint.2015.00005
Received: 24 November 2014; Paper pending published: 09 January 2015;
Accepted: 13 January 2015; Published online: 04 February 2015.
Edited by:
Janita Turchi, National Institute of Mental Health, National Institutes of Health, USAReviewed by:
Erik Shapiro, Michigan State University, USAKatrien Vandoorne, Eindhoven University of Technology, Netherlands
Copyright © 2015 Gilad and Pelled. This is an open-access article distributed under the terms of the Creative Commons Attribution License (CC BY). The use, distribution or reproduction in other forums is permitted, provided the original author(s) or licensor are credited and that the original publication in this journal is cited, in accordance with accepted academic practice. No use, distribution or reproduction is permitted which does not comply with these terms.
*Correspondence: pelled@kennedykrieger.org