- 1Institute for Biomagnetism and Biosignalanalysis, University of Münster, Münster, Germany
- 2Department of Integrative Physiology, National Institute for Physiological Sciences, Okazaki, Japan
Over the past 15 years, we have studied plasticity in the human auditory cortex by means of magnetoencephalography (MEG). Two main topics nurtured our curiosity: the effects of musical training on plasticity in the auditory system, and the effects of lateral inhibition. One of our plasticity studies found that listening to notched music for 3 h inhibited the neuronal activity in the auditory cortex that corresponded to the center-frequency of the notch, suggesting suppression of neural activity by lateral inhibition. Subsequent research on this topic found that suppression was notably dependent upon the notch width employed, that the lower notch-edge induced stronger attenuation of neural activity than the higher notch-edge, and that auditory focused attention strengthened the inhibitory networks. Crucially, the overall effects of lateral inhibition on human auditory cortical activity were stronger than the habituation effects. Based on these results we developed a novel treatment strategy for tonal tinnitus—tailor-made notched music training (TMNMT). By notching the music energy spectrum around the individual tinnitus frequency, we intended to attract lateral inhibition to auditory neurons involved in tinnitus perception. So far, the training strategy has been evaluated in two studies. The results of the initial long-term controlled study (12 months) supported the validity of the treatment concept: subjective tinnitus loudness and annoyance were significantly reduced after TMNMT but not when notching spared the tinnitus frequencies. Correspondingly, tinnitus-related auditory evoked fields (AEFs) were significantly reduced after training. The subsequent short-term (5 days) training study indicated that training was more effective in the case of tinnitus frequencies ≤ 8 kHz compared to tinnitus frequencies >8 kHz, and that training should be employed over a long-term in order to induce more persistent effects. Further development and evaluation of TMNMT therapy are planned. A goal is to transfer this novel, completely non-invasive and low-cost treatment approach for tonal tinnitus into routine clinical practice.
Introduction
Chronic tinnitus is a prevalent symptom/syndrome that can severely affect a patient's ability to lead a normal life and can induce psychiatric distress, which may even be associated with the risk of suicide (Coles, 1984). Chronic tinnitus is one of the most common auditory disorders, currently affecting 10–15% of the general adult population (Heller, 2003). In Germany, for instance, there are about 3 Million tinnitus sufferers in need of medical help, and about 1 Million people with tinnitus fail to cope with or compensate for their tinnitus.
The contemporary view on tinnitus biology is that, although tinnitus may be triggered by injury to the inner ear, the neural generators are most readily found centrally. While the neural generators may be primarily auditory, non-auditory centers often participate. Studies of noise-induced tinnitus have given rise to the general theory that tinnitus is triggered by injury to inner ear hair-cell populations. One consequence of such injury is a loss of lateral inhibition in the cortical frequency areas which map to those areas which have been primarily damaged in the periphery; this leads, in turn, to augmented excitation in the regions spectrally neighboring the lesion. This change projects to plastic adjustments in the central auditory system, culminating in altered cortical activity. The theory also holds that central auditory system plasticity is the main centerpiece of these adjustments, whereby reduced auditory nerve input triggers a shift in the balance of excitation and inhibition centrally. This shift leads to the emergence of a tripartite complex of changes that includes hyperactivity, increased bursting activity, and increased synchrony. Such changes reflect a loss of inhibitory drive to neurons, particularly of glycinergic and GABAergic systems, however, increases in excitation via upregulations of glutamatergic and cholinergic systems may also be involved. Such changes are found at multiple levels of the auditory pathway and even in some non-auditory centers, including somatosensory and limbic regions (Martin et al., 1993; Ochi and Eggermont, 1997; Cazals et al., 1998; Norena and Eggermont, 2005; Bauer et al., 2008). This view is consistent with the three-sided nature of tinnitus, which includes auditory, attentional, and emotional components.
To date, there is no standard cure for tinnitus (Rauschecker et al., 2010). One major problem is that there are several different treatment target candidates in the brain (e.g., auditory cortex, thalamus, dorsal/ventral cochlear nuclei, inferior colliculus, cochlear nerve, and the limbic system (Langguth et al., 2010). However, it appears plausible to assume that the auditory cortex should be a treatment target because changes in the auditory cortex must exist when tinnitus is consciously perceived.
During recent years, music has been intensively used as a tool for human brain investigations. Music relates to many human brain functions, such as perception, action, cognition, emotion, learning, and memory, and is therefore an ideal tool to investigate how the human brain works and how different brain functions interact. The positive effects which music, in its various forms, has on the human brain, are not only important in the framework of basic neuroscience but they also have considerable neuro-rehabilitative potential (Pantev and Herholz, 2011).
One of the key features in the processing of auditory information in central auditory structures is lateral inhibition. The afferent auditory pathway is not only formed by excitatory neural connections but also by inhibitory networks. A central auditory pathway neuron is characterized by its tuning curve. Each neuron has a characteristic frequency (CF) to which it is most responsive and is surrounded by other neurons, so that, together, they tonotopically span a range of CFs. If the neuron is excited from a lower level, it not only projects excitation to higher levels but also distributes inhibition laterally via interneuron collaterals to adjacent neurons with higher or lower CFs. This inhibition effect depends on the firing rate of the neuron and on the number of collaterals.
Based on two major foundations—music-induced plasticity and lateral inhibition of the human auditory cortex (which will be discussed in more detail in subsequent sections)—we have developed and evaluated a novel, individualized music training procedure capable of significantly alleviating the perceived loudness of tinnitus. The strategy is based on the notion that circumscribed, hyper-synchronous neuronal activity in the auditory cortex is critical for the emergence of tinnitus perception and it is motivated by neuro-scientific data indicating that maladaptive cortical reorganization processes are associated with tinnitus generation and maintenance. The treatment regimen consists of regularly listening to so-called “tailor-made notched music training (TMNMT),” which is characterized by a suppressed frequency band centered at the individual tinnitus frequency. By having patients listen to this modified music, we intend to decrease tinnitus-related hyper-synchronous auditory cortical activity through the attraction of lateral inhibition. As a consequence, the tinnitus would be perceived as becoming less loud and less distressing. Cumulative tinnitus alleviation would indicate long-term plasticity effects that could be expressed in both primary and secondary auditory cortical structures. A crucial aspect of the TMNMT is that patients are motivated to, and able to, select and listen to their favorite music. Listening to enjoyable music is likely to activate cortical attention networks and also the reward mechanisms of the brain, both of which would promote long-term plasticity. Moreover, in our TMNMT evaluation studies we have not relied solely on behavioral outcome measures; we also recorded tinnitus-related primary and non-primary auditory cortical neuronal activity by means of magnetoencephalography (MEG). We hypothesized that behavioral markers of tinnitus alleviation would correlate positively with electrophysiological markers of tinnitus-related change in auditory cortical neural activity.
Music-Induced Plasticity in the Human Auditory Cortex
In higher mammals including humans, neurons as well as some of their interconnections are formed prenatally into neural networks. For many years, the prevailing opinion was that network connections between neurons are generated primarily during cerebral maturation processes and that they would not change later. However, humans respond with considerable flexibility to new challenges throughout their entire life. Over the last three decades, experimental evidence has demonstrated that the connectivity of the adult brain is only partially determined by genetics and development during the childhood, and may be substantially modified through sensory experiences during adulthood. Thus, the functional organization of the adult brain adjusts in response to the alteration of behaviorally relevant input and processing. This was first demonstrated in a series of classical animal studies (Merzenich et al., 1983a,b; Jenkins et al., 1990; Kaas et al., 1990; Gilbert and Wiesel, 1992; Recanzone et al., 1993; Irvine and Rajan, 1995; Rauschecker et al., 1995, 1997).
The development of new, non-invasive techniques for recording brain activity has enabled neuroscientists to prove the existence of plasticity in functional neuronal networks in humans. One of these techniques, MEG [the magnetic counterpart of electroencephalography (EEG)], has become an established method for the non-invasive study of the spontaneous and evoked activity of the human cortex (Hari, 1990). The main sources of this activity are the pyramidal cells, which generate currents that flow tangentially to the surface of the head. Although MEG measurements provide only a macroscopic view of brain function, the spatial and, especially, the temporal-resolution achieved with this technique is sufficient to give indications of the functional organization and reorganizational plasticity of the human cortex by localizing the sources of evoked magnetic fields, which are elicited by different peripheral stimulation. Thus, using MEG, which allows non-invasive measurement in human subjects, changes in the cortical maps similar to those observed in the primate cortex can be demonstrated.
Auditory Plasticity in Musicians
Music relates to many brain functions and is therefore an ideal tool to investigate how the human brain works. Zatorre et al. (Zatorre et al., 2007) showed that playing a musical instrument, for example the violin, is a highly complex task (cf. Figure 1). The whole body, including almost all sensory systems, is involved in the performance process and has to be coordinated to high degree of synchrony and accuracy. As the arms support the violin and move the bow, and the hand fingers the strings, feedback from the somatosensory perception of the body posture and fingertips is constantly integrated to fine-tune each and every movement. The auditory system has the major control function in this process, analyzing the musical correctness of the sounds produced by the violin, and using this auditory feedback to fine-tune the motor functions and therefore improve the sounds produced. Apart from the motor and sensory systems, memory and attentional, as well as emotional, systems are also involved. Because of this complex interaction, music has developed, over the last 10 years, as a tool for studying brain plasticity in different sensory modalities and as an effective way of changing the brain's functional organization and interaction between brain areas for the purpose of neuro-rehabilitation. It has been demonstrated, in a series of experiments, that musical training has pronounced effects on functional and structural human brain plasticity.
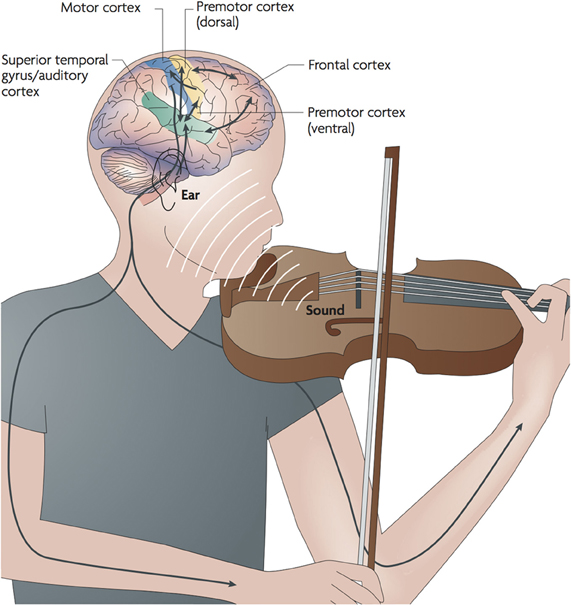
Figure 1. (From Zatorre et al., 2007) The figure illustrates the feedback and the feedforward interactions that occur during music performance. As a musician plays an instrument, motor systems control the fine movements needed to produce sound. The sound is processed by auditory circuitry, which, in turn, is used to adjust motor output to achieve the desired effect. Output signals from premotor cortices are also thought to influence responses within the auditory cortex, even in the absence of sound, or prior to sound; conversely, motor representations are thought to be active on hearing sound, even in the absence of movement. There is, therefore, a tight linkage between sensory and production mechanisms.
On the structural level, larger brain volume has been demonstrated in musicians compared to non-musicians in several brain areas; (auditory processing; Schlaug et al., 1995a,b; Schneider et al., 2002; Gaser and Schlaug, 2003; Bermudez et al., 2009); (visuo-spatial processing; Gaser and Schlaug, 2003; Bermudez et al., 2009); motor control: corpus callosum and precentral gyrus (Schlaug et al., 1995a; Gaser and Schlaug, 2003; Bangert and Schlaug, 2006); and cerebellum (Hutchinson et al., 2003). Such differences not only apply to gray matter: differences in white matter, between musicians and non-musicians, have also been found (Oechslin et al., 2010).
On the functional level, fundamental differences between musicians and non-musicians regarding the processing of sounds, were found not only in the auditory (Pantev et al., 1998; Tervaniemi et al., 2001; Koelsch et al., 2002; Shahin et al., 2003; Fujioka et al., 2004, 2005; Van Zuijen et al., 2004, 2005; Besson et al., 2007), but also in the somatosensory and the motor cortices (Elbert et al., 1995; Lotze et al., 2003). Therefore, in addition to the main modality, neural interactions between modalities are also enhanced in musically trained people (Schulz et al., 2003). The interplay and integration of several modalities are key elements of musical training and performance, and the multimodal integration and co-activation of the cortical areas involved during training might be an important mechanism supporting the training effects within each modality. Generally, the conditions which are most important for cortical reorganization to occur are: (1) the expansion of cortical representation through the increased use, or behaviorally relevant stimulation, of the respective receptor pool and (2) a heavy training schedule, coupled with high-levels of motivation, in a behaviorally relevant context. The results of these different studies performed on musicians confirm that extensive training has a specific and profound effect on the functional organization of the auditory cortex.
Laboratory Demonstrations of Auditory Plasticity
Most studies of musically induced plasticity of the auditory cortex have been based on musicians with years of extensive training. However, training musically naive subjects for a short time in a laboratory environment is even better suited to the direct evaluation of short-term training effects and the possibility of inducing short-term plastic changes.
In one study we investigated this question by having adults, in the laboratory, learning to discriminate spectral versus virtual modes of pitch perception in ambiguous “virtual” melodies (Schulte et al., 2002). A clear change in perception was accompanied by a distinct increase in the transient gamma band response that has been found to be associated with integrative cognitive functions, such as the binding process during object recognition. An independent component analysis, which was performed on the MEG data, indicated greater synchronization of the cortical networks involved in the generation of the evoked gamma band activity after participants had achieved the ability to perceive the virtual melody. In a further study (Menning et al., 2000), we found that frequency discrimination training over the course of 3 weeks led to rapid behavioral improvements that were accompanied by enhanced N1m and mismatch responses to pitch deviations, therefore demonstrating rapid, short-term training-induced plastic changes in the human auditory cortex. In a similar fMRI study, two randomly assigned groups were compared, one of which received auditory discrimination training over the course of 1 week (Jancke et al., 2001). The results showed a differential pattern. Subjects, who improved over the course of the training and also showed improved auditory acuity, had decreased neural activity in auditory areas (planum temporale and superior temporal sulcus) on fMRI testing. Subjects who did not improve over the course of the training, and subjects in the control group (no training), did not have changes in their auditory cortical activity on fMRI measurements before and after 1 week. These results demonstrate that short-term auditory training leads, in general, to plastic changes within the human auditory cortex for the task that is trained, however, these also depend upon the effectiveness of the training for the individual concerned.
As described in Figure 1, playing music is all about the multimodal integration of different sensory modalities and motor functions. We, therefore, asked the following question: is it this multimodal integration that makes musical training so effective in promoting plasticity in the human auditory cortex? Multisensory integration was defined by Meredith and Stein (Meredith and Stein, 1983) as a greater neuronal response to a stimulus consisting of a combination of modalities, compared with the sum of neuronal responses to each stimulus modality separately. The different sensory modalities interact, functionally reorganize, and contribute to new qualities of perception that convey information not inherent in each single modality. We, therefore, put the hypothesis forward that the strong effects of musical training on cortical reorganization might be due to the multimodal nature of musical training. Specifically, we hypothesized that sensorimotor-auditory training, in the context of piano playing, leads to greater plasticity in the human auditory cortex than mere auditory training. This hypothesis was tested in a study of twenty-three non-musicians with no formal musical training, who were assigned randomly to either sensorimotor-auditory or to auditory experimental groups (Lappe et al., 2008). Training-induced plasticity was evaluated by comparing the mismatch negativity responses (MMN) and the performance in an auditory melody discrimination test before and after training.
For the MEG measurements before and after training, we used three- and six-tone piano sequences (Figure 2A). Deviant sequences differed from the standard sequence in that the last tone of the sequence was three semitones lower. During a 2 week training period, the sensorimotor-auditory group learned to play the I–IV–V–I chord progression (Figure 2B) in broken chords, using both hands, from a visual template that was easy to read for musical novices (Figure 2C).
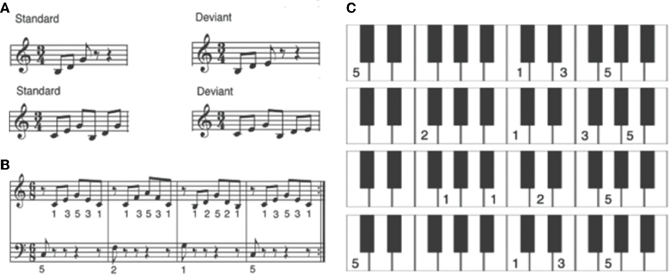
Figure 2. (From Lappe et al., 2008). (A) Tone sequences for the standard and deviant stimuli that were used in the MEG measurements before and after training. (B) Musical score of the I–IV–V–I chord progression in c-major in broken chords that was used as a training sequence for SA and A training. (C) Visual templates for the SA training for each broken chord of the training sequence. Numbers represent the fingers (thumb, 1; index finger, 2; etc.) with which the subjects were supposed to press the corresponding piano keys. On each template, the image of the piano keyboard was depicted and the finger placement was marked. For each chord, the notes were to be played in ascending order first, and then descending again (compare score in B).
In contrast, subjects in the auditory group merely listened to all of the training sessions of one randomly assigned subject from the sensorimotor-auditory group and had to judge the correctness of the heard sequences. As expected, auditory discrimination of short melodies improved more strongly in the group that received the piano training, compared to the listening group, as assessed by both the behavioral test and the electro-physiological MMN data, which are displayed in Figure 3. The source waveforms and statistical analysis clearly showed that the training effect, as seen in the increase from pre- to post-training sessions, was much larger in the sensorimotor-auditory group than in the auditory group. The multimodal sensorimotor-auditory training in non-musicians therefore resulted in greater plastic changes in the auditory cortex than auditory-only training, and this indicates the strength of the effect of sensory-motor practice on auditory representations. In this study we manipulated the subjects' experiences in a well-controlled laboratory setting, randomly assigned them to different groups, and the auditory input was identical for both the sensorimotor-auditory and the auditory group. The study, therefore, enabled us to conclude that musical instrument training which involves both the sensorimotor and the auditory system leads to stronger functional changes in auditory cortical areas than mere auditory training.
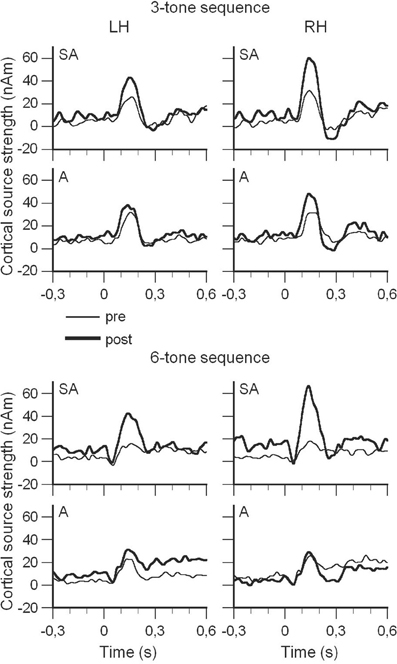
Figure 3. (Modified from Lappe et al., 2008) Group averages of the source waveforms which were obtained after performing source space projection before and after training for both groups (SA = Sensorimotor-Auditory; A = Auditory), stimulus conditions, and hemispheres. Data for the three-tone sequences are shown in the top four panels and data for the six-tone sequences in the bottom four panels. Within each set of four panels, SA group data are shown in the top row, and A group data are shown in the bottom row. Data from the left hemisphere (LH) are presented on the left and those of the right hemisphere (RH) on the right. Thin lines indicate pre-training (pre) data and thick lines post-training (post) data.
Plasticity Induced by Functional Deafferentation
The experiments describe above show the influence of long-term (musical) and short-term (laboratory) training on the processes of cortical reorganization within the human auditory cortex. A complementary approach to investigating plasticity is to remove a band of frequencies from familiar sounds such as music, and determine how brain responses to the eliminated sounds are affected (a functional deafferentation approach).
In a study following this approach (Pantev et al., 1999), normal-hearing subjects listened attentively, for 3 h on 3 consecutive days, to music of their choice from which a narrow frequency band centered at 1 kHz had been removed (notching). Immediately before and after listening to the notched music, MEG measurements of auditory cortical representations were measured for a “test” stimulus (band-passed noise centered at the notched region) and a “control” stimulus (band-passed noise centered one octave below the notched region). The music had been manipulated in such a way that a notch between 0.7 and 1.3 kHz, centered on 1 kHz, was produced using a band rejection filter. It was presented binaurally through earphones at a moderate loudness (about 60–70 dB SPL). The presence of the notch ensured that, during this period of music listening, there was practically no afferent input to cortical neurons tuned to frequencies around 1 kHz. After listening to notched music, the root-mean-square (RMS) values and strength of the corresponding N1m cortical source were decreased in the case of the test stimulus, whereas they remained almost unchanged for the control stimulus. This demonstrated that the notching-out of a band of frequency-specific auditory input decreased the cortical representation of these same frequencies in just a short time period.
These results provide evidence that different organizational structures of cortical representational maps can occur or develop within a time frame as short as a few hours, in this case following functional deafferentation of the adult human auditory cortex. The time course of this notching effect is consistent with animal studies in which selected regions of the cochlea have been deafferented by electrolytic lesions (Robertson and Irvine, 1989). While the reduction in responsiveness at the functionally deafferented cortical region cannot be explained by a habituation effect, lateral inhibition is a very likely explanation (cf. Kadner et al., 2002; Pantev et al., 2004). In this case the neurons with CFs within the notched area of the music were inhibited by their neighboring neurons with CFs outside of the notch, which were stimulated by the music. This short-term plasticity effect, however, reversed within 24 h. An important question is whether longer exposure would extend the duration of this effect. We will return to this question later, where the method of notching is applied to the treatment of tonal tinnitus. That research was based on foundation studies of lateral inhibition in the human auditory cortex, to which we turn next.
Lateral Inhibition of the Human Auditory Cortex
Lateral inhibition plays an important role in the functioning of our sensory modalities. In 1865, Ernst Mach described the “Mach bands” in the visual modality, which are explained by lateral inhibition (Mach, 1865). The model of lateral inhibition within the auditory system was derived from the classic visual lateral inhibition scheme (Von Békésy, 1967). The afferent auditory pathway is formed not only from excitatory neural connections; inhibitory networks also play an important role (as described in the Introduction section). On the basis of one of our previous studies (Pantev et al., 2004), we proposed that inhibition in the human auditory cortex, as mediated by lateral connections, is an active mechanism that innervates inhibitory neurons and causes the attenuation of the auditory evoked response.
Habituation, in contrast, is a neural mechanism that suppresses the activity of neurons, which are repeatedly activated. Habituation seems to play an important role both in suppressing irrelevant neural activity and in enhancing the neural activity elicited by irregular sensory inputs. The “tinnitus retraining therapy” (Jastreboff and Hazell, 1993) focuses on this habituation mechanism. In our first study (Pantev et al., 2004) we compared the decrease in auditory cortical activity induced by habituation vs. lateral inhibition within the same experiment and within the same subjects.
Lateral Inhibition and Habituation
In this study we used a forward masking paradigm and examined the effects of white noise (WN) and comb filtered noise (CFN) maskers. The amplitude spectrum of the CFN consisted of a series of pass and eliminated-band sections with identical widths on the logarithmic frequency scale (Figure 4).
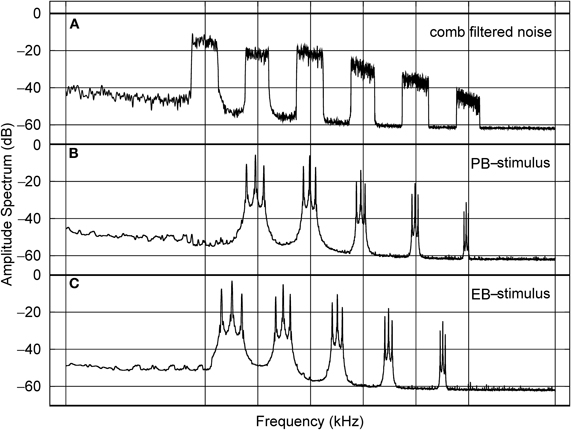
Figure 4. (Modified from Pantev et al. (2004) Amplitude spectra of the comb filtered noise (CFN), the pass-band stimulus (PB), and the eliminated-band stimulus (EB) (rows a, b, and c, respectively). The frequency components of the PB-stimulus correspond to pass-band sections of the CFN, whereas those of the EB-stimulus correspond to eliminated-band sections of the CFN.
Each complex sound contained five spectral components corresponding either to the pass-band sections of the CFN pass-band stimulus (PB stimulus) or to the eliminated-band sections of the CFN eliminated-band stimulus (EB stimulus). The PB- and EB- stimuli evoked N1m responses originating mainly from non-primary auditory structures (Pantev et al., 1995) and, in both cases, this response was reduced in amplitude following a preceding masker (CFN or WN) The main finding, however, was that when a preceding CFN masker was used, the source strengths of the N1m responses elicited by the EB stimuli were significantly lower than those responses that were elicited by the PB stimuli. In contrast, the N1m amplitudes elicited by the both stimuli were not significantly different following the WN masker. Thus, the neural mechanisms causing the N1m decrements depended on the combination of the masking sound and the test sound. The PB stimulus was composed of frequencies corresponding to the pass-bands of the CFN, meaning that those frequencies occurring within the pass-bands were stimulated throughout the presentation of the masker and PB stimulus. Consequently, in this case, the habituation effect must have played a major role in decreasing the N1m response following the CFN masker. On the other hand, the EB stimulus was composed of frequencies corresponding to the eliminated-bands of the CFN and thus only contained the frequency bands neighboring the CFN spectral components. This meant that the neurons responding to the EB stimulus had not been excited during the preceding CFN masker, the consequence of which was that lateral inhibition must have caused the main N1m decrement. In summary, these results indicated that the inhibitory effect mediated by the lateral connections (EB condition) was stronger than the habituation effect (PB condition).
We have also investigated the amount to which the lateral inhibition effect may be dependent on the inter-stimulus interval. Using CFN forward-maskers of 3 s duration and the same test stimuli (TS) (PB and EB) as in the previously described experiment, we then varied the inter-stimulus interval between them (0.5, 1, and 2 s). In this experiment (Okamoto et al., 2004), we demonstrated again that the lateral inhibition effect on the N1m evoked response was significantly larger than the corresponding habituation effect. The N1m decrement was maximal when the time interval between the CFN and the TS was short (0.5 s) but was still detectable at intervals up to 2 s. From this experiment we concluded that stimulating the auditory system for several seconds with a sound containing sharply defined spectral contrasts (CFN) results in a temporal change in the responsiveness of the auditory cortex to complex spectral pattern sounds. Cortical responses corresponding to the non-stimulated frequency bands were more strongly reduced than those responses corresponding to the TS. Thus, the lateral inhibition effect at the level of the auditory cortex was a reasonable explanation, and the experimental results we obtained provided evidence that the effect of inhibition decayed over several seconds.
Several electroencephalographic studies on habituation of the auditory cortex showed that the amplitude of the N1 response was most strongly attenuated when the preceding and subsequent sound stimuli had the same frequency as the test frequency (Butler, 1968; Picton et al., 1974; Pantev et al., 1975; Näätänen and Picton, 1987; Näätänen et al., 1988). However, in addition to this effect upon the neural group with the same receptive field, neural activity corresponding to frequencies adjacent to the test stimulus might play an important role for the neural response (Sutter and Loftus, 2003; Jääskeläinen et al., 2004; Okamoto et al., 2004; Pantev et al., 2004). In the previous experiments the eliminated bandwidths of the CFN were always the same (i.e., 1/4 octave) and so the effect of the width of the eliminated-band itself on the test stimulus remained unknown. We devised a further study to investigate whether an explanation in terms of lateral inhibition in the region of the eliminated-band frequency could be experimentally proved. Therefore, interfering masking sounds, which differed in the frequency domain, were presented between two successive 1 kHz pure tone TS. The masking sounds were WN and various band-eliminated noises (BENs) (1/8, 1/4, 1/2, and 1 octave bandwidths) centered at 1 kHz. The main finding here was that the N1m amplitude corresponding to the 1 kHz test tone was most strongly reduced when the preceding BEN had a 1/4 octave eliminated bandwidth. These experimental results can be explained mainly by the effect of lateral inhibition (Von Békésy, 1967; Ehret and Merzenich, 1988; Burrows and Barry, 1990; Rhode and Greenberg, 1994; Suga, 1995; Sutter et al., 1999) because, in this case, the eliminated bandwidth corresponds to one critical band (CB).
Simultaneous Narrow-Band Noise
The aforementioned studies used forward masking paradigms to investigate lateral inhibitory effects in the human auditory cortex. However, inhibitory neural interactions within human cortical tonotopic maps elicited by complex sounds continue to be poorly understood. A well-known concept in this context is the “CB” introduced by Fletcher (Fletcher, 1940). This concept describes neural groups with similar receptive fields as being part of the same CB and assumes only slight interactions between frequency-distant neuronal populations. However, neural activities elicited by distinct frequencies can influence each other. Jääskeläinen et al. (Jääskeläinen et al., 2004) observed a significant decrease in N1m-induced by a preceding masker despite the TS and masker differing by two octaves. Single cell recording studies applying simultaneous masking paradigms also demonstrated that the frequency tuning curves of auditory cortical neurons had lower and upper inhibitory side bands (Sutter et al., 1999; Sutter and Loftus, 2003; O'Connor et al., 2005). These results indicated that when sounds are presented simultaneously their interaction has non-linear frequency characteristics.
We investigated this aspect of neural interaction further using two simultaneously presented narrow-band noises (NBNs). TS1 consisted of one single NBN with a 40 Hz bandwidth centered at 1000 Hz. The five other TS consisted of two simultaneously presented NBNs each with a 20 Hz bandwidth and center frequencies set equally apart from 1000 Hz. The center-frequency differences between the two NBNs were 1/4 (TS2), 1/2 (TS3), one (TS4), two (TS5), and four (TS6) CBs, respectively. The N1m amplitude was maximal in the case of TS4 (1 CB) and minimal in the case of TS1 (single NBN). The N1m source strength increased gradually until the frequency difference between NBNs matched the CB (from TS1 to TS4) and then decreased with further increment of the frequency difference (Okamoto et al., 2005).
Two simultaneously presented sound stimuli normally activate two distinct neural groups with receptive fields corresponding to the frequency characteristics of these stimuli. However, if the stimulating sounds have very similar frequencies, the receptive fields might overlap, meaning that, within this overlapping region, a single neural group may be excited by the separate stimuli. The frequency overlap gradient between conditions might have caused a gradient in N1m source strength (TS1 > TS2 > TS3 > TS4). However, the receptive field organization of the auditory system alone cannot explain the results we obtained (TS4 > TS5; TS4 > TS6). Auditory neurons corresponding to the frequency components of one of the NBNs of TS5 or TS6 not only receive excitatory ascending inputs from that NBN, but also receive lateral inhibitory inputs from the other NBN, which was separated by more than one CB. Our finding that the N1m amplitude again reduced in response to these stimuli, which were separated by more than one CB, indicates that lateral inhibition mainly works between separate neural groups, which are activated through different peripheral band-pass filters. The results also provide some new insights into these mechanisms, which cause non-linear interactions between excitatory and inhibitory neurons when activated by sounds of different frequencies.
Asymmetric Lateral Inhibition
In summary, lateral inhibition in the auditory system seems to contribute to improving the perceptual contrast by enhancing the spectral edge of the sound stimuli, as we have demonstrated in the human auditory cortex. However, it is not so well-known whether the lateral inhibitory effects of neural connections from the lower and higher spectral regions are similar or different. The auditory peripheral organ, the cochlea, has an asymmetric anatomical composition (Kiang and Moxon, 1974), however, the frequency tuning curve asymmetry becomes less evident in the central auditory neurons (Katsuki et al., 1958; Suga, 1995).
We hypothesized that lateral inhibition in the central auditory pathway might also be asymmetric in order to compensate for the frequency tuning curve asymmetry originating in the cochlea. To test this hypothesis, we measured auditory evoked fields (AEFs) elicited by a TS following exposure to various maskers with single-octave bandwidths eliminated and center frequencies differing in 1/6 octave steps. The goal was to investigate lateral inhibitory effects of the lower and higher spectral edges of BENs on the auditory evoked responses to a subsequent TS. We used a 1000 Hz TS throughout this experiment. The differences in the frequency domain between the TS at 1000 Hz and the lower spectral edges of the BENs were 1/6 octave (BEN1), 2/6 octave (BEN2), 3/6 octave (BEN3), 4/6 octave (BEN4), and 5/6 octave (BEN5). The smallest N1m response occurred after exposure to BEN1 and the largest after exposure to BEN3. The N1m response was also smaller following BEN4 and BEN5 than BEN3, however, the decrease was not symmetric, and was more pronounced for the lower-frequency spectral edge than the higher edge.
This result (Okamoto et al., 2007a) implied that the lower spectral edge of the BEN caused a larger decrease in N1m than the higher spectral edge. We therefore conclude that the asymmetric lateral inhibition in the central auditory pathway contributes by adjusting the asymmetric neural activities originating in the cochlea. This adjustment results in sharper frequency contrasts and better auditory performance.
Effects of Attention on Lateral Inhibition
Another very important issue is the relationship between lateral inhibition and attention. In most day-to-day situations we are exposed to many different types of sound signals simultaneously, yet we can easily perceive selected sounds simply by paying attention to them. This process can be tuned by the enhancement of neural responses corresponding to task-relevant stimuli (gain), by suppression of task-irrelevant neural activities via lateral inhibition (sharpening), or by both.
Previous EEG (Hillyard et al., 1973; Picton et al., 1974) and MEG (Woldorff et al., 1993) studies observed that focused auditory attention increased the N1 auditory response. The sharpening effect of attention, however, had not been demonstrated. We therefore investigated the sharpening effects of focused auditory attention on the population-level frequency tuning in the human auditory cortex by means of MEG. We posited that attention might strengthen not only the excitatory neural connections but also the inhibitory networks (cf. Figure 5), which would contribute to finer frequency tuning and better auditory performance.
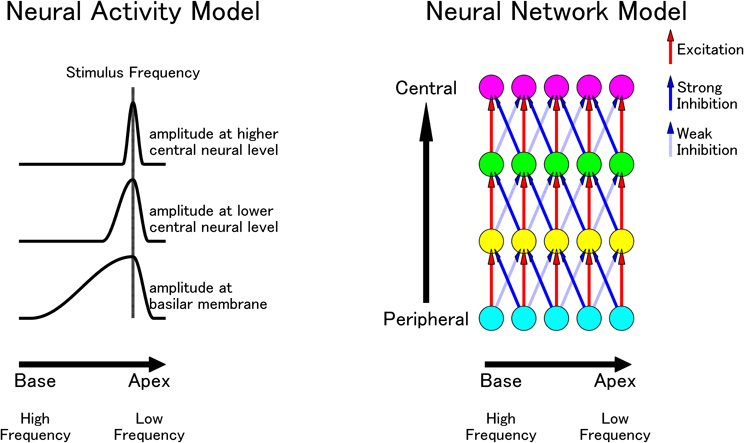
Figure 5. (Modified from Okamoto et al., 2007a,b) A hypothesized neural network in the human auditory system. Left: a schematic diagram of hypothesized neural activity corresponding to a stimulus frequency from the peripheral to the central auditory pathway. Neural activity becomes sharper at more central levels, especially in the high-frequency range. Right: a hypothetical excitatory and inhibitory neural network from the peripheral to the central auditory pathway. Red lines indicate excitatory neural connections and blue lines indicate inhibitory connections. Solid blue lines projecting from lower to higher frequencies have stronger inhibitory effects than the dashed blue lines projecting from higher to lower frequencies.
In this experiment, we used a TS that was presented either independently or simultaneously with four different BENs queued in a random sequence. Neuronal activities evoked by BEN and TS could be divided into three categories: activity evoked exclusively by BEN, activity evoked exclusively by TS, or activity evoked by both BEN and TS (Figures 6B1–B4, light gray, dark gray, black areas, respectively). As shown in Figure 6, the activity of auditory neurons, which can be activated by both BEN and TS decreases as BEN becomes wider and/or frequency tuning becomes sharper. Thus, the diminution of overlapping areas (Figure 6, black areas) and the enlargement of areas activated solely by TS (Figure 6, dark gray areas) illustrate improved population-level frequency tuning. The TS was a 1000 Hz amplitude-modulated tone (40 Hz), and the BENs were prepared as follows: spectral frequency bands with widths of 20 Hz (BEN20), 40 Hz (BEN40), 80 Hz (BEN80), or 160 Hz (BEN160) centered on the 1000 Hz TS carrier frequency were eliminated from WN. To investigate the effects of attention, we contrasted two different attentional conditions: active listening and distracted listening. The results indicated that the population-level frequency tuning became sharper when attention was directed to the auditory domain (Okamoto et al., 2007b).
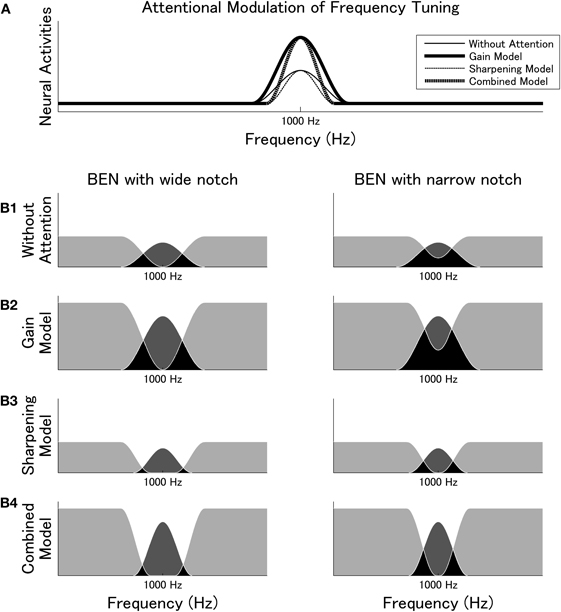
Figure 6. (Modified from Okamoto et al., 2007a,b) Attentional modulation of frequency tuning. (A) Different effects of attention (gain, sharpening, and combined (gain plus sharpening) models) modulate the population-level neural activities corresponding to the 1000 Hz test stimulus. (B1–B4) The relationship between neural activities elicited by BEN and TS as predicted by the different attention models. Light gray areas represent neural activities exclusively elicited by BEN and dark gray areas represent neural activities exclusively elicited by TS. Black areas indicate overlap: neurons in these areas had already been activated by BEN when TS appeared. Dark gray areas represent N1m source strength reflecting TS onset. B1 displays neural activities evoked without focused auditory attention. B2, B3, and B4 illustrate the gain model, the sharpening model, and the combined (gain plus sharpening) model, respectively. Diagrams on the left illustrate BENs with broad spectral notches; diagrams on the right illustrate BENs with narrow spectral notches. It is notable that the size ratios of the dark gray areas between the narrow BEN and the wide BEN differ between models: B3 and B4 have ratios much closer to one than B1 and B2, reflecting the sharpening effect of attention on population-level frequency tuning.
Previous studies have shown that lateral inhibition in the auditory system (Von Békésy, 1967; Suga, 1995; Pantev et al., 2004; Okamoto et al., 2005, 2007a) can sharpen frequency tuning in the auditory cortex. The inhibitory system, intensified by focused auditory attention, might have sharpened the population-level frequency tuning via the top-down auditory pathway. Therefore, focused auditory attention cannot only amplify excitatory neural activity (gain effect) but also inhibitory neural activity, leading to the sharpening of population-level frequency tuning in the human auditory cortex.
Although top-down auditory focused attention can amplify and sharpen neural activity in the human auditory cortex, the question is still unsettled about whether these attentional effects depend upon the specific location of neurons within the tonotopic maps. Psychoacoustic studies have indicated that frequency-specific auditory attention sharpens the tuning for an attended relative to an unattended frequency. This was reflected in a detection advantage for the former compared to the latter (Hafter et al., 1993; Hubner and Hafter, 1995).
Spectral cues are definitely important for neural processing in noisy environments. Based on the results described previously, we investigated whether population-level frequency tuning can be modulated by differential stimulus sequencing under auditory focused attention in awake humans (Okamoto et al., 2010a). We presented various pure tones of different frequencies (TS) simultaneously with BENs. BENs were notched with widths of one quarter (1/4 CB), one half (1/2 CB), or one CB (1 CB), centered at the frequency of the simultaneously presented TS (Figure 7). We contrasted two different attentional conditions within subjects: “constant sequencing” and “random sequencing.” In the constant sequencing session, identical frequency TS were presented successively, with simultaneous presentation of either the 1/4, 1/2, or the 1 CB BEN. In the random sequencing session, TS with different frequencies were randomly presented. Crucially, the overall bottom-up auditory input was identical between constant sequencing and random sequencing conditions. During all conditions, subjects were instructed to focus their attention on the auditory stimuli.
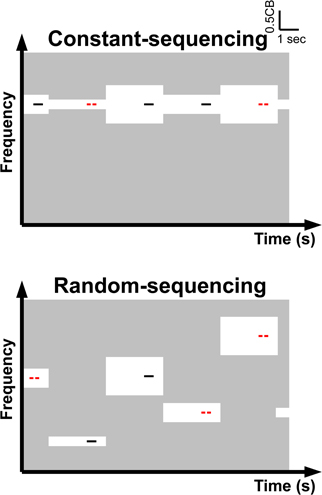
Figure 7. (Modified from Okamoto et al., 2009) Concept and time course of auditory stimulation in the constant sequencing and random sequencing conditions. Pass-bands and stop-bands of the band-eliminated noises (BENs) are represented by the light gray and white areas, respectively. The notch-bandwidth of a BEN (white area) is either 1/4, 1/2, or 1 critical band. Target and non-target test stimuli (TS) are represented as red lines with gap (target TS, requiring a button press) and black lines without gap (non-target TS), respectively. During the constant sequencing condition (upper graph), the TS is a series of identical frequencies, whereas during the random sequencing condition (lower graph) the TS has different frequencies. The TS frequencies differed between constant sequencing blocks. In total, identical bottom-up auditory inputs are provided during the constant sequencing and random sequencing conditions.
The N1m responses were larger when the TS had a constant frequency, compared to random TS frequencies, particularly when BENs with narrow eliminated-bands were simultaneously presented. Moreover, while there was no sequencing effect in the wide BEN condition, the narrow BENs showed an enhanced N1m source strength for the constant sequencing as compared to the random sequencing condition.
These results suggest that constant stimulus sequencing under focused auditory attention may cause sharper and larger neural activity at the attended (constantly presented) frequency, and broader and smaller neural responses at the other frequencies, compared to the random sequencing condition. Our findings further suggest that constant stimulus sequencing during auditory focused attention can improve population-level frequency tuning in humans in a frequency-specific manner by enhancing lateral inhibition around the attended frequency.
Unanswered Questions
The results discussed above support the hypothesis that N1m amplitude evoked by test tones centered in the notch of BEN stimuli is reduced compared to control conditions by the distribution of lateral inhibition into the notch region from the activated side band regions. A question raised by the findings is how they relate with the results of other studies investigating the effects of BEN stimuli on brain activity and with studies investigating the effect of BEN stimuli on auditory perception. Norena and Eggermont (2003) reported that the spontaneous firing rates of neurons coding the eliminated-band frequencies increased during an interval of BEN in the primary auditory cortex of ketamine-anesthetized cats, compared to an unnotched noise condition. Further, detection thresholds for the band-eliminated frequencies are decreased (improved) in human subjects following notched compared to broadband noise (Wiegrebe et al., 1996; Norena et al., 2000). Norena and Eggermont (2003) suggested that a shift of the balance of excitation and inhibition toward reduced inhibition in the notched region could explain an increase in spontaneous firing rates in this region and lead to an increase in driven activity accounting for threshold improvement. However, this interpretation is not favored by our findings, which point to an increase in lateral inhibition distributed into the notched region. Alternatively it could be proposed that enhanced spontaneous activity or Zwicker tones experienced in the notched region reduce N1m responses by a forward masking mechanism. However, this account does not appear to explain the scope of our findings as efficiently as does the simple hypothesis of lateral inhibition distributed to the notched region. It may be relevant that the cortical sources of the N1m localize predominantly to the auditory belt area and may be modulated “top-down” by attention as well as by changes in the response properties of neurons in primary auditory cortex that have been investigated in anesthetized animals.
The Bridge Between Cortical Plasticity, Lateral Inhibition and the Treatment of Tonal Tinnitus
In general, adaptive plasticity in the auditory cortices of musicians as well as non-musicians, based on and induced by musical training, is a very positive phenomenon. Training-induced alterations in the cortical map correspond to perceptual correlates, which indicate superior performance. These changes are excellent examples of the “bright side” of auditory cortical plasticity, which can enable extraordinary proficiencies. However, cortical plasticity also has “dark sides” (Elbert and Heim, 2001). When auditory cortical plasticity goes wrong, serious symptoms and pathologies can result, for example, if a peripheral lesion, which is normally by itself manageable, is influenced by an intense and stressful experience, such as the fear of serious disease, in conjunction with particular limbic system activation. In such a case, maladaptive auditory cortical reorganization, such as that which underlies tinnitus, can be triggered.
When auditory cortical plasticity has negative effects, such as tinnitus, this is, fortunately, not completely irreversible; the process of plasticity itself can be utilized in order to reverse or reduce these maladaptive changes. Such rehabilitative plasticity functions by reestablishing normal cortical functional organization to a certain degree. In order to accomplish this goal in tinnitus patients, we use the phenomenon of lateral inhibition. As demonstrated in Chapter 2, lateral inhibition can suppress interfering neural activity in the human auditory cortex. Subjective tinnitus is among the most prevalent symptoms likely to be caused by aberrant and interfering neuronal activity.
Recent auditory neuroscience research has suggested that an imbalance of excitatory and inhibitory neural interactions within the auditory cortex could lead to the perception of tinnitus (Eggermont and Roberts, 2004), however, neurophysiological tinnitus treatment has so far focused mainly on habituation mechanisms. We suggest that making use of lateral inhibition within the auditory cortex could be a valuable alternative strategy for suppressing tinnitus perception (Pantev et al., 1999, 2004). Our goal was to develop a highly specific auditory treatment strategy using an acoustic carrier (music) that could be listened to for a long time with pleasure and attention, and which would have the potential to specifically enhance lateral inhibitory impact in the human auditory cortex. In the following section, we are introducing and describing our treatment approach in detail.
“Long-Term” and “Short-Term” TMNMT for Tonal Tinnitus
Based on hypotheses derived from previous findings regarding tinnitus [e.g., Eggermont and Roberts (2004); Roberts et al. (2010)], music-induced cortical plasticity [e.g., Pantev et al. (1999)], and lateral inhibition [e.g., Pantev et al. (2004); Okamoto et al. (2005, 2007a,b)], we developed a novel tinnitus treatment strategy which specifically targets tonal tinnitus, the most frequent tinnitus subtype (http://www.tinnitusarchive.org).
This section will briefly introduce our treatment rationale. Firstly, tinnitus is likely to be the result of maladaptive plasticity in the central auditory pathway (Eggermont and Roberts, 2004). It is reasonable to assume that maladaptive changes in the auditory cortex must exist if tinnitus is present, because the tinnitus perception arises here (Eggermont, 2006). More specifically, neuronal populations in the auditory cortex which code external sounds with similar acoustic properties to the tinnitus frequency reported by patients with tonal tinnitus, are very likely to be involved in tinnitus perception (Muhlnickel et al., 1998; Diesch et al., 2004). These neuronal populations are presumably characterized by hyperactivity and hyper synchronicity (Rauschecker et al., 2010) and are, obviously, potential treatment targets but we require a non-invasive method of working specifically with them. An acoustic input with specific properties would allow us to target those particular neural populations in a non-invasive way. Secondly, maladaptive plastic changes are generally reversible (Flor et al., 1995; Candia et al., 1999; Giraux et al., 2001) and behavioral (re-)training may be one way to achieve this. Thirdly, music is a powerful agent that can be used as a neuro-rehabilitative strategy to induce adaptive cortical plasticity and reverse maladaptive plasticity (Wan and Schlaug, 2010). Music itself is not only a dynamic broadband acoustic stimulus but can also capture the attention of the listener and trigger positive emotional responses. Finally, the attenuation effects of lateral inhibition on the amplitude and synchronicity of neuronal activity in the human auditory cortex appear to be stronger than the attenuation effects of habituation (Okamoto et al., 2004; Pantev et al., 2004).
On the basis of these core assumptions and the series of experimental results reviewed in the previous sections, we developed TMNMT. This training strategy focuses on tonal tinnitus, which patients usually describe as sounding “beep”-like or “whistle”-like. In our experience the individual tinnitus frequency, in most cases of tonal tinnitus, can be matched psycho-acoustically. We assume that neuronal populations in the auditory cortex that code the tinnitus frequency are involved in tinnitus perception (Muhlnickel et al., 1998; Diesch et al., 2004; Roberts et al., 2010), and it is thus the goal of TMNMT to specifically target these neurons in the individual tinnitus patient.
From our perspective, based on the previously outlined knowledge about functional cortical reorganization and plasticity, we feel it is reasonable to assume that the maladaptively reorganized auditory cortex of a tinnitus patient can be retrained to certain degree by frequent stimulation using specifically and individually tailored, attractive and behaviorally relevant auditory input (her/his favorite music).
We believed that regular training over the course of several months was necessary because many examples from the literature on human cortical plasticity [e.g., Candia et al. (1999)] indicate that training is most effective and its effects are most persistent if the training is performed intensively and with perseverance.
Regarding the auditory input for the treatment, we are convinced that music is an excellent choice for several reasons. Firstly, music is enjoyable: almost everyone can name songs or musical works that he or she likes to listen to. Positive emotions are experienced during musical listening (Salimpoor et al., 2011) and this implies that attention is attracted to preferred music. Various evidence shows that focused attention promotes cortical plasticity [e.g., Polley et al. (2006)] and this suggests to us that if the listener really enjoys the training by, for example, being able to choose to listen to their favorite music, they will be most open to attention-induced plasticity.
Secondly, most types of contemporary music, e.g., pop or rock music, cover a broad frequency spectrum, with considerable amounts of energy in the higher-frequency range. With such music it is therefore possible to target typical tinnitus frequencies, which are usually high (http://www.tinnitusarchive.org). The aim of modifying the music is to reduce the activity/synchronicity of neuronal populations involved in tinnitus perception. One feasible way to achieve this would be to stimulate these neurons less than all other auditory neurons. In practice we believe this can be achieved by removing part of the energy spectrum from the training music, specifically the spectrum of energy corresponding to the tinnitus frequency range. The result is literally “notched” music, exposure to which would deprive the specific neuronal population that codes the tinnitus frequency, relative to the populations responsible for coding other frequencies. We thus hypothesize that TMNMT would induce a circumscribed auditory functional deafferentation (Pantev et al., 1999) and transient sensory input deprivation. This deprivation would lead to a reduction in the level of excitation of auditory cortical neurons coding the notched frequencies, among them the tinnitus frequency. This reduction in excitation level may be caused by the (transient) strengthening of locally weakened inhibitory impact in the auditory cortex. The consequence for sufferers of chronic tinnitus should be reduction in tinnitus loudness.
TMNMT Evaluation
To date, we have completed two major studies by means of which we evaluated the efficacy of TMNMT. The first study (Okamoto et al., 2010b; Stracke et al., 2010) was a proof-of-concept trial. The second study (Teismann et al., 2011, for details see below) was conducted in order to extend the results of the initial study, to advance the training strategy and to gain further insight regarding potential patient characteristics that may modulate the training outcome. From our perspective, the strength of both studies was that we not only evaluated tinnitus perception behaviorally but we also analyzed and evaluated tinnitus-related auditory cortical neuronal activity electrophysiologically, by means of MEG.
Long-Term TMNMT
For the proof-of-concept trial (Okamoto et al., 2010b; Stracke et al., 2010), we recruited a relatively small, but homogeneous with respect to their tinnitus, group of 39 patients. The participants, aged between 18 and 60 years, suffered from chronic (>12 months duration), unilateral, tonal tinnitus, with tinnitus frequencies ≤ 8000 Hz. The patients exhibited neither severe hearing loss nor neurological or psychiatric diseases. Before beginning training, the patients were assigned to one of three groups: target, placebo or monitoring. The assignment to target or placebo was executed pseudo-randomly and was double-blinded. Patients selected the music they most enjoyed. The target group received the real TMNMT: from their music, the frequency band of one octave width surrounding the individual tinnitus frequency was removed. The placebo group received placebo notched music therapy: the frequency band of one octave width around the tinnitus frequency remained unfiltered and, instead, the frequency range above and below this band was notched with a slowly moving filter of one octave width. The moving notch did not change the music more than the constant notch thus it was not possible to differentiate between the target and placebo music. The patients of the monitoring group were just listening to their usual music.
The TMNMT was performed over the course of 12 consecutive months. During this time period, the patients listened to their notched music for 1–2 h every day, using closed headphones supplied by us and characterized by an appropriate frequency response. The patients were instructed to listen to their notched music in a quiet environment, selecting their own moderate loudness. It was not mandatory to focus on the training music, however, if one is listening to his/her favorite music, it is very likely that attention will be directed to it. Once per week, following a strict routine, the patients assessed their perceived tinnitus loudness (as well as tinnitus-related annoyance, awareness, and handicapping) by means of a visual analogue scale. Every 6 months, tinnitus-related auditory cortical evoked activity was measured by means of MEG.
The results of this proof-of-concept study demonstrated the specific efficacy of TMNMT. After completing their training, the perceived tinnitus loudness (Figure 8), as well as tinnitus-related primary and non-primary auditory cortical evoked activity (Figure 9), was significantly reduced for patients in the target group, compared to their pre-training baseline. In contrast, in both the placebo and monitoring groups, no significant changes from the baseline were found. One interesting finding was the significant positive correlation between tinnitus loudness change and change in primary auditory cortical evoked activity. Those patients who showed more pronounced tinnitus loudness reductions demonstrated the stronger ASSR source strength ratio reductions. Approximately 80% of patients in the target group experienced loudness alleviation to some degree, and the average reduction in tinnitus loudness in this group was approximately 25%.
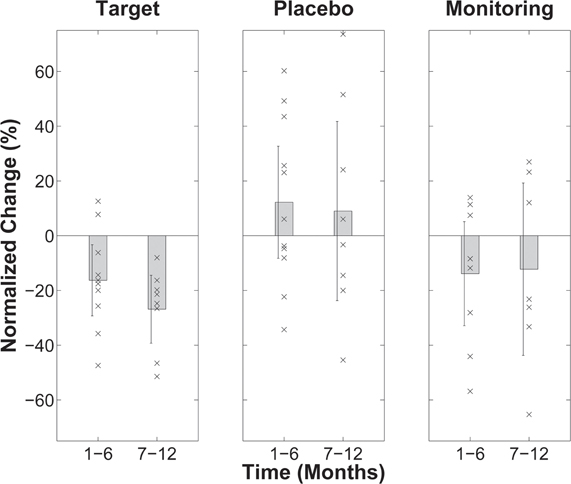
Figure 8. (Modified from Okamoto et al., 2010a,b) Normalized tinnitus loudness change after 6 and 12 months of treatment (or monitoring) relative to baseline (=0) for the three patient groups (target TMNMT, placebo TMNMT, and monitoring). Positive change values reflect impairment; negative change values reflect improvement. The bars indicate group averages and each “×” indicates an individual data point. The error bars denote confidence intervals. As indicated by the confidence interval bars, only the changes in the target group were statistically significant.
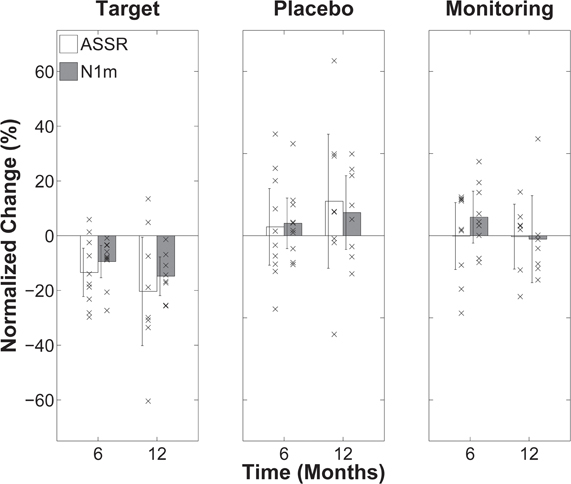
Figure 9. (Modified from Okamoto et al., 2010a,b) Change in normalized tinnitus-related auditory cortical evoked activity after 6 and 12 months of treatment (or monitoring) relative to baseline (=0) for the three patient groups (target TMNMT, placebo TMNMT, and monitoring). Positive change values reflect increase in activity; negative change values reflect decrease in activity. The bars indicate group averages and each “×” indicates an individual data point. The error bars denote confidence intervals. Auditory steady state response (ASSR) change values are reflected by white bars and N1m change values are reflected by gray bars. As indicated by the confidence interval bars, only the changes in the target group were statistically significant.
Short-Term Intensive TMNMT
Motivated by the findings of the proof-of-concept trial, we ran a second study (Teismann et al., 2011) in order to further develop and evaluate TMNMT. In the previous study, based on theoretical and practical considerations, we had included only patients with tinnitus frequencies ≤ 8000 Hz. This time, we also included patients with tinnitus frequencies > 8000 Hz. In order to better target these patients with notched music, we “flattened” the energy spectrum of the training music, i.e., we digitally redistributed energy from lower to higher-frequency ranges and thus enriched the energy spectrum in the higher-frequency range (Figure 10).
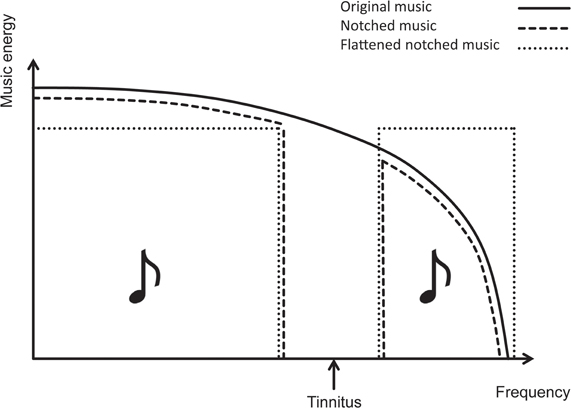
Figure 10. Schematic illustration of the energy spectra of original (i.e., unmodified) music (black line), notched music (green line), and flattened notched music (blue line). The red arrow indicates the tinnitus frequency, at which the notches are centered.
To investigate whether tinnitus frequency modulates TMNMT efficacy, we divided the patients into two groups on the basis of their tinnitus frequency, with 8000 Hz as the grouping criterion. We also shortened and intensified the TMNMT considerably. In the previous study, the patients had listened to approximately 720 h of notched music over the course of 12 months. In contrast, in the second study, the patients listened to 24 h of notched music over the course of 5 consecutive days. In order to study the temporal dynamics of the training effects, we assessed tinnitus perception in the second study quite intensively. Finally, to investigate the persistency of the training effects, we applied a post training observation phase of 31 days.
In the second study we did not apply a placebo TMNMT. All patients received the target TMNMT, including the selection of their most enjoyable music and the removal of the frequency band of one octave width centered at the individual tinnitus frequency. However, to minimize potential placebo effects, patients were told, prior to the onset of the study, that they would receive either the target or a placebo TMNMT. After the completion of the study, the patients were debriefed.
The results of this study demonstrated that the short and intense TMNMT had been effective, though only for the patient group characterized by tinnitus frequencies ≤ 8000 Hz. TMNMT efficacy was reflected by reduction in perceived tinnitus loudness and tinnitus-related distress, as well as by a reduction in tinnitus-related non-primary auditory cortical evoked neuronal activity. Furthermore, the results indicated that the reductions in tinnitus loudness and evoked cortical activity achieved in this very short TMNMT scenario persisted for no longer than 2 weeks. The tinnitus-related distress alleviation, however, appeared to be longer lasting.
On the basis of these two studies, it can currently be concluded that TMNMT is effective in reducing reported tinnitus loudness by an average of about 20% (with greater reductions in some cases), specifically for patients who suffer from chronic tonal tinnitus and who do not exhibit a greater than moderate hearing loss. Importantly, TMNMT efficacy could be demonstrated on different time scales and with varied training intensities. There are hints from pertinent literature on training-induced cortical reorganization, however, that longer-term training would probably induce more persistent and possibly permanent effects. In addition, there are hints that certain patient prerequisites, such as a very high tinnitus frequency, could counteract TMNMT efficacy. Crucially, TMNMT can specifically influence tinnitus-related auditory cortical activity.
Known TMNMT Related Issues
There are several issues associated with the application of TMNMT. In the following section we will discuss some of the most relevant challenges.
Tinnitus frequency determination
Obviously, the success of TMNMT will stand and fall by the reliable determination of the tinnitus frequency. Unfortunately, identifying the tinnitus frequency is not a trivial task (Tyler and Conrad-Armes, 1983; Moore et al., 2010). Firstly, the tinnitus frequency cannot be measured objectively, but has to be matched subjectively by the patient who has to compare test tones of different frequencies to his tinnitus percept. Secondly, depending upon certain prerequisites, like the actual tonal quality of the tinnitus percept (for example, rather clear tone vs. “dirty” tone, ringing, or chirping) or hearing loss [which is often associated with frequency selectivity impairment (Okamoto et al., 2011)], it is more or less difficult for patients to compare the frequency of their tinnitus to the frequency of a test tone. Thirdly, in our experience, patients vary in their ability to compare the frequency of their tinnitus to the frequency of a test tone, probably depending upon factors such as musical education. Fourthly, there are certain typical pitfalls, such as octave confusion, that need to be considered (Moore et al., 2010). Finally, it is also our experience that tinnitus frequency matches become more reliable (i.e., vary less) with training.
In our studies, in order to match the patient's tinnitus frequency we use a high-frequency audiometer (up to 16 kHz), testing with a frequency resolution of 1/24th octave. In cases of bilateral tinnitus, we perform tinnitus matching according to the ear in which the tinnitus is perceived as being loudest. In the case of unilateral tinnitus, we use the ear in which tinnitus is perceived. If tinnitus is perceived as being “in the head,” or if it is equally loud in both ears, we use the better hearing ear. During the matching procedure we start at different frequencies in order to first collect a number of potential tinnitus frequency “candidates.” In a second step, we test these candidates against each other in a two-forced-choice procedure. After identifying the “winner” candidate, we test this winner against all its octaves between 1000 and 16000 Hz, again applying a two-forced choice procedure. We usually repeat this procedure on different days before the tinnitus frequency is finally determined.
Music energy spectrum
The energy spectrum of the acoustic stimulus is one critical factor that would influence TMNMT efficacy. Unfortunately, unlike WN, for example, music does not contain an equal amount of energy evenly distributed over the whole frequency range. Rather, the amount of energy generally drops considerably with increasing frequency. Thus, in terms of energy distribution, music is a more appropriate training stimulus for patients with a rather low tinnitus frequency than those with rather high tinnitus frequencies. Fortunately, from our experience, music is surprisingly “robust” against alterations of its energy spectrum so that, even though modifications like the removal of a whole octave or the “flattening” of the energy spectrum may appear to be aggressive manipulations of the music, the results most often still sound like enjoyable music to which we are able to pay attention for a considerable time.
Worthy of note is our observation that the energy distribution across frequency differs markedly between different types of music. While rock or pop music usually already contains a relatively flat spectrum, including a comparatively significant amount of high-frequency energy, this does not hold true for other music types, such as vocal music or single instruments. Even the spectrum of classical orchestral music is, from our experience, less suitable compared to common, contemporary popular music because of having less evenly distributed energy.
Equipment
The equipment used for TMNMT also appears to be a critical factor in the efficacy of the treatment. Ideally, the carefully, individually tailored acoustic input should be transmitted through the ear-canal, middle-ear, and cochlea into the central auditory pathway with as little distortion as possible. The frequency responses and transferring characteristics of the devices used to play and transduce the notched music are, therefore, critical. This includes the music player, the amplifier, the headphones, and specific device configurations such as the equalizer settings. The goal would be to achieve true-to-the original, authentic tailor-made notched music replay. We therefore use headphones with maximally linear and flat frequency response. In addition, it is important to ensure that ambient noises, which are usually of broadband type and, of course, not specifically notched, would not interact with the music modification in an unwanted manner. Thus, in addition to instructing the patients to perform the TMNMT in a preferably quiet environment, we also have recommendations regarding the necessary equipment.
Outstanding Questions
Several important TMNMT-related questions remain to be answered. In the following sections we will focus on three of these questions.
Non-Tonal Tinnitus
It remains to be determined whether TMNMT could be effective in the case of non-tonal tinnitus types. For instance, a substantial number of patients report that their tinnitus sounds in some way “noise-like” (http://www.tinnitusarchive.org). From attempts at tinnitus matching it is known that, in such cases, the tinnitus percept can be compared to narrowband or broadband noise stimuli. It is usually far more difficult to match noise-like compared to tonal tinnitus percepts, and this implies that matches that are obtained in the former case would be less reliable. Moreover, a practical problem associated with the notched music treatment of noise-like tinnitus would be that rather wide frequency bands (e.g., two or three octaves) would have to be removed from the training music, which may result in qualitatively changed, distorted and less enjoyable music.
Severe Hearing Loss
Obviously, TMNMT can only be effective if the acting frequencies of the stimulus are actually perceived by the patient. Unfortunately, almost all tinnitus patients have hearing loss to a certain degree (Eggermont and Roberts, 2004). In our TMNMT evaluation studies, we have so far focused on patients with no greater than moderate hearing loss (i.e., ≤ 35 dB HL in study one and ≤ 50 dB HL in study two). Many patients with tinnitus, however, have severe hearing losses, especially older patients, and this usually affects the frequencies that need to be perceived in order for TMNMT to be effective. It therefore appears unlikely that music modification alone could be enough to effectively treat such patients. From our perspective, it would be important to think about strategies for making TMNMT available for tinnitus patients with more severe hearing losses.
How Does TMNMT Work?
We have argued that the beneficial effects of TMNMT are due to the suppression of tinnitus-related neural activity by lateral inhibition distributed into the notched region. Unlike our initial 3 day study of notched sounds described earlier (Pantev et al., 1999) in which brain responses reverted to their pre-listening levels over 24 h, TMNMT was experienced daily for 12 months (Okamoto et al., 2010a,b), and the effect on brain responses persisted after period of 6 and 12 months (cf. Figure 9) implying persisting changes in auditory cortex. Noteworthy, there are other treatment studies, which have reported improvements in tinnitus (Davis et al., 2007; Tass et al., 2012) and reductions in loudness growth in individuals with hyperacusis (Norena and Chery-Croze, 2007). Davis et al. (2007) report reduction of tinnitus loudness after exposure to complex sounds covering the hearing loss regions where tinnitus frequencies localize, whereas Tass et al. (2012) demonstrate tinnitus loudness reduction to sequential presentation of four pure tones (coordinated reset) with a distance of one or two octaves to the tinnitus frequency (for reviews see Hoare et al., 2011; Roberts and Bosnyak, 2011). Because the sounds used in these studies covered a considerable frequency range, our results with TMNMT raise the possibility that notching out the specific tinnitus frequencies in cases of tonal tinnitus may confer added benefit. Additional research will be needed to assess this question. It should also be noted that listening to background sound for extended periods (which our subjects did) could rescale auditory sensitivity (Formby et al., 2003; Norena and Chery-Croze, 2007). Thus, listening to notched music could reduce the perceived loudness of sound in the notched frequency region, which could have been reflected in our brain measurements. Several models of tinnitus suggest that enhanced sensitivity to sound and tinnitus go together, and that procedures that benefit one will also benefit the other. Therefore, further evaluations of the different approaches are necessary in order to be able to make a final decision, which are most important factors contributing to the outcome of customized sound treatment of tonal tinnitus.
A further question concerns the effect of long-term exposure to background sound on auditory neural representations. Until recently it was believed that passive auditory experience did not change auditory representations in the mature brain unless the sounds possessed behavioral relevance (Polley et al., 2006). However, recent animal studies (Pienkowski and Eggermont, 2011, 2012; Zhou and Merzenich, 2012) have demonstrated that long-term stimulation with band-pass noise or multi-frequency stimuli resulted in reduced spontaneous firing rates and reduced responsiveness of primary auditory cortex neurons corresponding to the stimulated frequencies. At the low- or high-frequency edge region (about one octave wide) enhanced neural activity was found. At first glance, these results appear to be contradictory to our own results where reductions in neural activity were observed in the band-eliminated regions of the listened to sound. However, there are many differences between the two experimental approaches. In the aforementioned animal studies neither hearing loss nor tinnitus was present, whereas in our experiments tinnitus was present and hearing loss as well. The effect of exposure to environmental sounds could be different depending on the level of background neural activity, which is likely elevated in tinnitus. In our study we used favorite music, a behaviorally and emotionally relevant sound stimulus for the subjects that may have elevated neural activity in the band-pass regions but suppressed it in the band-eliminated regions. In contrast, the above-mentioned animal studies used irrelevant noises as sound stimuli. In order to perceive behaviorally relevant environmental sound signals through the noise, the animals may have needed to increase the sensitivity of neurons, whose characteristic frequencies were not covered by the spectrum of the TS. Clearly, understanding the neural effects of long-term sound exposure is important not only for the prevention of hearing disorders but also for treating tinnitus (Okamoto et al., 2010b, 2011; Pienkowski and Eggermont, 2012).
Looking Ahead
We strive for two important goals. The first is the further optimization of the TMNMT strategy. This includes, among other things, examining the effectiveness of the tinnitus treatment as a function of the width of the spectral notch in TMNMT and experimentally testing the combination of TMNMT with complementary strategies, including tinnitus counseling. We assume that the enrichment of TMNMT with further effective strategies should induce a significant increase in the effects of rehabilitative reorganizational cortical plasticity in the tinnitus networks, and might potentially prolong the alleviation of the tinnitus percept.
The second goal is to transfer the optimized TMNMT strategy into clinical practice. This step involves evaluating the treatment efficacy in a large sample of tinnitus patients. The applicability of TMNMT depends substantially on its practicability, an important aspect of which is the quality and usability of the tools, which provide the treatment to patients. The goal is to develop professional and high-quality “state of the art” hard- and software-solutions, which are easy for the tinnitus patients to use and would win their acceptance. We plan to carefully evaluate the efficacy of the optimized TMNMT in a large sample of patients with tonal tinnitus [about 65% of tinnitus patients suffer from tonal tinnitus (Stouffer and Tyler, 1990)]. The clinical trial will involve regular audiological, psychophysical, neuroimaging, and psychometric outcome evaluations, and the optimized treatment is expected to induce significant reductions of tinnitus loudness, distress, and handicap.
In summary, the TMNMT approach can be considered as an enjoyable, low-cost, and presumably causal treatment that is capable of specifically alleviating tinnitus perception. In these times of restricted resources within the health system, it is of paramount importance to develop cost-efficient rehabilitation programs. Another important potential advantage of TMNMT is that, by being taught to perform the training on their own, patients are educated to carry the responsibility for their own future well-being. This is in line with current trends in healthcare systems, to treat the patient as a responsible partner able to undertake shared decision-making. The expected results will be of great interest for the community of oto-rhino-laryngologists, audiologists, neurologists, and psychotherapists far beyond the current research environment.
Conflict of Interest Statement
The authors declare that the research was conducted in the absence of any commercial or financial relationships that could be construed as a potential conflict of interest.
Acknowledgments
We thank Bernhard Ross and Andreas Wollbrink for technical support, Karin Berning for organizing the data acquisition, Ross Parfitt for valuable discussions and proof-reading, and our test subjects for their diligent collaboration. This work has been supported by IZKF CRA05, Deutsche Forschungsgemeinschaft (Pa 392/10-2, Pa 392/10-3, Pa 392/14-1, Pa 392/13-1), TRI Pantev, CIHR 2001–2003 “MEG studies of the auditory cortex,” Pantev, Canada Research Chair “Human Cortical Plasticity,” 2001–2003; CIHR “Understanding, treating and preventing tinnitus”; Japan Society for the Promotion of Science for Young Scientists (23689070), and “Strategic Research Program for Brain Sciences” (Development of biomarker candidates for social behavior).
References
Bangert, M., and Schlaug, G. (2006). Specialization of the specialized in features of external human brain morphology. Eur. J. Neurosci. 24, 1832–1834.
Bauer, C. A., Turner, J. G., Caspary, D. M., Myers, K. S., and Brozoski, T. J. (2008). Tinnitus and inferior colliculus activity in chinchillas related to three distinct patterns of cochlear trauma. J. Neurosci. Res. 86, 2564–2578.
Bermudez, P., Lerch, J. P., Evans, A. C., and Zatorre, R. J. (2009). Neuroanatomical correlates of musicianship as revealed by cortical thickness and voxel-based morphometry. Cereb. Cortex 19, 1583–1596.
Besson, M., Schon, D., Moreno, S., Santos, A., and Magne, C. (2007). Influence of musical expertise and musical training on pitch processing in music and language. Restor. Neurol. Neurosci. 25, 399–410.
Burrows, D. L., and Barry, S. J. (1990). Electrophysiological evidence for the critical band in humans: middle-latency responses. J. Acoust. Soc. Am. 88, 180–184.
Butler, R. A. (1968). Effect of changes in stimulus frequency and intensity on habituation of the human vertex potential. J. Acoust. Soc. Am. 44, 945–950.
Candia, V., Elbert, T., Altenmuller, E., Rau, H., Schafer, T., and Taub, E. (1999). Constraint-induced movement therapy for focal hand dystonia in musicians. Lancet 353, 42–42.
Cazals, K., Horner, C., and Huang, Z. W. (1998). Alterations in average spectrum of cochleoneural activity by long-term salicylate treatment in the guinea pig, a plausible index of tinnitus. J. Neurophysiol. 80, 2113–2120.
Davis, P. B., Paki, B., and Hanley, P. J. (2007). Neuromonics tinnitus treatment: third clinical trial. Ear Hear. 28, 242–259.
Diesch, E., Struve, M., Rupp, A., Ritter, S., Hulse, M., and Flor, H. (2004). Enhancement of steady-state auditory evoked magnetic fields in tinnitus. Eur. J. Neurosci. 19, 1093–1104.
Eggermont, J. J. (2006). Cortical tonotopic map reorganization and its implications for treatment of tinnitus. Acta Otolaryngol. Suppl. 556, 9–12.
Eggermont, J. J., and Roberts, L. E. (2004). The neuroscience of tinnitus. Trends Neurosci. 27, 676–682.
Ehret, G., and Merzenich, M. M. (1988). Complex sound analysis (frequency resolution, filtering and spectral integration) by single units of the inferior colliculus of the cat. Brain Res. 472, 139–163.
Elbert, T., Pantev, C., Wienbruch, C., Rockstroh, B., and Taub, E. (1995). Increased cortical representation of the fingers of the left hand in string players. Science 270, 305–307.
Flor, H., Elbert, T., Knecht, S., Wienbruch, C., Pantev, C., Birbaumer, N., Larbig, W., and Taub, E. (1995). Phantom-limb pain as a perceptual correlate of cortical reorganization following arm amputation. Nature 375, 482–484.
Formby, C., Sherlock, L. P., and Gold, S. L. (2003). Adaptive plasticity of loudness induced by chronic attenuation and enhancement of the acoustic background. J. Acoust. Soc. Am. 114, 55–58.
Fujioka, T., Trainor, L. J., Ross, B., Kakigi, R., and Pantev, C. (2004). Musical training enhances automatic encoding of melodic contour and interval structure. J. Cogn. Neurosci. 16, 1010–1021.
Fujioka, T., Trainor, L. J., Ross, B., Kakigi, R., and Pantev, C. (2005). Automatic encoding of polyphonic melodies in musicians and nonmusicians. J. Cogn. Neurosci. 17, 1578–1592.
Gaser, C., and Schlaug, G. (2003). Brain structures differ between musicians and non-musicians. J. Neurosci. 23, 9240–9245.
Gilbert, C. D., and Wiesel, T. N. (1992). Receptive field dynamics in adult primary visual cortex. Nature 356, 150–152.
Giraux, P., Sirigu, A., Schneider, F., and Dubernard, J. M. (2001). Cortical reorganization in motor cortex after graft of both hands. Nat. Neurosci. 4, 691–692.
Hafter, E. R., Schlauch, R. S., and Tang, J. (1993). Attending to auditory filters that were not stimulated directly. J. Acoust. Soc. Am. 94, 743–747.
Hari, R. (1990). Magnetic evoked fields of the human brain: basic principles and applications. Electroencephalogr. Clin. Neuro-physiol. Suppl. 41, 3–12.
Heller, A. (2003). Classification and epidemiology of tinnitus. Otolaryngol. Clin. North Am. 36, 239.
Hillyard, S. A., Hink, R. F., Schwent, V. L., and Picton, T. W. (1973). Electrical signs of selective attention in the human brain. Science 182, 177–180.
Hoare, D. J., Kowalkowski, V. L., Kang, S., and Hall, D. A. (2011). Systematic review and meta-analyses of randomized controlled trials examining tinnitus management. Laryngoscope 121, 1555–1564.
Hubner, R., and Hafter, E. R. (1995). Cuing mechanisms in auditory signal detection. Percept. psychophys. 57, 197–202.
Hutchinson, S., Lee, L. H., Gaab, N., and Schlaug, G. (2003). Cerebellar volume of musicians. Cereb. Cortex 13, 943–949.
Irvine, D. R. F., and Rajan, R. (1995). “Plasticity in the mature auditory system,” in Advances in Hearing Research, eds G. A. Manley, G. M. Klump, C. Köppl, H. Fastl, and H. Oeckinhaus (Singapore: World Scientific Publishing Co.), 3–23.
Jancke, L., Gaab, N., Wustenberg, T., Scheich, H., and Heinze, H. J. (2001). Short-term functional plasticity in the human auditory cortex: an fMRI study. Cogn. Brain Res. 12, 479–485.
Jastreboff, P. J., and Hazell, J. W. (1993). A neurophysiological approach to tinnitus: clinical implications. Br. J. Audiol. 27, 7–17.
Jenkins, W. M., Merzenich, M. M., Ochs, M. T., Allard, T., and Guic-Robles, E. (1990). Functional reorganization of primary somatosensory cortex in adult owl monkeys after behaviorally controlled tactile stimulation. J. Neurophysiol. 63, 82–104.
Jääskeläinen, I. P., Ahveninen, J., Bonmassar, G., Dale, A. M., Ilmoniemi, R. J., Levanen, S., Lin, F. H., May, P., Melcher, J., Stufflebeam, S., Tiitinen, H., and Belliveau, J. W. (2004). Human posterior auditory cortex gates novel sounds to consciousness. Proc. Natl. Acad. Sci. U.S.A. 101, 6809–6814.
Kaas, J. H., Krubitzer, L. A., Chino, Y. M., Langston, A. L., Polley, E. H., and Blair, N. (1990). Reorganization of retinotopic cortical maps in adult mammals after lesions of the retina. Science 248, 229–231.
Kadner, A., Viirre, E., Wester, D. C., Walsh, S. F., Hestenes, J., Vankov, A., and Pineda, J. A. (2002). Lateral inhibition in the auditory cortex: an EEG index of tinnitus? Neuroreport 13, 443–446.
Katsuki, Y., Sumi, T., Uchiyama, H., and Watanabe, T. (1958). Electric responses of auditory neurons in cat to sound stimulation. J. Neurophysiol. 21, 569–588.
Kiang, N. Y., and Moxon, E. C. (1974). Tails of tuning curves of auditory-nerve fibers. J. Acoust. Soc. Am. 55, 620–630.
Koelsch, S., Schmidt, B. H., and Kansok, J. (2002). Effects of musical expertise on the early right anterior negativity: an event-related brain potential study. Psychophysiology 39, 657–663.
Langguth, B., Kleinjung, T., Landgrebe, M., De Ridder, D., and Hajak, G. (2010). rTMS for the treatment of tinnitus: the role of neuronavigation for coil positioning. Neurophysiol. Clin. 40, 45–58.
Lappe, C., Herholz, S. C., Trainor, L. J., and Pantev, C. (2008). Cortical plasticity induced by short-term unimodal and multimodal musical training. J. Neurosci. 28, 9632–9639.
Lotze, M., Scheler, G., Tan, H. R., Braun, C., and Birbaumer, N. (2003). The musician's brain: functional imaging of amateurs and professionals during performance and imagery. Neuroimage 20, 1817–1829.
Mach, E. (1865). Über die Wirkung der räumlichen Vertheilung des Lichtreizes auf die Netzhaut. Akademie der Wissenschaften in Wien, Sitzungberichte, math.-nat. Cl. 52, 303–322.
Martin, W. H., Schwegler, J. W., Scheibelhoffer, J., and Ronis, M. L. (1993). Salicylate-induced changes in cat auditory nerve activity. Laryngoscope 103, 600–604.
Menning, H., Roberts, L. E., and Pantev, C. (2000). Plastic changes in the auditory cortex induced by intensive frequency discrimination training. Neuroreport 11, 817–822.
Meredith, M. A., and Stein, B. E. (1983). Interactions among converging sensory inputs in the superior colliculus. Science 221, 389–391.
Merzenich, M. M., Kaas, J. H., Wall, J., Nelson, R. J., Sur, M., and Felleman, D. (1983a). Topographic reorganization of somatosensory cortical areas 3b and 1 in adult monkeys following restricted deafferentation. Neuroscience 8, 33–55.
Merzenich, M. M., Kaas, J. H., Wall, J. T., Sur, M., Nelson, R. J., and Felleman, D. J. (1983b). Progression of change following median nerve section in the cortical representation of the hand in areas 3b and 1 in adult owl and squirrel monkeys. Neuroscience 10, 639–665.
Moore, B. C., Vinay, and Sandhya. (2010). The relationship between tinnitus pitch and the edge frequency of the audiogram in individuals with hearing impairment and tonal tinnitus. Hear. Res. 261, 51–56.
Muhlnickel, W., Elbert, T., Taub, E., and Flor, H. (1998). Reorganization of auditory cortex in tinnitus. Proc. Natl. Acad. Sci. U.S.A. 95, 10340–10343.
Norena, A. J., and Chery-Croze, S. (2007). Enriched acoustic environment rescales auditory sensitivity. Neuroreport 18, 1251–1255.
Norena, A., Micheyl, C., and Chery-Croze, S. (2000). An auditory negative after-image as a human model of tinnitus. Hear. Res. 149, 24–32.
Norena, A. J., and Eggermont, J. J. (2003). Neural correlates of an auditory afterimage in prmary auditory cortex. J. Assoc. Res. Otolaryngol. 4, 312–328.
Norena, A. J., and Eggermont, J. J. (2005). Enriched acoustic environment after noise trauma reduces hearing loss and prevents cortical map reorganization. J. Neurosci. 25, 699–705.
Näätänen, R., and Picton, T. (1987). The N1 wave of the human electric and magnetic response to sound: a review and an analysis of the component structure. Psychophysiology 24, 375–425.
Näätänen, R., Sams, M., Alho, K., Paavilainen, P., Reinikainen, K., and Sokolov, E. N. (1988). Frequency and location specificity of the human vertex n1-wave. Electroencephalogr. Clin. Neurophysiol. 69, 523–531.
O'Connor, K. N., Petkov, C. I., and Sutter, M. L. (2005). Adaptive stimulus optimization for auditory cortical neurons. J. Neurophysiol. 94, 4051–4067.
Ochi, K., and Eggermont, J. J. (1997). Effects of quinine on neural activity in cat primary auditory cortex. Hear. Res. 105, 105–118.
Oechslin, M. S., Imfeld, A., Loenneker, T., Meyer, M., and Jancke, L. (2010). The plasticity of the superior longitudinal fasciculus as a function of musical expertise: a diffusion tensor imaging study. Front. Hum. Neurosci. 3:76. doi: 10.3389/neuro.09.076.2009
Okamoto, H., Kakigi, R., Gunji, A., Kubo, T., and Pantev, C. (2005). The dependence of the auditory evoked N1m decrement on the bandwidth of preceding notch-filtered noise. Eur. J. Neurosci. 21, 1957–1961.
Okamoto, H., Kakigi, R., Gunji, A., and Pantev, C. (2007a). Asymmetric lateral inhibitory neural activity in the auditory system: a magnetoencephalographic study. BMC Neurosci. 8, 33.
Okamoto, H., Stracke, H., Wolters, C. H., Schmael, F., and Pantev, C. (2007b). Attention improves population-level frequency tuning in human auditory cortex. J. Neurosci. 27, 10383–10390.
Okamoto, H., Ross, B., Kakigi, R., Kubo, T., and Pantev, C. (2004). N1m recovery from decline after exposure to noise with strong spectral contrasts. Hear. Res. 196, 77–86.
Okamoto, H., Stracke, H., Lagemann, L., and Pantev, C. (2010a). Bottom-up driven involuntary auditory evoked field change: constant sound sequencing amplifies but does not sharpen neural activity. J. Neurophysiol. 103, 244–249.
Okamoto, H., Stracke, H., Stoll, W., and Pantev, C. (2010b). Listening to tailor-made notched music reduces tinnitus loudness and tinnitus-related auditory cortex activity. Proc. Natl. Acad. Sci. U.S.A. 107, 1207–1210.
Okamoto, H., Stracke, H., Zwitserlood, P., Roberts, L. E., and Pantev, C. (2009). Frequency-specific modulation of population-level frequency tuning in human auditory cortex. BMC Neurosci. 10, 1.
Okamoto, H., Teismann, H., Kakigi, R., and Pantev, C. (2011). Broadened population-level frequency tuning in human auditory cortex of portable music player users. PLoS ONE 6:e17022. doi: 10.1371/journal.pone.0017022
Pantev, C., Bertrand, O., Eulitz, C., Verkindt, C., Hampson, S., Schuierer, G., and Elbert, T. (1995). Specific tonotopic organizations of different areas of the human auditory cortex revealed by simultaneous magnetic and electric recordings. Electroencephalogr. Clin. Neurophysiol. 94, 26–40.
Pantev, C., Kevanishvili, Z. S., Galle, E., and Khachidze, O. (1975). ERA-stimulation with free-programable frequency and intensity sequence – a method to reduce the examination time. Arch. Otorhinolaryngol. 211, 43–49.
Pantev, C., Okamoto, H., Ross, B., Stoll, W., Ciurlia-Guy, E., Kakigi, R., and Kubo, T. (2004). Lateral inhibition and habituation of the human auditory cortex. Eur. J. Neurosci. 19, 2337–2344.
Pantev, C., Oostenveld, R., Engelien, A., Ross, B., Roberts, L. E., and Hoke, M. (1998). Increased auditory cortical representation in musicians. Nature 392, 811–814.
Pantev, C., Wollbrink, A., Roberts, L. E., Engelien, A., and Lutkenhoner, B. (1999). Short-term plasticity of the human auditory cortex. Brain Res. 842, 192–199.
Pantev, C., and Herholz, S. C. (2011). Plasticity of the human auditory cortex related to musical training. Neurosci. Biobehav. Rev. 35, 2140–2154.
Picton, T. W., Hillyard, S. A., Krausz, H. I., and Galambos, R. (1974). Human auditory evoked potentials. I. Evaluation of components. Electroencephalogr. Clin. Neurophysiol. 36, 179–190.
Pienkowski, M., and Eggermont, J. J. (2011). Cortical tonotopic map plasticity and behavior. Neurosci. Biobehav. Rev. 35, 2117–2128.
Pienkowski, M., and Eggermont, J. J. (2012). Reversible long-term changes in auditory processing in mature auditory cortex in the absence of hearing loss induced by passive, moderate-level sound exposure. Ear Hear. 33, 305–314.
Polley, D. B., Steinberg, E. E., and Merzenich, M. M. (2006). Perceptual learning directs auditory cortical map reorganization through top-down influences. J. Neurosci. 26, 4970–4982.
Rauschecker, J. P., Leaver, A. M., and Muhlau, M. (2010). Tuning out the noise: limbic-auditory interactions in tinnitus. Neuron 66, 819–826.
Rauschecker, J. P., Tian, B., Pons, T., and Mishkin, M. (1997). Serial and parallel processing in rhesus monkey auditory cortex. J. Comp. Neurol. 382, 89–103.
Rauschecker, J. P., Tian, B., and Hauser, M. (1995). Processing of complex sounds in the macaque nonprimary auditory cortex. Science 268, 111–114.
Recanzone, G., Schreiner, C., and Merzenich, M. (1993). Plasticity in the frequency representation of primary auditory cortex following discrimination training in adult owl monkeys. J. Neurosci. 13, 87–103.
Rhode, W. S., and Greenberg, S. (1994). Lateral suppression and inhibition in the cochlear nucleus of the cat. J. Neurophysiol. 71, 493–514.
Roberts, L. E., and Bosnyak, D. J. (2011). “Auditory training in tinnitus,” in Textbook of Tinnitus, eds A. Møller, T. Kleinjung, B. Langguth and D. De Ridder (New York, NY: Springer), 563–573.
Roberts, L. E., Eggermont, J. J., Caspary, D. M., Shore, S. E., Melcher, J. R., and Kaltenbach, J. A. (2010). Ringing ears: the neuroscience of tinnitus. J. Neurosci. 30, 14972–14979.
Robertson, D., and Irvine, D. R. (1989). Plasticity of frequency organization in auditory cortex of guinea pigs with partial unilateral deafness. J. Comp. Neurol. 282, 456–471.
Salimpoor, V. N., Benovoy, M., Larcher, K., Dagher, A., and Zatorre, R. J. (2011). Anatomically distinct dopamine release during anticipation and experience of peak emotion to music. Nat. Neurosci. 14, 257–262.
Schlaug, G., Jäncke, L., Huang, Y., Staiger, J. F., and Steinmetz, H. (1995a). Increased corpus callosum size in musicians. Neuropsychologia 33, 1047–1055.
Schlaug, G., Jancke, L., Huang, Y., and Steinmetz, H. (1995b). In vivo evidence of structural brain asymmetry in musicians. Science 267, 699–701.
Schneider, P., Scherg, M., Dosch, H. G., Specht, H. J., Gutschalk, A., and Rupp, A. (2002). Morphology of Heschl's gyrus reflects enhanced activation in the auditory cortex of musicians. Nat. Neurosci. 5, 688–694.
Schulte, M., Knief, A., Seither-Preisler, A., and Pantev, C. (2002). Different modes of pitch perception and learning-induced neuronal plasticity of the human auditory cortex. Neural Plast. 9, 161–175.
Schulz, M., Ross, B., and Pantev, C. (2003). Evidence for training-induced crossmodal reorganization of cortical functions in trumpet players. Neuroreport 14, 157–161.
Shahin, A. J., Bosnyak, D. J., Trainor, L. J., and Roberts, L. E. (2003). Enhancement of neuroplastic P2 and N1c auditory evoked potentials in musicians. J. Neurosci. 23, 5545–5552.
Stouffer, J. L., and Tyler, R. S. (1990). Characterization of tinnitus by tinnitus patients. J. Speech Hear. Disord. 55, 439–453.
Stracke, H., Okamoto, H., and Pantev, C. (2010). Customized notched music training reduces tinnitus loudness. Commun. Integr. Biol. 3, 274–277.
Suga, N. (1995). Sharpening of frequency tuning by inhibition in the central auditory system: tribute to Yasuji Katsuki. Neurosci. Res. 21, 287–299.
Sutter, M. L., Schreiner, C. E., Mclean, M., O'connor, K. N., and Loftus, W. C. (1999). Organization of inhibitory frequency receptive fields in cat primary auditory cortex. J. Neurophysiol. 82, 2358–2371.
Sutter, M. L., and Loftus, W. C. (2003). Excitatory and inhibitory intensity tuning in auditory cortex: evidence for multiple inhibitory mechanisms. J. Neurophysiol. 90, 2629–2647.
Tass, P. A., Adamchic, I., Freund, H.-J., von Stackelberg, T., and Hauptmann, C. (2012). Counteracting tinnitus by acoustic coordinated reset neuromodulation. Restor. Neurol. Neurosci. 30, 135–159.
Teismann, H., Okamoto, H., and Pantev, C. (2011). Short and intense tailor-made notched music training against tinnitus: the tinnitus frequency matters. PLoS ONE 6:e24685. doi: 10.1371/journal.pone.0024685
Tervaniemi, M., Rytkonen, M., Schroger, E., Ilmoniemi, R. J., and Naatanen, R. (2001). Superior formation of cortical memory traces for melodic patterns in musicians. Learn. Mem. 8, 295–300.
Tyler, R. S., and Conrad-Armes, D. (1983). Tinnitus pitch: a comparison of three measurement methods. Br. J. Audiol. 17, 101–107.
Van Zuijen, T. L., Sussman, E., Winkler, I., Näätänen, R., and Tervaniemi, M. (2004). Grouping of sequential sounds–an event-related potential study comparing musicians and nonmusicians. J. Cogn. Neurosci. 16, 331–338.
Van Zuijen, T. L., Sussman, E., Winkler, I., Näätänen, R., and Tervaniemi, M. (2005). Auditory organization of sound sequences by a temporal or numerical regularity–a mismatch negativity study comparing musicians and non-musicians. Brain Res. Cogn. Brain Res. 23, 270–276.
Wan, C. Y., and Schlaug, G. (2010). Music making as a tool for promoting brain plasticity across the life span. Neuroscientist 16, 566–577.
Wiegrebe, L., Kossl, M., and Schmidt, S. (1996). Auditory enhancement at the absolute threshold of hearing and its relationship to the Zwicker tone. Hear. Res. 100, 171–180.
Woldorff, M. G., Gallen, C. C., Hampson, S. A., Hillyard, S. A., Pantev, C., Sobel, D., and Bloom, F. E. (1993). Modulation of early sensory processing in human auditory cortex during auditory selective attention. Proc. Natl. Acad. Sci. U.S.A. 90, 8722–8726.
Zatorre, R. J., Chen, J. L., and Penhune, V. B. (2007). When the brain plays music: auditory-motor interactions in music perception and production. Nat. Rev. Neurosci. 8, 547–558.
Keywords: human auditory cortex, lateral inhibition, music-induced cortical plasticity, tonal tinnitus treatment, tailor-made notched music training
Citation: Pantev C, Okamoto H and Teismann H (2012) Music-induced cortical plasticity and lateral inhibition in the human auditory cortex as foundations for tonal tinnitus treatment. Front. Syst. Neurosci. 6:50. doi: 10.3389/fnsys.2012.00050
Received: 16 March 2012; Accepted: 05 June 2012;
Published online: 27 June 2012.
Edited by:
Larry Roberts, McMaster University, CanadaCopyright: © 2012 Pantev, Okamoto and Teismann. This is an open-access article distributed under the terms of the Creative Commons Attribution Non Commercial License, which permits non-commercial use, distribution, and reproduction in other forums, provided the original authors and source are credited.
*Correspondence: Christo Pantev, Institute for Biomagnetism and Biosignalanalysis, University of Münster, Malmedyweg 15, 48129 Muenster, Germany. e-mail:cGFudGV2QHVuaS1tdWVuc3Rlci5kZQ==