- Carl-Ludwig Institute for Physiology, Medical Faculty, University of Leipzig, Leipzig, Germany
Parallel fiber (PF) synapses show pronounced and lasting facilitation during bursts of high-frequency activity. They typically connect to their target neurons via a single active zone (AZ), harboring few release sites (~2–8) with moderate initial vesicular release probability (~0.2–0.4). In light of these biophysical characteristics, it seems surprising that PF synapses can sustain facilitation during high-frequency periods of tens of action potentials (APs). Recent findings suggest an increase in the number of occupied release sites due to ultra-rapid (~180 s−1), Ca2+ dependent recruitment of synaptic vesicles (SVs) from replenishment sites as major presynaptic mechanism of this lasting facilitation. On the molecular level, Synaptotagmin 7 or Munc13s have been suggested to be involved in mediating facilitation at PF synapses. The recruitment of SVs from replenishment sites appears to be reversible on a slower time-scale, thereby, explaining that PF synapses rapidly depress and ultimately become silent during low-frequency activity. Hence, PF synapses show high-frequency facilitation (HFF) but low-frequency depression (LFD). This behavior is explained by regulation of the number of occupied release sites at the AZ by AP frequency.
Introduction
Parallel fiber (PF) synapses are major sites for conveying sensory information to the cerebellar cortical output neurons, the Purkinje cells (PCs), and to interneurons. They are formed by granule cells, which fire bursts of action potentials (APs) over a broad range of frequencies up to ~1 kHz in response to sensory input (Chadderton et al., 2004; Rancz et al., 2007; Ritzau-Jost et al., 2014). PF synapses in turn are adapted to reliably respond to these high-frequency bursts of APs with sustained and facilitating transmission (Valera et al., 2012). This puts substantial demands on the mechanisms of synaptic vesicle (SV) supply.
Briefly, an AP invading a presynaptic terminal opens voltage-gated Ca2+ channels and the inflowing Ca2+ ions trigger the fusion of SVs with the presynaptic plasma membrane and transmitter release. Fusion of SVs is a probabilistic process that takes place at the presynaptic active zone (AZ). The AZ is thought to harbor one or more release sites (N) that constitute the individual entities at which a single SV can fuse with a certain vesicular release probability (pv; Südhof, 2013; Kaeser and Regehr, 2017).
For a single AP, the presynaptic efficacy depends on the number of release sites occupied by release-ready SVs (Nocc) at the time of the AP and on the pv of these SVs. The pv, in turn, depends on several factors, including the diffusional distance between the Ca2+ channels and the SV and the intrinsic Ca2+ sensitivity of its release machinery (Eggermann et al., 2012; Bornschein and Schmidt, 2019). While in experiments typically only an average pv can be estimated (Clements and Silver, 2000), the pv need not be homogeneous across release sites (Neher, 2015).
During a train of APs, the regulation of presynaptic efficacy gets more complex. Occupied release sites are continuously emptied by the fusion processes, which, without further mechanisms, would result in synaptic depression due to progressive depletion of the pool of release-ready SVs. How effectively the information transfer can be maintained during an AP train now depends on the speed with which Nocc can be restored or newly recruited and on their pv, which may increase. If the latter outcompetes SV consumption, the synapse may show facilitation rather than depression during the train (Jackman and Regehr, 2017; Neher and Brose, 2018).
This mini review article focusses on recent results from PF synapses suggesting that during high-frequency trains of APs the rate of restoration or recruitment of release sites exceeds the fusion rate, resulting in an activity-dependent increase in Nocc as major presynaptic mechanism of facilitation at PF synapses (Valera et al., 2012; Brachtendorf et al., 2015; Miki et al., 2016; Doussau et al., 2017).
Parallel-Fiber Synapses
Biophysics of Parallel Fiber Terminals
The target neurons of PFs include PCs and molecular layer interneurons (MLIs). PFs contact their targets typically by a single presynaptic bouton harboring a single AZ only (Xu-Friedman et al., 2001). Presynaptic Ca2+ transients are reliably induced by single APs, show very little trial-to-trial variability for a given bouton and linear summation during a train of APs (Brenowitz and Regehr, 2007; Schmidt et al., 2013; Baur et al., 2015; Miki et al., 2016; Kusch et al., 2018). Mature PF terminals gate release with P/Q-type channel nanodomains (Schmidt et al., 2013; Kusch et al., 2018) that develop from P/Q- and N-type channel microdomains gating release from young terminals (Mintz et al., 1995; Baur et al., 2015). Depending on their target neuron, PFs release SVs with pv ~0.25–0.4 in 2 mM extracellular Ca2+ concentration ([Ca2+]e; Sims and Hartell, 2005; Valera et al., 2012; Schmidt et al., 2013; Ishiyama et al., 2014; Baur et al., 2015). The number of release sites per synapse is small and has been estimated by amplitude fluctuation analysis of excitatory postsynaptic currents (EPSCs) to be on average in the range of ~2–5, perhaps with some target- or species-dependent differences (Schmidt et al., 2013; Ishiyama et al., 2014; Malagon et al., 2016). In electron microscopy ~8 docked vesicles were found in PF terminals (Xu-Friedman et al., 2001). These results indicate that single PF AZs harbor more than one release site, consistent with multi-vesicular release (Crowley et al., 2007).
High-Frequency Facilitation and Low-Frequency Depression
PF synapses show paired-pulse facilitation (PPF) with paired-pulse ratios (PPRs) between the first and the second EPSC amplitude (A2/A1) of ~2–3 at small interstimulus intervals (ISIs) of 5–10 ms (Figure 1). PPRs (Ai/A1) remain at this level even during longer lasting high-frequency bursts and under conditions of elevated initial pv (pv1; Atluri and Regehr, 1996; Sims and Hartell, 2005; Valera et al., 2012; Ishiyama et al., 2014; Brachtendorf et al., 2015; Turecek and Regehr, 2018). In light of the above brief overview of biophysical characteristics, this is surprising at first glance. Assuming N of three, pv1 of 0.25 and pv2 of 0.84 as estimated for PF to PC synapses in 2 mM [Ca2+]e (Valera et al., 2012; Schmidt et al., 2013; Brachtendorf et al., 2015), the theoretical maximum for the PPR between second and first pulse in the absence of SV replenishment [PPR = (pv2/pv1)*(1 − pv1) = 2.52; the term (1 − pv1) accounts for the reduction in Nocc during the first AP] is close to or even lower than the experimentally found values and subsequent pulses cannot be explained. Consistently, it has been suggested early that SV replenishment at PF terminals is very rapid (Crowley et al., 2007).
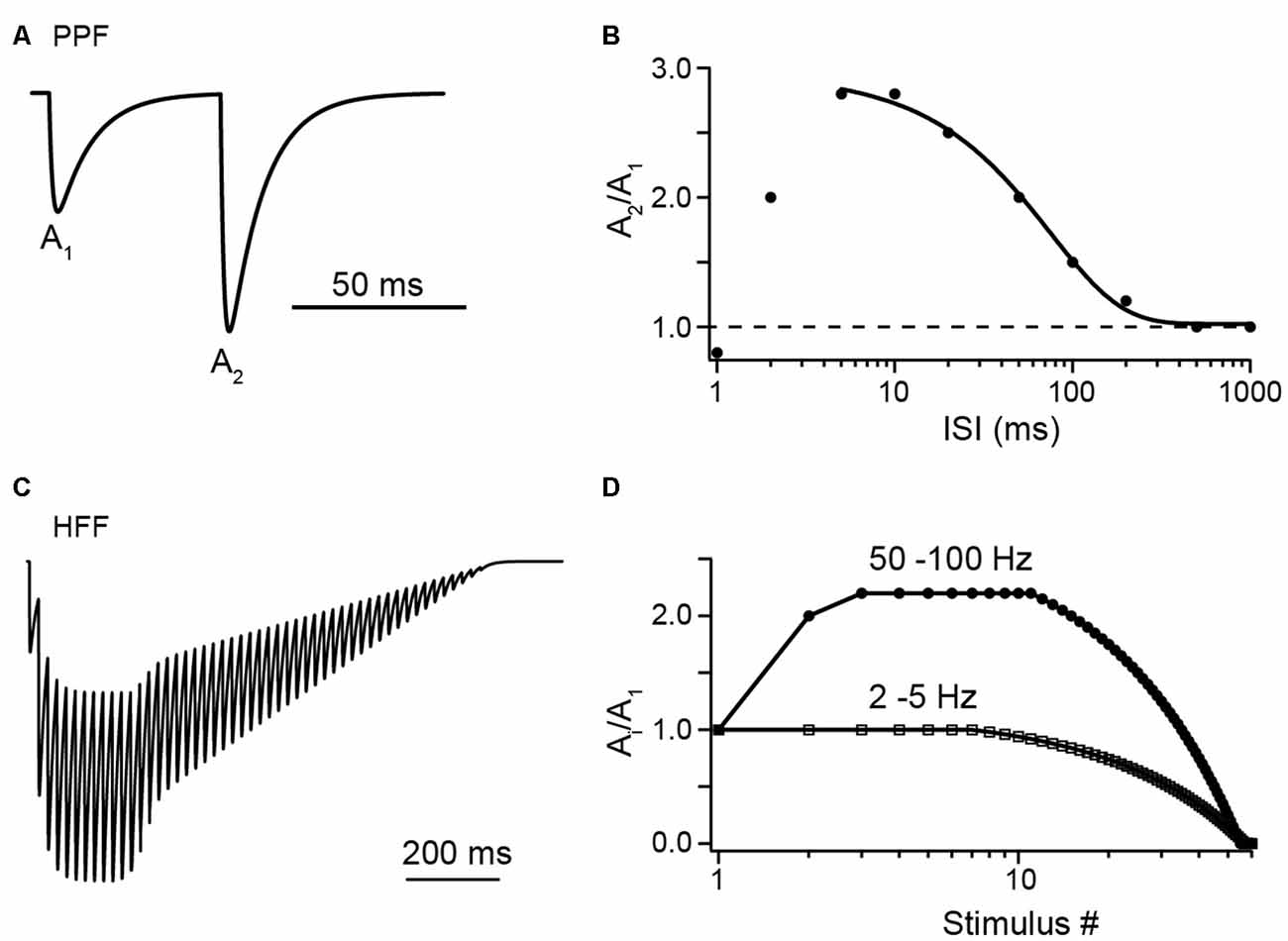
Figure 1. Illustrations of facilitation vs. depression at Parallel fiber (PF) to Purkinje cell (PC) synapses with synthetic data. (A) A pair of excitatory postsynaptic currents (EPSCs), normalized to the first amplitude (A1), as would be evoked by PF tract stimulation at an interstimulus interval (ISI) of 50 ms, illustrating paired-pulse facilitation (PPF). (B) Illustration of paired-pulse ratio (PPR) as a function of ISI. Synthetic data in the range of 5 ms to 1 s were fit by a sigmoidal function (see Valera et al., 2012). Note the decline in PPR at ISI <5 ms, which may indicate a limit in the speed of synaptic vesicle (SV) replenishment (see text for detail). (C) Illustration of high-frequency facilitation (HFF) of normalized EPSCs during a 50 Hz train of action potentials (APs). (D) Illustration of HFF (circles) and low-frequency depression (LFD; squares) as a function of stimulus number as would be observed at the indicated frequencies (see Doussau et al., 2017).
Rapid replenishment alone, however, is unlikely to fully account for PPF at PF synapses. It was recognized that even if pv2 of one and full replenishment between APs (i.e., Nocc,1 = Nocc,2; PPR = pv2/pv1) are assumed the experimentally determined values frequently exceed the theoretical maxima (Valera et al., 2012; Ishiyama et al., 2014; Brachtendorf et al., 2015; Miki et al., 2016). Consistently, Valera et al. (2012) found evidence for changes in N during activity of PF to PC synapses. They found that N, as estimated by the binominal parameter in fluctuation analysis, increased during high-frequency trains of APs. In particular N during the second AP was larger than during the first AP (N2 > N1), suggesting incremental N as a substantial factor of PPF. These findings were subsequently confirmed by stationary fluctuation analysis at single PF to PC synapses in paired recordings (Brachtendorf et al., 2015).
In the latter study, it was proposed that the PPR of PF to PC synapses can be explained by a model with sequential SV pools originally proposed for crayfish motoneuron synapses (Millar et al., 2005). In the adaptation for the PF terminal, it was assumed that release sites are restored from replenishment sites in a Ca2+ dependent manner (Brachtendorf et al., 2015). The model well predicted the experimental PPF over a broad range of ISIs of 5 ms to 1 s if a transient increase in N between the two APs of a paired-pulse experiment was permitted rather than an increase in pv alone. Morphologically, additional N appear possible since the area of the PF AZ (0.068 μm2; Kusch et al., 2018) is sufficiently large to harbor more than 2–8 release-ready SVs (r = 21 nm; Wilhelm et al., 2014).
Two recent studies investigated the mechanisms of sustained release reliability at PF terminals during trains of APs in great depth (Miki et al., 2016; Doussau et al., 2017; Figure 2). Miki et al. (2016) challenged PF to MLI synapses with trains of eight APs delivered at small ISI of 5 ms in elevated [Ca2+]e of 3 mM. Based on these data they suggest a sequential two-pool model (plus an implicit reserve pool) that explains facilitation mainly based on increasing Nocc (Figure 2A). They suggest an initially incomplete resting occupancy of release sites (referred to as docking sites), such that Nocc,1 < N1. N1 was estimated to be ~4–5 with a resting occupancy of 0.45, such that Nocc,1 is ~2–3. Replenishment sites of about the same number (4–5) were considered to be fully occupied and the transition probability between the two pools was estimated to be 0.6 during activity. Based on EGTA effects, this high transition probability was Ca2+ dependent and gave rise to the increase in Nocc during the train.
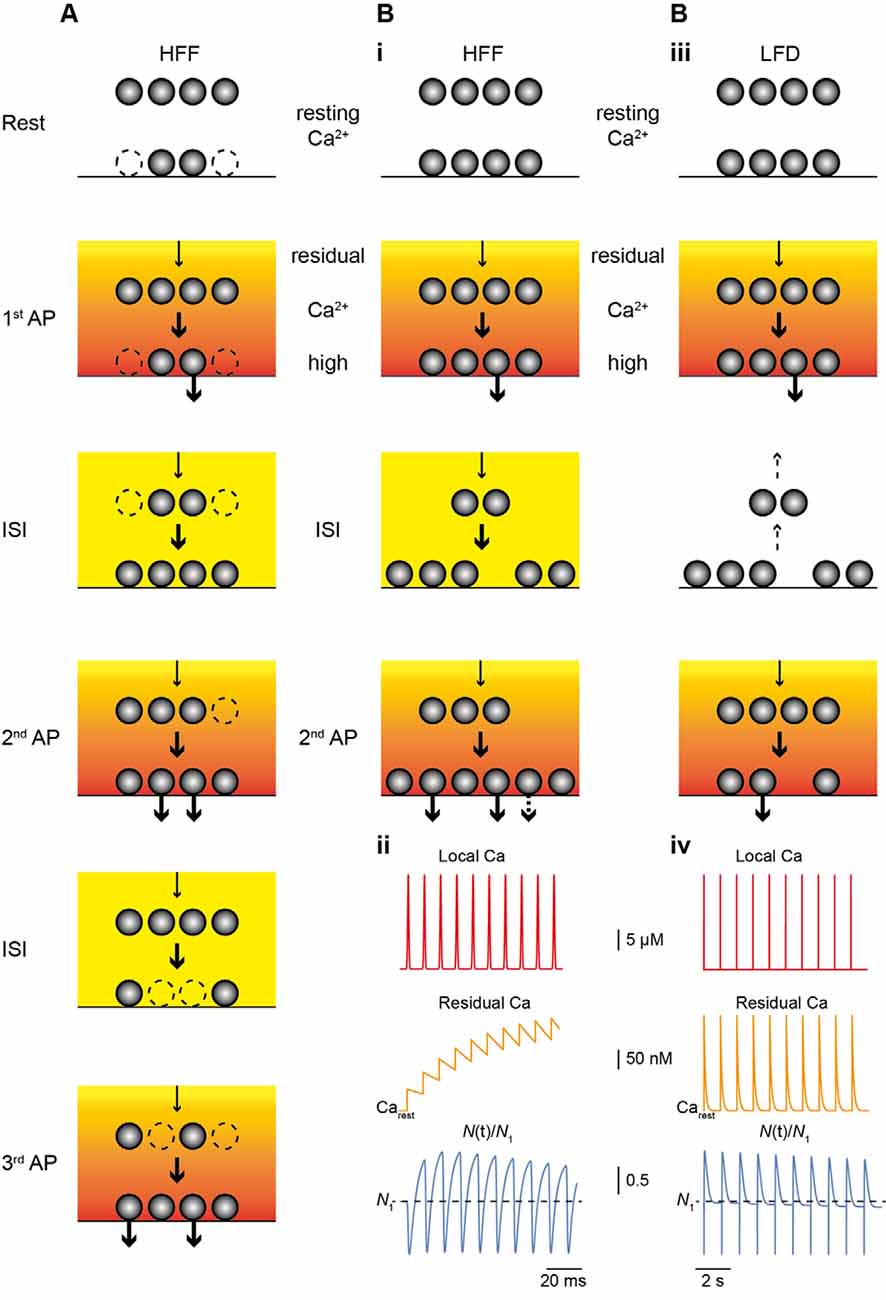
Figure 2. Main mechanism of facilitation and depression. (A) Scheme of the model of HFF proposed by Miki et al. (2016). The resting occupancy of release sites (lower vesicles) is ~50% (dashed vesicles; Nocc,1 < N), while a replenishment pool (upper vesicles) is fully occupied. The reserve pool is not shown. During high-frequency stimulation Nocc increases (Nocc,train = N), thereby, giving rise to HFF. Release and the transition of vesicles from replenishment sites to release sites (arrows) is driven by the Ca2+ gradient (red to yellow gradient) and residual Ca2+ (orange to yellow). (B) As in (A) but for the model proposed by Doussau et al. (2017) for HFF and LFD. At rest, all release sites are occupied (Nocc,1 = N1). (i) During HFF, N increases (Ntrain > N1); an increase in pv makes a smaller contribution to HFF (indicated by the dashed arrow in the lower panel). (ii) Illustrations of local Ca2+ at the release sensor (red, top), build-up of residual Ca2+ (orange, middle), and N(t) (blue, bottom), normalized to N1 (dashed line), plotted over time for 10 APs at 100 Hz. Note the increase in N. (iii) During low-frequency activation Ca2+ drops back to resting level between pulses and vesicles return from release sites to replenishment sites (dashed arrows), giving rise to PPR of ~1 in the second pulse. (iv) As in (ii) but for 10 APs at 2 Hz. Note that there is no build-up in residual Ca2+ (middle) and that N is no longer increased at the time of stimulation and progressively declines (lower), resulting in LFD during continuing activation.
Doussau et al. (2017) challenged PF synapses by long-lasting trains of 50 to >100 APs delivered either at high (ISI 10 or 20 ms) or low (ISI 0.2, 0.5 or 2 s) frequency. They found sustained high-frequency facilitation (HFF) for a large number of 20–30 APs before synapses progressively depressed and frequently became “silent.” Remarkably, during low-frequency activation, synapses no longer facilitated but had Ai/A1 PPRs of 1 for the first ~7 APs. Subsequently, EPSC amplitudes progressively depressed over tens of APs and eventually the synapses became silent. Hence, PF terminals show HFF but low-frequency depression (LFD; Figures 1C,D). In agreement with the above studies, the authors provide evidence that these bidirectional short-term plasticity characteristics are explained by the presence of two sequential SV pools (termed fully releasable and reluctant pool, plus an implicit reserve pool). During high-frequency trains, N, which is equal to Nocc in this study, increased via rapid recruitment from the reluctant pool while release sites became progressively depleted during low-frequency stimulation. The results with EGTA and simulations indicated that this rapid recruitment is Ca2+ dependent and slowly reversible within ~200 ms, such that it effectively increased N contributing to release with high-frequency but not low-frequency AP firing (Figure 2B).
In summary, while there is some controversy about the resting occupancy of release sites (Miki et al., 2016; Doussau et al., 2017), several lines of evidence from the recent literature suggest that facilitation at PF synapses mainly results from a presynaptic mechanism that increases the number of release sites or their occupancy during high-frequency trains of APs. This increase is the result of a very rapid, activity-dependent supply of SVs from replenishment sites, also referred to a “overfilling” of the ready releasable pool (RRP; Neher and Brose, 2018). The forward transition of SVs from replenishment sites is likely to be reversible on a slower time-scale, thereby, explaining the finding of LFD in addition to HFF at PF synapses.
Mechanisms of Rapid Replenishment
The very rapid, Ca2+ dependent forward transition of SVs from replenishment sites to release sites requires a mechanism that operates on the ms time-scale. PPR experiments indicate that the speed of the replenishment process reaches its limit at ISI <5 ms (Figure 1B). At shorter ISI PPF declined and eventually turned to depression (Valera et al., 2012).
Assuming an exponential process, Miki et al. (2016) estimate a very rapid rate constant of ~180 s−1, corresponding to τ of ~5.5 ms per release site for an ISI of 5 ms. This is faster than would be obtained by mere diffusion of SVs, suggesting an active process. Consistently, they found evidence for an involvement of actin and myosin cytoskeleton in rapid replenishment based on the inhibitory effects of latrunculin B and blebbistatin. Additional experiments with EGTA-AM revealed the Ca2+ dependency of replenishment.
As detailed above, Doussau et al. (2017) found evidence that the replenishment process is reversible on a slower time scale of ~200 ms. Interestingly, in a recent manuscript reporting results from electron microscopic analysis of hippocampal synapses, following stimulation and rapid freezing of cultured neurons, new SVs were recruited to the plasma membrane and fully replenished the docked pool of SVs within ~10 ms after stimulation (Kusick et al., 2018). The docking of these SVs was transient and they either undocked or fused within 100 ms. These ultra structural results are in notable agreement with the findings at PF synapses, suggesting that recruitment of SVs to release sites is rapid and reversible.
Already 20 years ago it has been suggested that facilitation at PF synapses requires a Ca2+ dependent facilitation sensor separate from the release sensor (Atluri and Regehr, 1996). The molecular identity and mode of action of this sensor, however, remained elusive until recently. Recent results suggest that Synaptotagmin 7 (Syt7) acts as facilitation sensor at PF terminals. Syt7 knock-out mice displayed reduced PPF, while their pv and presynaptic Ca2+ signaling were not affected (Turecek and Regehr, 2018). Mechanistically, Ca2+ binding to the C2A domain of Syt7 is required for facilitation at different synapses (Jackman et al., 2016). Interestingly, Syt7 was also found to promote SV replenishment during trains of APs in a Ca2+ dependent manner by interaction with Ca2+ bound calmodulin (Liu et al., 2014). For other functions of Syt7, e.g., in asynchronous release (Turecek and Regehr, 2018), and proposed relationships between different functions I refer the reader to recent reviews (e.g., Chen and Jonas, 2017; Bornschein and Schmidt, 2019; Volynski and Krishnakumar, 2018).
At Syt7 mutant PF synapses, a substantial amount of PPF remained at short ISI (Turecek and Regehr, 2018). This indicates that other mechanisms are operational in addition, which may involve other proteins with C2 domains such as Munc13s (Neher and Brose, 2018). The cerebellum-specific Munc13-3, for example, increases pv and alters PPR by “superpriming” (Augustin et al., 2001; Ishiyama et al., 2014). Experiments in a developmental context indicated that Munc13-3 tightens the coupling distance between SVs and P/Q-type channels (Kusch et al., 2018). Whether coupling distance tightening and Munc13-3 can establish newly occupied release sites during high-frequency activity is unclear at present. For further details on molecular mechanisms of short-term plasticity and the role of Synaptotagmins and other molecular players I refer the reader to recent comprehensive reviews (Jackman and Regehr, 2017; Bornschein and Schmidt, 2019; Neher and Brose, 2018; Volynski and Krishnakumar, 2018).
Concluding Remarks
PPF was discovered more than 70 years ago and its mechanisms may differ between synapses (Jackman and Regehr, 2017). At different synapses different conceptions were suggested to account for facilitation. Originally, it has been proposed that the “active Ca2+,” which is “Ca2+ remaining attached to specific sites on the inner axon membrane” causes facilitation (Katz and Miledi, 1968). Reminiscent of the active Ca2+ are slow Ca2+ unbinding from the release sensor (Bornschein et al., 2013) and Ca2+ binding to the facilitation sensor Syt7 (Atluri and Regehr, 1996; Jackman et al., 2016). In addition, elevated release site [Ca2+]i due to AP broadening (Geiger and Jonas, 2000) or effects of endogenous Ca2+ buffers can cause facilitation (Rozov et al., 2001). Finally, the very rapid, activity-dependent increase in the number of occupied release sites added to the mechanisms of facilitation (Valera et al., 2012; Brachtendorf et al., 2015; Miki et al., 2016; Doussau et al., 2017). At PF synapses buffering by their major endogenous buffer Calretinin increases PPF by reducing pv1 (Schmidt et al., 2013; Brachtendorf et al., 2015). The effect is attenuated by the concomitant reduction in the Ca2+ dependent recruitment process such that the net effect of Calretinin on PPF is rather moderate (Schiffmann et al., 1999; Brachtendorf et al., 2015). Also, Ca2+ unbinding from the release sensor likely makes a small contribution (Brachtendorf et al., 2015; Doussau et al., 2017). The majority of facilitation, however, results from an ultra-rapid and reversible increase in occupied release sites during high-frequency activity (Miki et al., 2016; Doussau et al., 2017). Hence, release sites at AZs of PF synapses are very dynamic entities that can be reversibly recruited or replenished on a millisecond time scale.
Author Contributions
HS wrote the manuscript.
Funding
This work was supported by the German Research Foundation (Deutsche Forschungsgemeinschaft, DFG, SCHM1838/2) and the DFG and University of Leipzig within the program of open access publishing.
Conflict of Interest Statement
The author declares that the research was conducted in the absence of any commercial or financial relationships that could be construed as a potential conflict of interest.
Acknowledgments
The author would like to thank Philippe Isope, Bernard Poulain, Takafumi Miki and Stefan Hallermann for their helpful comments and critical discussion of the manuscript.
Abbreviations
A, amplitude of EPSC; AP, action potential; AZ, active zone; BC, Basket cell; EPSC, excitatory postsynaptic current; MLI, molecular layer interneuron; N, number of release sites; Nocc, number of release sites occupied by release-ready SVs; pv, vesicular release probability; SV, synaptic vesicle; PC, Purkinje cell; PF, parallel fiber; PPR, paired pulse ratio; PPF, paired pulse facilitation; RRP, ready releasable pool.
References
Atluri, P. P., and Regehr, W. G. (1996). Determinants of the time course of facilitation at the granule cell to Purkinje cell synapse. J. Neurosci. 16, 5661–5671. doi: 10.1523/JNEUROSCI.16-18-05661.1996
Augustin, I., Korte, S., Rickmann, M., Kretzschmar, H. A., Südhof, T. C., Herms, J. W., et al. (2001). The cerebellum-specific Munc13 isoform Munc13–3 regulates cerebellar synaptic transmission and motor learning in mice. J. Neurosci. 21, 10–17. doi: 10.1523/JNEUROSCI.21-01-00010.2001
Baur, D., Bornschein, G., Althof, D., Watanabe, M., Kulik, A., Eilers, J., et al. (2015). Developmental tightening of cerebellar cortical synaptic influx-release coupling. J. Neurosci. 35, 1858–1871. doi: 10.1523/JNEUROSCI.2900-14.2015
Bornschein, G., Arendt, O., Hallermann, S., Brachtendorf, S., Eilers, J., and Schmidt, H. (2013). Paired-pulse facilitation at recurrent Purkinje neuron synapses is independent of calbindin and parvalbumin during high-frequency activation. J. Physiol. 591, 3355–3370. doi: 10.1113/jphysiol.2013.254128
Bornschein, G., and Schmidt, H. (2019). Synaptotagmin Ca2+ sensors and their spatial coupling to presynaptic Cav channels in central cortical synapses. Front. Mol. Neurosci. 11:494. doi: 10.3389/fnmol.2018.00494
Brachtendorf, S., Eilers, J., and Schmidt, H. (2015). A use-dependent increase in release sites drives facilitation at calretinin-deficient cerebellar parallel-fiber synapses. Front. Cell. Neurosci. 9:27. doi: 10.3389/fncel.2015.00027
Brenowitz, S. D., and Regehr, W. G. (2007). Reliability and heterogeneity of calcium signaling at single presynaptic boutons of cerebellar granule cells. J. Neurosci. 27, 7888–7898. doi: 10.1523/JNEUROSCI.1064-07.2007
Chadderton, P., Margrie, T. W., and Häusser, M. (2004). Integration of quanta in cerebellar granule cells during sensory processing. Nature 428, 856–860. doi: 10.1038/nature02442
Chen, C., and Jonas, P. (2017). Synaptotagmins: that’s why so many. Neuron 94, 694–696. doi: 10.1016/j.neuron.2017.05.011
Clements, J. D., and Silver, R. A. (2000). Unveiling synaptic plasticity: a new graphical and analytical approach. Trends Neurosci. 23, 105–113. doi: 10.1016/s0166-2236(99)01520-9
Crowley, J. J., Carter, A. G., and Regehr, W. G. (2007). Fast vesicle replenishment and rapid recovery from desensitization at a single synaptic release site. J. Neurosci. 27, 5448–5460. doi: 10.1523/JNEUROSCI.1186-07.2007
Doussau, F., Schmidt, H., Dorgans, K., Valera, A. M., Poulain, B., and Isope, P. (2017). Frequency-dependent mobilization of heterogeneous pools of synaptic vesicles shapes presynaptic plasticity. Elife 6:e28935. doi: 10.7554/elife.28935
Eggermann, E., Bucurenciu, I., Goswami, S. P., and Jonas, P. (2012). Nanodomain coupling between Ca2+ channels and sensors of exocytosis at fast mammalian synapses. Nat. Rev. Neurosci. 13, 7–21. doi: 10.1038/nrn3125
Geiger, J. R., and Jonas, P. (2000). Dynamic control of presynaptic Ca2+ inflow by fast-inactivating K+ channels in hippocampal mossy fiber boutons. Neuron 28, 927–939. doi: 10.1016/s0896-6273(00)00164-1
Ishiyama, S., Schmidt, H., Cooper, B. H., Brose, N., and Eilers, J. (2014). Munc13–3 superprimes synaptic vesicles at granule cell-to-basket cell synapses in the mouse cerebellum. J. Neurosci. 34, 14687–14696. doi: 10.1523/JNEUROSCI.2060-14.2014
Jackman, S. L., and Regehr, W. G. (2017). The mechanisms and functions of synaptic facilitation. Neuron 94, 447–464. doi: 10.1016/j.neuron.2017.02.047
Jackman, S. L., Turecek, J., Belinsky, J. E., and Regehr, W. G. (2016). The calcium sensor synaptotagmin 7 is required for synaptic facilitation. Nature 529, 88–91. doi: 10.1038/nature16507
Kaeser, P. S., and Regehr, W. G. (2017). The readily releasable pool of synaptic vesicles. Curr. Opin. Neurobiol. 43, 63–70. doi: 10.1016/j.conb.2016.12.012
Katz, B., and Miledi, R. (1968). The role of calcium in neuromuscular facilitation. J. Physiol. 195, 481–492. doi: 10.1113/jphysiol.1968.sp008469
Kusch, V., Bornschein, G., Loreth, D., Bank, J., Jordan, J., Baur, D., et al. (2018). Munc13–3 is required for the developmental localization of Ca2+ channels to active zones and the nanopositioning of Cav2.1 near release sensors. Cell Rep. 22, 1965–1973. doi: 10.1016/j.celrep.2018.02.010
Kusick, G. F., Chin, M., Lippmann, K., Adula, K. P., Davis, M. W., Jorgensen, E. M., et al. (2018). Synaptic vesicles undock and then transiently dock after an action potential. bioRxiv [Preprint]. doi: 10.1101/509216
Liu, H., Bai, H., Hui, E., Yang, L., Evans, C. S., Wang, Z., et al. (2014). Synaptotagmin 7 functions as a Ca2+-sensor for synaptic vesicle replenishment. Elife 3:e01524. doi: 10.7554/elife.01524
Malagon, G., Miki, T., Llano, I., Neher, E., and Marty, A. (2016). Counting vesicular release events reveals binomial release statistics at single glutamatergic synapses. J. Neurosci. 36, 4010–4025. doi: 10.1523/JNEUROSCI.4352-15.2016
Miki, T., Malagon, G., Pulido, C., Llano, I., Neher, E., and Marty, A. (2016). Actin- and myosin-dependent vesicle loading of presynaptic docking sites prior to exocytosis. Neuron 91, 808–823. doi: 10.1016/j.neuron.2016.07.033
Millar, A. G., Zucker, R. S., Ellis-Davies, G. C., Charlton, M. P., and Atwood, H. L. (2005). Calcium sensitivity of neurotransmitter release differs at phasic and tonic synapses. J. Neurosci. 25, 3113–3125. doi: 10.1523/JNEUROSCI.4717-04.2005
Mintz, I. M., Sabatini, B. L., and Regehr, W. G. (1995). Calcium control of transmitter release at a cerebellar synapse. Neuron 15, 675–688. doi: 10.1016/0896-6273(95)90155-8
Neher, E. (2015). Merits and limitations of vesicle pool models in view of heterogeneous populations of synaptic vesicles. Neuron 87, 1131–1142. doi: 10.1016/j.neuron.2015.08.038
Neher, E., and Brose, N. (2018). Dynamically primed synaptic vesicle states: key to understand synaptic short-term plasticity. Neuron 100, 1283–1291. doi: 10.1016/j.neuron.2018.11.024
Rancz, E. A., Ishikawa, T., Duguid, I., Chadderton, P., Mahon, S., and Hausser, M. (2007). High-fidelity transmission of sensory information by single cerebellar mossy fibre boutons. Nature 450, 1245–1248. doi: 10.1038/nature05995
Ritzau-Jost, A., Delvendahl, I., Rings, A., Byczkowicz, N., Harada, H., Shigemoto, R., et al. (2014). Ultrafast action potentials mediate kilohertz signaling at a central synapse. Neuron 84, 152–163. doi: 10.1016/j.neuron.2014.08.036
Rozov, A., Burnashev, N., Sakmann, B., and Neher, E. (2001). Transmitter release modulation by intracellular Ca2+ buffers in facilitating and depressing nerve terminals of pyramidal cells in layer 2/3 of the rat neocortex indicates a target cell-specific difference in presynaptic calcium dynamics. J. Physiol. 531, 807–826. doi: 10.1111/j.1469-7793.2001.0807h.x
Schiffmann, S. N., Cheron, G., Lohof, A., d’Alcantara, P., Meyer, M., Parmentier, M., et al. (1999). Impaired motor coordination and Purkinje cell excitability in mice lacking calretinin. Proc. Natl. Acad. Sci. U S A 96, 5257–5262. doi: 10.1073/pnas.96.9.5257
Schmidt, H., Brachtendorf, S., Arendt, O., Hallermann, S., Ishiyama, S., Bornschein, G., et al. (2013). Nanodomain coupling at an excitatory cortical synapse. Curr. Biol. 23, 244–249. doi: 10.1016/j.cub.2012.12.007
Sims, R. E., and Hartell, N. A. (2005). Differences in transmission properties and susceptibility to long-term depression reveal functional specialization of ascending axon and parallel fiber synapses to Purkinje cells. J. Neurosci. 25, 3246–3257. doi: 10.1523/JNEUROSCI.0073-05.2005
Südhof, T. C. (2013). Neurotransmitter release: the last millisecond in the life of a synaptic vesicle. Neuron 80, 675–690. doi: 10.1016/j.neuron.2013.10.022
Turecek, J., and Regehr, W. G. (2018). Synaptotagmin 7 mediates both facilitation and asynchronous release at granule cell synapses. J. Neurosci. 38, 3240–3251. doi: 10.1523/jneurosci.3207-17.2018
Valera, A. M., Doussau, F., Poulain, B., Barbour, B., and Isope, P. (2012). Adaptation of granule cell to Purkinje cell synapses to high-frequency transmission. J. Neurosci. 32, 3267–3280. doi: 10.1523/JNEUROSCI.3175-11.2012
Volynski, K. E., and Krishnakumar, S. S. (2018). Synergistic control of neurotransmitter release by different members of the synaptotagmin family. Curr. Opin. Neurobiol. 51, 154–162. doi: 10.1016/j.conb.2018.05.006
Wilhelm, B. G., Mandad, S., Truckenbrodt, S., Kröhnert, K., Schäfer, C., Rammner, B., et al. (2014). Composition of isolated synaptic boutons reveals the amounts of vesicle trafficking proteins. Science 344, 1023–1028. doi: 10.1126/science.1252884
Keywords: parallel fiber, faciliatation, replenishment, release probability, vesicle pools, residual calcium, synaptotagmin 7, Munc13
Citation: Schmidt H (2019) Control of Presynaptic Parallel Fiber Efficacy by Activity-Dependent Regulation of the Number of Occupied Release Sites. Front. Syst. Neurosci. 13:30. doi: 10.3389/fnsys.2019.00030
Received: 11 April 2019; Accepted: 01 July 2019;
Published: 17 July 2019.
Edited by:
Conor J. Houghton, University of Bristol, United KingdomReviewed by:
Stephen Rayport, Columbia University, United StatesUri Ashery, Tel Aviv University, Israel
Copyright © 2019 Schmidt. This is an open-access article distributed under the terms of the Creative Commons Attribution License (CC BY). The use, distribution or reproduction in other forums is permitted, provided the original author(s) and the copyright owner(s) are credited and that the original publication in this journal is cited, in accordance with accepted academic practice. No use, distribution or reproduction is permitted which does not comply with these terms.
*Correspondence: Hartmut Schmidt, aGFydG11dC5zY2htaWR0QG1lZGl6aW4udW5pLWxlaXB6aWcuZGU=